- 1Reef Biology Research Group, Department of Marine Science, Faculty of Science, Chulalongkorn University, Pathumwan, Thailand
- 2Aquatic Resources Research Institute, Chulalongkorn University, Pathumwan, Thailand
- 3Department of Microbiology, Faculty of Science, Chulalongkorn University, Pathumwan, Thailand
- 4Microbiome Research Unit for Probiotics in Food and Cosmetics, Chulalongkorn University, Pathumwan, Thailand
- 5Department of Nuclear Engineering, Faculty of Engineering, Chulalongkorn University, Pathumwan, Thailand
- 6Centre National de la Recherche Scientifique, Institut de Chimie des Substances Naturelles ICSN, Gif-sur-Yvette, France
As seawater temperature rises, repeated thermal bleaching events have negatively affected the reefs of the Andaman Sea for over decades. Studies on the coral-associated microbial diversity of prokaryotes and microbial eukaryotes (microbiome) in healthy and bleached corals are important to better understand the coral holobionts that involved augmented resistance to stresses, and this information remains limited in the Andaman Sea of Thailand. The present study thereby described the microbiomes of healthy (unbleached) and bleached colonies of four prevalent corals, Acropora humilis, Platygyra sp., Pocillopora damicornis, and Porites lutea, along with the surrounding seawater and sediments, that were collected during a 2016 thermal bleaching event, using 16S and 18S rRNA genes next-generation sequencing (NGS). Both prokaryotic and eukaryotic microbes showed isolated community profiles among sample types (corals, sediment, and seawater) [analysis of similarities (ANOSIM): p = 0.038 for prokaryotes, p < 0.001 for microbial eukaryotes] and among coral genera (ANOSIM: p < 0.001 for prokaryotes and microbial eukaryotes). In bleached state corals, we found differences in microbial compositions from the healthy state corals. Prevalent differences shared among bleached coral genera (shared in at least three coral genera) included a loss of reported coral-beneficial microbes, such as Pseudomonadales, Alteromonadales, and Symbiodinium; meanwhile an increase of putative coral-pathogenic Malassezia and Aspergillus. This difference could affect carbon and nitrogen availability for coral growth, reflective of a healthy or bleached state. Our findings in part supported previously microbial dysbiosis knowledge of thermal bleaching coral microbiomes around South East Asia marine geography, and together ongoing efforts are to support the understanding and management of microbial diversity to reduce the negative impacts to corals in massive thermal bleaching events.
Introduction
Coral bleaching is defined as a breakdown of coral-algae symbiosis, when the corals are stressed by a variety of factors, in which the increased atmosphere temperature (thus seawater temperature) is the major triggering cause, and the endosymbiotic algae (in particular photosynthetic dinoflagellate Symbiodinium) expel (Hoegh-Guldberg and Smith, 1989; Bruno et al., 2001; Eakin et al., 2010). This results in the loss of coral color (become white) and of food that is supplied through photosynthesis by the dinoflagellates for coral growth, reproduction, resistance to diseases, and survivability (Baker et al., 2008). Nowadays, thermal bleaching events are considered as the most problematic coral situation worldwide, including in the Andaman Sea coast of Thailand where the first widespread thermal bleaching event was recorded in 1991 and subsequently recurred in 1995, 1998, 2003, 2010 (severe event), and 2016, respectively (Phongsuwan and Chansang, 2012). The 2010 bleaching event caused 44.2–99.0% coral mortality (Brown et al., 1996; Phongsuwan and Chansang, 2012). In the 2016 bleaching event, approximately 50% of the corals were affected; this was considered moderate bleaching-induced mortality (Pootakham et al., 2018). The mortality of corals affects the nursing habitats of diverse marine fish and invertebrates (Boilard et al., 2020).
Other factors to cause coral bleaching include water salinity increase (Berkelmans et al., 2012; Kuanui et al., 2015), water pH decrease (ocean acidification) (Anthony et al., 2011; Burke et al., 2012), increased level of sediment till become covering corals and blocking sunlight to coral (affect photosynthesis of coral-photobionts) (Peters, 1984), and dysbiosis of the coral-associated microbiome (Ritchie, 2006; Bourne D. G. et al., 2008). This symbiotic relationship involves algal Symbiodinium (also known as zooxanthellae), prokaryotes (bacteria and archaea), eukaryotic microbes, and viruses and was reported dynamic and differentially associated in healthy vs. bleaching state, and bleaching-sensitive vs. -tolerant corals (Rohwer et al., 2002; Rosenberg et al., 2007; Boilard et al., 2020). Corals provide habitat to the coral symbionts (algae and microbes), while the microbial symbionts provide corals with nutrients through photosynthesis and/or decomposition of organic and inorganic substances, competition for space and nutrients against coral pathogens, and antibiotics or antioxidants to promote coral resistance against pathogens and coral bleaching (Lesser et al., 2004; Rosenberg et al., 2007; Lema et al., 2012; Webster and Reusch, 2017). The compositions of these beneficial microbes and negatively impacted microbes affect how well different coral hosts (i.e., coral species) sensitive to thermal bleaching (Csaszar et al., 2010; Leggat et al., 2011; Gardner et al., 2019). Among the coral genera affected by bleaching, some genera were more bleaching tolerant than the others (e.g., Porites > Acropora), likely due to the differences in host genetic and their symbiont compositions (Phongsuwan and Chansang, 2012; Gardner et al., 2019). The severe 2010 and 2016 bleaching events found that Acropora spp. is more prone to bleaching-induced mortality (75–100%) than Porites spp. (25–49%), in which scientists had suggested to be associated with the different Symbiodinium clades between Acropora and Porites (C3 and C15, respectively) (Fisher et al., 2012; Phongsuwan and Chansang, 2012; Gardner et al., 2019). Additionally, a shift of Symbiodinium clades from type C in healthy Acropora millepora to type D in bleached A. millepora (Berkelmans and van Oppen, 2006; Oliver and Palumbi, 2011) and the higher abundance of coral pathogens had been reported in bleached Acropora (Roder et al., 2014; Kusdianto et al., 2021).
Besides, studies reported different coral-associated microbiome patterns among coral genera and sites (Gardner et al., 2019). For instance, Pogoreutz et al. (2018) discovered the consistent pattern of, in particular, the dominance of order Oceanospirillales in coral Pocillopora verrucosa in Saudi Arabia coastal and other marine resources albeit healthy to bleaching and mortality state corals and suggested that the range in microbial flexibility may correlate an ability of corals to serve as hosts. This microbiome specificity to coral genera (or coral species) might affect the corals in adaptive to environmental changes. Many scientists, including Morrow et al. (2018) described the diversity of microbiomes associated with coral abilities to respond to thermal bleaching events and other environmental stresses (e.g., nutrient limitation). For sites, different marine geographies in the world affect reef types and climates, and thus, the microbiome diversity (Somboonna et al., 2017). Further, van Oppen and Blackall (2019) also pointed the temporal factor on microbiome changes, and specific microbiomes could support acclimatization of the corals to climate warming, heightening the coral insensitivity to bleaching (Gardner et al., 2019). Supportively, scientists found that microbiome transplantation using homogenized coral tissues of heat-tolerant donors Porites and Pocillopora in the wild could allow the recipient corals to bleach at lower rates (Doering et al., 2021). In vice versa, certain coral-associated microbiomes might cause coral to bleach at normal to high rates. Nevertheless, specifically preferred microbiomes for bleaching resilience remained none classified to date in the Andaman Sea of Thailand.
Following the aforementioned reports that successfully utilized universal 16S and 18S rRNA genes to analyze coral associated prokaryote and eukaryote diversities (Hadziavdic et al., 2014; Wang et al., 2014; Banos et al., 2018; Kusdianto et al., 2021), this report, therefore, utilized similar methods [16S and 18S rRNA genes next-generation sequencing (NGS)] to describe prokaryotic and eukaryotic microbiomes in healthy and bleached corals Acropora humilis, Platygyra sp., Pocillopora damicornis, and Porites lutea, in the Andaman Sea around Racha Yai Island, Phuket province, along with the microbiomes of the surrounding seawater and sediments, during a thermal bleaching event in 2016. Previously, the coral-associated microbiome of prokaryotes during the 2016 bleaching event in the Andaman Sea had been investigated (Pootakham et al., 2018); however, that study only reported on P. lutea leaving other three coral genera that are prevalent in the studied locations and also did not include analyses of the eukaryote microbiomes. Therefore, the present study included alpha and beta biodiversity analyses of both prokaryotic and eukaryotic microbiota of healthy and bleached colonies of four prevalent coral species. Our and other data will be crucial to allow characterization of the coral-beneficial microbes (or microbiome) against thermal bleaching events in the Andaman Sea, supporting the microbe-mediated strategies to protect and recover corals from thermal bleaching events (van Oppen and Blackall, 2019).
Materials and Methods
Sample Collections
Corals of species A. humilis (abbreviated as CA), Platygyra sp. (CT), P. damicornis (CC), and P. lutea (CR), seawater (W), and sediment (S) samples were collected from reef around Racha Yai Island (7°36′27.9″N 98°22′40.9″E), in Phuket province, Thailand (Figure 1A). Three independent replicates were collected for each sample. Healthy (H) and bleached (B) coral colonies were determined via their appearance [healthy coral colony appeared light or dark brown color (natural color, 0% sign of bleaching), and bleached coral colony appeared white color by on-site marine scientist divers (S. Chavanich, S. Jandang, and V. Viyakarn) (Bulan et al., 2018a,b; Kusdianto et al., 2021)]. The four coral genera had an identical bleached appearance, and the bleached sample was collected in the bleached area (completely white area) of coral color that bleached more than 50%. Of each coral sample, a fragment approximately 5 cm in length and 5 cm in diameter (for branching corals Acropora and Pocillopora, the samples were approximately 5 cm in length, while the compound colony Porites, approximately 5 cm in diameter of each sample was collected), at approximately 8–10 m depth from the sea surface, was collected haphazardly using hands in gloves, and the distance between each sampled colony was approximately 5 m. Seawater (6 L) was collected directly above each of the sampled coral colonies, and sediment (approximately 50 g) was collected directly below each of the sampled coral colonies. All samples were collected at the same day and time (around noon) during the global thermal bleaching event in May 2016, and at the same site and thus, the environmental factors were the same. All samples were transported immediately within the same day to the laboratory and stored in −20°C. The abbreviations for samples are sample (CA, CT, CC, CR, W, or S) followed by coral condition (H or B) (for coral) and independent replicate number (1, 2, or 3). For example, CAH1 represents healthy Acropora coral of independent replicate number 1.
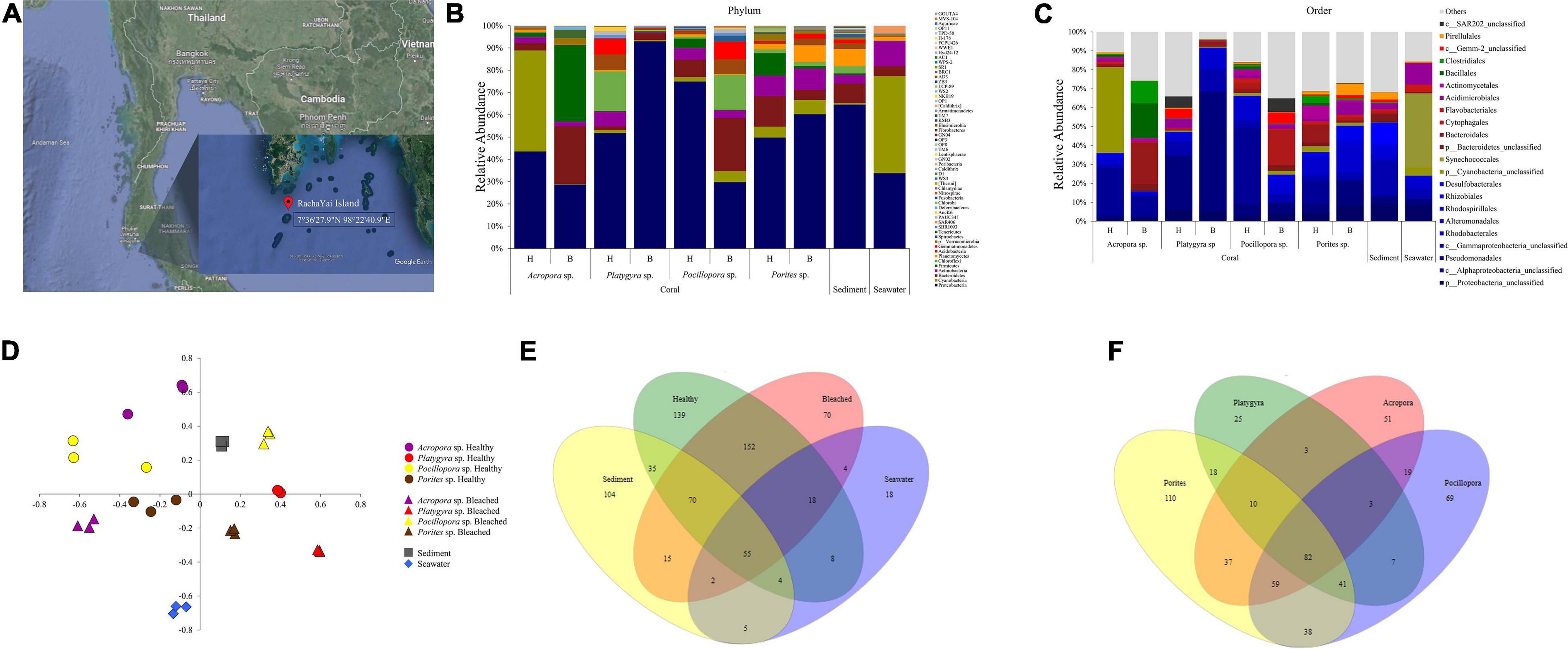
Figure 1. (A) Map representing sampling site of Racha Yai Island in the Andaman Sea; average bacterial compositions in coral, sediment, and seawater samples at (B) phylum level and (C) order level; (D) their non-metric multidimensional scaling analyses based on ThetaYC index at genus level; and Venn diagrams showing the numbers of shared and unique OTUs (E) among healthy corals, bleached corals, and surrounding sediment and seawater; and (F) among four coral species Acropora humilis, Platygyra sp., Pocillopora damicornis, and Porites lutea. In panel C, “Others” represent order OTUs with <1% abundance. For OTU classification where order could not be identified, the deepest classification level was given (abbreviated p for phylum and c for class).
Metagenomic Extraction
Coral samples (containing surface mucus layer, tissue, and skeleton) were ground using a sterile mortar and pestle, and 1 g of coral fragment and 1 g of sediment were used. Noted several sampling techniques to profiling the coral microbiome, including grinding entire coral like ours (Bulan et al., 2018a,b; Hernandez-Agreda et al., 2018; Pootakham et al., 2018; Rosales et al., 2019), collecting only surface mucus layer (Badhai et al., 2016), or scraping some tissue (Glasl et al., 2017), were reported. The surface mucus layer and tissue are important as an interface habitat between the coral and the surrounding (Glasl et al., 2016; Hadaidi et al., 2017), while the skeleton showed roles in physico-chemical nutritional exchanges and decalcification, which coral bleaching also affected alteration in skeleton microbiome and scientists discussed the skeleton may function as a microbial reservoir for tissue microbiome recolonization, i.e., following bleaching dysbiosis to support coral microbiome return to homeostasis (Ainsworth et al., 2015; Ricci et al., 2019). For seawater, 2.5 L was filtered through a sterile 0.22-μm filter membrane (Merck Millipore, MA, United States) and the filtered membrane was used. Metagenomic DNA was extracted using Power Soil DNA Isolation Kit for coral and sediment samples, and Power Water DNA Isolation Kit for seawater samples (MoBio, Carlsbad, CA, United States), following the instructions of the manufacturer and previous works of literature (Bulan et al., 2018a,b; Kusdianto et al., 2021). The quantity and quality of the extracted DNA were analyzed by NanoDrop™ 2000 spectrophotometer (Thermo Fisher Scientific, Waltham, MA, United States) and agarose gel electrophoresis (0.55% agarose gel w/v). The metagenomic DNA was stored at −20°C until further analyses.
16S and 18S rRNA Gene Library Preparation and Next-Generation Sequencing
The 16S rRNA gene V4 region and 18S rRNA gene V9 region were amplified by PCR using universal prokaryotic primers 515F (5′-GTGCCAGCMGCCGCGGTAA-3′) and 806R (5′-GGACTACHVGGGTWTCTAAT-3′), and universal eukaryotic primers Illumina_Euk_1391F (5′-GTACACACCGCCCGTC-3′) and lllumina_EukBr (5′-TGATCCTTCTGCAGGTTCACCTAC-3′), to create libraries of prokaryotes and microbial eukaryotes (fungi and small eukaryotes), respectively, according to Caporaso et al. (2012). Noted the 18S rRNA gene V9 is used elsewhere as adequate for analyzing microbial eukaryotic lineages (Vestheim and Jarman, 2008; Amaral-Zettler et al., 2009; Stoeck et al., 2010; Hadziavdic et al., 2014; Wang et al., 2014; Gong and Marchetti, 2019) and including the standard Earth Microbiome Project protocols (earthmicrobiome.org); and the 18S rRNA gene sequence database is increasingly large to support operational taxonomic units (OTUs) classification for microbial eukaryotes (Banos et al., 2018). For each sample, a 25-μl PCR reaction comprised 1 × EmeraldAmp ® GT PCR Master Mix (TaKaRa, Shiga, Japan), 0.3 μM of each primer, and 75 ng of the metagenome DNA. The 18S rRNA gene PCR also included 10 μM of a mammal-blocking primer (5′-GCCCGTCGCTACTACCGATTGGIIIIITTAGTGAGGCCCT3S pC3-3′) (Caporaso et al., 2012). The 16S rRNA gene PCR conditions were 94°C for 3 min, and 30 cycles of 94°C for 45 s, 50°C for 60 s, and 72°C for 1 min 30 s, followed by 72°C for 10 min; and the 18S rRNA gene PCR conditions were 94°C for 3 min, and 30 cycles of 94°C for 45 s, 65°C for 15 s, 57°C for 30 s, 72°C for 90 s, followed by 72°C for 10 min. Of each sample, independent triplicate PCRs were performed and pooled to prevent stochastic bias. PCR products of ≈381 bp (16S rDNA) and ≈260 bp (18S rDNA) in length were excised from agarose gels and purified using GF-1 Gel Extraction Kit (Vivantis Technologies Sdn Bhd, Selangor, Malaysia). Qubit™ dsDNA HS assay kit (Invitrogen, Waltham, MA, United States) and Qubit 3.0 Fluorometer (Thermo Fisher Scientific) were used to quantify the dsDNA of the PCR products, and 200 ng of each sample was sequenced on Miseq 300 NGS platform (Illumina, San Diego, CA, United States) at OMICS Sciences and Bioinformatics Center, Faculty of Science, Chulalongkorn University (Bangkok, Thailand).
Bioinformatics and Statistical Analyses
Sequences were processed according to Mothur’s standard operating procedure (SOP) (Schloss et al., 2009). For quality screening, reads containing (i) ambiguous bases; (ii) >1 mismatch base in the reverse primer region, (iii) >10 homopolymer, (iv) sequence length <100 bp, and (v) chimera sequence, were removed. Silva databases (version 1.32) were used to align the sequences and remove contaminated sequences, such as mitochondria and chloroplast sequences. For taxonomic classification into OTUs, the quality 16S and 18S rDNA sequences were determined using Mothur (Schloss et al., 2009) and Greengenes (version 13.8); and Good’s coverage index was computed to estimate a sequencing coverage (sequencing depth) of each sample. Then, these data samples for 16S and 18S rRNA genes sequences were normalized at 9,045 and 38,429 sequences per sample to prevent a bias from various sequencing depth across samples when comparisons among samples and groups were performed; noted a data sample with fewer sequences than the normalization (samples CAH2-3, CCH1-3, and CRH1-3) was pooled with other independently replicate sample followed by subsampling to normalization or using all those sequences. Venn diagram, alpha diversity (Chao1 richness, and Shannon diversity indices), and beta diversity [thetaYC dissimilarity index and non-metric multidimensional scaling (NMDS)], with default parameters, were determined using Mothur (Schloss et al., 2009; Kusdianto et al., 2021).
Mean and SD were computed. For statistical analysis, the Student’s t-test and analysis of similarities (ANOSIM) were used to test for significance between and among groups (p < 0.05).
Availability of Supporting Data
Nucleic acid sequences of 16S and 18S rDNA were deposited in an NCBI open access Sequence Read Archive database, accession number PRJNA700134.
Results
16S and 18S rRNA Gene Sequencings and Alpha Diversity
From the sequencing results, a total of 766,434 quality reads for 16S rRNA gene sequences and 6,103,914 quality reads for 18S rDNA sequences were obtained. The average quality reads per sample were 25,548 and 203,464 for 16S and 18S rRNA genes sequences, respectively; and hence, the Good’s coverage indices demonstrated relatively sufficient sequencing depth: genus-level OTUs were averaged at 98.89 and 99.99% in 16S and 18S rRNA genes, respectively (Supplementary Tables 1, 2).
The alpha diversity (a measurement of biodiversity to a single sample) of corals and sediments demonstrated the greater genus richness (represented by OTUs and Chao index) for prokaryotes than microbial eukaryotes, meanwhile in seawater demonstrated similar genus richness between prokaryotes and microbial eukaryotes (Supplementary Tables 1, 2 and Supplementary Figures 1A,C). In corals and at the genus level, prokaryotes had an average (avg.) of 252.71 OTUs, microbial eukaryotes avg. 60.42 OTUs; in sediments, prokaryotes had avg. 504.67 OTUs, microbial eukaryotes avg. 143.67 OTUs; and in seawater, prokaryotes had avg. 189.00 OTUs, and microbial eukaryotes avg. 227.67 OTUs. Hence, the highest richness was observed for prokaryotes in sediment samples (avg. Chao 581.05) followed by corals (avg. Chao 318.48) and seawater (avg. Chao 254.00; Supplementary Table 1). Analyzing together with the genus evenness (represented by Shannon index) found a similar trend: corals and sediments had the higher biodiversity for prokaryotes than microbial eukaryotes (with the highest in coral Porites (avg. Shannon 4.06) than the other three coral genera (avg. Chao 2.87)), meanwhile, seawater demonstrated similar biodiversity between prokaryotes and microbial eukaryotes (Supplementary Tables 1, 2 and Supplementary Figures 1B,D). For microbial eukaryotes, both genus richness and evenness were observed the highest in seawater (avg. Chao 245.89, avg. Shannon 2.92), followed by sediment (avg. Chao 164.73, avg. Shannon 1.96), and corals (avg. Chao 74.19, avg. Shannon 1.00) (Supplementary Figures 1C,D).
Interestingly, comparing between healthy and bleached coral conditions showed an alteration in their biodiversity, both in genus richness and evenness (Supplementary Figures 1, 2). For prokaryotes, bleached corals showed less biodiversity in Platygyra and Porites. For microbial eukaryotes, bleached corals showed less biodiversity in Acropora, Platygyra, and Pocillopora.
Beta Diversity Analyses for Prokaryotic Communities
While the relatively close biodiversity compositions within independently triplicate samples (p > 0.01) are shown in Supplementary Figure 2A, the diverse biodiversity compositions were observed for corals of different genera and sample types (corals vs. sediment vs. seawater). Analyzing the relative abundance at the phylum level revealed that Proteobacteria (avg. 53%) was the dominant phylum across all sample types and coral genera (Figure 1B). For the other dominant phyla, each sample group displayed quite a distinct prokaryotic composition; for example, the high abundance of Cyanobacteria was found only in seawater and healthy Acropora. Moreover, a dysbiosis of prokaryotic compositions was found specific to each bleached coral genus; and the dysbiosis between the healthy and bleached conditions was found more distinct in coral Acropora, Pocillopora, and Platygyra than Porites, in orderly (Figures 1B,D). For instance, the dominant Cyanobacteria (Figure 1B: avg. 45.34%) in healthy Acropora was changed to Bacteroidetes (avg. 25.93%) and Firmicutes (avg. 34.41%) in bleached Acropora, and the bacterial alteration was found in bleached Porites where the abundances of Firmicutes, Bacteroidales, Pseudomonadales, and Actinomycetales were reduced and the abundances of Planctomycetes, Rhodobacterales, Acidimicrobales, Rhizobiales, and Pirellules increased in proportion. Surprisingly, a contradictory trend was observed for Gemmatimonadetes, Acidobacteria, and Chloroflexi, which were present in relatively high abundances in healthy than in bleached Platygyra. It was noted that at phylum level analyses, the prokaryotic composition in bleached Porites to that in the sediment and the prokaryotic composition in CAH2 and CAH3 to that in seawater were quite similar (Figure 1B and Supplementary Figure 2A).
When analyzing the relative abundance at the order level, the prokaryotic compositions showed the clearer distinct in all sample types, and the similarity of bleached Porites to sediment and healthy Acropora to seawater was no longer observed (Figure 1C). The results also showed a specific dysbiosis of prokaryotic composition in bleached vs. healthy conditions of every coral genus; for example, in Acropora, an unclassified order of phylum Cyanobacteria was decreased in abundance while Bacteroidales, Bacillales, and Clostridiales were increased when undergoing bleaching. For Platygyra, an unclassified order of class Alphaproteobacteria and Gammaproteobacteria was predominantly present in healthy Platygyra; however, in bleached conditions, these bacterial taxa were increased even further along with Bacteroidales and Alteromonadales. For Pocillopora, healthy coral exhibited a greater proportion of Pseudomonadales and Rhodobacterales while bleached coral showed a higher proportion of Cytophagales, Rhodospirillales, and an unclassified order of class Gemm-2 and SAR202. Interestingly, a common bacterial alteration was observed among the coral genera, which is the reduction of order Pseudomonadales shared across three bleached coral genera (Acropora, Pocillopora, and Porites) (Figure 1C), which may suggest its importance in healthy corals.
The beta diversity (a measurement of biodiversity among samples) via NMDS analysis further demonstrated the community separation among coral genera. All bleached communities demonstrated separation from their healthy communities with the most separation being Acropora (Figure 1D), which is likely due to the aforementioned alteration seen in Figure 1C for each coral genus.
These results indicated that the prokaryotic profile of each coral genus is unique. Moreover, the results demonstrated that bleaching in coral may be associated with prokaryotic dysbiosis; however, the degree of alteration and species of prokaryotic changes may differ per coral species.
Beta Diversity Analyses for Microbial Eukaryotic Communities
The eukaryotic profiles obtained from 18S rRNA gene sequencing revealed multiple kingdoms of eukaryotes both unicellular and multicellular organisms, with the top abundance being Chromista (Supplementary Figure 2B: avg. 88.23%) followed by Animalia (9.10%), Plantae (0.86%), Fungi (0.23%), and then Protists (0.12%). At the phylum level, Dinoflagellata (avg. 73.49%) dominated the eukaryotic composition of all coral genera, while Ochrophyta (13.92%) dominated that of sediment and seawater. In addition to Ochrophyta, phyla Arthropoda, Protalveolata, and Dinoflagellata were also found to be dominant in seawater samples of Racha Yai Island. In certain coral genera (Platygyra, Pocillopora, and Porites), a decent abundance of Porifera (6.08%) was also observed as part of the dominant phyla; however, this is not the case for both coral states, such as bleached Pocillopora (0.0016%) and healthy Porites (0.007%) (Figure 2A). This result indicated the different eukaryotic profiles of coral, sediment, and seawater samples.
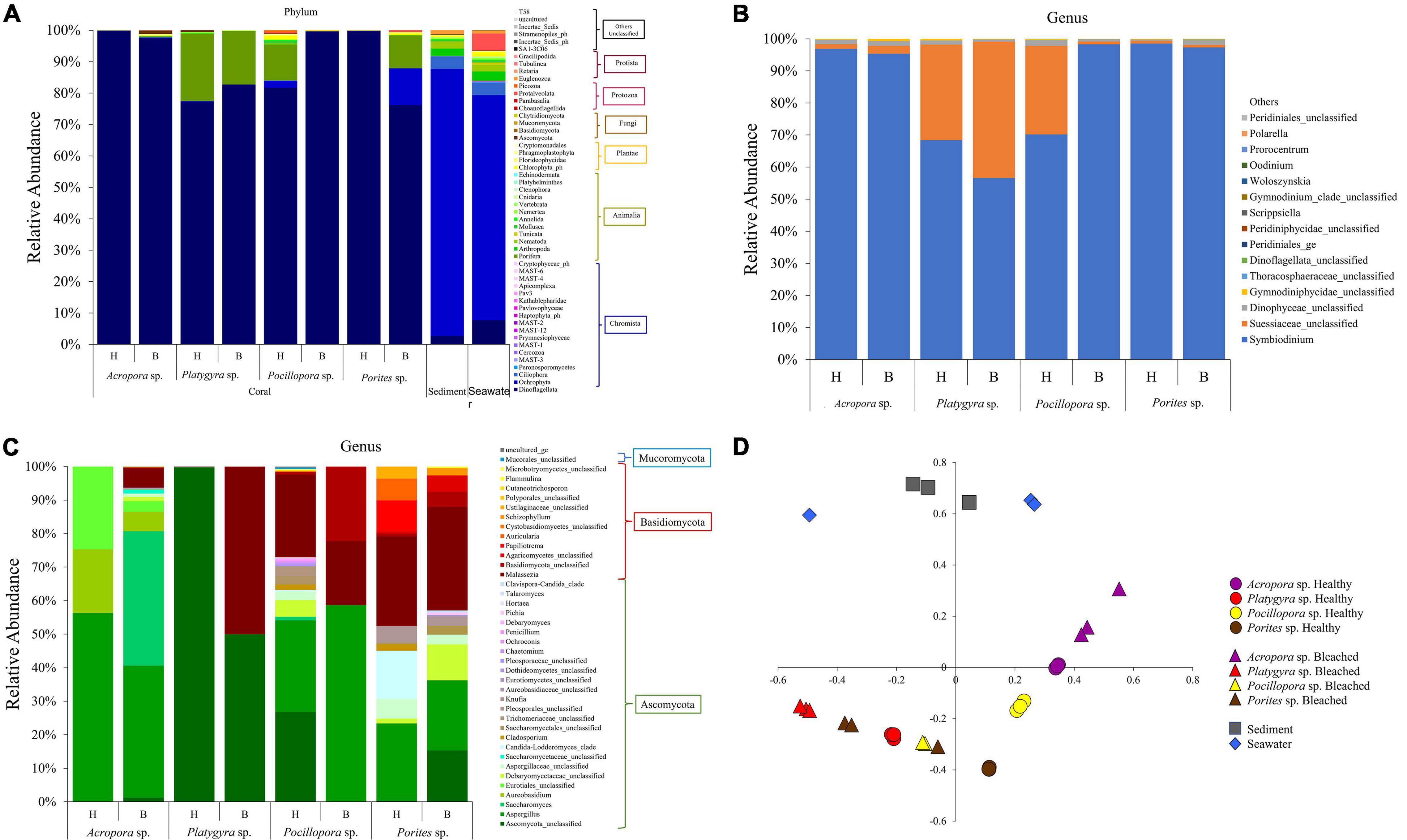
Figure 2. Average microbial eukaryotic compositions in coral, sediment, and seawater samples at (A) phylum level and (B,C) genus level (genus compositions of (B) Dinoflagellata and (C) fungi); and (D) their non-metric multidimensional scaling analyses based on ThetaYC index at the genus level. In panels (B,C), “Others” represent genus OTUs with <1% abundance. For OTU classification where genus could not be identified, the deepest classification level was given.
The composition of microbial eukaryotes Dinoflagellata (a single-cell protist and usually considered algae) and Fungi at a genus level was specifically analyzed to characterize the profiles at the genus level (Figures 2B,C, respectively). The result showed that most of the Dinoflagellata found were classified as Symbiodinium and that it is highly abundant in all coral genera. An unclassified genus of the family Suessiaceae was also observed to be highly abundant in healthy corals specifically in Platygyra and Pocillopora. Comparing healthy and bleached corals, the abundance of Symbiodinium was found to be reduced in all bleached coral genera except for bleached Pocillopora (Figure 2B and Supplementary Figure 2B). The finding supported previous knowledge of the symbiotic relationship of Symbiodinium to healthy state coral. However, for the Pocillopora genus, the replacement of Symbiodinium by an unclassified genus of the family Suessiaceae (from avg. 22.54% to avg. 0.87%), which was opposite to that in bleached Platygyra, might highlight one coral-holobiont advantageous for thermal-resistant Pocillopora.
Investigation into the fungal communities showed that each coral genus comprised quite a unique composition of fungal genera in mainly Ascomycota and Basidiomycota (Figure 2C). Overall, the result revealed that Pocillopora and Porites were more associated with diverse fungal communities than Acropora and Platygyra. For example, healthy Platygyra was revealed to compose solely of an unclassified genus of phylum Ascomycota while other coral genera were associated with a more diverse fungal community. Nevertheless, a shared fungal genus was observed for Aspergillus abundance in three coral genera (Acropora, Pocillopora, and Porites), which may suggest their association in the coral. When compared between healthy and bleached corals, the abundance of Malassezia was found to be commonly increased in bleached conditions of three coral genera (Acropora, Platygyra, and Porites) apart from Pocillopora, which suggested their pathogenicity in the coral (Amend, 2014; Kusdianto et al., 2021). Other observations include abundance shifts of Eurotiales (Supplementary Figure 2C) to Saccharomyces in bleached Acropora, declining fungal diversity in bleached Pocillopora (remaining genera: Aspergillus, Malassezia, and an unclassified genus of phylum Basidiomycota), and the fungal dysbiosis in bleached Porites where an unclassified genus of phylum Ascomycota and an unclassified genus of family Debaryomycetaceae both increased in their abundance while Candida-Lodderomyces, an unclassified genus of family Pleosporaceae and Chaetomium, diminished.
Non-metric multidimensional scaling analysis in Figure 2D showed that the microbial eukaryotic diversity between sample types (corals vs. sediment, seawater) was separate; and Platygyra, Pocillopora, and Porites shared some overlapping community positions, while Acropora communities were more disparate. Of each coral genus, slight separation between healthy and bleached profiles was observed. The results also indicated that the microbial eukaryotic composition obtained by 18S rRNA gene sequences in each coral genus is quite similar due to the dominant abundance of Dinoflagellata composition (Figure 2A). However, the dysbiosis caused by the bleaching event was observable and the compositions of fungi were revealed to be unique in each coral genus.
Identification of Important Bacteria and Fungi Operational Taxonomic Units in Healthy and Bleached Corals
Specific analyses were performed on the microbial taxa that were known to be associated with coral bleaching which include bacterial orders Vibrionales, Oceanospirillales, Alteromonadales, Rhodospirillales, Rhizobiales, and fungal genus Aspergillus, for example (Supplementary Figure 3). Vibrionales were reported to be associated with bleached corals and were found to increase during heat stress (Kushmaro et al., 1998; Bourne D. et al., 2008; Tout et al., 2015); however, in the present study, the Vibrionales abundance was relatively low in all coral genera and was almost absent in bleached corals. Oceanospirillales were shown to be involved in dimethylsulfoniopropionate (DMSP) degradation and antimicrobial compounds production, which may provide nutrients and resistance against coral diseases (Kirkwood et al., 2010; Raina et al., 2016). Apparently, this is likely the case for Pocillopora (from 0.49 to 0.09%) and Porites (from 0.84 to 0.13%) genera, which showed a significant reduction in Oceanospirillales in bleached corals; however, in bleached Acropora (from 0.40 to 0.72%) and bleached Platygyra (from 0.93 to 1.69%), Oceanospirillales were found to be increased instead. Alteromonadales were reported to be beneficial as nitrogen fixers and the nitrogen availability serves as one key for Symbiodinium growth, so they were generally associated with healthy state corals (Ceh et al., 2013; Rädecker et al., 2015); however, they were sometimes reported to be increased when corals are stressed or developing diseases, perhaps might mediate coral or algal parasitism growths (Sunagawa et al., 2009; Morris et al., 2019). In the present result, Acropora, Pocillopora, and Porites all exhibited a reduction of Alteromonadales in bleached corals; however, in Platygyra, Alteromonadales were significantly increased when bleached. Finally, Rhodospirillales and Rhizobiales were known for their role in nitrogen fixation (Lema et al., 2014; Tsoy et al., 2016). Interestingly, these bacterial orders (Rhodospirillales and Rhizobiales) were found to be increased in bleached Pocillopora and bleached Porites, suggesting their role in adapting to thermal stresses in these coral genera.
To date, the role of fungi in coral bleaching is very limited. Aspergillus sydowii was reported to be causing diseases in corals (Geiser et al., 1998; Smith and Weil, 2004). In analyzing the abundance of Aspergillus, the present result obtained a relatively low abundance of this fungal genus. However, the Aspergillus abundance was found to be increased in bleached Acropora, which could play its pathogenic role during a thermal bleaching event (Alker et al., 2001; Kusdianto et al., 2021). Additionally, increased temperature affects coral immune vulnerability and thus decreasing coral resistance and increasing coral pathogen diseases (Ward et al., 2007).
Discussion
Global warming has negatively impacted massive corals including those in the Andaman Sea for over decades since 1991 (Phongsuwan and Chansang, 2012). The importance of coral-associated microbiomes is recently being recognized as they function fundamentals to the coral developmental cycle, ranging from providing nutrients, antimicrobial substances, and microbial metabolites, to competition against pathogens, which together could support the ability of corals to resist or adapt to harsh environments, including thermal bleaching event (Lesser et al., 2004; Kelman et al., 2006; Rosenberg et al., 2007; Lema et al., 2012; Webster and Reusch, 2017). Phuket province is surrounded by coral reefs of four prevalent coral genera; however, their coral-associated microbiomes of prokaryotes and microbial eukaryotes have not been determined with respect to the thermal bleaching event. Subsequently, this study aimed to provide the prokaryotic and eukaryotic microbial profiles of these four prevalent coral genera (Acropora, Platygyra, Pocillopora, and Porites) in healthy and bleached status in Phuket province, Andaman Sea.
Revealing the microbial diversity at the genus level, a similar pattern to our previous study conducted in the upper Gulf of Thailand in the year 2016 was obtained where the sediment was evaluated to have the highest prokaryotic richness among all sample types and where seawater was shown to have the highest microbial eukaryote richness (Supplementary Figures 1A,C; Hoshino et al., 2020; Kusdianto et al., 2021). Moreover, a similar result to Kusdianto et al. (2021) was observed in microbial diversity in coral samples, which comprised a higher richness of bacteria than that of microbial eukaryotes (Supplementary Figures 1B,D). Compared to the coral genera, Porites displayed relatively high biodiversity in both prokaryotic and microbial eukaryotic profiles (Figure 1). This result is in good agreement with a previous study from Gardner et al. (2019) who revealed a higher microbial diversity in heat-tolerant corals Porites and Coelastrea, than in a heat-sensitive coral, Acropora. Interestingly, when bleached these coral genera, they demonstrated the unique dysbiosis to their prokaryotic and microbial eukaryotic biodiversity (Figure 1 and Supplementary Figure 1). This species alteration could be due to the specific microbial composition and unique coral genus host-bacterial interaction (Bourne D. et al., 2008; Carlos et al., 2013; McDevitt-Irwin et al., 2017; Osman et al., 2020; Sun et al., 2020). Venn diagrams showed that bleached corals had relatively fewer number of genus OTUs than healthy corals in approximately half the number (Figure 1E; Pollock et al., 2019); nonetheless, this lower alpha diversity was not held true to every coral species, in part highlighting the report that the alpha diversity of the stressed corals, regardless of the stressor, could carry the more alpha diversity (McDevitt-Irwin et al., 2017), and these differences might be associated with coral species and their associated microbiome (Kusdianto et al., 2021). Further, among four coral genera, Pocillopora and Porites had the highest number of OTU diversity (Figure 1F), which supported the previous reports of heat-tolerant Porites and Pocillopora in the wild and that the microbiota from the heat-tolerant Porites and Pocillopora in the wild could help the recipient corals to bleach at lower rates (Doering et al., 2021).
The symbiotic relationship between corals and photosynthetic dinoflagellates (Symbiodinium) is well recognized for the symbionts’ provision of nutrients and energy required by the corals (Falkowski et al., 1984; Mieog et al., 2009). Unfortunately, this positive interaction between corals and Symbiodinium can be easily broken with the slight increase of seawater temperature, leading to the bleaching phenomenon (Salih et al., 1997; Gardner et al., 2019). In profiling, the coral-associated microbiome during the 2016 thermal event in Racha Yai Island, a consistent reduction of Symbiodinium was found in three bleached corals genera (Acropora, Platygyra, and Porites) (Figure 2B and Supplementary Figure 2B). Even though the reduction of Symbiodinium was relatively low, this might make insufficient to support the healthy state (Sebastian et al., 2009; Cunning et al., 2017). The remaining Symbiodinium might also comprise of different clades/types and confer a different degree to support the healthy coral phenotype (Berkelmans and van Oppen, 2006; Stat et al., 2008; Gardner et al., 2019). This was previously reported in the case of Pocillopora that exhibited an increased proportion of putative thermal-resistant clade D Symbiodinium in a bleached state (Berkelmans and van Oppen, 2006; Panithanarak, 2015) and supported our finding of relative abundant Symbiodinium in bleached Pocillopora. Meanwhile, Pocillopora was previously reported to exhibit variable zooxanthellae species composition, concordant with inferred thermal stress, and thus, the smaller percentage of Symbiodinium in our reported healthy Pocillopora might merely be due to the great diversity of zooxanthellae species composition during its healthy state (Torres et al., 2021). Moreover, comparing among coral genera, it was observed that Platygyra and Pocillopora did not depend solely on Symbiodinium but that they also relied on an ancient photosynthetic dinoflagellate, Suessiaceae, which was suggested to be symbionts of the earliest Scleractinian corals, evolving through the Triassic period (Palliani and Riding, 2000; Boulotte et al., 2016; Janouškovec et al., 2017) and was present in a high proportion in healthy Platygyra and Pocillopora. Thus, in terms of supporting healthy state corals for Pocillopora could be explained by the abundance of Suessiaceae, while in Platygyra the proportion is relatively high yet in bleaching state (Figure 2B). However, our reported numbers are all in relative abundance percentage, and thus in future studies, we plan to include the copy numbers of each species (as known as quantitative microbiota) to reveal the microbial abundance in explicit numbers as copies (Steinert et al., 2020) and also may include the analyses of ITS and/or 18S rRNA gene V4 region together with the 18S rRNA gene V9 region to maximize the characterization for phototrophic communities (Bradley et al., 2016).
Differences in bleaching susceptibility among coral genera were not entirely resolved by Symbiodinium abundance and composition but were complemented by the associated bacterial communities (e.g., bacteria of carbon synthesis function to replace Symbiodinium’s photosynthesis function and bacteria of nitrogen synthesis function, for coral growth) (Rädecker et al., 2015; Cunning et al., 2017; Sweet and Bulling, 2017; Morrow et al., 2018). Compared to the microbial eukaryotic profiles, the prokaryotic community shifts between healthy and bleached corals are more apparent in all coral genera (Figure 1). This is likely due to the wide metabolisms and rapid generation times of the prokaryotes making them more rapid in responding to stresses than Symbiodinium (Pogoreutz et al., 2018). In examining the coral genera, each coral genus exhibited a unique bacterial composition as evidenced in relative abundance and NMDS analyses (Figure 1). This is likely due to the selection of host-specific microbiome, which finetuned a distinct microbial assemblage for each coral genus (Bourne D. et al., 2008; Bernasconi et al., 2019; Damjanovic et al., 2020; Kusdianto et al., 2021). Nevertheless, common bacterial community shifts of order Pseudomonadales and Alteromonadales were observed, which were present in more abundance across three healthy coral genera (Acropora, Pocillopora, and Porites) but declined in bleached corals (Figure 1 and Supplementary Figures 2A, 3). This is similar to the previous report of Pseudomonadales where its abundance was replaced by other pathogenic bacteria when undergoing a bleaching event (Rosenberg et al., 2007) and is also in good agreement with its role in inhibiting coral pathogens in soft coral Sarcophyton glaucum (ElAhwany et al., 2015). As Alteromonadales was reported to be able to degrade toxic DMSP to a carbon source of food (Reisch et al., 2011; Raina et al., 2013), this further supported the abundance of these taxa in healthy corals. Although relatively low in their abundance, Vibrionales was surprisingly found in healthy corals across all coral genera and was found to decline in bleached coral samples (Supplementary Figure 3). This was unexpected as Vibrionaceae were reported to be opportunistic and potentially pathogenic bacteria associated with coral diseases and coral bleaching (Bourne D. et al., 2008; Tout et al., 2015). However, this pattern was similar to that of our previous study in corals around the upper Gulf of Thailand (Kusdianto et al., 2021), which could be due to the nitrogen-fixing ability in certain Vibrio spp. (Criminger et al., 2007; Chimetto et al., 2008). Reshef and colleagues (Reshef et al., 2006; Rädecker et al., 2015) proposed “coral probiotic hypothesis” that the relationship between symbiotic microbes and their coral host was dynamic and might evolutionarily select toward the advantageous compositions of the coral holobionts under varying environmental conditions in a year. Other changes were found to be specific to coral genera, where Acropora and Platygyra shared a similar alteration while Pocillopora and Porites shared the same fate of bacterial dysbiosis when bleached to orders Oceanospirillales, Rhizobiales, and Rhodospirillales (Figure 1C and Supplementary Figure 3). It is still unclear how these bacterial taxa that were reported to play roles in carbon and sulfur recycling processes (Oceanospirillales) (Raina et al., 2016), and nitrogen fixation (Rhizobiales and Rhodospirillales) (Lema et al., 2014; Tsoy et al., 2016) were more abundant in a healthy state of certain coral genera but were also more abundant in a bleached state of other coral species. Nevertheless, it is hypothesized that the specific interaction put by these bacterial species and their host which was unique for each coral genus could be the underlining reason. Moreover, these specific community shifts for each coral genus were also evidenced in previous studies where specific bacterial taxa (e.g., Vibrionales, Rhodobacterales, Alteromonadales, Rhizobiales, Rhodospirillales, and Oceanospirillales) were found to be dominant in a healthy state of certain coral species but were also more abundant in a bleached state of other coral species (Bourne and Munn, 2005; McDevitt-Irwin et al., 2017; Sun et al., 2020).
For the fungal communities, although unique patterns were observed against coral genera, common increases of genus Malassezia for bleached Acropora, Platygyra, and Porites were observed. Another alteration in the fungal community was the decrease of genus Aspergillus for bleached Acropora and unclassified genus in family Aspergillaceae for bleached Pocillopora and Porites (Figure 2C). The association of Malassezia to coral bleaching is yet unknown; however, its pathogenic role had been reported in association with skin disorders in the human for Malassezia (McGinley et al., 1975; Velegraki et al., 2015). For Aspergillus, its role as an aspergillosis disease in coral communities had been reported (Geiser et al., 1998; Kim and Rypien, 2015). Moreover, the increase of these fungal taxa in bleached corals is similar to our previous study in 2016 thermal bleaching event in the upper Gulf of Thailand (Kusdianto et al., 2021).
Conclusion
In summary, as Thailand is bounded by the coastlines of the Andaman Sea on one side and the Gulf of Thailand on the other side (Figure 1A), this presents a slight difference in marine geography (e.g., the corals in the Andaman Sea have more hard corals than in the Gulf of Thailand). Following our first report of both prokaryotic and microbial eukaryotic profiles associated with coral genera and their sediment and seawater surroundings in the upper Gulf of Thailand (Kusdianto et al., 2021), the present study continued firstly characterizing the microbiome compositions (prokaryotes and microbial eukaryotes) of four coral genera exhibited in healthy and bleached corals collected from the Andaman Sea of Thailand during the 2016 thermal bleaching event to better understand the healthy and bleached coral-associated microbiome and in the slight different Thailand marine geography. This study found distinct prokaryotic and microbial eukaryotic profiles between Acropora, Platygyra, Pocillopora, and Porites and suggested microbiome composition that might support coral bleaching susceptibility or tolerance based on previous works of literature. Potential coral-beneficial microbes from the previous reports as aforementioned were found to be reduced in the bleaching state corals (e.g., Pseudomonadales and Alteromonadales) but the previously reported coral-pathogenic microbes were found to increase (e.g., Malassezia and Aspergillus). Overall, while similar findings across bleach vs. healthy microbiota were found between our studied locations in this study (the Andaman Sea) and the Gulf of Thailand by Kusdianto et al. (2021), new findings that were highlighted in this study were Pseudomonadales (no reduction in bleached state corals Acropora and Platygyra in the Gulf of Thailand), Alteromonadales (no reduction in bleached state Porites in the Gulf of Thailand), and Vibrionales (higher in bleached state Porites in the Gulf of Thailand). However, the present analysis is a time point analysis of coral microbiome in 2016 in the studied location, further studies of upcoming bleaching events and diverse locations in the Andaman Sea of Thailand should be performed to allow complete understanding and identification of microbiomes representing the healthy vs. bleaching corals and to interpret the associated microbiomes to support against thermal bleaching stress. In addition, future studies should separate coral tissue and coral skeleton microbiomes analyses; coral tissue represents a direct interface and coral skeleton represents a reservoir for inner microbes and physico-chemical nutrients via decalcification (Ainsworth et al., 2015; Glasl et al., 2016; Ricci et al., 2019). Eventually, understanding these coral-associated microbiomes would help in restoring sustainable reefs (i.e., understanding which core microorganisms support bleaching resistance and coral reef restoration in the Andaman Sea of Thailand).
Data Availability Statement
The datasets presented in this study can be found in online repositories. The names of the repository/repositories and accession number(s) can be found below: https://www.ncbi.nlm.nih.gov/, PRJNA700134.
Author Contributions
SC collected the samples, helped conceive of the study, and helped revise the manuscript. HK and CK did molecular biology experiments and data analysis. CK helped draft the manuscript. DW commented. SJ and VV collected the samples. JO and VV conceived of the study. VV helped revise the manuscript. NS conceived of the study, coordinated the experiments and data analysis, and wrote and revised the manuscript. All authors read and approved the final manuscript.
Funding
This research was supported by the Ratchadaphisek Sompoch Endowment Fund 2021 Chulalongkorn University (764002-DT), the 90th Anniversary Fund of Chulalongkorn University, EU-Horizon 2020 Project TASCMAR (634674), NRCT-JSPS Core-to-Core Program, Mubadala Petroleum (Thailand), Microbiome Research Unit for Probiotics in Food and Cosmetics, Chulalongkorn University, and Thailand Science Research and Innovation Fund Chulalongkorn University (CU_FRB65_dis(3)_091_23_23).
Conflict of Interest
The authors declare that the research was conducted in the absence of any commercial or financial relationships that could be construed as a potential conflict of interest.
Publisher’s Note
All claims expressed in this article are solely those of the authors and do not necessarily represent those of their affiliated organizations, or those of the publisher, the editors and the reviewers. Any product that may be evaluated in this article, or claim that may be made by its manufacturer, is not guaranteed or endorsed by the publisher.
Acknowledgments
We acknowledged the Plant Genetic Conservation Project under the Royal Initiative of Her Royal Highness Princess Maha Chakri Sirindhorn, and the Naval Special Warfare Command, Royal Thai Navy for their assistance in the field.
Supplementary Material
The Supplementary Material for this article can be found online at: https://www.frontiersin.org/articles/10.3389/fmars.2022.763421/full#supplementary-material
References
Ainsworth, T. D., Krause, L., Bridge, T., Torda, G., Raina, J.-B., Zakrzewski, M., et al. (2015). The coral core microbiome identifies rare bacterial taxa as ubiquitous endosymbionts. ISME J. 9, 2261–2274. doi: 10.1038/ismej.2015.39
Alker, A. P., Smith, G. W., and Kim, K. (2001). Characterization of Aspergillus sydowii (Thom et Church), a fungal pathogen of Caribbean sea fan corals. Hydrobiologia 460, 105–111. doi: 10.1007/978-94-017-3284-0_9
Amaral-Zettler, L. D., McCliment, E. A., Ducklow, H. W., and Huse, S. M. (2009). A method for studying protistan diversity using massively parallel sequencing of V9 hypervariable regions of small-subunit ribosomal RNA genes. PLoS One 4:e6372. doi: 10.1371/journal.pone.0006372
Amend, A. (2014). From dandruff to deep-sea vents: malassezia-like fungi are ecologically hyper-diverse. PLoS Pathog. 10:e1004277. doi: 10.1371/journal.ppat.1004277
Anthony, K. R., Maynard, J. A., Diaz-Pulido, G., Mumby, P. J., Marshall, P. A., Cao, L., et al. (2011). Ocean acidification and warming will lower coral reef resilience. Glob. Change Biol. 17, 1798–1808. doi: 10.1016/j.marpolbul.2013.06.011
Badhai, J., Ghosh, T. S., and Das, S. K. (2016). Composition and functional characterization of microbiome associated with mucus of the coral Fungia echinata collected from Andaman sea. Front. Microbiol. 7:936. doi: 10.3389/fmicb.2016.00936
Baker, A. C., Glynn, P. W., and Riegl, B. (2008). Climate change and coral reef bleaching: an ecological assessment of long-term impacts, recovery trends and future outlook. Estuar. Coast. Shelf Sci. 80, 435–471. doi: 10.1016/j.ecss.2008.09.003
Banos, S., Lentendu, G., Kopf, A., Wubet, T., Glöckner, F. O., and Reich, M. (2018). A comprehensive fungi-specific 18S rRNA gene sequence primer toolkit suited for diverse research issues and sequencing platforms. BMC Microbiol. 18:190. doi: 10.1186/s12866-018-1331-4
Berkelmans, R., and van Oppen, M. J. (2006). The role of zooxanthellae in the thermal tolerance of corals: a ‘nugget of hope’for coral reefs in an era of climate change. Proc. Biol. Sci. 273, 2305–2312. doi: 10.1098/rspb.2006.3567
Berkelmans, R., Jones, A. M., and Schaffelke, B. (2012). Salinity thresholds of Acropora spp. on the great barrier reef. Coral Reefs 31, 1103–1110. doi: 10.1007/s00338-012-0930-z
Bernasconi, R., Stat, M., Koenders, A., Paparini, A., Bunce, M., and Huggett, M. J. (2019). Establishment of coral-bacteria symbioses reveal changes in the core bacterial community with host ontogeny. Front. Microbiol. 10:1529. doi: 10.3389/fmicb.2019.01529
Boilard, A., Dubé, C. E., Gruet, C., Mercière, A., Hernandez-Agreda, A., and Derome, N. (2020). Defining coral bleaching as a microbial dysbiosis within the coral holobiont. Microorganisms 8:1682. doi: 10.3390/microorganisms8111682
Boulotte, N. M., Dalton, S. J., Carroll, A. G., Harrison, P. L., Putnam, H. M., Peplow, L. M., et al. (2016). Exploring the Symbiodinium rare biosphere provides evidence for symbiont switching in reef-building corals. ISME J. 10, 2693–2701. doi: 10.1038/ismej.2016.54
Bourne, D. G., and Munn, C. B. (2005). Diversity of bacteria associated with the coral Pocillopora damicornis from the Great Barrier Reef. Environ. Microbiol. 7, 1162–1174. doi: 10.1111/j.1462-2920.2005.00793.x
Bourne, D. G., Boyett, H. V., Henderson, M. E., Muirhead, A., and Willis, B. L. (2008). Identification of a ciliate (Oligohymenophorea: Scuticociliatia) associated with brown band disease on corals of the Great Barrier Reef. Appl. Environ. Microbiol. 74, 883–888. doi: 10.1128/AEM.01124-07
Bourne, D., Iida, Y., Uthicke, S., and Smith-Keune, C. (2008). Changes in coral-associated microbial communities during a bleaching event. ISME J. 2, 350–363. doi: 10.1038/ismej.2007.112
Bradley, I. M., Pinto, A. J., and Guest, J. S. (2016). Design and evaluation of Illumina MiSeq-compatible, 18S rRNA gene-specific primers for improved characterization of mixed phototrophic communities. Appl. Environ. Microbiol. 82, 5878–5891. doi: 10.1128/AEM.01630-16
Brown, B., Dunne, R., and Chansang, H. (1996). Coral bleaching relative to elevated seawater temperature in the Andaman Sea (Indian Ocean) over the last 50 years. Coral Reefs 15, 151–152.
Bruno, J., Siddon, C., Witman, J., Colin, P., and Toscano, M. (2001). El Niño related coral bleaching in Palau, western Caroline Islands. Coral Reefs 20, 127–136.
Bulan, D. E., Wilantho, A., Krainara, P., Viyakarn, V., Chavanich, S., and Somboonna, N. (2018a). Spatial and seasonal variability of reef bacterial communities in the upper Gulf of Thailand. Front. Mar. Sci. 5:1529.
Bulan, D. E., Wilantho, A., Tongsima, S., Viyakarn, V., Chavanich, S., and Somboonna, N. (2018b). Microbial and small eukaryotes associated with reefs in the upper Gulf of Thailand. Front. Mar. Sci. 5:436. doi: 10.3389/fmars.2018.00436
Burke, L., Reytar, K., Spalding, K., and Perry, A. (2012). “Reefs at risk revisited in the Coral Triangle: World Resources Institute,” in Proceedings of the Nature Conservancy. World-Fish Center, International Coral Reef Action Network (Nairobi: UNEP World Conservation Monitoring Centre and Global Coral Reef Monitoring).
Caporaso, J. G., Lauber, C. L., Walters, W. A., Berg-Lyons, D., Huntley, J., Fierer, N., et al. (2012). Ultra-high-throughput microbial community analysis on the Illumina HiSeq and MiSeq platforms. ISME J. 6, 1621–1624. doi: 10.1038/ismej.2012.8
Carlos, C., Torres, T. T., and Ottoboni, L. M. (2013). Bacterial communities and species-specific associations with the mucus of Brazilian coral species. Sci. Rep. 3:1624. doi: 10.1038/srep01624
Ceh, J., Kilburn, M. R., Cliff, J. B., Raina, J. B., van Keulen, M., and Bourne, D. G. (2013). Nutrient cycling in early coral life stages: Pocillopora damicornis larvae provide their algal symbiont (Symbiodinium) with nitrogen acquired from bacterial associates. Ecol. Evol. 3, 2393–2400.
Chimetto, L. A., Brocchi, M., Thompson, C. C., Martins, R. C., Ramos, H. R., and Thompson, F. L. (2008). Vibrios dominate as culturable nitrogen-fixing bacteria of the Brazilian coral Mussismilia hispida. Syst. Appl. Microbiol. 31, 312–319. doi: 10.1016/j.syapm.2008.06.001
Criminger, J., Hazen, T., Sobecky, P., and Lovell, C. (2007). Nitrogen fixation by Vibrio parahaemolyticus and its implications for a new ecological niche. Appl. Environ. Microbiol. 73, 5959–5961. doi: 10.1128/AEM.00981-07
Csaszar, N. B., Ralph, P. J., Frankham, R., Berkelmans, R., and van Oppen, M. J. (2010). Estimating the potential for adaptation of corals to climate warming. PLoS One 5:e9751. doi: 10.1371/journal.pone.0009751
Cunning, R., Muller, E. G., Gates, R. D., and Nisbet, R. M. (2017). A dynamic bioenergetic model for coral-Symbiodinium symbioses and coral bleaching as an alternate stable state. J. Theor. Biol. 431, 49–62. doi: 10.1016/j.jtbi.2017.08.003
Damjanovic, K., Blackall, L. L., Menéndez, P., and van Oppen, M. J. (2020). Bacterial and algal symbiont dynamics in early recruits exposed to two adult coral species. Coral Reefs 39, 189–202. doi: 10.1007/s00338-019-01871-z
Doering, T., Wall, M., Putchim, L., Rattanawongwan, T., Schroeder, R., Hentschel, U., et al. (2021). Towards enhancing coral heat tolerance: a “microbiome transplantation” treatment using inoculations of homogenized coral tissues. Microbiome 9:102. doi: 10.1186/s40168-021-01053-6
Eakin, C. M., Morgan, J. A., Heron, S. F., Smith, T. B., Liu, G., Alvarez-Filip, L., et al. (2010). Caribbean corals in crisis: record thermal stress, bleaching, and mortality in 2005. PLoS One 5:e13969. doi: 10.1371/journal.pone.0013969
ElAhwany, A. M., Ghozlan, H. A., ElSharif, H. A., and Sabry, S. A. (2015). Phylogenetic diversity and antimicrobial activity of marine bacteria associated with the soft coral Sarcophyton glaucum. J. Basic Microbiol. 55, 2–10. doi: 10.1002/jobm.201300195
Falkowski, P. G., Dubinsky, Z., Muscatine, L., and Porter, J. W. (1984). Light and the bioenergetics of a symbiotic coral. Bioscience 34, 705–709.
Fisher, P., Malme, M., and Dove, S. (2012). The effect of temperature stress on coral–Symbiodinium associations containing distinct symbiont types. Coral Reefs 31, 473–485.
Gardner, S. G., Camp, E. F., Smith, D. J., Kahlke, T., Osman, E. O., Gendron, G., et al. (2019). Coral microbiome diversity reflects mass coral bleaching susceptibility during the 2016 El Niño heat wave. Ecol. Evol. 9, 938–956. doi: 10.1002/ece3.4662
Geiser, D. M., Taylor, J. W., Ritchie, K. B., and Smith, G. W. (1998). Cause of sea fan death in the West Indies. Nature 394, 137–138.
Glasl, B., Bongaerts, P., Elisabeth, N. H., Hoegh-Guldberg, O., Herndl, G. J., and Frade, P. R. (2017). Microbiome variation in corals with distinct depth distribution ranges across a shallow-mesophotic gradient (15-85 m). Coral Reefs 36, 447–452. doi: 10.1007/s00338-016-1517-x
Glasl, B., Herndl, G. J., and Frade, P. R. (2016). The microbiome of coral surface mucus has a key role in mediating holobiont health and survival upon disturbance. ISME J. 10, 2280–2292. doi: 10.1038/ismej.2016.9
Gong, W., and Marchetti, A. (2019). Estimation of 18S gene copy number in marine eukaryotic plankton using a next-generation sequencing approach. Front. Mar. Sci. 6:219. doi: 10.3389/fmars.2019.00219
Hadaidi, G., Röthig, T., Yum, L. K., Ziegler, M., Arif, C., Roder, C., et al. (2017). Stable mucus-associated bacterial communities in bleached and healthy corals of Porites lobata from the Arabian seas. Sci. Rep. 7:45362. doi: 10.1038/srep45362
Hadziavdic, K., Lekang, K., Lanzen, A., Jonassen, I., Thompson, E. M., and Troedsson, C. (2014). Characterization of the 18S rRNA gene for designing universal eukaryote specific primers. PLoS One 9:e87624. doi: 10.1371/journal.pone.0087624
Hernandez-Agreda, A., Leggat, W., Bongaerts, P., Herrera, C., and Ainsworth, T. D. (2018). Rethinking the coral microbiome: simplicity exists within a diverse microbial biosphere. mBio 9:e00812-18. doi: 10.1128/mBio.00812-18
Hoegh-Guldberg, O., and Smith, G. J. (1989). The effect of sudden changes in temperature, light and salinity on the population density and export of zooxanthellae from the reef corals Stylophora pistillata Esper and Seriatopora hystrix Dana. J Exp. Mar. Biol. Ecol. 129, 279–303.
Hoshino, T., Doi, H., Uramoto, G.-I., Wörmer, L., Adhikari, R. R., et al. (2020). Global diversity of microbial communities in marine sediment. PNAS 117, 27587–27597. doi: 10.1073/pnas.1919139117
Janouškovec, J., Gavelis, G. S., Burki, F., Dinh, D., Bachvaroff, T. R., Gornik, S. G., et al. (2017). Major transitions in Dinoflagellate evolution unveiled by phylotranscriptomics. PNAS 114, E171–E180. doi: 10.1073/pnas.1614842114
Kelman, D., Kashman, Y., Rosenberg, E., Kushmaro, A., and Loya, Y. (2006). Antimicrobial activity of Red Sea corals. Mar. Biol. 149, 357–363.
Kim, K., and Rypien, K. L. (2015). “Aspergillosis of caribbean sea fan corals, Gorgonia spp,” in Coral Diseases, eds C. Woodley, C. A. Downs, A. Bruckner, J. Porter, and S. B. Galloway (Hoboken, NJ: Wiley), doi: 10.1002/9781118828502.ch16
Kirkwood, M., Todd, J. D., Rypien, K. L., and Johnston, A. W. (2010). The opportunistic coral pathogen Aspergillus sydowii contains dddP and makes dimethyl sulfide from dimethylsulfoniopropionate. ISME J. 4, 147–150. doi: 10.1038/ismej.2009.102
Kuanui, P., Chavanich, S., Viyakarn, V., Omori, M., and Lin, C. (2015). Effects of temperature and salinity on survival rate of cultured corals and photosynthetic efficiency of zooxanthellae in coral tissues. Ocean Sci. J 50, 263–268.
Kusdianto, H., Kullapanich, C., Palasuk, M., Jandang, S., Pattaragulwanit, K., Quazzani, J., et al. (2021). Microbiomes of healthy and bleached corals during a 2016 thermal bleaching event in the upper Gulf of Thailand. Front. Mar. Sci. 8:643962. doi: 10.3389/fmars.2021.643962
Kushmaro, A., Rosenberg, E., Fine, M., Haim, Y. B., and Loya, Y. (1998). Effect of temperature on bleaching of the coral Oculina patagonica by Vibrio AK-1. Mar. Scol. Prog. Ser. 171, 131–137. doi: 10.3354/meps171131
Leggat, W., Seneca, F., Wasmund, K., Ukani, L., Yellowlees, D., and Ainsworth, T. D. (2011). Differential responses of the coral host and their algal symbiont to thermal stress. PLoS One 6:e26687. doi: 10.1371/journal.pone.0026687
Lema, K. A., Bourne, D. G., and Willis, B. L. (2014). Onset and establishment of diazotrophs and other bacterial associates in the early life history stages of the coral Acropora millepora. Mol. Ecol. 23, 4682–4695. doi: 10.1111/mec.12899
Lema, K. A., Willis, B. L., and Bourne, D. G. (2012). Corals form characteristic associations with symbiotic nitrogen-fixing bacteria. Appl. Environ. Microbiol. 78, 3136–3144. doi: 10.1128/AEM.07800-11
Lesser, M. P., Mazel, C. H., Gorbunov, M. Y., and Falkowski, P. G. (2004). Discovery of symbiotic nitrogen-fixing cyanobacteria in corals. Science 305, 997–1000. doi: 10.1126/science.1099128
McDevitt-Irwin, J. M., Baum, J. K., Garren, M., and Vega Thurber, R. L. (2017). Responses of coral-associated bacterial communities to local and global stressors. Front. Mar. Sci. 4:262. doi: 10.3389/fmars.2017.00262
McGinley, K. J., Leyden, J. J., Marples, R. R., Path, M., and Kligman, A. M. (1975). Quantitative microbiology of the scalp in non-dandruff, dandruff, and seborrheic dermatitis. J Invest. Dermatol. 64, 401–405. doi: 10.1111/1523-1747.ep12512335
Mieog, J. C., Olsen, J. L., Berkelmans, R., Bleuler-Martinez, S. A., Willis, B. L., and van Oppen, M. J. (2009). The roles and interactions of symbiont, host and environment in defining coral fitness. PLoS One 4:e6364. doi: 10.1371/journal.pone.0006364
Morris, L. A., Voolstra, C. R., Quigley, K. M., Bourne, D. G., and Bay, L. K. (2019). Nutrient availability and metabolism affect the stability of coral-Symbiodiniaceae symbioses. Trends Microbiol. 27, 678–689. doi: 10.1016/j.tim.2019.03.004
Morrow, K. M., Muller, E., and Lesser, M. P. (2018). “How does the coral microbiome cause, respond to, or modulate the bleaching process?,” in Coral Bleaching. Ecological Studies (Analysis and Synthesis), Vol. 233, eds M. van Oppen and J. Lough (Cham: Springer), 153–188. doi: 10.1007/978-3-319-75393-5_7
Oliver, T., and Palumbi, S. (2011). Many corals host thermally resistant symbionts in high-temperature habitat. Coral Reefs 30, 241–250.
Osman, E. O., Suggett, D. J., Voolstra, C. R., Pettay, D. T., Clark, D. R., Pogoreutz, C., et al. (2020). Coral microbiome composition along the northern Red Sea suggests high plasticity of bacterial and specificity of endosymbiotic dinoflagellate communities. Microbiome 8:8. doi: 10.1186/s40168-019-0776-5
Palliani, R. B., and Riding, J. B. (2000). Subdivision of the dinoflagellate cyst family Suessiaceae and discussion of its evolution. J Micropalaeontol. 19, 133–137. doi: 10.1144/jm.19.2.133
Panithanarak, T. (2015). Effects of the 2010 coral bleaching on phylogenetic clades and diversity of zooxanthellae (Symbiodinium spp) in soft corals of the genus Sinularia. Plankton Benthos Res. 10, 11–17.
Peters, E. C. (1984). A survey of cellular reactions to environmental stress and disease in Caribbean scleractinian corals. Helgol. Meeresunters. 37, 113–137.
Phongsuwan, N., and Chansang, H. (2012). Repeated coral bleaching in the Andaman Sea, Thailand, during the last two decades. Phuket Mar. Biol. Cent. Res. Bull. 71, 19–41. doi: 10.1016/j.dsr2.2013.02.015
Pogoreutz, C., Rädecker, N., Cárdenas, A., Gärdes, A., Wild, C., and Voolstra, C. R. (2018). Dominance of Endozoicomonas bacteria throughout coral bleaching and mortality suggests structural inflexibility of the Pocillopora verrucosa microbiome. Ecol. Evol. 8, 2240–2252. doi: 10.1002/ece3.3830
Pollock, F. J., Lamb, J. B., van de Water, J. A. J. M., Smith, H. A., Schaffelke, B., Willis, B. L., et al. (2019). Reduced diversity and stability of coral-associated bacterial communities and suppressed immune function precedes disease onset in corals. R. Soc. Open Sci. 6:190355. doi: 10.1098/rsos.190355
Pootakham, W., Mhuantong, W., Putchim, L., Yoocha, T., Sonthirod, C., Kongkachana, W., et al. (2018). Dynamics of coral-associated microbiomes during a thermal bleaching event. MicrobiologyOpen 7:e00604. doi: 10.1002/mbo3.604
Rädecker, N., Pogoreutz, C., Voolstra, C. R., Wiedenmann, J., and Wild, C. (2015). Nitrogen cycling in corals: the key to understanding holobiont functioning? Trends Microbiol. 23, 490–497. doi: 10.1016/j.tim.2015.03.008
Raina, J.-B., Tapiolas, D. M., Forêt, S., Lutz, A., Abrego, D., Ceh, J., et al. (2013). DMSP biosynthesis by an animal and its role in coral thermal stress response. Nature 502, 677–680. doi: 10.1038/nature12677
Raina, J.-B., Tapiolas, D., Motti, C. A., Foret, S., Seemann, T., Tebben, J., et al. (2016). Isolation of an antimicrobial compound produced by bacteria associated with reef-building corals. PeerJ 4:e2275. doi: 10.7717/peerj.2275
Reisch, C. R., Moran, M. A., and Whitman, W. B. (2011). Bacterial catabolism of dimethylsulfoniopropionate (DMSP). Front. Microbiol. 2:172. doi: 10.3389/fmicb.2011.00172
Reshef, L., Koren, O., Loya, Y., Zilber-Rosenberg, I., and Rosenberg, E. (2006). The coral probiotic hypothesis. Environ. Microbiol. 8, 2068–2073.
Ricci, F., Marcelino, V. R., Blackall, L. L., Kühl, M., Medina, M., and Verbruggen, H. (2019). Beneath the surface: community assembly and functions of the coral skeleton microbiome. Microbiome 7:159. doi: 10.1186/s40168-01900762-y
Ritchie, K. B. (2006). Regulation of microbial populations by coral surface mucus and mucus-associated bacteria. Mar. Ecol. Prog. Ser. 322, 1–14.
Roder, C., Arif, C., Bayer, T., Aranda, M., Daniels, C., Shibl, A., et al. (2014). Bacterial profiling of white plague disease in a comparative coral species framework. ISME J. 8, 31–39. doi: 10.1038/ismej.2013.127
Rohwer, F., Seguritan, V., Azam, F., and Knowlton, N. (2002). Diversity and distribution of coral-associated bacteria. Mar. Ecol. Prog. Ser. 243, 1–10. doi: 10.3354/meps243001
Rosales, S. M., Miller, M. W., Williams, D. E., Traylor-Knowles, N., Young, B., and Serrano, X. M. (2019). Microbiome differences in disease-resistant vs. susceptible Acropora corals subjected to disease challenge assays. Sci. Rep. 9:18279. doi: 10.1038/s41598-019-54855-y
Rosenberg, E., Koren, O., Reshef, L., Efrony, R., and Zilber-Rosenberg, I. (2007). The role of microorganisms in coral health, disease and evolution. Nat. Rev. Microbiol. 5, 355–362. doi: 10.1038/nrmicro1635
Salih, A., Hoegh-Guldberg, O., and Cox, G. (1997). “Bleaching responses of symbiotic dinoflagellates in corals: the effects of light and elevated temperature on their morphology and physiology,” in Proceedings of the Australian Coral Reef Society, Heron Island 50 year Commemorative Meeting (Heron Island, HI).
Schloss, P. D., Westcott, S. L., Ryabin, T., Hall, J. R., Hartmann, M., Hollister, E. B., et al. (2009). Introducing mothur: open-source, platform-independent, community-supported software for describing and comparing microbial communities. Appl. Environ. Mircobiol. 75, 7537–7541. doi: 10.1128/AEM.01541-09
Sebastian, C. R., Sink, K., Mcclanahan, T. R., and Cowan, D. A. (2009). Bleaching response of corals and their Symbiodinium communities in south Africa. Mar. Biol. 156, 2049–2062.
Smith, G. W., and Weil, E. (2004). “Aspergillosis of gorgonians,” in Coral Health and Disease, eds E. Rosenberg and Y. Loya (New York, NY: Springer).
Somboonna, N., Wilantho, A., Monanunsap, S., Chavanich, S., Tangphatsornruang, S., and Tongsima, S. (2017). Microbial communities in the reef water at Kham island, lower Gulf of Thailand. PeerJ 5:e3625. doi: 10.7717/peerj.3625
Stat, M., Morris, E., and Gates, R. D. (2008). Functional diversity in coral–dinoflagellate symbiosis. PNAS 105, 9256–9261. doi: 10.1073/pnas.0801328105
Steinert, G., Busch, K., Bayer, K., Kodami, S., Arbizu, P. M., Kelly, M., et al. (2020). Compositional and quantitative insights into bacterial and archaeal communities of South Pacific deep-sea sponges (Demospongiae and Hexactinellida). Front. Microbiol. 11:716. doi: 10.3389/fmicb.2020.00716
Stoeck, T., Bass, D., Nebel, M., Christen, R., Jones, M. D. M., Breiner, H.-W., et al. (2010). Multiple marker parallel tag environmental DNA sequencing reveals a highly complex eukaryotic community in marine anoxic water. Mol. Ecol. 19, 21–31. doi: 10.1111/j.1365-294X.2009.04480.x
Sun, F., Yang, H., Wang, G., and Shi, Q. (2020). Combination analysis of metatranscriptome and metagenome reveal the composition and functional response of coral symbionts to bleaching during an El Niño Event. Front. Microbiol. 11:448. doi: 10.3389/fmicb.2020.00448
Sunagawa, S., DeSantis, T. Z., Piceno, Y. M., Brodie, E. L., DeSalvo, M. K., Voolstra, C. R., et al. (2009). Bacterial diversity and white plague disease-associated community changes in the Caribbean coral Montastraea faveolata. ISME J. 3, 512–521. doi: 10.1038/ismej.2008.131
Sweet, M. J., and Bulling, M. T. (2017). On the importance of the microbiome and pathobiome in coral health and disease. Front. Mar. Sci. 4:9. doi: 10.3389/fmars.2017.00009
Torres, A. F., Valino, D. A. M., and Ravago-Gotanco, R. (2021). Zooxanthellae diversity and coral-symbiont associations in the Philippine Archipelago: specificity and adatability across thermal gradients. Front. Mar. Sci. 8:731023. doi: 10.3389/fmars.2021.731023
Tout, J., Siboni, N., Messer, L. F., Garren, M., Stocker, R., Webster, N. S., et al. (2015). Increased seawater temperature increases the abundance and alters the structure of natural Vibrio populations associated with the coral Pocillopora damicornis. Front. Microbiol. 6:432. doi: 10.3389/fmicb.2015.00432
Tsoy, O. V., Ravcheev, D. A., Èuklina, J., and Gelfand, M. S. (2016). Nitrogen fixation and molecular oxygen: comparative genomic reconstruction of transcription regulation in Alphaproteobacteria. Front. Microbiol. 7:1343. doi: 10.3389/fmicb.2016.01343
van Oppen, M. J. H., and Blackall, L. L. (2019). Coral microbiome dynamics, functions and design in a changing world. Nat. Rev. Microbiol. 17, 557–567. doi: 10.1038/s41579-019-0223-4
Velegraki, A., Cafarchia, C., Gaitanis, G., Iatta, R., and Boekhout, T. (2015). Malassezia infections in humans and animals: pathophysiology, detection, and treatment. PLoS Pathog. 11:e1004523. doi: 10.1371/journal.ppat.1004523
Vestheim, H., and Jarman, S. N. (2008). Blocking primers to enhance PCR amplification of rare sequences in mixed samples - a case study on prey DNA in Antarctic krill stomachs. Front. Zool. 5:12. doi: 10.1186/1742-9994-5-12
Wang, Y., Tian, R. M., Gao, Z. M., Bougouffa, S., and Qian, P.-Y. (2014). Optimal eukaryotic 18S and universal 16S/18S ribosomal RNA primers and their application in a study of symbiosis. PLoS One 9:e90053. doi: 10.1371/journal.pone.0090053
Ward, J. R., Kim, K., and Harvell, C. D. (2007). Tempereature affects coral disease resistance and pathogen growth. Mar. Ecol. Prog. Ser. 329, 115–121. doi: 10.3354/meps329115
Keywords: coral bleaching, coral reefs, microbiome, bacteria, fungi, next generation sequencing, Andaman Sea
Citation: Chavanich S, Kusdianto H, Kullapanich C, Jandang S, Wongsawaeng D, Ouazzani J, Viyakarn V and Somboonna N (2022) Microbiomes of Healthy and Bleached Corals During a 2016 Thermal Bleaching Event in the Andaman Sea of Thailand. Front. Mar. Sci. 9:763421. doi: 10.3389/fmars.2022.763421
Received: 23 August 2021; Accepted: 10 January 2022;
Published: 21 February 2022.
Edited by:
Anthony Lee Dellinger, Kepley BioSystems, Inc., United StatesReviewed by:
Christine Ferrier-Pagès, Centre Scientifique de Monaco, MonacoKefu Yu, Guangxi University, China
Joseph Selvin, Pondicherry University, India
Copyright © 2022 Chavanich, Kusdianto, Kullapanich, Jandang, Wongsawaeng, Ouazzani, Viyakarn and Somboonna. This is an open-access article distributed under the terms of the Creative Commons Attribution License (CC BY). The use, distribution or reproduction in other forums is permitted, provided the original author(s) and the copyright owner(s) are credited and that the original publication in this journal is cited, in accordance with accepted academic practice. No use, distribution or reproduction is permitted which does not comply with these terms.
*Correspondence: Voranop Viyakarn, Vm9yYW5vcC5WQGNodWxhLmFjLnRo; Naraporn Somboonna, TmFyYXBvcm4uU0BjaHVsYS5hYy50aA==