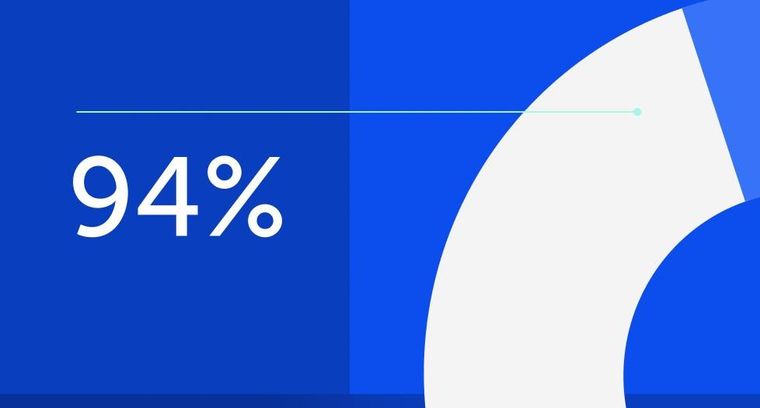
94% of researchers rate our articles as excellent or good
Learn more about the work of our research integrity team to safeguard the quality of each article we publish.
Find out more
REVIEW article
Front. Mar. Sci., 04 May 2022
Sec. Marine Pollution
Volume 9 - 2022 | https://doi.org/10.3389/fmars.2022.753391
This article is part of the Research TopicVulnerability and Resilience of Marine Ecosystems Affected by the Deepwater Horizon Oil SpillView all 6 articles
The scale of the Deepwater Horizon disaster was and is unprecedented: geographic extent, pollutant amount, countermeasure scope, and of most relevance to this Research Topic issue, range of ecotypes affected. These ecotypes include coastal/nearshore, continental shelf, deep benthic, and open-ocean domains, the last of which is the subject of this synthesis. The open-ocean ecotype comprises ~90% of the volume of the Gulf of Mexico. The exact percentage of this ecotype contaminated with toxins is unknown due to its three-dimensional nature and dynamics, but estimates suggest that the footprint encompassed most of its eastern half. Further, interactions between the water column and the deep benthos may be persistent, making this synthesis one of time (a decade) rather than event conclusion. Here we examine key elements of the open-ocean ecosystem, with emphasis on vulnerability and resilience. Of paramount importance relative to the Gulf nearshore and shelf ecotypes, pre-disaster baseline data were lacking for most of the fauna. In such cases, inferences were drawn from post-disaster assessments. Both phytoplankton and mesozooplankton vulnerabilities were quite high, but resilience appeared equally so. The phytoplankton situation was a bit more complex in that toxin-imposed reductions may have been offset by nutrient injection via high freshwater discharge in 2010. Intermediate trophic levels exhibited population-level depressions, ostensibly due to high vulnerability and low resilience. Apex predator impacts were variable. Certain large epipelagic fishes may have avoided the highest concentrations of hydrocarbons/dispersant, and thus larval abundances returned to pre-disaster levels of variability and abundance within a few years after a steep initial decline. Oceanic cetaceans, particularly shallow-diving stenellid dolphins, did not appear to avoid oiled waters and exhibited strong declines in the northern Gulf. Given that population declines of many open-ocean taxa appear to be ongoing a decade later, we conclude that this largest of Gulf ecosystem components, like its deep-benthic counterpart, is as fragile as it is voluminous. This is particularly concerning given the rapid, and likely irreversible, shift to deeper waters by the US and Mexican oil industries in concert with the higher likelihood of accidents with increasing platform depth.
The spatiotemporal scope of the Deepwater Horizon oil spill disaster (DWH) has been well characterized (Murawski et al., 2020 and references therein). By several key metrics it stands as the worst aquatic disaster to date; the disaster discharged 4.0-4.9 million barrels of oil, oiled 2,113 km of coastline, contaminated 110,000 km2 of benthic area, produced a deep-water plume ~400 km in length, and introduced a large amount of dispersant to the system [~2.1 million gallons] (Hsieh, 2010; Du and Kessler, 2012; McNutt et al., 2012; Payne and Driskell, 2015; U.S. District Court, 2015; Nixon et al., 2016; Romero et al., 2017; NASEM, 2020). In addition to these metrics, DWH was unique in the depth of ecosystem impact (~1500 m to the surface), the nature of mitigation measures (i.e., sub-sea dispersant injection; NASEM, 2020), and the magnitude of an unexpected oil-sedimentation event (MOSSFA: Marine Oil Snow Sedimentation and Flocculent Accumulation; Brooks et al., 2015; Romero et al., 2015; Daly et al., 2016; Diercks et al., 2021). While the surface manifestation of the oil spill and its subsequent coastal/nearshore impact received the most public attention and concern, the open-ocean pelagic domain received the most toxic pollutants due to the multiple fates of the released oil (Camilli et al., 2010; Ryerson et al., 2012; Wade et al., 2016; Romero et al., 2017). For example, some discharged oil ascended through the water column to the surface as buoyant droplets (~20%), some formed deep-sea plumes of dispersed and dissolved oil between ~1000-1300 m depth (~48%), and some sank from the surface to deep-sea plumes and the seafloor as contaminated particles (~4-9%) (Ryerson et al., 2012; Chanton et al., 2015; Romero et al., 2017). From a marine ecosystem perspective, the largest portion of the Gulf of Mexico (GoMex hereafter, to differentiate from the Gulf of Maine) affected by DWH, the deep (> 200 m) water column, was and still is its least understood portion - a hindrance to both the natural resource damage assessment process (NRDA) (Deepwater Horizon Natural Resource Damage Assessment Trustees, 2016) and the construction of this synthesis.
The strategy devised for a synthesis of ecological research relative to DWH (i.e., Gulf of Mexico Research Initiative [GoMRI] Core area 3; https://gulfresearchinitiative.org/gomri-synthesis/) was to subdivide the DWH-affected GoMex into four ‘ecotypes,’ each addressed in this Frontiers in Marine Science Research Topic on Vulnerability and Resilience of Marine Ecosystems Affected by the Deepwater Horizon Oil Spill: 1) coastal/nearshore habitat (Murawski et al., 2021); 2) continental shelf habitat (Patterson et al., in prep.); 3) the deep benthos (Schwing et al., 2020); and 4) the open ocean (this paper). In addressing the open-ocean GoMex, we must first consider ways in which it differs from the other ecotypes. The most obvious differentiating aspect is size. The pelagic domain - the water-column habitat in waters deeper than 200 m (i.e., seaward of the shelf-break) - contains ~97% of the GoMex’s total volume, with 90.4% accounted for by the “deep-pelagic” portion, i.e. the mesopelagic and bathypelagic zones (Figure 1). A second differentiating aspect is that the primary physical drivers of the GoMex pelagic ecosystem are the fluid features of water (e.g., temperature, density/stratification, light attenuation, pressure) and mesoscale oceanographic features (e.g., the Loop Current, its associated eddies, and frontal features; Biggs and Ressler, 2001; Muhling et al., 2013; Vecchione et al., 2015; Johnston et al., 2019; Boswell et al., 2020; Milligan and Sutton, 2020). In terms of research and synthesis, this structuring requires a sampling/analysis philosophy that is framed in a 4-D Lagrangian sense as much as, or more than, a geographical perspective. It is critical that both spatial and temporal aspects of variability be considered carefully when evaluating the effects of a point-source disturbance on a pelagic ecosystem, even a prolonged event such as DWH. A third primary difference between the open-ocean ecotype and the other three ecotypes is the degree to which the biota partition themselves vertically (i.e., by water column depth). As a rule, in the pelagic ocean the vertical scale of faunal distributions is finer by three to five orders of magnitude than the horizontal scale (i.e., 10’s to 100’s of meters vs. 10’s to 1000’s of kilometers, respectively; Angel, 1993; Sutton, 2013). This is not to say that the vertical strata are not highly connected, as we will see in the following discussions of vertical migration, but that low-latitude pelagic ecosystems such as the GoMex appear to be highly structured vertically in time and space (Hopkins et al., 1996).
Figure 1 High-resolution hypsography of the Gulf of Mexico, with delineation of total volume by depth zones. Figure created from ETOPO1 data (https://ngdc.noaa.gov/mgg/global//global.html) at 1 arc minute resolution.
Like pelagic ecosystems globally (Angel, 1993; Angel, 1997; Herring, 2002; Robison, 2004; Priede, 2017), the open-ocean GoMex is subdivided into three ecological depth realms based on the penetration of sunlight; the epipelagic, mesopelagic, and bathypelagic (Figure 2), each with a characteristic daytime fauna (reviewed in Sutton, 2013). The epipelagic zone (0-200 m) contains all phytoplankton production and most of the zooplankton production, including larval and juvenile fishes and invertebrates. Most of the larger, highly mobile oceanic predators are centered in this layer, either by the need for air (cetaceans, seabirds, sea turtles) or by the reliance on vision to locate patchy food resources. The mesopelagic zone (200 – 1000 m depth) of the GoMex contains a highly diverse and specialized fauna during daytime, with much of this fauna migrating into the epipelagic zone at night to feed on plankton or on those organisms feeding on plankton (Hopkins et al., 1996; Sutton and Hopkins, 1996a; D’Elia et al., 2016; Boswell et al., 2020; Frank et al., 2020; Judkins and Vecchione, 2020). Research since DWH has identified the GoMex as one of the most diverse mesopelagic ecosystems in the World Ocean (Sutton et al., 2017a; Sutton et al., 2020). The bathypelagic zone (below 1000 m) supports a wide variety of fauna adapted for life in total darkness (excepting the light from bioluminescence, which is contributed by many taxa). This fauna in the GoMex was almost completely unknown prior to DWH (Burghart et al., 2007). Summarizing the ecotype, the open-ocean GoMex contains a rich panoply of taxonomic and behavioral diversity, with the biota highly connected vertically through active and passive flux and horizontally through coupled behavior and current-mediated translocation (Milligan and Sutton, 2020; Timm et al., 2020a). This pelagic connectivity links the four subject ecotypes (most deep-benthic and demersal taxa have pelagic larvae, and many coastal and shelf taxa use the open ocean as nursery habitat) and ocean basins.
Figure 2 Schematic of the open-ocean ecosystem of the northern Gulf of Mexico. Solid arrows associated with key taxa represent active flux (vertical migrations); dashed arrows indicate passive flux (sinking). Nekton = fishes, cephalopods, and large pelagic crustaceans. Note: seabirds not treated in synthesis due to lack of time-series data but included here to represent aerial-to-deep-pelagic connectivity.
In this paper, we present synopses of research on key ecological taxa of the open-ocean GoMex, with summaries of patterns of abundance, taxonomic composition, and behavior (if known) relative to the timing and extent of DWH and ensuing offshore mitigation measures. We evaluate the vulnerability of these taxa to DWH effects, as well as their realized or hypothetical resilience and recovery potential.
The synthesis approach adopted herein generally follows that of Murawski et al. (2021), and Schwing et al. (2020), with modifications as necessary in cases of data deficiency. As with those papers, several “key taxa” (Figure 2; Table 1) were identified and investigated in detail. These taxa were chosen based on their ecological and/or economic importance, vertical distribution representation, and the availability of information with which to assess or infer DWH impact. The seven key taxa discussed in this synthesis include: 1) oceanic phytoplankton, 2) mesozooplankton, 3) early life history stages of large epipelagic fishes (billfishes, dolphinfishes, tunas, and swordfish), 4) deep-pelagic fishes, 5) deep-pelagic macrocrustacea (euphausiids, decapod shrimps, and lophogastrids), 6) deep-pelagic cephalopods, and 7) oceanic marine mammals. Core papers summarizing methods of data collection and analysis for key taxa include but are not limited to Quigg et al. (2021) [phytoplankton], Daly et al. (2021) [mesozooplankton], Meinert et al. (2020) [ichthyoplankton], Cook et al. (2020) [micronekton], Boswell et al. (2020) [acoustic sensing of deep-scattering layers], Frasier et al. (2019) [oceanic cetaceans], and Johnston et al. (2019) [pelagic habitat characterization].
Table 1 Gulf of Mexico open-ocean biota selected as “key taxa” for vulnerability/resilience (V/R) analyses relative to the Deepwater Horizon disaster (DWH).
In keeping with the Vulnerability-Resilience (V-R) approach used in the companion syntheses in this Research Topic (see Schwing et al., 2020; Murawski et al., 2021), we evaluated the vulnerability of key taxa to DWH based on 13 attributes (Table 2). These attributes, related to distributional overlap (time and space), ability to detect and/or avoid toxicants, exposure, sensitivity to toxins and/or response measures (if known), and effects on trophic linkages, were selected by a 30-member panel of oceanic ecology experts during two GoMRI synthesis workshops in 2019 (attendees included authorship and additional subject matter experts [SMEs] listed in Acknowledgments). Vulnerability was qualitatively scored by SMEs for each taxon on a scale of low, medium, and high for each attribute. Resilience potential was likewise scored by the SME panel for 11 attributes (Table 2) relating to the proportion of population exposure, generation time, rate of production, reproductive ecology, population connectivity with other regions, known co-varying stressors, and capacity for restoration. The overall vulnerability and resilience of each taxon was then assessed, taking into account the distribution of high, medium, and low attribute scores, uncertainty regarding specific attributes (i.e., data gaps), and the relevance of specific attributes to specific taxa.
Table 2 Attributes used for evaluating the vulnerability (V) and resilience (R) of the Gulf of Mexico open-ocean fauna to the effects of the Deepwater Horizon disaster.
Abundance declines in the open-ocean GoMex biota after DWH ranged from imperceptible (mesozooplankton) to ephemeral (phytoplankton) to extensive (deep-pelagic fishes, macrocrustaceans). Of those taxa that demonstrated declines, none could be ascribed unequivocally to DWH due to (1) lack of pre-spill data, (2) confounding effects of co-stressors and/or spill countermeasures (e.g., opening Bonnet Carré spillway), and/or (3) massive data gaps regarding organismal biology and ecology. This is not to say that DWH was not the primary or contributing cause of these declines. Encapsulation of what has been learned to date is important as a benchmark for future resource management in the GoMex. We conducted V-R analyses to provide a means of comparing the relative risks of oil spills to key taxa, populations, and ecological assemblages. This information can then guide near-field mitigation efforts and predict far-field consequences. In the open-ocean case, the execution of the V-R analysis also highlighted critical data gaps, particularly among the deeper-living fauna.
Results (Figure 3; Table 2) are briefly summarized here, followed by more detailed treatment by taxon. From the lower right corner of the V-R matrix (Figure 3), a counter-clockwise progression relating to body size and generation time was apparent. The drivers of oceanic phytoplankton production over time were difficult to disarticulate because of the interplay of physical forcing, riverine nutrient input, and DWH effects. Some phytoplankton taxa (e.g., chain diatoms) appear to have been stimulated after DWH due to several factors, while other taxa may have been depressed (inferred from lower satellite-sensed surface chlorophyll values). Overall, vulnerability was classified as high, but resilience also high due to short generation times, influx from surrounding waters, and potential enhancement by countermeasure activity. Mesozooplankton vulnerability was classified as high due to overlapping spatial distributions, limited avoidance capacity, consumption of oil-droplet-sized particles, and the laboratory-demonstrated lethal/sublethal effects of oil/dispersant on the fauna. Likewise, mesozooplankton resilience was also considered high, with high oceanic connectivity and fecundity, ergo rapid replenishment, considered most responsible for the lack of population declines after DWH. Vulnerability of the early life history (ELH) stages of large epipelagic fishes was classified as high but taxon-specific, with shelf-associated species (e.g., blackfin tuna, Thunnus atlanticus) being more vulnerable than offshore-spawning species (e.g., blue marlin, Makaira nigricans) due to greater overlap of high-quality ELH stages habitat of the former with the DWH footprint. Where ELH stages and oil/dispersant co-occurred, all taxa were considered highly vulnerable due to toxicity effects. Low inherent resilience of ELH stages to direct mortality or sublethal effects may have been offset by higher sensing and avoidance capacity of adult spawning stock, resulting in moderate net resilience of the taxa in total. Deep-pelagic fishes were classified as highly vulnerable to DWH given the prolonged exposure to oil/dispersant throughout the water column, the known deleterious effects of DWH-derived toxins on fishes (especially ELH stages), the likely consumption of contaminated food (zooplankton) by the assemblage, the tight linkages of predators and prey in the system, and the low resilience attributes of the assemblage. Pelagic macrocrustaceans and cephalopods were likewise classified as highly vulnerable due to similar reasons, with cephalopod vulnerability heightened by reduced depuration capacity. Classification of resilience of macrocrustaceans was problematic due to the large variations in lifespans, brooding behavior (spawners vs. brooders, large numbers of tiny eggs vs. small numbers of large eggs), and vertical migration behavior (non-migrators vs. weak migrators vs. strong migrators) within each taxon. However, as no macrocrustacean family has shown evidence of recovering to 2011 levels as of this writing, we conclude that resilience of the assemblage as a whole is relatively low. Cephalopods were considered moderately resilient due to shorter life spans, hence potentially faster replenishment. Oceanic cetacean vulnerability was classified as moderate, with multiple contamination vectors (cutaneous contact, respiration of volatile fraction, consumption of tainted prey) potentially offset by higher sensing and avoidance capacity and lower site fidelity. Cetacean resilience to DWH was difficult to assess given the number of co-stressors and the uncertainties regarding abundance trajectories but was tentatively classified as low given the high number of ‘low’ scores with respect to resilience attributes (e.g., long generations times, low fecundity).
Figure 3 Summary Vulnerability-Resilience matrix, with cumulative attribute designation of high, moderate, and low for key open-ocean taxa of the northern Gulf of Mexico. D-P, deep-pelagic (residing in water column below 200 m depth during daytime).
Phytoplankton form the base of the food web in ocean ecosystems and their productivity constrain the potential for productivity at higher trophic levels (Marshak and Link, 2021). Under the influence of remote and local forcing, phytoplankton in surface waters of the GoMex are dynamic in both assemblage composition and abundance. Remote forcing mechanisms include the Atlantic Multidecadal Oscillation (AMO) and El Nino Southern Oscillation (ENSO), while local forcing mechanisms include upwelling, river and non-point source nutrient inputs, vertical mixing, the Loop Current, and ocean eddies (Muller-Karger et al., 2015). Phytoplankton in the GoMex include a wide range of taxa, from diatoms and dinoflagellates to cyanobacteria (Dortch, 1997; Paul et al., 2013). Because all phytoplankton contain the photosynthetic pigment chlorophyll a (Chl a), and because it is more difficult to measure the abundance of individual phytoplankton taxa than Chl a, the latter is used to estimate phytoplankton biomass and distributions in surface waters.
Satellite-derived Chl a concentrations (Chl a in mg m-3) in the open-ocean GoMex are driven mainly by wind-induced mixing and ocean eddies, with winter highs of 0.2 – 0.4 mg m-3 and summer lows of ~ 0.1 mg m-3, corresponding to a winter mixed layer depth (MLD) of ~ 100 m and summer MLD of 20 – 30 m (Muller-Karger et al., 2015). One exception is the northern and eastern portions of the GoMex (north of 27.8°N and east of 89.9°W), where Mississippi River plumes often cause a secondary Chl a peak during summer. Offshore transport of river plumes is well known (e.g., Hu et al., 2003; Hu et al., 2005). The influence of the river plume on shelf waters (< 1000 m isobath) is stronger in this region, as shown in the satellite images of Figure 4. Indeed, the northern intrusion of the Loop Current, together with its eddies, can move nutrient-rich coastal waters offshore, enriching offshore waters with nutrients and higher Chl a (Androulidakis et al., 2019). This is especially true for the northeastern GoMex, where DWH occurred.
Figure 4 Distributions of surface chlorophyll a fronts (using a color index as the proxy) in July – September 2016 showing offshore advection and entrainment of nutrient-rich coastal and shelf waters by anti-cyclonic eddies, which influences both the eastern and western Gulf of Mexico. Figure adapted from Zhang and Hu (2021).
Vulnerability of phytoplankton to the DWH event, at least initially, was deemed high; six of nine scored vulnerability attributes were scored as such, namely due to high spatial overlap, no ability to detect and avoid pollutants, and susceptibility to toxicants and countermeasures. Resilience was more complicated due to confounding factors, detailed as follows, but was ultimately deemed high as well (seven of nine attributes scored as such). An initial assessment of the potential influence of DWH on surface water phytoplankton suggested that Chl a in the impacted area was significantly higher immediately after the oil spill and during August 2010 than all previous August months since 2002. To our knowledge, no samples of phytoplankton were collected off-shelf during the oil spill in spring 2010 due to the difficulty of sampling through surface oil. A Shadowed Image Profiling and Evaluation Recorder (SIPPER) camera system was used to collect images of large phytoplankton, along with data from environmental sensors (e. g., CTD, chlorophyll fluorescence) on a cruise during May and June 2010 (Daly et al., 2021). Large chain diatoms were relatively abundant with the highest concentrations in the upper 50 m. Physical samples collected during summer 2010 indicated that chain diatoms dominated the phytoplankton community, and included Thalassionema nitzschioides, Pseudo-nitzschia sp., Dactyliosolen fragilissimus, Leptocylindrus danicus, Leptocylindrus minimus, Dactyliosolen fragilissimus, Chaetoceros spp., Eucampia spp., and Cyclotella sp. (Quigg et al., 2021). Trichodesmium also was relatively abundant during late spring and summer. Subsequent analysis by O’Connor et al. (2016) confirmed the Hu et al. (2011) finding of Chl a anomalies, although O’Connor et al. (2016) attributed some of the anomalies to the river plume and to the opening of the Bonnet Carré spillway, which increased nutrient concentration and fluxes to the area. It is not possible to isolate the cause of increased Chl a. Observations of chlorophyll concentrations in this off-shelf region in follow-on years differ. Integrated chlorophyll fluorescence obtained from in situ samples and fluorescence sensors off-shelf in spring 2010 were not significantly different (p > 0.001) from that in spring 2011, 2012, and 2014, but were significantly lower (p < 0.001) than integrated chlorophyll fluorescence in spring 2013 when Mississippi River discharge was relatively high (Quigg et al., 2021). In contrast, Li et al. (2019) reported that time-series satellite data showed depressed solar-stimulated surface phytoplankton fluorescence in 2011 – 2014 in the vicinity of DWH, possibly due to inhibition of phytoplankton growth, altered nutrient inventories, or some combination of factors. After 2015, surface Chl a in the area, as revealed by satellites, returned to normal levels with natural variations similar to those before DWH (Li et al., 2019). Furthermore, Parsons et al. (2015) observed lower Chl a in 2010 on the Louisiana shelf than the previous 20 years. DWH may have stimulated some phytoplankton while inhibiting others (Ozhan and Bargu, 2014; Ozhan et al., 2014). In particular, large chain forming diatoms bloomed in the summer of 2010 (Quigg et al., 2021) and bloom-associated materials, including diatom remnants and oil components, were captured in deep sediment traps in the fall of 2010 (Yan et al., 2016).
The mesozooplankton community consists of a diverse array of marine organisms, ranging from 0.2 to 20 mm in size. In addition to holoplankton, this category includes larvae of diverse pelagic and benthic animals. Zooplankton are a key component of marine food webs as the primary grazers on phytoplankton and other microplankton and as prey for higher trophic levels, including commercially important fishes (Banse, 1995; Cushing, 1995). Zooplankton also play other important roles in biogeochemical cycles in marine systems, contributing fecal pellets, feeding structures (e.g., larvacean houses), exuviae, and dead organisms to sedimenting marine snow (Longhurst and Harrison, 1989).
The mesozooplankton species composition and spatial distribution in the GoMex was reviewed in Iverson and Hopkins (1981) and Biggs and Ressler (2001). In general, zooplankton abundance and biomass are relatively low off-shelf, where > 50% of the zooplankton biomass occurs in the upper 200 m, typical of oligotrophic waters (Hopkins, 1982). The highest zooplankton abundances in the northeastern quadrant of the GoMex occur in the productive regions of high outflow of the Mississippi, Atchafalaya, Mobile, and Apalachicola River systems and downstream river plumes and in the vicinity of the De Soto Canyon (Okolodkov, 2003). This region frequently also has cyclonic or anticyclonic eddies that persist for weeks to several months (Jochens and DiMarco, 2008; Schiller et al., 2011). These eddies influence biological productivity via cross-margin flow, entrainment of low-salinity shelf water, and uplift of isopycnals and nutrient fields by cyclonic eddies as well as uplift at the periphery of anticyclonic eddies (Belabbassi et al., 2005). River plumes, fronts, and eddies all act to retain and aggregate phytoplankton, zooplankton, and fish larvae, resulting in trophic hot spots (Grimes and Finucane, 1991).
While there was some information on zooplankton in this region prior to DWH (e.g., Turner, 1987; Ortner et al., 1989; Dagg and Whitledge, 1991; Dagg, 1995, in addition to the papers mentioned above), there were no baseline data on open-ocean zooplankton abundance and distribution based on systematic surveys over multiple years in the northeastern GoMex. The SEAMAP Program (NOAA/NMFS) has collected zooplankton along stations in the GoMex for several decades and NOAA/NRDA funded zooplankton surveys during and after DWH, but most of these data are not publicly available. Results from a spring 2010 NRDA survey, GoMRI-funded zooplankton surveys between 2010 and 2014, and selected SEAMAP samples collected between 2005 and 2009 are summarized below. The dominant open-ocean mesozooplankton taxa were copepods, larvaceans, and chaetognaths, with episodically high abundances of ostracods, and gelatinous zooplankton, such as hydromedusea, siphonophores, and doliolids (Daly et al., 2021). Euphausiids, pelagic shrimps, pteropods, heteropods, and echinoderm larvae were relatively common. The dominant off-shelf epipelagic copepods were variable seasonally and interannually, and included Centropages velificatus, Oithona spp., Corycaeus spp., Oncaea spp., Clausocalanus furcatus, Subeucalanus pileatus, Temora turbinata, Acartia danae, Ctenocalanus furcatus, Nanocalanus minor, and Paracalanus spp. Mesozooplankton abundances were spatially variable, with the highest densities typically in the upper 50 m, particularly during summer. Multivariate analyses indicated that river discharge, wind speed and direction, seawater temperature, and Chl a concentrations were the environmental factors that had the strongest impact on mesozooplankton community dynamics. The two years with the highest river discharge rates (2010 and 2013) also had the highest zooplankton abundances.
Vulnerability of mesozooplankton to DWH effects was deemed high, particularly regarding spatial overlap of exposure and sensitivity to toxins. Total polycyclic aromatic hydrocarbon (PAH) concentrations greater than 0.5 μg L-1 (ppb) are considered toxic to marine zooplankton; concentrations of PAH reached toxic levels in the upper 20 m of the water column for extended periods across much of the region affected by DWH (Deepwater Horizon Natural Resource Damage Assessment Trustees, 2016). The Trustees Report (2016) also estimated that 37 to 68 trillion zooplankton were killed over the entire oil spill region, including 4–6% of the zooplankton community in open-ocean waters. Since mesozooplankton are typically most abundant in the upper 50 m off shelf (Hopkins, 1982; Daly et al., 2021), there was persistent and significant overlap between zooplankton and toxic concentrations of spilled oil. Based on field data, about 40% of the zooplankton community in the upper 100 m of the water column may have been affected by the highest concentrations of oil during May and June 2010 (Daly et al., 2021). Laboratory experiments demonstrated that copepod and gelatinous zooplankton species exposed to Louisiana Sweet crude oil, a surrogate for Macondo (MC252) crude oil released during DWH, resulted in mortality or sublethal effects, such as reduced rates of feeding, growth, egg production, egg hatching, development time, and fecal pellet production, as well as impaired swimming behavior (Almeda et al., 2013a; Almeda et al., 2013b; Almeda et al., 2014b; Almeda et al., 2014c; Cohen et al., 2014; Lee et al., 2017) by zooplankton ingesting physically and chemically dispersed oil or through exposure to dissolved oil compounds. (Almeda et al. 2013b; Almeda et al. 2013a) found dispersed oil at the 1∶20 dispersant to oil ratio commonly used in the treatment of oil spills was 2-3 times more toxic than oil alone to stages of scyphozoans, Pelagia noctiluca and Aurelia aurita, the ctenophore, Mnemiopsis leidyi, and the copepod, Acartia tonsa. Almeda et al. (2014a) also determined that dispersant-treated crude oil was 1.6 times more toxic when ingested by adults and nauplii of the copepods, Acartia tonsa, Temora turbinata, and Parvocalanus crassirostris, than crude oil alone. The doliolid, Dolioletta gegenbauri, was shown after ingesting crude oil to release oil in fecal pellets (Lee et al., 2012), which then could be ingested by particle feeding zooplankton deeper in the water column. Furthermore, the dynamic nature of the northern GoMex, including interactions of the Loop Current, Mississippi River plume, fronts, and eddies, acts to retain and aggregate surface oil, particles, and zooplankton (Daly et al., 2020), increasing the opportunities for exposure to oil. Similarly, closer to the surface, toxicity of DWH oil to zooplankton increased in the presence of sunlight (Almeda et al., 2013b; Barron, 2017), further exacerbating the vulnerability of shallow-water zooplankton to oil spill impacts. Deeper dwelling zooplankton could have been exposed to DWH oil through several different pathways: interaction with the rising column of oil and gas from the wellhead before it was capped, predation on oil-affected prey in surface waters during vertical migration (Quintana-Rizzo et al., 2015), and consumption of oiled marine snow (Daly et al., 2016) and carcasses (Tolan, 2020) from surface waters.
As with phytoplankton, post-DWH surveys indicated a high degree of resilience of the open ocean mesozooplankton to DWH effects. Daly et al. (2021) showed that the abundance of zooplankton during the oil spill (spring 2010) was not significantly different from following springs (2011 and 2012) and summer 2010 abundances were the highest observed for the 2005 - 2014 period, likely due to high river discharge during the oil spill. Although experimental results indicated that all tested zooplankton experienced lethal or sublethal effects, net community impacts could not be detected in the months and years following DWH. Daly et al. (2021) concluded that zooplankton community resilience in the vicinity of the oil spill was primarily due to ecosystem connectivity (transport of zooplankton into the oil spill area), refuge in deeper waters, high fecundity, and relatively short generation times.
Although the mesozooplankton community appeared to be resilient to the oil spill, it should be noted that zooplankton may have contributed to other ecosystem impacts of oil. Some zooplankton, such as dinoflagellates (Noctiluca), copepods, and doliolids, ingest oil droplets and egest them in fecal pellets, which may be re-ingested by other particle feeders (Lee et al., 2012; Almeda et al., 2014a; Almeda et al., 2016). Fecal pellets and other zooplankton products may have contributed to the significant sedimentation of marine oil snow to the seafloor (Daly et al., 2016; Yan et al., 2016) and corresponding impacts on the benthos (White et al., 2012; Schwing et al., 2015). Zooplankton also may have contributed to bioaccumulation in upper-trophic-level animals through ingestion of oil or ingestion of suspended particulate matter. Carbon isotopic depletion in suspended material indicated that oil carbon was incorporated into the lower-trophic food web through biodegradation by bacteria (Graham et al., 2010; Chanton et al., 2012; Fernández-Carrera et al., 2016).
Large, highly migratory species of fishes that occupy the epipelagic (upper 200 m) zone of the water column play an important ecological role in the global open ocean and are important economically in the GoMex. Shifts in distribution, abundance, and assemblage diversity are known to be important determinants of ecosystem productivity and stability (Lindegren et al., 2016). Investigations centered on their ELH stages (larvae and early juveniles) are less common than those on the subadults/adults, but the former are critical for understanding population dynamics, spawning stock biomass (reproductive capacity), essential spawning and nursery areas (Rooker et al., 2012; Richardson et al., 2016), and environmental conditions that support early-life survival and recruitment (Rooker et al., 2012; Pritt et al., 2014; Houde, 2016; Richardson et al., 2016).
The larval epipelagic fish community of the GoMex is taxonomically diverse and comprises many species found throughout the larger Atlantic Ocean basin (Lyczkowski-Shultz et al., 2013; Randall et al., 2015; Cornic et al., 2018). In outer shelf and slope waters of the northern GoMex, Meinert et al. (2020) reported over 99 families of fish larvae in summer ichthyoplankton surveys, indicating a remarkably diverse assemblage. Numerically dominant taxa in the surface layer of the water column (upper 1 m) include several taxa that are also common in the epipelagic zone as adults. Apart from the numerically dominant family, Carangidae (jacks), which represented nearly one-third of the catch, other core components of the surface ichthyoplankton assemblage included families Clupeidae (herrings), Exocoetidae (flyingfishes), Hemiramphidae (halfbeaks), Istiophoridae (billfishes), and Scombridae (mackerels and tunas) (Rooker et al., 2013; Randall et al., 2015; Cornic and Rooker, 2018; Meinert et al., 2020; Pruzinsky et al., 2020; Cornic and Rooker, 2021). All of these taxa also occurred below the surface layer of the epipelagic zone. However, several deep-pelagic taxa not detected near the surface were common deeper in the epipelagic zone (mixed layer) down to 200 m; these included families Myctophidae (lanternfishes), Gonostomatidae (bristlemouths), and Sternoptychidae (marine hatchetfishes). In fact, myctophids were the most abundant family of fish larvae collected in oblique bongo tows within the mixed layer during surveys in the both the northern (Meinert et al., 2020) and southern (Daudén-Bengoa et al., 2020) GoMex. Similar results were reported by Wang et al. (2021) in their analysis of depth-discrete ichthyoplankton data collected during six NRDA plankton cruises in 2010 and 2011, which included tows down to depths of 1500 m. Four families (Myctophidae, Gonostomatidae, Sternoptychidae, Phosichthyidae) comprised the majority of the catch in both the epipelagic (63%) and combined mesopelagic and bathypelagic (97%) regions, and assemblage structure across all regions was largely determined by depth and seasonality. The high abundance of mesopelagic fish larvae within the epipelagic zone clearly signifies well-developed ecological connectivity between the epi- and deep-pelagic zones in the open GoMex, and this finding also highlights the value of the epipelagic zone as an essential nursery habitat of deep-pelagic fishes prior to ontogenic shifts to deeper habitats.
Time-series data from ichthyoplankton surveys of the epipelagic zone are limited in scope and nearly all investigations have focused on specific taxonomic groups rather than the entire epipelagic fish assemblage. Recent efforts in the northern GoMex have focused on species or taxa that are important to recreational or commercial fisheries in this region, including billfishes, dolphinfishes, tunas, and swordfish (Muhling et al., 2012; Kitchens and Rooker, 2014; Cornic et al., 2018; Meinert et al., 2020; Pruzinsky et al., 2020). Rooker et al. (2013) characterized the relative abundance of blackfin tuna (Thunnus atlanticus), blue marlin (Makaira nigricans), common dolphinfish (Coryphaena hippurus), and sailfish (Istiophorus platypterus) larvae pre- (2007-2009) and post- (2010) DWH to assess potential impacts of this event on early life survival and recruitment potential. The mean abundance for each species was lowest post-DWH in 2010, though the observed declines were often statistically similar to at least one year in the baseline surveys (i.e., pre-DWH years) for certain taxa and often within the range of expected natural variation for occurrence and/or mean density of these taxa (Rooker et al., 2013). More recent sampling through GoMRI’s DEEPEND Consortium has indicated a return to pre-spill abundances for nearly all of species assessed, although abundances of certain taxa (e.g., billfishes) remained low relative to mean abundances pre-DWH for several years after the event (Meinert et al., 2020).
The overall vulnerability of epipelagic fish ELH stages was deemed high due to acute sensitivity to DWH toxins and moderate spatial/temporal overlap of their habitats with areas impacted by DWH. Reductions in the abundance of pelagic fish larvae post-DWH are in accord with the premise that PAHs and other toxic components resulting from DWH negatively impacted the early life survival of these taxa (Berenshtein et al., 2020). Physical processes that move oil and dispersants also transport epipelagic fish larvae (Jolliff et al., 2014), increasing their exposure to these toxins. Larvae of epipelagic fishes exposed to toxic components of oil experienced a wide range of deleterious effects on an assortment of physiological parameters (Grosell and Pasparakis, 2021), including growth (Mager et al., 2017), sensory function (Xu et al., 2016; Xu et al., 2017), cardiac development and function (Incardona et al., 2014; Perrichon et al., 2018), metabolic demand (Pasparakis et al., 2016), and buoyancy control/swimming performance (Mager et al., 2014; Pasparakis et al., 2016). Moreover, sensitivity to oil toxicity is an order of magnitude higher for early life stages (larvae) compared to adults of these pelagic species (Pasparakis et al., 2019). Regarding spatiotemporal exposure, high-quality habitat for several species common in the surficial epipelagic zone during the first few weeks of life overlapped with the areal coverage of surface oil from DWH. Rooker et al. (2013) observed that high-quality spawning and/or nursery habitat of billfish, dolphinfish, and tuna larvae were impacted by DWH, with surface oil occurring across approximately 10-20% of available high-quality habitat in the northern GoMex used by these taxa during early life. In general, the degree of overlap between surface oil and high-quality habitat was markedly higher for species commonly inhabiting waters on the continental shelf (e.g., blackfin tuna, common dolphinfish) relative to open-ocean species on the continental slope and beyond (e.g., blue marlin), suggesting that species spawning farther offshore were less likely to be impacted by surface oil or associated toxins (e.g., from dispersants) from the DWH event.
The return of epipelagic fish ELH stages to pre-spill abundances after a several-year period of lower abundances indicates a moderate level of resilience for these taxa, with the proviso that the abundances of these fishes are impacted by a number of factors other than DWH, both natural (e.g., Loop Current position) and anthropogenic (e.g., fishing pressure on spawning stock). It is possible that resilience of epipelagic fish ELH stages is enhanced by the oil detection and avoidance capabilities of the adult spawning stock. Documented shifts in the spatial distribution of certain taxa (e.g., blue marlin) away from the northern GoMex in summer 2010, possibly due to DWH remediation activities, may have resulted in short-term reductions in ELH stage abundances (Rooker et al., 2013), but their return afterward may have helped offset initial losses.
The deep-pelagic (meso- and bathypelagic combined) fish assemblage of the GoMex is one of the most species-rich in the World Ocean (Gartner et al., 1987; Sutton and Hopkins, 1996a; Sutton et al., 2017a; Moore et al., 2020). This high species count results from both natural factors and sampling/analysis intensity. Natural factors include the GoMex’s ecotonal location (tropical influence from the south paired with winter cooling in the north accommodates a mix of tropical, subtropical, and temperate species), well-oxygenated conditions throughout the deep water column (a limiting factor in other low-latitude ecoregions; Sutton et al., 2017a), the use of deep-pelagic habitat by early life stages of coastal and deep-demersal taxa (Moore et al., 2020), and resource partitioning among its members (Hopkins et al., 1996; Hopkins and Sutton, 1998; McEachran and Fechhelm, 1998). Additionally, the mesopelagic zone of the GoMex is one of the world’s most intensively studied (Hopkins et al., 1996 and references therein), particularly since DWH (Cook et al., 2020; Sutton et al., 2020). The number of quantitative, discrete-depth net samples from the GoMex’s bathypelagic zone collected since DWH likely exceeds the sum of all other such sample sets in oceanographic history.
The majority of the deep-pelagic fish species occurring in the GoMex are widely distributed, many circumglobal. The mesopelagic ichthyofauna is similar to those of the connected Caribbean and Sargasso Seas, with alterations in the rank order of dominants rather than species replacements (Gartner et al., 1987; Craddock et al., 1992; Ross et al., 2010; Sutton et al., 2010). This similarity is derived from ocean circulation (Caribbean Current→Loop Current→Florida Current→Gulf Stream; Maul, 1977; Sturges and Evans, 1983; Gallegos, 1996) coupled with the ethology/ecology of the fauna (e.g., diel vertical migration, early life stage development in the epipelagic promoting dispersal; (Daudén-Bengoa et al., 2020; Milligan and Sutton, 2020). Though there are less data for the GoMex bathypelagic zone, some evidence indicates that the GoMex may house a unique assemblage, with the numerically dominant species (Cyclothone obscura; Sutton et al., 2017b; Sutton et al., 2017c; Cook and Sutton, 2017a; Cook and Sutton, 2017b; Cook and Sutton, 2018; Cook and Sutton, 2019) differing from that of the adjacent Sargasso Sea (Cyclothone braueri; Sutton et al., 2010), albeit with much deeper sampling in the Sargasso Sea (to 5000 m).
Given the full-water-column nature of DWH, the vertical migratory behavior of most of the fauna, and the aforementioned deleterious effects of oil/dispersants on fishes, the vulnerability of deep-pelagic fishes to DWH was rated high. Vertical migrations attuned to solar cycle are a defining feature of GoMex deep-pelagic fishes (and macrocrustaceans and cephalopods, see below); more species migrate than do not, including the vast majority of the mesopelagic (during daytime) taxa (Gartner et al., 1987; Boswell et al., 2020; Milligan and Sutton, 2020). The ecological, biogeochemical, and subsurface-oil-spill-exposure ramifications of vertical migration are profound. Diel vertical migration turns a relatively depauperate daytime epipelagic zone (from an abundance and diversity perspective) into a dynamic and species-rich subsystem during nighttime. Sunrise then cues a massive migration back to depth. At the same time, this behavior ostensibly amplified the exposure of the migrating taxa to DWH toxins. As layered plumes formed during the initial high-pressure ejection of hydrocarbons, later mixed with dispersant, lateral advection of these features expanded the footprint of DWH in the deep-pelagic domain well beyond the immediate vicinity of Macondo. If a taxon were to stay within one depth stratum, it might have been “lucky” enough to not contact a subsurface plume, but vertically migrating fauna would not have been so fortunate. This applies even to the fauna below the major subsurface oil plume centered at 1100 m (Camilli et al., 2010), as post-DWH sampling revealed that the upper bathypelagic fauna can and do migrate vertically into the mesopelagic, and perhaps the epipelagic, zones (Sutton et al., 2020).
In terms of detection and avoidance of DWH toxins by deep-pelagic fishes, the current thinking regarding their behavior is that movements are primarily vertical, not horizontal (Pearcy and Laurs, 1966; Benoit-Bird et al., 2009), though evidence of horizontal migrations has been reported near oceanic islands (Benoit-Bird and Au, 2006) and cannot be discounted in the GoMex, especially above the continental slope. That said, vulnerability to large-scale water column pollutants was putatively high due to extended spatial overlap for long periods of time (Romero et al., 2018) combined with likely consumption of contaminated prey (Quintana-Rizzo et al., 2015). Berenshtein et al. (2020) reported that the water-column manifestation of DWH toxins was much larger than the surface footprint, meaning that the total portion of the fish assemblage exposed may have been substantial.
Of all the global mesopelagic ecoregions (sensu Sutton et al., 2017a), the trophic ecology of the GoMex is among the best known, due largely to the work of T.L. Hopkins and colleagues (see Hopkins et al., 1996 for an extensive listing of published information). These studies reported a high-degree of ecological niche partitioning among the GoMex deep-pelagic fish taxa, which the authors posit as a mechanism for high species diversification and maintenance in a relatively featureless, food-limited, but highly stable low-latitude ecosystem (Hopkins and Sutton, 1998, and references therein). This finding, coupled with highly selective feeding and increasing tightness of trophic linkages between predators and prey as trophic levels increase (Sutton and Hopkins, 1996b; Davison et al., 2013), suggests that GoMex deep-pelagic fishes are highly vulnerable to sudden variations in prey abundance and/or composition
Given the data gaps related to the population dynamics and long-term abundance variation of most deep-pelagic fishes, the overall characterization of resilience of this taxon was heavily weighted by inferences based on post-DWH sampling and analysis. These data revealed an extensive and persistent decline in abundance of both meso- and bathypelagic fishes between 2011 (NOAA-supported NRDA sampling) and 2015-2018 (GoMRI-supported DEEPEND sampling) (Cook et al., 2020; Sutton et al., 2020; Sutton et al., in review). These declines have been detected separately via direct net sampling and multi-frequency acoustic sensing (Sutton et al., in review). Because pre-DWH data were not available, direct ascription of the declines to DWH is not possible. However, Romero et al. (2018) demonstrated large increases in PAH concentrations in mesopelagic species following DWH, and in particular, concentrated in gonads. No other known environmental or ecological (see plankton treatments above) correlates would explain this community-level decline. That 2011 abundances, representing a period from 6-14 months after the Macondo wellhead was capped, were higher than abundances from 2015-2018 suggests that the sharpest population declines after DWH were delayed, putatively due to recurrent recruitment failure rather than immediate spawning stock mortality. Similar lags in fish population declines have been noted after other large marine oil spills (e.g., Barron et al., 2020).
The ecological concept of “life in the slow lane” is often applied to deep-sea fishes, especially to deep-benthic and deep-demersal fishes (Drazen and Haedrich, 2012), to explain low resilience in the face of human disturbance (mainly fishing). This concept does not necessarily apply to deep-pelagic fishes, at least to those few for which age and growth information is available. A summary based on extrapolation from available information suggests that deep-pelagic fish generation times and replacement rates in the GoMex are intermediate, with planktivorous fishes living approximately one to a few years, and higher order predators living a few years to over a decade (but not as long as some large epipelagic apex predators or deep-demersal and benthic fishes). The data gap regarding reproduction dynamics of GoMex deep-pelagic fishes parallels that of age and growth. Available information suggests spawning in most taxa is protracted, with multiple stages of egg development year-round, with little evidence of seasonality (Marks et al., 2020). Thus, we tentatively rank this attribute of resilience as moderate – presumably higher than that of deep-demersal fishes, but not high enough to rapidly replace losses due to mortality. The aforementioned protracted PAH contamination via maternal deposition in eggs (Romero et al. (2018) in combination with tight trophic coupling among assemblage members suggests low resilience to disturbance. This follows a global-scale analysis of marine fish foods webs that found open-ocean community resilience to be lower than that of coastal systems due to lower food-web interaction redundancy (Albouy et al., 2019).
Deep-pelagic macrocrustaceans (decapod shrimps, lophogastrids, and large euphausiids), like their fish counterparts, are major components of the GoMex and global oceanic ecosystems, playing an important role in trophic dynamics as both predators of phytoplankton and zooplankton (Roe, 1984; Hopkins et al., 1994 and references therein) and prey for cephalopods, cetaceans, seabirds, deep-pelagic fishes, and many species of commercially important fishes (Borodulina, 1972; Deagle et al., 2007; Schramm, 2007; Jayalakshmi et al., 2011). Of the GoMex open-ocean macrocrustaceans, five families comprise 95% of the assemblage numbers: Oplophoridae (31%), Benthesicymidae (21%), Euphausiidae (17%), Sergestidae (16%), and Eucopiidae (10%). For two of the three families for which statistical analyses have been completed, Oplophoridae and Euphausiidae, both the abundance and biomass of the respective assemblages were significantly higher landward of the 1000-m isobath than seaward, but the faunal compositions were largely the same (Burdett et al., 2017; Frank et al., 2020).
In a recurrent theme, lack of pre-spill data for the deeper-living macrocrustaceans, coupled with data gaps regarding life-history dynamics, made it difficult to assess vulnerability to and resilience from DWH. Much of these characterizations are based on what is known regarding similar taxa, and on post-DWH population level assessments. Regarding vulnerability to toxins, we do know that both crude oil and dispersants can have deleterious effects on crustaceans. both lethal and sublethal, including altered reproduction, respiration rates, growth, feeding and locomotion (Almeda et al., 2013a; Peiffer and Cohen, 2015). One study that tested the effects of crude oil (specifically, 1-methylnaphthalene) on deep-sea crustaceans showed a higher sensitivity than that expressed in coastal species (Knap et al., 2017). That, plus extensive spatiotemporal overlap to oil/dispersant as described for deep-pelagic fishes, led us to rank pelagic macrocrustacean vulnerability as high.
Regarding resilience, there is less life history information on this taxa than even deep-pelagic fishes, again requiring inference from post-DWH sampling results. These analyses revealed that the abundance of pelagic macrocrustaceans declined markedly between 2011 and 2015-2018, with a decline onset lag like that seen in fishes, and with the decrease statistically significant at all depths and times of day (Sutton et al., in review). The severity of decline was taxon-specific – the Benthesicymidae, Sergestidae, Oplophoridae and Eucopiidae decline was sizeable, but the Euphausiidae decline was major (Sutton et al., in review). Timm et al. (2020b) analyzed genetic connectivity of two species of Oplophoridae and one species of Sergestidae between the GoMex and the Atlantic proper. They concluded that hydrographic flow interacting with life history (vertical migration behavior, brooders vs. spawners, ontogenetic migrations) likely determines taxon-specific resilience to disturbances such as DWH. Overall, as with fishes, the preponderance of evidence suggests that pelagic macrocrustacean resilience to DWH was low.
Judkins (2009) documented 131 species in the Wider Caribbean region (GoMex plus Caribbean Sea and eastern Florida seaboard). Records of other species from the northern GoMex have since been added (Judkins et al. 2016a; Judkins et al., 2016b; Judkins et al., 2020), indicating that more cephalopod species may yet be discovered in the region’s deep-pelagic environment. The deep-pelagic cephalopod assemblage of the GoMex includes muscular families (e.g., ommastrephids, onychoteuthids) as well as gelatinous representatives such as the pelagic octopods (e.g., Japetella diaphana) and the vampire “squid” (Vampyroteuthis infernalis). Cephalopods are aggressive predators of fishes, crustaceans, and other molluscs (Leite et al., 2016; Hoving and Haddock, 2017; Olmos-Perez et al., 2017). In turn, they are food for larger fishes, marine mammals, and birds (Croxall and Prince, 1996; Clarke, 1996; Logan et al., 2013; Xavier et al., 2013). Due to their importance in the oceanic GoMex food web, cephalopods are potentially important vectors not only for carbon transfer through the water column but for petroleum contaminants as well (Bustamante et al., 2006; Unger et al., 2008; Arkhipkin, 2013; Romero et al., 2020).
Prior to DWH, one cruise series, the Sperm Whale Acoustic Prey Survey (SWAPS, Jan-Mar, 2010), collected over 3000 midwater cephalopods to examine cephalopod species richness in the potential prey field of northern GoMex sperm whales. Before that survey, deep-pelagic cephalopods were collected from the GoMex, but only ancillary to other research (e.g., Voss, 1956; Lipka, 1975; Cairns, 1976; Passarella, 1990; Passarella and Hopkins, 1991; Judkins et al., 2009; Judkins at al., 2010). Our knowledge of cephalopods in the deep GoMex has advanced substantially since 2010, including valuable insights into their diversity, ecology, genetics, population connectivity, and potential to be vectors of contaminants. Zapp Sluis et al. (2021) used a generalized additive modeling framework on mesozooplankton data (bongo samples) collected during 2015-2017 to characterize the summer daytime distribution and abundance of squid paralarvae in the epipelagic zone of the northern GoMex. Thirteen families were broadly distributed over the sampling area and paralarvae were present in ~76% of the stations sampled, meaning that this region may be an important spawning area for oceanic squid species. At the population level, Timm et al. (2020a) found evidence of homogeneous populations and significant inbreeding in Cranchia scabra and Pyroteuthis margaritifera, both diel vertical migrators. Conversely, Vampyroteuthis infernalis, a deep-living non-migrator, exhibited population structure that did not appear to be related to geography.
While cephalopods are highly mobile, the deep-pelagic species were classified as highly vulnerable to DWH based on spatial exposure and duration, particularly related to the suspended oil plume located between 900-1200 m depth. Vertical-distribution analyses showed that 37 species utilize the 900-1200 m depth zone, either by migration through it or by living within it (Judkins and Vecchione, 2020). Some species (e.g., bathyteuthids, bolitaenids, mastigoteuthids) remain at that depth for most of their adult lives. Molluscs have a higher tendency to bioaccumulate and concentrate contaminants than other groups (Gomes et al., 2013; Semedo et al., 2014) because of their lack of a highly developed hepato-billary system (unlike for fishes), further increasing vulnerability. This is alarmingly relevant in the GoMex, as deep-pelagic cephalopods were exposed to PAHs for an extended period. In a study of five GoMex species (Japetella diaphana, Abralia redfieldi, Histioteuthis corona, Leachia atlantica, and Onychoteuthis banksii) Romero et al. (2020) found a shift in the composition of PAHs in the tissue of all cephalopods after 2010, with a more petrogenic source in 2011 that weathered and mixed with other sources in 2015–2016.
Regarding resilience, cephalopods’ rapid growth rates and short lifespans (Wells and Clarke, 1996; Wood and O’Dor, 2000; Jackson and O’Dor, 2001) have been interpreted as an evolutionary response to high mortality rates (primarily predation; O’Dor and Webber, 1986). For deep-pelagic species this may not be the case. For example, Hoving and Robison (2017) examined three deep-pelagic species in Monterey Canyon and found they had increased lifespans as well as slower growth rates than their shallow relatives; therefore, their population dynamics may be quite different than the well-known coastal species. These attributes of resilience were not scored due to insufficient data. Aspects of life histories, including life span, are still unknown for many deep-sea cephalopod species. Because of the dearth of detailed information about most oceanic cephalopod species, they are often treated as a single “black box” in most ecosystem models (de la Chesnais et al., 2017). We know enough about the variability among species and higher evolutionary groups to be confident that this “black-box” approach is inadequate. Because cephalopods are central components of oceanic food webs, impacts on this group likely had important repercussions for both their predators (e.g., fishes, mammals, birds) and their prey (fishes, crustaceans, other cephalopods, and possibly other taxa).
At least 17 species of oceanic cetaceans are regularly found in the deep GoMex beyond the continental shelf break (Würsig et al., 2000). The only mysticete (baleen whale) regularly observed in the GoMex is the newly defined Rice’s whale, Balaenoptera ricei (Rosel et al., 2021), which while critically endangered, does not venture far beyond the continental slope into deep water. This leaves only odontocetes (toothed whales) that fit broadly into two categories: shallow divers, including various dolphin species in the genus Stenella and other smaller delphinids, and deep divers, including sperm, pygmy sperm, beaked, and pilot whales. Shallow divers feed nocturnally on vertically migrating mesopelagic cephalopods and myctophids (Robertson and Chivers, 1998), and some may also forage opportunistically on epipelagic prey. Deep divers routinely prey primarily on meso- and bathypelagic cephalopods (Fertl et al., 1997; Judkins et al., 2013; West et al., 2017). Due to their high caloric needs, which require elevated prey consumption (Benoit-Bird, 2004; Farmer et al., 2018), these top predators serve as sentinel species whose population declines or spatial shifts may reflect broader but less readily observable changes in deep-pelagic ecosystems.
Shipboard visual surveys for oceanic cetaceans have been irregularly conducted in the GoMex since the since the early 1990’s (Mullin and Hoggard, 2000; Jochens et al., 2008). Oceanic GoMex cetaceans are designated as separate stocks from conspecifics in the broader Atlantic due to an overall lack of evidence of gene flow between the two systems. Pantropical spotted dolphins (Stenella attenuata) have historically been the most commonly observed offshore species during visual surveys, with stock size estimates near 50,000 (Waring et al., 2016), followed by spinner dolphins (S. longirostris) and oceanic bottlenose dolphins (Tursiops truncatus), with stock sizes estimated in the thousands to tens of thousands (Waring et al., 2013). Deep-diving odontocete stock sizes are generally smaller, the largest being approximately 2,000 pilot whales (Globicephala macrorhynchus) and fewer than 1,000 sperm whales (Physeter microcephalus) (Waring et al., 2016). Beaked whale (Ziphiidae) and pygmy/dwarf sperm whale (Kogia spp.) stock sizes have typically been estimated in the low hundreds, but these may be underestimates due to the cryptic surface behaviors of these species (Cholewiak et al., 2017; Hildebrand et al., 2019). Although survey efforts have documented cetacean diversity and spatial distributions in the region (Davis et al., 2002), resulting stock size estimates have high associated uncertainties, and estimates have been too infrequent to infer population trends. A lack of survey data from the Mexican portion of the GoMex further compounds overall uncertainty for these highly mobile species. Prior to 2018, surveys were primarily conducted in summer, and did not capture information relating to seasonal cycles or distributional shifts in response to oceanographic conditions. Intensive visual survey effort across seasons was conducted in 2018-2019 to address this data gap, however pre-DWH baseline measurements for comparison are lacking.
As part of the NRDA in 2010, a passive acoustic monitoring program was initiated to assess long-term trends in cetacean occurrence following DWH (Hildebrand et al., 2015; Sidorovskaia et al., 2016). This and other programs demonstrated the high temporal resolution needed to investigate long-term trends in species occurrence. Pre-DWH passive acoustic monitoring data are extremely limited. However, the passive acoustic monitoring record since 2010, in conjunction with increased visual survey effort, strongly suggests long-term declines in offshore Stenella dolphin occurrence within the US EEZ in the GoMex (Frasier et al., 2019). Due to lack of spatial coverage across the GoMex, we cannot discern whether observed declines represent a spatial shift or mortality.
The available information on the impacts of oil on odontocetes has been largely focused on coastal species and environments, specifically killer whales following the Exxon Valdez Oil Spill (EVOS; Matkin et al., 2008) and GoMex Bay, Sound, and Estuarine (BSE) bottlenose dolphins following DWH (Deepwater Horizon Natural Resource Damage Assessment Trustees, 2016; Schwacke et al., 2017). In both cases, long-term impacts on the observed populations were high, with elevated rates of chronic disease and mortality, and suppressed long-term reproductive capacity (Dahlheim and Matkin, 1994; Matkin et al., 2008; Schwacke et al., 2013; Schwacke et al., 2017; Smith et al., 2017; Takeshita et al., 2017). Population recovery time for Barataria Bay bottlenose dolphins following DWH is estimated to be 39 years (Smith et al., 2017).
Regarding vulnerability of oceanic cetaceans to DWH, attribute scoring ranged from low to moderate to high, resulting in a cumulative rating of moderate. Odontocetes possess biosonar and are likely capable of detecting oil slicks (Geraci et al., 1983), however there is no evidence of oil avoidance in coastal proxies (Smultea and Wursig, 1995). Similarly, oceanic cetaceans were observed, directly both visually and acoustically, within the DWH oil slick, with no evidence of avoidance or reduced presence (Hildebrand et al., 2015; Dias et al., 2017; Frasier et al., 2019; Morano et al., 2020). Initial acute exposure to surface oil through inhalation, ingestion, and dermal contact for a portion of the major stocks is certain. Exposure to the deep plume during foraging dives likely occurred for deep divers, which are known to forage at depths near or over 1000 m depth (Watwood et al., 2006; Shearer et al., 2019). Site fidelity of offshore cetaceans is likely considerably lower than that observed in coastal proxies, which could mitigate chronic exposure. Cetaceans are capable of metabolizing oil compounds including PAHs (Lee and Anderson, 2005). Most studies of the health impacts of oil ingestion in mammals consider ingestion of raw oil and visibly oiled prey (Engelhardt et al., 1977; Geraci, 1990; Matkin et al., 2008; Schwacke et al., 2013). The health effects of long-term ingestion of lower concentrations of oil-derived compounds through prey are not well studied but may be more relevant to offshore cetaceans. Deep divers may be more vulnerable than shallow foragers to chronic toxicity if feeding near the bottom on prey with strong trophic ties to contaminated sediments and/or deep suspended plumes. Limited available evidence suggests that dispersants may have toxic effects at the cellular level (Wise et al., 2014) but more research is needed to evaluate organismal effects. Odontocetes are well known to bioaccumulate fat-soluble contaminants from their prey (Aguilar et al., 1999). This may be a particular concern for myctophid-eating shallow divers (e.g. Lahaye et al., 2006), however fishes metabolize PAHs more effectively than cephalopods (Law and Whinnett, 1992; Unger et al., 2008; Romero et al., 2020).
DWH occurred against a backdrop of other chronic stressors for oceanic cetaceans, which may have increased vulnerability, and continue to affect resilience. An unusual mortality event had already begun among delphinids in early 2010, two months prior to the spill, and was then exacerbated by DWH, lasting until July 2014 (Litz et al., 2014; Venn-Watson et al., 2015). More broadly, ongoing stress related to excessive anthropogenic noise, fisheries bycatch and entanglement, prey pressure related to climate change and fishing, ship strikes, ocean plastics, and environmental pollutants may limit population recovery (Weilgart, 2007; Lewison et al., 2014; Carroll et al., 2017). Oceanic cetaceans make up only a small fraction of the stranding record, which is typically dominated by coastal species (Litz et al., 2014). This pattern risks being interpreted as a lack of impact; however, the lack of oceanic species representation is likely linked to a lower probability of stranding when mortalities occur offshore (Williams et al., 2011).
Our understanding of the system’s carrying capacity is clouded by both historic and modern pressures. Sperm whales, a species of primary concern, have been listed as endangered since 1970 following mortality due to commercial whaling (Reeves et al., 2011). The GoMex is believed to function as a nursery for sperm whale populations in the broader Atlantic (Englehaupt et al., 2009). Matriarchal groups with calves are prevalent in the region, while the large males typical of high latitudes are less commonly observed (Solsona Berga, 2019). Social groups are also common among the shallow divers, with group sizes ranging from half a dozen to hundreds of delphinids (Würsig et al., 2000). Odontocete calves inhabit the same waters as adults, and nurse until the age at which they can begin foraging, therefore young are highly dependent and trophically linked to their mothers as well as dependent on the social unit for protection (Perrin and Reilly, 1984). Population resilience and recovery is limited in these long-lived animals by long gestation times, elevated age at reproductive maturity, and low number of offspring produced (typically a single calf per cycle) at high energetic cost.
The current restoration paradigm for GoMex cetaceans relies on mitigation of compounding stressors to bolster stock resilience. Planned restoration actions include mitigating bycatch and fisheries interactions, vessel collisions, anthropogenic noise, illegal feeding and harassment, investing in coastal restoration, and expanding marine protected areas (Deepwater Horizon Natural Resource Damage Assessment Trustees, 2016). However, even in an optimal environment, recovery is likely to be slow. More information on the mechanisms of impacts is needed, particularly for offshore cetaceans, before effective management mechanisms can be identified (Smith et al., 2017; Ackleh et al., 2018).
Due to the nature and size of DWH, there were no open-ocean taxa that we considered low vulnerability and high resilience. Phyto- and mesozooplankton were classified as highly vulnerable due to high spatial overlap with DWH toxins, low relative mobility, and high sensitivity to DWH toxins, yet they were also highly resilient, with no detectible DWH effects months to a year after the event. Early life history stages of large epipelagic fishes exhibited temporal abundance trends similar to mesozooplankton, but with larger initial declines before returning to natural ranges within one or two years, and with the added factor that abundances and distributions may be tied to possible relocation out of the DWH-affected area by the adult spawning stock of some species. Examination of post-DWH time-series data for deep-pelagic micronekton (fishes, macrocrustaceans, and cephalopods) suggested that intermediate size classes were highly vulnerable and exhibited the least resilience, evidenced by the observed declines after DWH. Cases such as this, where the lack of pre-disaster data precluded definitive damage assessment, confound a confident synthesis. Regarding the largest-bodied taxon considered here, the oceanic cetaceans, although surveys were conducted prior to DWH, time-series trajectories have very high uncertainties due to the nature of the surveys. Those data that do exist, primarily post-DWH, strongly suggest long-term abundance declines in shallow-diving cetaceans in the northern GoMex. Overall, vulnerability of cetaceans was classified as moderate, while resilience was tentatively classified as low due to the low fecundity and long generation times. If open-ocean cetacean stock injuries scale with those of coastal bottlenose dolphins, recovery could take several decades (Smith et al., 2017).
In closing, the open-ocean GoMex exemplifies the complex situation of a very large, physically dynamic, and understudied ecosystem rapidly subjected to large volumes of hydrocarbons and dispersants. All taxa examined were deemed moderately to highly vulnerable to DWH effects, while resilience was highly variable and taxon-specific. We conclude that, for the intermediate and higher trophic levels of the open-ocean GoMex, even a decade after the event we have no evidence to suggest that impacts from DWH are “over.”
All authors collaborated in the analysis, evaluation, and publication writing and editing leading to this contribution. This publication was greatly aided by two workshops conducted by a subset of the authors (TS, RM, KF, DH, JH, SJ, HJ, SM, KR, IR, and MV, led by SM) and others in July (St. Petersburg, FL, United States) and October (Washington, D.C., United States) 2019.
This paper is a result of research funded by the National Oceanic and Atmospheric Administration’s RESTORE Science Program under award NA19NOS4510193 to Nova Southeastern University. Data acquisition, synthesis and manuscript preparation was also supported by grants from the Gulf of Mexico Research Initiative and the Gulf of Mexico Alliance.
The authors declare that the research was conducted in the absence of any commercial or financial relationships that could be construed as a potential conflict of interest.
All claims expressed in this article are solely those of the authors and do not necessarily represent those of their affiliated organizations, or those of the publisher, the editors and the reviewers. Any product that may be evaluated in this article, or claim that may be made by its manufacturer, is not guaranteed or endorsed by the publisher.
For their valuable input we thank the following participants of the Gulf of Mexico Research Initiative (GoMRI)-supported Core Area 3 (Ecology/Ecosystems) Synthesis Workshop I, July 23-25, 2019, St. Petersburg, Florida, and Workshop II, October 9-11, Washington D.C.: Cameron Ainsworth, Stacy Calhoun, Matt Campbell, Erik Cordes, Sherryl Gilbert, Thomas Guilment, Ken Halanych, David Hanisko, Jenny Litz, Craig McClain, Paul Montagna, Joel Ortega-Ortiz, Claire Paris, Will Patterson, Ernst Peebles, Adam Pollack, Lori Schwacke, and Verena Wang. We also thank the GoMRI Research Board Core 3 facilitators for their excellent guidance throughout the synthesis process: Rita Colwell, Richard Dodge, Michael Feldman, Ken Halanych, Bill Hogarth, Margaret Leinen, Jennifer Petitt, Kevin Shaw, Rick Shaw, Bob Shipp, and Chuck Wilson.
Ackleh A., Caswell H., Chiquet R., Tang T., Veprauskas A. (2018). Sensitivity Analysis of the Recovery Time for a Population Under the Impact of an Environmental Disturbance. Nat. Resour. Model. 32, e12166. doi: 10.1111/nrm.12166
Aguilar A., Borrell A., Pastor T. (1999). Biological Factors Affecting Variability of Persistent Pollutant Levels in Cetaceans. J. Cetacean Res. Manage. 1, 83–116. doi: 10.47536/jcrm.v1i1.264
Albouy C., Archambault P., Appeltans W., Araújo M. B., Beauchesne D., Cazelles K., et al. (2019). The Marine Fish Food Web Is Globally Connected. Nat. Ecol. Evol. 3 (8), 1153–1161. doi: 10.1038/s41559-019-0950-y
Almeda R., Baca S., Hyatt C., Buskey E. J. (2014a). Ingestion and Sublethal Effects of Physically and Chemically Dispersed Crude Oil on Marine Planktonic Copepods. Ecotoxicology 23, 988–1003. doi: 10.1007/s10646-014-1242-6
Almeda R., Connelly T. L., Buskey E. J. (2014b). Novel Insight Into the Role of Heterotrophic Dinoflagellates in the Fate of Crude Oil in the Sea. Sci. Rep. 4, 1–9. doi: 10.1038/srep07560
Almeda R., Connelly T. L., Buskey E. J. (2016). How Much Crude Oil can Zooplankton Ingest? Estimating the Quantity of Dispersed Crude Oil Defecated by Planktonic Copepods. Environ. Pollut. 208, 645–654. doi: 10.1016/j.envpol.2015.10.041
Almeda R., Hyatt C., Buskey E. J. (2014c). Toxicity of Dispersant Corexit 9500A and Crude Oil to Marine Microzooplankton. Ecotoxicol. Environ. Saf. 106, 76–85. doi: 10.1016/j.ecoenv.2014.04.028
Almeda R., Wambaugh Z., Chai C., Wang Z., Liu Z., Buskey E. J. (2013a). Effects of Crude Oil Exposure on Bioaccumulation of Polycyclic Aromatic Hydrocarbons and Survival of Adult and Larval Stages of Gelatinous Zooplankton. PloS One 8, e74476. doi: 10.1371/journal.pone.0074476
Almeda R., Wambaugh Z., Wang Z., Hyatt C., Liu Z., Buskey E. J. (2013b). Interactions Between Zooplankton and Crude Oil: Toxic Effects and Bioaccumulation of Polycyclic Aromatic Hydrocarbons. PloS One 8, e67212. doi: 10.1371/journal.pone.0067212
Androulidakis Y., Kourafalou V., Henaff M. L., Kang H. S., Sutton T. T., Chen S., et al. (2019). Offshore Spreading of Mississippi Waters: Pathways and Vertical Structure Under Eddy Influence. J. Geophysical Res.: Oceans 124, 1–27. doi: 10.1029/2018JC014661
Angel M. V. (1993). Biodiversity of the Pelagic Ocean. Conserv. Biol. 7, 760–772. doi: 10.1046/j.1523-1739.1993.740760.x
Angel M. V. (1997). “What is the Deep Sea?,” in Deep-Sea Fishes. Eds. Randall D. J., Farrell A. P. (New York, NY: Academic Press), 1–41.
Arkhipkin A. I. (2013). Squid as Nutrient Vectors Linking Southwest Atlantic Marine Ecosystems. Deep Sea Res. Pt II 95, 7–20. doi: 10.1016/j.dsr2.2012.07.003
Banse K. (1995). Zooplankton: Pivotal Role in the Control of Ocean Production. ICES J. Mar. Sci. 52, 265–277. doi: 10.1016/1054-3139(95)80043-3
Barron M. G. (2017). Photoenhanced Toxicity of Petroleum to Aquatic Invertebrates and Fish. Arch. Environ. Contam. Toxicol. 73, 40–46. doi: 10.1007/s00244-016-0360-y
Barron M. G., Vivian D. N., Heintz R. A., Yim U. H. (2020). Long-Term Ecological Impacts From Oil Spills: Comparison of Exxon Valdez, Hebei Spirit, and Deepwater Horizon. Environ. Sci. Technol. 54 (11), 6456–6467. doi: 10.1021/acs.est.9b05020
Belabbassi L., Chapman P., Nowlin W. D. Jr., Jochens A. E., Biggs D. C. (2005). Summertime Nutrient Supply to Near-Surface Waters of the Northeastern Gulf of Mexico: 1998, 1999, and 2000. Gulf Mex. Sci. 23 (2), 137–160. doi: 10.18785/goms.2302.01
Benoit-Bird K. J. (2004). Prey Caloric Value and Predator Energy Needs: Foraging Predictions for Wild Spinner Dolphins. Mar. Biol. 145, 435–444. doi: 10.1007/s00227-004-1339-1
Benoit-Bird K. J., Au W. W. (2006). Extreme Diel Horizontal Migrations by a Tropical Nearshore Resident Micronekton Community. Mar. Ecol. Prog. Ser. 319, 1–14. doi: 10.3354/meps319001
Benoit-Bird K. J., Au W. W., Wisdoma D. W. (2009). Nocturnal Light and Lunar Cycle Effects on Diel Migration of Micronekton. Limnol. Oceanogr. 54 (5), 1789–1800. doi: 10.4319/lo.2009.54.5.1789
Berenshtein I., Paris C. B., Perlin N., Alloy M. M., Joye S. B., Murawski S. (2020). Invisible Oil Beyond the Deepwater Horizon Satellite Footprint. Sci. Adv. 6, eaaw8863. doi: 10.1126/sciadv.aaw8863
Biggs D. C., Ressler P. H. (2001). Distribution and Abundance of Phytoplankton, Zooplankton, Ichthyoplankton, and Micronekton in the Deepwater Gulf of Mexico. Gulf Mex. Sci. 19, 1. doi: 10.18785/goms.1901.02
Borodulina O. D. (1972). The Feeding of Mesopelagic Predatory Fish in the Open Ocean. J. Ichthyol. 12, 692–703.
Boswell K. M., D’Elia M., Johnston M. W., Mohan J. A., Warren J. D., Wells R. J. D., et al. (2020). Oceanographic Structure and Light Levels Drive Patterns of Sound Scattering Layers in a Low-Latitude Oceanic System. Front. Mar. Sci. 7. doi: 10.3389/fmars.2020.00051
Brooks G. R., Larson R. A., Schwing P. T., Romero I. C., Moore C., Reichart G. J., et al. (2015). Sedimentation Pulse in the NE Gulf of Mexico Following the 2010 DWH Blowout. PloS One 10 (7), e0132341. doi: 10.1371/journal.pone.0132341
Burdett E., Fine C., Sutton T. T., Cook A. B., Frank T. (2017). Geographic and Depth Distributions, Ontogeny, and Reproductive Seasonality Decapod Shrimps (Caridea: Oplophoridae) From the Northeastern Gulf of Mexico. B. Mar. Sci. 93, 743–767. doi: 10.5343/bms.2016.1083
Burghart S. E., Hopkins T. L., Torres J. J. (2007). The Bathypelagic Decapoda, Lophogastrida, and Mysida of the Eastern Gulf of Mexico. Mar. Biol. 152, 315–327. doi: 10.1007/s00227-007-0691-3
Bustamante P., Lahaye V., Durnez C., Churland C., Caurnat F. (2006). Total and Organic Hg Concentrations in Cephalopods From the North Eastern Atlantic Waters: Influence of Geographical Origin and Feeding Ecology. Sci. Total Environ. 68, 585–596. doi: 10.1016/j.scitotenv.2006.01.038
Cairns S. (1976). Cephalopods Collected in the Straits of Florida by the R/V Gerda. Bull. Mar. Sci. 26 (2), 233–272.
Camilli R., Reddy C. M., Yoerger D. R., Van Mooy B. A. S., Jakuba M. V., Kinsey J. C., et al. (2010). Tracking Hydrocarbon Plume Transport and Biodegradation at Deepwater Horizon. Science 330, 201–204. doi: 10.1126/science.1195223
Carroll A. G., Przeslawski R., Duncan A., Gunning M., Bruce B. (2017). A Critical Review of the Potential Impacts of Marine Seismic Surveys on Fish & Invertebrates. Mar. Pollut. Bull. 114, 9–24. doi: 10.1016/j.marpolbul.2016.11.038
Chanton J. P., Cherrier J., Wilson R. M., Sarkodee-Adoo J., Bosman S., Mickle A., et al. (2012). Radiocarbon Evidence That Carbon From the Deepwater Horizon Spill Entered the Planktonic Food Web of the Gulf of Mexico. Environ. Res. Lett. 7, 045303. doi: 10.1088/1748-9326/7/4/045303
Chanton J., Zhao T., Rosenheim B. E., Joye S., Bosman S., Brunner C., et al. (2015). Using Natural Abundance Radiocarbon to Trace the Flux of Petrocarbon to the Seafloor Following the Deepwater Horizon Oil Spill. Environ. Sci. Technol. 49 (2), 847–854. doi: 10.1021/es5046524
Cholewiak D., DeAngelis A. I., Palka D., Corkeron P. J., Van Parijs S. M. (2017). Beaked Whales Demonstrate a Marked Acoustic Response to the Use of Shipboard Echosounders. R. Soc Open Sci. 4, 170940. doi: 10.1098/rsos.170940
Clarke M. R. (1996). The Role of Cephalopods in the World’s Oceans - Cephalopods as Prey. III. Cetaceans. Philos. Trans. R. Soc London Ser. B 351, 1053–1065. doi: 10.1098/rstb.1996.0093
Cohen J. H., McCormick L. R., Burkhardt S. M. (2014). Effects of Dispersant and Oil on Survival and Swimming Activity in a Marine Copepod. Bull. Environ. Contam. Toxicol. 92, 381–387. doi: 10.1007/s00128-013-1191-4
Cook A. B., Bernard A. M., Boswell K. M., Bracken-Grissom H., D’Elia M., DeRada S., et al. (2020). A Multidisciplinary Approach to Investigate Deep-Pelagic Ecosystem Dynamics in the Gulf of Mexico Following Deepwater Horizon. Front. Mar. Sci. 7. doi: 10.3389/fmars.2020.548880
Cook A., Sutton T. T. (2017a). Inventory of Gulf Oceanic Fauna Data Including Species, Weight, and Measurements. Cruises DP01 May 1-8, 2015 and DP02 August 9-21, 2015 R/V on the Point Sur in the Northern Gulf of Mexico (Distributed by: Gulf of Mexico Research Initiative Information and Data Cooperative (GRIIDC)) (Corpus Christi, TX: Harte Research Institute, Texas A&M University). doi: 10.7266/N70P0X3T
Cook A., Sutton T. T. (2017b). Inventory of Gulf of Mexico Oceanic Fauna Data Including Species, Weight, and Measurements From R/V Point Sur (Cruises DP03 and DP04) May-August 2016. Distributed by: Gulf of Mexico Research Initiative Information and Data Cooperative (GRIIDC) (Corpus Christi: Harte Research Institute, Texas A&M University). doi: 10.7266/N7XP7385
Cook A., Sutton T. T. (2018). Inventory of Oceanic Fauna Data Including Species, Weight, and Measurements From R/V Point Sur (Cruise DP05) in the Gulf of Mexico From 2017-05-01 to 2017-05-11. Distributed by: Gulf of Mexico Research Initiative Information and Data Cooperative (GRIIDC) (Corpus Christi, TX: Harte Research Institute, Texas A&M University). doi: 10.7266/N7902234
Cook A., Sutton T. T. (2019). Inventory of Oceanic Fauna Data Including Species, Weight, and Measurements From R/V Point Sur Cruise PS19-04 (DP06) in the Gulf of Mexico From 2018-07-19 to 2018-08-01. Distributed by: Gulf of Mexico Research Initiative Information and Data Cooperative (GRIIDC) (Corpus Christi: Harte Research Institute, Texas A&M University). doi: 10.7266/n7-ac8e-0240
Cornic M., Rooker J. R. (2018). Influence of Oceanographic Conditions on the Distribution and Abundance of Blackfin Tuna (Thunnus Atlanticus) Larvae in the Gulf of Mexico. Fish. Res. 201, 1–10. doi: 10.1016/j.fishres.2017.12.015
Cornic M., Rooker J. R. (2021). Temporal Shifts in the Abundance and Preferred Habitats of Yellowfin and Bigeye Tuna Larvae in the Gulf of Mexico. J. Marine Syst. 217, 103524. doi: 10.1016/j.jmarsys.2021.103524
Cornic M., Smith B. L., Kitchens L. L., Bremer J. R. A., Rooker J. R. (2018). Abundance and Habitat Associations of Tuna Larvae in the Surface Water of the Gulf of Mexico. Hydrobiologia 806, 29–46. doi: 10.1007/s10750-017-3330-0
Craddock J. E., Backus R. H., Daher M. A. (1992). Vertical Distribution and Species Composition of Midwater Fishes in Warm-Core Gulf Stream Meander/Ring 82-H. Deep Sea Res. 39, S203–S218. doi: 10.1016/S0198-0149(11)80012-9
Croxall J. P., Prince P. A. (1996). Cephalopods as Prey. I. Seabirds. Philos. Trans. R. Soc London Ser. B. 351, 1045–1052. doi: 10.1098/rstb.1996.0091
Cushing D. H. (1995). The Long-Term Relationship Between Zooplankton and Fish. ICES J. Mar. Sci. 52, 611–626. doi: 10.1016/1054-3139(95)80076-X
Dagg M. J. (1995). Copepod Grazing and the Fate of Phytoplankton in the Northern Gulf of Mexico. Cont. Shelf Res. 15, 1303–1317. doi: 10.1016/0278-4343(94)00086-3
Dagg M. J., Whitledge T. E. (1991). Concentrations of Copepod Nauplii Associated With the Nutrient-Rich Plume of the Mississippi River. Cont. Shelf Res. 11, 1409–1423. doi: 10.1016/0278-4343(91)90043-6
Dahlheim M. E., Matkin C. O. (1994). “Assessment of Injuries to Prince William Sound Killer Whales,” in Marine Mammals and the Exxon Valdez. Ed. Loughlin T. R. (San Diego, CA: Academic Press), 163–172.
Daly K. L., Passow U., Chanton J., Hollander D. (2016). Assessing the Impacts of Oil-Associated Marine Snow Formation and Sedimentation During and After the Deepwater Horizon Oil Spill. Anthropocene 13, 18–33. doi: 10.1016/j.ancene.2016.01.006
Daly K. L., Remsen A., Outram O., Broadbent H., Kramer K., Dubikas K. (2021). Resilience of the Zooplankton Community in the Northeast Gulf of Mexico During and After the Deepwater Horizon Oil Spill. Mar. Pollut. Bull. 163, 111882. doi: 10.1016/j.marpolbul.2020.111882
Daly K. L., Vaz A., Paris C. B. (2020). “Physical Processes Influencing the Sedimentation and Lateral Transport of MOSSFA in the NE Gulf of Mexico,” in Scenarios and Responses to Future Deep Oil Spills - Fighting the Next War. Eds. Murawski S. A., Hollander D., Ainsworth C., Gilbert S., Paris C. B., Schlüter M., Wetzel D. (Cham, Switzerland: Springer Nature), 300–314. doi: 10.1007/978-3-030-12963-7_18
Daudén-Bengoa G., Jiménez-Rosenberg S. P. A., Compaire J. C., del Pilar Echeverri-García L., Pérez-Brunius P., Herzka S. Z. (2020). Larval Fish Assemblages of Myctophids in the Deep Water Region of the Southern Gulf of Mexico Linked to Oceanographic Conditions. Deep Sea Res. Pt I 155, 103181. doi: 10.1016/j.dsr.2019.103181
Davison P. C., Checkley D. M. Jr., Koslow J. A., Barlow J. (2013). Carbon Export Mediated by Mesopelagic Fishes in the Northeast Pacific Ocean. Prog. Oceanogr. 116, 14–30. doi: 10.1016/j.pocean.2013.05.013
Davis R. W., Ortega-Ortiz J. G., Ribic C. A., Evans W. E., Biggs D. C., Ressler P. H., et al. (2002). Cetacean Habitat in the Northern Oceanic Gulf of Mexico. Deep Sea Res. Pt I 49, 121–142. doi: 10.1016/S0967-0637(01)00035-8
Deagle B., Gales N., Evans K., Jarman S., Robinson S., Trebilco R., et al. (2007). Studying Seabird Diet Through Genetic Analysis of Faeces: A Case Study on Macaroni Penguins (Eudyptes Chrysolophus). PloS One 2, e831. doi: 10.1371/journal.pone.0000831
Deepwater Horizon Natural Resource Damage Assessment Trustees (2016) Deepwater Horizon Oil Spill: Final Programmatic Damage Assessment and Restoration Plan and Final Programmatic Environmental Impact Statement. Available at: http://www.gulfspillrestoration.noaa.gov/restorationplanning/gulf-plan (Accessed March 11, 2021).
de la Chesnais T., Pecl G. T., Fulton E. A., Tracey S. (2017). Advancing Ecosystem Models to Incorporate Cephalopods and Assess Their Implications in Systems’ Evolution. Rev. Fish Biol. Fish. 29, 313–334. doi: 10.1007/s11160-019-09554-0
D’Elia M., Warren J. D., Rodriguez-Pinot I., Sutton T. T., Cook A. B., Boswell K. M. (2016). Diel Variation in the Vertical Distribution of Deep-Water Scattering Layers in the Gulf of Mexico. Deep Sea Res. Pt I 115, 91–102. doi: 10.1016/j.dsr.2016.05.014
Dias L. A., Litz J., Garrison L., Martinez A., Barry K., Speakman T. (2017). Exposure of Cetaceans to Petroleum Products Following the Deepwater Horizon Oil Spill in the Gulf of Mexico. Endanger. Species Res. 33, 119–125. doi: 10.3354/esr00770
Diercks A. R., Romero I. C., Larson R. A., Schwing P., Harris A., Bosman S., et al. (2021). Resuspension, Redistribution and Deposition of Oil-Residues to Offshore Depocenters After the Deepwater Horizon Oil Spill. Front. Mar. Sci. 8 doi: 10.3389/fmars.2021.630183
Dortch Q. (1997). “VII. Phytoplankton Characteristics,” in 1997. An Observational Study of the Mississippi-Atchafalaya Coastal Plume: Final Report. OCS Study MMS98-0040. Ed. Murray S. P. (New Orleans, La: U.S. Dept. of the Interior, Minerals Mgmt. Service, Gulf of Mexico OCS Region), 513pp.
Drazen J. C., Haedrich R. L. (2012). A Continuum of Life Histories in Deep-Sea Demersal Fishes. Deep Sea Res. Pt I 61, 34–42. doi: 10.1016/j.dsr.2011.11.002
Du M., Kessler J. D. (2012). Assessment of the Spatial and Temporal Variability of Bulk Hydrocarbon Respiration Following the Deepwater Horizon Oil Spill. Environ. Sci. Technol. 46, 10499–10507. doi: 10.1021/es301363k
Engelhardt F. R., Geraci J. R., Smith T. G. (1977). Uptake and Clearance of Petroleum Hydrocarbons in the Ringed Seal. Phoca hispida. J. Fish. Res. Board Can. 34, 1143–1147. doi: 10.1139/f77-171
Englehaupt D., Rus Hoelzel A., Nicholson C., Frantzis A., Mesnick S., Gero S., et al. (2009). Female Philopatry in Coastal Basins and Male Dispersion Across the North Atlantic in a Highly Mobile Marine Species, the Sperm Whale (Physeter Macrocephalus). Mol. Ecol. 18, 4193–4205. doi: 10.1111/j.1365-294X.2009.04355.x
Farmer N. A., Baker K., Zeddies D. G., Denes S. L., Noren D. P., Garrison L. P., et al. (2018). Population Consequences of Disturbance by Offshore Oil and Gas Activity for Endangered Sperm Whales (Physeter Macrocephalus). Biol. Conserv. 227, 189–204. doi: 10.1016/j.biocon.2018.09.006
Fernández-Carrera A., Rogers K. L., Weber S. C., Chanton J. P., Montoya J. P. (2016). Deep Water Horizon Oil and Methane Carbon Entered the Food Web in the Gulf of Mexico. Limnol. Oceanogr. 61, S387–S400. doi: 10.1002/lno.10440
Fertl D., Schiro A., Collier S., Worthy G. A. J. (1997). Stranding of a Cuvier’s Beaked Whale (Ziphius Cavirostris) in Southern Texas, With Comments on Stomach Contents. Gulf Mex. Sci. 15, 5. doi: 10.18785/goms.1502.05
Frank T. M., Fine C. D., Burdett E. A., Cook A. B., Sutton T. T. (2020). The Vertical and Horizontal Distribution of Deep-Sea Crustaceans in the Order Euphausiacea in the Vicinity of the Deepwater Horizon Oil Spill. Front. Mar. Sci. 7. doi: 10.3389/fmars.2020.00099
Frasier K. E., Solsona-Berga A., Stokes L., Hildebrand J. A. (2019). “Impacts of the Deepwater Horizon Oil Spill on Marine Mammals and Sea Turtles,” in Deep Oil Spills. Eds. Murawski S. A., Ainsworth C. H., Gilbert S., Hollander D. J., Paris C. B., Schlüter M., Wetzel D. L. (Cham, Switzerland:Springer Nature), 431–462. doi: 10.1007/978-3-030-11605-7_26
Gallegos A. (1996). “Descriptive Physical Oceanography of the Caribbean Sea,” in Small Islands: Marine Science and Sustainable Development, vol. 51 . Ed. Maul G. A. (Washington, DC: American Geophysical Union), 36–55. doi: 10.1029/CE051
Gartner J. V., Hopkins T. L., Baird R. C., Milliken D. M. (1987). The Lanternfishes (Pisces, Myctophidae) of the Eastern Gulf of Mexico. Fish. Bull. 85, 81–98.
Geraci J. R. (1990). “Physiologic and Toxic Effects on Cetaceans,” in Sea Mammals and Oil: Confronting the Risks. Eds. Geraci J. R., St. Aubin D. J. (San Diego, CA: Academic Press Inc), 167–192.
Geraci J., St. Aubin D. J., Reisman R. (1983). Bottlenose Dolphins, Tursiops Truncatus, Can Detect Oil. Can. J. Fish. Aquat. Sci. 40, 1516–1522. doi: 10.1139/f83-174
Gomes F., Oliveira M., Ramalhosa M. J., Delerue-Matos C., Morais S. (2013). Polycyclic Aromatic Hydrocarbons in Commercial Squids From Different Geographical Origins: Levels and Risks for Human Consumption. Food Chem. Toxicol. 59, 46–54. doi: 10.1016/j.fct.2013.05.034
Graham W. M., Condon R. H., Carmichael R. H., D’Ambra I., Patterson H. K., Linn L. J., et al. (2010). Oil Carbon Entered the Coastal Planktonic Food Web During the Deepwater Horizon Oil Spill. Environ. Res. Lett. 5, 045301. doi: 10.1088/1748-9326/5/4/045301
Grimes C. B., Finucane J. H. (1991). Spatial Distribution and Abundance of Larval and Juvenile fish, Chlorophyll and Macrozooplankton Around the Mississippi River Discharge Plume, and the Role of the Plume in Fish Recruitment. Mar. Ecol. Prog. Ser. 75, 109–119. doi: 10.3354/meps075109
Grosell M., Pasparakis C. (2021). Physiological Responses of Fish to Oil Spills. Ann. Rev. Mar. Sci. 13, 137–160. doi: 10.1146/annurev-marine-040120-094802
Hildebrand J. A., Baumann-Pickering S., Frasier K. E., Tricky J. S., Merkens K. P., Wiggins S. M., et al. (2015). Passive Acoustic Monitoring of Beaked Whale Densities in the Gulf of Mexico. Sci. Rep. 5, 6343. doi: 10.1038/srep16343
Hildebrand J. A., Frasier K. E., Baumann-Pickering S., Wiggins S. M., Merkens K. P., Garrison L. P., et al. (2019). Assessing Seasonality and Density From Passive Acoustic Monitoring of Signals Presumed to Be From Pygmy and Dwarf Sperm Whales in the Gulf of Mexico. Front. Mar. Sci. 6. doi: 10.3389/fmars.2019.00066
Hopkins T. L. (1982). The Vertical Distribution of Zooplankton in the Eastern Gulf of Mexico. Deep Sea Res. 29, 1069–1083. doi: 10.1016/0198-0149(82)90028-0
Hopkins T. L., Flock M. E., Gartner J. V., Torres J. J. (1994). Structure and Trophic Ecology of a Low Latitude Midwater Decapod and Mysid Assemblage. Mar. Ecol. Prog. Ser. 109, 143–156. doi: 10.3354/meps109143
Hopkins T. L., Sutton T. T. (1998). Midwater Fishes and Shrimps as Competitors in Low Latitude Oligotrophic Ecosystems. Mar. Ecol. Prog. Ser. 164, 37–45. doi: 10.3354/meps164037
Hopkins T. L., Sutton T. T., Lancraft T. M. (1996). Trophic Structure and Predation Impact of a Low Latitude Midwater Fish Community. Prog. Oceanogr. 38, 205–239. doi: 10.1016/S0079-6611(97)00003-7
Houde E. H. (2016). “Recruitment Variability: Implications for Assessment and Management,” in Fish Reproductive Biology: Implications for Assessment and Management. Eds. Jakobson T., Fogarty M. J., Megrey B. A., Moksness E. (Chichester, UK: John Wiley & Sons), 98–187.
Hoving H. J. T., Haddock S. D. H. (2017). The Giant Deep-Sea Octopus Haliphron Atlanticus Forages on Gelatinous Fauna. Sci. Rep. 7, 44952. doi: 10.1038/srep44952
Hoving H. J. T., Robison B. (2017). The Pace of Life in Deep-Dwelling Squids. Deep Sea Res. Pt I 126, 40–49. doi: 10.1016/j.dsr.2017.05.005
Hsieh P. A. (2010) Computer Simulation of Reservoir Depletion and Oil Flow From the Macondo Well Following the Deepwater Horizon Blowout. USGS Open-File Report 2010-1266. Available at: https://pubs.er.usgs.gov/publication/ofr20101266.
Hu C., Muller-Karger F. E., Biggs D. C., Carder K. L., Nababan B., Nadeau D., et al. (2003). Comparison of Ship and Satellite Bio-Optical Measurements on the Continental Margin of the NE Gulf of Mexico. Intl J. Rem. Sens. 24, 2597–2612. doi: 10.1080/0143116031000067007
Hu C., Nelson J. R., Johns E., Chen Z., Weisberg R. H., Muller-Karger F. E. (2005). Mississippi River Water in the Florida Straits and in the Gulf Stream Off Georgia in Summer 2004. Geophys. Res. Lett. 32 (10), L14606. doi: 10.1029/2005GL022942
Hu C., Weisberg R. H., Liu Y., Zheng L., Daly K., English D., et al. (2011). Did the Northeastern Gulf of Mexico Become Greener After the Deepwater Horizon Oil Spill? Geophys. Res. Lett. 38, L09601. doi: 10.1029/2011GL047184
Incardona J. P., Gardner L. D., Linbo T. L., Brown T. L., Esbaugh A. J., Mager E. M., et al. (2014). Deepwater Horizon Crude Oil Impacts the Developing Hearts of Large Predatory Pelagic Fish. Proc. Natl. Acad. Sci. U.S.A. 111, e1510–e1518. doi: 10.1073/pnas.1320950111
Iverson R. L., Hopkins T. L. (1981). “A Summary of Knowledge of Plankton Production in the Gulf of Mexico: Recent Phytoplankton and Zooplankton Research,” in Proceedings of a Symposium of Environmental Research Needs in the Gulf of Mexico (GOMEX), NOAA/ADML, Miami, FL, 30 September – 3 October 1979. (Miami, USA: National Oceanic and Atmospheric Administration) 147–211.
Jackson G. D., O’Dor R. K. (2001). Time, Space, and the Ecophysiology of Squid Growth, Life in the Fast Lane. Vie Milieu 51, 205–215.
Jayalakshmi K. J., Jasmine P., Muraleedharan K. R., Prabhakaran M. P., Habeebrehman H., Jacob J., et al. (2011). Aggregation of Euphausia Sibogae During Summer Monsoon Along the Southwest Coast of India. J. Mar. Biol. 2011, 1–12. doi: 10.1155/2011/945734
Jochens A., Biggs D., Benoit-Bird K., Engelhaupt D., Gordon J., Hu C., et al. (2008). Sperm Whale Seismic Study in the Gulf of Mexico: Synthesis Report (OCS Study MMS 2008-006, U.S (New Orleans, LA: Dept. of Interior, Minerals Management Service, Gulf of Mexico OCS Region), 341.
Jochens A. E., DiMarco S. F. (2008). Physical Oceanographic Conditions in the Deepwater Gulf of Mexico in Summer 2000-2002. Deep Sea Res. Pt II 55, 2541–2554. doi: 10.1016/j.dsr2.2008.07.003
Johnston M., Milligan R., Easson C., DeRada S., Penta B., Sutton T. (2019). An Empirically-Validated Method for Characterizing Pelagic Habitats in the Gulf of Mexico Using Ocean Model Data. Limnol Oceanogr. Methods 17, 362–375. doi: 10.1002/lom3.10319
Jolliff J. K., Smith T. A., Ladner S., Arnone R. A. (2014). Simulating Surface Oil Transport During the Deepwater Horizon Oil Spill: Experiments With the BioCast System. Ocean Model. 75, 84–99. doi: 10.1016/j.ocemod.2014.01.004
Judkins H. (2009). Cephalopods of the Broad Caribbean: Distribution, Abundance and Ecological Importance. [Dissertation] (St. Petersburg (FL: University of South Florida).
Judkins H., Arbuckle S., Vecchione M., Garrison L., Martinez A. (2013). Cephalopods in the Potential Prey Field of Sperm Whales (Physeter Macrocephalus) in the Northern Gulf of Mexico. J. Nat. Hist. 49, 1267–1280 doi: 10.1080/00222933.2013.802045
Judkins H., Lindgren A., Villeneuva R., Clark K., Vecchione M. (2020). A Description of Three New Bathyteuthid Species in the Northern Gulf of Mexico. Bull. Mar. Sci. 96, 281–295. doi: 10.5343/bms.2019.0051
Judkins H., Rosario K., Vecchione M. (2016b). Morphological and Molecular Evidence of Heteroteuthis Dagamensis in the Gulf of Mexico. Bull. Mar. Sci. 92, 51–57. doi: 10.5343/bms.2015.1061
Judkins H., Vecchione M. (2020). Vertical Distribution Patterns of Cephalopods in the Northern Gulf of Mexico. Front. Mar. Sci. 7. doi: 10.3389/fmars.2020.00047
Judkins H., Vecchione M., Cook A., Sutton T. T. (2016a). Diversity of Midwater Cephalopods in the Northern Gulf of Mexico: Comparison of Two Collecting Methods. Mar. Biodivers. 47, 647–657. doi: 10.1007/s12526-016-0597-8
Judkins H., Vecchione M., Roper C. F. E. (2009). Cephalopods (Mollusca: Cephalopoda) of the Gulf of Mexico. Gulf of Mexico Origin, Waters, and Biota Biodiversity Vol. 1. Eds. Felder D., Camp D. A. (Texas: Texas A&M University Press, College Station), 701–710. Ch. 34.
Judkins H., Vecchione M., Torres J., Roper C. F. E. (2010). Cephalopod Species Richness in the Wider Caribbean Region. ICES J. Mar. Sci. 67, 1392–1400. doi: 10.1093/icesjms/fsq092
Kitchens L. L., Rooker J. R. (2014). Habitat Associations of Dolphinfish in the Gulf of Mexico. Fish. Oceanogr. 23, 460–471. doi: 10.1111/fog.12081
Knap A., Turner N. R., Bera G., Renegar D. A., Frank T., Sericano J., et al. (2017). Short-Term Toxicity of 1-Methylnaphthalene to Americamysis Bahia and 5 Deep-Sea Crustaceans. Env. Tox. Chem. 36, 3415–3423. doi: 10.1002/etc.3926
Lahaye V., Bustamante P., Dabin W., Van Canneyt O., Dhermain F., Cesarini C., et al. (2006). New Insights From Age Determination on Toxic Element Accumulation in Striped and Bottlenose Dolphins From Atlantic and Mediterranean Waters. Mar. Pollut. Bull. 52, 1219–1230. doi: 10.1016/j.marpolbul.2006.02.020
Law R. J., Whinnett J. A. (1992). Polycyclic Aromatic Hydrocarbons in Muscle Tissue of Harbour Porpoises (Phocoena Phocoena) From UK Waters. Mar. Pollut. Bull. 24, 550–553. doi: 10.1016/0025-326X(92)90707-D
Lee R. F., Anderson J. W. (2005). Significance of Cytochrome P450 System Responses and Levels of Bile Fluorescent Aromatic Compounds in Marine Wildlife Following Oil Spills. Mar. Pollut. Bull. 50, 705–723. doi: 10.1016/j.marpolbul.2005.04.036
Lee R. F., Koster M., Paffenhoffer G. A. (2012). Ingestion and Defecation of Dispersed Oil Droplets by Pelagic Tunicates. J. Plankton Res. 34, 1058–1063. doi: 10.1093/plankt/fbs065
Lee C. E., Remfert J. L., Opgenorth T., Lee K. M., Stanford S., Connolly J. W., et al. (2017). Evolutionary Response to Crude Oil From the Deepwater Horizon Oil Spill by the Copepod Eurytemora Affinis. Evol. Appl. 10, 813–828. doi: 10.1111/eva.12502
Leite T. S., Batista B. T., Lima F. D., Barbosa J. C., Mather J. (2016). Geographic Variability of Octopus Insularis Diet: From Oceanic Island to Continental Populations. Aquat. Biol. 25, 17–27. doi: 10.3354/ab00655
Lewison R. L., Crowder L. B., Wallace B. P., Moore J. E., Cox T., Zydelis R., et al. (2014). Global Patterns of Marine Mammal, Seabird, and Sea Turtle Bycatch Reveal Taxa-Specific and Cumulative Megafauna Hotspots. P. Nat. Acad. Sci. U.S.A. 111, 5271–5276. doi: 10.1073/pnas.1318960111
Li Y., Hu C., Quigg A., Gao H. (2019). Potential Influence of the Deepwater Horizon Oil Spill on Phytoplankton Primary Productivity in the Northern Gulf of Mexico. Environ. Res. Lett. 14, 094018. doi: 10.1088/1748-9326/ab3735
Lindegren M., Checkley D. M. Jr., Ohman M. D., Koslow J. A., Goericke R. (2016). Resilience and Stability of a Pelagic Marine Ecosystem. Proc. R. Soc B Biol. Sci. 283, 20151931. doi: 10.1098/rspb.2015.1931
Lipka D. (1975). The Systematics and Zoogeography of Cephalopods From the Gulf of Mexico (Texas A&M: Texas A&M University, College Station, United States). Dissertation.
Litz J. A., Baran M. A., Bowen-Stevens S. R., Carmichael R. H., Colegrove K. M., Garrison L. P., et al. (2014). Review of Historical Unusual Mortality Events (UMEs) in the Gulf of Mexic- 2009): Providing Context for the Multi-Year Northern Gulf of Mexico Cetacean UME Declared in 2010. Dis. Aquat. Organ. 112, 161–175. doi: 10.3354/dao02807
Logan M., Toppin R., Smith S., Galuardi B., Porter J., Lutcavage M. (2013). Contribution of Cephalopod Prey to the Diet of Large Pelagic Fish Predators in the Central North Atlantic Ocean. Deep Sea Res. Pt II 95, 74–82. doi: 10.1016/j.dsr2.2012.06.003
Longhurst A. R., Harrison W. G. (1989). The Biological Pump: Profiles of Plankton and Consumption in the Upper Ocean. Prog. Oceanogr. 22, 46–123. doi: 10.1016/0079-6611(89)90010-4
Lyczkowski-Shultz J., Hanisko D. S., Sulak K. J., Konieczna M., Bond P. J. (2013). Characterization of Ichthyoplankton in the Northeastern Gulf of Mexico From SEAMAP Plankton Survey-1999. Gulf Caribb. Res. 25, 43–98. doi: 10.18785/gcr.2501.05
Mager E. M., Esbaugh A. J., Stieglitz J. D., Hoenig R., Bodinier C., Incardona J. P., et al. (2014). Acute Embryonic or Juvenile Exposure to Deepwater Horizon Crude Oil Impairs the Swimming Performance of Mahi-Mahi (Coryphaena Hippurus). Environ. Sci. Technol. 48, 7053–7061. doi: 10.1021/es501628k
Mager E. M., Pasparakis C., Schlenker L. S., Yao Z., Bodinier C., Stieglitz J. D., et al. (2017). Assessment of Early Life Stage Mahi-Mahi Windows of Sensitivity During Acute Exposures to Deepwater Horizon Crude Oil. Environ. Sci. Technol. 36, 1887–1895. doi: 10.1002/etc.3713
Marks A. D., Kerstetter D. W., Wyanski D. M., Sutton T. T. (2020). Reproductive Ecology of Dragonfishes (Stomiiformes: Stomiidae) in the Gulf of Mexico. Front. Mar. Sci. 7, 101. doi: 10.3389/fmars.2020.00101
Marshak A. R., Link J. S. (2021). Primary Production Ultimately Limits Fisheries Economic Performance. Sci. Rep. 11, 12154. doi: 10.1038/s41598-021-91599-0
Matkin C. O., Saulifis E. L., Ellis G. M., Olesiuk P., Rice S. D. (2008). Ongoing Population-Level Impacts on Killer Whales Orcinus Orca Following the ’Exxon Valdez’ Oil Spill in Prince William Sound, Alaska. Mar. Ecol. Prog. Ser. 356, 269–281. doi: 10.3354/meps07273
Maul G. A. (1977). The Annual Cycle of the Gulf Loop Current Part I: Observations During a One-Year Time Series. J. Mar. Res. 35, 29–47.
McEachran J., Fechhelm J. D. (1998). Fishes of the Gulf of Mexico, Vol. 1: Myxiniformes to Gasterosteiformes (Austin, Texas: University of Texas Press).
McNutt M. K., Camilli R., Crone T. J., Guthrie G. D., Hsieh P., Ryerson T. B., et al. (2012). Review of Flow Rate Estimates of the Deepwater Horizon Oil Spill. Proc. Natl. Acad. Sci. U.S.A. 109, 20260–20267. doi: 10.1073/pnas.1112139108
Meinert C. R., Clausen-Sparks C., Cornic M., Sutton T. T., Rooker J. R. (2020). Taxonomic Richness and Diversity of Larval Fish Assemblages in the Oceanic Gulf of Mexico: Links to Oceanographic Conditions. Front. Mar. Sci. 7. doi: 10.3389/fmars.2020.00579
Milligan R. J., Sutton T. T. (2020). Dispersion Overrides Environmental Variability as a Primary Driver of the Horizontal Assemblage Structure of the Mesopelagic Fish Family Myctophidae in the Northern Gulf of Mexico. Front. Mar. Sci. 7. doi: 10.3389/fmars.2020.00015
Moore J. A., Fenolio D. B., Cook A. B., Sutton T. T. (2020). Hiding in Plain Sight: Elopomorph Larvae are Important Contributors to Fish Biodiversity in a Low-Latitude Oceanic Ecosystem. Front. Mar. Sci. 7. doi: 10.3389/fmars.2020.00169
Morano J. L., Tielens J. T., Muirhead C. A., Estabrook B. J., Sullivan P. J., Dugan P. J., et al. (2020). Seasonal Movements of Gulf of Mexico Sperm Whales Following the Deepwater Horizon Oil Spill and the Limitations of Impact Assessments. Mar. Pollut. Bull. 161, 111627. doi: 10.1016/j.marpolbul.2020.111627
Muhling B. A., Reglero P., Ciannelli L., Alvarez-Berastegui D., Alemany F., Lamkin J. T., et al. (2013). Comparison Between Environmental Characteristics of Larval Bluefin Tuna Thunnus Thynnus Habitat in the Gulf of Mexico and Western Mediterranean Sea. Mar. Ecol. Prog. Ser. 486, 257–276. doi: 10.3354/meps10397
Muhling B. A., Roffer M. A., Lamkin J. T., Ingram G. W. Jr., Upton M. A., Gawlikowski G., et al. (2012). Overlap Between Atlantic Bluefin Tuna Spawning Grounds and Observed Deepwater Horizon Surface Oil in the Northern Gulf of Mexico. Mar. Pollut. Bull. 64, 679–687. doi: 10.1016/j.marpolbul.2012.01.034
Muller-Karger F. E., Smith J. P., Werner S., Chen R., Roffer M., Liu Y., et al. (2015). Natural Variability of Surface Oceanographic Conditions in the Offshore Gulf of Mexico. Prog. Oceanogr. 134, 54–76. doi: 10.1016/j.pocean.2014.12.007
Mullin K. D., Hoggard W. (2000). “Visual Surveys of Cetaceans and Sea Turtles From Aircraft and Ships,” in Cetaceans, Sea Turtles and Seabirds in the Northern Gulf of Mexico: Distribution, Abundance and Habitat Associations, Vol. Ii. Eds. Davis R. W., Evans W. E., Wursig D. (New Orleans, LA: Tech. Rep. OCS Study MMS 96-0027. USGS/BRD/CR-1999-0006, U.S. Dept. of Interior, Minerals Management Service, Gulf of Mexico OCS Region), 111–172.
Murawski S. A., Ainsworth C. H., Gilbert S., Hollander D. J., Paris C. B., Schlüter M., et al. (2020). Scenarios and Responses to Future Deep Oil Spills - Fighting the Next War (Charm, Switzerland: Springer Nature). doi: 10.1007/978-3-030-12963-7
Murawski S. A., Kilborn J. P., Bejarano A. C., Chagaris D., Donaldson D., Hernandez F. J. Jr., et al. (2021). A Synthesis of Deepwater Horizon Impacts on Coastal and Nearshore Living Marine Resources. Front. Mar. Sci. 7, 594862. doi: 10.3389/fmars.2020.594862
National Academies of Science, Engineering, and Medicine [NASEM] (2020). The Use of Dispersants in Marine Oil Spill Response (Washington, DC: The National Academies Press).
Nixon Z., Zengel S., Baker M., Steinhoff M., Fricano G., Rouhani S., et al. (2016). Shoreline Oiling From the Deepwater Horizon Oil Spill. Mar. Poll. Bull. 107, 170–178. doi: 10.1016/j.marpolbul.2016.04.003
O’Connor B. S., Muller-Karger F. E., Nero R. W., Hu C., Peebles E. B. (2016). The Role of Mississippi River Discharge in Offshore Phytoplankton Blooming in the Northeastern Gulf of Mexico During August 2010. Remote Sens. Environ. 173, 133–144. doi: 10.1016/j.rse.2015.11.004
O’Dor R. K., Webber D. M. (1986). The Constraint on Cephalopods: Why Squid Aren’t Fish. Can. J. Zool. 64, 1591–1605. doi: 10.1139/z86-241
Okolodkov Y. B. (2003). A Review of Russian Plankton Research in the Gulf of Mexico and the Caribbean Sea in the 1960s-1980s. Hidrobiologica 13, 207–221.
Olmos-Pérez L., Roura Á., Pierce G. J., Boyer S., González Á. (2017). Diet Composition and Variability of Wild Octopus Vulgaris and Alloteuthis Media (Cephalopoda) Paralarvae: A Metagenomic Approach. Front. Physiol. 8. doi: 10.3389/fphys.2017.00321
Ortner P. B., Hill L. C., Cummings S. R. (1989). Zooplankton Community Structure and Copepod Species Composition in the Northern Gulf of Mexico. Cont. Shelf Res. 9, 387–402. doi: 10.1016/0278-4343(89)90040-X
Ozhan K., Bargu S. (2014). Distinct Responses of Gulf of Mexico Phytoplankton Communities to Crude Oil and the Dispersant Corexit® Ec9500A Under Different Nutrient Regimes. Ecotoxicology 23, 370–384. doi: 10.1007/s10646-014-1195-9
Ozhan K., Parsons M. L., Bargu S. (2014). How Were Phytoplankton Affected by the Deepwater Horizon Oil Spill? BioScience 64, 829–836. doi: 10.1093/biosci/biu117
Parsons M., Morrison W., Rabalais N., Turner R., Tyre K. (2015). Phytoplankton and the Macondo Oil Spill: A Comparison of the 2010 Phytoplankton Assemblage to Baseline Conditions on the Louisiana Shelf. Environ. Pollut. 207, 152–160. doi: 10.1016/j.envpol.2015.09.019
Pasparakis C., Esbaugh A. J., Burggren W., Grosell M. (2019). Physiological Impacts of Deepwater Horizon Oil on Fish. Comp. Biochem. Phys. C 224, 108558. doi: 10.1016/j.cbpc.2019.06.002
Pasparakis C., Mager E. M., Stieglitz J. D., Benetti D., Grosell M. (2016). Effects of Deepwater Horizon Crude Oil Exposure, Temperature and Developmental Stage on Oxygen Consumption of Embryonic and Larval Mahi-Mahi (Coryphaena Hippurus). Aquat. Toxicol. 181, 113–123. doi: 10.1016/j.aquatox.2016.10.022
Passarella K. C. (1990). Oceanic Cephalopod Assemblage in the Eastern Gulf of Mexico. Department of Marine Science (St. Petersburg, Fl: University of South Florida).
Passarella K. C., Hopkins T. L. (1991). Species Composition and Food Habits of the Micronektonic Cephalopod Assemblage in the Eastern Gulf of Mexico. Bull. Mar. Sci; 49 (1-2), 638–659.
Paul J. H., Hollander D., Coble P., Daly K. L., Murasko S., English D., et al. (2013). Toxicity and Mutagenicity of Gulf of Mexico Waters During and After the Deepwater Horizon Oil Spill. Env. Sci. Technol. 47, 9651–9659. doi: 10.1021/es401761h
Payne J. R., Driskell W. B. (2015) 2010 DWH Offshore Water Column Samples — Forensic Assessments and Oil Exposures. U.S. Dept. Of Interior, Deepwater Horizon Response & Restoration, Admin. Record. Available at: www.doi.gov/deepwaterhorizon/adminrecord.
Pearcy W. G., Laurs R. M. (1966). Vertical Migration and Distribution of Mesopelagic Fishes Off Oregon. Deep-Sea Res. 13 (2), 153–165. doi: 10.1016/0011-7471(66)91096-5
Peiffer R. F., Cohen J. H. (2015). Lethal and Sublethal Effects of Oil, Chemical Dispersant, and Dispersed Oil on the Ctenophore Mnemiopsis Leidyi. Aquat. Biol. 23, 237–250. doi: 10.3354/ab00625
Perrichon P., Mager E. M., Pasparakis C., Stieglitz J. D., Benetti D. D., Grosell M., et al. (2018). Combined Effects of Elevated Temperature and Deepwater Horizon Oil Exposure on the Cardiac Performance of Larval Mahi-Mahi, Coryphaena Hippurus. PloS One 13, e0203949. doi: 10.1371/journal.pone.0203949
Perrin W. F., Reilly S. B. (1984). Reproductive Parameters of Dolphins and Small Whales of the Family Delphinidae. Rep. Int. Whal. Commn. 6, 97–133.
Priede I. G. (2017). Deep-Sea Fishes: Biology, Diversity, Ecology and Fisheries (Cambridge, UK: Cambridge University Press).
Pritt J. J., Roseman E. F., O’Brien T. P. (2014). Mechanisms Driving Recruitment Variability in Fish: Comparisons Between the Laurentian Great Lakes and Marine Systems. ICES J. Mar. Sci. 71, 2252–2267. doi: 10.1093/icesjms/fsu080
Pruzinsky N. M., Milligan R. J., Sutton T. T. (2020). Pelagic Habitat Partitioning of Late-Larval and Juvenile Tunas in the Oceanic Gulf of Mexico. Front. Mar. Sci. 7. doi: 10.3389/fmars.2020.00257
Quigg A., Parsons M., Bargu S., Ozhan K., Daly K. L., Chakraborty S., et al. (2021). Marine Phytoplankton Responses to Oil and Dispersant Exposures: Knowledge Gained Since the Deepwater Horizon Oil Spill. Mar. Poll. Bull. 164, 112074. doi: 10.1016/j.marpolbul.2021.112074
Quintana-Rizzo E., Torres J. J., Ross S. W., Romero I., Watson K., Goddard E., et al. (2015). δ13c and δ15 N in Deep-Living Fishes and Shrimps After the Deepwater Horizon Oil Spill, Gulf of Mexico. Mar. Pollut. Bull. 94, 241–250. doi: 10.1016/j.marpolbul.2015.02.002
Randall L. L., Smith B. L., Cowan J. H., Rooker J. R. (2015). Habitat Characteristics of Bluntnose Flyingfish Prognichthys Occidentalis (Actinopeterygii, Exocoetidae), Across Mesoscale Features in the Gulf of Mexico. Hydrobiologia 749, 97–111. doi: 10.1007/s10750-014-2151-7
Reeves R. R., Lund J. N., Smith T. D., Josephson E. A. (2011). Insights From Whaling Logbooks on Whales, Dolphins, and Whaling in the Gulf of Mexico. Gulf Mex. Sci. 9, 4. doi: 10.18785/goms.2901.04
Richardson D. E., Marancik K. E., Guyon J. R., Lutcavage M. E., Galuardi B., Lam C. H., et al. (2016). Discovery of a Spawning Ground Reveals Diverse Migration Strategies of Atlantic Bluefin Tuna. (Thunnus Thynnus). Proc. Nat. Acad. Sci. U.S.A. 113, 3299–3304. doi: 10.1073/pnas.1525636113
Robertson K. M., Chivers S. J. (1998). Prey Occurrence in Pantropical Spotted Dolphins, Stenella Attenuata, From the Eastern Tropical Pacific. Oceanogr. Lit. Rev. 1, 125.
Robison B. H. (2004). Deep Pelagic Biology. J. Exp. Mar. Biol. Ecol. 300, 253–272. doi: 10.1016/j.jembe.2004.01.012
Roe H. S. J. (1984). The Diel Migrations and Distributions Within a Mesopelagic Community in the North East Atlantic. 2. Vertical Migrations and Feeding of Mysids and Decapod Crustacea. Prog. Oceanogr. 13, 269–318. doi: 10.1016/0079-6611(84)90011-9
Romero I., Judkins H., Vecchione M. (2020). Temporal Variability of Polycyclic Aromatic Hydrocarbons in Deep-Sea Cephalopods of the Northern Gulf of Mexico. Front. Mar. Sci. 7. doi: 10.3389/fmars.2020.0054
Romero I. C., Schwing P. T., Brooks G. R., Larson R. A., Hastings D. W., Flower B. P., et al. (2015). Hydrocarbons in Deep-Sea Sediments Following the 2010 Deepwater Horizon Blowout in the Northeast Gulf of Mexico. PloS One 10, 1–23. doi: 10.1371/journal.pone.0128371
Romero I. C., Sutton T., Carr B., Quintana-Rizzo E., Ross S. W., Hollander D. J., et al. (2018). Decadal Assessment of Polycyclic Aromatic Hydrocarbons in Mesopelagic Fishes From the Gulf of Mexico Reveals Exposure to Oil-Derived Sources. Environ. Sci. Technol. 52, 10985–10996. doi: 10.1021/acs.est.8b02243
Romero I. C., Toro-Farmer G., Diercks A.-R., Schwing P., Muller-Karger F., Murawski S., et al. (2017). Large-Scale Deposition of Weathered Oil in the Gulf of Mexico Following a Deep-Water Oil Spill. Env. Poll. 228, 179–189. doi: 10.1016/j.envpol.2017.05.0190269-749
Rooker J. R., Kitchens L. L., Dance M. A., Wells R. J. D., Falterman B., Cornic M. (2013). Spatial, Temporal, and Habitat-Related Variation in Abundance of Pelagic Fishes in the Gulf of Mexico: Potential Implications of Deepwater Horizon Oil Spill. PloS One 8, e76080. doi: 10.1371/journal.pone.0076080
Rooker J. R., Simms J. R., Wells R. J. D., Holt S. A., Holt G. J., Graves J. E., et al. (2012). Distribution and Habitat Associations of Billfish and Swordfish Larvae Across Mesoscale Features in the Gulf of Mexico. PloS One 7, e34180. doi: 10.1371/journal.pone.0034180
Rosel P. E., Wilcox L. A., Yamada T. K., Mullin K. D. (2021). A New Species of Baleen Whale (Balaenoptera) From the Gulf of Mexico, With a Review of Its Geographic Distribution. Mar. Mammal Sci. 37 (2), 577–610. doi: 10.1111/mms.12776
Ross S. W., Quattrini A. M., Roa-Varón A. Y., McClain J. P. (2010). Species Composition and Distributions of Mesopelagic Fishes Over the Slope of the North-Central Gulf of Mexico. Deep Sea Res. Pt II 57, 1926–1956. doi: 10.1016/j.dsr2.2010.05.008
Ryerson T. B., Camilli R., Kessler J. D., Kujawinski E. B., Reddy C. M., Valentine D. L., et al. (2012). Chemical Data Quantify Deepwater Horizon Hydrocarbon Flow Rate and Environmental Distribution. Proc. Natl. Acad. Sci. U.S.A. 109, 20246–20253. doi: 10.1073/pnas.1110564109
Schiller R. V., Kourafalou V. H., Hogan P., Walker N. D. (2011). The Dynamics of the Mississippi River Plume: Impact of Topography, Wind and Offshore Forcing on the Fate of Plume Waters. J. Geophys. Res. 116, c06029. doi: 10.1029/2010JC006883
Schramm M. J. (2007). Tiny Krill: Giants in Marine Food Chain (Silver Spring, USA: NOAA National Marine Sanctuary Program). Available at: http://sanctuaries.noaa.gov/news/pdfs/sanctuarywatch/sw8_3.pdf.
Schwacke L. H., Smith C. R., Townsend F. I., Wells R. S., Hart L. B., Balmer B. C., et al. (2013). Health of Common Bottlenose Dolphins (Tursiops Truncatus) in Barataria Bay, Louisiana, Following the Deepwater Horizon Oil Spill. Environ. Sci. Technol. 48, 93–103. doi: 10.1021/es403610f
Schwacke L. H., Thomas L., Wells R. S., McFee W. E., Hohn A. A., Mullin K. D., et al. (2017). Quantifying Injury to Common Bottlenose Dolphins From the Deepwater Horizon Oil Spill Using an Age-, Sex-and Class-Structured Population Model. Endanger. Species Res. 33, 265–279. doi: 10.3354/esr00777
Schwing P. T., Montagna P. A., Joye S. B., Paris C. B., Cordes E. E., McClain C. R., et al. (2020). A Synthesis of Deep Benthic Faunal Impacts and Resilience Following the Deepwater Horizon Oil Spill. Front. Mar. Sci. 7, 560012. doi: 10.3389/fmars.2020.560012
Schwing P. T., Romero I. C., Brooks G. R., Hastings D. W., Larson R. A., Hollander D. J. (2015). A Decline in Benthic Foraminifera Following the Deepwater Horizon Event in the Northeastern Gulf of Mexico. PloS One 10, e0120565. doi: 10.1371/journal.pone.0120565
Semedo M., Oliveira M., Gomes F., Reis-Henriques M. A., Delerue-Matos C., Moralis S., et al. (2014). Seasonal Patterns of Polycyclic Aromatic Hydrocarbons in Digestive Gland and Arm of Octopus (Octopus Vulgaris) From the Northwestern Atlantic. Sci. Total Environ. 481, 488–497. doi: 10.1016/j.scitotenv.2014.02.088
Shearer J. M., Quick N. J., Cioffi W. R., Baird R. W., Webster D. L., Foley H. J., et al. (2019). Diving Behaviour of Cuvier’s Beaked Whales (Ziphius Cavirostris) Off Cape Hatteras, North Carolina. R. Soc Open Sci. 6, 181728. doi: 10.1098/rsos.181728
Sidorovskaia N., Li K., Tiemann C., Ackleh A., Tang T. (2016). Long-Term Spatially Distributed Observations of Deep Diving Marine Mammals in the Northern Gulf of Mexico Using Passive Acoustic Monitoring. J. Acoust. Soc Am. 140, 3073–3073. doi: 10.1121/1.4969575
Smith C. R., Rowles T. K., Hart L. B., Townsend F. I., Wells R. S., Zolman E. S., et al. (2017). Slow Recovery of Barataria Bay Dolphin Health Following the Deepwater Horizon Oil Spil-2014), With Evidence of Persistent Lung Disease and Impaired Stress Response. Endanger. Species Res. 33, 127–142. doi: 10.3354/esr00778
Smultea M. A., Wursig B. (1995). Behavioral Reactions of Bottlenose Dolphins to the Mega Borg Oil Spill, Gulf of Mexico 1990. Aquat. Mamm. 21, 171–181.
Solsona Berga A. (2019). Advancement of Methods for Passive Acoustic Monitoring : A Framework for the Study of Deep-Diving Cetacean. [Tesis Doctoral Dr. En Xaraxa] (Barcelona (España: Universitat Politècnica de Catalunya). Available at: http://hdl.handle.net/2117/129269.
Sturges W., Evans J. C. (1983). On the Variability of the Loop Current in the Gulf of Mexico. J. Mar. Res. 41, 639–653. doi: 10.1357/002224083788520487
Sutton T. T. (2013). Vertical Ecology of the Pelagic Ocean: Classical Patterns and New Perspectives. J. Fish Biol. 83, 1508–1527. doi: 10.1111/jfb.12263
Sutton T. T., Clark M. R., Dunn D. C., Halpin P. N., Rogers A. D., Guinotte J., et al. (2017a). A Global Biogeographic Classification of the Mesopelagic Zone. Deep Sea Res. Pt I 126, 85–102. doi: 10.1016/j.dsr.2017.05.006
Sutton T. T., Cook A., Moore J. A., Frank T., Judkins H., Vecchione M., et al. (2017b). Inventory of Gulf Oceanic Fauna Data Including Species, Weight, and Measurements. Meg Skansi Cruises From Jan. 25 – Sept. 30, 2011 in the Northern Gulf of Mexico (Distributed by: Gulf of Mexico Research Initiative Information and Data Cooperative (GRIIDC)) (Corpus Christi, TX: Harte Research Institute, Texas A&M University). doi: 10.7266/N7VX0DK2
Sutton T. T., Cook A., Moore J. A., Frank T., Judkins H., Vecchione M., et al. (2017c). Inventory of Gulf Oceanic Fauna Data Including Species, Weight, and Measurements, Pisces Cruises From Dec. 1 2010 – Sept. 29, 2011 in the Northern Gulf of Mexico (Distributed by: Gulf of Mexico Research Initiative Information and Data Cooperative (GRIIDC)) (Corpus Christi, TX: Harte Research Institute, Texas A&M University). doi: 10.7266/N7R49NTN
Sutton T. T., Frank F., Judkins H., Romero I. C. (2020). “As Gulf Oil Extraction Goes Deeper, Who Is at Risk? Community Structure, Distribution, and Connectivity of the Deep-Pelagic Fauna,” in Scenarios and Responses to Future Deep Oil Spills - Fighting the Next War. Eds. Murawski S. A., Hollander D., Ainsworth C., Gilbert S., Paris C. B., Schlüter M., Wetzel D. (Cham, Switzerland: Springer Nature), 403–418. doi: 10.1007/978-3-030-12963-7_24
Sutton T. T., Hopkins T. L. (1996a). The Species Composition, Abundance and Vertical Distribution of the Stomiid (Pisces: Stomiiformes) Fish Assemblage of the Gulf of Mexico. Bull. Mar. Sci. 59 (3), 530–542.
Sutton T. T., Hopkins T. L. (1996b). Trophic Ecology of the Stomiid (Pisces, Stomiidae) Assemblage of the Eastern Gulf of Mexico: Strategies, Selectivity and Impact of a Mesopelagic Top Predator Group. Mar. Biol. 127, 179–192. doi: 10.1007/BF00942102
Sutton T. T., Wiebe P. H., Madin L., Bucklin A. (2010). Diversity and Community Structure of Pelagic Fishes to 5000 M Depth in the Sargasso Sea. Deep Sea Res. Pt II 57, 2220–2233. doi: 10.1016/j.dsr2.2010.09.024
Takeshita R., Sullivan L., Smith C., Collier T., Hall A., Brosnan T., et al. (2017). The Deepwater Horizon Oil Spill Marine Mammal Injury Assessment. Endanger. Species Res. 33, 95–106. doi: 10.3354/esr00808
Timm L. E., Bracken-Grissom H. D., Sosnowski A., Breitbart M., Vecchione M., Judkins H. (2020a). Population Genomics of Three Deep-Sea Cephalopod Species Reveals Connectivity Between the Gulf of Mexico and Northwestern Atlantic Ocean. Deep Sea Res. Pt I 158, 103222. doi: 10.1016/j.dsr.2020.103222
Timm L. E., Isma L. M., Johnston M. W., Bracken-Grissom H. D. (2020b). Comparative Population Genomics and Biophysical Modeling of Shrimp Migration in the Gulf of Mexico Reveals Current-Mediated Connectivity. Front. Mar. Sci. 7. doi: 10.3389/fmars.2020.00019
Tolan J. L. (2020). Temporal and Spatial Trends of Dead Copepods in the Northern Gulf of Mexico in Relation to the Deepwater Horizon Oil Spill. [Master’s Thesis] (Baton Rouge, (LA: Louisiana State University and Agricultural and Mechanical College). doi: 10.13140/RG.2.2.33624.11529
Turner J. T. (1987). Zooplankton Feeding Ecology: Contents of Fecal Pellets of the Copepod Centropages Velificatus From Waters Near the Mouth of the Mississippi River. Biol. Bull. 173, 377–386. doi: 10.2307/1541550
Unger M. A., Harvey E., Vadaas G. G., Vecchione M. (2008). Persistent Pollutants in Nine Species of Deep-Sea Cephalopods. Mar. Poll. Bull. 56, 1486–1512. doi: 10.1016/j.marpolbul.2008.04.018
U.S. District Court (2015) Findings of Facts and Conclusions of Law - Phase 2 Trial. Case 2: 10-Md-02179-Cjb-Ss. Available at: http://www.laed.uscourts.gov/sites/default/files/OilSpill/Orders/1152015FindingsPhaseTwo.pdf.
Vecchione M., Falkenhaug T., Sutton T. T., Cook A. B., Gislason A., Hansen H. Ø., et al. (2015). The Effect of the North Atlantic Subpolar Front as a Boundary in Pelagic Biogeography Decreases With Increasing Depth and Organism Size. Prog. Oceanogr. 138, 105–115. doi: 10.1016/j.pocean.2015.08.006
Venn-Watson S., Garrison L., Litz J., Fougeres E., Mase B., Rappucci G., et al. (2015). Demographic Clusters Identified Within the Northern Gulf of Mexico Common Bottlenose Dolphin (Tursiops Truncatus) Unusual Mortality Event: January 2010-June 2013. PloS One 10, e0117248. doi: 10.1371/journal.pone.0117248
Wade T. L., Sericano J. L., Sweet S. T., Knap A. H., Guinasso N. L. (2016). Spatial and Temporal Distribution of Water Column Total Polycyclic Aromatic Hydrocarbons (PAH) and Total Petroleum Hydrocarbons (TPH) From the Deepwater Horizon (Macondo) Incident. Mar. Pollut. Bull. 103, 286–293. doi: 10.1016/j.marpolbul.2015.12.002
Wang V. H., Zapfe C. R., Hernandez F. J. (2021). Assemblage Structure of Larval Fishes in Epipelagic and Mesopelagic Waters of the Northern Gulf of Mexico. Front. Mar. Sci. 8, 766369. doi: 10.3389/fmars.2021.766369
Waring G. T., Josephson E., Maze-Foley K., Rosel P. E. (2013). U.S. Atlantic and Gulf of Mexico Marine Mammal Stock Assessments—2012. NOAA Tech. Memo. NMFS NE. 219, 598.
Waring G., Josephson E., Maze-Foley K., Rosel P. (2016). U.S. Atlantic and Gulf of Mexico Marine Mammal Stock Assessments—2015. NOAA Tech. Memo. NMFS NE. 238, 501. doi: 10.7289/V57S7KTN02543-01026
Watwood S. L., Miller P. J. O., Johnson M., Madsen P. T., Tyack P. L. (2006). Deep-Diving Foraging Behaviour of Sperm Whales (Physeter macrocephalus). J. Anim. Ecol. 75, 814–825. doi: 10.1111/j.1365-2656.2006.01101.x
Weilgart L. S. (2007). The Impacts of Anthropogenic Ocean Noise on Cetaceans and Implications for Management. Can. J. Zool. 85, 1091–1116. doi: 10.1139/Z07-101
Wells M. J., Clarke A. (1996). Energetics: The Costs of Living and Reproducing for an Individual Cephalopod. Philos. T. R. Soc B 351, 1083–1104. doi: 10.1098/rstb.1996.0095
West K. L., Walker W. A., Baird R. W., Mead J. G., Collins P. W. (2017). Diet of Cuvier’s Beaked Whales Ziphius Cavirostris From the North Pacific and a Comparison With Their Diet World-Wide. Mar. Ecol. Prog. Ser. 574, 227–242. doi: 10.3354/meps12214
White H. K., Hsing P.-Y., Cho W., Shank T. M., Cordes E. E., Quattrini A. M., et al. (2012). Impact of the Deepwater Horizon Oil Spill on a Deep-Water Coral Community in the Gulf of Mexico. Proc. Natl. Acad. Sci. 109, 20303–20308. doi: 10.1073/pnas.1118029109
Williams R., Gero S., Bejder L., Calambokidis J., Kraus S. D., Lusseau D., et al. (2011). Underestimating the Damage: Interpreting Cetacean Carcass Recoveries in the Context of the Deepwater Horizon/BP Incident. Conserv. Lett. 4, 228–233. doi: 10.1111/j.1755-263X.2011.00168.x
Wise C. F., Wise J. T., Wise S. S., Thompson W. D., Wise J. P. Jr., Wise S. J.P. (2014). Chemical Dispersants Used in the Gulf of Mexico Oil Crisis Are Cytotoxic and Genotoxic to Sperm Whale Skin Cells. Aquat. Toxicol. 152, 335–340. doi: 10.1016/j.aquatox.2014.04.020
Wood J. B., O’Dor R. K. (2000). Do Larger Cephalopods Live Longer? Effects of Temperature and Phylogeny on Interspecific Comparisons of Age and Size at Maturity. Mar. Biol. 136, 91–99. doi: 10.1007/s002270050012
Würsig B., Jefferson T. A., Schmidly D. J. (2000). The Marine Mammals of the Gulf of Mexico (College Station, Texas: Texas A&M University Press).
Xavier J. C., Cherel Y., Roberts J., Piatkowski U. (2013). How do Cephalopods Become Available to Seabirds: Can Fish Gut Contents From Tuna Fishing Vessels be a Major Food Source of Deep-Dwelling Cephalopods? ICES J. Mar. Sci. 70, 46–49. doi: 10.1093/icesjms/fss167
Xu E. G., Mager E. M., Grosell M., Hazard E. S., Hardiman G., Schlenk D. (2017). Novel Transcriptome Assembly and Comparative Toxicity Pathway Analysis in Mahi-Mahi (Coryphaena Hippurus) Embryos and Larvae Exposed to Deepwater Horizon Oil. Sci. Rep. 7, 44546. doi: 10.1038/srep44546
Xu E. G., Mager E. M., Grossell M., Pasparakis C., Schlenker L. S., Stieglitz J. D., et al. (2016). Time- and Oil-Dependent Transcriptomic and Physiological Responses to Deepwater Horizon Oil in Mahi-Mahi (Coryphaena hippurus) Embryos and Larvae. Environ. Sci. Technol. 50, 7842–7851. doi: 10.1021/acs.est.6b02205
Yan B., Passow U., Chanton J. P., Nöthig E., Asper V., Sweet J., et al. (2016). Sustained Deposition of Contaminants From the Deepwater Horizon Spill. Proc. Natl. Acad. Sci. 113 (24), E3332–E3340. doi: 10.1073/pnas.1513156113
Zapp Sluis M., Judkins H., Dance M. A., Vecchione M., Cornic M., Sutton T., et al. (2021). Family Composition, Abundance, and Habitat Associations of Squid Paralarvae in the Northern Gulf of Mexico. Deep Sea Res. I. 174, 103572. doi: 10.1016/j.dsr.2021.103572
Keywords: epipelagic, mesopelagic, bathypelagic, oil spill accident, pollution, deep sea
Citation: Sutton TT, Milligan RJ, Daly K, Boswell KM, Cook AB, Cornic M, Frank T, Frasier K, Hahn D, Hernandez F, Hildebrand J, Hu C, Johnston MW, Joye SB, Judkins H, Moore JA, Murawski SA, Pruzinsky NM, Quinlan JA, Remsen A, Robinson KL, Romero IC, Rooker JR, Vecchione M and Wells RJD (2022) The Open-Ocean Gulf of Mexico After Deepwater Horizon: Synthesis of a Decade of Research. Front. Mar. Sci. 9:753391. doi: 10.3389/fmars.2022.753391
Received: 04 August 2021; Accepted: 31 March 2022;
Published: 04 May 2022.
Edited by:
Vanessa F. Fonseca, Center for Marine and Environmental Sciences (MARE), PortugalReviewed by:
Jianfeng Feng, Nankai University, ChinaCopyright © 2022 Sutton, Milligan, Daly, Boswell, Cook, Cornic, Frank, Frasier, Hahn, Hernandez, Hildebrand, Hu, Johnston, Joye, Judkins, Moore, Murawski, Pruzinsky, Quinlan, Remsen, Robinson, Romero, Rooker, Vecchione and Wells. This is an open-access article distributed under the terms of the Creative Commons Attribution License (CC BY). The use, distribution or reproduction in other forums is permitted, provided the original author(s) and the copyright owner(s) are credited and that the original publication in this journal is cited, in accordance with accepted academic practice. No use, distribution or reproduction is permitted which does not comply with these terms.
*Correspondence: Tracey T. Sutton, dHN1dHRvbjFAbm92YS5lZHU=
Disclaimer: All claims expressed in this article are solely those of the authors and do not necessarily represent those of their affiliated organizations, or those of the publisher, the editors and the reviewers. Any product that may be evaluated in this article or claim that may be made by its manufacturer is not guaranteed or endorsed by the publisher.
Research integrity at Frontiers
Learn more about the work of our research integrity team to safeguard the quality of each article we publish.