- 1Institute of Marine Sciences, University of California, Santa Cruz, Santa Cruz, CA, United States
- 2NOAA Southwest Fisheries Science Center, San Diego, CA, United States
- 3Department of Biological Sciences, Thomas More University, Crestview Hills, KY, United States
- 4NOAA Southwest Fisheries Science Center, Monterey, CA, United States
- 5Department of Aquatic Resources, Swedish University of Agricultural Sciences, Uppsala, Sweden
- 6Department of Earth and Environmental Sciences, Jeonbuk National University, Jeonju-si, South Korea
- 7Geophysical Fluid Dynamics Laboratory, National Oceanic and Atmospheric Administration, Princeton, NJ, United States
- 8Hopkins Marine Station, Stanford University, Pacific Grove, CA, United States
North Pacific albacore (Thunnus alalunga) is a commercially important tuna species known to undertake extensive migratory movements between nearshore waters of the California Current and offshore environments in the central Pacific. However, these migration behaviors are highly variable, with some individuals traveling thousands of kilometers within a season, and others largely resident in the southern California Current throughout the year. In this study, we use data from 33 archival-tagged albacore (released between 2003 and 2011) to examine the movements, physiology and ecology of tuna following different migratory pathways. We used direct measurements of body temperature and ambient water temperature from internal archival tags to estimate energy intake via the Heat Increment of Feeding (HIF), the increased internal heat production associated with digestion of a meal. Our results indicate that HIF was variable in space and time, but it was highest for individuals foraging in the offshore North Pacific Transition Zone and southern California Current during spring and summer, and lowest in the Transition Zone in fall. None of the migratory strategies examined appeared to confer consistently higher energetic benefits than the others. Fish remaining resident in the southern California Current year-round incurred lower migration costs, and could access favorable foraging conditions off Baja California in spring and summer. In contrast, fish which undertook longer migrations had much higher energetic costs during periods of faster transit times, but were able to reach highly productive foraging areas in the central and western Pacific. HIF was generally higher in larger fish, and when ambient temperatures were cooler, but was not strongly correlated with other environmental covariates. Our analyses offer new avenues for studying the physiology of wild tuna populations, and can complement diet and isotopic studies to further understanding of fish ecology.
Introduction
Many large marine vertebrates exhibit long-distance migration behaviors as part of their life cycle. While these movements have energetic costs, they allow animals to follow favorable thermal gradients, access productive foraging habitats, avoid predation, or reproduce in areas that maximize the survival of early life history stages (Alerstam et al., 2003; Winkler et al., 2014). The cues that initiate migrations are complex and not always well understood. Organisms may respond to seasonal cycles of local environmental conditions or prey concentrations, leaving an area when it becomes energetically unfavorable (Kotwicki et al., 2005; Whitlock et al., 2015). Conversely, animals may migrate to time their movements to take advantage of favorable conditions for foraging or reproduction at their destination, potentially following learned or genetically hard-wired routes (Block et al., 2011; Secor, 2015; Hays et al., 2016; Abrahms et al., 2019).
Migratory behaviors are frequently shaped by evolutionary history, and may maximize performance measures such as reproductive success, energy gain, or persistence of a population (Hays et al., 2016). Despite these selection pressures, individuals within a species can show high variability in migratory timing and routes (Abrahms et al., 2018; Patterson et al., 2018). Migration traits can be very plastic, and migratory behaviors can be rapidly lost and gained among species and populations within relatively short time periods (Alerstam and Bäckman, 2018). This plasticity could potentially allow animals to adapt to spatiotemporal mismatches between migration routes and favorable habitats, resulting from environmental variability and climate change. However, to better assess this potential, improved understanding of the interactions between environmental conditions, energetic benefits, and physiological costs is required. While migrations timed to coincide with seasonal cycles of forage availability can confer rewards such as increased fitness (Whitlock et al., 2015; Abrahms et al., 2021), long-distance movements can be energetically risky. The risk is amplified when animals must expend substantial resources to reach distant foraging grounds, or when environmental variability results in mismatched timing between arrival to a foraging area and favorable conditions (Lennox et al., 2016).
Quantifying the costs and benefits of migration strategies in wild organisms can be difficult. This is particularly the case for large marine animals such as sharks, tunas, sea turtles and pinnipeds, which can migrate large distances from coastal to pelagic seas as part of their life cycles (Block et al., 2011; Cooke et al., 2016). However, recent studies have shown that telemetry technologies can provide insight, using (for example) measurements of 3-dimensional movement behaviors (Jonsen et al., 2007), buoyancy (Thums et al., 2011; Del Raye et al., 2013), or internal temperature fluctuations (Bestley et al., 2008) to estimate foraging behavior and energy gain. For regionally endothermic tunas, the Heat Increment of Feeding (HIF) has been used to quantify foraging success, using internal and external temperature records from archival tags (Whitlock et al., 2013, 2015; Aoki et al., 2017; Snyder et al., 2017; Carroll et al., 2021). HIF is based on the increase in visceral temperature observed due to specific dynamic action, the sum total of the metabolic processes required to digest and assimilate a meal (Carey et al., 1984; Bestley et al., 2008; Clark et al., 2008, 2010). Studies on captive tunas have shown that HIF is proportional to the caloric value of a meal, and also influenced by prey species, mass, meal fat or protein content, predator size, and ambient temperature (Whitlock et al., 2013; Aoki et al., 2017).
Regional endothermy allows many tuna species to retain metabolic heat produced in the viscera, muscle, brain and eyes. Some tunas can maintain an elevated and relatively stable internal body temperature, particularly as they increase in body size (Brill et al., 1994; Kitagawa et al., 2006; Aoki et al., 2020). By directly measuring metabolic rate in Pacific bluefin tuna (T. orientalis), Blank et al. (2007a,b) showed the presence of a “thermal minimum zone”: an ambient water temperature range within which this species incurs the lowest metabolic costs. The energetic risks and rewards of foraging migrations in tunas may thus depend on the spatiotemporal interactions between distributions of prey, the thermal habitats that the predator encounters when targeting these prey, and the tuna’s metabolic optimum temperature.
Albacore tuna (Thunnus alalunga, albacore hereafter) is a highly migratory species distributed globally in temperature and tropical waters, including the North Pacific Ocean. Many immature albacore (<5–6 years old) undertake extensive seasonal migrations between temperate coastal ecosystems in the California Current and the central North Pacific (Childers et al., 2011). These movements are assumed to maximize foraging opportunities within suitable thermal habitats, but the energetic costs and benefits associated with these large-scale movements are not yet known (Ichinokawa et al., 2008; Snyder, 2016). A distinctive feature of albacore in the North Pacific is the existence of a resident, “non-migrating” sub-group, which is located in some years in waters off Baja California and the southern California Bight (Childers et al., 2011). This group has historically supported substantial fisheries landings in the region, but can be largely absent for many years at a time (Clemens and Craig, 1965), as it has been since the mid-2000s (Frawley et al., 2021). There has been speculation that these southern California Current fish may represent a separate sub-stock, but recent genetic analyses found no significant genetic structure throughout the North Pacific (Vaux et al., 2021).
The high-frequency sampling of both ambient and internal temperatures by implanted archival tags provides an opportunity to apply principles of thermal and metabolic physiology to unanswered questions on albacore migration. In this study, we used an archival tagging dataset to examine spatiotemporal variability in HIF events for albacore tagged between 2003 and 2011 at lengths between 63 and 96 cm. Our study objectives were to (1) define spatiotemporal variability in North Pacific albacore HIF events, using records from archival-tagged fish, (2) compare energetic costs and benefits between migratory and resident fish, and (3) assess the cross- and within-region predictability of HIF using a suite of environmental and behavioral predictors.
Materials and Methods
Archival Tagging
Albacore archival tagging data were available through two programs. The Albacore Archival Tagging Program is a collaborative effort between the NOAA Southwest Fisheries Science Center and the American Fishermen’s Research Foundation, which has been tagging and releasing albacore since 2002 (Childers et al., 2011; Snyder et al., 2017). The TOPP (Tagging Of Pacific Predators) project is a field program of the Census of Marine Life, and has deployed over 4500 tags on more than 20 species since 2000, including albacore (Block et al., 2011). Three types of archival tags (Lotek LTD2310 and LAT2810, and Wildlife Computers MK9) were surgically implanted in 1315 albacore between 2003 and 2011, with a total of 43 tags recovered and assessed for inclusion in this study.
Daily light-based geolocations for all tags were estimated using manufacturer-provided software. A sea surface temperature (SST) inclusive unscented Kalman filter (ukfsst package version 0.3, Lam et al., 2008; Nielsen et al., 2012), was then fitted to all tracks using the NOAA Optimum Interpolation SST V2 product (Reynolds et al., 2002) in R 3.6.3 (R Core Team, 2020). A bathymetric correction constraining estimated locations based on daily fish depth was then applied using the analyzepsat package (Galuardi et al., 2010). Tags with post-recapture temperature calibration issues, or with poor fit between upper 10 m external temperatures and satellite SST even after geolocation correction were removed from further analyses, as were tags with < 30 days of temperature sensor data available. This resulted in 33 tags for HIF analyses herein.
Albacore lengths taken at time of release under the TOPP program were curved fork lengths (CFL), whereas fish released under the Albacore Archival Tagging Program were measured using straight fork length (SFL). No CFL to SFL conversions have been published for albacore, and so we used the piecewise regression described for the closely related Pacific bluefin tuna by Estess et al. (2014). Albacore were released off the U.S. West Coast and Baja California at SFLs between 63.2 cm and 96.2 cm, between July 2003 and August 2011, and spent between 43 and 752 days at large (Table 1). Due to the potential impacts of tagging-related stress on foraging and movement behaviors (Itoh et al., 2003; Bestley et al., 2008), we discarded the first 30 days of data post-tagging for each fish from our analyses.
Albacore in the California Current are primarily immature juveniles, and spawning has not been recorded in the region (Clemens and Craig, 1965; Sund et al., 1981). However, two-thirds of the fish in this study were released at fork lengths with the 78 – 94 cm size range at maturity reported for the western North Pacific (Table 1, Chen et al., 2010). Albacore typically spawn where SSTs are warmer than 24°C (Farley et al., 2012), although larvae are occasionally collected in waters as cool as ∼ 21 – 22°C (Alvarez-Berastegui et al., 2017). Of the albacore in this study, only one spent more than a few days where SST was >22°C. This fish (tag 2864) was comparatively large (93.3 cm SFL at release), and had a markedly different migration pattern to the other albacore, moving into warm waters well south of Hawaii during spring and summer (Figure 1). As our analyses focused on foraging migrations rather than spawning migrations, we calculated HIF for tag 2864, but did not include this fish in further statistical comparisons of HIF among migratory groups.
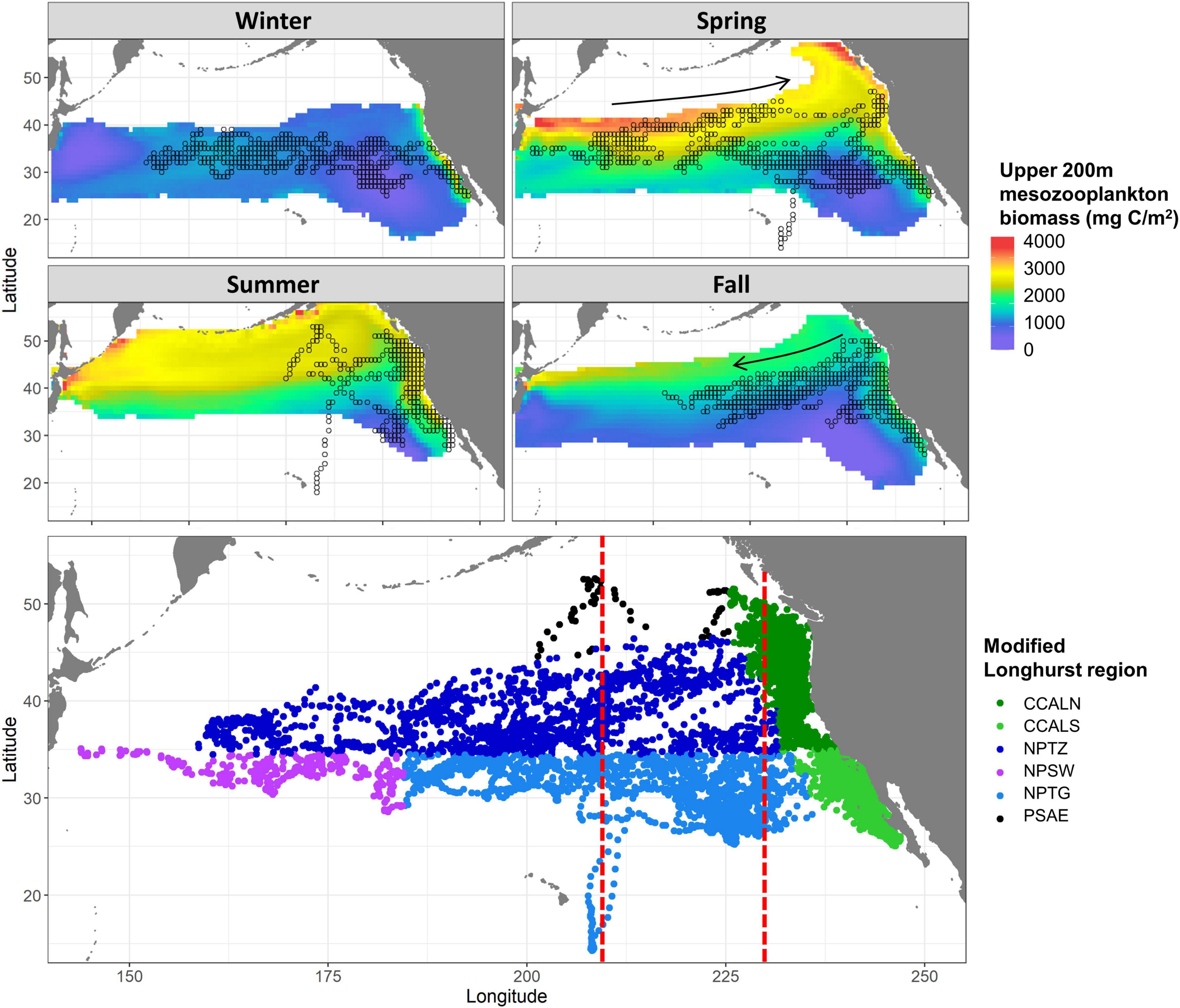
Figure 1. Estimated daily positions for 33 tagged albacore from 2003 to 2013. Top: positions shown by quarter at 1 × 1 resolution, overlaid on 2000 – 2017 climatological integrated upper 200 m mesozooplankton biomass (Park et al., 2018). Locations where SST from the Park et al. (2018) model is outside the range of 12–22°C (which contains > 99% of tag locations) are masked. Arrows denote typical directions of migrating fish. Bottom: positions shown by modified Longhurst region (Longhurst, 2007): The Coastal California Current province was split into north (“CCALN”), and south (“CCALS”) of 35°N, “NPTZ” is North Pacific Transition Zone (also known as North Pacific Polar Front), “NPSW” is North Pacific Subtropical Gyre Province (West), “NPTG” is North Pacific Subtropical Gyre, and “PSAE” is Pacific Subarctic Gyres Province (East). Twenty points in the Alaska Coastal Downwelling province were also assigned to CCALN, and 16 points in the Kuroshio Current province were assigned to NPSW. The Bering Sea and Sea of Okhotsk are masked, as albacore are not known to occur in these areas. The vertical red lines denote 210°E and 230°E: the cutoffs between “Long-migratory,” “Short-migratory,” and “Resident” fish (see Table 1).
Each fish was assigned a migratory pattern based on the maximum distance traveled westwards from their North American release location (Table 1 and Figure 1). “Long-migratory” fish went west of 210°E during some part of their time at large. These animals always left the California Current region in fall, and returned again in late spring. “Short-migratory” albacore traveled west of 230°E, but not west of 210°E. Some of these fish also left the California Current in fall or winter and returned in early spring, while others moved offshore in spring and returned in summer. “Resident” albacore did not move west of 230°E. These fish were located off southern California and Baja California during all months of the year. Fish with <90 days of temperature data available were not assigned a migratory pattern. Most fish in the long-migratory group were released in the northern California Current, while most short-migratory and resident fish were released in the south (Table 1). Long-migratory fish were on average smaller at release than short-migratory or resident fish, but estimated lengths during times at large (see below) overlapped substantially across all three groups.
Estimating Heat Increment of Feeding
As no laboratory data exist for HIF in albacore, due to the difficulties involved with keeping this species in captivity, all measurements were made in the field on wild animals. However, extensive work on the closely related Pacific bluefin tuna provides the basis for modeling and use of HIF in both laboratory and field studies (Whitlock et al., 2013, 2015). In these previous experiments, visual observations of feeding in a captive tank facility were used to identify the total food consumed by individual laboratory fish, and archival tags similar to the ones used in this study were implanted to record body temperatures. This work verified that HIF events are easily visible in Pacific bluefin tuna from fish internal body temperature (Tb). Post feeding, a steady rise in Tb occurs, increasing the thermal excess (Te) between Tb and ambient water temperature (Ta). Fish Tb then falls back to a baseline minimum, usually representing the completion of digestion. Experimental work on Pacific bluefin tuna (Blank et al., 2007b; Clark et al., 2008), and the model of Whitlock et al. (2013), indicate that this baseline represents the routine metabolic rate of the fish in a non-feeding or fasted state. The first step toward quantifying albacore HIF in the current study was therefore to estimate this baseline, fasting Tb in the absence of visceral warming due to feeding.
Whitlock et al. (2013) showed that in the laboratory, baseline Tb in Pacific bluefin tuna is strongly and positively correlated with Ta. However, wild juvenile albacore often show dynamic diving behavior during the day, rapidly moving across thermal gradients of several degrees (Childers et al., 2011). During these times, the lag in Tb in response to Ta across different temperature gradients makes it difficult to calculate the baseline Tb. In contrast, albacore typically stay in the upper mixed layer of the water column during the night, within relatively constant ambient temperatures (Snyder et al., 2017). A steady decrease in Tb during this time is usually clearly visible, with the minimum Tb reached in the hours before dawn (Figure 2). This decrease likely corresponds to the post-absorptive part of the HIF event measured in the laboratory (Clark et al., 2010; Whitlock et al., 2013). We thus used the relationship between the empirically observed minimum Tb during these nightly periods of stable water temperature throughout each fish’s deployment to estimate their baseline Tb as a function of Ta for the entire time-series. Times of nautical dawn and dusk were calculated using dates and estimated daily locations in the R suncalc package version 0.5 (Thieurmel and Elmarhraoui, 2019).
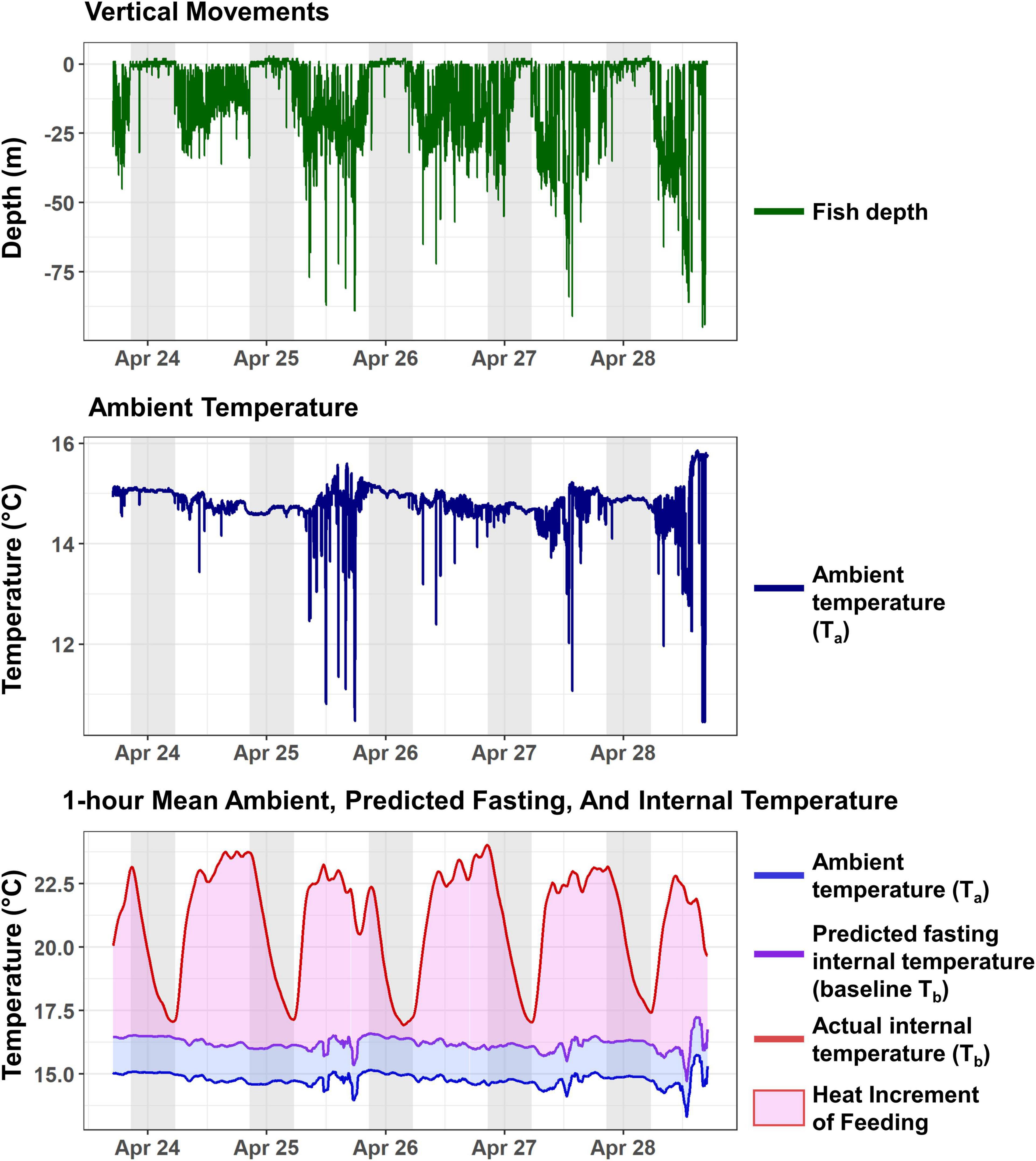
Figure 2. Five days of HIF events for tag 1973 in the CCALS, April 24–28, 2004. Top: depth occupied at 1-min intervals. Middle: ambient water temperature recorded by the tag at 1-min intervals. Bottom: 1-h moving mean of ambient temperature, estimated baseline (fasting) internal temperature, and measured internal temperature. The light pink area denotes the internal temperature increase due to HIF, while the purple area is the estimated thermal excess maintained by the fish under fasting conditions. Gray shading denotes nighttime, defined as time between nautical dusk and nautical dawn.
These night-time observations were fit to the following equation using non-linear least squares (nls) in R 3.6.3 (R Core Team, 2020), such that:
Where Ta is ambient external temperature, and a and b are constants. Although albacore do not usually show diving or cross-frontal behavior at night, calculation of baseline Tb excluded any data where these behaviors could be occurring, defined as periods when Ta changed by more than 1°C over a 30-min time interval. The relationship in equation (1) was established for each fish, and estimates of a and b were then used to calculate the predicted baseline Tb across the entire tag record. For calculations of predicted baseline Tb and Te, we followed Whitlock et al. (2013) and Snyder et al. (2017) in using centered 1-h moving means of both Ta and Tb, to minimize the effect of any high-frequency temperature fluctuations. HIF was then calculated as the area under the curve between the baseline Tb and observed Tb at 1-min intervals, across each 24-h period. These 24 h periods started and stopped at nautical dawn, when a rapid increase in Tb was usually clearly visible as fish commenced foraging behavior (Figure 2).
Estimating Metabolic Costs of Movement
To estimate the energetic costs of movement incurred by each fish, we used the metabolic oxygen demand model of Muhling et al. (2017; Supplementary Figure 1). This model was developed using experimental laboratory data from juvenile Pacific bluefin tuna (Blank et al., 2007a,b; Clark et al., 2013), as there are few comparable data available for albacore where fish were not artificially stressed during metabolic measurements by recent capture (e.g., Graham and Laurs, 1982). Comparative studies suggest that bluefin tuna have higher metabolic rates than other Thunnus species (Blank et al., 2007a), and likely have higher aerobic capacity than albacore due to large aerobic fiber volumes in the fast and slow twitch swimming musculature. However, as both albacore and bluefin tuna are temperate tunas, inhabiting similar thermal ranges as juveniles (Childers et al., 2011; Snyder, 2016; Fujioka et al., 2018), the metabolic data from Pacific bluefin tuna in the Muhling et al. (2017) model is likely the closest approximation available for estimating juvenile albacore metabolic rates. Although they grow much larger as adults, the size range of the bluefin tuna used to develop the model (70–84 cm) was also within the size range of the tagged albacore in this study (Table 1). In addition, the predicted thermal minimum metabolic zone from the oxygen demand model overlaps strongly with the locations of tagged albacore from this study (Supplementary Figure 1). Lastly, as we primarily use the predicted metabolic oxygen demand in a ratio (see below), the interspecific differences between bluefin tuna and albacore should not impact our results substantially.
The metabolic model is a simple Generalized Additive Model (GAM) that predicts the oxygen demands of movement (mg O2 kg–1 hour–1) in fasted animals, given Ta and swimming speed [body lengths (BL)/second]. We estimated the metabolic oxygen demands of movement (abbreviated to “metabolic movement costs” hereafter) for albacore at daily resolution for each fish, using daily mean Ta, and distance traveled in both horizontal and vertical dimensions. Horizontal distance was calculated as the great-circle distance between consecutive daily positions, smoothed using a 7-day moving mean to reduce the effects of substantial error in geolocation estimates (Braun et al., 2018). These estimates do not capture departures from the shortest path, and are thus conservative. Following Aoki et al. (2017), we calculated vertical distance as half the total distance traveled in the vertical plane, assuming that descending movements require minimal energy. The total daily distance traveled in three dimensions was then converted to BL/s, using the estimated daily length of each fish. Changes in albacore length-at-age across the size ranges examined in this study are quite close to linear (Xu et al., 2016). We therefore estimated daily lengths for each fish using growth rates calculated as the difference between the recorded length at release, and the recorded length at recapture, divided by the number of days at large. Where no recapture length was recorded (n = 17 fish), we used mean growth rates (cm/day) from the fish where both measurements were available (Table 1).
The lack of information on albacore diet composition across different locations and seasons prevents the exact determination of daily energy intake, as the relationship between HIF and calories is dependent on the prey species (Whitlock et al., 2013). To examine the costs and benefits of different migration strategies, we therefore used a simple ratio of daily HIF (°C hour) against daily metabolic movement costs (mg O2 kg–1 hour–1) for each fish on each day, hereafter termed the “HIF-cost ratio”:
We assessed whether both HIF, and the HIF-cost ratio, differed between migratory and non-migratory fish using a linear mixed-effects model built using the nlme package (Pinheiro et al., 2020). These models included estimated daily fish length, the migratory strategy of each fish (Table 1) and month as predictors, as well as an interaction between migratory strategy and month. The HIF-cost ratio was right-skewed, and so was natural log transformed before analysis. We included tag number as a random effect, and a first-order temporal autocorrelation process by date. Acceptable fit of each model was assessed using scatterplots of residuals, Q-Q plots, and examination of the autocorrelation function (ACF) of the residuals. Pairwise tests comparing migratory strategies within months were conducted using the emmeans package (Lenth, 2020).
Drivers of Spatial and Temporal Variation in Heat Increment of Feeding
We used Generalized Additive Mixed Models (GAMMs) built in the mgcv package (Wood, 2011) to assess the ability of a suite of environmental variables to predict daily HIF. Predictors were selected to approximate both the temperature and productivity of the fish’s immediate environment, as well as behavioral parameters. We included satellite sea surface chlorophyll from reanalyses developed through the Ocean-Color Climate Change Initiative (OCCCI) using multiple ocean color sensors (Sathyendranath et al., 2019). Surface values were converted to estimates of total euphotic zone chlorophyll using the relationship described in Morel and Berthon (1989). We also included estimates of integrated mesozooplankton biomass and temperature in the upper 200 m from a data-assimilative retrospective physical ocean simulation integrated with a non-assimilative biogeochemical/plankton food web model (Park et al., 2018). These variables were extracted at monthly temporal resolution, and 3 × 3 degree spatial resolution, to account for inaccuracies in geolocation estimates, and the capacity of albacore to travel large distances in short periods of time. Several additional predictors were extracted from the tag records: mean daytime fish depth, and the mean and standard deviation of daytime ambient temperature, the latter of which can highlight the use of strong temperature gradients such as fronts (Snyder et al., 2017). We also included fraction of the moon illuminated, to capture changes in prey and predator behavior in response to the lunar cycle, and fish length, to account for potentially slower rate of heat transfer in larger animals (Kitagawa et al., 2006; Boye et al., 2009). GAMMs were fit with a Gaussian distribution and a log link function. Tag number was included as a random effect in each GAMM, as well as a first-order temporal autocorrelation process by date. Redundancy in the suite of predictors was examined using linear correlations among predictors, and the concurvity function, which shows whether some smooth terms could be approximated by one or more of the other smooth terms in the model (Wood, 2011). Based on these analyses, we removed upper 200m temperature from all GAMMs, and retained all other variables. As before, acceptable fit of each model was assessed using scatterplots of residuals, Q-Q plots, and examination of the ACF of the residuals.
Environmental drivers of HIF variations were evaluated at two different scales. First, we considered HIF across the full study area, encompassing a broad range of temperature and productivity states across six distinct Longhurst regions (Figure 1). Second, we considered drivers of HIF variations within each region separately, to assess whether determinants of successful foraging varied among ecosystems. For the region-specific GAMMs, only data from fish that spent at least 14 days within the selected region were included, which still resulted in > 15 fish and > 1600 fish/days in each region-specific model. We note that our environmental driver estimates, particularly those derived from ocean/biogeochemical simulations, have been evaluated most extensively for cross-regional contrasts that are reasonably captured for the variables used (e.g., Stock et al., 2014; Park et al., 2018). Greater uncertainty exists for more subtle within-region gradients. Predictor importance was determined by re-running the GAMMs with each predictor sequentially dropped, and recording the change in adjusted R2.
Results
Albacore tagged with archival tags occupied a broad range of ocean biomes in the North Pacific. During their deployment time periods, these 33 fish covered a latitudinal range of more than 4,000 km, and a longitudinal range of more than 9,000 km, occupying multiple biogeographic regions (Figure 1). Their migratory paths overlapped with areas of estimated high mesozooplankton biomass in the California Current and in the offshore North Pacific Polar Front, more commonly known as the North Pacific Transition Zone (Polovina et al., 2001). In addition, some fish also moved through more oligotrophic areas, such as the North Pacific Subtropical Gyre. Most albacore were located within the California Current ecosystem during summer, or a short distance offshore. Fish were more dispersed longitudinally during winter, with some resident albacore off the coast of Baja California, and long-migratory fish as far west as 160°E. More than 99% of tag locations were in locations where SST was between 12 and 21°C, consistent with the approximate thermal metabolic minimum zone predicted by the metabolic movement costs model (Supplementary Figure 1). Based on this temperature range, thermal habitat available in the California Current contracted latitudinally to the area south of approximately 40°N in winter, and expanded in spring and summer. However, some fish migrated much further from shore in fall and winter than would be required to simply avoid SSTs of <12°C in the northern California Current.
The increase in fish thermal excess (Te) due to HIF was clearly visible from tag internal temperature sensors (Figure 2). An example from an albacore (fish 1973) located in the southern California Current in spring 2004 shows Te values during daylight hours as large as 6 – 7°C. Te generally reached a maximum during the day, in association with more dynamic diving behavior. Te and Tb slowly declined overnight, reaching a minimum just before dawn. During the night, albacore stayed much shallower in the water column and within much more constant temperatures than those observed during the day.
The mean HIF across all tagged albacore was strongly variable in space, with both high and low values occurring in nearly all regions (Figure 3). During spring, HIF was high across most of the temperate north Pacific, and in the southern California Current. In contrast, HIF was generally low in fall, especially offshore of the California Current. Offshore locations in the North Pacific Transition Zone region in fall represent fish migrating westwards away from the North American coast (Figure 1). During the spring-summer months, albacore tended to use either the southern California Current or the Transition Zone, but not both. Of the 14 fish classified as resident or short-migratory (Table 1), 13 used the southern California Current region in spring or summer, and none used the Transition Zone region during the same seasons. In contrast, of the 10 fish classified as long-migratory, 9 used the Transition Zone region in spring or summer but only 2 used the southern California Current. A more migratory movement pattern was thus associated with greater use of the Transition Zone region, and less use of the southern California Current.
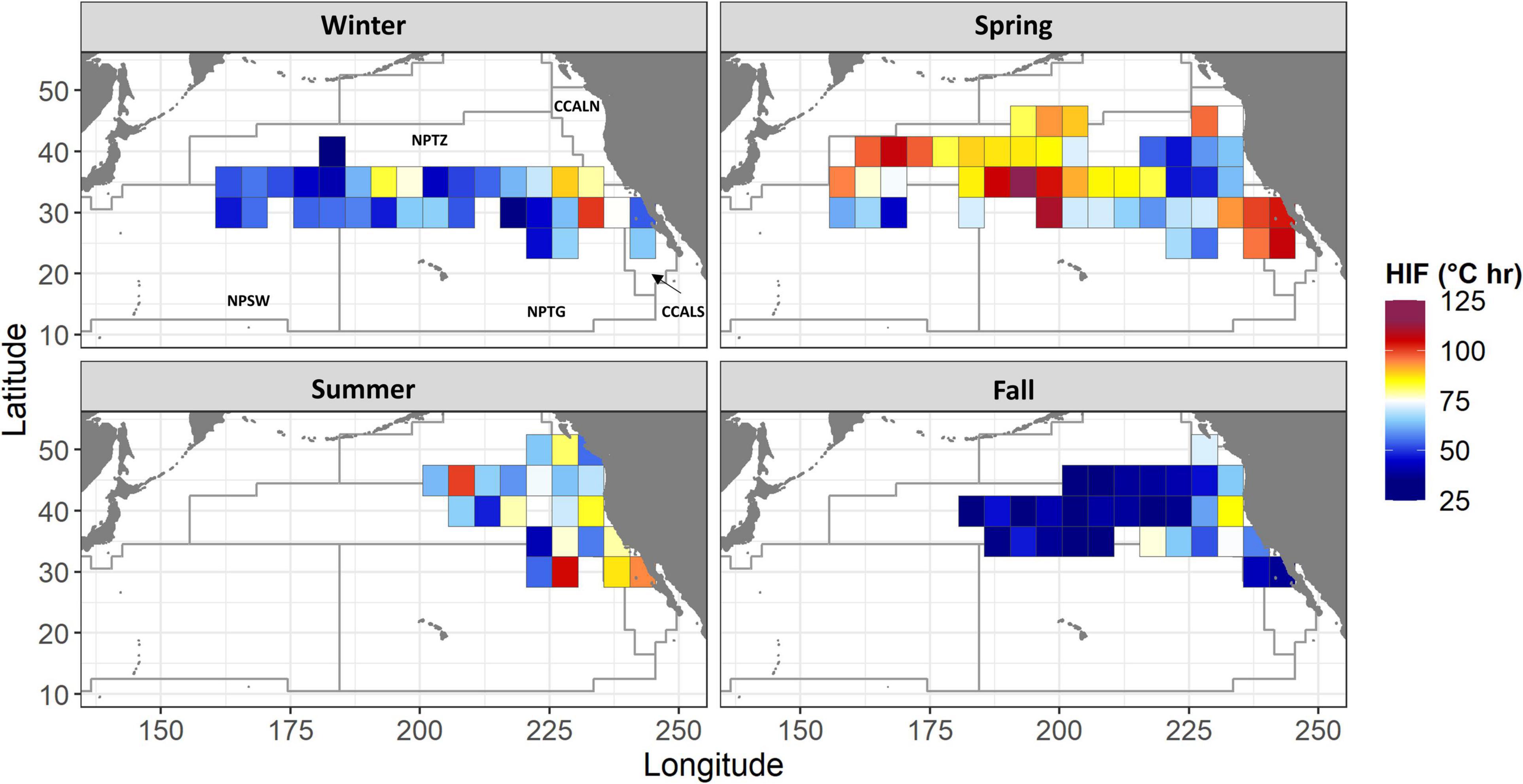
Figure 3. Mean daily HIF by season at 5 × 5 degree resolution, 2003–2013. Only grid cells with data from at least two fish are plotted. Boundaries of modified Longhurst regions from Figure 1 are also shown.
Example time-series from four fish with different migration strategies highlight the substantial spatiotemporal variability in HIF (Figure 4). Fish 1973 is an example of a resident fish, remaining off the coast of Baja California and southern California between December 2003 and August 2004. This fish showed generally low HIF (<50°C hr) during winter months, increasing into the spring and summer. Fish 1987 migrated a short distance offshore in spring of 2004 before returning to the southern California Current. This albacore also showed higher HIF of up to 150°C hr during warmer months, similarly to fish 1973. Fish 1475 was located off southern California in late 2004, before moving offshore in early 2005. Although this fish showed some higher HIF values offshore in January, it appeared to gain the most energy in coastal waters in early summer. Fish 1045 showed long-migratory behavior, moving offshore in November 2006, and reaching ∼190°E by January 2007. It showed highest HIF values (>100°C hr) in early spring while offshore in the North Pacific Transition Zone region, with high values also evident in the California Current in summer and fall. HIF values were lowest during the offshore migration in November – December 2006 (Figure 4).
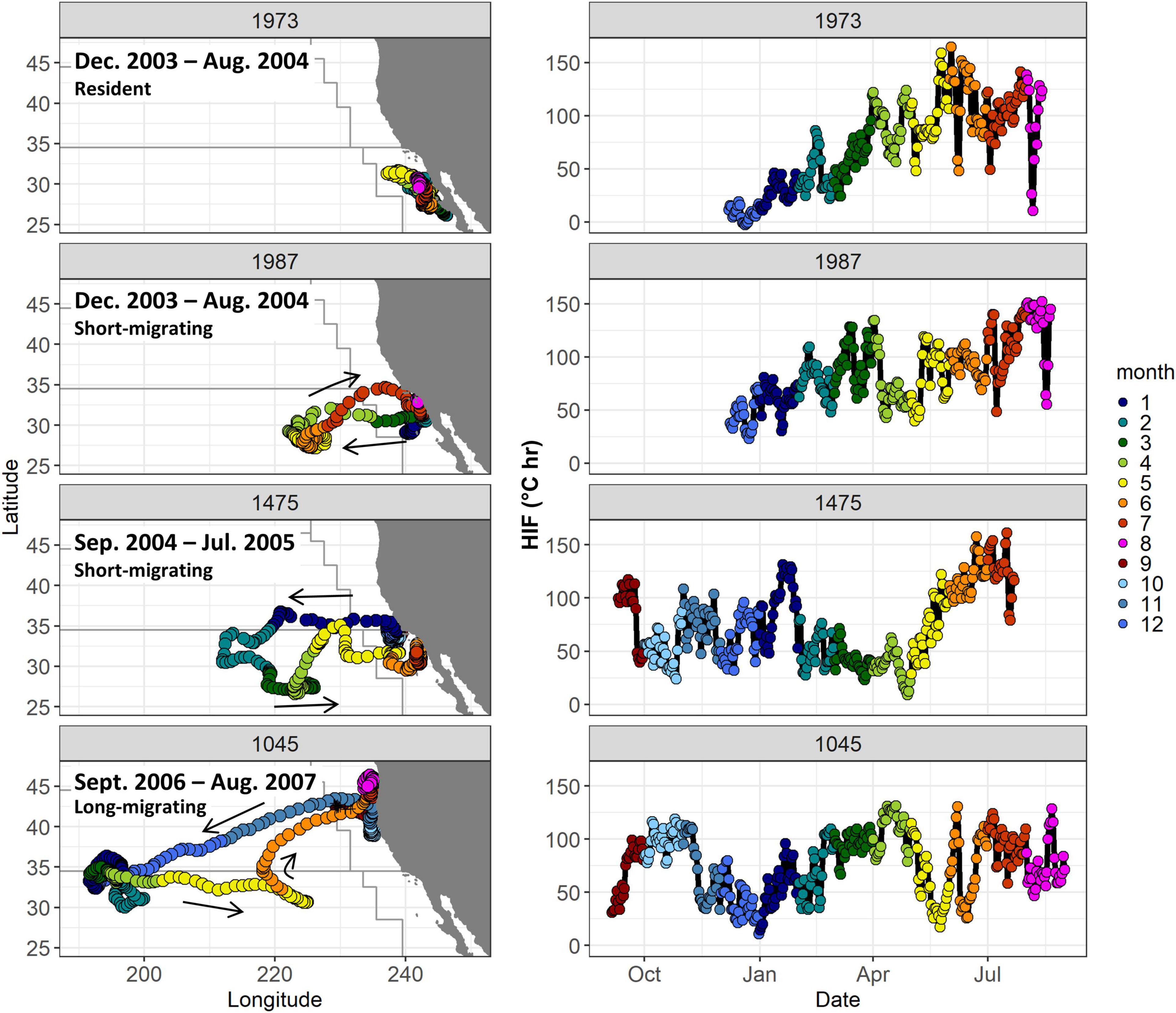
Figure 4. Daily locations (left), and daily mean HIF (right) for four example fish showing different migration patterns (see Table 1). Colors denote month, arrows show migration directions. Boundaries of modified Longhurst regions from Figure 1 are also shown.
The majority of tags recovered were from fish with around a year or less of temperature data available (Table 1). However, some fish were at large for long enough to allow the observation of repeat migrations. Where this was possible, the fidelity of fish to similar migration routes across years was striking (Figure 5). Fish 1045 and fish 2393 showed very similar tracks between consecutive years, leaving the northern California Current in October, spending winter and early spring in the central North Pacific, and then returning to the California Current via the subtropical gyre in May and June. Both fish showed high values of HIF in October before leaving the California Current, and high values again in April–May while in the subtropical gyre, with lowest in late fall and early winter. Fish 1090251 and 1090269 also showed similar migration tracks across consecutive years, but in contrast to fish 1045 and fish 2393, migrated much further west (Figure 5). Fish 1090521 and 1090269 also showed a very strong seasonal signal in HIF, which was consistent between years. These two fish had much lower HIF values in fall and winter, and higher values in spring and early summer, when they were in the western North Pacific and moving eastwards back toward the California Current.
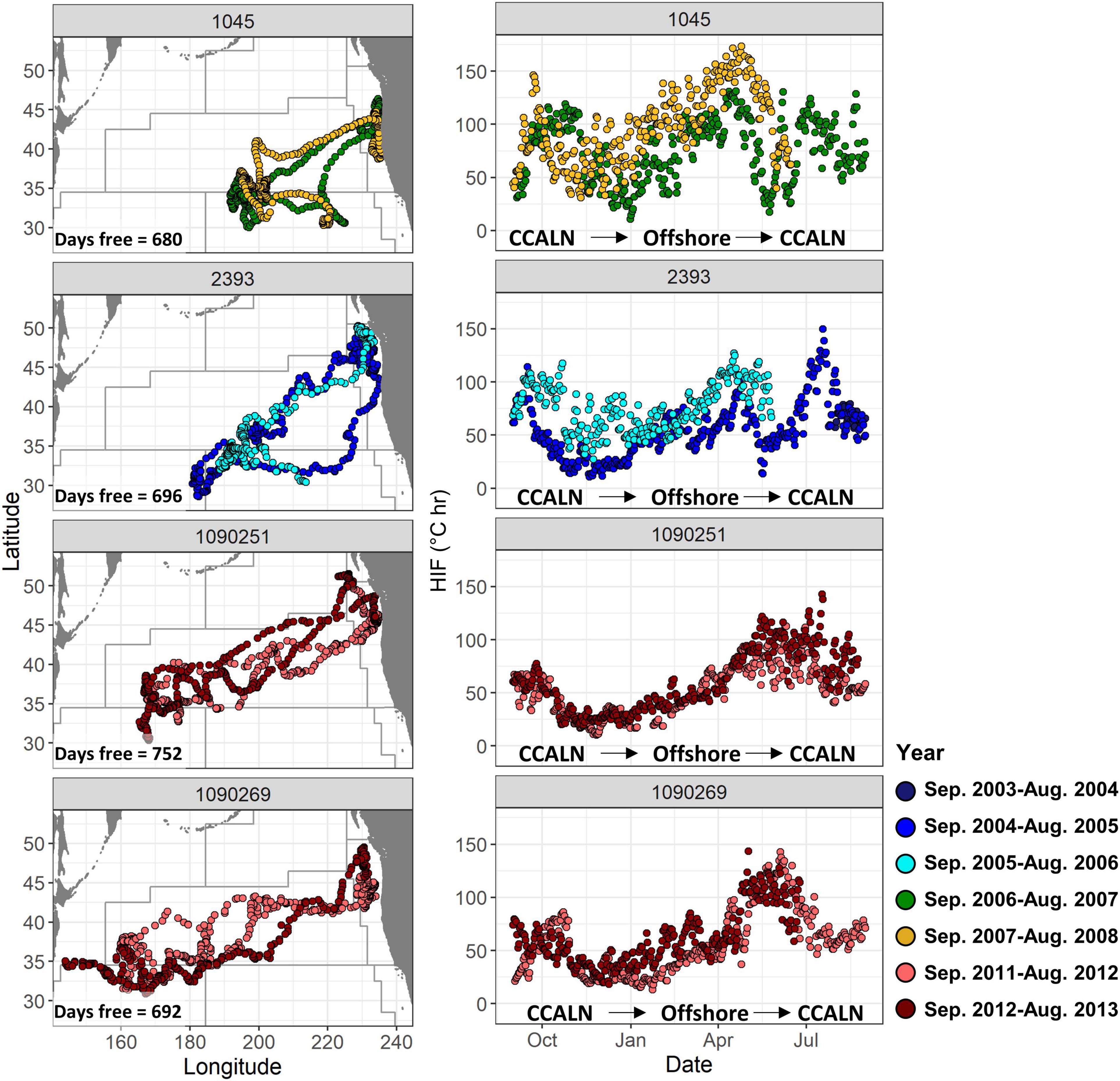
Figure 5. Left: daily locations, and right: daily mean HIF for the 4 fish which were at large the longest, during both years at liberty. Years were defined from September 1st through August 31st, to coincide with typical migration timing leaving and returning to the West Coast. Boundaries of modified Longhurst regions from Figure 1 are also shown. “CCALN” denotes the northern California Current.
Of the 18 albacore that showed migratory behavior (Table 1), 15 moved offshore during the fall, and returned to the California Current during spring (The other three moved only a short distance offshore of southern California during spring and returned in summer). For these 15 fish, HIF recorded during fall and winter was correlated with the farthest distance traveled westwards. Albacore with lower fall/winter HIF moved further westwards away from the North American coast during their migrations (Figure 6). The latitude at which fish left the California Current to begin their offshore migration was also important in determining their movements, with fish leaving from the southern California Current not migrating as far west as those leaving from the north.
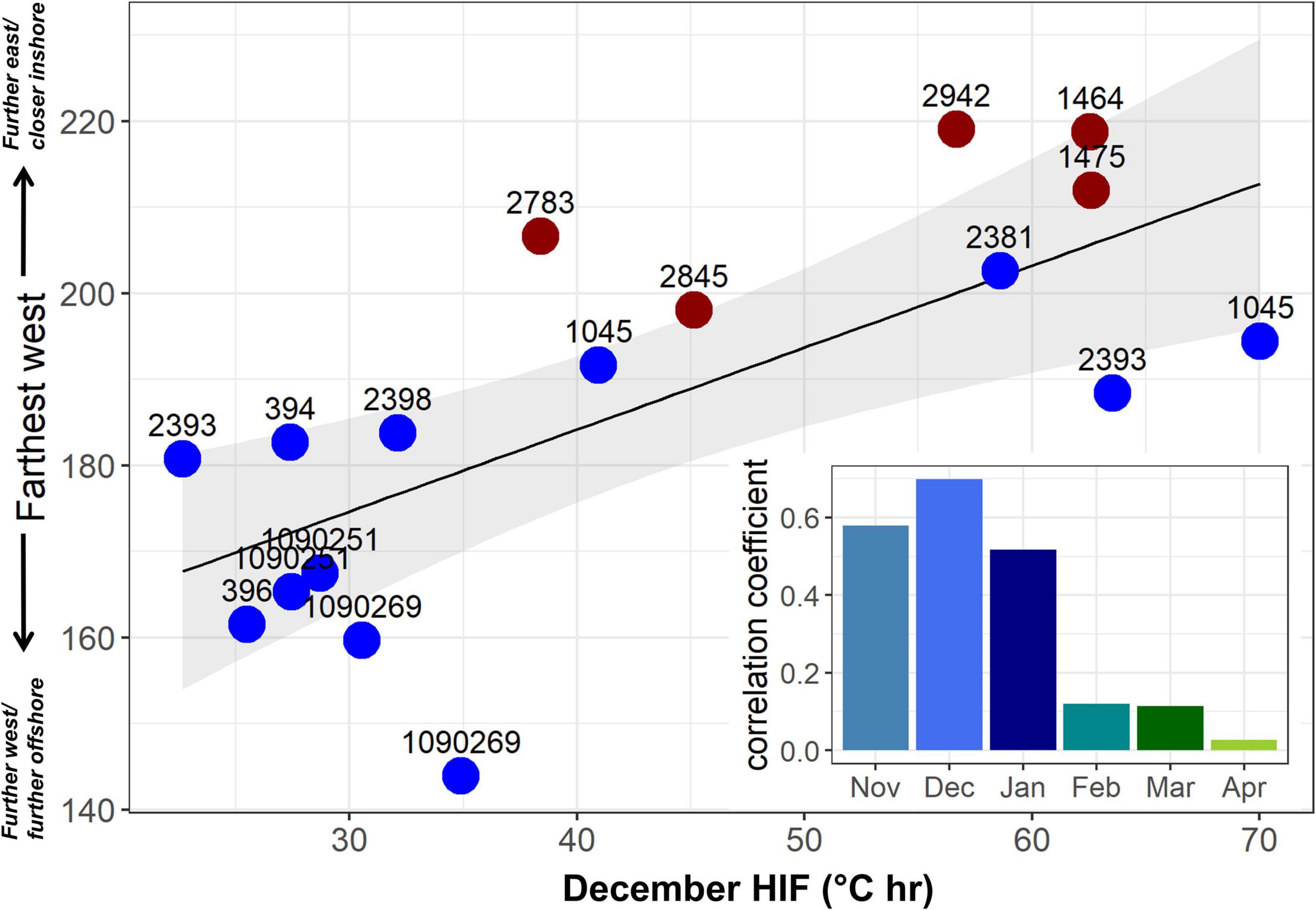
Figure 6. Mean December HIF versus farthest westward location (degrees east, reached between January and June) for the 15 fish which moved offshore of the California Current in fall, and returned during spring, with tag number also shown. Blue circles show fish which departed the California Current in fall north of 40°N, red circles show fish which departed the California Current south of 40°N. Inset shows linear correlation coefficient between mean HIF and farthest west (degrees east) by month.
The diversity of movement patterns in tagged albacore clearly resulted in different seasonal patterns in HIF, and in movement costs. Long-migratory albacore incurred much greater movement costs on average during their onshore migration from March through July, and again during November during offshore migration (Figure 7). However, both HIF and HIF-cost ratios for long-migratory fish were more stable throughout the year, showing a less pronounced seasonal cycle compared to short-migratory and resident fish. The linear mixed-effects model (Supplementary Figure 2) showed that there were few significant (p < 0.05) differences in HIF by month across migration types. Resident fish showed significantly higher values than short migratory fish in May, whereas short-migratory fish showed higher values than resident fish in December. Long-migratory fish also had higher mean HIF values than short-migratory fish in September, and higher HIF than resident fish in December. When mean HIF-cost ratios were examined, mixed models showed that values were significantly higher in resident fish than in long-migratory fish from May through July, and higher than short-migratory fish from April to June. Mean HIF-cost ratios were higher in short-migratory fish than long-migratory fish in August, but the reverse was true in September. These results suggest that the resident strategy may have been the most advantageous energetically, when both energy gain from foraging and energetic costs of movement were considered. However, the weak results for the model comparing HIF only suggest that these differences were primarily driven by the higher movement costs estimated for the migratory fish. Although sample sizes in the current study were very small, we note that there was no difference in estimated daily growth rates (Table 1) across the three migratory strategies (ANOVA, p = 0.65).
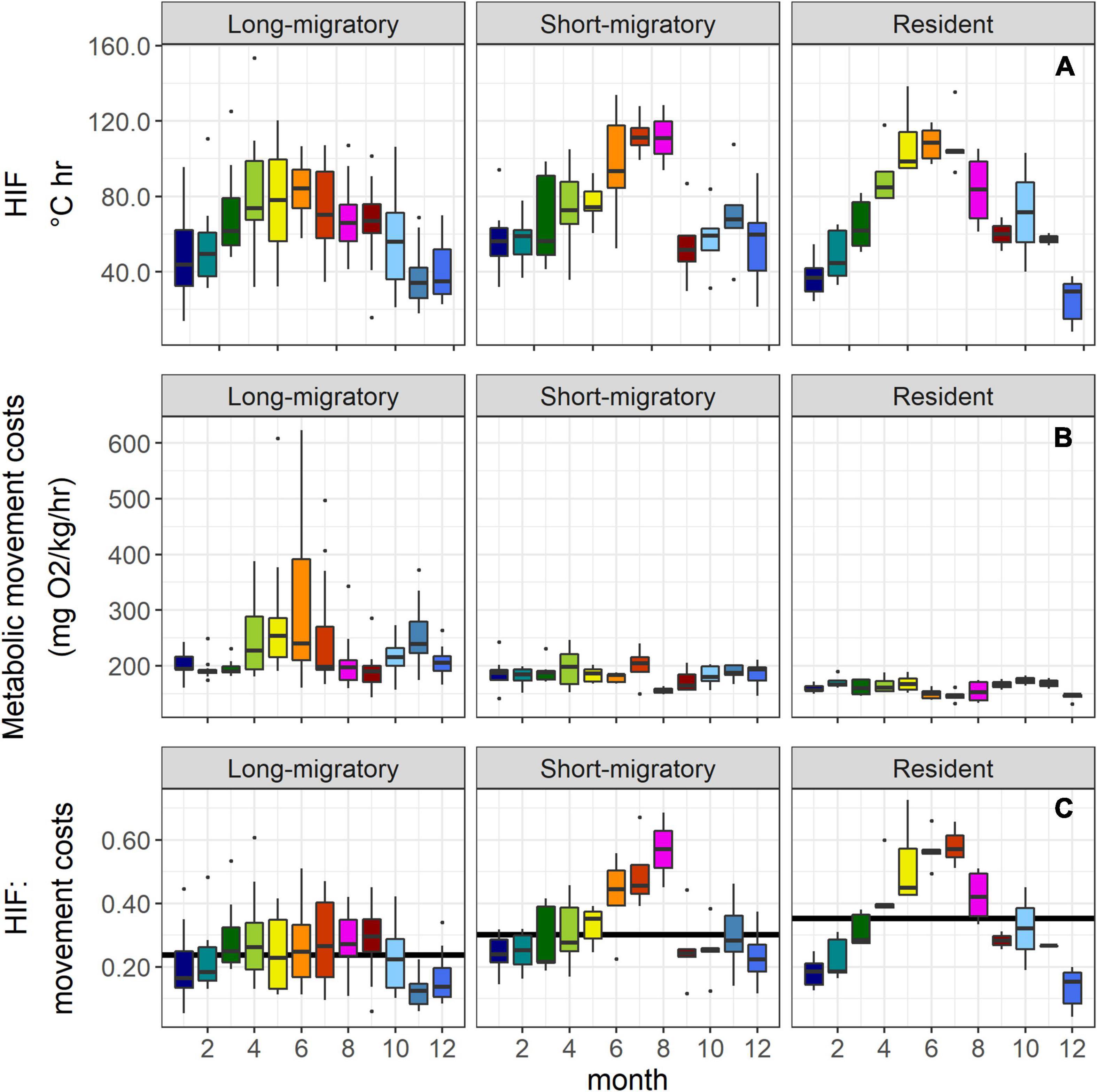
Figure 7. (A) Mean monthly HIF, (B) mean monthly movement costs based on water temperature and swimming speed, and (C) HIF-cost ratio, normalized against the maximum value. Annual mean values of (C) are shown as solid black lines. Tagged fish are split into “long-migratory” fish, “short-migratory” fish, and “resident” fish (see Table 1 and Figure 1). Note that fish at large for more than one migration year always showed the same migratory pattern.
The GAMM predicting daily HIF across all regions highlighted fish length as the most influential predictor, with higher HIF predicted for larger fish (Supplementary Figures 3, 4 and Supplementary Table 1). HIF was also predicted to be greater when fish foraged shallower in the water column, when they encountered less variable ambient temperatures, and when mesozooplankton biomass was higher. GAMMs within the four main Longhurst regions showed that fish length was strongly important in the southern California Current and Transition Zone (also positive relationships), but less so for the other regions. Mesozooplankton biomass was an influential predictor in the Transition Zone region, but was much less important in the California Current (Supplementary Table 1). However, of the two estimates of productivity, mesozooplankton biomass was always more influential to the GAMMs than was depth-integrated chlorophyll. Partial relationships for the region-specific GAMMs generally reflected those from the cross-region GAMM (figures not shown). The fixed effects portion of each GAMM generally had a moderate to low ability to predict daily HIF (except for in the NPTZ, where adjusted R2 was 0.49). Re-running the GAMMs without the first-order temporal autocorrelation process improved predictive ability substantially (Supplementary Table 1), suggesting that the serial autocorrelation in HIF observations (see Figures 4, 5) was not well captured by the environmental and behavioral predictors included.
A closer examination of HIF and the most influential environmental predictor (mesozooplankton biomass) for the more migratory albacore showed strongly fish-dependent and track-dependent relationships (Supplementary Figure 5). Fish 1090251 and 1090269 migrated along the Transition Zone between the California Current in summer and the western North Pacific in winter. Seasonal variability in HIF for these two fish was strongly correlated with the predicted mesozooplankton biomass along their tracks. The raw correlation coefficient was >0.70 for both fish, but only the relationship for tag 1090269 remained significant at p < 0.05 after the substantial (>0.8) temporal autocorrelation was accounted for. In contrast, fish 1045 and 2845 used the eastern Transition Zone in fall before moving into the subtropical gyre in winter and spring. HIF observed for these two fish was not well predicted by mesozooplankton biomass (r < 0.2), particularly in the subtropical gyre. In this region, mesozooplankton biomass was predicted to be low, but HIF could be as high as that recorded in the California Current.
Discussion
Spatiotemporal Structure of Heat Increment of Feeding in Albacore
Foraging migrations allow mobile animals to increase their fitness by accessing caloric resources in spatially distant ecosystems (Alerstam et al., 2003; Abrahms et al., 2021). Here, we use biologging tags that provide high-frequency environmental and physiological data to quantify the movement costs and foraging benefits associated with migration for juvenile albacore in the North Pacific. As the fish included in our analyses were likely not spawning, the primary motivators of movement are assumed to be energetic drivers. These include remaining in thermal environments that reduce metabolic costs while keeping internal body temperature optimum for locomotion and digestion, and maximizing energy acquisition. By estimating both foraging success and movement costs, we were able to examine the energetics of juvenile albacore in time and space across three distinct migratory strategies. Our results show that daily increases in albacore internal temperature on foraging grounds are clearly visible from internal archival tag measurements, and can be substantial (>5 – 7°C above predicted baseline body temperature). Thermal excess was usually at a maximum during the day, and decreased steadily overnight, consistent with most foraging activity taking place during daylight hours.
The albacore included in this study were distributed across foraging areas separated by thousands of kilometers, ranging from productive coastal upwelling systems to epipelagic offshore environments. Examination of HIF across these areas suggested that the most favorable areas were in the southern California Current in spring and summer, and along the offshore North Pacific Transition Zone in spring. All but one fish with at least a month of data available overlapped with one of these two areas during their time at large. Albacore that used the southern California Current foraging area tended to display more residential behavior, either staying in the area throughout the year or undertaking shorter migrations offshore. In contrast, fish that used the transition zone foraging area were often more migratory, moving offshore in fall and back toward the California Current in spring. Three of these highly migratory albacore crossed to the west of the 180° meridian during their time at large, a round trip of more than 8,000 km.
The modeled energetic movement costs among each of the three migratory strategies shown by albacore in this study were clearly distinct. Albacore exhibiting the long-migratory pattern were estimated to spend as much as twice the energy on locomotion between fall and spring each year than did fish that were resident in the southern California Current year-round. This difference was primarily due to the much larger distances traveled by long-migratory albacore: all three movement patterns allowed fish to occupy thermal environments that enabled them to stay approximately within their metabolic thermal minimal zone. However, based on the ratio of HIF to movement costs, migratory behavior did not necessarily lead to fish obtaining more resources. While mean HIF by month was similar among the three migratory strategies, the HIF-cost ratio was higher for the resident fish during spring and early summer. This suggests that the resident migratory strategy may have been more advantageous than the other two, at least for the years where we had data.
Although our results suggest that a non-migrating strategy may be a beneficial one for albacore, this subgroup of the population is not always present. Abundance of albacore off southern California and Baja California has been low since the mid-2000s, with most fisheries landings of this species in the northern California Current (Frawley et al., 2021). This decadal-scale variability is not necessarily a new phenomenon, as low landings of albacore off California were also recorded in the 1930s and early 1940s (Clemens and Craig, 1965), and in the late 1980s (Glaser, 2011). It is possible that the foraging environment in the southern California Current is periodically unfavorable for albacore, due to fluctuating abundance of key prey species such as anchovy (Pinkas et al., 1971) or variability in other components of the ocean environment such as water temperature or persistence of fronts. Other factors such as vertical distributions, varying migration pathways and recruitment to the fishery, or stock size may also influence fisheries landings in the region. However, due to the short time period covered by this study, it is difficult to determine the relative importance of local conditions in the California Current versus drivers of migration routes or other large-scale phenomena on the persistence of the southern subgroup. There are several other uncertainties associated with this analysis. For example, Snyder et al. (2017) showed that several albacore in the southern California Current foraged successfully across thermal fronts. These sub-daily horizontal movements would not be captured by our coarse daily distance traveled estimates. In addition, repeated movements across strong temperature gradients may incur higher metabolic costs than those captured by our simple models, if they require a fish to expend more energy to maintain its preferred internal temperature. If so, this could somewhat offset the energetic advantages of the resident migratory strategy.
Other key uncertainties in our results include the lack of available parameters for physiological modeling of HIF and metabolic processes. Albacore are difficult to keep in captivity, and so we chose to use a metabolic model developed using experimental data for Pacific bluefin tuna. Although the two species are quite similar ecologically, they are far from identical, and the uncertainty introduced by this choice could be substantial. Incorporating physiological processes into ecological models is widely considered to be advantageous, and to provide more robust results when compared to models which are purely correlative. However, our study demonstrates one of the main drawbacks with mechanistic models: they can require detailed experimental data to properly parameterize, and where these are not available, researchers may have to resort to “parameter stealing” (Peck et al., 2018), where measurements from one species are used to represent another.
In addition, we also did not have direct information on albacore diet composition, competition with other large pelagic predators which may target similar prey, energy intake, and caloric costs of movement and digestion. We also assume that mechanisms of HIF in albacore are similar to those recorded in the laboratory for Pacific bluefin tuna. Some previous work has shown that juvenile albacore sampled in the southern California Current (i.e., fish more likely to be resident than migratory) had higher growth rates than those from the northern California Current (Wetherall et al., 1987; Renck et al., 2014). Although sample sizes in the current study were very small, we found no difference in estimated daily growth rates across the migratory strategies. Analyzing otolith growth rates or nutritional condition from additional archival tagged fish could provide much more insight into this possibility, but would be difficult to achieve given the low recapture rates for tagged juvenile albacore, and the low probability of recovering the fish (rather than just the tag) after recapture.
Drivers of Spatial and Temporal Variation in Heat Increment of Feeding
Spatiotemporal structure in HIF was only modestly predicted by a suite of environmental and behavioral variables. Fish size was positively correlated with HIF, particularly in the southern California Current. This may be due to larger stomach volumes in larger fish allowing the ingesting of more calories, or increased thermal inertia with mass resulting in a slower rate of heat transfer (Boye et al., 2009). Satellite-based chlorophyll measurements were not especially useful to the GAMMs, but mesozooplankton biomass from the food web model of Park et al. (2018) provided some skill, particularly in the offshore Transition Zone region. The daily HIF of some albacore that migrated long distances along the Transition Zone was strongly related to predicted mesozooplankton biomass along their tracks. In contrast, mesozooplankton biomass lost its ability to predict HIF in the southern Transition Zone region and in the subtropical gyre. In these areas, HIF was often much higher than would be expected in such oligotrophic waters (see Supplementary Figure 5).
The productivity of these foraging areas may not be well represented by satellite measurements or ocean models. Studies using biogeochemical drifters in the subtropical gyre suggest that eddies may facilitate episodic vertical transport of nutrients into the euphotic zone, in some cases leading to a surface expression of chlorophyll (Johnson et al., 2010; Wilson, 2021). Oceanographic mechanisms supporting productivity in this ecosystem may therefore be fine-scale enough to be missed by the coarse spatial resolution of our analyses, or be occurring largely below the surface. This part of the subtropical gyre is also an important foraging area for many other animals, including white sharks, seabirds, whales, and cephalopods (Jorgensen et al., 2010; Costa et al., 2012; Domeier et al., 2012) and is a productive fishing ground for bigeye tuna (Choy et al., 2016). Additional sampling of the region, in particular subsurface measurements of biogeochemistry and lower trophic levels, could thus clearly improve our understanding of migration dynamics and foraging ecology of multiple species in the North Pacific.
Additional complexity in relationships between ocean productivity and HIF could result from the broad prey assemblage upon which albacore forage. Their diet includes a wide variety of taxa, from coastal pelagic fishes associated with highly productive upwelling systems to vertically migrating pelagic squid associated with the deep scattering layer of more offshore, oligotrophic regions (Laurs and Lynn, 1991; Glaser, 2010). Studies in the California Current show that albacore primarily eat fairly small prey, particularly young-of-the-year finfish and cephalopods (Glaser, 2010). Peak abundance of these prey is more closely related to spawning phenology than to overall ecosystem productivity. Previous work from the western North Pacific has shown a high prevalence of Japanese anchovy (E. japonicus) in the diets of albacore collected west of the 180° meridian (Watanabe et al., 2004). Some of the longest long-migrating fish in our study (e.g., tags 1090251 and 1090269: Figure 5) showed very high HIF in this area during late spring: a potential energetic payoff after the high energetic costs incurred to reach the area. The presence of anchovy so far from their coastal spawning grounds is likely due to strong offshore transport from the Kuroshio Extension, rather than primary productivity characteristics at locations where albacore were sampled (Komatsu et al., 2002).
Foraging Behavior and Migratory Strategies: Risks and Rewards
Other tuna species also undertake migrations to reach foraging areas, but the extent of these movements varies strongly by species. For some, these are relatively short movements within a region, such as for skipjack tuna (Katsuwonus pelamis) and yellowfin tuna (T. albacares) (Schaefer et al., 2007; Aoki et al., 2017). Others, such as Pacific bluefin tuna and Atlantic bluefin tuna (T. thynnus) make trans-ocean movements in a matter of weeks to months (Block et al., 2005; Kitagawa et al., 2009; Fujioka et al., 2018). Some species, such as Pacific bluefin tuna, travel long distances from natal spawning grounds to reach juvenile foraging grounds but are then resident in these areas for many years at a time (Boustany et al., 2010; Carroll et al., 2021). In contrast, immature southern bluefin tuna (T. maccoyii) undertake large-scale, seasonal, out-and-back movements between coastal and offshore foraging grounds (Patterson et al., 2018), a pattern more similar to the long-migratory albacore described in the present study. In the northeast Atlantic, juvenile Atlantic bluefin tuna have also been observed to aggregate in the Bay of Biscay during summer, before dispersing far offshore in cooler months (Arregui et al., 2018). Migration patterns can be flexible, and shift in response to thermal habitats, abundance of prey, or other unknown drivers (Patterson et al., 2018; Chust et al., 2019; Jansen et al., 2021).
Drivers of migratory timing remains one of the main unanswered questions in movement ecology (Nathan et al., 2008). Migratory movements in animals can be initiated by local conditions at the origin of the migration, or anticipated conditions at a distant target. The most predictable foraging migration routes with highest track fidelity should result when resources have the most predictable variation in time and space (Mueller and Fagan, 2008). This can allow “resource tracking,” in which organisms can optimize their energy intake by tracking phenological variation in resources across space (Abrahms et al., 2021). A key question, though, is how animals can locate and return to favorable foraging environments, especially those separated by large distances, and without obvious waypoints (Lennox et al., 2019). In this study, many long-migratory albacore moved offshore of the North American coast in fall, and appeared to show relatively low energy intake through early winter. These fish were then able to access apparently favorable foraging grounds along the offshore Transition Zone in spring. Similar patterns were observed in numerous animals during the tracking of multiple species from distinct taxa during the Census of Marine Life TOPP program (Block et al., 2011), indicating there were optimal periods for being within the California Current and more favorable times to be in the adjacent subtropical gyre. Water temperatures in the northern California Current in winter are likely below optimal metabolic levels for albacore (Snyder, 2016), and cooling temperatures in fall may initiate their offshore migration. However, long-migrating albacore moved much further westward than would be required purely to avoid low temperatures. They may therefore be migrating in anticipation of favorable foraging conditions several months into the future: an energetic reward to balance the risks associated with long migrations through unfavorable foraging habitat.
The source of this prior knowledge of favorable foraging areas remains unclear. The interplay between inherited characteristics (Jorgensen et al., 2010; Kess et al., 2019), responses to environmental cues (Olsson et al., 2006; Horton et al., 2017; Carroll et al., 2021), memory (Abrahms et al., 2019) and learning (Huse et al., 2002) is a key source of uncertainty in studies of animal migration (Lennox et al., 2019). In our study, the degree of track fidelity between years was striking for the few fish with times at large longer than 18 months. However, our data are not sufficient to show whether these fish were following similar environmental gradients across consecutive years, or whether memory or school dynamics were more influential. The negative relationship between HIF in fall as fish moved offshore, and eventual maximum distance from the West Coast, suggests that albacore may respond to foraging conditions en route by modifying their migration speed. However, the mechanisms behind these relationships are not clear from our data. Fish may have modified their westward movements based on foraging success as they moved offshore. Alternatively, other non-foraging cues may have caused fish to follow different migration routes across different years, and their foraging success then varied depending on their spatial overlap with suitable prey.
Overall, our results further demonstrate the value of HIF measurements in endothermic tunas and highlight the value of archival tagging studies in understanding the ecology and physiology of highly migratory fishes. We show how the three migratory strategies observed are associated with diverse risks and rewards in terms of energy expended on locomotion, and energy gained from foraging. Many questions remain regarding the plasticity of migration routes in albacore, the impacts of a variable forage base, and drivers of the appearance and disappearance of substantial numbers of albacore in the southern California Current. In the future, incorporation of data from studies on diet, nutritional condition, and growth rates will likely help us to better understand the energetic consequences of albacore migrations, and how these may be shaped by climate variability and change.
Data Availability Statement
The data analyzed in this study is subject to the following licenses/restrictions: Albacore tracks from the TOPP program are available through the TOPP data interface (https://gtopp.org/). Additional data from tags available through the Albacore Archival Tagging Program are not posted publicly at the request of the American Fishermens Research Foundation, but are available upon request to BM. The mesozooplankton biomass fields are described in Park et al. (2018), and available upon request to J-YP. Chlorophyll re-analysis fields are available on the Coastwatch ERDDAP server at: https://coastwatch.pfeg.noaa.gov/erddap. The albacore metabolic movement demand model is described in Muhling et al. (2017), and is available upon request to BM. Requests to access these datasets should be directed to BM, Barbara.Muhling@noaa.gov.
Ethics Statement
The animal study was reviewed and approved by the Stanford University Administrative Panel on Laboratory Animal Care, and all animal research was conducted in accordance with IACUC protocols.
Author Contributions
BM conceived and designed the study. BM, RW, EH, and SS conducted the statistical analyses. J-YP and CS contributed the biogeochemical model outputs for the study. SS, BB, HD, and RW contributed essential physiological knowledge, and improved the initial HIF modeling and physiological inputs to the manuscript. BB participated in all tagging cruises for albacore at TOPP and conducted surgery on fish. All authors wrote the manuscript, and approved the submitted version.
Funding
BM was partially supported by the Future Seas I & II projects (funded by NOAA’s Coastal and Ocean Climate Applications and Climate and Fisheries Adaptation Programs: grant numbers NA17OAR4310268 and NA20OAR4310507), as well as NOAA internal funding. Funding for TOPP tagging of albacore tuna and data processing was from the Moore and Packard Foundations.
Author Disclaimer
The scientific results and conclusions, as well as any views or opinions expressed herein, are those of the author(s) and do not necessarily reflect the views of NOAA or the Department of Commerce.
Conflict of Interest
The authors declare that the research was conducted in the absence of any commercial or financial relationships that could be construed as a potential conflict of interest.
Publisher’s Note
All claims expressed in this article are solely those of the authors and do not necessarily represent those of their affiliated organizations, or those of the publisher, the editors and the reviewers. Any product that may be evaluated in this article, or claim that may be made by its manufacturer, is not guaranteed or endorsed by the publisher.
Acknowledgments
We thank the captains and crew of all vessels which released and recaptured albacore, including commercial and sport fishermen. We acknowledge the American Fishermen’s Research Foundation, the Tagging of Pacific Predators (TOPP) program, the Inter-American Tropical Tuna Commission, students and staff from the Tuna Research and Conservation Center at Stanford University, and the Monterey Bay Aquarium for assistance with tag deployments aboard the F/V Shogun and tagging training. Animal Care was provided by Stanford IACUC to BB, and by California Department of Fish and Wildlife Special Use permits obtained specifically for the cruises. John Childers and Suzy Kohin were instrumental in developing the NOAA albacore tagging program, and assisting with data interpretation for this study. Tim Lam provided helpful assistance with the UKFSST package, while Camrin Braun and Martin Arostegui contributed valuable advice on data processing and geolocation estimates. Michael Castleton assisted with TOPP data access and data processing. Gemma Carroll and Fernando Gonzalez Taboada provided helpful comments and feedback on an earlier draft of the manuscript. We also thank the two peer-reviewers, whose insightful comments further improved the manuscript.
Supplementary Material
The Supplementary Material for this article can be found online at: https://www.frontiersin.org/articles/10.3389/fmars.2022.730428/full#supplementary-material
References
Abrahms, B., Aikens, E. O., Armstrong, J. B., Deacy, W. W., Kauffman, M. J., and Merkle, J. A. (2021). Emerging perspectives on resource tracking and animal movement ecology. Trends Ecol. Evol. 36, 308–320. doi: 10.1016/j.tree.2020.10.018
Abrahms, B., Hazen, E. L., Aikens, E. O., Savoca, M. S., Goldbogen, J. A., Bograd, S. J., et al. (2019). Memory and resource tracking drive blue whale migrations. Proc. Natl. Acad. Sci. U. S. A. 116, 5582–5587. doi: 10.1073/pnas.1819031116
Abrahms, B., Hazen, E. L., Bograd, S. J., Brashares, J. S., Robinson, P. W., Scales, K. L., et al. (2018). Climate mediates the success of migration strategies in a marine predator. Ecol. Lett. 21, 63–71. doi: 10.1111/ele.12871
Alerstam, T., and Bäckman, J. (2018). Ecology of animal migration. Curr. Biol. 28, R968–R972. doi: 10.1016/j.cub.2018.04.043
Alerstam, T., Hedenström, A., and Åkesson, S. (2003). Long-distance migration: evolution and determinants. Oikos 103, 247–260. doi: 10.1034/j.1600-0706.2003.12559.x
Alvarez-Berastegui, D., Ingram, G. W. Jr., Reglero, P., Macías, D., and Alemany, F. (2017). Albacore (Thunnus alalunga) larval index in the Western Mediterranean Sea, 2001-2015. Collect. Vol. Sci. Pap. ICCAT 74, 687–707.
Aoki, Y., Aoki, A., Ohta, I., and Kitagawa, T. (2020). Physiological and behavioural thermoregulation of juvenile yellowfin tuna Thunnus albacares in subtropical waters. Mar. Biol. 167:71.
Aoki, Y., Kitagawa, T., Kiyofuji, H., Okamoto, S., and Kawamura, T. (2017). Changes in energy intake and cost of transport by skipjack tuna (Katsuwonus pelamis) during northward migration in the northwestern Pacific Ocean. Deep Sea Res. II Top. Stud. Oceanogr. 140, 83–93.
Arregui, I., Galuardi, B., Goñi, N., Lam, C. H., Fraile, I., Santiago, J., et al. (2018). Movements and geographic distribution of juvenile bluefin tuna in the Northeast Atlantic, described through internal and satellite archival tags. ICES J. Mar. Sci. 75, 1560–1572.
Bestley, S., Patterson, T. A., Hindell, M. A., and Gunn, J. S. (2008). Feeding ecology of wild migratory tunas revealed by archival tag records of visceral warming. J. Anim. Ecol. 77, 1223–1233. doi: 10.1111/j.1365-2656.2008.01437.x
Blank, J. M., Farwell, C. J., Morrissette, J. M., Schallert, R. J., and Block, B. A. (2007a). Influence of swimming speed on metabolic rates of juvenile Pacific bluefin tuna and yellowfin tuna. Physiol. Biochem. Zool. 80, 167–177. doi: 10.1086/510637
Blank, J. M., Morrissette, J. M., Farwell, C. J., Price, M., Schallert, R. J., and Block, B. A. (2007b). Temperature effects on metabolic rate of juvenile Pacific bluefin tuna Thunnus orientalis. J. Exp. Biol. 210, 4254–4261. doi: 10.1242/jeb.005835
Block, B. A., Jonsen, I. D., Jorgensen, S. J., Winship, A. J., Shaffer, S. A., Bograd, S. J., et al. (2011). Tracking apex marine predator movements in a dynamic ocean. Nature 475, 86–90. doi: 10.1038/nature10082
Block, B. A., Teo, S. L., Walli, A., Boustany, A., Stokesbury, M. J., Farwell, C. J., et al. (2005). Electronic tagging and population structure of Atlantic bluefin tuna. Nature 434, 1121–1127. doi: 10.1038/nature03463
Boustany, A. M., Matteson, R., Castleton, M., Farwell, C., and Block, B. A. (2010). Movements of Pacific bluefin tuna (Thunnus orientalis) in the Eastern North Pacific revealed with archival tags. Prog. Oceanogr. 86, 94–104. doi: 10.1016/j.pocean.2010.04.015
Boye, J., Musyl, M., Brill, R., and Malte, H. (2009). Transectional heat transfer in thermoregulating bigeye tuna (Thunnus obesus) – a 2D heat flux model. J. Exp. Biol. 212, 3708–3718. doi: 10.1242/jeb.031427
Braun, C. D., Galuardi, B., and Thorrold, S. R. (2018). HMMoce: an R package for improved geolocation of archival-tagged fishes using a hidden Markov method. Methods Ecol. Evol. 9, 1212–1220. doi: 10.1111/2041-210x.12959
Brill, R. W., Dewar, H., and Graham, J. B. (1994). Basic concepts relevant to heat transfer in fishes, and their use in measuring the physiological thermoregulatory abilities of tunas. Environ. Biol. Fish. 40, 109–124. doi: 10.1007/bf00002538
Carey, F. G., Kanwisher, J. W., and Stevens, E. D. (1984). Bluefin tuna warm their viscera during digestion. J. Exp. Biol. 109, 1–20. doi: 10.1152/ajpregu.1984.246.4.R487
Carroll, G., Brodie, S., Whitlock, R., Bograd, S., Hazen, E., and Block, B. A. (2021). Flexible foraging migrations buffer a marine predator against energetic costs of extreme climate variability. Proc. R. Soc. B Biol. Sci. 288:20210671. doi: 10.1098/rspb.2021.0671
Chen, K. S., Crone, P. R., and Hsu, C. C. (2010). Reproductive biology of albacore Thunnus alalunga. J. Fish Biol. 77, 119–136. doi: 10.1111/j.1095-8649.2010.02662.x
Childers, J., Snyder, S., and Kohin, S. (2011). Migration and behavior of juvenile North Pacific albacore (Thunnus alalunga). Fish. Oceanogr. 20, 157–173. doi: 10.1111/j.1365-2419.2011.00575.x
Choy, C. A., Wabnitz, C. C., Weijerman, M., Woodworth-Jefcoats, P. A., and Polovina, J. J. (2016). Finding the way to the top: how the composition of oceanic mid-trophic micronekton groups determines apex predator biomass in the central North Pacific. Mar. Ecol. Prog. Ser. 549, 9–25. doi: 10.3354/meps11680
Chust, G., Goikoetxea, N., Ibaibarriaga, L., Sagarminaga, Y., Arregui, I., Fontán, A., et al. (2019). Earlier migration and distribution changes of albacore in the Northeast Atlantic. Fish. Oceanogr. 28, 505–516. doi: 10.1111/fog.12427
Clark, T. D., Brandt, W. T., Nogueira, J., Rodriguez, L. E., Price, M., Farwell, C. J., et al. (2010). Postprandial metabolism of Pacific bluefin tuna (Thunnus orientalis). J. Exp. Biol. 213, 2379–2385. doi: 10.1242/jeb.043455
Clark, T. D., Farwell, C. J., Rodriguez, L. E., Brandt, W. T., and Block, B. A. (2013). Heart rate responses to temperature in free-swimming Pacific bluefin tuna (Thunnus orientalis). J. Exp. Biol. 216, 3208–3214. doi: 10.1242/jeb.086546
Clark, T. D., Taylor, B. D., Seymour, R. S., Ellis, D., Buchanan, J., Fitzgibbon, Q. P., et al. (2008). Moving with the beat: heart rate and visceral temperature of free-swimming and feeding bluefin tuna. Proc. R. Soc. B Biol. Sci. 275, 2841–2850. doi: 10.1098/rspb.2008.0743
Clemens, H. B., and Craig, W. L. (1965). An Analysis of California’s Albacore Fishery. Sacramento: Resources Agency of California.
Cooke, S. J., Brownscombe, J. W., Raby, G. D., Broell, F., Hinch, S. G., Clark, T. D., et al. (2016). Remote bioenergetics measurements in wild fish: opportunities and challenges. Comp. Biochem. Physiol. A Mol. Integr. Physiol. 202, 23–37. doi: 10.1016/j.cbpa.2016.03.022
Costa, D. P., Breed, G. A., and Robinson, P. W. (2012). New insights into pelagic migrations: implications for ecology and conservation. Ann. Rev. Ecol. Evol. Syst. 43, 73–96. doi: 10.1146/annurev-ecolsys-102710-145045
Del Raye, G., Jorgensen, S. J., Krumhansl, K., Ezcurra, J. M., and Block, B. A. (2013). Travelling light: white sharks (Carcharodon carcharias) rely on body lipid stores to power ocean-basin scale migration. Proc. R. Soc. B Biol. Sci. 280:20130836. doi: 10.1098/rspb.2013.0836
Domeier, M. L., Nasby-Lucas, N., and Palacios, D. M. (2012). “The northeastern Pacific white shark shared offshore foraging area (SOFA) a first examination and description from ship observations and remote sensing,” in Global Perspectives on the Biology and Life History of the Great White Shark, ed. M. L. Domerier (Boca Raton: CRC Press), 147–158.
Estess, E. E., Coffey, D. M., Shimose, T., Seitz, A. C., Rodriguez, L., Norton, A., et al. (2014). Bioenergetics of captive Pacific bluefin tuna (Thunnus orientalis). Aquaculture 434, 137–144.
Farley, J. H., Ashley, J. W., Cambell, R. D., Naomi, P. C. J., Paige, E., Simon, D. H., et al. (2012). Population Biology of Albacore Tuna in the Australian Region. Canberra: CSIRO.
Frawley, T. H., Muhling, B. A., Brodie, S., Fisher, M. C., Tommasi, D., Le Fol, G., et al. (2021). Changes to the structure and function of an albacore fishery reveal shifting social-ecological realities for Pacific Northwest fishermen. Fish Fish. 2, 280–297.
Fujioka, K., Fukuda, H., Tei, Y., Okamoto, S., Kiyofuji, H., Furukawa, S., et al. (2018). Spatial and temporal variability in the trans-Pacific migration of Pacific bluefin tuna (Thunnus orientalis) revealed by archival tags. Prog. Oceanogr. 162, 52–65. doi: 10.1016/j.pocean.2018.02.010
Galuardi, B., Royer, F., Golet, W., Logan, J., Neilson, J., and Lutcavage, M. (2010). Complex migration routes of Atlantic bluefin tuna (Thunnus thynnus) question current population structure paradigm. Can. J. Fish. Aquat. Sci. 67, 966–976.
Glaser, S. M. (2010). Interdecadal variability in predator–prey interactions of juvenile North Pacific albacore in the California Current System. Mar. Ecol. Prog. Ser. 414, 209–221.
Glaser, S. M. (2011). Do albacore exert top-down pressure on northern anchovy? Estimating anchovy mortality as a result of predation by juvenile North Pacific albacore in the California current system. Fish. Oceanogr. 20, 242–257.
Graham, J. B., and Laurs, R. M. (1982). Metabolic rate of the albacore tuna Thunnus alalunga. Mar. Biol. 72, 1–6.
Hays, G. C., Ferreira, L. C., Sequeira, A. M., Meekan, M. G., Duarte, C. M., Bailey, H., et al. (2016). Key questions in marine megafauna movement ecology. Trends Ecol. Evol. 31, 463–475. doi: 10.1016/j.tree.2016.02.015
Horton, T. W., Hauser, N., Zerbini, A. N., Francis, M. P., Domeier, M. L., Andriolo, A., et al. (2017). Route fidelity during marine megafauna migration. Front. Mar. Sci. 4:422. doi: 10.3389/fmars.2017.00422
Huse, G., Railsback, S., and Feronö, A. (2002). Modelling changes in migration pattern of herring: collective behaviour and numerical domination. J. Fish Biol. 60, 571–582.
Ichinokawa, M., Coan, A. L., and Takeuchi, Y. (2008). Transoceanic migration rates of young North Pacific albacore, Thunnus alalunga, from conventional tagging data. Can. J. Fish. Aquat. Sci. 65, 1681–1691.
Itoh, T., Tsuji, S., and Nitta, A. (2003). Migration patterns of young Pacific bluefin tuna (Thunnus orientalis) determined with archival tags. Fish. Bull. 101, 514–534.
Jansen, T., Nielsen, E. E., Rodríguez-Ezpeleta, N., Arrizabalaga, H., Post, S., and MacKenzie, B. R. (2021). Atlantic bluefin tuna (Thunnus thynnus) in Greenland–mixed-stock origin, diet, hydrographic conditions and repeated catches in this new fringe area. Can. J. Fish. Aquat. Sci. 78, 400–408. doi: 10.1139/cjfas-2020-0156
Johnson, K. S., Riser, S. C., and Karl, D. M. (2010). Nitrate supply from deep to near-surface waters of the North Pacific subtropical gyre. Nature 465, 1062–1065. doi: 10.1038/nature09170
Jonsen, I. D., Myers, R. A., and James, M. C. (2007). Identifying leatherback turtle foraging behaviour from satellite telemetry using a switching state-space model. Mar. Ecol. Prog. Ser. 337, 255–264.
Jorgensen, S. J., Reeb, C. A., Chapple, T. K., Anderson, S., Perle, C., Van Sommeran, S. R., et al. (2010). Philopatry and migration of Pacific white sharks. Proc. R. Soc. B Biol. Sci. 277, 679–688. doi: 10.1098/rspb.2009.1155
Kess, T., Bentzen, P., Lehnert, S. J., Sylvester, E. V., Lien, S., Kent, M. P., et al. (2019). A migration-associated supergene reveals loss of biocomplexity in Atlantic cod. Sci. Adv. 5:eaav2461. doi: 10.1126/sciadv.aav2461
Kitagawa, T., Kimura, S., Nakata, H., and Yamada, H. (2006). Thermal adaptation of Pacific bluefin tuna Thunnus orientalis to temperate waters. Fish. Sci. 72, 149–156.
Kitagawa, T., Kimura, S., Nakata, H., Yamada, H., Nitta, A., Sasai, Y., et al. (2009). Immature Pacific bluefin tuna, Thunnus orientalis, utilizes cold waters in the Subarctic Frontal Zone for trans-Pacific migration. Environ. Biol. Fish. 84, 193–196. doi: 10.1007/s10641-008-9409-8
Komatsu, T., Sugimoto, T., Ishida, K. I., Itaya, K., Mishra, P., and Miura, T. (2002). Importance of the Shatsky Rise Area in the Kuroshio Extension as an offshore nursery ground for Japanese anchovy (Engraulis japonicus) and sardine (Sardinops melanostictus). Fish. Oceanogr. 11, 354–360. doi: 10.1046/j.1365-2419.2002.00218.x
Kotwicki, S., Buckley, T. W., Honkalehto, T., and Walters, G. (2005). Variation in the distribution of walleye pollock (Theragra chalcogramma) with temperature and implications for seasonal migration. Fish. Bull. 103, 574–587.
Lam, C. H., Nielsen, A., and Sibert, J. R. (2008). Improving light and temperature based geolocation by unscented Kalman filtering. Fish. Res. 91, 15–25. doi: 10.1016/j.fishres.2007.11.002
Laurs, R. M., and Lynn, R. J. (1991). “North Pacific albacore ecology and oceanography,” in Biology, Oceanography and Fisheries and the North Pacific Transition Zone and Subarctic Frontal Zone, ed. J. A. Wetherall (Washington: NOAA), 69–87.
Lennox, R. J., Chapman, J. M., Souliere, C. M., Tudorache, C., Wikelski, M., Metcalfe, J. D., et al. (2016). Conservation physiology of animal migration. Conserv. Physiol. 4:cov072. doi: 10.1093/conphys/cov072
Lennox, R. J., Paukert, C. P., Aarestrup, K., Auger-Méthé, M., Baumgartner, L., Birnie-Gauvin, K., et al. (2019). One hundred pressing questions on the future of global fish migration science, conservation, and policy. Front. Ecol. Evol. 7:286. doi: 10.3389/fevo.2019.00286
Lenth, R. V. (2020). emmeans: Estimated Marginal Means, aka Least-Squares Means. R Package Version 1.5.3. Available online at: https://CRAN.R-project.org/package=emmeans.
Morel, A., and Berthon, J.-F. (1989). Surface pigments, algal biomass profiles, and potential production of the euphotic layer: relationships reinvestigated in view of remote-sensing applications. Limnol. Oceanogr. 34, 1545–1562. doi: 10.4319/lo.1989.34.8.1545
Mueller, T., and Fagan, W. F. (2008). Search and navigation in dynamic environments–from individual behaviors to population distributions. Oikos 117, 654–664. doi: 10.1111/j.0030-1299.2008.16291.x
Muhling, B. A., Brill, R., Lamkin, J. T., Roffer, M. A., Lee, S. K., Liu, Y., et al. (2017). Projections of future habitat use by Atlantic bluefin tuna: mechanistic vs. correlative distribution models. ICES J. Mar. Sci. 74, 698–716. doi: 10.1093/icesjms/fsw215
Nathan, R., Getz, W. M., Revilla, E., Holyoak, M., Kadmon, R., Saltz, D., et al. (2008). A movement ecology paradigm for unifying organismal movement research. Proc. Natl. Acad. Sci. U. S. A. 105, 19052–19059. doi: 10.1073/pnas.0800375105
Nielsen, A., Sibert, J. R., Ancheta, J., Galuardi, B., and Lam, C. H. (2012). ukfsst: Kalman Filter tracking including Sea Surface Temperature. R package version 0.3.
Olsson, I. C., Greenberg, L. A., Bergman, E., and Wysujack, K. (2006). Environmentally induced migration: the importance of food. Ecol. Lett. 9, 645–651. doi: 10.1111/j.1461-0248.2006.00909.x
Park, J. Y., Stock, C. A., Yang, X., Dunne, J. P., Rosati, A., John, J., et al. (2018). Modeling global ocean biogeochemistry with physical data assimilation: a pragmatic solution to the equatorial instability. J. Adv. Model. Earth Syst. 10, 891–906. doi: 10.1002/2017ms001223
Patterson, T. A., Eveson, J. P., Hartog, J. R., Evans, K., Cooper, S., Lansdell, M., et al. (2018). Migration dynamics of juvenile southern bluefin tuna. Sci. Rep. 8:14553. doi: 10.1038/s41598-018-32949-3
Peck, M. A., Arvanitidis, C., Butenschön, M., Canu, D. M., Chatzinikolaou, E., Cucco, A., et al. (2018). Projecting changes in the distribution and productivity of living marine resources: a critical review of the suite of modelling approaches used in the large European project VECTORS. Estuar. Coast. Shelf Sci. 201, 40–55. doi: 10.1016/j.ecss.2016.05.019
Pinheiro, J., Bates, D., DebRoy, S., Sarkar, D., and R Core Team (2020). nlme: Linear and Nonlinear Mixed Effects Models. R Package Version 3.1-148. Available online at: https://CRAN.R-project.org/package=nlme.
Pinkas, L., Oliphant, M. W., and Iverson, I. L. K. (1971). Food Habits of Albacore, Bluefin Tuna and Bonito in California Waters. California: California Department of Fish and Game.
Polovina, J. J., Howell, E., Kobayashi, D. R., and Seki, M. P. (2001). The transition zone chlorophyll front, a dynamic global feature defining migration and forage habitat for marine resources. Prog. Oceanogr. 49, 469–483. doi: 10.1016/s0079-6611(01)00036-2
R Core Team (2020). R: A Language and Environment for Statistical Computing. Vienna: R Foundation for Statistical Computing.
Renck, C. L., Wells, R. J. D., Dewar, H., and Talley, D. M. (2014). Regional growth patterns of juvenile albacore (Thunnus alalunga) in the eastern North Pacific. Calif. Cooperat. Ocean. Fish. Investig. Rep. 55, 135–143.
Reynolds, R. W., Rayner, N. A., Smith, T. M., Stokes, D. C., and Wang, W. (2002). An improved in situ and satellite SST analysis for climate. J. Clim. 15, 1609–1625.
Sathyendranath, S., Brewin, R. J., Brockmann, C., Brotas, V., Calton, B., Chuprin, A., et al. (2019). An ocean-colour time series for use in climate studies: the experience of the ocean-colour climate change initiative (OC-CCI). Sensors 19:4285. doi: 10.3390/s19194285
Schaefer, K. M., Fuller, D. W., and Block, B. A. (2007). Movements, behavior, and habitat utilization of yellowfin tuna (Thunnus albacares) in the northeastern Pacific Ocean, ascertained through archival tag data. Mar. Biol. 152, 503–525.
Simpson, G. L. (2021). gratia: Graceful ‘ggplot’-Based Graphics and Other Functions for GAMs Fitted Using ‘mgcv’. R Package Version 0.6.0. Available online at: https://CRAN.R-project.org/package=gratia.
Snyder, S. M. (2016). Navigating a Seascape: Physiological and Environmental Motivations Behind Juvenile North Pacific Albacore Movement Patterns. Ph.D. thesis. San Diego: University of California.
Snyder, S., Franks, P. J., Talley, L. D., Xu, Y., and Kohin, S. (2017). Crossing the line: tunas actively exploit submesoscale fronts to enhance foraging success. Limnol. Oceanogr. Lett. 2, 187–194.
Stock, C. A., Dunne, J. P., and John, J. J. (2014). Global-scale carbon and energy flows through the marine planktonic food web: an analysis with a coupled physical–biological model. Prog. Oceanogr. 120, 1–28.
Sund, P. N., Blackburn, M., and Williams, F. (1981). Tunas and their environment in the Pacific Ocean: a review. Oceanogr. Mar. Biol. Ann. Rev. 19, 443–512.
Thieurmel, G., and Elmarhraoui, A. (2019). Package ‘suncalc’: Compute Sun Position, Sunlight Phases, Moon Position and Lunar Phase. R Package Version 0.5. Available online at: https://cran.r-project.org/web/packages/suncalc/suncalc.pdf.
Thums, M., Bradshaw, C. J., and Hindell, M. A. (2011). In situ measures of foraging success and prey encounter reveal marine habitat-dependent search strategies. Ecology 92, 1258–1270. doi: 10.1890/09-1299.1
Vaux, F., Bohn, S., Hyde, J. R., and O’Malley, K. G. (2021). Adaptive markers distinguish North and South Pacific Albacore amid low population differentiation. Evol. Appl. 14, 1343–1364. doi: 10.1111/eva.13202
Watanabe, H., Kubodera, T., Masuda, S., and Kawahara, S. (2004). Feeding habits of albacore Thunnus alalunga in the transition region of the central North Pacific. Fish. Sci. 70, 573–579.
Wetherall, J. A., Laurs, R. M., Nishimoto, R. N., and Yong, M. Y. (1987). “Growth variation and stock structure in North Pacific albacore,” in Albacore Workshop Working Paper, (Shimizu: Far Seas Fisheries Research Laboratory), 21.
Whitlock, R. E., Hazen, E. L., Walli, A., Farwell, C., Bograd, S. J., Foley, D. G., et al. (2015). Direct quantification of energy intake in an apex marine predator suggests physiology is a key driver of migrations. Sci. Adv. 1:e1400270. doi: 10.1126/sciadv.1400270
Whitlock, R. E., Walli, A., Cermeño, P., Rodriguez, L. E., Farwell, C., and Block, B. A. (2013). Quantifying energy intake in Pacific bluefin tuna (Thunnus orientalis) using the heat increment of feeding. J. Exp. Biol. 216, 4109–4123. doi: 10.1242/jeb.084335
Wilson, C. (2021). Episodic subsurface injections in the oligotrophic north pacific observed from biogeochemical-argo data. J. Geophys. Res. Oceans 126:e2021JC017169.
Winkler, D. W., Jørgensen, C., Both, C., Houston, A. I., McNamara, J. M., Levey, D. J., et al. (2014). Cues, strategies, and outcomes: how migrating vertebrates track environmental change. Mov. Ecol. 2:10.
Wood, S. N. (2011). Fast stable restricted maximum likelihood and marginal likelihood estimation of semiparametric generalized linear models. J. R. Stat. Soc. 73, 3–36.
Keywords: North Pacific albacore, foraging ecology, highly migratory species, tuna migration, heat increment of feeding
Citation: Muhling BA, Snyder S, Hazen EL, Whitlock RE, Dewar H, Park J-Y, Stock CA and Block BA (2022) Risk and Reward in Foraging Migrations of North Pacific Albacore Determined From Estimates of Energy Intake and Movement Costs. Front. Mar. Sci. 9:730428. doi: 10.3389/fmars.2022.730428
Received: 24 June 2021; Accepted: 22 February 2022;
Published: 29 March 2022.
Edited by:
Alberto Basset, University of Salento, ItalyReviewed by:
José Lino Vieira De Oliveira Costa, University of Lisbon, PortugalIgor Arregui, Marine Research Division, Technology Center Expert in Marine and Food Innovation (AZTI), Spain
Copyright © 2022 Muhling, Snyder, Hazen, Whitlock, Dewar, Park, Stock and Block. This is an open-access article distributed under the terms of the Creative Commons Attribution License (CC BY). The use, distribution or reproduction in other forums is permitted, provided the original author(s) and the copyright owner(s) are credited and that the original publication in this journal is cited, in accordance with accepted academic practice. No use, distribution or reproduction is permitted which does not comply with these terms.
*Correspondence: Barbara A. Muhling, QmFyYmFyYS5NdWhsaW5nQG5vYWEuZ292