- 1Institute of Marine Science and Technology, Shandong University, Qingdao, China
- 2Southern Marine Science and Engineering Guangdong Laboratory, Zhuhai, China
- 3Jiangsu Institute of Marine Resources Development, Jiangsu Key Laboratory of Marine Bioresources and Environment, Jiangsu Ocean University, Lianyungang, China
Climate change can increase riverine discharge, which will promote terrigenous particle transportation and deeply impact microbe-mediated biogeochemical processes in the estuarine ecosystem. However, little is known about the long-term impacts of terrigenous particles on autotrophic and heterotrophic microbial community structures due to in situ continuous particle input. To solve this problem, a large-volume indoor incubation experiment was set up for over 40 days to simulate terrigenous particle input scenario. The activity and community structures of keystone groups were largely correlated with biochemical components derived from the terrigenous particles. The ecosystem was maintained by chemoautotrophic nitrifiers before the addition of terrigenous particles. The system was then functionally dominated by heterotrophic microorganisms after the input of terrigenous particles because terrigenous particles created environments that allowed heterotrophs to proliferate better than chemoautotrophs. The input of terrigenous particles increased the relative intensity of humic-like compounds mainly through releasing nutrients and biological labile organic matter to the seawater, which promoted the microbial transformation of organic matter. This study illustrates that terrigenous particles can impact the balance between heterotrophic and chemoautotrophic microbes and play an important role in humic-like compound transformation in seawater.
1. Introduction
Estuaries connect two of the Earth’s most important carbon sinks, terrestrial and marine ecosystems. Annually, 150 Mt of terrigenous particulate organic carbon (POC) is transported to global oceans via rivers (Smeaton and Austin, 2022). Precipitation is predicted to increase under climate change (Meier, 2006), leading to higher flood risks (Hirabayashi et al., 2013) and consequently the transportation of more terrigenous particles to the ocean (Bauer et al., 2013). Before entering the open ocean, terrigenous particulate organic matter (POM) passes through the estuarine filter (Canuel et al., 2012). It has been suggested that terrigenous organic matter plays a vital role in maintaining coastal carbon cycling (Bianchi et al., 2011; Bauer et al., 2013; Ward et al., 2016). Despite the huge input of terrigenous particles, chemical biomarker and stable isotopic data indicated that there were little terrigenous particles in the ocean (Hedges et al., 1997; Bianchi, 2011; Kandasamy and Nath, 2016). This large discrepancy indicates that a large proportion of terrigenous organic matter is mineralized or photo-degraded in the estuary (Dalzell et al., 2009; Fichot and Benner, 2014).
The degradation of terrigenous organic matter will contribute numerous nutrients and organic matter to the oceans, thus strongly impacting marine ecosystems (Jeandel and Oelkers, 2015). Many studies believe that nutrients limit phytoplankton biomass, and increased discharge of terrigenous nutrients promotes phytoplankton productivity (Rabalais et al., 2002; Smith, 2006). However, it has been reported that increased river discharge would suppress phytoplankton biomass production and promote carbon flow to microbial heterotrophy (Wikner and Andersson, 2012). It can be inferred that heterotrophy will play a more important role after terrigenous organic matter input. However, they ignore chemoautotrophic microorganisms that are universal and important players of biogeochemical processes in the seawater (Pachiadaki et al., 2017; Sebastián et al., 2018). Nitrifiers are the most important chemoautotrophic groups (Middelburg, 2011; Hutchins and Capone, 2022), and their activities are largely determined by the concentration of ammonium and nitrite (Kemp and Dodds, 2002). For example, ammonia-oxidizing archaea (AOA) could account for over 20% of 16S rRNA amplicons in coastal water (Rasmussen and Francis, 2022). A positive correlation between nitrite-oxidizing bacteria (NOB) and the potential nitrification rates was also found in estuaries (Monteiro et al., 2017), highlighting the importance of nitrifiers in coastal systems. To evaluate the impacts of terrigenous particles on the balance between heterotrophy and autotrophy, chemoautotrophs should be taken into account.
Studies suggested that an increase in riverine input would increase prokaryotic C production rate and significantly alter phytoplankton communities (Bittar et al., 2016; Zoppini et al., 2019). However, the impact of riverine input on marine microbial communities, especially the balance between chemoautotrophic and heterotrophic microbes, remains poorly understood. Moreover, it is not clear how terrigenous particles would impact various biochemical parameters that reflected the ecological functions of the estuarine ecosystem in the long term. This is largely attributed to the complex hydrodynamic processes present in natural environments and continuous terrigenous particle input. Interactions among different microbial groups are complex and easily influenced by the surrounding environments (Constable et al., 2014; Trevathan-Tackett et al., 2019). Because microorganisms play a fundamental role in mediating biogeochemical element cycles (Azam and Malfatti, 2007), it is necessary to learn how marine microbes respond to terrigenous organic matter input, especially under current situations where terrigenous organic matter are increasingly transported into the ocean.
To solve this problem, a large-volume and long-term indoor incubation experiment was set up for over 40 days to simulate the process that terrigenous particles sank to the seawater. A previous study based on this system found that nitrifiers are fundamental in maintaining a starved ecosystem (Zhang et al., 2021), but it is not clear whether chemoautotrophy remains important after terrigenous particle input. Further studies indicated that terrigenous organic matter influenced marine ecosystems, including increasing prokaryote abundance (Chen et al., 2022) and promoting organic matter transformation (Xiao et al., 2022). Recent results based on this system clearly explicated how microbes modified particles in the seawater (Zhang et al., 2022). The composition and activity of microbial communities are the central issues in microbial ecology (Falkowski et al., 2008; Schimel and Schaeffer, 2012; Aronson et al., 2013). However, the intricacies of how terrigenous particle input influences microbes, especially the balance between autotrophic and heterotrophic microorganisms and associated biogeochemical processes, are unclear. Based on the previous results in this system, this study further improves our understanding of the functional properties and biogeochemical cycles of the marine ecosystem under situations where increasing terrigenous particles will be transported to the seawater.
2. Materials and methods
2.1. Experiment setup
To study the responses of marine ecosystems to terrigenous POM input, a long-term and large-volume indoor incubation experiment was set up (Figure S1). About 100,000 L of seawater was collected from the coast of Halifax to fill the Aquatron tower tank (https://www.dal.ca/dept/aquatron.html). After 80 days, about 20,000 L of river water from the Ingramport River was gently added to the surface of the seawater. Both seawater and river water were filtered with 300-μm pore size bolting cloth before being added to the Aquatron tower tank. The incubation was conducted entirely under dark conditions to avoid disturbances caused by photoautotrophic activities. This study chose the 40-day phases before and after adding freshwater for a total study period of 80 days (Figure S1). A previous study indicated that there was little change in dissolved inorganic nitrogen (DIN) and microbial community structures during the 40 days before the addition of freshwater, reflecting that the system was relatively steady (Zhang et al., 2021), thus allowing this phase to be used as background. This study only focused on the dynamics of various ecological parameters in the seawater layer.
2.2. Sample collection and biogeochemical analysis
Time-series sample was collected from 1 m above the bottom for the following analysis of physicochemical parameters and characteristics of POM. Details on sample collection and measurements were described in previous studies (Zhang et al., 2021; Zhang et al., 2022).
Briefly, about 40-ml water samples were filtered with 0.45-μm pore size polyvinylidene difluoride (PVDF) filters (Millipore). Nutrients , , and were then measured using the Skalar SAN++ autoanalyzer at Dalhousie University. We used the Winkler method to measure the concentration of dissolved oxygen (DO) and the biological oxygen demand (BOD) (Carpenter, 1965). BOD5 was the reduction in DO after a 5-day dark incubation period (Grigoryeva et al., 2020). The chemical oxygen demand (COD) was recorded using the alkaline potassium permanganate method (Liu et al., 2018). Temperature and salinity were recorded using Multiparameter Sonde (YSI EXO, YSI Inc.).
Seawater samples for fluorescent dissolved organic matter (FDOM) analysis were collected after filtering through precombusted (450°C, 6 h) GF/F (GF refers to Glass Microfiber and F represents the pore size is 0.7 µm) membrane and stored in 40-ml Amber-certified volatile organic analysis (VOA) vials (Thermo Scientific, USA). The sample was analyzed with the Yvon Horiba Aqualog system. Five parallel factor analysis (PARAFAC) components (C1–C5) were decomposed and validated. The positions of C1–C5 were 319/440 nm, 304/380 nm, <240/523 nm, 388 (250)/487 nm, and 271/329 nm, respectively. Therefore, C1–C4 were cataloged as humic-like components and C5 as a protein-like component. Please refer to the original work for more details about sample detection and analysis (Xiao et al., 2022).
2.3. Biological sample collection and measurements
To collect microbial communities, seawater was successively filtered through 3-, 0.8-, and 0.2-μm pore size polycarbonate membranes (47-mm diameter; Millipore) at a pressure of no more than 0.03 MPa. Membranes were stored in RNase-free tubes, and samples used for active community analysis were filled with RNAlater (RNA stabilization solution, Ambion). All membranes were kept at -20°C until nucleic acid extraction. Please refer to the original work for detailed methods (Zhang et al., 2021; Zhang et al., 2022). Nucleotide sequence datasets used in this study are available in the NCBI Sequence Read Archive under the accession number PRJNA751271 (Zhang et al., 2022).
2.4. Statistical analysis
The variations in bacterial communities at the genus level under the constraint of biogeochemical parameters were analyzed by redundancy analysis (RDA). Variables with variance inflation factor (VIF) >10 were removed, and the remaining factors were used for further analysis. The statistical significance of parameters was estimated using Monte Carlo permutation tests (999 permutations), and only significant explanatory variance (p < 0.05) was shown in the plots.
β-diversity is an important index reflecting the variation in species composition among sites and can be the result of turnover (species replacement between sites) and nestedness (species loss or gain from site to site) (Baselga and Orme, 2012). To determine how terrigenous particle input impact community diversity, we used the betapart package in R to calculate turnover and nestedness components (Baselga and Orme, 2012). The significance of the difference between the stabilization (before freshwater treatment) and stratification phases (after freshwater treatment) was calculated using T-test.
The changes in the relative abundance of the bacterial groups during incubation were divided into increase (p < 0.05), decrease (p < 0.05), and no significant change (p > 0.05) based on the results of the linear fitness.
The correlation among different bacterial groups was calculated using FastSpar (Watts et al., 2019) with a bootstrap procedure repeated 1,000 times. Only robust (correlation coefficient ≥0.8 or ≤-0.8) and statistically significant (p < 0.01) correlations were considered. The topological roles of the different groups can be described by within-module connectivity (Zi) and among-module connectivity (Pi) (Guimera and Nunes Amaral, 2005). Zi and Pi were calculated in R (Cao et al., 2018). According to the values of Zi and Pi, the nodes in a network were divided into four subcategories: peripheral nodes (Zi ≤ 2.5, Pi ≤ 0.62), connectors (Zi ≤ 2.5, Pi > 0.62), module hubs (Zi > 2.5, Pi ≤ 0.62), and network hubs (Zi > 2.5, Pi > 0.62) (Guimera and Nunes Amaral, 2005; Zhou et al., 2011).
Ecological functional profiles of bacterial communities were predicted using FAPROTAX (Louca et al., 2016). Nonmetric multidimensional scaling (NMDS) analysis based on Bray–Curtis dissimilarities of predicted functional profiles was used to determine the β-diversity of the different samples.
To link microbial diversity with environmental variables and biogeochemical processes, partial least squares path modeling (PLS-PM) was performed using package plspm (version 0.4.9) in R (Gao et al., 2019; Tian et al., 2019). Variables used in PLS-PM analysis were divided into eight blocks: α-diversity (Simpson, Shannon, and Chao1), β-diversity (scores of the first two NMDS axes as numeric variables for bacterial community composition), DIN (including ammonium, nitrite, and nitrate), particles (concentration of POC, ratio of POC:PN, and δ13C of POM), BOD, COD, humic-like components (PARAFAC components C1–C4), and protein-like components (PARAFAC component C5). Variables with VIFs <10 were selected to do PLS-PM analysis. Variables with loadings <0.7 based on the results of initial PLS-PM structure equation were removed, and the remaining variables were used to perform the final PLS-PM structure equation (Gao et al., 2019). R2 and the goodness of fit (GOF) were used to evaluate the quality of the model.
3. Results
3.1. Bacterial community composition
The bacterial community structure was investigated based on DNA and RNA libraries from three size fractions at the genus level. In both DNA and RNA libraries, the relative abundance of Unclassified Rhodospirillaceae was higher in the <3-μm-size fractions than that in the >3-μm-size fractions, while the reverse was true for Planctomyces (Figure 1). A peak in relative abundance appeared on day 11 after terrigenous particle input in the <3-μm-size fractions for Stenotrophomonas, Unclassified Enterobacteriaceae, and Enterobacter when the concentration of POC in the seawater also reached a peak (Figures 1; S2). The highest relative abundance of these groups occurred on day 1 in the >3-μm-size fractions due to a faster sinking speed (Figure 1). However, this situation only appeared in DNA libraries, and the relative abundances of these groups were very low throughout the experiment in the RNA libraries (Figure 1). With the input of terrestrial particles, the relative abundance of Porticoccus and Unclassified Moraxellaceae increased, especially in the <3-μm-size fractions based on both DNA and RNA libraries (Figure 1). In contrast, the relative abundances of Schlesneria and Zavarzinella reached their peaks on day 11 in the >3-μm-size fractions in both DNA and RNA libraries (Figure 1). It was worth noting that the relative abundance of Nitrospina decreased with incubation in the DNA and RNA libraries (Figure 1). OM27 clade also showed a significant decreased trend over time in >0.8-μm-size fractions based on the DNA and RNA libraries (Figure 1). Terrigenous particles caused the dynamics of microbial communities in the seawater.
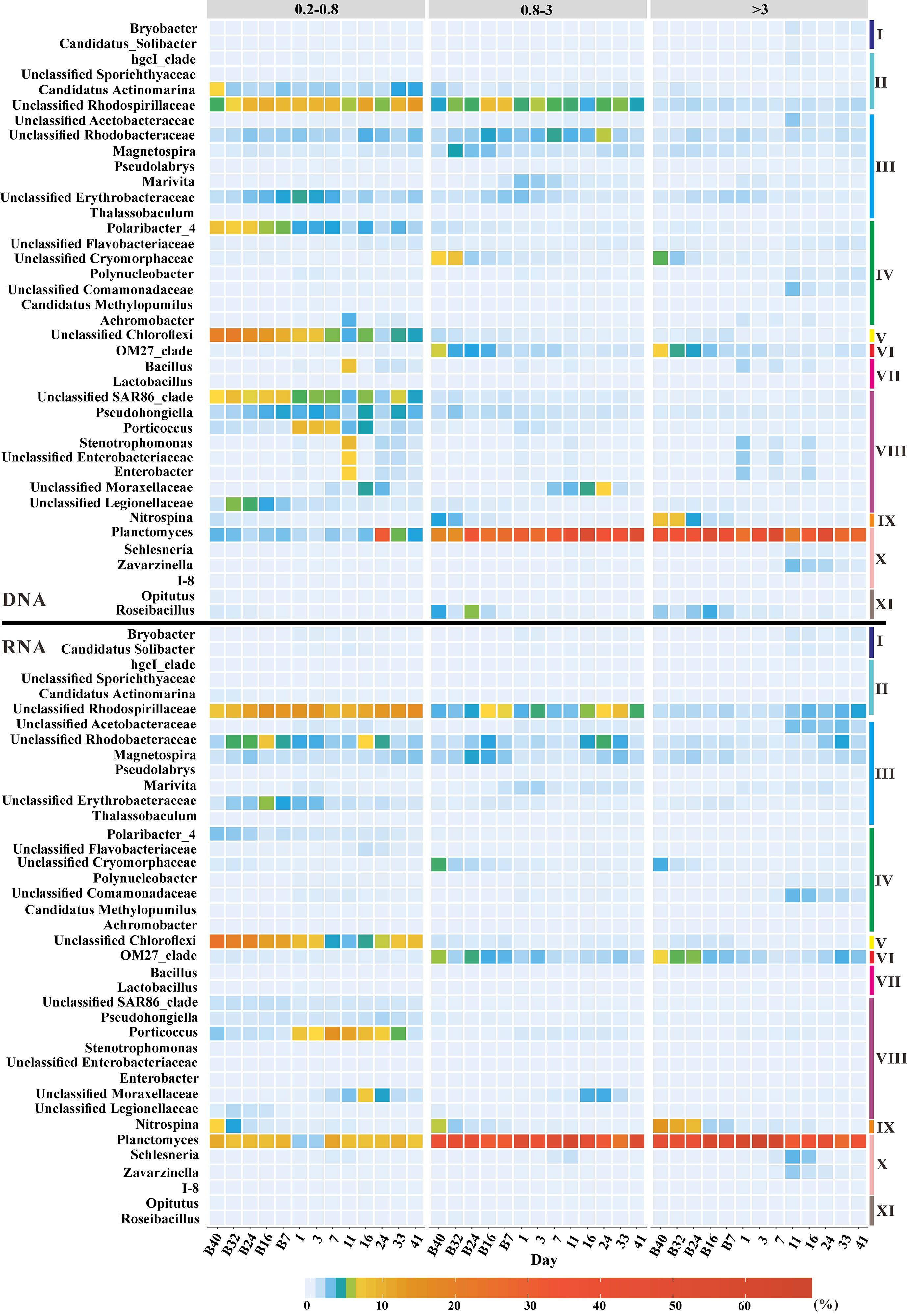
Figure 1 Bacterial communities at the genus level based on DNA (top panel) and RNA (bottom panel) libraries from the 0.2–0.8-µm-, 0.8–3-µm-, and >3-µm-size fractions. Groups with relative abundance of not less than 5% in at least one sample were shown in the graph. I, Acidobacteria; II, Alphaproteobacteria; III, Bacteroidetes; IV, Betaproteobacteria; V, Chloroflexi; VI, Deltaproteobacteria; VII, Firmicutes; VIII, Gammaproteobacteria; IX, Nitrospinae; X, Planctomycetes; XI, Verrucomicrobia.
3.2. Relationships between bacterial community and biogeochemical parameters
The relationships between bacterial communities and biogeochemical variables were assessed by RDA (Figure 2). According to the RDA scores, concentrations of nitrate and POC were significant factors determining the distribution of bacterial communities in all libraries (Figure 2), except for POC in the 0.2–0.8-μm-size fraction RNA libraries (Figure 2D). Additionally, δ15N of POM significantly contributed to the variation in the bacterial communities of 0.8–3-μm DNA libraries (Figure 2B) and 0.2–0.8-μm RNA libraries (Figure 2D). Concentrations of ammonium and BOD5 were also significant variables explaining the pattern of the bacterial community structures in the 0.2–0.8-μm RNA libraries (Figure 2D). Nitrite concentration and δ13C of POM only impacted the bacterial communities in the >3-μm RNA libraries (Figure 2F).
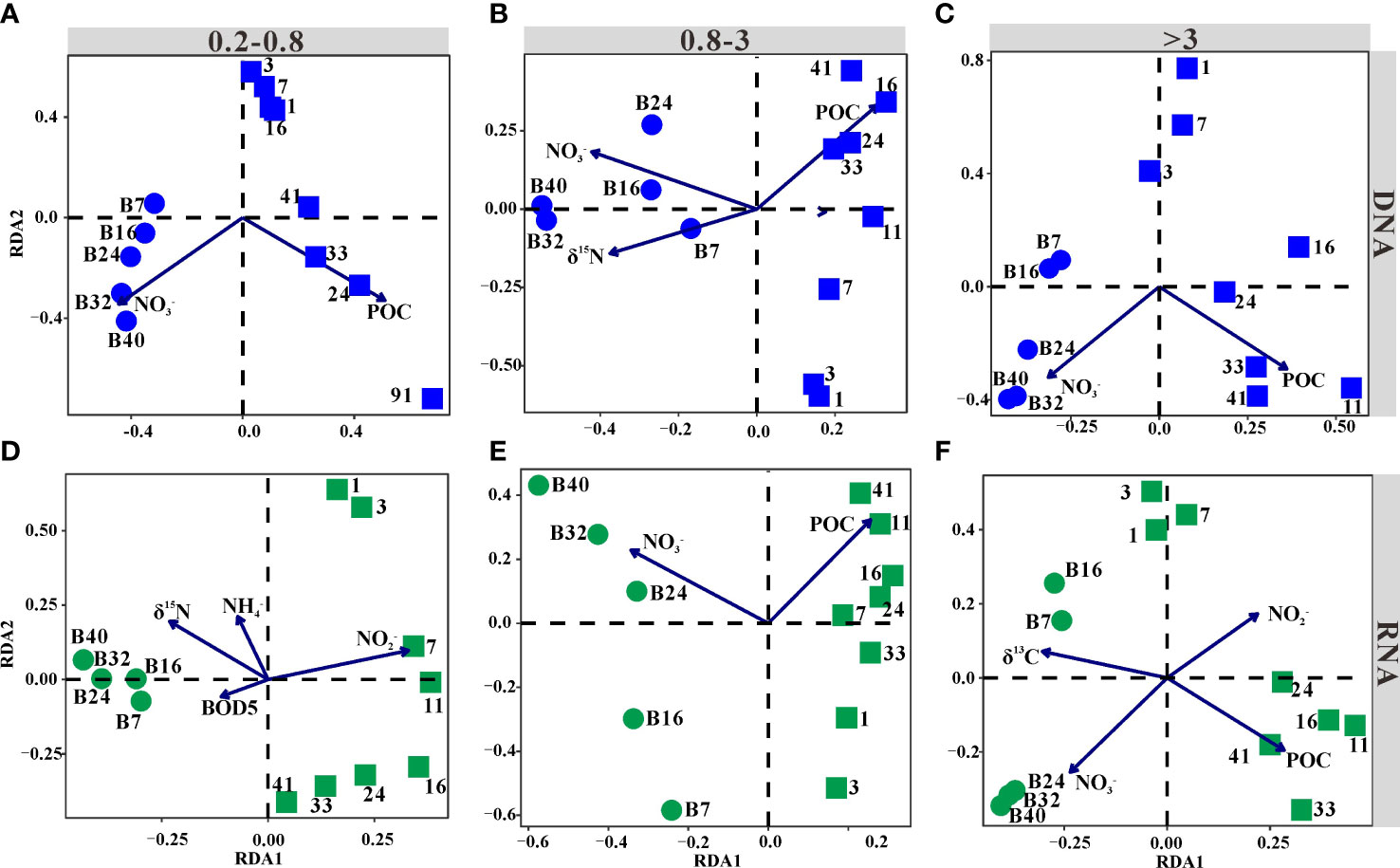
Figure 2 Ordination plots of the results from the redundancy analysis (RDA). Blue points represented DNA libraries, while green points represented RNA libraries. Communities were from the 0.2–0.8-μm (A, D)-, 0.8–3-μm (B, E)-, and >3-μm (C, F)-size fractions (p < 0.05). The circle and square represented samples collected before and after the addition of freshwater, respectively.
Importantly, samples were divided into two groups along the first axis. Samples collected before and after adding freshwater formed distinct clusters (Figure 2). The bacterial communities collected after adding freshwater were positively correlated with POC but negatively correlated with nitrate that was the production of nitrification, except in the 0.2–0.8-μm RNA libraries. In comparison, samples collected before adding freshwater exhibited a completely opposite pattern (Figure 2).
3.3. Nestedness and turnover components of β-diversity
β-diversity can be divided into two components, namely, turnover and nestedness. The former reflects species replacement, whereas the latter refers to β-diversity attributable to species loss or gain (Baselga, 2010). The mean value for pairwise βturnover was larger than that for pairwise βnestedness, regardless of size fraction or incubation time (Figure 3). This indicated that the dissimilarities among samples over time were dominated by in situ species replacement, instead of terrigenous particle-attached microbe input. The average βnestedness of the stabilization phase increased with size based on both DNA and RNA libraries (Figure 3). The average βnestedness of the stratification phase slightly increased with size based on DNA libraries, whereas the differences among the three size fractions were little based on RNA libraries (Figure 3).
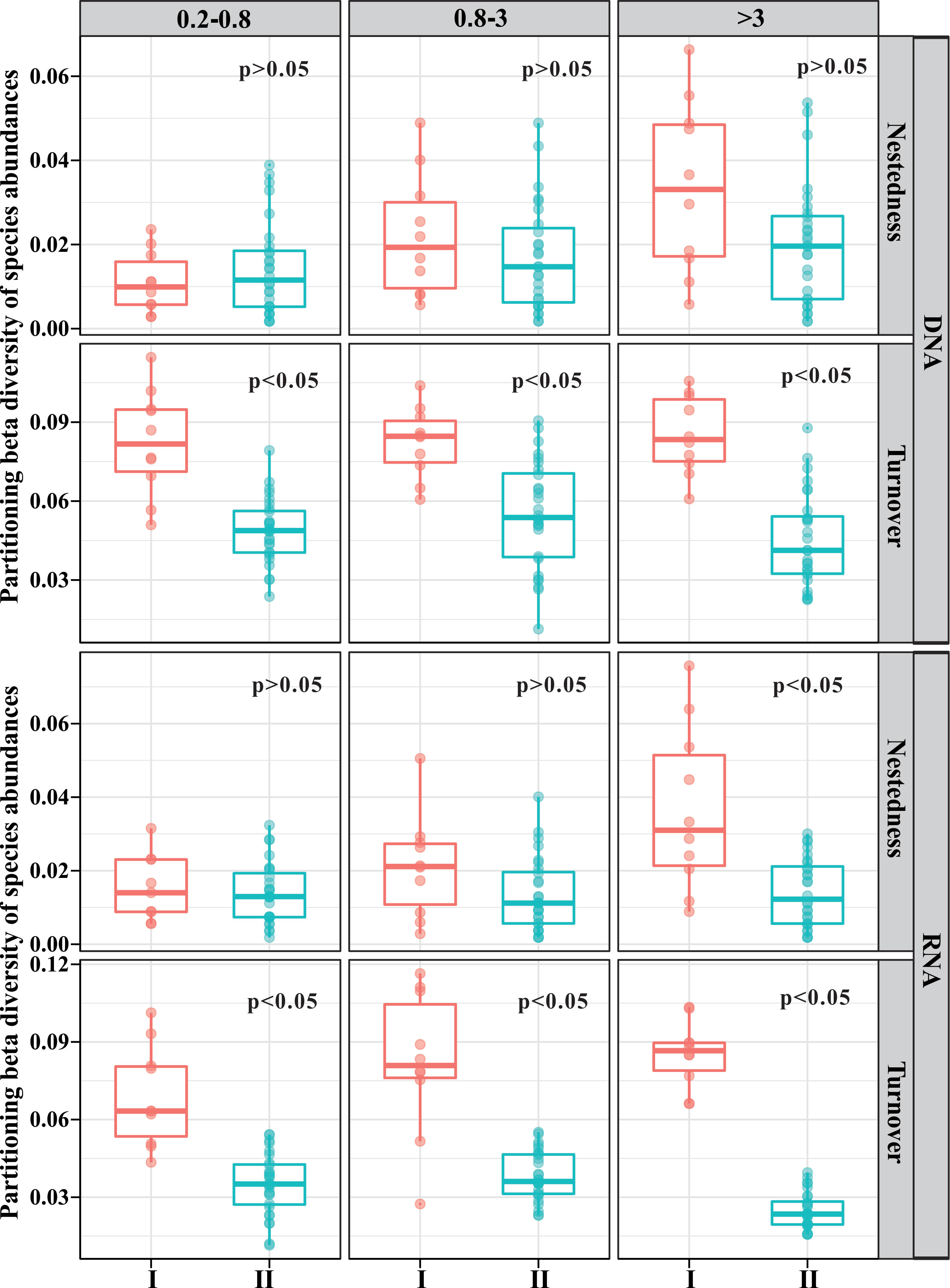
Figure 3 Pairwise turnover and nestedness component of β-diversity across incubation time. I, stabilization phase; II, stratification phase.
The average βturnover of stabilization showed little differences among the three size fractions based on DNA libraries, while it displayed an increasing trend with size based on RNA libraries (Figure 3). The βturnover of stratification was almost the same between 0.2–0.8-μm- and 0.8–3-μm-size fractions, which was obviously higher than that in the >3-μm-size fractions based on DNA and RNA libraries (Figure 3).
Notably, βturnover was higher in the stabilization phase compared to the stratification phase in all size fractions (Figure 3). βnestedness was higher in the stabilization phase compared to the stratification phase in only >3-μm-size fractions based on RNA libraries, while the βnestedness did not show significant differences between the two phases in other libraries (Figure 3).
3.4. Detection of the topological roles of nodes for the bacterial network
The topological roles of the groups identified in the six networks were shown as a Zi-Pi plot (Figure 4). It was observed that most of the groups were peripherals, with most of their links inside their modules. Furthermore, among these peripherals, plenty of nodes (38% for 0.2–0.8 μm, 36% for 0.8–3 μm, and 28% for >3 μm in DNA libraries; 38% for 0.2–0.8 μm, 33% for 0.8–3 μm, and 23% for >3 μm in RNA libraries) had no links outside their own modules (Pi = 0) (Figure S3). Most of these nodes were affiliated to groups that did not significantly change with incubation, except in the >3-μm-size fraction DNA libraries, where almost half of the nodes were assigned to groups that increased significantly with time (Figure S3). It was worth noting that the percentage of these nodes decreased with size fraction in both DNA and RNA libraries (Figure S3). It might be that microbial communities from large size fractions were easily impacted by terrigenous particle input. Moreover, the percentage of nodes without links outside their own modules was higher in DNA libraries than that in RNA libraries of >0.8-μm-size fractions, while the difference was small in the 0.2–0.8-μm-size fraction (Figure S3). The connectors (nodes linking different modules) could be regarded as keystone nodes that play crucial roles in shaping the network structure (Banerjee et al., 2018). Based on the DNA libraries, 19 (14%), 8 (6%), and 3 (2%) groups were identified as connectors in the 0.2–0.8-μm-, 0.8–3-μm-, and >3-μm-size fractions, respectively (Figure 4; Table S1). For the RNA libraries, 5 (4%), 46 (35%), and 1 (1%) group was identified as connectors in the 0.2–0.8-μm-, 0.8–3-μm-, and >3-μm-size fractions, respectively (Figure 4; Table S2). Among these keystone nodes, groups that significantly decreased with time were dominant in the <3-μm DNA and >3-μm RNA libraries (Tables S1, S2). In comparison, groups that significantly increased or decreased significantly over time were dominant in the >3-μm DNA and <3-μm RNA libraries (Tables S1, S2). Furthermore, no network hubs and module hubs were observed in all of the networks (Figure 4).
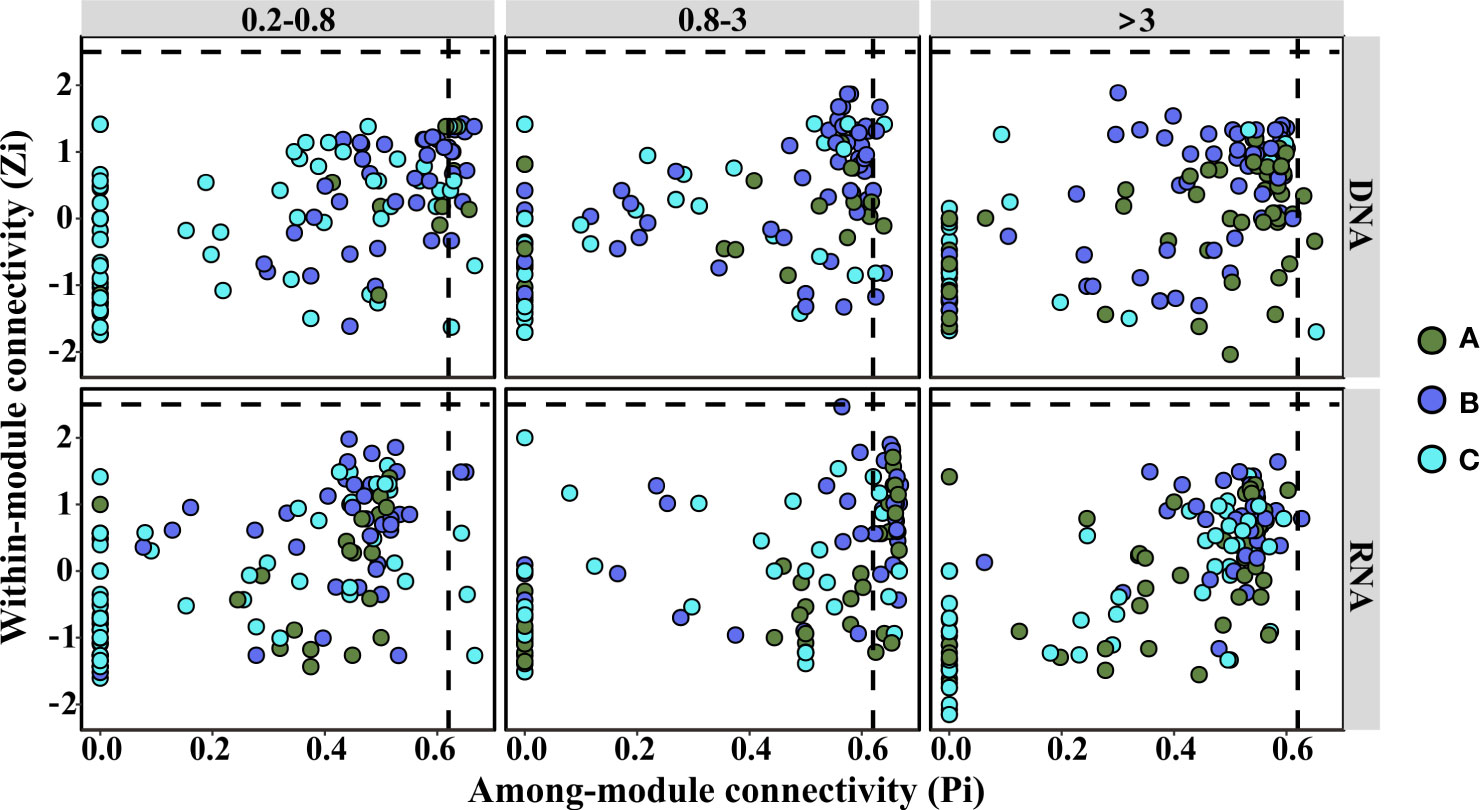
Figure 4 Zi-Pi plot showing the distribution of groups based on their topological roles. Dots represent bacterial groups with different trends over the incubation period. (A) Increasing (p < 0.05); (B) decreasing (p < 0.05); (C) not significant (p > 0.05). The horizontal dashed line represented a Zi of 2.5, while the vertical dashed line represented a Pi of 0.62.
3.5. Changes of ecological functions of bacterial communities
Two-dimensional NMDS ordination was used to illustrate the similarities among predicted bacterial functional profiles. The functional profiles were clearly divided into three groups according to size fraction (Figures 5A, B). Notably, samples collected from the stabilization phase tended to cluster together, while samples from the stratification phase formed another cluster (Figures 5A, B). Moreover, the differences between 0.2–0.8-μm- and 0.8–3-μm-size fractions were larger than those between 0.8–3-μm- and >3-μm-size fractions (Figures 5A, B).
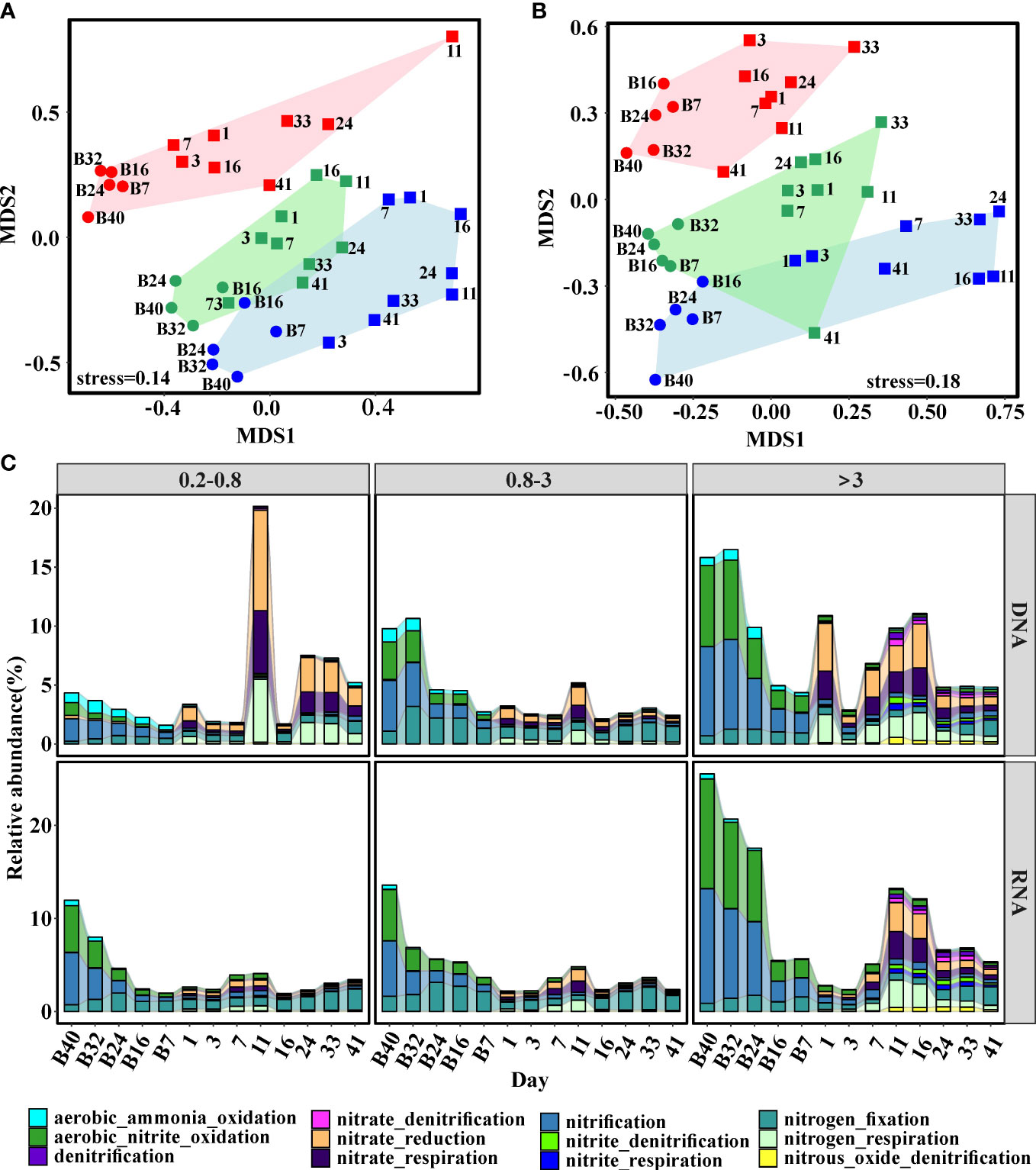
Figure 5 Nonmetric multidimensional scaling (NMDS) of bacterial function composition based on Bray–Curtis dissimilarities. (A) DNA libraries; (B) RNA libraries. Points represent bacterial groups from different size fractions. Red: 0.2–0.8 μm; Green: 0.8–3 μm; Blue: >3 μm. (C) Changes of genes involved in the predicted nitrogen metabolism. Functional profiles were predicted by FAPROTAX. The circle and square represented samples collected before and after the addition of freshwater, respectively.
A previous study indicated that nitrification played a fundamental role in the driving succession of active communities and biogeochemical elements cycle before terrigenous particles addition (Zhang et al., 2021); thus, it was necessary to explore functional profiles of bacterial communities associated with nitrogen. Clearly, the metabolism related to nitrogen changed after terrigenous particles sank to the seawater (Figure 5C). In the stabilization phase, chemoautotrophy (nitrification, ammonia oxidation, and nitrite oxidization) was the dominant process involved in nitrogen metabolism (Figure 5C). With the input of terrigenous particles, metabolism related to nitrogen shifted from chemoautotrophy to heterotrophy (Figure 5C). During the stabilization phase, ammonium and nitrite were oxidized to nitrate by nitrifiers, suppling energy and fresh organic carbon to the system. After terrigenous particles sank to the seawater, energy from the degradation of terrigenous organic carbon supported this system and heterotrophy became the dominant process (Figure 5C).
3.6. The direct and indirect effects of terrigenous particles on various ecological parameters
To explore the direct and indirect effects of terrigenous particles on various ecological parameters, we conducted PLS-PM structure equation models. Terrigenous particles greatly affected nutrients via direct (path coefficient = 1.07) and indirect (path coefficient = -1.16) ways (Figure 6B). This indicated that although the total effect of terrigenous particles on nutrients was tiny (path coefficient = -0.08), the dynamics of nutrients were deeply impacted by terrigenous particles. Besides nutrients, humic-like and protein-like components were also deeply impacted by terrigenous particles (Figure 6B). The humic-like components received more indirect (path coefficient = 0.93) than direct (path coefficient = -0.25) contributions from terrigenous particles, and the total effect was positive (path coefficient = 0.68) (Figure 6B). The opposite situation occurred in the protein-like component, and the total effect of terrigenous particles on this component was negative (path coefficient = -0.52) (Figures 6A, B). Terrigenous particles had more direct effects on the protein-like component than the humic-like components, whereas the situation was opposite for indirect effects (Figure 6B). It was interesting to note that terrigenous particles had a positive impact on the β-diversity but a negative impact on the α-diversity of bacterial communities (Figures 6A, B). The positive direct effect of terrigenous particles on BOD (path coefficient = 0.45) was greater than their negative indirect effect (path coefficient = -0.31), leading to an overall positive impact of terrigenous particles on BOD (Figures 6A, B).
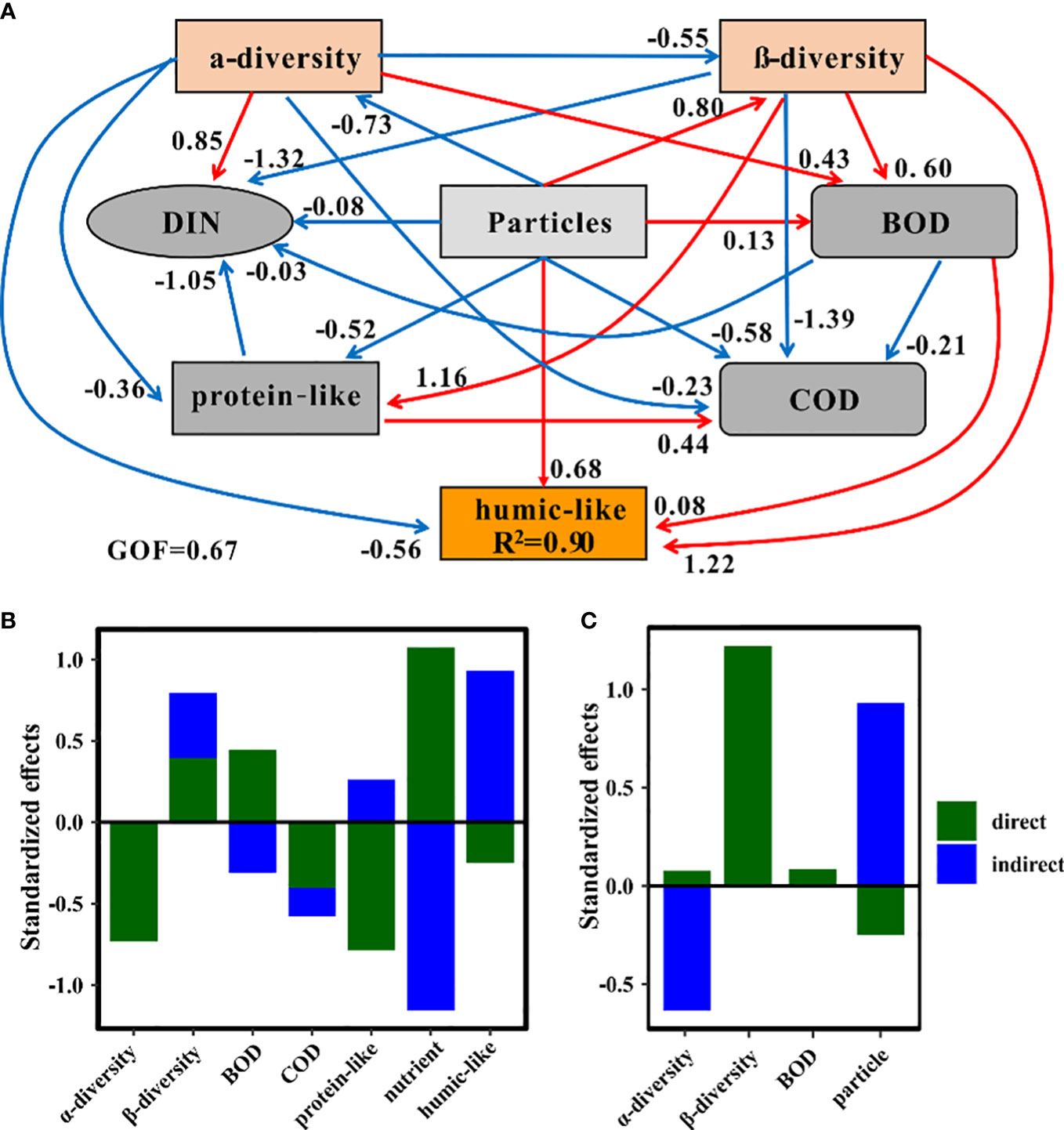
Figure 6 Partial least squares path models showing the effects of terrigenous particles on other variables. (A) Effects among different factors monitored in this system based on partial least squares path analysis. GOF, goodness of fit. The blue arrows represent the negative effect, while the red arrows indicate the positive effect. (B) Standardized effects of particles on other factors. (C) Standardized effects of factors on the humic-like components.
The relative intensity of the humic-like components was well explained by our block variables (R2 = 0.90) (Figure 6A). Among these variables, the β-diversity of bacterial communities was the most important factor affecting the humic-like components (path coefficient = 1.22) (Figure 6C). The terrigenous particles were also a key factor influencing the humic-like components (Figure 6C). Although terrigenous particles could indirectly increase the relative intensity of this component, they simultaneously reduced the relative intensity of the humic-like components through direct ways (Figure 6C). The α-diversity of bacterial communities also exhibited an important contribution to the humic-like components through mainly indirect effects (Figure 6C). Overall, the β-diversity of bacterial communities, terrigenous particles, and BOD had positive impacts while the α-diversity of bacterial communities had negative impacts on the humic-like components (Figures 6A, C).
4. Discussion
4.1. Impacts of terrigenous particulates on the bacterial diversity and community structures in seawater
Microorganisms are the foundation of ecosystems and regulate global biogeochemical cycles (Falkowski et al., 2008). Not only are marine microbes largely determined by terrigenous organic matter input (Bittar et al., 2016; Zoppini et al., 2019), they also impact the cycle of carbon and other elements (Falkowski et al., 2008; Schimel and Schaeffer, 2012; Aronson et al., 2013). However, previous studies only divided the microbial groups into autotrophic and heterotrophic subgroups, without finer taxonomic information about microbial communities (Wikner and Andersson, 2012). The influences of terrigenous input on microbial communities were also uncertain. This study clearly illustrated how terrigenous particles changed bacterial communities at the genus level (Figure 1). Previous studies found that the genus Stenotrophomonas was particularly closely associated with terrestrial plants (Ryan et al., 2009). The changes in relative abundance of Stenotrophomonas, Unclassified Enterobacteriaceae, and Enterobacter supported that terrigenous sinking particles carried bacteria from the river to the seawater, but they did not remain active in the seawater (Figure 1).
The value of turnover was higher than that of nestedness in all size fractions (Figure 3). This phenomenon was consistent with most studies that the dominant component has been turnover in various ecosystems (Soininen et al., 2018). High βturnover suggested that a difference in richness played a smaller role in generating β-diversity patterns, while compositional dissimilarities of communities between samples were the dominant factors influencing β-diversity (Soininen et al., 2018). Terrigenous particles can change the β-diversity in seawater through two ways: 1) river particle-attached bacteria can be transported to the seawater by sinking terrigenous particles; 2) terrigenous particles are an important organic matter source, and the input of organic matter will change the bacterial community (Sipler et al., 2017; Figueroa et al., 2021). The input of terrigenous POM significantly decreased the average βturnover (Figure 3) without significant impacts on the average βnestedness (except >3 μm, RNA libraries), implying that the input of terrigenous particles changed the relative abundance of the original bacterial groups. This was the dominant way for terrigenous particles to impact the bacterial community structure in the seawater. Although terrigenous particles can carry river bacteria to the seawater, this has minor effects on marine bacterial communities.
4.2. Impacts of terrigenous particles on the balance between chemoautotrophic and heterotrophic microbes
The RDA showed that POC was a significant environment factor that structured bacterial communities after the addition of freshwater, while nitrate holds the main role in explaining bacterial community variations before adding freshwater (Figure 2). A previous study indicated that nitrification performed by nitrifiers played a fundamental role in maintaining this starved ecosystem (Zhang et al., 2021), and there was no doubt that nitrate was a significant factor shaping bacterial communities before adding freshwater. Functional profiles also supported that nitrification was the dominant metabolism before adding freshwater (Figure 5). However, the metabolism related to nitrogen shifted from chemoautotrophy to heterotrophy with the input of terrigenous POM. Terrigenous particles carried nutrients to the seawater, which might promote phytoplankton productivity (Smith, 2006). Further research showed that riverine discharge had a negative effect on phytoplankton production and a positive effect on heterotrophic production (Wikner and Andersson, 2012). It is hard to study the impacts of terrigenous particles on chemoautotrophic microorganisms in the field because of the disturbance caused by light and continuous terrigenous particle input. This study found that although terrigenous particles transported DIN to the seawater that might promote the activity of chemoautotrophic microorganisms, this process was not as important as the enhanced activity of heterotrophic microorganisms caused by terrigenous organic matter input. Because the concentration of DIN was lower in freshwater than that in seawater, terrigenous particles could transport little nutrient to the seawater. Moreover, heterotrophic microorganisms can assimilate ammonium (e.g., SAR86) (Middelburg and Nieuwenhuize, 2000; Dupont et al., 2012), and previous studies proved that nitrifiers were less competitive for ammonium (Verhagen et al., 1992; Van Niel et al., 1993). Thus, terrigenous particles obviously promoted the activity of heterotrophic but not autotrophic bacteria.
Sixty-five groups were identified as connectors of the network (Figure 4; Tables S1, S2) and could be regarded as keystone nodes (Banerjee et al., 2018). Although some of the keystone groups were rare, previous studies documented that rare microorganisms were important for many biogeochemical processes (Banerjee et al., 2018). Some keystone nodes were shared between DNA and RNA libraries and accounted for 60% and 20% of all keystone nodes in these two libraries, respectively (Tables S1, S2). This implied that connectors in the RNA-based network made much tighter interactions among modules than those in the DNA-based network, which confirmed that the network structures were different between these two libraries.
Nearly 30% of network nodes were affiliated to groups that did not significantly change with incubation (Tables S1, S2). Previous opinions believed that keystone taxa were consistently present regardless of changes in environmental conditions (Shade and Handelsman, 2012). More than 40% of network nodes were involved in groups that decreased significantly over time, and these nodes were dominated by proteobacteria, many of which were nitrifiers. A previous study showed that nitrifiers played a fundamental role in maintaining this starved ecosystem (Zhang et al., 2021). This study also showed that nitrifiers (Nitrosomonas, Nitrosospira, and Nitrospira) were involved in keystone groups (Tables S1, S2). However, their importance to the ecosystem was weakened after terrigenous particles sank to the seawater, apparent in their significant decrease with time (Figure 1). Nearly 30% of the keystone groups increased significantly with incubation. These keystone groups were mainly affiliated with Bacteroidetes, Planctomycetes, and Proteobacteria (Tables S1, S2). Bacteroidetes are generally specialized in degrading high-molecular weight compounds and prefer to attach to particles (Fernández-Gomez et al., 2013). Planctomycetes are also important in particle degradation (Orsi et al., 2016). These groups were pioneers in particle degradation and supply substrates to others, thus they played a keystone role in maintaining the bacterial communities. This indicated that the metabolisms involved in the particle degradation were key processes in this system. Terrigenous sinking particles carried organic carbon to the seawater, and there was no doubt that POC was the significant factor shaping bacterial community structures during the stratification phase (Figure 2).
It was worth mentioning that the abundant group SAR86 (Figure 1), a clade of proteobacteria, was a connector in the networks (Tables S1, S2). It significantly decreased in DNA libraries (Table S1) but did not change significantly in RNA libraries (Table S2). Studies about this clade indicated that it could degrade lipids and carbohydrates, but the genomes of SAR86 lack nitrate reductase and nitrite reductase (Dupont et al., 2012). The stable relative abundance of this clade in the RNA libraries implied that terrigenous particles impacted the bacterial activity via synergistic interactions among nutrients and organic matter.
Terrigenous particles can alter keystone groups and impact whole bacterial communities. A previous study showed that terrigenous input increased the production of heterotrophic instead of photosynthetic microorganisms (Wikner and Andersson, 2012). This study further supported that terrigenous particles weakened the importance of chemoautotrophic microorganisms in marine ecosystems, and increased riverine discharge would lead to domination of the food web by heterotrophic microorganisms.
4.3. Impacts of terrigenous particles on various ecological parameters in seawater
Changes in ecological function induced by terrigenous particles will impact diverse ecological parameters in seawater. Bacteria often display “feast and famine” strategies (Pedler et al., 2014; Sebastián et al., 2019), where the input of sinking particles can promote special species to grow rapidly, thus reducing the α-diversity of bacterial communities (Figures 6A, B). β-diversity represents the change in species diversity between communities. The results of RDA indicated that terrigenous particles were a significant factor shaping bacterial communities during the stratification phase (Figure 2). On one hand, terrigenous particles carried riverine bacterial communities to the seawater, where most of them disappeared with incubation, causing divergences and increasing the β-diversity of bacterial communities. On the other hand, as discussed above, opportunistic groups grew fast under the stimulation of terrigenous particles and dominated the bacterial communities, reducing the β-diversity of bacterial communities.
In addition to the bacterial community, terrigenous particles can strongly impact many physicochemical parameters. Terrigenous particles carried and released biologically available organic matter when they entered oceans (Butman et al., 2007; Bianchi, 2011), thus terrigenous particles directly and positively impacted BOD (Figure 6B). Moreover, the priming effect caused by the addition of active terrigenous POM promoted microbes to mineralize in situ organic matter and cause the depletion of organic matter (Turnewitsch et al., 2007; Bianchi, 2011), illustrated by the indirect and negative impacts of terrigenous particles on BOD and COD (Figure 6B). Furthermore, previous studies showed that sinking particles could be the core for dissolved organic matter (DOM) to attach (Hwang and Druffel, 2003; Roland et al., 2008). This scavenging process transported organic matter from the water column to the sediments, causing a decrease in COD. Therefore, terrigenous particles had a direct and negative impact on COD (Figure 6B).
Terrigenous POM was composed of numerous components (Tremblay et al., 2011). The input of terrigenous particles directly diluted the relative intensity of humic- and protein-like components. However, a previous study based on this system indicated that terrigenous particles promoted the growth of prokaryotic microorganisms (Chen et al., 2022), which likely produced more protein-like compounds. Therefore, terrigenous particles also indirectly and positively impacted the protein-like component (Figure 6B). Interactions among different components in FDOM can potentially take place. It was found that although the fluorescence of protein-like components was largely quenched by the humic-like components, the fluorescence of humic-like components was not influenced by the protein-like components (Wang et al., 2015). Thus, the impacts of terrigenous particles on protein-like components might be stronger.
Previous studies showed that terrigenous particles could carry nutrients to the ocean (Seitzinger et al., 2005), so there was no doubt that nutrients were directly and positively contributed by terrigenous particles (Figure 6B). Enhanced nutrients (ammonium and nitrate) could increase organic matter transformation (Jiao et al., 2010), causing the depletion of organic matter in the system. Heterotrophic bacteria with nitrate reductase genes can use dissolved nutrients (Jiang and Jiao, 2016), reducing the concentration of DIN. The predicted function profiles also showed that nitrate reduction played a more important role after terrigenous particles sank to the seawater (Figure 5C). The utilization of terrigenous organic matter stimulated nitrate reduction, thus terrigenous particles indirectly and negatively affected the nutrients (Figure 6B).
Marine DOM is approximately equivalent to the stock of carbon resident in atmospheric CO2 (Hansell et al., 2009; Quéré et al., 2018), and therefore even a tiny disturbance to it can have large implications for the global carbon cycle (Lønborg et al., 2020). Thus, we paid special attention to the impacts of terrigenous particles on DOM, especially the humic-like component that was an important part of recalcitrant DOM (Cory and Kaplan, 2012; Catalá et al., 2015). Previous studies supported that high riverine discharge corresponded with changes in DOM quality, including an elevated proportion of humic-like FDOM (Walker et al., 2009; Bittar et al., 2016). A previous study based on this system showed that the production rate of the humic-like components increased after freshwater addition (Xiao et al., 2022). However, they did not find microbial evidence to explain this observation. This was illustrated by our results that indirect effects were more important than direct effects (Figures 6B, C). As discussed above, terrigenous particles carried nutrients and organic matter to the seawater, which then promoted the transformation of organic matter, ultimately causing the accumulation of humic-like FDOM components. Terrigenous particles promoted the activity of heterotrophic microorganisms and associated transformation of organic matter, thus causing the accumulation of humic-like components.
5. Conclusions
Although terrigenous particles can carry riverine microorganisms to the seawater, this study finds that the terrigenous matter derived from the particles was a more important factor than microbes in driving bacterial community structures in the seawater. Because the activity of keystone groups was largely correlated with terrigenous particles, it is likely that terrigenous particles determined the bacterial communities through shaping keystone groups. Terrigenous particles deeply impacted the balance between chemoautotrophic and heterotrophic groups and weakened the importance of chemoautotrophy in seawater. Coupled with results from previous studies, it can be inferred that increased riverine discharge would lead to an ecosystem dominated by heterotrophic microorganisms (Berglund et al., 2007; Wikner and Andersson, 2012). Humic-like compounds are thought to be important parts of recalcitrant organic carbon and play an essential role in the marine carbon cycle. Although terrigenous particles did carry humic-like compounds to the seawater, stimulated transformation of in situ organic matter in seawater caused by terrigenous nutrients and organic matter contributed more humic-like compounds. This will increase carbon sequestration in the seawater because these compounds are generally recalcitrant to microbial degradation. Our study implied that terrigenous particles transported nutrients and organic matter to the seawater and triggered a series of ecological dynamics. Because the river basin in this study is relatively undisturbed, future studies can consider ecosystems strongly impacted by human activities for a more general conclusion.
Data availability statement
The datasets presented in this study can be found in online repositories. The names of the repository/repositories and accession number(s) can be found below: https://www.ncbi.nlm.nih.gov/, PRJNA751271.
Author contributions
HS designed and coordinated the study. LZ, and YL performed the experiments. LZ and YL analyzed the data and wrote the manuscript with contributions from all co-authors. All authors contributed to the article and approved the submitted version.
Funding
This work was funded by Southern Marine Science and Engineering Guangdong Laboratory (Zhuhai) (SML2020SP004), the Key Research and Development Program of Shandong Province (2020ZLYS04) and National Key Research and Development Program of China (2018YFA0605800).
Acknowledgments
We sincerely thank TingYat Lee for his assistance in editing original manuscript. We also thank Nianzhi Jiao, Jihua Liu and Xilin Xiao for their support during this experiment.
Conflict of interest
The authors declare that the research was conducted in the absence of any commercial or financial relationships that could be construed as a potential conflict of interest.
Publisher’s note
All claims expressed in this article are solely those of the authors and do not necessarily represent those of their affiliated organizations, or those of the publisher, the editors and the reviewers. Any product that may be evaluated in this article, or claim that may be made by its manufacturer, is not guaranteed or endorsed by the publisher.
Supplementary material
The Supplementary Material for this article can be found online at: https://www.frontiersin.org/articles/10.3389/fmars.2022.1116286/full#supplementary-material
References
Aronson E. L., Allison S. D., Helliker B. R. (2013). Environmental impacts on the diversity of methane-cycling microbes and their resultant function. Front. Microbiol. 4. doi: 10.3389/fmicb.2013.00225
Azam F., Malfatti F. (2007). Microbial structuring of marine ecosystems. Nat. Rev. Microbiol. 5 (10), 782–791. doi: 10.1038/nrmicro1747
Banerjee S., Schlaeppi K., van der Heijden M. G. A. (2018). Keystone taxa as drivers of microbiome structure and functioning. Nat. Rev. Microbiol. 16 (9), 567–576. doi: 10.1038/s41579-018-0024-1
Baselga A. (2010). Partitioning the turnover and nestedness components of beta diversity. Global Ecol. Viogeog. 19 (1), 134–143. doi: 10.1111/j.1466-8238.2009.00490.x
Baselga A., Orme C. D. L. (2012). Betapart: an r package for the study of beta diversity. Methods Ecol. Evol. 3 (5), 808–812. doi: 10.1111/j.2041-210X.2012.00224.x
Bauer J. E., Cai W. J., Raymond P. A., Bianchi T. S., Hopkinson C. S., Regnier P. A. (2013). The changing carbon cycle of the coastal ocean. Nature. 5047478, 61–70. doi: 10.1038/nature12857
Berglund J., Müren U., Båmstedt U. (2007). Efficiency of a phytoplankton-based and a bacterial-based food web in a pelagic marine system. Limnol. Oceanog. 52 (1), 121–131. doi: 10.4319/lo.2007.52.1.0121
Bianchi T. S (2011). The role of terrestrially derived organic carbon in the coastal ocean: A changing paradigm and the priming effect. Proc. Natl. Acad. Sci. USA 108 (49), 19473–19481. doi: 10.1073/pnas.1017982108
Bianchi T. S., Wysocki L. A., Schreiner K. M., Filley T. R., Corbett D. R., Kolker A. S. (2011). Sources of terrestrial organic carbon in the Mississippi plume region: Evidence for the importance of coastal marsh inputs. Aquat. Geochem 17 (4), 431–456. doi: 10.1007/s10498-010-9110-3
Bittar T. B., Berger S. A., Birsa L. M., Walters T. L., Thompson M. E., Spencer R. G. M., et al. (2016). Seasonal dynamics of dissolved, particulate and microbial components of a tidal saltmarsh-dominated estuary under contrasting levels of freshwater discharge. Estuar. Coast. Shelf Sci. 182, 72–85. doi: 10.1016/j.ecss.2016.08.046
Butman D., Raymond P., Oh N. H., Mull K. (2007). Quantity, 14C age and lability of desorbed soil organic carbon in fresh water and seawater. Organic Geochem. 38 (9), 1547–1557. doi: 10.1016/j.orggeochem.2007.05.011
Canuel E. A., Cammer S. S., McIntosh H. A., Pondell C. R. (2012). Climate change impacts on the organic carbon cycle at the land-ocean interface. Annu. Rev. Earth Planet. Sci. 40 (685), 2012. doi: 10.1146/annurev-earth-042711-105511
Cao X., Zhao D., Xu H., Huang R., Zeng J., Yu Z. (2018). Heterogeneity of interactions of microbial communities in regions of taihu lake with different nutrient loadings: A network analysis. Sci. Rep. 8 (1), 1–11. doi: 10.1038/s41598-018-27172-z
Carpenter J. H. (1965). The Chesapeake bay institute technique for the winkler dissolved oxygen method. Limnol. Oceanog. 10 (1), 141–143. doi: 10.4319/lo.1965.10.1.0141
Catalá T. S., Reche I., Fuentes-Lema A., Romera-Castillo C., Nieto-Cid M., Ortega-Retuerta E., et al. (2015). Turnover time of fluorescent dissolved organic matter in the dark global ocean. Nat. Commun. 6 (1), 1–9. doi: 10.1038/ncomms6986
Chen X., Wei W., Xiao X., Wallace D., Hu C., Zhang L., et al. (2022). Heterogeneous viral contribution to dissolved organic matter processing in a long-term macrocosm experiment. Environ. Int. 158, 106950. doi: 10.1016/j.envint.2021.106950
Constable A. J., Melbourne-Thomas J., Corney S. P., Arrigo K. R., Barbraud C., Barnes D. K., et al. (2014). Climate change and southern ocean ecosystems I: How changes in physical habitats directly affect marine biota. Global Change Biol. 20 (10), 3004–3025. doi: 10.1111/gcb.12623
Cory R. M., Kaplan L. A. (2012). Biological lability of streamwater fluorescent dissolved organic matter. Limnol. Oceanog. 57 (5), 1347–1360. doi: 10.4319/lo.2012.57.5.1347
Dalzell B., Minor E., Mopper K. (2009). Photodegradation of estuarine dissolved organic matter: A multi-method assessment of DOM transformation. Organic Geochem. 40 (2), 243–257. doi: 10.1016/j.orggeochem.2008.10.003
Dupont C. L., Rusch D. B., Yooseph S., Lombardo M. J., Alexander Richter R., Valas R., et al. (2012). Genomic insights to SAR86, an abundant and uncultivated marine bacterial lineage. ISME J. 6 (6), 1186–1199. doi: 10.1038/ismej.2011.189
Falkowski P. G., Fenchel T., Delong E. F. (2008). The microbial engines that drive earth's biogeochemical cycles. Science 3205879, 1034–1039. doi: 10.1126/science.11532
Fernández-Gomez B., Richter M., Schüler M., Pinhassi J., Acinas S. G., González J. M., et al. (2013). Ecology of marine bacteroidetes: A comparative genomics approach. ISME J. 7 (5), 1026–1037. doi: 10.1038/ismej.2012.169
Fichot C. G., Benner R. (2014). The fate of terrigenous dissolved organic carbon in a river-influenced ocean margin. Global Biogeochem. Cycles. 28 (3), 300–318. doi: 10.1002/2013GB004670
Figueroa D., Capo E., Lindh M. V., Rowe O. F., Paczkowska J., Pinhassi J., et al. (2021). Terrestrial dissolved organic matter inflow drives temporal dynamics of the bacterial community of a subarctic estuary (northern Baltic Sea). Environ. Microbiol. 23 (8), 4200–4213. doi: 10.1111/1462-2920.15597
Gao X., Chen H., Govaert L., Wang W., Yang J. (2019). Responses of zooplankton body size and community trophic structure to temperature change in a subtropical reservoir. Ecol. Evol. 9 (22), 12544–12555. doi: 10.1002/ece3.5718
Grigoryeva N. I., Zhuravel E. V., Mazur A. A. (2020). Seasonal variations of water quality in the vostok bay, Peter the great gulf, the Sea of Japan. Water Res. 47 (2), 249–256. doi: 10.1134/s0097807820020062
Guimera R., Nunes Amaral L. A. (2005). Functional cartography of complex metabolic networks. Nature 4337028, 895–900. doi: 10.1038/nature03288
Hansell D. A., Carlson C. A., Repeta D. J., Schlitzer R. (2009). Dissolved organic matter in the ocean: A controversy stimulates new insights. Oceanography 22 (4), 202–211. doi: 10.5670/oceanog.2009.109
Hedges J. I., Keil R. G., Benner R. (1997). What happens to terrestrial organic matter in the ocean? Organic Geochem. 27 (5-6), 195–212. doi: 10.1016/s0146-6380(97)00066-1
Hirabayashi Y., Mahendran R., Koirala S., Konoshima L., Yamazaki D., Watanabe S., et al. (2013). Global flood risk under climate change. Nat. Climate Change. 3 (9), 816–821. doi: 10.1038/nclimate1911
Hutchins D. A., Capone D. G. (2022). The marine nitrogen cycle: new developments and global change. Nat. Rev. Microbiol. 20, 1–14. doi: 10.1038/s41579-022-00687-z
Hwang J., Druffel E. R. M. (2003). Lipid-like material as the source of the uncharacterized organic carbon in the ocean? Science 2995608, 881–884. doi: 10.1126/science.1078508
Jeandel C., Oelkers E. H. (2015). The influence of terrigenous particulate material dissolution on ocean chemistry and global element cycles. Chem. Geol. 395, 50–66. doi: 10.1016/j.chemgeo.2014.12.001
Jiang X., Jiao N. (2016). ). nitrate assimilation by marine heterotrophic bacteria. Sci. China Earth Sci. 59 (3), 477–483. doi: 10.1007/s11430-015-5212-5
Jiao N., Tang K., Cai H., Mao Y. (2010). Ncreasing the microbial carbon sink in the sea by reducing chemical fertilization on the land. Nat. Rev. Microbiol. 9 (1). doi: 10.1038/nrmicro2386-c2
Kandasamy S., Nath B. N. (2016). Perspectives on the terrestrial organic matter transport and burial along the land-deep Sea continuum: Caveats in our understanding of biogeochemical processes and future needs. Front. Mar. Sci. 3. doi: 10.3389/fmars.2016.00259
Kemp M. J., Dodds W. K. (2002). The influence of ammonium, nitrate, and dissolved oxygen concentrations on uptake, nitrification, and denitrification rates associated with prairie stream substrata. Limnol. Oceanog. 47 (5), 1380–1393. doi: 10.4319/lo.2002.47.5.1380
Lønborg C., Carreira C., Jickells T., Álvarez-Salgado X. A. (2020). Impacts of global change on ocean dissolved organic carbon (DOC) cycling. Front. Mar. Sci. 7. doi: 10.3389/fmars.20
Liu Z., Qiuqian L., Yao Z., Wang X., Huang L., Zheng J., et al. (2018). Effects of a commercial microbial agent on the bacterial communities in shrimp culture system. Front. Microbiol 9. doi: 10.3389/fmicb.2018.02430
Louca S., Parfrey L. W., Doebeli M. (2016). Decoupling function and taxonomy in the global ocean microbiome. Science 3536305, 1272–1277. doi: 10.1126/science.aaf4507
Meier H. E. M. (2006). Baltic Sea Climate in the late twenty-first century: A dynamical downscaling approach using two global models and two emission scenarios. Climate Dynam. 27 (1), 39–68. doi: 10.1007/s00382-006-0124-x
Middelburg J. J. (2011). Chemoautotrophy in the ocean. Geophys. Res. Lett. 38 (24), L24604. doi: 10.1029/2011GL049725
Middelburg J. J., Nieuwenhuize J. (2000). Nitrogen uptake by heterotrophic bacteria and phytoplankton in the nitrate-rich Thames estuary. Mar. Ecol. Prog. Series. 203, 13–21. doi: 10.3354/meps203013
Monteiro M., Séneca J., Torgo L., Cleary D., Gomes N., Santoro A., et al. (2017). Environmental controls on estuarine nitrifying communities along a salinity gradient. Aquat. Microbial Ecol. 80 (2), 167–180. doi: 10.3354/ame01847
Orsi W. D., Smith J. M., Liu S., Liu Z., Sakamoto C. M., Wilken S., et al. (2016). Diverse, uncultivated bacteria and archaea underlying the cycling of dissolved protein in the ocean. ISME J. 10 (9), 2158–2173. doi: 10.1038/ismej.2016.20
Pachiadaki M. G., Sintes E., Bergauer K., Brown J. M., Record N. R., Swan B. K., et al. (2017). Major role of nitrite-oxidizing bacteria in dark ocean carbon fixation. Science 358 (6366), 1046–1051. doi: 10.1126/science.aan8260
Pedler B. E., Aluwihare L. I., Azam F. (2014). Single bacterial strain capable of significant contribution to carbon cycling in the surface ocean. Proc. Natl. Acad. Sci. 111 (20), 7202–7207. doi: 10.1073/pnas.1401887111
Quéré C., Andrew R. M., Friedlingstein P., Sitch S., Hauck J., Pongratz J., et al. (2018). Global carbon budget 2018. Earth System Sci. Data. 10 (4), 2141–2194. doi: 10.5194/essd-10-2141-2018
Rabalais N. N., Turner R. E., Dortch Q., Justic D., Bierman V. J., Wiseman W. J. (2002). “Nutrient-enhanced productivity in the northern gulf of Mexico: Past, present and future,” in Nutrients and eutrophication in estuaries and coastal waters (Dordrecht: Springer), 39–63.
Rasmussen A. N., Francis C. A. (2022). Genome-resolved metagenomic insights into massive seasonal ammonia-oxidizing archaea blooms in San Francisco bay. Msystems 7 (1), e01270–e01221. doi: 10.1128/msystems.01270-21
Roland L. A., McCarthy M. D., Guilderson T. (2008). Sources of molecularly uncharacterized organic carbon in sinking particles from three ocean basins: A coupled Δ14C and δ13C approach. Mar. Chem. 111 (3-4), 199–213. doi: 10.1016/j.marchem.2008.05.010
Ryan R. P., Monchy S., Cardinale M., Taghavi S., Crossman L., Avison M. B., et al. (2009). The versatility and adaptation of bacteria from the genus stenotrophomonas. Nat. Rev. Microbiol. 7 (7), 514–525. doi: 10.1038/nrmicro2163
Schimel J. P., Schaeffer S. M. (2012). Microbial control over carbon cycling in soil. Front. Microbiol. 3. doi: 10.3389/fmicb.20
Sebastián M., Auguet J., Ortiz C., Sala M., Marrasé C., Gasol J. M. (2018). Deep ocean prokaryotic communities are remarkably malleable when facing long term starvation. Environ. Microbiol. 20 (2), 713–723. doi: 10.1111/1462-2920.14002
Sebastián M., Estrany M., Ruiz-González C., Forn I., Sala M. M., Gasol J. M., et al. (2019). High growth potential of long-term starved deep ocean opportunistic heterotrophic bacteria. Front. Microbiol. 10. doi: 10.3389/fmicb.20
Seitzinger S., Harrison J., Dumont E., Beusen A. H., Bouwman A. (2005). Sources and delivery of carbon, nitrogen, and phosphorus to the coastal zone: An overview of global nutrient export from watersheds (NEWS) models and their application. Global Biogeochem. Cycles 19 (4), GB4S01. doi: 10.1029/2005GB002606
Shade A., Handelsman J. (2012). Beyond the Venn diagram: The hunt for a core microbiome. Environ. Microbiol. 14 (1), 4–12. doi: 10.1111/j.1462-2920.2011.02585.x
Sipler R. E., Kellogg C. T., Connelly T. L., Roberts Q. N., Yager P. L., Bronk D. A. (2017). Microbial community response to terrestrially derived dissolved organic matter in the coastal arctic. Front. Microbiol. 8. doi: 10.3389/fmicb.2017.01018
Smeaton C., Austin W. E. N. (2022). Distribution of particulate and dissolved organic carbon in surface waters of northern scottish fjords. Estuar. Coast. Shelf Sci. 274, 107952. doi: 10.1016/j.ecss.2022.107952
Smith V. H. (2006). Responses of estuarine and coastal marine phytoplankton to nitrogen and phosphorus enrichment. Limnol. Oceanog. 51 (1part2), 377–384. doi: 10.4319/lo.2006.51.1_part_2.0377
Soininen J., Heino J., Wang J. (2018). A meta-analysis of nestedness and turnover components of beta diversity across organisms and ecosystems. Global Ecol. Biogeograph. 27 (1), 96–109. doi: 10.1111/geb.12660
Tian J., Dungait J. A., Lu X., Yang Y., Hartley I. P., Zhang W., et al. (2019). Long-term nitrogen addition modifies microbial composition and functions for slow carbon cycling and increased sequestration in tropical forest soil. Global Change Biol. 25 (10), 3267–3281. doi: 10.1111/gcb.14750
Tremblay L., Alaoui G., Léger M. N. (2011). Characterization of aquatic particles by direct FTIR analysis of filters and quantification of elemental and molecular compositions. Environ. Sci. technol. 45 (22), 9671–9679. doi: 10.1021/es202607n
Trevathan-Tackett S. M., Sherman C. D., Huggett M. J., Campbell A. H., Laverock B., Hurtado-McCormick V., et al. (2019). A horizon scan of priorities for coastal marine microbiome research. Nat. Ecol. Evol. 3 (11), 1509–1520. doi: 10.1038/s41559-019-0999-7
Turnewitsch R., Domeyer B., Graf G. (2007). Experimental evidence for an effect of early-diagenetic interaction between labile and refractory marine sedimentary organic matter on nitrogen dynamics. J. Sea Res. 57 (4), 270–280. doi: 10.1016/j.seares.2006.08.001
Van Niel E., Arts P., Wesselink B., Robertson L., Kuenen J. G. (1993). Competition between heterotrophic and autotrophic nitrifiers for ammonia in chemostat cultures. FEMS Microbiol. Ecol. 11 (2), 109–118. doi: 10.1111/j.1574-6968.1993.tb05802.x
Verhagen F. J., Duyts H., Laanbroek H. J. (1992). Competition for ammonium between nitrifying and heterotrophic bacteria in continuously percolated soil columns. Applied Environ. Microbiol. 58, 10, 3303–3311. doi: 10.1128/aem.58.10.3303-3311.1992
Walker S. A., Amon R. M., Stedmon C., Duan S., Louchouarn P. (2009). The use of PARAFAC modeling to trace terrestrial dissolved organic matter and fingerprint water masses in coastal Canadian Arctic surface waters. J. Geophys. Res.: Biogeosci. 114 (G4), G00F06. doi: 10.1029/2009JG000990
Wang Z., Cao J., Meng F. (2015). Interactions between protein-like and humic-like components in dissolved organic matter revealed by fluorescence quenching. Water Res. 68, 404–413. doi: 10.1016/j.watres.2014.10.024
Ward N. D., Bianchi T. S., Sawakuchi H. O., Gagne-Maynard W., Cunha A. C., Brito D. C., et al. (2016). The reactivity of plant-derived organic matter and the potential importance of priming effects along the lower Amazon river. J. Geophys. Res.: Biogeosci. 121 (6), 1522–1539. doi: 10.1002/2016JG003342
Watts S. C., Ritchie S. C., Inouye M., Holt K. E. (2019). FastSpar: rapid and scalable correlation estimation for compositional data. Bioinformatics 35 (6), 1064–1066. doi: 10.1093/bioinformatics/bty734
Wikner J., Andersson A. (2012). Increased freshwater discharge shifts the trophic balance in the coastal zone of the northern Baltic Sea. Global Change Biol. 18 (8), 2509–2519. doi: 10.1111/j.1365-2486.2012.02718.x
Xiao X., Powers L. C., Liu J., Gonsior M., Zhang R., Zhang L., et al. (2022). Biodegradation of terrigenous organic matter in a stratified Large-volume water column: Implications of the removal of terrigenous organic matter in the coastal ocean. Environ. Sci. Technol. 56 (8), 5234–5246. doi: 10.1021/acs.est.1c08317
Zhang L., Chen M., Chen X., Wang J., Zhang Y., Xiao X., et al. (2021). Nitrifiers drive successions of particulate organic matter and microbial community composition in a starved macrocosm. Environ. Int. 157, 106776. doi: 10.1016/j.envint.2021.106776
Zhang L., Chen M., Zheng Y., Wang J., Xiao X., Chen X., et al. (2022). Microbially driven fate of terrigenous particulate organic matter in oceans. Limnol. Oceanog. 68, 1–17. doi: 10.1002/lno.12255
Zhou J., Deng Y., Luo F., He Z., Yang Y. (2011). Phylogenetic molecular ecological network of soil microbial communities in response to elevated CO2. MBio 2 (4), e00122–e00111. doi: 10.1128/mBio.00122-11
Keywords: terrigenous particulate organic matter, chemoautotrophy and heterotrophy communities, microbial transformation, coastal ecosystem, carbon sequestration
Citation: Zhang L, Liu Y, Chen L and Song H (2023) Terrigenous particles regulate autotrophic and heterotrophic microbial assembly and induce humic-like FDOM accumulation in seawater. Front. Mar. Sci. 9:1116286. doi: 10.3389/fmars.2022.1116286
Received: 05 December 2022; Accepted: 28 December 2022;
Published: 19 January 2023.
Edited by:
Xiangbin Ran, Ministry of Natural Resources, ChinaReviewed by:
Shengwei Hou, Southern University of Science and Technology, ChinaQingyun Yan, Sun Yat-sen University, China
Copyright © 2023 Zhang, Liu, Chen and Song. This is an open-access article distributed under the terms of the Creative Commons Attribution License (CC BY). The use, distribution or reproduction in other forums is permitted, provided the original author(s) and the copyright owner(s) are credited and that the original publication in this journal is cited, in accordance with accepted academic practice. No use, distribution or reproduction is permitted which does not comply with these terms.
*Correspondence: Hui Song, c29uZ2h1aTIwMThAZm94bWFpbC5jb20=