- 1Guangdong Provincial Key Laboratory of Marine Disaster Prediction and Prevention, and Guangdong Provincial Key Laboratory of Marine Biotechnology, Shantou University, Shantou, China
- 2Southern Marine Science and Engineering Guangdong Laboratory (Guangzhou), Guangzhou, China
The agricultural use of neonicotinoids is increasing worldwide and poses a threat to non-target organisms. The existing toxicity data of neonicotinoids that is mainly focused on widely used neonicotinoids ignores the influence of environmental factors, like temperature, related to climate changes, etc. To fill this data gap, the present study assessed the temperature-dependent toxicity of six neonicotinoids at four temperatures. Briefly, a fish embryo toxicity test was performed at the following temperatures—20, 23, 28, and 33°C—on zebrafish embryos to evaluate the lethal and sublethal effects of these toxicants. At 28°C, the lethal toxicity (LC50) values for these toxicants were cycloxaprid—3.36 mg/L, nitenpyram—7.08 mg/L, paichongding—17.2 mg/L, imidaclothiz—738.6 mg/L, dinotefuran—2,096 mg/L, and thiamethoxam—4,293 mg/L, respectively. Among the sublethal effects, the enzymatic activities changed significantly in neonicotinoid treatments, which revealed oxidative stress, metabolic disorders, and neurotoxicity. Particularly, acetylcholinesterase inhibition and glutathione S-transferase activation showed a significant dose–response relationship. However, cycloxaprid, nitenpyram, and paichongding were found to be more potent compared with imidaclothiz and thiamethoxam. The influence of temperature on these neonicotinoids demonstrated an inverted V-shaped relationship, in which toxicity decreased with the increase of temperature and then increased with the increase of temperature after exceeding the optimum temperature. This study provides a reference for the multiscale effects and potential mechanisms of neonicotinoids. Temperature-dependent toxicity is of great significance for future toxicity testing and risk assessment of chemicals in the face of global climate changes.
Highlights
● The temperature-dependent lethal and sublethal effects of neonicotinoids were investigated by a fish embryo toxicity test.
● Multi-biomarkers including AChE, GPX, GST, and ATPase were examined.
● Temperature-dependent thresholds for multi-endpoint were determined.
● The temperature-dependent chemical toxicity for neonicotinoids followed an inverted V-shaped relationship.
● Our results can support data dossier development, toxicity testing, and ecological risk assessment.
1. Introduction
The extensive use of neonicotinoid insecticides and their massive residues in aquatic environments are a serious threat to non-target organisms. To date, neonicotinoid toxicity testing for aquatic species has been limited to the most commonly used categories in major markets (e.g., imidacloprid and thiamethoxam) and some aquatic invertebrates like Chironomus riparius, Mysidopsis bahia, and Daphnia (Hayasaka et al., 2013; Anderson et al., 2015; Miles et al., 2017). Several studies reported that neonicotinoid insecticides could induce acute lethal toxicity to fish species like Oryzias latipes, Lepomis macrochirus, Oncorhynchus mykiss, and Danio rerio (Ohi et al., 2004; Ocampo and Sagun, 2007; Clasen et al., 2011; Gibbons et al., 2014). Some studies demonstrated that neonicotinoids can induce sublethal toxicity, e.g., reduced body length and weight and inhibition in the growth and development of Pimephales promelas (DeCant and Barrett, 2010), and can influence the development of gonads and the reproductive system in Oreochromis niloticus (Ocampo and Sagun, 2007; Lauan et al., 2013) as well as immunotoxicity in O. latipes (Sánchez-Bayo and Goka, 2005). Moreover, inhibition in the activity of acetylcholinesterase (AChE) and the development of the nervous system in brain tissues, resulting in neurotoxicity in O. mykiss and Gobiocypris rarus, was reported by Topal et al. (2017); Tian et al. (2018), and Ma et al. (2019). Oxidative stress, DNA damage, inhibition in protein synthesis, and disruption of amino acid metabolism and tricarboxylic acid cycle, leading to energy metabolism imbalance in D. rerio, were reported by Yan et al. (2015); Yan et al. (2016); Zhang and Zhao (2017). However, the toxicity data of neonicotinoids is scarce, especially for some newly developed neonicotinoids including nitenpyram, cycloxaprid, paichongding, and imidaclothiz, and the sublethal effects of these neonicotinoids on non-target fishes (e.g., D. rerio) are largely unknown.
Temperature variations by climatic changes have profound implications on the chemical toxicity to individual organisms by influencing their physical and chemical properties (e.g., thermal dynamics, solubility, aggregation, ion dissolution, precipitation, partition coefficient, diffusion rates, chemical bonding, reaction rate, chemical speciation, and bioavailability) and biological reactions including growth and development, physiology and biochemistry, energy metabolism, chemical uptake, detoxification, and biological elimination (Heugens et al., 2003; Brocchi et al., 2013; Bourgeault et al., 2013; Wang et al., 2019). However, the toxicity of pesticides such as neonicotinoids under different temperature exposure scenarios is scarcely explored. The toxicity data and risk thresholds obtained from laboratory standard toxicity testing ignore the influence of temperature variations on neonicotinoid toxicity. These factors could potentially bring large uncertainty into the derivation of appropriate water quality guidelines or the risk assessment of chemicals (Zhou et al., 2014). Keeping in view the increasingly global climatic changes and the emergence of a wide variety of pollutants like neonicotinoids in the environment with relatively poor safety information, it is necessary to investigate the influence of temperature changes on the multi-endpoint toxicity induced by neonicotinoids to provide safety information and theoretical basis for a comprehensive ecological risk assessment (ERA) of chemicals.
The data based on some traditional individual-level endpoints (e.g., mortality, growth, development, behavior, and morphology) or considering a single toxic effect as endpoint may not provide sufficient protection for aquatic organisms exposed to low doses of neonicotinoids over long time periods (Wang et al., 2021) because neonicotinoids at low concentrations may not directly cause mortality at the individual level but could potentially cause some chronic or sublethal effects at the cellular/molecular level (e.g., biochemistry, enzymes, and hormones). Some previous studies reported that neonicotinoids can induce oxidative stress (Priya and Maruthi, 2013), followed by changes in cells/tissues/organs like the liver and spleen (Nicolas and Ocampo, 2006) and gonads (Lauan et al., 2013), ultimately leading to developmental toxicity, immunotoxicity, and thus mortality (Morrissey et al., 2015), whereas the mode of action (MOA) and the relationship among these effects (i.e., adverse outcome pathway—AOP) induced by neonicotinoids are unclear. Meanwhile, the selection of test endpoints is another key factor for toxicity identification and evaluation (TIE) and the ERA of pollutants. However, the traditional toxicity test using adult fish is time-consuming and resource-wasting and violates the 3R principles (reduction, refinement, and replacement of animal testing). The embryonic life stage could supplement enough to cover these deficiencies because an embryo is the simplest multicellular individual developed from a single cell, which not only complies with the 3R principles but also could allow a convenient study of its toxic effects from cell/tissue level to the ontogenetic level. Therefore, the fish embryo toxicity test (FET) may be a rapid and effective alternative test method to examine multiscale effects from the cellular/molecular level, including neurotoxicity, energy metabolism, and oxidative stress, to the individual level like hatching rate, mortality, and potential MOA of neonicotinoids (OECD, 2013; Su et al., 2021).
In this study, FET was performed with the exposure of neonicotinoids (nitenpyram, cycloxaprid, paichongding, imidaclothiz, dinotefuran, and thiamethoxam) at different temperature levels (20, 23, 28, and 33°C) to investigate the temperature-dependent effects of neonicotinoids on the development of zebrafish embryo. Firstly, this study provides a reference for the toxic effects of neonicotinoids and their potential mechanisms on zebrafish embryos as well as the theoretical basis for the construction of AOPs of the chemicals. Secondly, the determination of temperature-dependent thresholds [e.g., LC/EC10/50, no observed effect concentration (NOEC), and lowest observed effect concentration (LOEC)] at diverse endpoints can enrich the toxicity database for TIE and the ERA of neonicotinoids. Finally, the influences of temperature on neonicotinoid toxicity to the embryonic development of zebrafish were systematically investigated by analyzing the relationship between the temperature and toxicity thresholds of each test endpoint. It could provide vital information/guidelines for the construction of predictive models based on temperature-dependent chemical toxicity as well as the derivation of temperature-dependent or region-specific risk thresholds for neonicotinoids.
2. Materials and methods
2.1. Zebrafish maintenance and embryo collection
Adult wild-type (AB) zebrafish (D. rerio) were obtained from China Zebrafish Resource Center (Wuhan, China). All fish were reared in a recirculation system at 28 ± 0.5°C under 14:10-h light/dark photoperiod and 54–324-lx light intensity following the standard zebrafish breeding protocols. Meanwhile, NaHCO3 and NaCl were added in reverse osmosis-filtered water to maintain the pH and conductivity at 7.5 ± 0.5 and 500–800 μS/cm, respectively. The adult zebrafish were fed twice a day with live brine shrimps. The male and female fishes were kept separately at each designated temperature conditions for more than 2 weeks before they were determined to spawn. On the night before spawning, the paired adult fishes were placed in spawning boxes with a male/female ratio of 1:1 and separated by a partition overnight. The partition was removed earlier the next day, and spawning was induced at the beginning of the light cycle. Within 0.5 h of spawning, embryos were collected, rinsed with standard diluent (Appendix I) thrice, and transferred to the embryo medium before use. Fertilized and healthy embryos were selected for toxicity testing under the stereomicroscope SZX 7 (Olympus, Tokyo, Japan) following Kimmel et al. (1995).
2.2. Chemicals and reagents
Cycloxaprid (CAS number: 1203791416), dinotefuran (165252700), imidaclothiz (105843365), nitenpyram (150824478), paichongding (948994169), and thiamethoxam (153719234) with 98% purity were purchased from HaoRong Biological Technology Co., Ltd. (Wuhan, China). Stock solutions were prepared by dissolving the neonicotinoids in standard diluent (Appendix I) prior to the commencement of an exposure experiment to ensure the proper dissolution of the substance. The tested concentrations were obtained by further diluting the stocks with standard diluents (Appendix I). To prevent photodegradation, both solutions (test and stock) were prepared in glass bottles wrapped in aluminum foil and stored in a refrigerator at 4°C until each experiment is finished.
2.3. Standard acute lethal toxicity test
Standard acute lethal toxicity tests were conducted following OECD TG 236 (OECD, 2013). The highest tested concentration should preferably result in 100% lethality, and the lowest concentration tested should preferably give no observable effect. Range-finding tests were conducted with concentrations ranging from 0.1 to 10,000 mg/L (10 embryos per concentration) before the definitive test to allow the selection of an appropriate concentration range. After 96 h of exposure, the approximate concentration range of the embryonic acute lethal toxicity of each neonicotinoid could be preliminarily determined to set the formal test concentration gradient.
According to the results of the preliminary experiment, five test concentrations were set between 0% and 100% lethal concentration, and standard diluent was used as the blank control group. The embryos were immersed in the test solutions before cleavage of the blastodisc commences or, at the latest, by the 16-cell stage. Thus, embryos at 1 h of post-fertilization (1 hpf) were randomly selected and individually exposed to the neonicotinoids in 24-well plates, and the acute toxicity test was terminated after 96 h of exposure (static exposure with no renewal over the period of 96 h). Each well on the plate was refilled with 2 ml of freshly prepared test solutions and one embryo (24 embryos per plate). The test was performed under four temperatures (20, 23, 28, and 33°C) and under 14:10-h light/dark photoperiod. Each neonicotinoid exposure concentration at each temperature was set as one treatment, and each treatment was performed in triplicates (three 24-well plates for each treatment and 72 embryos per treatment). Coagulation of embryos, malformation, and lack of heartbeat were used to determine the lethality. Additionally, hatching was recorded in the treatment and control groups every day starting from 48 h. The observations of embryos hatching and mortality under a microscope were recorded every 24 h until the end of the test. Finally, the mortality rate was computed to generate the log (dose) mortality curves, and the 96-h embryonic 10%/50% lethal concentration (LC10/50) values under different temperatures were calculated.
2.4. Standard sublethal FET test
Following the OECD TG 236 and based on the acute toxicity test results, the exposure concentrations of neonicotinoids for the sublethal toxicity test were set as 1/2×, 1/4×, 1/8×, 1/16×, and 1/32 × 96-h LC50. Standard diluent without chemicals was used as the blank control group. The 1-hpf embryos were randomly selected for exposure to different neonicotinoid test solutions under four temperatures (20, 23, 28, and 33°C) and 14:10-h light/dark photoperiod. Each treatment was performed in triplicates (24 embryos per replicate). The homogenate of the embryo tissue was prepared after exposure for 96 h. Firstly, 24 embryos were rinsed in cold normal saline (pH 7.4, 0.6% sodium chloride solution), dried with filter paper, and then weighed at 0.2 g. The weighed embryos (0.2 g) were added into 1.8 ml 0.6% NaCl solution by ratio of weight (g) to volume (ml) = 1:9 in a 5-ml glass homogenizer (also called tissue grinding tube, which consisted of a grinding rod with an outer surface of ground sand and a glass tube with an inner surface of ground sand) for homogenizing. The homogenizing tube was placed on ice and fully ground for a dozen times up to 6–8 min to make 10% homogenate (the embryonic tissue was crushed and ground by manually pushing and pulling and by using a rotating rod). Finally, the prepared 10% homogenate was centrifuged at 4°C, 2,500 rpm for 15 min, and the supernatant was taken for determination. The embryo homogenate of each test group was used to detect the different endpoints, including the following: (1) oxidative stress: GPX and GST activity, (2) metabolic disorders/ATPase activity, and (3) neurotoxicity/AChE activity.
Total protein content and enzyme activity were determined by various kits, including Bradford Protein Content Assay Kit (Beyotime), Total GPX Activity Detection Kit (Beyotime), GST Activity Detection Kit (Solarbio), ATPase Activity Detection Kit (Nanjing Jiancheng), and AChE Activity Detection Kit (Solarbio). The enzyme activity in each sample was expressed as the enzyme activity in protein per unit mass (U/mg protein). The contents of the above-mentioned enzyme activities were detected by a TECAN microplate analyzer.
2.5. Statistical analysis
Experimental data was analyzed using GraphPad Prism (version 5.0, San Diego, CA, USA), and the results were expressed as mean ± standard deviation (SD). One-way ANOVA was performed to compare the significant difference between the control and the neonicotinoid treatment groups as well as to determine the LOEC and NOEC. Two-way ANOVA was computed to test the significance of temperature and exposure concentration and their interactions on the changes of indicators. To determine LC10/50 (10%/50% lethal concentration) and EC10/50 (10%/50% effective concentration), the data were fitted to a sigmoidal (log) dose–response curve (variable slope) with a four-parameter logistic equation. All analyses were conducted in SPSS (version 23, Chicago, IL, USA), and the significance level α was set at 0.05.
3. Results and discussion
3.1. Acute lethal toxicity of neonicotinoids to zebrafish embryos
Based on the preliminary experimental results, the five exposure concentrations for each neonicotinoid were set as follows: cycloxaprid—0.1, 1, 10, 50, and 100 mg/L; dinotefuran—1, 10, 100, 1,000, and 10,000 mg/L; imidaclothiz—0.1, 1, 10, 50, and 100 mg/L; nitenpyram—0.1, 1, 10, 100, and 1,000 mg/L; paichongding—0.1, 1, 10, 50, and 100 mg/L; and thiamethoxam—1,000, 2,000, 3,000, 4,000, and 5,000 mg/L, respectively. After 96 h of exposure under four temperatures (20, 23, 28, and 33°C), the mortality rate of zebrafish embryos was calculated (Supplementary Table S1). The dose–response curves at four temperatures for the six neonicotinoids were plotted and demonstrated in Supplementary Figure S5. The 96-h LC50 of the six neonicotinoids under different temperatures was calculated and listed in Table 1—for example, at 28°C, the LC50 of cycloxaprid, dinotefuran, imidaclothiz, nitenpyram, paichongding, and thiamethoxam was 3.36, 2,096, 738.6, 7.08, 17.2, and 4,293 mg/L, respectively. The acute lethal toxicity of these neonicotinoids to zebrafish embryos is presented in the following order: cycloxaprid > nitenpyram > paichongding > imidaclothiz > dinotefuran > thiamethoxam. However, a similar toxicity trend was also found at three other temperatures (20, 23, and 33°C).
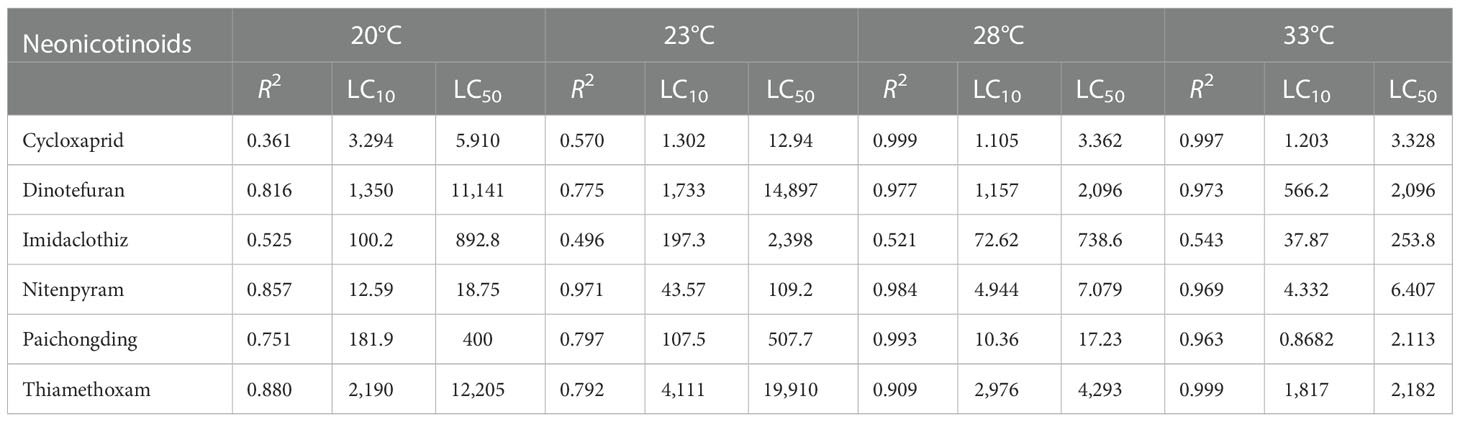
Table 1 The embryonic 96-h 10% and 50% lethal concentration (LC10/50, mg/L) of six neonicotinoids under different temperature conditions [curves were fitted to sigmoidal (log) dose–response curve (variable slope) with a four-parameter logistic equation in GraphPad Prism (version 5.0, San Diego, CA, USA)].
3.2. Sublethal effects of neonicotinoids to zebrafish embryos
According to the LC50 values of the neonicotinoids, five sublethal concentrations for each neonicotinoid were set as follows: cycloxaprid—0.125, 0.25, 0.5, 1, and 2 mg/L; imidaclothiz—6.25, 12.5, 25, 50, and 100 mg/L; nitenpyram—0.125, 0.25, 0.5, 1, and 2 mg/L; paichongding—0.625, 1.25, 2.5, 5, and 10 mg/L; and thiamethoxam—62.5, 125, 250, 500, and 1,000 mg/L, respectively. After 96 h of exposure, the enzyme activities in all neonicotinoid treatment groups and in the control group under different temperatures were measured (Supplementary Tables S2–S5).
3.2.1. AChE activity
The tested neonicotinoids at sublethal concentrations (1/32–1/2 LC50) showed no significant effect on the survival of zebrafish embryos. However, the AChE activity followed a dose–effect relationship of being significantly decreased by increasing the neonicotinoid concentrations, which indicated that the neonicotinoids are potentially neurotoxic to zebrafish embryos (Supplementary Figure S1). Sigmoidal dose–response curves (variable slope) were fitted with a four-parameter logistic equation to obtain EC50 (Supplementary Figures S6A–E). One-way ANOVA was also performed to determine the NOEC/LOEC of neonicotinoids inhibiting the AChE activity of zebrafish embryos at four temperatures (Table 2). At 28°C, the EC50 of cycloxaprid, imidaclothiz, nitenpyram, paichongding, and thiamethoxam AChE activity was 0.998 (0.786, 1.244), 52.55 (29.74, 189.8), 0.957 (0.546, 2.184), 0.695 (0.019, 1.442), and 24.33 (17.09, 32.7) mg/L, whereas at 33°C the EC50 of these neonicotinoids was 0.693 (0.595, 0.806), 6.57 (2.10, 11.15), 0.494 (0.416, 0.587), 0.503 (0.137, 0.824), and 128.0 (96.21, 163.2) mg/L, respectively. However, it is worth mentioning that the inhibitory effect of cycloxaprid, nitenpyram, and paichongding on the AChE activity of zebrafish embryo was significantly greater than those of imidaclothiz and thiamethoxam.
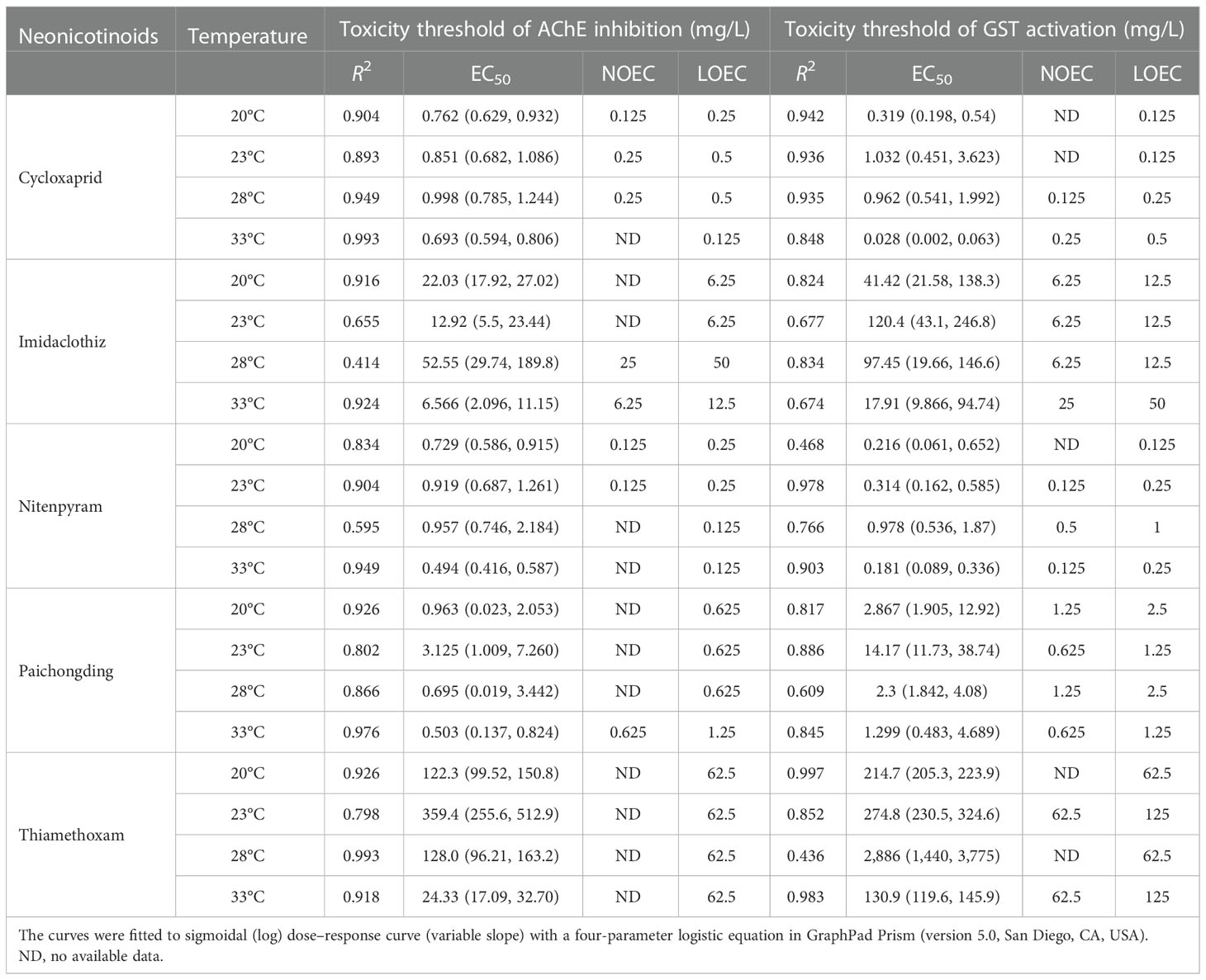
Table 2 Toxicity threshold of AChE inhibition and GST activation (EC50, NOEC, LOEC, mg/L) of five neonicotinoids under different temperature conditions.
As nicotinic acetylcholine receptor (nAChR) agonists, neonicotinoids selectively bind to the nAChR and compete with the insect’s acetylcholine, thus blocking the normal transmission between neurons and thereby causing paralysis and the ultimately death of the organism (Mitchell et al., 2017). AChE is a multi-molecule glycoprotein that hydrolyzes acetylcholine and can terminate the transmission of acetylcholine in synapses. It exists in nerve tissue, liver, muscle, red blood cell, or spleen in animals and plays an important role in nerve conduction and functional regulation of the neurotransmitter. Changes in the activity of AChE are sensitive toxicological indicators for pesticide pollution in an aquatic environment, which can be further used as molecular biomarkers for toxicity evaluation and risk assessment as well as in the environmental monitoring of pesticides in water (Lionetto et al., 2013; Gravato et al., 2021). Neonicotinoids particularly inhibit the AChE activity, resulting in the accumulation of acetylcholine which causes inhibition in the normal functioning of the central nervous system (Lu et al., 2018). According to pioneer studies, neonicotinoids can cause neurotoxic effects (e.g., motor behavior) to invertebrates and aquatic vertebrates by inhibiting the AChE activity, e.g., exposure of imidacloprid resulted in decreased AChE activity in Chironomus riparius (Azevedo-Pereira et al., 2011), Eisenia foetida (Wang et al., 2015), and Caenorhabditis elegans (Meyer and Williams, 2014) as well as in the brain of D. rerio (Guerra et al., 2021), O. mykiss (Topal et al., 2017), and Rhamdia quelen (Marins et al., 2021). In the muscle of Astyanax altiparanae (Almeida et al., 2021), sulfoxaflor exposure can also significantly decrease AChE enzyme activity in the brain and muscle tissues of zebrafish (Piner Benli and Çelik, 2021). In this study, the inhibitory effect on AChE activity of zebrafish embryos indirectly reflects the neurotoxic effect of neonicotinoids on zebrafish, which can be used as a quantitative indicator to evaluate the sublethal toxicity of neonicotinoid pesticides (Sheets et al., 2015).
3.2.2. ATPase activity
Compared with the control group, under four temperatures (20, 23, 28, and 33°C), the ATPase activity of zebrafish embryos was slightly increased in the low concentration (p > 0.05) and significantly decreased (p < 0.05) in the high concentration of the six neonicotinoid treatment groups (Supplementary Figure S2)—for example, at 33°C, the ATPase activity was decreased in 1 mg/L and increased in 0.5 mg/L, whereas the enzyme activity was increased at other temperatures in 0.125 and 0.25 cycloxaprid treatments (Supplementary Figure S2A). At 20 and 33°C, the enzyme activity was decreased in 6.25 mg/L and decreased at two other temperatures in 12.5- and 25-mg/L treatments of imidaclothiz (Supplementary Figure S2B). Furthermore, at all four temperatures, the ATPase activity was activated by nitenpyram exposure in 0.125 and 0.25 mg/L and decreased in the 0.5-, 1-, and 2-mg/L treatment groups (Supplementary Figure S2C). Meanwhile, at 23°C, the ATPase activity was promoted in 0.625-mg/L treatment and inhibited by 0.625-, 1.25-, and 2.5-mg/L paichongding treatments at three other temperatures (Supplementary Figure S2D). However, at 20 and 28°C, the ATPase activity was enhanced in 0.625 mg/L, followed by a decrease at 250 and 500 mg/L, and inhibited at 23 and 33°C by 0.625- and 1.25-mg/L treatments of thiamethoxam (Supplementary Figure S2E).
ATPase (divided into Na+K+–ATPase and Ca2+Mg2+–ATPase), a protease in the membrane of a cell or an organelle, can regulate the membrane potential and maintain the intracellular ion gradients which are essential for signal transduction, excitability, conductivity, and release of neurotransmitters in nerve cells (Ji et al., 2009). Na+K+–ATPase is a sodium pump on the cell membrane, which can transport Na+ and K+ to maintain the normal physiological function of cells. When Na+K+–ATPase activity is inhibited, the intracellular and extracellular Na+ concentration will be imbalanced, and excessive intracellular Na+ can inhibit the uptake of excitatory amino acids in the synaptic membrane (Davis and Wedemeyer, 1971; Farley et al., 1984; Agrahari and Gopal, 2008), whereas Ca2+Mg2+–ATPase is a calcium pump on the membrane, which can hydrolyze the ATP to maintain a low intracellular Ca2+ concentration which may help in maintaining cell homeostasis. When the activity of Ca2+Mg2+–ATPase inhibits, the activity of a large number of intracellular enzymes will be reduced, and this will cause an increase of free radical production, ultimately leading to peroxidation damage of the membrane, destruction of the mitochondria, and cell death (Al-Rajhi, 1990; Blasiak, 1995; Li et al., 2006). The inhibition in energy conversion—such as inhibition in the activity of mitochondrial enzyme—may be the key component of neonicotinoid insecticide mechanism (Kumar et al., 2018; Li et al., 2022; Sevgiler and Atlı, 2022; Veedu et al., 2022). The results of our study indicated that the high-concentration exposure of neonicotinoids inhibited the ATPase activity in zebrafish embryos, which may cause the functional disturbance of the membrane ion pump (e.g., sodium pump and calcium pump). Because the dysfunction of a membrane ion pump leads to a massive outflow of K+ and Mg2+, there will be a massive influx of Na+, Ca2+, and H2O into the cell, resulting in the retention of water and sodium, energy metabolism disorders, and mitochondrial dysfunction, ultimately leading to nerve cell damage and dysfunction of the central nervous system (Suvetha et al., 2010; Serafini et al., 2019; Oyovwi et al., 2021). However, at present, the mechanism which demonstrates how neonicotinoid induces the inhibition of ATPase activity is not clear. It could be either the direct inhibition of ATPase after neonicotinoid pesticides enter into the cell or by indirectly inhibiting the activity of ATPase by structural and functional disorders of the biomembrane through oxidative/peroxidation damage, or both possibilities could have happened (Chauhan et al., 2002; Akbar et al., 2016; Kumar et al., 2018).
3.2.3. Antioxidant enzymes
Oxidative stress refers to oxidative damage caused by the accumulation of reactive oxygen species (ROS) and reactive nitrogen species when there is an imbalance between the generation and the elimination of oxygen-free radicals in the body or cells, resulting in the damage of lipids, amino acids/proteins, nucleic acids, and chromosomes and, ultimately, cell death (Droge, 2002; Deng et al., 2021). Antioxidant enzymes, including superoxide dismutase, catalase, peroxidase, GPX, and GST, are important indexes to detect oxidative stress (Lacourse et al., 2009). GPX can remove oxygen metabolites and maintain the activity of glutathione (GSH). GST is a dual-function enzyme acting as an antioxidant and phase II detoxification enzyme. The combination of GSH with various toxic substances and their metabolites by catalyzing them into hydrophobic substances which are excreted in the form of urine or bile is a vital mechanism of GSH. This mechanism helps GSH to achieve the detoxification effect of degrading and discharging various toxic substances in the body. Meanwhile, GST can also repair macromolecular proteins, DNA, and other substances, remove lipid peroxides, and inhibit lipid peroxidation, which can protect the body from damage by exogenous stimulation (Ullah et al., 2022). Thus, GPX and GST are commonly known as biochemical indicators or biomarkers for evaluating the oxidative damage of environmental pollutants (Pan et al., 2022; Ullah et al., 2022).
In this study, at four temperatures (20, 23, 28, and 33°C), the GPX activity was promoted or not affected by the lower concentrations of the six neonicotinoids compared with the higher concentrations which cause the inhibition in GPX activity (Supplementary Figure S3)—for example, at 20, 23, and 28°C, the GPX activity was enhanced in 0.125-, 0.25-, and 0.5-mg/L concentrations and inhibited in 1- and 2-mg/L concentrations of cycloxaprid (Supplementary Figure S3A); at all four temperatures, enzyme activity was inhibited in 6.25-, 12.5-, and 25-mg/L imidaclothiz concentrations (Supplementary Figure S3B); at 20 and 33°C, enzyme activity increased in 0.125-mg/L concentration and decreased in 0.5- and 1-mg/L concentrations of nitenpyram (Supplementary Figure S3C); at 20°C, the enzyme activity was promoted in 0.625-, 1.25-, and 2.5-mg/L paichongding concentrations and inhibited in 10-mg/L concentration (Supplementary Figure S3D); and at 20, 23, and 28°C, enzyme activity was activated in 62.5- and 125-mg/L concentrations and inhibited in 250- and 500-mg/L concentrations of thiamethoxam (Supplementary Figure S3E).
These findings demonstrated that the inhibition of GPX activity by cycloxaprid and nitenpyram is much stronger than by the other neonicotinoids (especially imidaclothiz and thiamethoxam). The overall effect of neonicotinoids demonstrated a strong dose–response relationship between GPX activity and neonicotinoid concentrations. At low-concentration exposures of the six neonicotinoids, the GPX activity was generally promoted, whereas at higher concentrations the enzyme activity was inhibited. One possible reason could be the excessive production of ROS after neonicotinoids entered the embryos (Shukla et al., 2017). GPX can participate in controlling ROS homeostasis by regulating the intracellular H2O2 levels, antioxidant enzyme activities, and gene transcription levels and by maintaining the GSH/GSSG balance (Zhang et al., 2020). In turn, GPX activities can be regulated by ROS level. An increased concentration of intracellular ROS caused by external stimulus (neonicotinoid exposure) can increase the expression level of the gpx gene and thus promote GPX activities (Li et al., 2015), whereas with increased pesticide concentration and continuous action on cells, excessive ROS cannot be eliminated by the GPX enzyme, leading to the accumulation of ROS, which will oxidize the amino acid residues of the GPX enzyme protein to produce a carbonyl group and change its secondary structure (reducing its helicity) and thus reducing the enzyme activity (Lin et al., 2021). Meanwhile, the excessive ROS can damage lipid, DNA, protein, and other biological macromolecules as well as the structure and functions of membranes, which can directly or indirectly affect the synthesis of the GPX enzyme (Tian et al., 2020; Benchaâbane et al., 2022).
The findings of our study demonstrated that the GST activity was activated by neonicotinoids in a dose-dependent manner (Supplementary Figure S4). It can be noticed that, at 20°C, the GST activity was increased by 0.125 mg/L cycloxaprid, 12.5 mg/L imidaclothiz, 1 mg/L nitenpyram, 2.5 mg/L paichongding, and 62.5 mg/L thiamethoxam, while at 33°C the enzyme activity was promoted by 0.5 mg/L cycloxaprid, 50 mg/L imidaclothiz, 0.25 mg/L nitenpyram, 1.25 mg/L paichongding, and 125 mg/L thiamethoxam. It should be noted that the fluctuations in GST activity may be caused by oxidative stress and the detoxification is induced by pollutant stimulation because the activity of GST could be activated to catalyze the binding of GSH with lipid peroxides or other harmful metabolites produced by oxidative damage and exert antioxidant and detoxification effects (Qi et al., 2013; Benchaâbane et al., 2022).
Dose–response curve and one-way ANOVA were employed to obtain the EC10/50 and the LOEC/NOEC of neonicotinoids, which revealed that the GST activity was promoted in zebrafish embryos at different temperatures (Table 2 and Supplementary Figures S6F–J). At 28°C, the EC50 of GST activation induced by cycloxaprid, imidaclothiz, nitenpyram, paichongding, and thiamethoxam was 0.9619 (0.5409, 1.992), 17.91 (9.866, 94.74), 0.9780 (0.5363, 1.870), 2.300 (1.842, 14.08), and 2,886 (1,440, 9,775) mg/L, respectively, whereas the oxidative damage by cycloxaprid and nitenpyram to zebrafish embryo was stronger compared that of with thiamethoxam. Previous studies have shown that antioxidant and detoxification enzymes are crucial in maintaining cell homeostasis and can used as a biochemical marker to evaluate the potential toxicity of the contaminants—inducing oxidative stress in aquatic organisms, especially in fish (Santana et al., 2018; Gallego-Ríos et al., 2021; Solomando et al., 2022). In this study, the changes of antioxidant enzyme (GPX) and detoxification enzyme (GST) revealed that the neonicotinoids induce oxidative damage to zebrafish embryo. These responses can be used as molecular biomarkers to evaluate the oxidative stress and toxicity mechanisms of neonicotinoids or other pollutants.
Overall, the neonicotinoids potentially caused neurotoxicity and CNS dysfunction by AChE inhibition. Furthermore, neonicotinoid toxicity can cause the peroxidation of biological macromolecules including lipid, protein, and DNA by inducing oxidative damage. This phenomenon can destroy the structure and function of biological membranes, including those of the mitochondrial membrane with the potential inhibition of respiratory enzyme activity, ultimately leading to cellular metabolic disorders (Meiliana and Wijaya, 2012). This implies that neurotoxicity, oxidative damage, and metabolic disorders may be the key components of MOA of neonicotinoid insecticides. These biomarkers—including AChE, GST, GPX, and ATPase activity at the molecular/cellular level—can be utilized as signal indicators for monitoring pesticide contamination in aquatic environments at the early stages.
3.3. Temperature-dependent effects of neonicotinoids to zebrafish embryos
3.3.1. Effects of temperature on embryonic development
Temperature can profoundly influence the physiological and ecological performance of organisms such as during embryonic development—including growth and development (Brett, 1979), malformation and death (Kamler et al., 1998), adaptations and acclimation (Burleson and Silva, 2011), resource acquisition and behavior (Van der Have, 2002), metabolic and ventilation rate (Vinagre et al., 2014), and survivorship and fecundity (Frazier et al., 2006; Angilletta et al., 2009; Kingsolver, 2009; Amarasekare and Savage, 2012). In this study, the hatching rate of zebrafish embryos which are maintained as the control group at four temperatures was recorded for 192 h. The hatching curves of zebrafish embryos at different temperatures (20, 23, 28, and 30°C) were plotted and demonstrated in Supplementary Figure S7. The results showed that embryos started hatching at 20 and 23°C within 108 and 84 h and completely hatched after 192 h at both of these temperatures, while at the two other temperatures (28 and 33°C) the embryos started hatching at 36 h and completely hatched after 72 h. These findings clearly indicated that a low temperature has an inhibitory effect on embryo hatching, and a higher temperature can reduce the hatching time of embryos. Meanwhile, in the control group, the enzyme activities of AChE, ATPase, GPX, and GST were highest at 28°C, decreased at 33°C, and significantly inhibited at low temperatures of 20 and 23°C (Supplementary Tables S2–S5). As demonstrated in Supplementary Figure S7, low temperature inhibited the enzyme activity and high temperature enhanced the enzyme activity. Therefore, it can be assumed that temperatures below or above Topt can inhibit embryonic development.
3.3.2. Temperature-dependent chemical toxicity of neonicotinoids
In this study, a significant interaction was found between various concentrations of the tested neonicotinoids and the embryonic responses (lethal including mortality and sublethal like changes of AChE, ATPase, GPX, and GST activities) of zebrafish at different temperatures. Furthermore, the findings of this study also demonstrated that the interaction between neonicotinoid toxic concentrations and temperature significantly influenced the embryonic mechanisms (Supplementary Table S6).
Nonlinear regression curves were plotted on the toxicity threshold values of lethal (LC50) and sublethal effect (EC50) of neonicotinoids at different temperatures and fitted by Gaussian distribution (Figure 1). Significant variations in LC50 and EC50 can be noticed at variable temperatures. The effect of temperature on neonicotinoid toxicity showed an inverted V-shaped relationship, where the toxicity decreased with the increase of temperature and then increased with the increase of temperature after exceeding the Topt. The temperature corresponding to the highest amplitude of the Gaussian curve represents the Topt, at which toxicity is the least (toxicity is enhanced when the temperature is above/below the Topt). Topt values were also obtained at 23.5, 22.3, 23.8, 23.6, 21.9, and 22.9°C for cycloxaprid, dinotefuran, imidaclothiz, nitenpyram, paichongding, and thiamethoxam, respectively, when considering acute lethal toxicity (Table 3), while for the sublethal effects of AChE inhibition, Topt values were derived at 26.3, 27.3, 25.4, 23.8, and 24.0°C for cycloxaprid, imidaclothiz, nitenpyram, paichongding, and thiamethoxam, respectively. Temperature can affect the toxicity of neonicotinoids (e.g., imidacloprid and thiacloprid) on the embryonic level by enhancing the respiratory efficiency or energy metabolism (Osterauer and Köhler, 2008; Scheil and Köhler, 2009). It is generally accepted that elevated temperatures tend to increase the rate of chemical contaminant uptake by increasing the metabolic and ventilation rate of ectotherms, hence boosting the bioaccumulation of chemicals in body tissues (Cherkasov et al., 2006; Tsui and Wang, 2006; Cherkasov et al., 2007; Schiedek et al., 2008). This mechanism ultimately leads to a higher chemical toxicity at higher temperature levels (Cairns et al., 1975; Bourgeault et al., 2013).
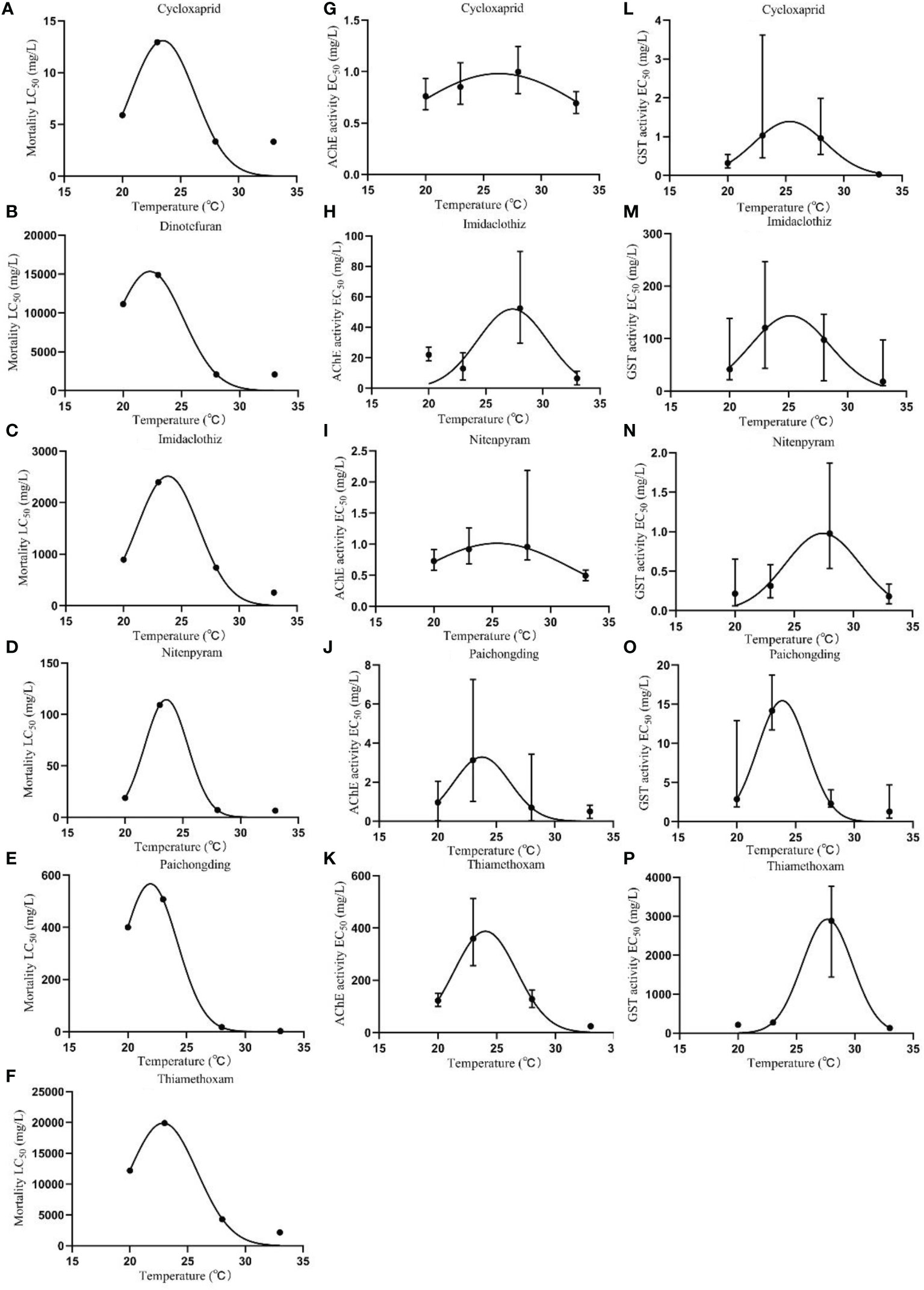
Figure 1 Temperature-dependent chemical toxicity of neonicotinoids to zebrafish embryos. (A–F) Effect of temperature on the lethal toxicity (LC50). (G–K) Effect of temperature on the toxicity threshold (EC50) of AChE inhibitory effect. (L–P) Effect of temperature on the toxicity threshold (EC50) of glutathione S-transferase activation.
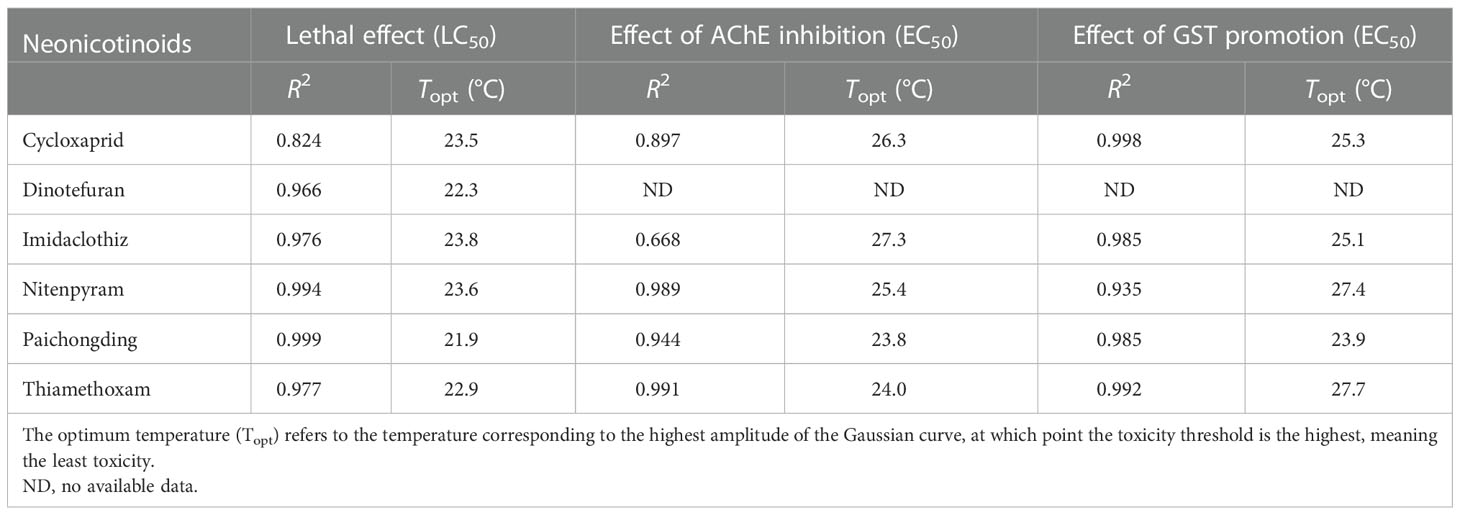
Table 3 Effect of temperature on the lethal and sublethal toxicity of neonicotinoids (R2 represents the goodness of nonlinear regression curves fitted by Gaussian distribution in GraphPad Prism (version 5.0, San Diego, CA, USA).
It is commonly reported that chemical toxicity increases with increasing temperature (linear model) (Leung et al., 2000; McConnachie and Alexander, 2004; Kwok and Leung, 2005), but a non-linear function (e.g., inverted V-shaped model) tends to describe the relationship between temperature and chemical toxicity more adequately with wider temperature ranges as demonstrated in further comprehensive toxicity studies (Heugens et al., 2003; Li et al., 2006; Bao et al., 2008; Osterauer and Köhler, 2008; Prato et al., 2008; Wong and Leung, 2013). Following the inverted V-shaped model, chemical toxicity to aquatic organisms was lowest at Topt and increased at a temperature below or above Topt. In general, aquatic organisms living in optimal conditions are more tolerant to chemical toxicity than those living in conditions near their thermal tolerance limits (Heugens et al., 2001; Li et al., 2014). Every aquatic organism has a thermal tolerance range (Cairns et al., 1975; Cairns et al., 1978); when aquatic ectotherms (e.g., fish) generally live within an optimum range of temperatures, they have sufficient energy and oxygen supply and strong activities of detoxification enzymes and antioxidant enzymes (Pörtner, 2010), resulting in a high clearance rate of exogenous toxins and low sensitivity to their toxicity (Frederich and Pörtner, 2000). When the temperature exceeds (higher/lower than) the optimal range, its internal energy budget cannot maintain its normal growth state (energy deficit) (Sokolova and Pörtner, 2007), the tolerance of the organism is reduced, and such a thermal stress at temperature extremes may further enhance the toxicity of a chemical (Jusup et al., 2016). Therefore, such an integrative inverted V-shaped response can be typically explained by the temperature-regulated mechanisms (Mu et al., 2018).
4. Conclusion
In this study, the multi-scale toxic effects of neonicotinoids on zebrafish embryos under exposure at different temperatures were investigated by using standard FET test. The neonicotinoids significantly inhibited the AChE activity but significantly promoted the GST activity, while GPX activity and ATPase activity were enhanced in the low-concentration treatment group and inhibited by high concentrations of neonicotinoids. Dinotefuran, imidaclothiz, and thiamethoxam were less toxic than cycloxaprid, nitenpyram, and paichongding. Neurotoxicity, oxidative stress responses such as changes of GPX and GST activities, and metabolic dysfunction indicators such as ATPase activities may be the key endpoints of neonicotinoid toxicity as revealed from our study. These multi-biomarkers can be used for monitoring the early contamination or long-term exposure of trace contaminants in water environments. Temperature-dependent toxicity thresholds (e.g., LC/EC50, LOEC, and NOEC) at multi-endpoints were determined by lethal and sublethal toxicity tests to fill the data gaps on neonicotinoids. The influence of temperature on neonicotinoid toxicity to zebrafish embryos represented an inverted V-shaped relationship where toxicity increased with increasing and decreasing temperature from Topt. Therefore, the influence of environmental parameters, especially temperature, should be considered in future toxicity testing and risk assessment of contaminants of emerging concern, including neonicotinoids, and such an inverted V-shaped model can support the prediction of temperature-dependent chemical toxicity to aquatic organisms and the enactment of appropriate water quality guidelines for protecting aquatic ecosystems under different thermal scenarios.
Data availability statement
The original contributions presented in the study are included in the article/Supplementary Material. Further inquiries can be directed to the corresponding authors.
Ethics statement
The animal study was reviewed and approved by the Ethics Committee in Shantou University (Approval Number: 20221205).
Author contributions
YYLW: investigation, methodology, and writing—original draft. Y-EC: investigation and writing—review and editing. SK, JY, PL, and WL: writing—review and editing. YW: investigation, supervision, and writing—review and editing. ZW: conceptualization, supervision, funding acquisition, and writing—review and editing. All authors contributed to the article and approved the submitted version.
Funding
This work research was supported by the National Natural Science Foundation of China, China (21906067), 2020 Li Ka Shing Foundation Cross-Disciplinary Research Grant, China (2020LKSFG03E), Shantou University Scientific Research Foundation for Talents, China (NTF19044), Innovation and Entrepreneurship Project of Shantou, China (201112176541391), and Science and Technology Plan Projects of Guangdong Province, China (Grant No. 2021B1212050025, STKJ2021125).
Conflict of interest
The authors declare that the research was conducted in the absence of any commercial or financial relationships that could be construed as a potential conflict of interest.
Publisher’s note
All claims expressed in this article are solely those of the authors and do not necessarily represent those of their affiliated organizations, or those of the publisher, the editors and the reviewers. Any product that may be evaluated in this article, or claim that may be made by its manufacturer, is not guaranteed or endorsed by the publisher.
Supplementary material
The Supplementary Material for this article can be found online at: https://www.frontiersin.org/articles/10.3389/fmars.2022.1101737/full#supplementary-material
References
Agrahari S., Gopal K. (2008). Inhibition of na+–K+-ATPase in different tissues of freshwater fish Channa punctatus (Bloch) exposed to monocrotophos. Pesticide Biochem. Physiol. 92, 57–60. doi: 10.1016/j.pestbp.2008.06.003
Akbar S. M. D., Sreeramulu K., Sharma H. C. (2016). “Monitoring biosafety of pharmaceutical drugs, insecticides, and other bioactive molecules to mitochondria,” in Atlas of science (Karnataka, India), 01–03.
Almeida É.C., Passos L. S., Vieira C. E. D., Acayaba R. D., Montagner C. C., Pinto E., et al. (2021). Can the insecticide imidacloprid affect the health of the Neotropical freshwater fish Astyanax altiparanae (Teleostei: Characidae)? Environ. Toxicol. Pharmacol. 85, 103634. doi: 10.1016/j.etap.2021.103634
Al-Rajhi D. H. (1990). Properties of Ca2+-Mg2+-ATPase from rat brain and its inhibition by pyrethroids. Pesticide Biochem. Physiol. 37, 116–120. doi: 10.1016/0048-3575(90)90116-J
Amarasekare P., Savage V.m. (2012). A framework for elucidating the temperature dependence of fitness. Am. Nat. 179, 178–191. doi: 10.1086/663677
Anderson J. C., Dubetz C., Palace V. P. (2015). Neonicotinoids in the Canadian aquatic environment: A literature review on current use products with a focus on fate, exposure, and biological effects. Sci. Total Environ. 505, 409–422. doi: 10.1016/j.scitotenv.2014.09.090
Angilletta M., Huey R., Frazier M. (2009). Thermodynamic effects on organismal performance: Is hotter better? Physiol. Biochem. Zool.: PBZ 83, 197–206. doi: 10.1086/648567
Azevedo-Pereira H., Lemos M., Soares A. (2011). Effects of imidacloprid exposure on Chironomus riparius meigen larvae: Linking acetylcholinesterase activity to behaviour. Ecotoxicol. Environ. Saf. 74, 1210–1215. doi: 10.1016/j.ecoenv.2011.03.018
Bao V., Koutsaftis A., Leung K. (2008). Temperature-dependent toxicities of chlorothalonil and copper pyrithione to the marine copepod Tigriopus japonicus and dinoflagellate Pyrocystis lunula. Australas. J. Ecotoxicol. 14, 45–54. doi: 10.1007/s10646-014-1297-4
Benchaâbane S., Ayad A. S., Loucif-Ayad W., Soltani N. (2022). Multibiomarker responses after exposure to a sublethal concentration of thiamethoxam in the African honeybee (Apis mellifera intermissa). Comp. Biochem. Physiol. Part C: Toxicol. Pharmacol. 257, 109334. doi: 10.1016/j.cbpc.2022.109334
Blasiak J. (1995). Inhibition of erythrocyte membrane (Ca2+-Mg2+)-ATPase by the organophosphorus insecticides parathion and methylparathion. Comp. Biochem. Physiol. Part C: Pharmacol. Toxicol. Endocrinol. 110, 119–125. doi: 10.1016/0742-8413(95)00004-8
Bourgeault A., Ciffroy P., Garnier C., Cossu-Leguille C., Masfaraud J.-F., Charlatchka R., et al. (2013). Speciation and bioavailability of dissolved copper in different freshwaters: Comparison of modelling, biological and chemical responses in aquatic mosses and gammarids. Sci. Total Environ. 452-453, 68–77. doi: 10.1016/j.scitotenv.2013.01.097
Brett J. R. (1979). “Environmental factors and growth,” in Fish physiology. Eds. Hoar W. S., Randall D. J., Brett J. R. (London and New York: Academic Press), 599–675.
Brocchi E., Navarro R., Moura F. (2013). A chemical thermodyanamics review applied to V2O5 chlorination. Thermochimica Acta 559, 1–16. doi: 10.1016/j.tca.2013.01.025
Burleson M., Silva P. (2011). Cross tolerance to environmental stressors: effects of hypoxic acclimation on cardiovascular responses of channel catfish (Ictalurus punctatus) to a thermal challenge. J. Thermal Biol. 36, 250–254. doi: 10.1016/j.jtherbio.2011.03.009
Cairns J., Buikema A., Heath A., Parker B. (1978). Effects of temperature on aquatic organism sensitivity to selected chemicals. Bulletin-Virginia Water Resour. Res. Center 106, 1–88.
Cairns J., Heath A., Parker B. (1975). The effects of temperature upon the toxicity of chemicals to aquatic organisms. Hydrobiologia 47, 135–171. doi: 10.1007/BF00036747
Chauhan V., Tsiouris J., Chauhan A., Sheikh A., Brown W., Vaughan M. (2002). Increased oxidative stress and decreased activities of Ca/Mg-ATPase and Na/K-ATPase in the red blood cells of the hibernating black bear. Life Sci. 71, 153–161. doi: 10.1016/S0024-3205(02)01619-3
Cherkasov A., Biswas P., Ridings D., Ringwood A., Sokolova I. (2006). Effects of acclimation temperature and cadmium exposure on cellular energy budgets in the marine mollusk Crassostrea virginica: Linking cellular and mitochondrial responses. J. Exp. Biol. 209, 1274–1284. doi: 10.1242/jeb.02093
Cherkasov A. S., Grewal S., Sokolova I. (2007). Combined effects of temperature and cadmium exposure on haemocyte apoptosis and cadmium accumulation in the eastern oyster Crassostrea virginica (Gmelin). J. Thermal Biol. 32, 162–170. doi: 10.1016/j.jtherbio.2007.01.005
Clasen B., Loro V., Cattaneo R., Moraes B., Lópes T., Avila L., et al. (2011). Effects of the commercial formulation containing fipronil on the non-target organism Cyprinus carpio: Implications for rice - fish cultivation. Ecotoxicol. Environ. Saf. 77, 45–51. doi: 10.1016/j.ecoenv.2011.10.001
Davis P. W., Wedemeyer G. A. (1971). Na+, k+-activated-ATPase inhibition in rainbow trout: A site for organochlorine pesticide toxicity? Comp. Biochem. Physiol. Part B: Comp. Biochem. 40, 823–827. doi: 10.1016/0305-0491(71)90157-X
DeCant J., Barrett M. (2010). Environmental fate and ecological risk assessment for the registration of clothianidin for use as a seed treatment on mustard seed (oilseed and condiment. and cotton). United States Environ. Prot. Agency.
Deng S., Wu Y., Duan H., Cavanagh J.-A. E., Wang X., Qiu J., et al. (2021). Toxicity assessment of earthworm exposed to arsenate using oxidative stress and burrowing behavior responses and an integrated biomarker index. Sci. Total Environ. 800, 149479. doi: 10.1016/j.scitotenv.2021.149479
Droge W. (2002). Free radicals in the physiological control of cell function. Physiol. Rev. 82, 47–95. doi: 10.1152/physrev.00018.2001
Farley R. A., Tran C. M., Carilli C. T., Hawke D., Shively J. E. (1984). The amino acid sequence of a fluorescein-labeled peptide from the active site of (Na,K)-ATPase. J. Biol. Chem. 259, 9532–9535. doi: 10.1016/S0021-9258(17)42732-3
Frazier M., Huey R., Berrigan D. (2006). Thermodynamics constrains the evolution of insect population growth rates: “Warmer is better”. Am. Nat. 168, 512–520. doi: 10.1086/506977
Frederich M., Pörtner H.-O. (2000). Oxygen limitation of thermal tolerance defined by cardiac and ventilatory performance in spider crab, Maja squinado. American journal of physiology. Regulatory Integr. Comp. Physiol. 279, R1531–R1538. doi: 10.1152/ajpregu.2000.279.5.R1531
Gallego-Ríos S. E., Peñuela G. A., Martínez-López E. (2021). Updating the use of biochemical biomarkers in fish for the evaluation of alterations produced by pharmaceutical products. Environ. Toxicol. Pharmacol. 88, 103756. doi: 10.1016/j.etap.2021.103756
Gibbons D., Morrissey C., Mineau P. (2014). A review of the direct and indirect effects of neonicotinoids and fipronil on vertebrate wildlife. Environ. Sci. pollut. Res. Int. 22, 103–118. doi: 10.1007/s11356-014-3180-5
Gravato C., Abe F. R., de Oliveira D. P., Soares A. M. V. M., Domingues I. (2021). Acetylcholinesterase (AChE) activity in embryos of zebrafish. Methods Mol. Biol. 2240, 119–124. doi: 10.1007/978-1-0716-1091-6_10
Guerra L. J., do Amaral A. M. B., de Quadros V. A., da Luz Fiuza T., Rosemberg D. B., Prestes O. D., et al. (2021). Biochemical and behavioral responses in zebrafish exposed to imidacloprid oxidative damage and antioxidant responses. Arch. Environ. Contamination Toxicol. 81, 255–264. doi: 10.1007/s00244-021-00865-9
Hayasaka D., Suzuki K., Nomura T., Nishiyama M., Nagai T., Sánchez-Bayo F., et al. (2013). Comparison of acute toxicity of two neonicotinoid insecticides, imidacloprid and clothianidin, to five cladoceran species. J. Pesticide Sci. 38, 44–47. doi: 10.1584/jpestics.D12-061
Heugens E. H., Hendriks A. J., Dekker T., van Straalen N. M., Admiraal W. (2001). A review of the effects of multiple stressors on aquatic organisms and analysis of uncertainty factors for use in risk assessment. Crit. Rev. Toxicol. 31, 247–284. doi: 10.1080/20014091111695
Heugens E. H., Jager T., Creyghton R., Kraak M. H., Hendriks A. J., Van Straalen N. M., et al. (2003). Temperature-dependent effects of cadmium on Daphnia magna: Accumulation versus sensitivity. Environ. Sci. Technol. 37, 2145–2151. doi: 10.1021/es0264347
Ji L., Chauhan A., Brown W., Chauhan V. (2009). Increased activities of Na+/K+-ATPase and Ca2+/Mg2+-ATPase in the frontal cortex and cerebellum of autistic individuals. Life Sci. 85, 788–793. doi: 10.1016/j.lfs.2009.10.008
Jusup M., Sousa T., Domingos T., Labinac V., Marn N., Wang Z., et al. (2016). Physics of metabolic organization. Phys. Life Rev. 20, 1–39. doi: 10.1016/j.plrev.2016.09.001
Kamler E., Keckei H., Bauer-Nemeschkal E. (1998). Temperature-induced changes of survival, development and yolk partitioning in Chondrostoma nasus. J. Fish Biol. 53, 658–682. doi: 10.1111/j.1095-8649.1998.tb01009.x
Kimmel C., Ballard W., Kimmel S., Ullmann B., Schilling T. (1995). Stages of embryonic-development of the zebrafish. Dev. Dynamics 203, 253–310. doi: 10.1002/aja.1002030302
Kumar N., Md S., Sharma H. C., Jayalakshmi S., Sreeramulu K. (2018). Imidacloprid impedes mitochondrial function and induces oxidative stress in cotton bollworm, Helicoverpa armigera larvae (Hubner: Noctuidae). J. Bioenergetics Biomembranes 50, 1–12. doi: 10.1007/s10863-017-9739-3
Kwok K., Leung K. (2005). Toxicity of antifouling biocides to the intertidal harpacticoid copepod Tigriopus japonicus (Crustacea, copepoda): Effects of temperature and salinity. Mar. pollut. Bull. 51, 830–837. doi: 10.1016/j.marpolbul.2005.02.036
Lacourse E. J., Hernandez-Viadel M., Jefferies J. R., Svendsen C., Spurgeon D. J., Barrett J., et al. (2009). Glutathione s-transferase (GST) as a candidate molecular-based biomarker for soil toxin exposure in the earthworm Lumbricus rubellus. Environ. pollut. 157, 2459–2469. doi: 10.1016/j.envpol.2009.03.015
Lauan M., Claret B., Ocampo, Pablo P. (2013). Low-dose effects of carbaryl, chlorpyrifos and imidacloprid insecticides on the gonad and plasma testosterone level of male juvenile and adult Nile tilapia (Oreochromis niloticus linnaeus). Asia Life Sci. 22.
Leung K., Taylor A., Furness R. (2000). Temperature-dependent physiological responses of the dogwhelk Nucella lapillus to cadmium exposure. J. Mar. Biol. Assoc. UK 80, 647–660. doi: 10.1017/S0025315400002472
Li X., Bai Y., Zhu W., Shi X., Xu S. (2022). The endoplasmic reticulum-mitochondrial crosstalk is involved in the mitigation mechanism of eucalyptol on imidacloprid toxicity in Ctenopharyngodon idellus kidney cells. Fish Shellfish Immunol. 127, 99–108. doi: 10.1016/j.fsi.2022.06.014
Li H., Feng T., Liang P., Shi X., Gao X., Jiang H. (2006). Effect of temperature on toxicity of pyrethroids and endosulfan, activity of mitochondrial na+-K+-ATPase and Ca2+-Mg2+-ATPase in Chilo suppressalis (Walker) (Lepidoptera: Pyralidae). Pesticide Biochem. Physiol. 86, 151–156. doi: 10.1016/j.pestbp.2006.03.001
Li J. A., Leung P., Bao V., Lui G., Leung K. (2014). Temperature-dependent physiological and biochemical responses of the marine medaka Oryzias melastigma with consideration of both low and high thermal extremes. J. Thermal Biol. 54, 98–105. doi: 10.1016/j.jtherbio.2014.09.011
Lin H., Lin F., Yuan J., Cui F., Chen J. (2021). Toxic effects and potential mechanisms of fluxapyroxad to zebrafish (Danio rerio) embryos. Sci. Total Environ. 769, 144519. doi: 10.1016/j.scitotenv.2020.144519
Lionetto M. G., Caricato R., Calisi A., Giordano M. E., Schettino T. (2013). Acetylcholinesterase as a biomarker in environmental and occupational medicine: new insights and future perspectives. BioMed. Res. Int. 2013, 321213. doi: 10.1155/2013/321213
Li C., Shi L., Chen D., Ren A., Gao T., Zhao M. (2015). Functional analysis of the role of glutathione peroxidase (GPx) in the ROS signaling pathway, hyphal branching and the regulation of ganoderic acid biosynthesis in ganoderma lucidum. Fungal Genet. Biol. 82, 168–180. doi: 10.1016/j.fgb.2015.07.008
Lu C., Chang C. H., Palmer C., Zhao M., Zhang Q. (2018). Neonicotinoid residues in fruits and vegetables: An integrated dietary exposure assessment approach. Environ. Sci. Technol. 52, 3175–3184. doi: 10.1021/acs.est.7b05596
Ma X., Li H., Xiong J., Mehler W. T., You J. (2019). Developmental toxicity of a neonicotinoid insecticide, acetamiprid to zebrafish embryos. J. Agric. Food Chem. 67, 2429–2436. doi: 10.1021/acs.jafc.8b05373
Marins A. T., Cerezer C., Leitemperger J. W., Severo E. S., Costa M. D., Fontoura D. O., et al. (2021). A mixture of pesticides at environmental concentrations induces oxidative stress and cholinergic effects in the neotropical fish Rhamdia quelen. Ecotoxicol. (London England) 30, 164–174. doi: 10.1007/s10646-020-02300-6
McConnachie S., Alexander G. (2004). The effect of temperature on digestive and assimilation efficiency, gut passage time and appetite in an ambush foraging lizard, cordylus melanotus. J. Comp. Physiol. B Biochemical Systemic Environ. Physiol. 174, 99–105. doi: 10.1007/s00360-003-0393-1
Meiliana A., Wijaya A. (2012). Mitochondrial dysfunction in metabolic disease. Indonesian Biomed. J. 4, 119. doi: 10.18585/inabj.v4i3.172
Meyer D., Williams P. L. (2014). Toxicity testing of neurotoxic pesticides in Caenorhabditis elegans. J. Toxicol. Environ. Health B: Crit. Rev. 17, 284–306. doi: 10.1080/10937404.2014.933722
Miles J., Hua J., Sepulveda M., Krupke C., Hoverman J. (2017). Effects of clothianidin on aquatic communities: Evaluating the impacts of lethal and sublethal exposure to neonicotinoids. Public Library Sci. One 12, e0174171. doi: 10.1371/journal.pone.0174171
Mitchell E. A. D., Mulhauser B., Mulot M., Mutabazi A., Glauser G., Aebi A. (2017). A worldwide survey of neonicotinoids in honey. Science 358, 109–111. doi: 10.1126/science.aan3684
Morrissey C. A., Mineau P., Devries J. H., Sanchez-Bayo F., Liess M., Cavallaro M. C., et al. (2015). Neonicotinoid contamination of global surface waters and associated risk to aquatic invertebrates: a review. Environ. Int. 74, 291–303. doi: 10.1016/j.envint.2014.10.024
Mu Y., Wang Z., Wu F., Zhong B., Yang M., Sun F., et al. (2018). Model for predicting toxicities of metals and metalloids in coastal marine environments worldwide. Environ. Sci. Technol. 52, 4199–4206. doi: 10.1021/acs.est.7b06654
Nicolas M. C. F., Ocampo P. P. (2006). Blood acetylcholinesterase (AChE) inhibition and melanomacrophage centers (MMC) formation in Nile tilapia (Oreochromis niloticus linn) as induced by exposure to chlorpyrifos insecticide. Philippine Entomologist 20, 187.
Ocampo P. P., Sagun V. G. (2007). Gonadal changes in male tilapia (Oreochromis niloticus linn.) after exposure to imidocloprid insecticide. Philippine Entomologist 21, 199–200.
OECD (2013). Test No. 236: OECD guidelines for the testing of chemicals–Fish embryo acute toxicity (FET) test. Organ. Econ. Coop. Dev.(Paris, France) pp. 1–22.
Ohi M., Dalsenter P., Martino-Andrade A., Nascimento A. (2004). Reproductive adverse effects of fipronil in wistar rats. Toxicol. Lett. 146, 121–127. doi: 10.1016/j.toxlet.2003.08.008
Osterauer R., Köhler H.-R. (2008). Temperature-dependent effects of the pesticides thiacloprid and diazinon on the embryonic development of zebrafish (Danio rerio). Aquat. Toxicol. (Amsterdam Netherlands) 86, 485–494. doi: 10.1016/j.aquatox.2007.12.013
Oyovwi M. O., Ben-Azu B., Tesi E. P., Oyeleke A. A., Uruaka C. I., Rotu R. A., et al. (2021). Repeated endosulfan exposure induces changes in neurochemicals, decreases ATPase transmembrane ionic-pumps, and increased oxidative/nitrosative stress in the brains of rats: Reversal by quercetin. Pesticide Biochem. Physiol. 175, 104833. doi: 10.1016/j.pestbp.2021.104833
Pan Y., Tian L., Zhao Q., Tao Z., Yang J., Zhou Y., et al. (2022). Evaluation of the acute toxic effects of crude oil on intertidal mudskipper (Boleophthalmus pectinirostris) based on antioxidant enzyme activity and the integrated biomarker response. Environ. pollut. 292, 118341. doi: 10.1016/j.envpol.2021.118341
Piner Benli P., Çelik M. (2021). In vivo effects of neonicotinoid-sulfoximine insecticide sulfoxaflor on acetylcholinesterase activity in the tissues of zebrafish (Danio rerio). Toxics 9, 73. doi: 10.3390/toxics9040073
Pörtner H.-O. (2010). Oxygen- and capacity-limitation of thermal tolerance: A matrix for integrating climate-related stressor effects in marine ecosystems. J. Exp. Biol. 213, 881–893. doi: 10.1242/jeb.037523
Prato E., Scardicchio C., Biandolino F. (2008). Effects of temperature on the acute toxicity of cadmium to Corophium insidiosum. Environ. Monit. Assess. 136, 161–166. doi: 10.1007/s10661-007-9672-8
Priya B., Maruthi Y. (2013). Imidacloprid toxicity on biochemical constituents in liver tissue of fresh water teleost Channa punctatus. Int. J. Pharma Bio Sci. 4, B50–B54.
Qi S., Wang C., Chen X., Qin Z., Li X., Wang C. (2013). Toxicity assessments with Daphnia magna of guadipyr, a new neonicotinoid insecticide and studies of its effect on acetylcholinesterase (AChE), glutathione s-transferase (GST), catalase (CAT) and chitobiase activities. Ecotoxicol. Environ. Saf. 98, 339–344. doi: 10.1016/j.ecoenv.2013.09.013
Sánchez-Bayo F., Goka K. (2005). Unexpected effects of zinc pyrithione and imidacloprid on Japanese medaka fish (Oryzias latipes). Aquat. Toxicol. (Amsterdam Netherlands) 74, 285–293. doi: 10.1016/j.aquatox.2005.06.003
Santana M. S., Sandrini-Neto L., Filipak Neto F., Oliveira Ribeiro C. A., Di Domenico M., Prodocimo M. M. (2018). Biomarker responses in fish exposed to polycyclic aromatic hydrocarbons (PAHs): Systematic review and meta-analysis. Environ. pollut. 242, 449–461. doi: 10.1016/j.envpol.2018.07.004
Scheil V., Köhler H.-R. (2009). Influence of nickel chloride, chlorpyrifos, and imidacloprid in combination with different temperatures on the embryogenesis of the zebrafish Danio rerio. Arch. Environ. Contamination Toxicol. 56, 238–243. doi: 10.1007/s00244-008-9192-8
Schiedek D., Sundelin B., Readman J., Macdonald R. (2008). Interactions between climate change and contaminants. Mar. pollut. Bull. 54, 1845–1856. doi: 10.1016/j.marpolbul.2007.09.020
Serafini S., de Freitas Souza C., Baldissera M. D., Baldisserotto B., Segat J. C., Baretta D., et al. (2019). Fish exposed to water contaminated with eprinomectin show inhibition of the activities of AChE and Na+/K+-ATPase in the brain, and changes in natural behavior. Chemosphere 223, 124–130. doi: 10.1016/j.chemosphere.2019.02.026
Sevgiler Y., Atlı G. (2022). Sulfoxaflor, Zn2+ and their combinations disrupt the antioxidant and osmoregulatory (Ca2+-ATPase) system in Daphnia magna. J. Trace Elements Med. Biol. 73, 127035. doi: 10.1016/j.jtemb.2022.127035
Sheets L., Li A., Minnema D., Collier R., Creek M., Peffer R. (2015). A critical review of neonicotinoid insecticides for developmental neurotoxicity. Crit. Rev. Toxicol. 46, 1–38. doi: 10.3109/10408444.2015.1090948
Shukla S., Jhamtani R. C., Dahiya M. S., Agarwal R. (2017). Oxidative injury caused by individual and combined exposure of neonicotinoid, organophosphate and herbicide in zebrafish. Toxicol. Rep. 4, 240–244. doi: 10.1016/j.toxrep.2017.05.002
Sokolova I., Pörtner H.-O. (2007). The role of thermal environment in stress adaptation and tolerance: Integration of multiple stressors - preface. J. Thermal Biol. 32, 117. doi: 10.1016/j.jtherbio.2007.01.006
Solomando A., Cohen-Sánchez A., Box A., Montero I., Pinya S., Sureda A. (2022). Microplastic presence in the pelagic fish, Seriola dumerili, from Balearic islands (Western Mediterranean), and assessment of oxidative stress and detoxification biomarkers in liver. Environ. Res. 212, 113369. doi: 10.1016/j.envres.2022.113369
Su T., Lian D., Bai Y., Wang Y. Y. L., Zhang D., Wang Z., et al. (2021). The feasibility of the zebrafish embryo as a promising alternative for acute toxicity test using various fish species: A critical review. Sci. Total Environ. 787, 147705. doi: 10.1016/j.scitotenv.2021.147705
Suvetha L., Ramesh M., Saravanan M. (2010). Influence of cypermethrin toxicity on ionic regulation and gill Na+/K+-ATPase activity of a freshwater teleost fish Cyprinus carpio. Environ. Toxicol. Pharmacol. 29, 44–49. doi: 10.1016/j.etap.2009.09.005
Tian X., Hong X., Yan S., Li X., Wu H., Lin A., et al. (2020). Neonicotinoids caused oxidative stress and DNA damage in juvenile Chinese rare minnows (Gobiocypris rarus). Ecotoxicol. Environ. Saf. 197, 110566. doi: 10.1016/j.ecoenv.2020.110566
Tian X., Yang W., Wang D., Zhao Y., Yao R., Ma L., et al. (2018). Chronic brain toxicity response of juvenile Chinese rare minnows (Gobiocypris rarus) to the neonicotinoid insecticides imidacloprid and nitenpyram. Chemosphere 210, 1006–1012. doi: 10.1016/j.chemosphere.2018.06.083
Topal A., Alak G., Ozkaraca M., Yeltekin A. C., Comaklı S., Acıl G., et al. (2017). Neurotoxic responses in brain tissues of rainbow trout exposed to imidacloprid pesticide: Assessment of 8-hydroxy-2-deoxyguanosine activity, oxidative stress and acetylcholinesterase activity. Chemosphere 175, 186–191. doi: 10.1016/j.chemosphere.2017.02.047
Tsui M. T., Wang W. X. (2006). Acute toxicity of mercury to Daphnia magna under different conditions. Environ. Sci. Technol. 40, 4025–4030. doi: 10.1021/es052377g
Ullah S., Ahmad S., Altaf Y., Dawar F. U., Anjum S. I., Baig M. M. F. A., et al. (2022). Bifenthrin induced toxicity in Ctenopharyngodon idella at an acute concentration: A multi-biomarkers based study. J. King Saud Univ. - Sci. 34, 101752. doi: 10.1016/j.jksus.2021.101752
Van der Have T. (2002). A proximate model for thermal tolerance in ectotherms. Oikos 98, 141–155. doi: 10.1034/j.1600-0706.2002.980115.x
Veedu S. K., Ayyasamy G., Tamilselvan H., Ramesh M. (2022). Single and joint toxicity assessment of acetamiprid and thiamethoxam neonicotinoids pesticides on biochemical indices and antioxidant enzyme activities of a freshwater fish Catla catla. Comp. Biochem. Physiol. Part C: Toxicol. Pharmacol. 257, 109336. doi: 10.1016/j.cbpc.2022.109336
Vinagre C., Madeira D., Mendonça V., Dias M., Roma J., Diniz M. S. (2014). Effect of increasing temperature in the differential activity of oxidative stress biomarkers in various tissues of the rock goby, Gobius paganellus. Mar. Environ. Res. 97, 10–14. doi: 10.1016/j.marenvres.2014.01.007
Wang Y. Y. L., Li P., Ohore O. E., Wang Y., Zhang D., Bai Y., et al. (2021). Life stage and endpoint sensitivity differences of fathead minnow (Pimephales promelas) to chemicals with various modes of action. Environ. pollut. 290, 117995. doi: 10.1016/j.envpol.2021.117995
Wang Z., Lui G. C., Leung K. (2019). Thermal extremes can intensify chemical toxicity to freshwater organisms and hence exacerbate their impact to the biological community. Chemosphere 224, 256–264. doi: 10.1016/j.chemosphere.2019.02.103
Wang K., Qi S., Mu X., Chai T., Yang Y., Wang D., et al. (2015). Evaluation of the toxicity, AChE activity and DNA damage caused by imidacloprid on earthworms, Eisenia fetida. Bull. Environ. Contamination Toxicol. 95, 475–480. doi: 10.1007/s00128-015-1629-y
Wong S., Leung K. (2013). Temperature-dependent toxicities of nano zinc oxide to marine diatom, amphipod and fish in relation to its aggregation size and ion dissolution. Nanotoxicology 8, 24–35. doi: 10.3109/17435390.2013.848949
Yan S., Wang J., Zhu L., Chen A., Wang J. (2015). Toxic effects of nitenpyram on antioxidant enzyme system and DNA in zebrafish (Danio rerio) livers. Ecotoxicol. Environ. Saf. 122, 54–60. doi: 10.1016/j.ecoenv.2015.06.030
Yan S. H., Wang J. H., Zhu L. S., Chen A. M., Wang J. (2016). Thiamethoxam induces oxidative stress and antioxidant response in zebrafish (Danio rerio) livers. Environ. Toxicol. 31, 2006–2015. doi: 10.1002/tox.22201
Zhang J., Hao H., Wu X., Wang Q., Chen M., Feng Z., et al. (2020). The functions of glutathione peroxidase in ROS homeostasis and fruiting body development in hypsizygus marmoreus. Appl. Microbiol. Biotechnol. 104, 10555–10570. doi: 10.1007/s00253-020-10981-6
Zhang H., Zhao L. (2017). Influence of sublethal doses of acetamiprid and halosulfuron-methyl on metabolites of zebra fish (Brachydanio rerio). Aquat. Toxicol. 191, 85–94. doi: 10.1016/j.aquatox.2017.08.002
Zhou G. J., Wang Z., Lau E. T. C., Xu X. R., Leung K. M. Y. (2014). Can we predict temperature-dependent chemical toxicity to marine organisms and set appropriate water quality guidelines for protecting marine ecosystems under different thermal scenarios? Mar. pollut. Bull. 87, 11–21. doi: 10.1016/j.marpolbul.2014.08.003
Keywords: test alternatives, fish embryo toxicity test, insecticides, temperature-dependent chemical toxicity, ecological risk assessment
Citation: Wang YYL, Cai Y-E, Kazmi SSUH, Yang J, Wang Y, Li P, Liu W and Wang Z (2023) Temperature-dependent effects of neonicotinoids on the embryonic development of zebrafish (Danio rerio). Front. Mar. Sci. 9:1101737. doi: 10.3389/fmars.2022.1101737
Received: 18 November 2022; Accepted: 21 December 2022;
Published: 17 January 2023.
Edited by:
Carlos Gravato, University of Lisbon, PortugalReviewed by:
Gaetana Napolitano, University of Naples Parthenope, ItalyKathryn Hassell, RMIT University, Australia
Copyright © 2023 Wang, Cai, Kazmi, Yang, Wang, Li, Liu and Wang. This is an open-access article distributed under the terms of the Creative Commons Attribution License (CC BY). The use, distribution or reproduction in other forums is permitted, provided the original author(s) and the copyright owner(s) are credited and that the original publication in this journal is cited, in accordance with accepted academic practice. No use, distribution or reproduction is permitted which does not comply with these terms.
*Correspondence: Yuwen Wang, d2FuZ3l1d2VuQHN0dS5lZHUuY24=; Zhen Wang, emhlbndhbmdAc3R1LmVkdS5jbg==