- 1Department of Oceanography, College of Natural Sciences, Pusan National University, Busan, Republic of Korea
- 2Global Ocean Research Center, Korea Institute of Ocean Science and Technology, Busan, Republic of Korea
- 3Deep-Sea and Seabed Mineral Resources Research Center, Korea Institute of Ocean Science and Technology, Busan, Republic of Korea
- 4Department of Geological Science, College of Natural Sciences, Pusan National University, Busan, Republic of Korea
- 5Department of Oceanography and Marine Research Institute, Pusan National University, Busan, Republic of Korea
This study investigated ferromanganese oxide (Fe-Mn oxide) precipitated on porifera located on the tabletop of the Magellan seamount (OSM17, 1571 m depth) in the western Pacific. As the growth rate of Fe-Mn oxide is several mm/Myr and porifera skeletons are difficult to preserve posthumously, geochemical and mineralogical studies have not yet been conducted on this topic. Fe-Mn oxides from porifera have a morphological differentiation from general ferromanganese deposits because porifera act as substrates for the Fe-Mn oxide. The thickness of Fe-Mn oxide cannot be visually confirmed because it thinly precipitates on the skeletons of porifera. Therefore, high-resolution fluctuations are reflected in marine environmental factors, such as redox conditions and surface productivity over tens of thousands of years, compared to ferromanganese deposits representing tens of millions of years. Hence, the geochemical and mineralogical characteristics were investigated. Porifera skeletons were confirmed, displaying a stratified lattice-like structure, thickness of Fe-Mn oxide of up to 110 μm, and age of approximately 55,000 years. Irregular distribution of major elements of Fe-Mn oxide, Mn, Fe, Ni, and Co was due to the characteristics of the structures. The presence of Fe-vernadite, identified by quantitative and mineralogical analysis, indicates oxidative environmental conditions at the tabletop of OSM17. Biomineralization was identified by confirming the presence of Fe-Mn oxides with a globular, sheath-like structure. Because of its conservation state, species identification of porifera was not based on the shape of the spicule, but was confirmed to be Farrea occa. This study verified that the tabletop of OSM17 has been in an oxidative environment for approximately 55,000 years, and through species identification, high dissolved silica (DSi) concentrations provide appropriate conditions for Farrea occa to survive. The sample in this study can be used as a new indicator in paleo-environment research.
1 Introduction
Ferromanganese nodules are mostly found in the abyssal plain and ferromanganese crusts covering the surface of seamount slopes. The hydrogenetic origin of ferromanganese deposits refers to the formation of these deposits by direct precipitation of Mn oxide and Fe oxyhydroxide from ambient seawater. Oxygen rich bottom water reacts with dissolved Mn and Fe in the Oxygen minimum zone (OMZ) (Hein et al., 2000; Hein and Koschinsky, 2014). Mn oxide and Fe oxyhydroxide produce secondary colloids, and alternating layers appear because of the negative and positive surface charges, respectively (Wang and Müller, 2009). Their adsorption capacity is high; thus, Ni, Cu, Zn, and other rare earth elements, which are considered potential future resources, are adsorbed from seawater to Mn oxide and Fe oxyhydroxide (Glasby, 2006; Hein et al., 2013). Ferromanganese deposits are considered a closed system wherein seawater cannot not easily enter the center of the deposit. These deposits are formed at an extremely slow growth rate of several millimeters per million years; each layer reflects several paleo-environmental factors, such as the bottom current (Hein and Koschinsky, 2014), redox condition (Yang et al., 2019), and surface productivity (Jiang et al., 2019). These features are suitable for reconstructing paleo-environments through ferromanganese deposits.
Porifera that served as substrates for ferromanganese (Fe-Mn) oxide precipitation in this study are typical filter feeders and the oldest metazoans (Van Soest et al., 2012); records exist since the beginning of the Phanerozoic Eon (approximately 540 Mya; Łukowiak, 2020). In addition, porifera are distributed worldwide and more than 8,500 species have been discovered, with new species continuously being reported (Van Soest et al., 2012). Siliceous species, Demosponges, Hexactinellida, and Homoscleromorpha take up Si from the ambient seawater through complex enzymatic and metabolic processes (Alvarez et al., 2017). These classes of porifera play an important role in the global silicon cycle due to their global distribution (Alvarez et al., 2017). Si uptake depends on the availability of highly dissolved silica (DSi) in the ambient seawater; therefore, DSi is a limiting factor in controlling porifera survival. Therefore, it is important to reconstruct the fluctuations of the past DSi with the δ30Si isotope of the spicule that serves as the skeleton of the porifera; however, high-resolution reconstruction is difficult due to the rare occurrence of spicule fossils in sediment cores (Fontorbe et al., 2017) compared to that of radiolarian in the sediment core.
This study sampled Fe-Mn oxide precipitated on skeletal porifera located at the tabletop of OSM (open seamount)17, western Pacific. In the case of porifera, spicules, which are hard parts composed of silica, may remain posthumously (Benton and Harper, 2009); however, porifera with preserved structures in fossils are rarely found. Both porifera and Fe-Mn oxides show a global distribution (Taylor et al., 2007; Mizell and Hein, 2018), which makes research on this topic possible because paleo-environmental research has not yet been conducted through morphological, geochemical, and mineralogical studies on these types of samples. Iyer (1999) conducted mineralogical and geochemical analyses of samples of Fe-Mn oxide precipitated on shark teeth. In addition, previous studies have been undertaken on Fe-Mn oxide precipitated on soft substrates, which are the least abundant substrate type of the common substrates (Hein and Morgan, 1999; Hein et al., 2000; Marino et al., 2017; Ortiz Kfouri et al., 2021); however, there are currently no studies on species identification of substrates and Fe-Mn oxide precipitated on porifera (FMP).
FMP differs from ferromanganese deposits in that the skeleton of porifera acts as a substrate for Fe-Mn oxide precipitation, which indicates that seawater can flow into and out of the substrate. A thinner Fe-Mn oxide layer on the porifera skeleton than that of ferromanganese deposits reflects a relatively recent environment. In addition, porifera have various internal symbiotic microorganisms (Taylor et al., 2007), which can facilitate biomineralization during Fe-Mn oxide precipitation. In this study, the geochemical and mineralogical analyses of Fe-Mn oxide were performed to investigate the formation mechanism and to reconstruct the paleo-environment at the OSM17 tabletop. In addition, the study aimed to confirm survival conditions through porifera species identification and verify environmental factors, such as dissolved silica concentration, that cannot be observed in general ferromanganese deposits. Therefore, FMP can be applied as a new indicator in the reconstruction of the Magellan seamount cluster paleo-environment within tens of thousands of years, as well as suggest the correct approach for future research.
2 Material and methods
2.1 Study area
The western Pacific Magellan seamount cluster, OSM17, was identified as the study area. This Magellan seamount cluster provides suitable conditions for the formation of ferromanganese deposits due to its low sedimentation rate (0.4–4 mm/Ky) and oxygen-rich Antarctic bottom waterflows. The Magellan seamount cluster was created by volcanic activity south of the equator during the Cretaceous period (Glasby et al., 2007), which is now located in the region due to the movement of the Pacific plate (Clouard and Bonneville, 2015).
2.2 Sample
The FMP used in this study was obtained using an epibenthic sledge at the tabletop of OSM17, in the western Pacific (location: 151°38′40″E, 19°44′40″N; depth: 1571 m; Figure 1). Black ferromanganese oxides appeared to be precipitated on the outsides of the skeletons of the porifera but did not appear to be densely formed into ferromanganese nodules or crusts (Figure 2). The overall appearance of the FMP was cylindrical, with the osculum having a diameter of 10–13 mm and length of approximately 7.3 cm. The inside of the FMP had a stratified lattice-like structure and thickness of approximately 3 mm, including the precipitated ferromanganese oxide and skeleton of the porifera.
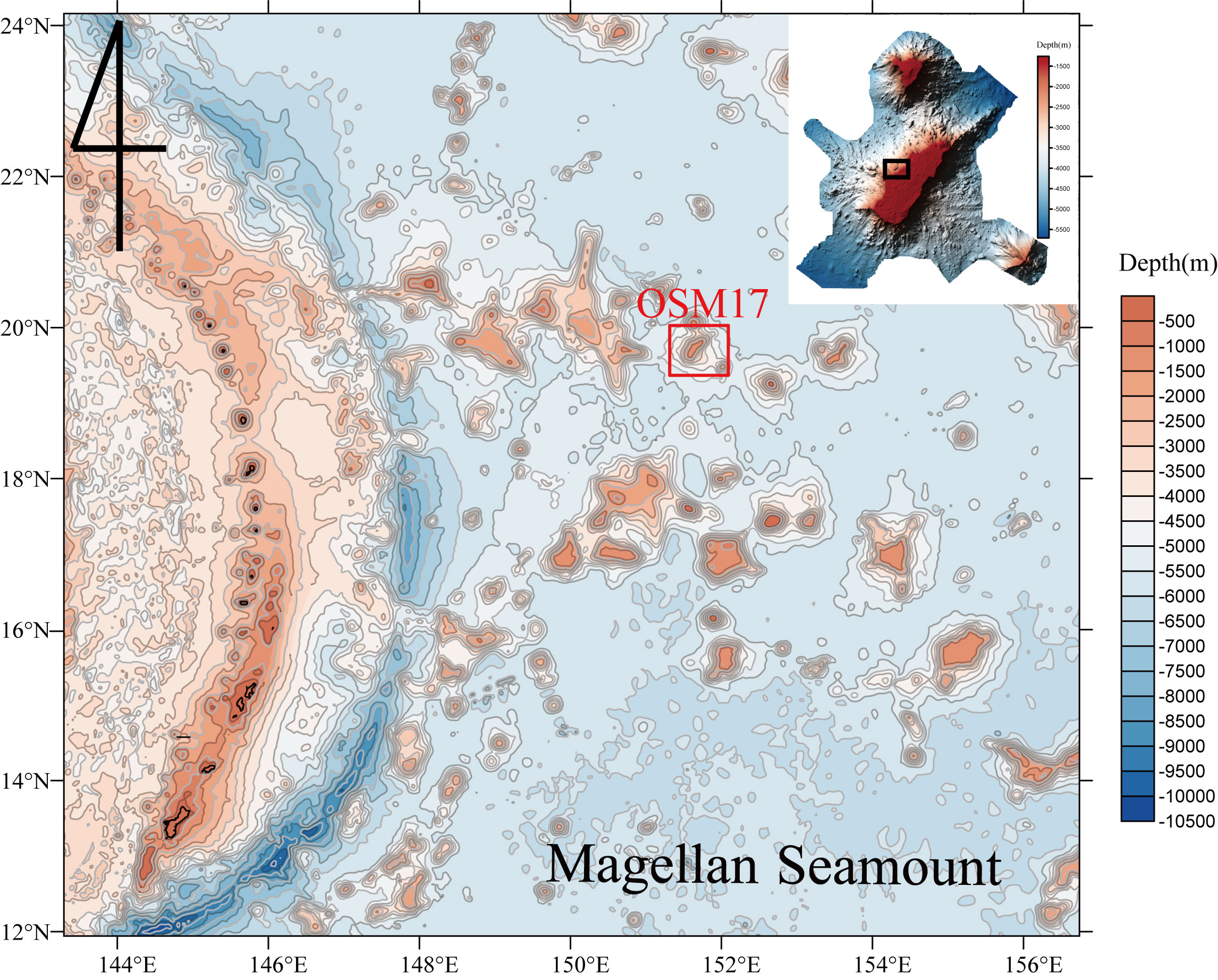
Figure 1 Bathymetry map of the western Pacific. Upper right of the map is a detailed bathymetry map of the study area, OSM17, using multibeam data. The black square denotes the epibenthic sledge site.
2.3 Micro-CT
Because of the stratified lattice-like structure of the FMP, which is the substrate specificity of FMP, it is impossible to visually measure the thickness of Fe-Mn oxide precipitated on the porifera skeleton; therefore, this study attempted to directly measure the thickness of the Fe-Mn oxide using Micro-computed tomography (Micro-CT) analysis. The sample used for the analysis was a 1 × 1 cm portion of the FMP. Micro-CT used Xradia Versa 510 (ZEISS, Germany) from the Wonju Center of the Korea Institute of Industrial Technology (KITECH). The analysis was conducted with a voltage of 139.77 kV, 9.8 W X-ray source, and the detector resolution was 2048 × 2048 pixels, with each pixel being 5 μm. The program used for the analysis was the Digital Imaging and Communications (DICOM) file in the Dragonfly software (Object Research Systems, Canada, version 2022.1).
2.4 μ-XRF
Micro-X-ray fluorescence (μ-XRF), used for geochemical analysis, was measured using the Bruker M4 Tornado μ-XRF (Bruker, Germany) from the Wonju Center of the Korea Institute of Industrial Technology (KITECH) with the Mo-anode tube operating at 10 kV, a spot size of 25 μm, and accuracy of ±5%. In order to confirm the growth mechanism of Fe-Mn oxide, a portion of the FMP was cut with a cutter and polished using film sandpaper for analysis. The distribution of the main elements of FMP, such as Si, Mn, Fe, Ni, Co, and Cu, was confirmed. Through quantitative analysis using built-in software, the genesis of Fe-Mn oxide was confirmed by the ratio of Mn/Fe (Halbach et al., 1981).
2.5 HR-XRD
To analyze the mineral species of Fe-Mn oxide that could not be confirmed through conventional XRD analysis due to its low crystallinity, a portion of the FMP was ground and powdered with a mortar for HR-XRD analysis, which was performed at the Pohang Accelerator Laboratory (PAL) 5A MS-XRS beamline. The powdered sample was loaded into a 0.5 mm diameter Boron-rich capillary (Charles Super Company, Inc., Natick, Ma, USA) and irradiated with a 0.69265 Å X-ray wavelength for 15 s from 2θ angles from 5°–70°. Crystallographica Search-Match (Golden Software, USA, version 2.0.3.1) was then used to identify the mineral species.
2.6 SEM-EDS
To investigate the effect of biomineralization during precipitation of Fe-Mn oxide, this study attempted to confirm the morphological characteristics of Fe-Mn oxide through scanning electron microscope- energy dispersive spectroscopy (SEM-EDS) analysis (Thomas et al., 2016). A sample of platinum coated and fixed on a carbon tape underwent SEM-EDS analysis to determine the occurrence of biomineralization. The SEM GEMINI500 (ZEISS, Germany) was used at the Core Research Facilities at Pusan National University, Busan, Korea. Secondary electron images were obtained using a voltage of 15 keV, working distance of 8.5 mm, and aperture of 20 μm, and EDS profiles were obtained using a voltage of 15 keV, working distance of 8.5 mm, and aperture of 60 μm.
3 Results
3.1 Micro-CT
In FMP, since Fe-Mn oxide is precipitated on the skeleton of the stratified lattice-like structure, the thickness of the cross-section of an FMP does not truly reflect the thickness of the precipitated Fe-Mn oxide. The Fe-Mn oxide layer is extremely thin and, therefore, cannot be distinguished by the naked eye; hence, the thickness of Fe-Mn oxide was measured using Micro-CT. The results of the Micro-CT analysis (Figure 3) confirm that the skeleton of the lattice-like structure existed through the xy and yz scanning axes. When exposed to X-rays, Fe-Mn oxides have an attenuation coefficient considerably different from that of the porifera skeletons. Bright colors indicate high X-ray absorption, indicating more dense material, whereas dark shades indicate low X-ray absorption and less dense material (Benites et al., 2018). The bright appearance and high density in the FMP is the precipitated Fe Mn oxide. In contrast, the darker shades, which are the most common, indicate the porifera skeletons. The thickest layer of Fe-Mn oxide, which indicated the commencement of the precipitation of Fe-Mn oxide, was determined to be 110 μm. The growth rate at the outermost edge of the ferromanganese crust found in OSM7 of the Magellan seamount cluster was approximately 2 mm/Myr (Kim et al., 2006), and it can be deduced that the Fe-Mn oxide precipitated approximately 55,000 years ago following the death of the porifera.
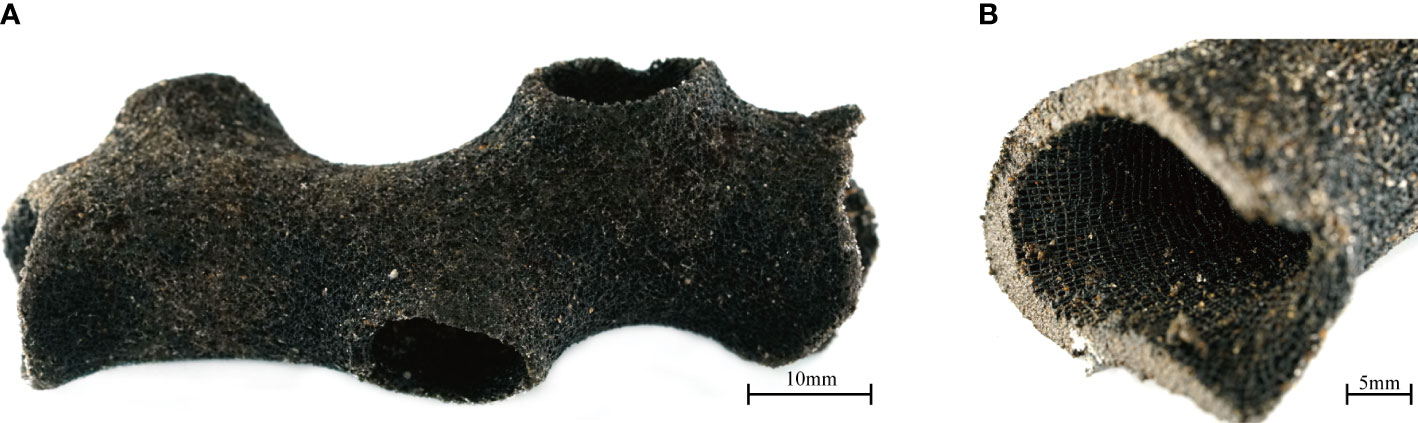
Figure 2 Photographs of the FMP sample: (A) Black colored ferromanganese oxide precipitated on the porifera and (B) section of FMP showing stratified lattice-like structure inside.
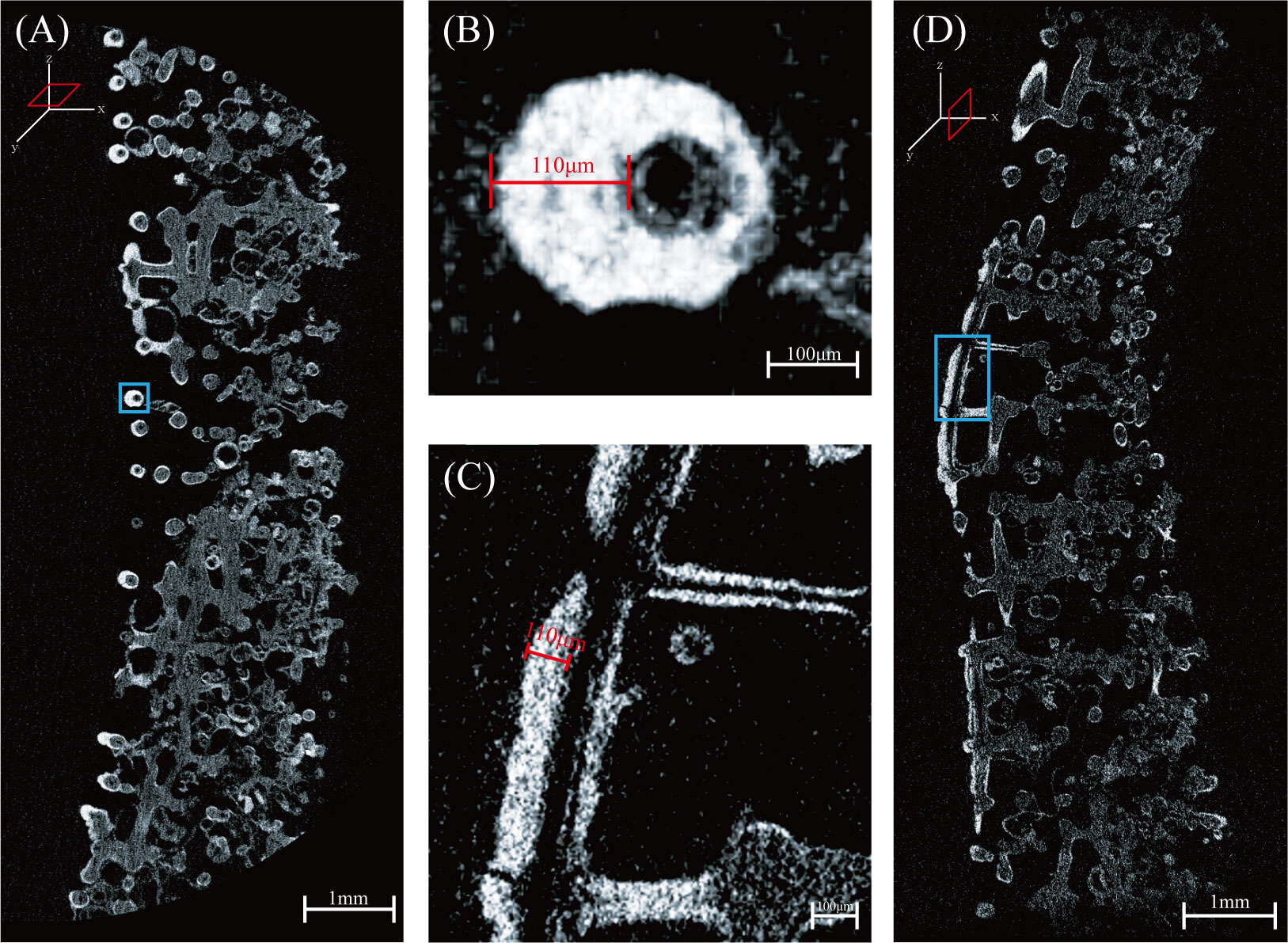
Figure 3 Three-dimensional cross sectional micro-computed tomography scan images of FMP: (A) and (D) are images scanned in the xy and yz scanning axes, respectively, and (B) and (C) are enlarged images of (A) and (D), respectively. Bright areas indicate ferromanganese oxide with a thickness of approximately 110 μm.
3.2 μ-XRF
The μ-XRF results (Figure 4), suggest a very high Si intensity; this is because the spicules that make up the skeletons of the porifera comprise biomineralized Si (Müller et al., 2007). Unlike general ferromanganese deposits, Mn and Fe, which are the main elements of Fe-Mn oxide, showed an irregular distribution and did not form a layer. Co and Ni showed a similar distribution to that of Mn because they were incorporated into the Mn-oxide (Hein et al., 2000; Yang and Jung, 2022). Cu showed a similar distribution layer to Si and was distributed along the skeleton of the porifera; this distribution occurs because Cu acts as a micronutrient for symbiotic microorganisms in the porifera (Coale and Bruland, 1988), and Cu is assumed to be involved in the structure of the multicopper oxidase (Yang and Jung, 2022). Based on this, it is evident that Cu appeared via a biogenic process (Hein and Morgan, 1999; Hein et al., 2000). The major elements of FMP are irregularly distributed because porifera, which acts as a substrate for the oxidized form of Mn and Fe, has a lattice-like structure that allows seawater to enter and exit the structure; therefore, major elements are not precipitated sequentially due to different skeletal shapes.
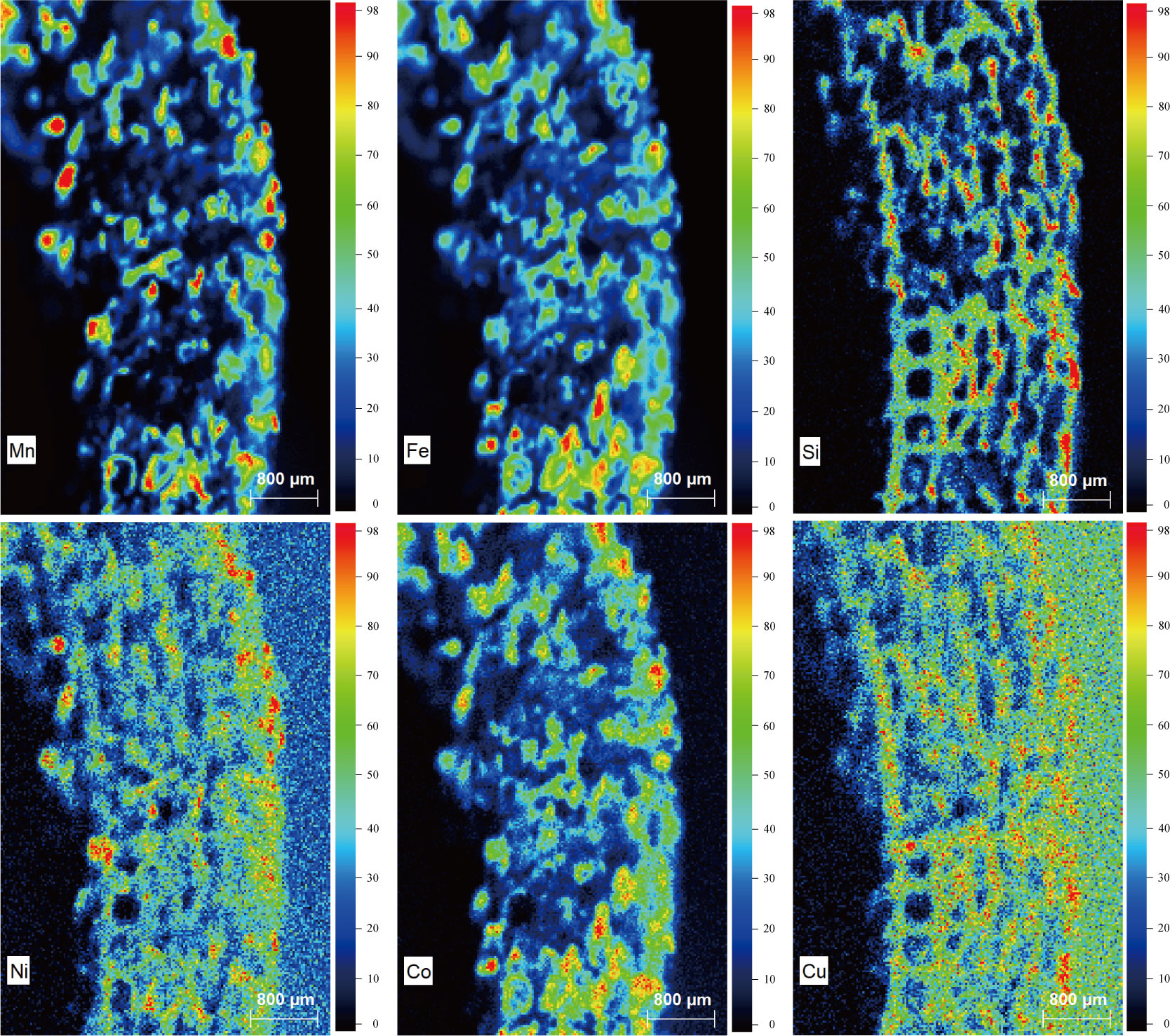
Figure 4 Major elemental distribution of FMP. Intensities are indicated by the color index at the right of each illustration. Mn, Fe, Ni and Co are of hydrogenetic origin and Si and Cu are of biogenic origin.
Quantitative analyses of the major elements constituting FMP were conducted by dividing the lattice-like structure into the inner, where it appeared rough, and outer portions, where it appeared dense (Table 1). In the inner portion, Fe was the highest at 31.00 wt%, followed by Si (24.99 wt%), Mn (18.96 wt%), and Ca (18.04 wt%). In the outer portion, Si was the highest at 50.83 wt%, followed by Fe (22.86 wt%), Mn (12.42 wt%), and Ca (7.85 wt%). The Mn/Fe ratio (which can confirm the genesis of formation) was 0.61 and 0.54 for the inner and outer portions, respectively, and the genesis of precipitation of the Fe-Mn oxide on the porifera was confirmed to be of hydrogenetic origin (Halbach et al., 1981; Glasby, 2006; Hein and Koschinsky, 2014; Menendez et al., 2019).
3.3 HR-XRD
The high-resolution X-ray diffractometer (HR-XRD) results for the Fe-Mn oxide and porifera skeleton powder samples (Figure 5) confirm that vernadite (V, δ-MnO2), quartz (Q), calcite (C), and feldspar (F) were present. Vernadite is formed by direct precipitation of Fe oxyhydroxide and Mn oxide on the substrate. It is evident that the Fe-Mn oxide precipitated on the FMP, which was confirmed by HR-XRD, formed via hydrogenesis, which is consistent with the mineralogical analysis of the outermost part of the ferromanganese deposits from the Magellan seamount cluster (Glasby, 2006; Yang et al., 2019).
Moreover, this result is consistent with the Mn/Fe ratio results analyzed by μ-XRF. Silicate minerals were easily detected because they constitute the skeleton of Hexactinellida (Drozdov et al., 2021). Feldspar was determined to be the detrital component (Zawadzki et al., 2022), and calcite appeared to have originated from calcareous ooze sediments at the tabletop of OSM17. The fact that Fe was identified in the μ-XRF analysis but did not appear in the XRD is a typical characteristic of Fe-vernadite; it is not detected in XRD analysis because it is filled with an amorphous form, which is commonly epitaxially intergrown with δ-MnO2 (Hein et al., 2000; Machida et al., 2021; Deng et al., 2022).
3.4 SEM
The results of the secondary electron images and EDS analysis of the FMP are displayed in Figure 6. Figure 6A shows the analysis conducted by targeting a place presumed to be a skeleton of porifera that exists in a smooth form and hardly shows sediments. Observation in the EDS spectrum showed peaks of C, Na, Pt, and Si, whereas Mn and Fe, assumed to be in the form of Fe-Mn oxide, were not identified. The high levels of Si in the EDS point, shown in Figure 6A, were attributed to the silicate mineral composition of the porifera skeletons (Uriz et al., 2003; Drozdov et al., 2021), which is the result of the influence of enzymatically controlled biomineralization of DSi in the ambient seawater (Wang et al., 2009; Arasuna et al., 2018).
Figure 6B shows an image of the Fe-Mn oxide portion where the precipitate was present on the skeleton. EDS analysis was conducted on the portion that had a globular and sheath-like structure; this is a known characteristic of biomineralization, which has been identified in previous studies (Wang et al., 2009; Wang et al., 2012; Jiang et al., 2019; Park and Kim, 2020). From the analysis results, it was possible to confirm the EDS profile with high Mn and Fe that did not appear in the other EDS points. In addition, the results show that biomineralization occurred to a certain extent under the influence of microorganisms, but precipitation of Fe-Mn oxide was primarily of hydrogenetic origin.
Figures 6C, D confirm the presence of foraminifera and coccolith, respectively, in the morphological and high Ca, C, and O profiles (Langer et al., 2011; Weiner et al., 2016). The presence of foraminifera and coccolith was attributed to the calcareous ooze sediment in the study area.
4 Discussion
4.1 Identification of porifera species and reconstruction of the paleo-environment through porifera life cycle
The identification of porifera species proceeds with the appearance of the porifera and formation of spicules that make up its skeleton (Van Soest et al., 2012). Depending on the size, spicules can be typically divided into macrosclere and microsclere, but there are some exceptions, which make differentiation difficult (Łukowiak, 2020). In addition, it is difficult to proceed with the follow-up process to determine the form of spicules because the SEM analysis of FMP does not confirm the complete shape of spicules. Therefore, the species of porifera were identified based on the overall appearance, internal characteristics, porifera distribution revealed in previous studies, water depth, and DSi concentration.
The μ-XRF analysis results indicate that the species of porifera could potentially be Demospongiae, Hexactinellida, or Homosclerophorida based on its siliceous skeleton (Van Soest et al., 2012). Due to its internal lattice-like structure (identified in Figure 2B.), it was narrowed down to a species of Hexactinellida (Leys et al., 2007). As oscula were identified in Figure 2A, which were separated from the main tube in the FMP, it was determined that the porifera were of the class Hexactinellida, order Sceptrulophora, family Farreidae, genus Farrea, and species Farrea occa.
Although Farrea occa has a global distribution and the depth at which it is found varies depending on the region (Reiswig, 2002), most of the Farreidae family survive at depths greater than 1000 m and a DSi greater than 100 μM (Alvarez et al., 2017). The OSM17 tabletop was located at a depth of 1000 m during the late-Cretaceous-early-Paleocene period and now exists at a deeper depth due to the movement of the Pacific plate (Smith et al., 1989). The DSi concentrations of the Magellan seamount increased after 37 Ma due to the expansion of the Tasman Gateway and opening of the Drake Passage, which allowed the inflow of Antarctic bottom water containing high concentrations of DSi (Fontorbe et al., 2017). In addition, the deep water in the pacific ocean flows along the marine conveyor belt and accumulates DSi formed from the dissolution of biogenic silica, which results in higher DSi concentrations compared to that in the north Atlantic and Indian Oceans (Yu et al., 2022).
Based on these results, it is evident that the summit of OSM17 is a region of high DSi suitable for the survival and growth of Farrea occa. Farrea occa species were identified at a depth of 1935 m in Weijia Guyot in the Magellan seamount cluster (Chen et al., 2021), and the FMP was similar to that of previous studies on the oscular diameter, thickness, and length of the main tube (Reiswig, 2002; Ehrlich et al., 2007; Ehrlich, 2019). The results of this study are consistent with those of previous studies on the species identification of porifera.
After 1.2 Ma, deep water in the Pacific Ocean showed rapid flow during the glacial period, and approximately 55,000 years later, Fe-Mn oxide began to precipitate, showing rapid currents during the last glacial period (Hillaire-Marcel and De Vernal, 2007). At the tabletop of the Magellan seamount, the current flows faster than at the bottom (Yang et al., 2020). Biofouling, due to rock outcrops, appears where rapid ocean currents are present, which is the preferred environment for porifera, a filter feeder (Yang et al., 2020). As benthic organisms causing bioturbation prefer an environment with slow currents (Yang et al., 2020), we believe that it is thought that the tabletop of OSM17 has less bioturbation than the seamount foot. Therefore, it can be assumed that Farrea occa is porifera with precipitated ferromanganese oxide that forms at the summit of OSM17. The study area has a high DSi concentration, fast current, and relatively weaker bioturbation than the seamount foot, which is suitable for the survival of Farrea occa.
4.2 Reconstruction of the paleo-environment using ferromanganese oxide
Age dating is important in paleo-environment research and is commonly used in dating ferromanganese deposits (Glasby, 2006; Hein and Koschinsky, 2014; Benites et al., 2020). It was difficult to apply the Co-chronometer age dating method to the sample used in this study. The Co-chronometer method calculates the growth rate by assuming that the Co content supplied to the ferromanganese oxide (formed by hydrogenesis) is constant (Puteanus and Halbach, 1988) through the wt% of Co, Mn, and Fe (Manheim and Lane-Bostwick, 1988; Puteanus and Halbach, 1988). However, it is difficult to apply the Co-chronometer method to the Fe-Mn oxide sample because the wt% of Co, Mn, and Fe cannot be calculated for the thin Fe-Mn oxide layer with a maximum thickness of 110 μm in the FMP; therefore, a growth rate of 2 mm/Myr, measured at the outermost part of the ferromanganese crust of hydrogenetic genesis found in OSM7 of the Magellan seamount cluster, was applied to this sample (Kim et al., 2006).
The impact of microorganisms on the globular shape and sheath-like structure was identified through SEM analysis. The large surface area and substrate specificity accelerated the precipitation of Fe-Mn oxide (Tebo et al., 2004), and thus it is possible that it has been formed more recently than the estimated value. The effect of biomineralization, confirmed through SEM-EDS analysis (Figure 6B), indicates that the surface productivity was high when Fe-Mn oxide was precipitated on the tabletop of OSM17 and the supply of organic matter for microbial growth was unobstructed. Consequentially, it can be confirmed through Fe-Mn oxide analysis that the tabletop of OSM17 has been in an oxidative state and had high surface productivity for the last tens of thousands of years, which was difficult to identify in the existing ferromanganese deposits.
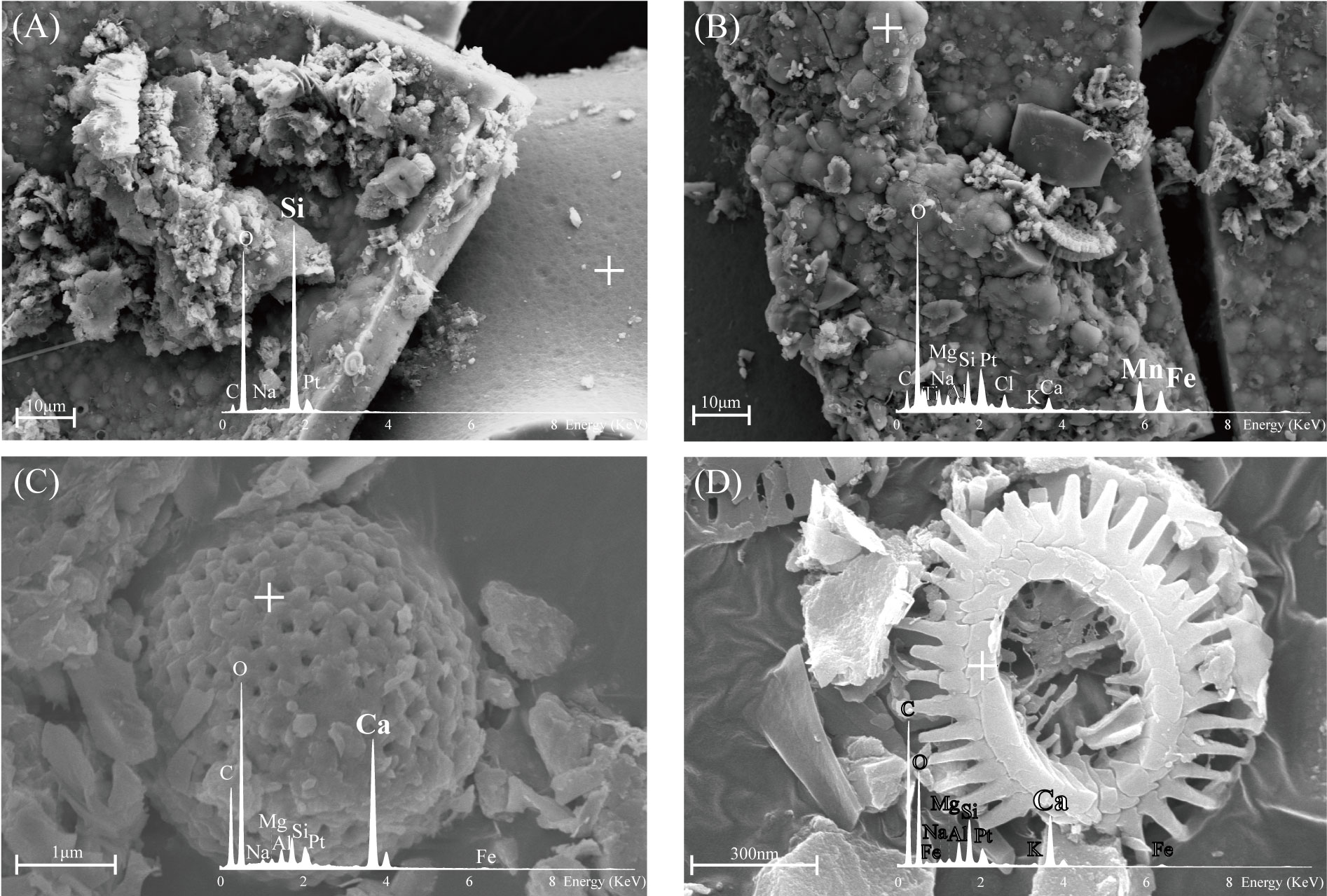
Figure 6 Representative SEM secondary electron images and EDS profiles of FMP: (A) Smooth surface with high concentration of Si; (B) Globular-like morphology and presence of Mn and Fe; and (C, D) Morphology and EDS profiles indicate foraminifera and coccolith, respectively (the crosses indicate the EDS point).
4.3 FMP formation mechanism and future research direction
Although porifera and Fe-Mn oxides constituting FMP show a global distribution (Taylor et al., 2007; Mizell and Hein, 2018), there have been no studies on samples with atypical forms in which Fe-Mn oxides are precipitated in the skeleton of porifera. In addition, porifera fossils usually exist in buried forms in sediments and are found very rarely with their skeletons preserved (Łukowiak, 2020); this is because porifera form siliceous deposits by binding or burying posthumously (Łukowiak, 2020), particularly Farrea occa, which have brittle skeletons (Krautter et al., 2001).
The FMP, in which the skeletal structure was preserved, was formed due to three main factors: (1) The low sedimentation rate in the study area: The Magellan seamount cluster exhibits a low sedimentation rate of 0.4–4 mm/Ky (Yang et al., 2020). High sedimentation rates hinder the precipitation of Fe-Mn oxide (Marino et al., 2017); therefore, the tabletop of OSM17 is a suitable environment for the precipitation of Fe-Mn oxide. (2) The substrate of ferromanganese oxide with a large surface area: Unlike ordinary ferromanganese deposits, the stratified lattice-like structure has a larger surface area than nuclei or substrate; therefore, precipitation can occur faster even if precipitation occurs at the same rate in the early stages of Fe-Mn oxide precipitation. This large surface area of the substrate can also be found in Si element distribution data from μ-XRF and Micro-CT images. In addition, the fact that porifera, which are organisms that do not have rolling properties, such as ferromanganese nodules, are not buried in sediments also serves as a substrate for Fe-Mn oxide precipitation. (3) Rapid precipitation due to the effects of microorganisms: Biomineralization effects precipitated dissolved manganese faster than abiotic processes (Tebo et al., 2004), and SEM-EDS analysis shows that there was an effect of microorganisms. Therefore, due to the three abovementioned factors, the skeletons could have been preserved by FMP, which protected them from collapsing or becoming siliceous sediment due to the rapid precipitation of Fe-Mn oxide on the porifera skeleton following death.
5 Conclusion
The FMP sampled in this study comprised Fe-Mn oxide precipitated on the skeletons of Farrea occa belonging to the Hexactinellida class, a species of porifera. Morphological, geochemical, and mineralogical results of FMP show that the tabletop of OSM17, a Magellan seamount cluster in the western Pacific, has been subjected to an oxidative environment with high DSi concentration, fast current flow, slow sedimentation rate, and relatively weak bioturbation, which has been suitable for the survival of porifera and precipitation of Fe-Mn oxide.
In addition, the FMP, which is up to 55,000 years old, is complementary to existing studies in that it can reconstruct the environment tens of thousands of years ago, which is difficult to identify in the ferromanganese deposits. Since porifera fossils that exist with skeletons in the marine environment are rare, it is believed that FMP will contribute to the investigation of past porifera species and DSi fluctuations in the marine environment, which have been limited due to relatively insufficient spicule fossils in the sediment core. Because only one sample was found in this study, reconstructing the DSi fluctuations by geological period at the tabletop of OSM17 is not possible. However, DSi reconstruction could be possible through future research. Moreover, FMP can be considered a new indicator to research the paleo-environment of the marine environment.
Data availability statement
The raw data supporting the conclusions of this article will be made available by the authors, without undue reservation.
Author contributions
All authors listed have made a substantial, direct, and intellectual contribution to the work and approved it for publication.
Funding
This research was supported by the Korea Institute of Marine Science & Technology Promotion (KIMST), funded by the Ministry of Oceans and Fisheries (No. 20220509) and supported by the National Research Foundation of Korea (NRF) grant funded by the Korea government (MIST) (No. 202006710003) and supported by the Korea Institute of Ocean & Technology (KIOST) research program (Grant no. PE99986).
Conflict of interest
The authors declare that the research was conducted in the absence of any commercial or financial relationships that could be construed as a potential conflict of interest.
Publisher’s note
All claims expressed in this article are solely those of the authors and do not necessarily represent those of their affiliated organizations, or those of the publisher, the editors and the reviewers. Any product that may be evaluated in this article, or claim that may be made by its manufacturer, is not guaranteed or endorsed by the publisher.
References
Alvarez B., Frings P. J., Clymans W., Fontorbe G., Conley D. J. (2017). Assessing the potential of sponges (Porifera) as indicators of ocean dissolved Si concentrations. Front. Mar. Sci. 4. doi: 10.3389/fmars.2017.00373
Arasuna A., Kigawa M., Fujii S., Endo T., Takahashi K., Okuno M. (2018). Structural characterization of the body frame and spicules of a glass sponge. Minerals 8. doi: 10.3390/min8030088
Benites M., Hein J. R., Mizell K., Blackburn T., Jovane L. (2020). Genesis and evolution of ferromanganese crusts from the summit of Rio grande rise, southwest Atlantic ocean. Minerals 10. doi: 10.3390/min10040349
Benites M., Millo C., Hein J., Nath B. N., Murton B., Galante D., et al. (2018). Integrated geochemical and morphological data provide insights into the genesis of ferromanganese nodules. Minerals 8, 488. doi: 10.3390/min8110488
Benton M. J., Harper D. A. (2009). Introduction to paleobiology and the fossil record. 1st ed. (Willey: Blackwell Publishing).
Chen W., Na J., Shen C., Zhang R., Lu B., Cheng H., et al. (2021). Ophiuroid fauna of cobalt-rich crust seamounts in the northwest pacific ocean. Acta Oceanol. Sin. 40, 55–78. doi: 10.1007/s13131-021-1887-y
Clouard V., Bonneville A. (2015). Ages of seamounts, islands, and plateaus on the pacific plate. Geological Soc. America. 388. doi: 10.1130/SPE388
Coale K. H., Bruland K. W. (1988). Copper complexation in the northeast pacific. Limnol. Oceanogr. 33, 1084–1101. doi: 10.4319/lo.1988.33.5.1084
Deng X., He G., Xu Y., Liu Y., Wang F., Zhang X. (2022). Oxic bottom water dominates polymetallic nodule formation around the caiwei guyot, northwestern pacific ocean. Ore Geol. Rev. 143. doi: 10.1016/j.oregeorev.2022.104776
Drozdov A. L., Zemnukhova L. A., Panasenko A. E., Polyakova N. V., Slobodyuk A. B., Ustinov A. Y., et al. (2021). Silicon compounds in sponges. Appl. Sci. 11. doi: 10.3390/app11146587
Ehrlich H. (2019). Marine biological materials of invertebrate origin (Cham: Springer International Publishing).
Ehrlich H., Krautter M., Hanke T., Simon P., Knieb C., Heinemann S., et al. (2007). First evidence of the presence of chitin in skeletons of marine sponges. part II. glass sponges (Hexactinellida: Porifera). J. Exp. Zool. B Mol. Dev. Evol. 308, 473–483. doi: 10.1002/jez.b.21174
Fontorbe G., Frings P. J., de la Rocha C. L., Hendry K. R., Carstensen J., Conley D. J. (2017). Enrichment of dissolved silica in the deep equatorial pacific during the Eocene-oligocene. Paleoceanography 32, 848–863. doi: 10.1002/2017PA003090
Glasby G. P. (2006). “Manganese: Predominant role of nodules and crusts,” in Marine geochemistry (Cham: Springer International Publishing).
Glasby G. P., Ren X., Shi X., Pulyaeva I. A. (2007). Co–Rich Mn crusts from the Magellan seamount cluster: The long journey through time. Geo-Mar. Lett. 27, 315–323. doi: 10.1007/s00367-007-0055-5
Halbach P., Hebisch U., Scherhag C. (1981). Geochemical variations of ferromanganese nodules and crusts from different provinces of the pacific ocean and their genetic control. Chem. Geol. 34, 3–17. doi: 10.1016/0009-2541(81)90067-X
Hein J. R., Koschinsky A. (2014). Deep-ocean ferromanganese crusts and nodules. Treatise Geochem 13, 273–291. doi: 10.1016/B978-0-08-095975-7.01111-6
Hein J. R., Koschinsky A., Bau M., Manheim F., Kang J. K., Roberts L. (2000). Cobalt-rich ferromanganese crusts in the pacific.
Hein J. R., Mizell K., Koschinsky A., Conrad T. A. (2013). Deep-ocean mineral deposits as a source of critical metals for high- and green-technology applications: Comparison with land-based resources. Ore Geol. Rev. 51, 1–14. doi: 10.1016/j.oregeorev.2012.12.001
Hein J. R., Morgan C. L. (1999). Influence of substrate rocks on fe-Mn crust composition. Deep Sea Res. Part I: Oceanogr. Res. Pap. 46, 855–875. doi: 10.1016/S0967-0637(98)00097-1
Hillaire-Marcel C., De Vernal A. (2007). Proxies in late Cenozoic paleoceanography (Amsterdam: Elsevier).
Iyer S. (1999). Ferromanganese oxides on sharks’ teeth from central Indian ocean basin. Indian J. Mar. Sci. 28. 263–269
Jiang X.-D., Sun X.-M., Guan Y. (2019). Biogenic mineralization in the ferromanganese nodules and crusts from the south China Sea. J. Asian Earth Sci. 171, 46–59. doi: 10.1016/j.jseaes.2017.07.050
Kim J., Hyeong K., Jung H.-S., Moon J.-W., Kim K.-H., Lee I. (2006). Southward shift of the intertropical convergence zone in the western pacific during the late tertiary: Evidence from ferromanganese crusts on seamounts west of the Marshall islands. Paleoceanography 21. doi: 10.1029/2006PA001291
Krautter M., Conway K. W., Barrie J. V., Neuweiler M. (2001). Discovery of a “Living dinosaur”: Globally unique modern hexactinellid sponge reefs off British Columbia, Canada. Facies 44, 265–282. doi: 10.1007/BF02668178
Langer G., Probert I., Nehrke G., Ziveri P. (2011). The morphological response of emiliania huxleyi to seawater carbonate chemistry changes: An interstrain comparison. J. Nannoplankton Res. 32, 29–34.
Leys S. P., Mackie G. O., Reiswig H. M. (2007). The biology of glass sponges. Adv. Mar. Biol. 52, 1–145. doi: 10.1016/S0065-2881(06)52001-2
Łukowiak M. (2020). Utilizing sponge spicules in taxonomic, ecological and environmental reconstructions: A review. PeerJ 8, e10601. doi: 10.7717/peerj.10601
Machida S., Nakamura K., Kogiso T., Shimomura R., Horinouchi K., Okino K., et al. (2021). Fine-scale chemostratigraphy of cross-sectioned hydrogenous ferromanganese nodules from the western north pacific. Isl. Arc. 30. doi: 10.1111/iar.12395
Manheim F. T., Lane-Bostwick C. M. (1988). Cobalt in ferromanganese crusts as a monitor of hydrothermal discharge on the pacific sea floor. Nature 335, 59–62. doi: 10.1038/335059a0
Marino E., Gonzãlez F. J., Somoza L., Lunar R., Ortega L., Vãzquez J. T., et al. (2017). Strategic and rare elements in Cretaceous-Cenozoic cobalt-rich ferromanganese crusts from seamounts in the canary island seamount province (northeastern tropical Atlantic). Ore Geol. Rev. 87, 41–61. doi: 10.1016/j.oregeorev.2016.10.005
Menendez A., James R. H., Lichtschlag A., Connelly D., Peel K. (2019). Controls on the chemical composition of ferromanganese nodules in the clarion-clipperton fracture zone, eastern equatorial pacific. Mar. Geol. 409, 1–14. doi: 10.1016/j.margeo.2018.12.004
Mizell K., Hein J. R. (2018). Ferromanganese crusts and nodules: Rocks that grow, in encyclopedia of geochemistry: A comprehensive reference source on the chemistry of the earth. Ed. White W. M. (Cham: Springer International Publishing).
Müller W. E. G., Wang X., Belikov S. I., Tremel W., Schloámacher U., Natoli A., et al. (2007). “Formation of siliceous spicules in demosponges: Example suberites domuncula,” in Handbook of biomineralization (New York: Wiley Online Library).
Ortiz Kfouri L., Millo C., Estela De Lima A., Silveira C. S., Sant’anna L. G., Marino E., et al. (2021). Growth of ferromanganese crusts on bioturbated soft substrate, tropic seamount, northeast Atlantic ocean. Deep Sea Res. Part I: Oceanogr. Res. Pap. 175, 103586. doi: 10.1016/j.dsr.2021.103586
Park H., Kim J. (2020). Application of scanning electron microscopy (SEM) for biotically induced microstructure observation in sedimentary sample of natural condition. Korean J. Mineral. Petrol. 33, 165–173. doi: 10.22807/KJMP.2020.33.3.165
Puteanus D., Halbach P. (1988). Correlation of Co concentration and growth rate — a method for age determination of ferromanganese crusts. Chem. Geol. 69, 73–85. doi: 10.1016/0009-2541(88)90159-3
Reiswig H. M. (2002). Family Coreidae Gray 1872, in Systema Porifera: A guide to the classification of sponges. Eds. Hooper J. N. A., Soest R.W.M.V., Willenz P. (Massachusetts: Springer International Publishing).
Smith W. H. F., Staudigel H., Watts A. B., Pringle M. S. (1989). The Magellan seamounts: Early Cretaceous record of the south pacific isotopic and thermal anomaly. J. Geophys. Res. 94, 10501–10523. doi: 10.1029/JB094iB08p10501
Taylor M. W., Radax R., Steger D., Wagner M. (2007). Sponge-associated microorganisms: Evolution, ecology, and biotechnological potential. Microbiol. Mol. Biol. Rev. 71, 295–347. doi: 10.1128/MMBR.00040-06
Tebo B. M., Bargar J. R., Clement B. G., Dick G. J., Murray K. J., Parker D., et al. (2004). BIOGENIC manganese oxides: Properties and mechanisms of formation. Annu. Rev. Earth Planet. Sci. 32, 287–328. doi: 10.1146/annurev.earth.32.101802.120213
Thomas T., Moitinho-Silva L., Lurgi M., Björk J. R., Easson C., Astudillo-García C., et al. (2016). Diversity, structure and convergent evolution of the global sponge microbiome. Nat. Commun. 7, 11870. doi: 10.1038/ncomms11870
Uriz M. J., Turon X., Becerro M. A., Agell G. (2003). Siliceous spicules and skeleton frameworks in sponges: Origin, diversity, ultrastructural patterns, and biological functions. Microsc. Res. Tech. 62, 279–299. doi: 10.1002/jemt.10395
Van Soest R. W., Boury-Esnault N., Vacelet J., Dohrmann M., Erpenbeck D., De Voogd N. J., et al. (2012). Global diversity of sponges (Porifera). PloS One 7, e35105. doi: 10.1371/journal.pone.0035105
Wang X.-H., Gan L., Müller W. E. G. (2009). Contribution of biomineralization during growth of polymetallic nodules and ferromanganese crusts from the pacific ocean. Front. Mater. Sci. China. 3, 109–123. doi: 10.1007/s11706-009-0033-0
Wang X., Gan L., Wiens M., Schlossmacher U., Schröder H. C., Müller W. E. (2012). Distribution of microfossils within polymetallic nodules: Biogenic clusters within manganese layers. Mar. Biotechnol. (NY). 14, 96–105. doi: 10.1007/s10126-011-9393-4
Wang X., Müller W. E. G. (2009). Marine biominerals: perspectives and challenges for polymetallic nodules and crusts. Trends Biotechnol. 27, 375–383. doi: 10.1016/j.tibtech.2009.03.004
Weiner A. K. M., Morard R., Weinkauf M. F. G., Darling K. F., Andrë A., Quillëvërë F., et al. (2016). Methodology for single-cell genetic analysis of planktonic foraminifera for studies of protist diversity and evolution. Front. Mar. Sci. 3. doi: 10.3389/fmars.2016.00255
Yang K., Jung J. (2022). Mineralogical-geochemical characteristics of manganese nodules in the deep subseafloor sediments at site U1371 in the western south pacific gyre area. Ocean Polar Res. 44, 139–145. doi: 10.4217/OPR.2022012
Yang K., Park H., Son S.-K., Baik H., Park K., Kim J., et al. (2019). Electron microscopy study on the formation of ferromanganese crusts, western pacific Magellan seamounts. Mar. Geol. 410, 32–41. doi: 10.1016/j.margeo.2019.01.001
Yang Z., Qian Q., Chen M., Zhang R., Yang W., Zheng M., et al. (2020). Enhanced but highly variable bioturbation around seamounts in the northwest pacific. Deep Sea Res. I., 156. doi: 10.1016/j.dsr.2019.103190
Yu X., Zhuang Y., Cai X., Qi D. (2022). Role of marginal seas in deep ocean regeneration of dissolved silica: A case study in the marginal seas of the western pacific. Front. Mar. Sci. 9. doi: 10.3389/fmars.2022.925919
Keywords: porifera, Magellan seamount tabletop, ferromanganese oxide, micro-computed tomography, biomineralization
Citation: Park K, Jung J, Park J, Ko Y, Lee Y and Yang K (2023) Geochemical-mineralogical analysis of ferromanganese oxide precipitated on porifera in the Magellan seamount, western Pacific. Front. Mar. Sci. 9:1086610. doi: 10.3389/fmars.2022.1086610
Received: 01 November 2022; Accepted: 30 November 2022;
Published: 06 January 2023.
Edited by:
Cinzia Corinaldesi, Marche Polytechnic University, ItalyReviewed by:
Luigi Jovane, University of São Paulo, BrazilGianni Barucca, Marche Polytechnic University, Italy
Copyright © 2023 Park, Jung, Park, Ko, Lee and Yang. This is an open-access article distributed under the terms of the Creative Commons Attribution License (CC BY). The use, distribution or reproduction in other forums is permitted, provided the original author(s) and the copyright owner(s) are credited and that the original publication in this journal is cited, in accordance with accepted academic practice. No use, distribution or reproduction is permitted which does not comply with these terms.
*Correspondence: Kiho Yang, a3lhbmdAcHVzYW4uYWMua3I=