- 1Key Laboratory of Integrated Regulation and Resource Development on Shallow Lakes, Ministry of Education, Hohai University, Nanjing, Jiangsu, China
- 2College of Environment, Hohai University, Nanjing, Jiangsu, China
In recent decades, damming has become one of the most important anthropogenic activities for river regulation, and reservoirs have become hotspots for biogeochemical cycling. The construction of dams changes riverine hydrological conditions and alters the physical, chemical, and biological characteristics of rivers, eventually leading to significant variations in nutrient cycling. This review mainly explores the effects of river damming on nutrient transport and transformation, including i) nutrient (N, P, Si, and C) retention in reservoirs, ii) greenhouse gas (GHG) emissions, and iii) interactions between the nutrient stoichiometry ratio and the health of the reservoir ecosystem. The important drivers of nutrient transport and transformation, such as river connectivity, hydraulic residence time, hydropower development mode, microbial community variation, and anthropogenic pollution, have also been discussed. In addition, strategies to recover from the negative effects of damming on aquatic ecosystems are summarized and analyzed. To provide theoretical and scientific support for the ecological and environmental preservation of river-reservoir systems, future studies should focus on nutrient accumulation and GHG emissions in cascade reservoirs.
Introduction
With the development of human society, dam construction has developed rapidly owing to its applications in power generation, water resource utilization, navigation, and flood control. At the end of the 19th century, Europe and the United States started the first boom in hydropower development as a clean, efficient, and stable source of energy. The 21st century witnessed a second boom in dam construction, mainly occurring in developing countries, among which Asia was a hot spot with an installed capacity of more than 15 GW (Glibert, 2020). Although the center of dam construction has shifted from developed to developing countries since 2016, hydropower has always been the main source of renewable energy, accounting for 71% of the renewable energy supply (Moran et al., 2018). Currently, there are more than 3,700 hydropower stations with a capacity of at least 1 MW planned or under construction worldwide (Grill et al., 2019). Although dam construction has many functions, it will change the original state of the watershed. (Song et al., 2018). According to previous studies, the connectivity of more than half of the large rivers in the world has been influenced by damming (Van Cappellen and Maavara, 2016), and the number will increase to 93% by 2030 (Glibert, 2020).
It has been reported that dam construction flattens flood variation, alters downstream flow, and affects total runoff, leading to changes in the hydrological conditions of river estuaries and nearby marine areas (Baumgartner et al., 2022). Effects of dams on ecosystems occur through reduced river flow, reduced sediment flux, altered water temperature, changed estuary delta, and blocked migration routes in river sections and adjacent seas (Zhang X. et al., 2022). Moreover, damming alters the matter and energy fluxes in river and marine ecosystems, which has a direct impact on nutrient biogeochemistry (Liermann et al., 2012). Dams and reservoirs are hotspots for biogeochemical activity in river networks. The interference of human regulation on the circulation of river biomass affects the migration and transformation characteristics of river biomass and the eutrophication response of water. In addition to nutrient flow, the nutrient ratios are also affected. Changes in nutrient content and proportion impact river ecosystems, including the microbial communities in them. Therefore, the main contents of this review are as follows: 1) variation in the migration and transformation processes of nutrients, 2) important driving factors for the transport of river nutrients, and 3) potential strategies to mitigate the environmental consequences of river damming.
Transport and transformation of nutrients affected by river damming
River damming is one of the primary anthropogenic disruptions to the Earth’s natural water and matter cycles (Gilbert et al., 2020). Physical factors such as water level, flux, and velocity show notable changes after reservoir construction. For example, water velocity decreases as it approaches the dam, transforming the system from a natural “river” to an artificial “lake” (Winton et al., 2019). These modifications in physical parameters further impact biogeochemical cycles, affecting water quality and jeopardizing ecosystems in the downstream reaches and adjacent wetlands.
Nitrogen (N), phosphorus (P), silicon (Si), and carbon (C) cycling, the four most essential nutrient cycles in river ecosystems, are crucial for preserving aquatic biological balance (Atkins, 2020). Dam construction artificially complicates changes in the trophic state of rivers (Winemiller et al., 2016). To fully understand the changes of nutrient load fluxes and chemical compositions, it is necessary to review the nutrient cycling variation and relevant mechanism after damming.
Variations in hydraulic conditions
Dams intercept large amounts of sediment in reservoirs, and there is a general decrease in particle size along the flow direction within the reservoir as suspended particles settle down owing to the reduction in river velocity (Yi et al., 2017; Tang et al., 2018). Coarse particles deposit easily, whereas fine particles can travel long distances downstream. Since fine particles typically have a higher capacity for adsorption than coarse particles (Pan et al., 2013), the total nitrogen and organic carbon in the sediment would increase from upstream to downstream of the reservoir. Meanwhile, slower flow rates caused by damming can limit sediment sliding and suspension violently, reducing the release of nutrients from sediments in reservoirs (Van Cappellen and Maavara, 2016).
Reservoir construction can also result in vertical thermal stratification because the density gradient of reservoir water formed by solar heating on the surface effectively prevents the water temperature from mixing (Noori et al., 2021). Thermal stratification indirectly affects the dissolved oxygen (DO) content (Winton et al., 2019). In short, hydrodynamic weakening of the reservoir area causes water temperature and DO stratification, affecting biological reactions.
Sediment retention and thermal stratification in reservoirs can further affect the cyclic nutrient transformation and change the trophic status of the river system, which presents both benefits and drawbacks for the trophic condition of river systems. Nutrient retention after dam construction can benefit the receiving water by reducing the risk of eutrophication in downstream areas; however, it increases the risk of eutrophication in the reservoir (Maavara et al., 2020a). To manage river nutrients, it is crucial to understand how damming affects the four fundamental nutrients—N, P, Si, and C.
Variation in nitrogen cycling in reservoirs
Nitrogen is an important nutrient in river systems. Nitrogen transformation and transport processes include ammonification, nitrification, nitrogen fixation, denitrification, decomposition, and organic nitrogen synthesis (Kreiling et al., 2021; Zhou et al., 2022). The biogeochemical cycle of nitrogen is influenced by dam construction, especially in terms of nitrogen flux and concentration (Nair and Yu, 2020).
Atmospheric deposition, upstream inflow, and biological nitrogen fixation are the major sources of nitrogen input in reservoirs (Jankowski et al., 2012; Horvath et al., 2013). The percentage of atmospheric deposition that contributes to the nitrogen load in reservoirs changes with the climatic rainfall and is higher during rainy seasons (Liu et al., 2019). Agricultural discharge is the dominant source of upstream inflow. Nitrogen fixation (conversion of N2 to NH4+)—the only biological way of nitrogen generation— contributes to 39–47% of the nitrogen in the water column inside the reservoir (Maavara et al., 2020a). Runoff and gaseous emissions constitute the majority of the nitrogen transported out of reservoirs. Ammonia volatilization and denitrification are two of these gaseous emissions (Wei et al., 2022). The nitrogen flux from the water was found to be greatly reduced after passing through a reservoir (Wollheim et al., 2008). According to Harrison et al., in the mid-1990s, global reservoirs eliminated 464 Gmol N annually (Harrison et al., 2009; Harrison et al., 2010; Van Cappellen and Maavara, 2016). Denitrification, the major nitrogen removal mechanism in reservoirs, contributes to approximately 58–59% of the total removal (Zhu et al., 2022).
Reservoir development alters nitrogen fluxes and changes the reactive rate of nitrogen. For instance, the dam construction in recent years has significantly enhanced the nitrogen fixation in reservoirs, increasing the primary production and rate of nitrification and nitrogen assimilation in reservoirs (Cook et al., 2010; Grantz et al., 2014). The three nitrogen biogeochemical processes of the reservoir (Figure 1)—ammonification, nitrification, and denitrification have a significant impact on river ecosystems. Damming increases the nitrogen concentration in reservoirs through nitrogen retention and fixation, ultimately resulting in eutrophication (Moutinho et al., 2021). However, changes in the N:P ratio can reduce the N limitation in receiving water. According to Akbarzadeh (Akbarzadeh et al., 2019), dams increase the ratio of N:P in rivers globally due to nitrogen fixation in reservoirs, reducing N limitation in water (Ramirez-Zierold et al., 2010; Molisani et al., 2013). The decrease in the nitrogen concentration in water due to denitrification and burial in reservoirs makes nitrogen one of the main variables limiting the primary productivity in water (Hamilton et al., 2001; Galloway, 2005; Vanni et al., 2011; Bi et al., 2017). Therefore, understanding the beneficial and detrimental effects of the various biological actions of nitrogen is crucial for identifying and addressing the issues of nutrient balance in the water column.
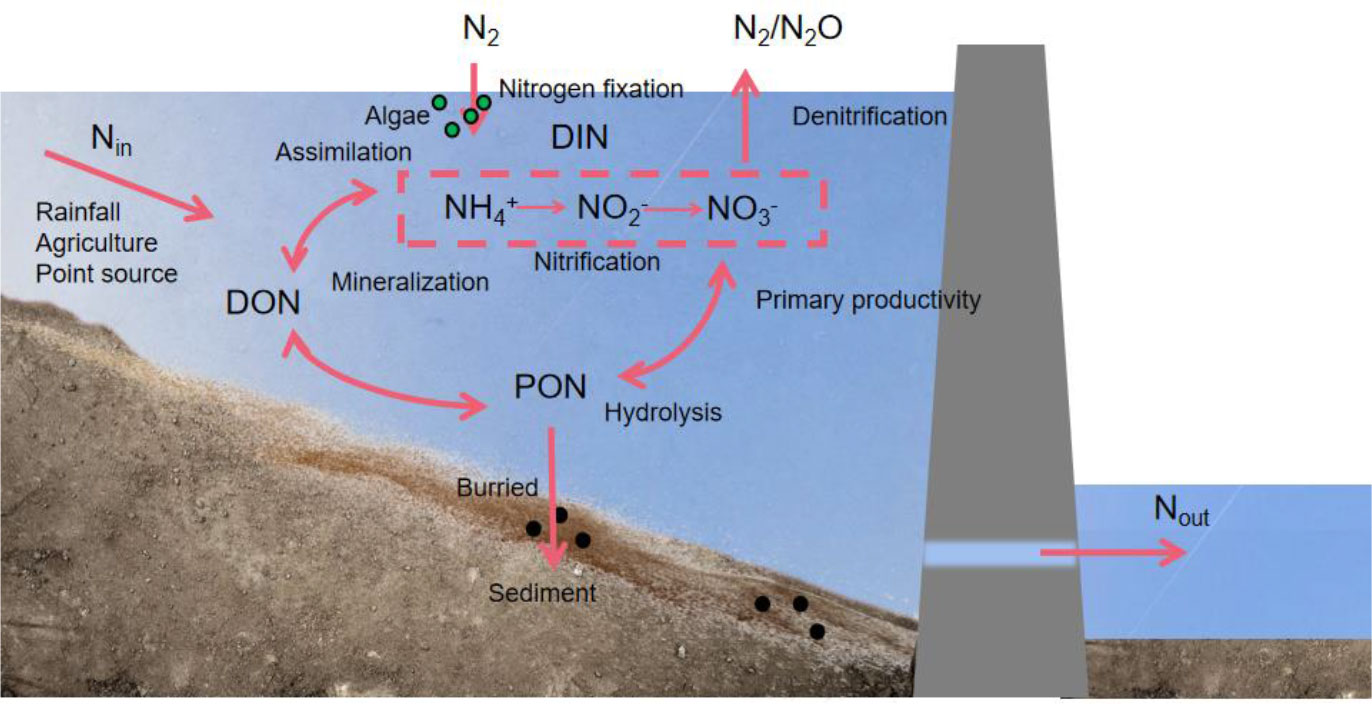
Figure 1 Biogeochemical transformation mechanism of nitrogen in a reservoir. DON:dissolved organic nitrogen; PON:particle organic nitrogen; DIN:dissolved inorganic nitrogen.
The third most long-lived GHG is N2O; therefore, climate change is largely impacted by massive N2O production. Reservoirs significantly increase GHG emissions because N2O can be produced during denitrification (Hansen et al., 2016). Globally, natural lakes and reservoirs produce 4.5 ± 2.9 Gmol N2O per year (Zhang L. et al., 2022), and reservoirs account for nearly 50% of all fluxes, although the area of reservoirs only makes up 9% of all standing water. Additionally, after reservoir construction, the riparian zone often declines as the water level rises, which may result in an expansion of the denitrification zone for the same magnitude of water level variation (Thouvenot-Korppoo et al., 2009; Turner et al., 2016). Moreover, damming causes fluctuations in reservoir water levels, which alternates between wet and dry cycles (Shi et al., 2020a) and introduces nitrate to the riparian surface. This cyclic dry-wet process can significantly accelerate the riparian denitrification process by controlling oxygen infiltration, nitrogen substrate, and carbon availability (Ranalli and Macalady, 2010; McPhillips and Walter, 2015; Wang et al., 2018).
Variation in phosphorus cycling in reservoirs
Sources of P are divided into natural and anthropogenic parts. Natural sources are mainly phosphate rocks, which enter the water cycle through erosion and weathering. Anthropogenic sources are further divided into point and non-point sources, such as domestic sewage discharge and agricultural fertilizers (Smith and Schindler, 2009; Huang et al., 2015). The transport and transformation of P (Figure 2) in reservoirs mainly include sediment adsorption, desorption, and resuspension processes, the transformation between different phases (water-particle-sediment), assimilation and absorption of organisms, and release from decomposition, all of which determine the form and distribution of P (Withers and Jarvie, 2008). Adsorbed P moves with suspended sediment particles, whereas dissolved P is transported by convective diffusion (Huang et al., 2015). Adsorbed P may accumulate on the riverbed surface due to sediment deposition and then be released during sediment re-suspension.
Reservoir construction changes hydrological and environmental conditions, making it difficult to understand how P would be transported, transformed, and retained in rivers. The transport of P involves various physical and chemical processes, such as convective diffusion, adsorption-desorption, and deposition-resuspension (Figure 2). However, damming slows down the flow rate of water, causing the P adsorbed by particles to settle and sediment easily, increasing P retention in reservoirs, and thereby reducing the downstream flux of P (Teodoru and Wehrli, 2005; Harrison et al., 2010). Vertical physicochemical and biological stratification phenomena further change P forms (Friedl and Wuest, 2002). For instance, thermal stratification leads to anaerobic conditions in the bottom layer of reservoir, which facilitate the release of sediment P. Since reservoirs are mostly drained from the bottom layer, it will result in the leakage of high concentrations of P in downstream of dam (Wang et al., 2009; Keitel et al., 2016).
Generally, P retention is significantly increased by reservoir construction. Because the reservoir has a large area of stable water, it reduces the flow of the river and traps a large amount of P. Based on previous studies, the reservoir dams in the upper Seine River watershed in France retained 60% of the phosphate adsorbed by sediment particles and benthic organisms (Yan et al., 2021). According to comparative studies of river networks in the midwest of the United States, the yearly flux of total P in downstream water decreased by approximately 20% after reservoir construction (Powers et al., 2015). With more dams and reservoirs being built, P retention in rivers continues to increase globally. Due to the surge in dam construction, the total P (TP) roughly doubled between 1970 and 2000, making up 12% of the global river TP load (Filippelli, 2008; Maavara et al., 2015).
Although reservoirs can serve as P sinks and reduce nutrient loading into the receiving waters downstream, P accumulates in the sediment as the reservoir’s operation time increases. In summer flood conditions, the bottom sediment is easily eroded, and P is released into the overlying water, causing serious environmental problems (Huang et al., 2015). In addition, reservoir retention can reduce the downstream pollution load; however, in rivers with lower nutrient levels, it may lead to nutrient depletion in the downstream areas. For example, Sweden’s most severely dammed and reservoir-regulated river, the Lulealven River, exhibits a progressive decline in P export, endangering primary productivity downstream (Humborg et al., 2002).
Variation in silicon cycling in reservoirs
Silicon is a crucial biogenic component of various freshwater aquatic species, particularly diatoms. The primary limiting element in many aquatic systems is Si despite its abundance (Koszelnik and Tomaszek, 2008; Tavernini et al., 2011) because the majority of Si is bound as quartz and silicate minerals, making it difficult for living organisms to utilize (Cornelis et al., 2011; Durr et al., 2011). The predominant form of biogenic dissolved Si in river systems is H4SiO4 (Maavara et al., 2018). It is critical to understand the riverine Si cycle because diatoms contribute to 25% of the primary productivity of water and 40% of the primary productivity of oceans (Triplett et al., 2008).
The main sources of Si in reservoirs are river inflows, rock weathering, and sediment erosion in bedrock aquifers. Besides, groundwater is also a major path for Si transport to surface waters (Maavara et al., 2018). Reactive Si enters the reservoir in two different states: dissolved and particulate. Dissolved reactive Si (DSi) entirely consists of Si hydroxide (H4SiO4) or monomeric silica. In contrast, particulate reactive Si (PSi) contains all Si associated with particles and may be dissolved before being removed by burial in bottom sediments or river outflow. Although solid phases, such as quartz and silicate minerals, are not bioavailable, once they enter the reservoir, a portion of the particulate Si may be dissolved to produce extra DSi. The pool of active particulate Si in reservoirs is further increased by the transformation of dissolved Si into biogenic Si by organisms, such as diatoms and riparian plants (mostly diatoms) (Mosseri et al., 2005; Sauer et al., 2006). Biogenic Si (BSi) is composed of silica with protective organic matter. When organic matter degrades, soluble BSi is converted to DSi, while insoluble BSi is exported downstream or buried in reservoir sediments (Loucaides et al., 2012). Details of the Si cycling process within the reservoir are shown in Figure 3. Compared to PSi, sediment silicon (SSi) has a substantially lower dissolution rate constant, and as a result, reactive PSi diminishes quickly, that is, aging occurs. The insoluble part of the SSi is permanently removed by burial in the reservoir sediments. Therefore, the total Si retention is the sum of the exogenous active Si and the interactions between the dissolution and retention of BSi and the PSi formed in reservoirs (Teodoru et al., 2006; Jansen et al., 2010).
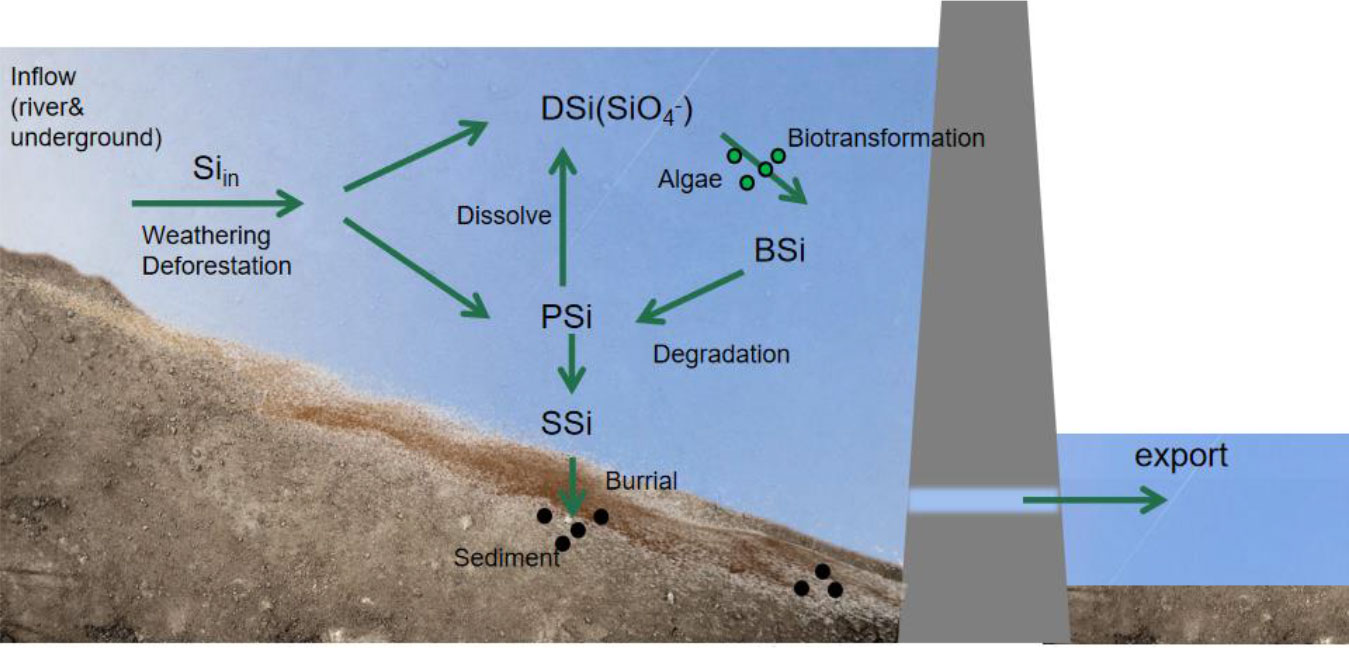
Figure 3 Circulation process of silicon in a reservoir. DSi, dissolved reactive silicon; SSi, sediment silicon; PSi, particulate reactive silicon; BSi, biogenic silicon.
Silicon is easily trapped in reservoirs (Syvitski et al., 2005). Silicon retention primarily occurs in the transition zone between the rivers and reservoirs (Mugidde et al., 2003). In Diefenbaker Lake in Saskatchewan, the retention of dissolved Si is approximately 28% of the inflow (Koszelnik and Tomaszek, 2008). Dams typically sequester 20% of the active Si that is added to reservoirs (Znachor et al., 2013). Globally, reservoirs store 5.3% of the active Si in the basin. Rivers, lakes, and coastal areas are among the many aquatic habitats whose ecology and health are significantly influenced by Si (Garnier et al., 2010; Tavernini et al., 2011). Compared with nitrogen and phosphorus, Si is more crucial for the growth of phytoplankton and the health of aquatic ecosystems. However, because of dam construction, Si is retained in the reservoir, reducing Si flux into the ocean and affecting coastal productivity owing to Si limitation (Maavara et al., 2020a). As a result, we should pay more attention to how humans have altered river systems, particularly how the construction of dams affects Si cycling and biological processes at all scales, from the river basin to the global level (Hartmann et al., 2011).
Variation in carbon cycling in reservoirs
The increasing atmospheric CO2 concentrations have led to increased global warming in the 21st century (Cole et al., 2007). Climate change affects the hydrological cycle and water quality, and global warming is playing an increasingly important role in aquatic systems. Reservoirs constantly produce methane and carbon dioxide, which may exacerbate global warming (Glibert, 2012). Whether reservoirs serve as sources or sinks of GHGs has been hotly debated. To control global C balance, it is crucial to understand the profound modifications caused by damming (Mendonca et al., 2012; Grill et al., 2015).
Reservoir construction initially causes an increase in GHG emissions and later causes eutrophication in downstream areas. After damming, carbon retention in the reservoir begins, leading to an increase in the primary productivity of the reservoir (Figure 4). As primary productivity increases, the production of CH4 by microbes also increase. Therefore, eutrophic reservoirs often have higher CH4 emissions than oligotrophic reservoirs (Deemer et al., 2016). Tropical reservoirs typically emit more gaseous C than temperate reservoirs because of their larger surface area, higher soil organic C content, and warmer water temperatures (Fearnside, 2002; Abril et al., 2005; Kemenes et al., 2011; Colas et al., 2020). Over time, long-term sediment buildup in aging reservoirs may increase nutrients downstream, which could result in large-scale hazardous algal blooms (HABs) (Justic et al., 2002; Paerl et al., 2006; Fox and Ford, 2016; Fernandez-Alias et al., 2022; Liu et al., 2022).
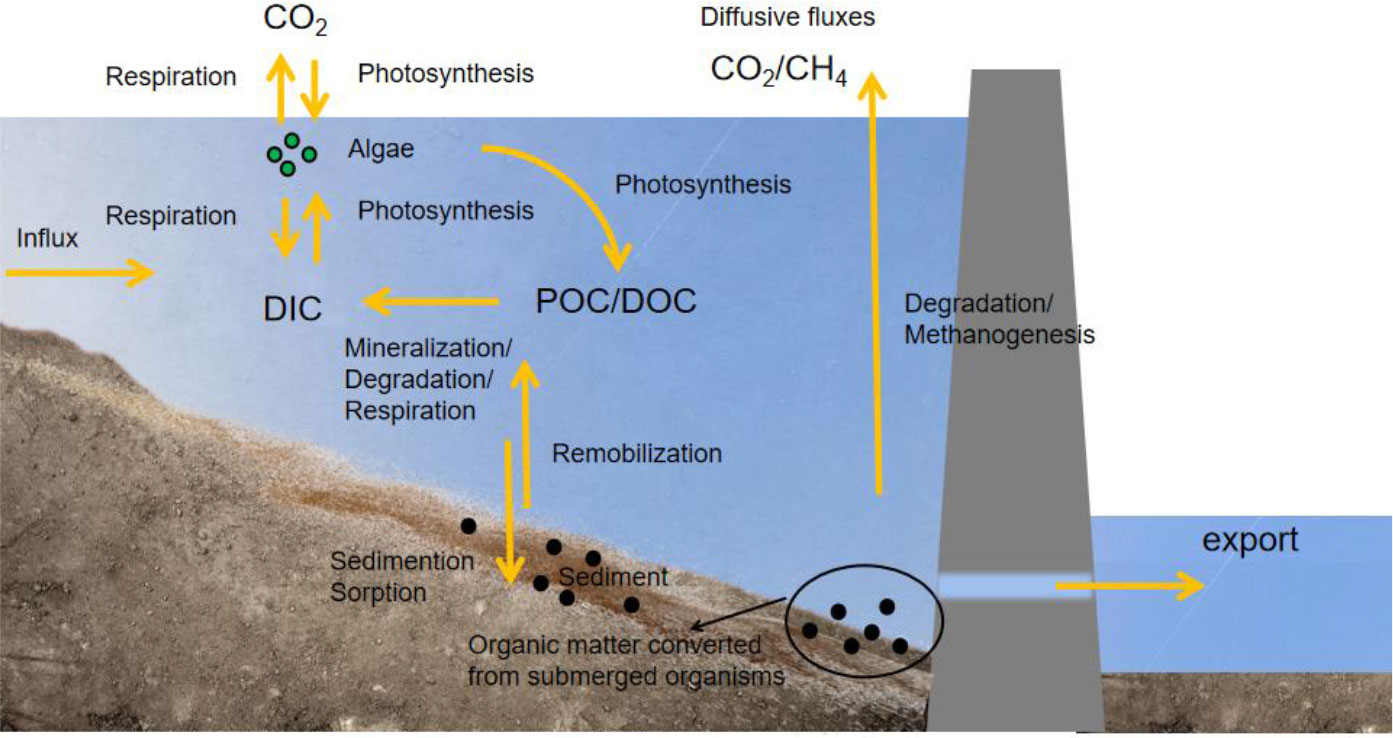
Figure 4 Circulation process of carbon in a reservoir. DOC, dissolved organic carbon; POC, particle organic carbon; DIC, dissolved inorganic carbon.
Compared with natural rivers, C in reservoirs shows a greater spatial and temporal variation (Han et al., 2018) due to thermal stratification, increased primary productivity, and the discharge of reservoir bottom water. Thermal stratification in the reservoir water causes differences in factors such as vertical illumination, DO, and nutrients (Winton et al., 2019). The differences in the environment provide the conditions for chemical and biological stratification. Increased photosynthesis results in higher particle organic carbon (POC) concentrations and lower CO2 (aq) concentrations in the reservoir surface water, whereas the increase in biological activity and organic C mineralization results in higher CO2 (aq) concentrations in the reservoir bottom water. As a result, the CO2 concentrations in the water released from the bottom of the reservoir to downstream areas are higher (Sugiyama et al., 2004; Kim et al., 2006; Stimson et al., 2017). In addition, seasonal changes in water levels may cause higher GHG emissions through repeated wetting and drying cycles. After dam removal, sediments with abundant organic matters and frequent water-level changes may also be hotspots for GHG emissions. According to previous studies, CO2 emissions after dam removal will be nine times higher than the reservoir’s initial emissions after the dam was built for 100 years (Pacca, 2007). Therefore, multi-objective optimization is advised to increase power generation while guaranteeing minimal GHG emissions for diverse basin features.
Nutrient ratio
Although reservoirs have a retention effect on all four major nutrients, different retention efficiencies can change the stoichiometric ratio of nutrients, leading to different nutrient limitations and ultimately affecting the trophic status of the reservoirs. According to Maavara et al., in 2000, dams retained an average of 21% of biogenic silica, 43% of reactive phosphorus, and 35.5% of total nitrogen (Maavara et al., 2014; Maavara et al., 2020a). Reservoir construction modifies the quantity of biogenic material entering downstream and changes the nutrient ratios because reservoirs preferentially eliminate phosphorus and nitrogen and are more effective at retaining phosphorus than nitrogen (Calic et al., 2013). In freshwater, phosphorus is frequently considered as the limiting element, whereas nitrogen is considered as the limiting element in coastal and marine environments (Elser et al., 2022). Nowadays, fertilizer and wastewater discharge lead to N and P enrichment, and the removal of Si-rich plants during deforestation and agricultural production, combined with the effects of dam construction, is likely to result in significantly higher N: Si and P: Si ratios than before, contributing to Si limitation downstream, which may cause downstream eutrophication and promote the growth of harmful algae (Struyf et al., 2010; Clymans et al., 2011).
The trophic dynamics and biogeochemical processes are fundamentally coupled. The imbalances in stoichiometric ratios of various elements (C, N, P, Si, etc.) caused by damming lead to short- and long-term consequences on ecosystems, from altering primary productivity to breaking water homeostasis (Vitousek et al., 2010). For instance, the structure of the phytoplankton community changes, and harmful algae may become the dominant species if the ratio of DSi and dissolved inorganic nitrogen (DSi : DIN)< 1 in reservoir water (Lane et al., 2004; Zervoudaki et al., 2009), causing risks to the aquatic environment. Consequently, ecosystem modifications can affect the recycling of nutrients in reservoirs. The nutrient cycle can also be altered by changing the physical characteristics of the habitat, such as turbidity, flux, light, oxygen, pH, and substrate availability (Glibert et al., 2011; Gao et al., 2012). For instance, in eutrophic reservoirs, an increase in algal productivity causes a decrease in DO, which may affect N and P cycling (Glibert, 2012; Wurtsbaugh, 2020).
A management approach that considers various nutrients must be devised to control nutrients effectively. After 37 years of research in an experimental lake, it has been determined that a single nutrient removal approach can cause the ecosystem to become stoichiometrically unbalanced (Schindler et al., 2008). Control of either N or P is ineffective in controlling algal blooms; a joint control of these nutrients is essential for effectively managing eutrophication. Leiblich’s minimum law and Redfield stoichiometry are the salt restriction parameters for the eutrophication of water, which must be considered for any specific management (Klausmeier et al., 2008; Allen and Gillooly, 2009; Mebane et al., 2021).
Influence factors of damming on nutrient cycles
Influence of river location and connectivity
Location is the most crucial physical parameter for evaluating water flow, connectivity, and shape (Schmadel et al., 2018). The hydrological characteristics of a river, such as flux and velocity, are largely determined by the location of the river network. It influences environmental factors, such as water temperature, DO, and hydraulic residence time, and ultimately affects nutrient cycling (Lautz and Fanelli, 2008). A key assurance that water, organisms, nutrients, and energy can flow throughout the river environment is the integrity of the river’s connectivity (Grill et al., 2019). For instance, the level of connectivity of a river network can influence the variability in nitrogen removal. Figure 5 depicts the combined factors affecting the migration and transformation of nutrients. In terms of connectivity, lower connectivity means less N elimination, so downstream N transport will be greater. Reservoirs that are well-connected would remove more nitrogen because these reservoirs are more likely to be well-mixed and can remove nitrogen more effectively (Worman and Kronnas, 2005). This is because the control of nutrient levels greatly depends on the shape of the reservoir, particularly its surface area (Seitzinger et al., 2002). Changes in nutrient elements further affect biological processes; therefore, it is necessary to study the location and connectivity of reservoirs.
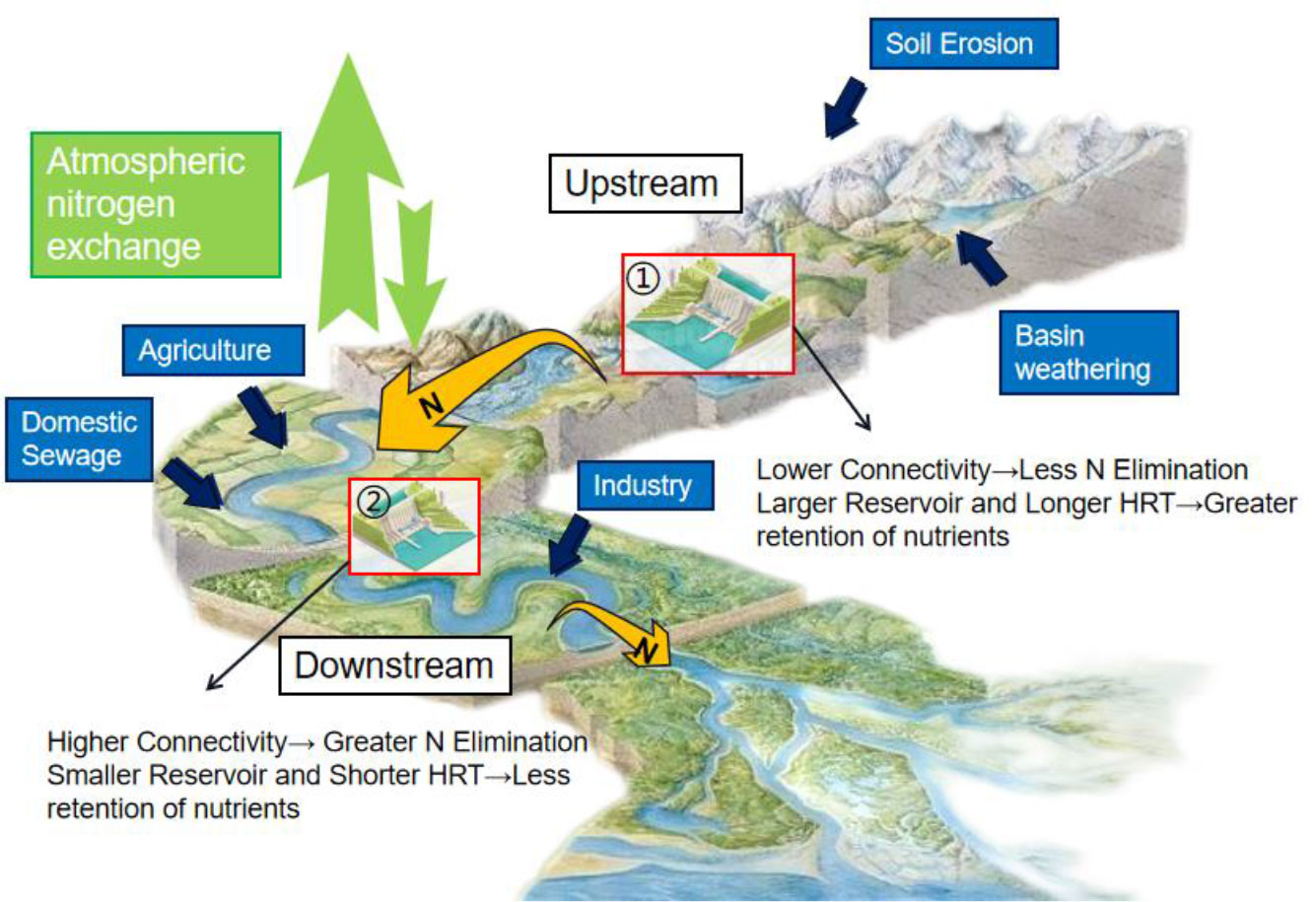
Figure 5 The influence of river hydrological factors on the migration and transformation of nutrients. HRT, hydraulic retention time.
Influence of storage capacity and hydraulic residence time
An increase in hydraulic retention time (HRT) is one of the most significant hydrological alterations caused by damming (Messager et al., 2016). Studies have shown that after the construction of a reservoir, the HRT may grow to months or even years (Prairie et al., 2018). Hydraulic retention time is frequently referred to as the “master variable,” which regulates biogeochemical reactions.
The water depth and HRT are not the same because the reservoir’s size (reservoir capacity) and hydraulic load are not equal. Typically, there is a positive correlation between nutrient retention and the HRT of reservoirs. This is mainly because seasonal thermal stratification is more pronounced in reservoirs with longer HRT. Significant chemical and biological stratification are altered by significant thermal stratification, which impacts primary productivity (Liu et al., 2012) and further changes the nutrient cycling within the reservoir (Van Cappellen and Maavara, 2016). The HRT in large reservoirs is often longer than those in small reservoirs, leading to a higher nutrient retention efficiency in large reservoirs.
However, according to other studies, reservoirs with short HRT may have abnormally high biogeochemical reactivity per unit area or time. An inverse link between HRT and the elimination rates of TP, TN, nitrate, and phosphate was discovered in more than 200 small reservoirs (Hejzlar et al., 2009). It is hypothesized that as the volume of the reservoir decreases, the contact area between the sediment and water increases, thereby increasing the nutrient retention efficiency.
Extensive research has shown that the HRT in reservoirs is an important first-order predictor (Maavara et al., 2020b). Increases in nutrient (N, P, Si, and C) retention are generally predicted by increases in the HRT. For instance, Akbarzadeh et al. claimed that a positive correlation could be found between the average HRT of the reservoir and its apparent net nitrogen removal efficiency, which is defined as the difference between the annual flux of N carried with the river inflow and the flux of N flowing out through the dam (Grantz et al., 2014; Akbarzadeh et al., 2019). With respect to phosphorus, the function of HRT is more pronounced. In reservoirs with longer HRTs, particle sedimentation of adsorbed phosphorus increases and algae and bacteria further consume dissolved phosphorus. In contrast, phosphorus retention is lower in new reservoirs without a buffer plant zone (Suchowolec and Gorniak, 2009). When hydropower stations generate electricity, they must discharge floods for safety; therefore, the HRT will be much shorter than that of reservoirs. This explains why an increasing number of dams do not increase the retention of P. Silicon and HRT also have a similar relationship. The DSi retention efficiency is significantly and negatively correlated with both the average reservoir depth and hydraulic load (the hydraulic load is negatively correlated with HRT) (Maavara et al., 2014). Similarly, the ability of reservoirs to retain and remove C is strongly related to its HRT. When HRT increases, the release rate of methane gas increases (Zhang et al., 2020). The longer the reservoir HRT, the lower the flow velocity, and thus, the better the sunlight mineralization in shallow waters with dissolved organic carbon (DOC)-rich mineral complexes. Moreover, the greater contact between water and the riverbed allows the complete decomposition of the DOC-rich material into carbon dioxide and methane gas (Prairie et al., 2018). Following a review of the literature, the HRT and retention of the four fundamental nutrients were gathered. The fitting curves showing the link between HRT and nutrient retention efficiency are shown in Figure 6.
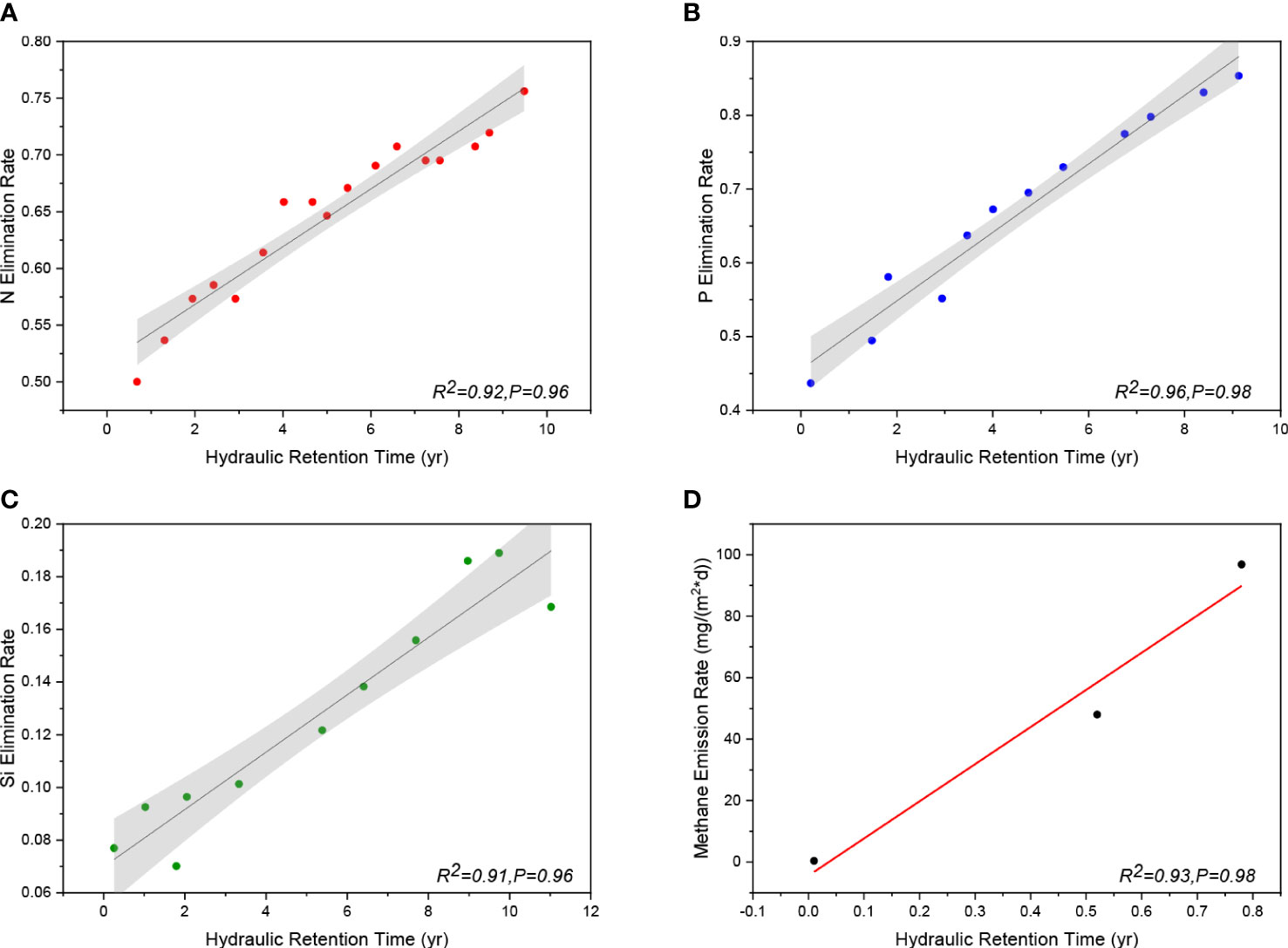
Figure 6 The graph names in order: (A): Fitting curve of HRT and nitrogen removal efficiency (R2 = 0.92, P = 0.96); (B): Fitting curve of HRT and phosphorus removal efficiency (R2 = 0.96, P = 0.98); (C): Fitting curve of HRT and silicon removal efficiency (R2 = 0.91, P = 0.96); (D): Fitting curve of HRT and methane gas emission rate (water depth = 3m) (Data are from Li et al., 2018; Maavara et al., 2020b).
The nutrient load and ratio are also controlled by HRT. Under most conditions, P is often removed from reservoirs more effectively than N and Si (Boesch, 2002; Howarth and Marino, 2006; Schaefer and Alber, 2007). The dominance of P retention in freshwater systems or the simplicity of adsorption of dissolved phosphorus to mineral surfaces may be the driving mechanisms for the preferential removal of P in reservoirs (Maranger et al., 2018). The removal of Si can be more effective than those of P and N at an HRT of less than 50 days (Barrera-Herrera et al., 2020). For instance, Si retention (72% DSi and 16% BSi) occurred before P retention (50%) in the Three Gorges Reservoir with an HRT of 27 days (Boesch, 2002; Ran et al., 2013; Huang et al., 2015). Under short HRT conditions, Si elimination may be prioritized because of the ability of diatoms to establish communities more quickly than other phytoplankton communities (Paerl et al., 2006).
Influence of hydropower development mode (single or cascade reservoirs)
Cascade reservoirs can jointly control flows, so the water level fluctuation is smaller than that of a single reservoir (Shi et al., 2020b); however, cascade reservoirs cause higher nutrients and water retention. The retention of N, P, and Si within cascade reservoirs is cumulative (Descloux et al., 2017). For example, cascade hydropower development reduced the output of TP in the Yellow River basin by approximately 84% (Ouyang et al., 2011). The numerous dams and reservoirs built in the upper Yangtze River basin, particularly after the construction of the Three Gorges Dam, have reduced the delivery of TP to the middle and lower basins by 77% (Zhou et al., 2013). The retention of reactive P in Luxembourg’s terrace dams increases progressively after passing through each dam (Salvia-Castellvi et al., 2001). Currently, research on the cumulative effects of cascades is insufficient, which is an urgent problem to solve.
Global warming is accelerated by the construction of cascade reservoirs around rivers. Methane and carbon dioxide emissions from cascade hydropower were measured by Shi et al. in Lancang River (Shi et al., 2017). The results showed that the CH4/CO2 ratio of the first reservoir (0.023) was higher than those of the other reservoirs. The comparatively higher nutrient retention in the first reservoir has the potential to cause eutrophication, which introduces exogenous organic carbon into the sediment. This potentially amplifies the warming potential of the first reservoir (Shi et al., 2017). Another study on nitrous oxide emissions from heavily dammed cascade hydropower stations in the upper Mekong River using a thin boundary layer model reached similar conclusions (Shi et al., 2020b). Thus, greater attention should be paid to GHG emissions from the first reservoir of cascade reservoirs, and methods should be adopted to reduce the potential for global warming (Wei et al., 2022).
Influence of microbial community variation
As key drivers of biogeochemical cycles, riverine microbes are essential for biogeochemical cycles that influence energy transport, food chain transmission, and water self-purification (Kuypers et al., 2018; Sohrt et al., 2019). In aquatic environments, some microorganisms play important roles in nutrient removal. For instance, Aspergillus is crucial to the metabolism of carbon and nitrogen (Hou et al., 2018). Actinobacteria are essential for nitrification, denitrification, and organic carbon decomposition (Droppo et al., 2016). Bacteroides are primarily responsible for the nitrification and degradation of organic matter (Wu et al., 2018).
Because microorganisms are involved in many significant biogeochemical reactions, it is critical to understand the effects of dams on microbial succession and environmental driving factors (Hanson et al., 2012; Ren et al., 2017). Environmental factors have a significant impact on the composition and function of microbial communities (Shade et al., 2012; Brasell et al., 2021). According to a study on the relationship between plankton and nutrients in regulating reservoirs of the South-North Water Diversion Project, temperature, DO, pH, chlorophyll-a, TP, nitrate, and ammonia nitrogen significantly influence the bacterial community and functional composition of water (Zhou et al., 2020). After reservoir construction, stratification changes the distribution of physical characteristics (temperature, DO), which may result in the formation of vertical nutrient (TDP, NH4+, etc.) gradients and alter the structure of the microbial communities. This results in spatial heterogeneity of bacterial communities under reservoir stratification (Yu et al., 2014; Yang et al., 2015; Xue et al., 2017). Furthermore, reservoir construction changes the riverine aquatic ecology from a “riverine” heterotrophic system to a “lacustrine” autotrophic system dominated by plankton (Thomson et al., 2005). In addition, the photosynthetic ability of planktonic bacteria is improved by the lower turbidity and higher light transmission in the surface water, which leads to the increase of planktonic bacteria over time, further strengthening the “lake-type” autotrophic system (Saito et al., 2001).
The enrichment of nutrients within the reservoir converts biological activity into a crucial element that can speed up material cycling, especially during the warm season. After the impoundment of the Wujiangdu reservoir in the upper reaches of the Wujiang River, it has been claimed that the biogeochemical cycle of carbon has been enhanced and that there has been a large increase in phytoplanktonic bacterial biomass (Han et al., 2018). However, because of the change in nutrient contents over time, the nutrients that control phytoplankton development can change over time (Paerl et al., 2011; Teixeira et al., 2018), and microbial communities can exhibit patterns that are harmful to the river’s aquatic ecosystem. For instance, unlike phosphorus limitation in spring, the water in summer is nitrogen-limited, and the temperature is more favorable for algal growth (Paerl et al., 2014; Bullerjahn et al., 2016). With the growth of algae, the turbidity of water increases, promoting hypoxia in deeper areas of the lake and impacting riverine ecosystems (Feist et al., 2016). Therefore, it is critical to pay close attention to changes in the microbial community, particularly the development of hazardous algae such as cyanobacteria that frequently cause water blooms.
Influence of anthropogenic nutrient input
Phosphorus export is directly affected by anthropogenic phosphorus sources, sewage treatment, and dam building (Powers et al., 2016). In addition to reservoir construction activities, exogenous pollution inputs from human activities also greatly affect nutrient transport and balance—major factors influencing the ecological health of rivers and nutrient cycling. Reservoirs retain nutrients, but the increase in nutrient flux caused by anthropogenic discharge still exceeds the amount retained after damming (Sun et al., 2022). For example, reservoir retention can significantly reduce P exports to downstream areas and coastal zones. However, P retention by reservoirs does not always result in a reduction in P export from the watershed. Instead, exports may increase because of the significant influence of anthropogenic P sources (Kumar et al., 2022). Moreover, exogenous input changes the proportion of nutrients available in different forms. In the Yangtze River, between 1970 and 2010, dissolved P export increased ten times, but a 44% decline in TP export indicated a long-term decline in particulate P export (Powers et al., 2016).
When additional steps were taken to reduce exogenous inputs from anthropogenic discharges, the cycle of nutrients in the river significantly changed. For instance, the output of P decreased by 86% in the Thames after an improvement in sewage treatment efficiency (Haygarth et al., 2014). Due to the reduction of domestic sewage and industrial wastewater, the overall phosphorus loads in the Rhine decreased by more than six times between 1977 and 2005 (Van Nieuwenhuyse, 2007; Leuven et al., 2009). This indicates that river nutrient status can be improved by restricting anthropogenic external inputs or enhancing sewage treatment efficiency in addition to dam management to control nutrient status.
Countermeasures to reduce the impact of reservoir on nutrients
Dam construction has a significant impact on river ecosystems. This study focuses on two engineering solutions to lessen the effect of reservoirs on nutrients in watersheds where dam construction has disrupted the system. These are dam removal and tributary habitat replacement.
Dam removal
Because of the aging of building materials and the accumulation of sediments after water storage, dams are dismantled when they can no longer serve their intended purpose (Ding et al., 2019). Dams are rarely completely destroyed, but maintaining them can be expensive (Hansen et al., 2020). Therefore, dam removal is viewed as a realistic option when the expense of management is greater than the cost of removal (O'Connor et al., 2015), particularly in cases where there is a high possibility of river ecological restoration. So far, 3,450 dams have been taken down in Sweden, the United Kingdom and some other European countries (Moran et al., 2018).
Dam removal has two distinct effects. As depicted in Figure 7, removing dams offers the opportunity for the ecological restoration of the river (Doyle et al., 2003; Brown et al., 2022; Leisher et al., 2022). However, dam removal exposes and transports huge amounts of sedimentary pollutants accumulated in the reservoir to downstream areas because of the greater flow when the dam is dismantled. This causes an increase in N, P, and toxic pollution downstream (Hitt et al., 2012; Pess et al., 2014). For instance, the demolition of the Fort Edward Dam in 1973 led to the flow of sediments containing polychlorinated biphenyls downstream in New York (Ryan Bellmore et al., 2017). In addition, groundwater levels decrease because of reservoir runoff and dam removal (Inamdar et al., 2021). As a result of this decline, downstream streams become deeper and larger in cross-section, which has permanent effects such as stream bed degradation, stream surface depression, and erosion by nutrient-rich sediments (Stanley and Doyle, 2003). However, choosing not to dismantle dams does not guarantee that the nutrients are always retained in upstream reservoirs. When an aging dam remains in place, the removal efficiency of sediments and nutrients decreases over time. Therefore, weighing these risks and advantages is necessary to determine whether and how to remove a dam (Kirchherr and Charles, 2016; Bohrerova et al., 2017).
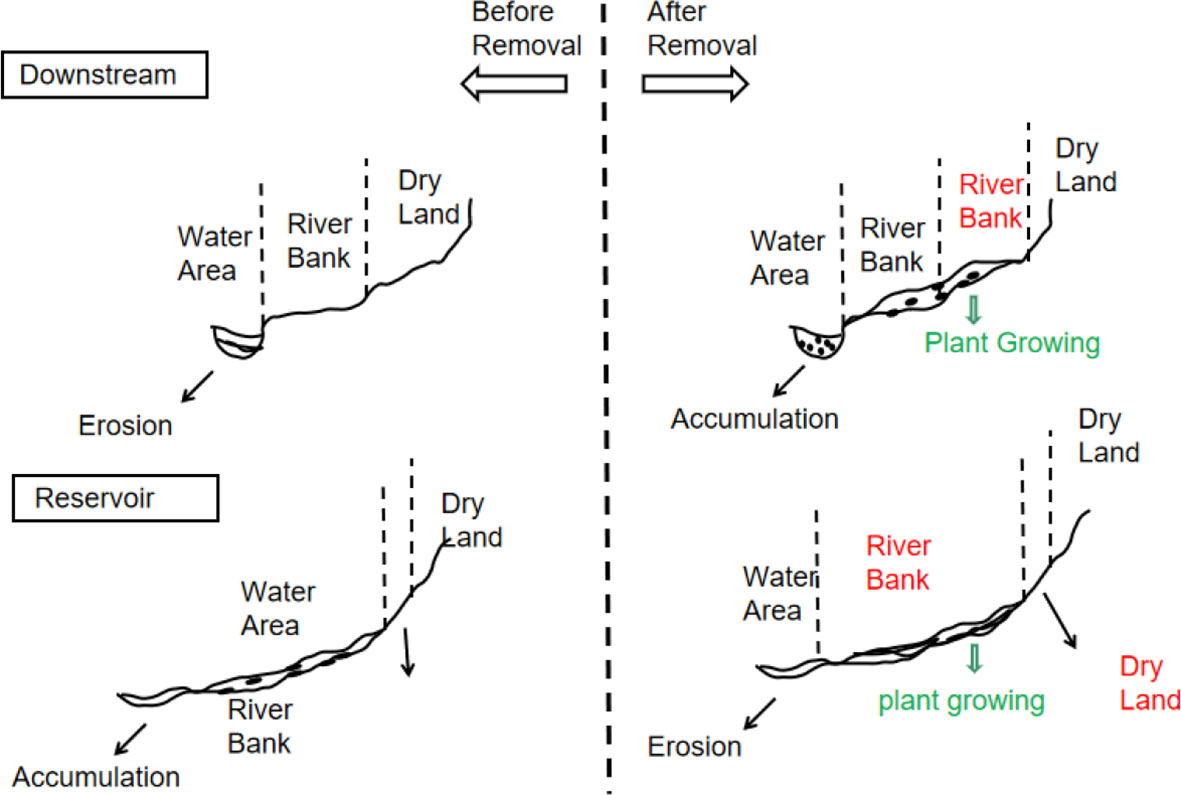
Figure 7 Two possible outcomes of dam removal. The red words in the figure show the cross-section areas that have changed after the dam removal, the green words show the riparian plant zone, and black particles represent sediment and nutrients.
Although people are becoming more conscious of the consequences of dam removal, the assessment and monitoring of river dam removal remain insufficient. According to Ryan Bellmore et al. (Ryan Bellmore et al., 2017), only 9% of the dams in the US had received a scientific evaluation before removal by the end of 2014. Pre-removal monitoring was conducted on a small percentage of dams, although it often lasted only one or two years. Post-removal monitoring was also scarce, with only a few dams observed for more than five years. However, it can take decades to observe an ecological response after dam removal (Beechie et al., 2010). To better manage river ecosystems, it is advised to consider five fundamental issues: goals of the river ecosystem, obstacles to achieving these goals, key issues of implementation, and the long-term effects of climate change and land and water management (Acreman, 2016).
Tributary habitat replacement
To strike a balance between the requirements for resource utilization and biodiversity conservation, emphasis should be placed on long-term ecological restoration and tributary habitat replacement. Tributary habitat replacement is one of the current environmental protection strategies for hydropower production (Nel et al., 2007). It has two definitions: (i) habitat replacement, which refers to replacing mainstream habitats with tributary habitats to provide suitable habitats for aquatic organisms impacted by mainstream development; (ii) alternative measures, which refer to tributary protection as alternative or complementary measures to the traditional protection measures for the mainstream, to alleviate the conflict between mainstream development and in-situ conservation of mainstream ecosystems (Caissie and Smith, 2022). As shown in Figure 8, the introduction of tributaries not only increases biological exchange and migratory species in the land-water connection zone, but also reduces HRT and sediment transport. This leads to better river conditions after several decades.
Currently, many ecosystem evaluations are based exclusively on major rivers and ignore the conservation potential of their important tributaries. However, these tributaries typically represent the same type of ecosystem with better conditions. Some river ecosystems between the main rivers and tributaries are considered to be less dangerous when tributaries are taken into account in ecosystem assessments, demonstrating the value of tributaries in maintaining biodiversity (da Silva et al., 2019). Downstream tributaries play a significant role in restoring rivers to a more “natural” state by offering a more suitable source of sediments and better water flow.
Protecting aquatic organisms in tributary habitats can compensate for affected mainstream habitats and balance development and conservation between the mainstream and tributaries (Poff et al., 2007; Ribeiro et al., 2022). Tributaries have successfully mitigated the negative effects of damming. The import from tributaries has reduced nutrient loading, successfully controlling cyanobacterial blooms that once plagued Portugal (Chicharo et al., 2006). Four tributaries in the upper Hun River, a significant water supply for the Dayefang Reservoir in northeastern China, are the main controls for lowering nitrogen loading (Ma et al., 2015). The overall discharge of DOC into the receiving water can be decreased via tributary diversions (Rohlfs et al., 2016). In addition, in the Tennessee Valley, the protection of tributaries for dam management improved downstream DO and biodiversity (Bednarik et al., 2017).
Conclusion
This review evaluated the direct impact of damming on hydro-physical factors, such as the river water flux and velocity, which further influences the balance of the fluxes of the main nutrients (N, P, Si, and C). Due to the changes in nutrient concentrations, the magnitude of the stoichiometric ratios of different nutrients is altered, which in turn induces the risk of eutrophication in reservoirs and the oligotrophication of downstream waters. In addition, damming leads to an increase in the emission of GHGs and thus worsens global warming, especially in cascade reservoirs.
In addition, the impact of reservoirs on nutrient cycling is mainly influenced by river connectivity, HRT, hydropower development mode, microbial community, and anthropogenic nutrient input. Dam retention restricts the movement and exchange of water, organisms, nutrients, and energy throughout the river environment. HRT is significantly positively correlated with nutrient retention, which causes higher retention of water and nutrients in cascade reservoirs than in single reservoirs. Furthermore, exogenous inputs from human activities have a significant impact on nutrient transport and balance. Under the influence of damming, changes in the spatial and temporal succession of microbial communities influence microbial functions related to nutrient cycling, further affecting nutrient transport and transformation. Two typical engineering solutions—dam removal and tributary habitat replacement—are suggested to reduce the negative effects of reservoirs on river ecosystems. However, compared to dam removal, tributary habitat replacement is a better option for balancing resource utilization and protecting biodiversity. Future investigations are required on 1) changes in nutrient cycling in cascade reservoirs, 2) GHG discharge from cascade reservoirs, and 3) prospects for tributary habitat substitution.
Author contributions
XW performed the conceptualization, writing, supervision, and funding acquisition. YC contributed to the methodology, formal analysis, and writing. QY and XX performed methodology and writing. BH, JG, YZ, and YL carried out the writing. All authors contributed to the article and approved the submitted version.
Funding
This study was supported by the National Natural Science Foundation of China (42007149, 92047201, 92047303).
Conflict of interest
The authors declare that the research was conducted in the absence of any commercial or financial relationships that could be construed as a potential conflict of interest.
Publisher’s note
All claims expressed in this article are solely those of the authors and do not necessarily represent those of their affiliated organizations, or those of the publisher, the editors and the reviewers. Any product that may be evaluated in this article, or claim that may be made by its manufacturer, is not guaranteed or endorsed by the publisher.
References
Abril G., Guerin F., Richard S., Delmas R., Galy-Lacaux C., Gosse P., et al. (2005). Carbon dioxide and methane emissions and the carbon budget of a 10-year old tropical reservoir (Petit saut, French Guiana). Global Biogeochemical Cycles 19 (4), GB4007. doi: 10.1029/2005gb002457
Acreman M. (2016). Environmental flowsbasics for novices. Wiley Interdiscip. Reviews-Water 3 (5), 622–628. doi: 10.1002/wat2.1160
Akbarzadeh Z., Maavara T., Slowinski S., Van Cappellen P. (2019). Effects of damming on river nitrogen fluxes: A global analysis. Global Biogeochemical Cycles 33 (11), 1339–1357. doi: 10.1029/2019gb006222
Allen A. P., Gillooly J. F. (2009). Towards an integration of ecological stoichiometry and the metabolic theory of ecology to better understand nutrient cycling. Ecol. Lett. 12 (5), 369–384. doi: 10.1111/j.1461-0248.2009.01302.x
Atkins E. (2020). Contesting the 'greening' of hydropower in the Brazilian Amazon. Political Geogr. 80, 102179. doi: 10.1016/j.polgeo.2020.102179
Barrera-Herrera J. A., Aranguren-Riaño N., Páez-Ruíz Y. M., Molina-Pacheco L. B., Pedroza-Ramos A., Díaz-Ballesteros C. A. (2020). Incidencia del tiempo de retención hidráulica en el plancton del reservorio la chapa (Santana, boyacá), Colombia. Rev. la Academia Colombiana Cienc. Exactas Físicas y Naturales 44 (171), 407–422. doi: 10.18257/raccefyn.1022
Baumgartner L. J., Marsden T., Duffy D., Horta A., Ning N. (2022). Optimizing efforts to restore aquatic ecosystem connectivity requires thinking beyond large dams. Environ. Res. Lett. 17 (1), 014008. doi: 10.1088/1748-9326/ac40b0
Bednarik A., Blaser M., Matousu A., Hekera P., Rulik M. (2017). ). effect of weir impoundments on methane dynamics in a river. Sci. Total Environ. 584, 164–174. doi: 10.1016/j.scitotenv.2017.01.163
Beechie T. J., Sear D. A., Olden J. D., Pess G. R., Buffington J. M., Moir H., et al. (2010). Process-based principles for restoring river ecosystems. Bioscience 60 (3), 209–222. doi: 10.1525/bio.2010.60.3.7
Bi R., Ismar S., Sommer U., Zhao M. (2017). Environmental dependence of the correlations between stoichiometric and fatty acid-based indicators of phytoplankton nutritional quality. Limnology Oceanography 62 (1), 334–347. doi: 10.1002/lno.10429
Boesch D. F. (2002). Challenges and opportunities for science in reducing nutrient over-enrichment of coastal ecosystems. Estuaries 25 (4B), 886–900. doi: 10.1007/bf02804914
Bohrerova Z., Park E., Halloran K., Lee J. (2017). Water quality changes shortly after low-head dam removal examined with cultural and microbial source tracking methods. River Res. Appl. 33 (1), 113–122. doi: 10.1002/rra.3069
Brasell K. A., Howarth J., Pearman J. K., Fitzsimons S. J., Zaiko A., Pochon X., et al. (2021). Lake microbial communities are not resistant or resilient to repeated large-scale natural pulse disturbances. Mol. Ecol. 30 (20), 5137–5150. doi: 10.1111/mec.16110
Brown R. L., Thomas C. C., Cubley E. S., Clausen A. J., Shafroth P. B. (2022). Does large dam removal restore downstream riparian vegetation diversity? testing predictions on the elwha river, Washington, USA. Ecol. Appl. 32 (6). doi: 10.1002/eap.2591
Bullerjahn G. S., McKay R. M., Davis T. W., Baker D. B., Boyer G. L., D'Anglada L. V., et al. (2016). Global solutions to regional problems: Collecting global expertise to address the problem of harmful cyanobacterial blooms. A Lake Erie Case study. Harmful Algae 54, 223–238. doi: 10.1016/j.hal.2016.01.003
Caissie D., Smith A. (2022). Water temperature variability at culvert replacement sites and river thermal impacts related to the removal of an old sediment pond: application on the Barnet brook and a tributary of the nerepis river (New Brunswick, Canada). Environ. Monit. Assess. 194 (7), 470. doi: 10.1007/s10661-022-10117-5
Calic M., Caric M., Krsinic F., Jasprica N., Pecarevic M. (2013). Controlling factors of phytoplankton seasonal succession in oligotrophic Mali ston bay (south-eastern Adriatic). Environ. Monit. Assess. 185 (9), 7543–7563. doi: 10.1007/s10661-013-3118-2
Chicharo L., Chicharo M. A., Ben-Hamadou R. (2006). Use of a hydrotechnical infrastructure (Alqueva dam) to regulate planktonic assemblages in the guadiana estuary: Basis for sustainable water and ecosystem services management. Estuar. Coast. Shelf Sci. 70 (1-2), 3–18. doi: 10.1016/j.ecss.2006.05.039
Clymans W., Struyf E., Govers G., Vandevenne F., Conley D. J. (2011). Anthropogenic impact on amorphous silica pools in temperate soils. Biogeosciences 8 (8), 2281–2293. doi: 10.5194/bg-8-2281-2011
Colas F., Chanudet V., Daufresne M., Buchet L., Vigouroux R., Bonnet A., et al. (2020). Spatial and temporal variability of diffusive CO2 and CH4 fluxes from the Amazonian reservoir Petit-saut (French Guiana) reveals the importance of allochthonous inputs for long-term c emissions. Global Biogeochemical Cycles 34 (12), e2020GB006602. doi: 10.1029/2020gb006602
Cole J. J., Prairie Y. T., Caraco N. F., McDowell W. H., Tranvik L. J., Striegl R. G., et al. (2007). Plumbing the global carbon cycle: Integrating inland waters into the terrestrial carbon budget. Ecosystems 10 (1), 171–184. doi: 10.1007/s10021-006-9013-8
Cook P. L. M., Aldridge K. T., Lamontagne S., Brookes J. D. (2010). Retention of nitrogen, phosphorus and silicon in a large semi-arid riverine lake system. Biogeochemistry 99 (1-3), 49–63. doi: 10.1007/s10533-009-9389-6
Cornelis J. T., Delvaux B., Georg R. B., Lucas Y., Ranger J., Opfergelt S. (2011). Tracing the origin of dissolved silicon transferred from various soil-plant systems towards rivers: a review. Biogeosciences 8 (1), 89–112. doi: 10.5194/bg-8-89-2011
da Silva P. S., Miranda L. E., Makrakis S., de Assumpcao L., Dias J. H. P., Makrakis M. C. (2019). Tributaries as biodiversity preserves: An ichthyoplankton perspective from the severely impounded upper parana river. Aquat. Conservation-Marine Freshw. Ecosyst. 29 (2), 258–269. doi: 10.1002/aqc.3037
Deemer B. R., Harrison J. A., Li S. Y., Beaulieu J. J., Delsontro T., Barros N., et al. (2016). Greenhouse gas emissions from reservoir water surfaces: A new global synthesis. Bioscience 66 (11), 949–964. doi: 10.1093/biosci/biw117
Descloux S., Chanudet V., Serca D., Guerin F. (2017). Methane and nitrous oxide annual emissions from an old eutrophic temperate reservoir. Sci. Total Environ. 598, 959–972. doi: 10.1016/j.scitotenv.2017.04.066
Ding L., Chen L., Ding C., Tao J. (2019). Global trends in dam removal and related research: A systematic review based on associated datasets and bibliometric analysis. Chin. Geographical Sci. 29 (1), 1–12. doi: 10.1007/s11769-018-1009-8
Doyle M. W., Harbor J. M., Stanley E. H. (2003). Toward policies and decision-making for dam removal. Environ. Manage. 31 (4), 453–465. doi: 10.1007/s00267-002-2819-z
Droppo I. G., Krishnappan B. G., Lawrence J. R. (2016). Microbial interactions with naturally occurring hydrophobic sediments: Influence on sediment and associated contaminant mobility. Water Res. 92, 121–130. doi: 10.1016/j.watres.2016.01.034
Durr H. H., Meybeck M., Hartmann J., Laruelle G. G., Roubeix V. (2011). Global spatial distribution of natural riverine silica inputs to the coastal zone. Biogeosciences 8 (3), 597–620. doi: 10.5194/bg-8-597-2011
Elser J. J., Devlin S. P., Yu J., Baumann A., Church M. J., Dore J. E., et al. (2022). Sustained stoichiometric imbalance and its ecological consequences in a large oligotrophic lake. Proc. Natl. Acad. Sci. U.S.A. 119 (30). doi: 10.1073/pnas.2202268119
Fearnside P. M. (2002). Greenhouse gas emissions from a hydroelectric reservoir (Brazil's tucurui dam) and the energy policy implications. Water Air Soil pollut. 133 (1-4), 69–96. doi: 10.1023/a:1012971715668
Feist T. J., Pauer J. J., Melendez W., Lehrter J. C., DePetro P. A., Rygwelski K. R., et al. (2016). Modeling the relative importance of nutrient and carbon loads, boundary fluxes, and sediment fluxes on gulf of Mexico hypoxia. Environ. Sci. Technol. 50 (16), 8713–8721. doi: 10.1021/acs.est.6b01684
Fernandez-Alias A., Montano-Barroso T., Conde-Cano M.-R., Manchado-Perez S., Lopez-Galindo C., Quispe-Becerra J.-I., et al. (2022). Nutrient overload promotes the transition from top-down to bottom-up control and triggers dystrophic crises in a Mediterranean coastal lagoon. Sci. Total Environ. 846, 157388. doi: 10.1016/j.scitotenv.2022.157388
Filippelli G. M. (2008). The global phosphorus cycle: Past, present, and future. Elements 4 (2), 89–95. doi: 10.2113/gselements.4.2.89
Fox J. F., Ford W. I. (2016). Impact of landscape disturbance on the quality of terrestrial sediment carbon in temperate streams. J. Hydrology 540, 1030–1042. doi: 10.1016/j.jhydrol.2016.07.016
Friedl G., Wuest A. (2002). Disrupting biogeochemical cycles - consequences of damming. Aquat. Sci. 64 (1), 55–65. doi: 10.1007/s00027-002-8054-0
Gao Y., Cornwell J. C., Stoecker D. K., Owens M. S. (2012). Effects of cyanobacterial-driven pH increases on sediment nutrient fluxes and coupled nitrification-denitrification in a shallow fresh water estuary. Biogeosciences 9 (7), 2697–2710. doi: 10.5194/bg-9-2697-2012
Garnier J., Beusen A., Thieu V., Billen G., Bouwman L. (2010). N:P:Si nutrient export ratios and ecological consequences in coastal seas evaluated by the ICEP approach. Global Biogeochemical Cycles 24, GB0A05. doi: 10.1029/2009gb003583
Gilbert M. C., Akama A., Fernandes C. C., Albertson R. C. (2020). Rapid morphological change in multiple cichlid ecotypes following the damming of a major clearwater river in Brazil. Evolutionary Appl. 13 (10), 2754–2771. doi: 10.1111/eva.13080
Glibert P. M. (2012). Ecological stoichiometry and its implications for aquatic ecosystem sustainability. Curr. Opin. Environ. Sustainability 4 (3), 272–277. doi: 10.1016/j.cosust.2012.05.009
Glibert P. M. (2020). Harmful algae at the complex nexus of eutrophication and climate change. Harmful Algae 91, 101583. doi: 10.1016/j.hal.2019.03.001
Glibert P. M., Fullerton D., Burkholder J. M., Cornwell J. C., Kana T. M. (2011). Ecological stoichiometry, biogeochemical cycling, invasive species, and aquatic food webs: San Francisco estuary and comparative systems. Rev. Fisheries Sci. 19 (4), 358–417. doi: 10.1080/10641262.2011.611916
Grantz E. M., Haggard B. E., Scott J. T. (2014). Stoichiometric imbalance in rates of nitrogen and phosphorus retention, storage, and recycling can perpetuate nitrogen deficiency in highly-productive reservoirs. Limnology Oceanography 59 (6), 2203–2216. doi: 10.4319/lo.2014.59.6.2203
Grill G., Lehner B., Lumsdon A. E., MacDonald G. K., Zarfl C., Liermann C. R. (2015). An index-based framework for assessing patterns and trends in river fragmentation and flow regulation by global dams at multiple scales. Environ. Res. Lett. 10 (1), 015001. doi: 10.1088/1748-9326/10/1/015001
Grill G., Lehner B., Thieme M., Geenen B., Tickner D., Antonelli F., et al. (2019). Mapping the world's free-flowing rivers. Nature 569 (7755), 215–21+. doi: 10.1038/s41586-019-1111-9
Hamilton S. K., Tank J. L., Raikow D. F., Wollheim W. M., Peterson B. J., Webster J. R. (2001). Nitrogen uptake and transformation in a midwestern US stream: A stable isotope enrichment study. Biogeochemistry 54 (3), 297–340. doi: 10.1023/a:1010635524108
Hansen E., Chan K. S., Jones C. S., Schilling K. (2016). Assessing the relative importance of nitrogen-retention processes in a large reservoir using time-series modeling. J. Agric. Biol. Environ. Stat 21 (1), 152–169. doi: 10.1007/s13253-015-0218-1
Hansen H. H., Forzono E., Grams A., Ohlman L., Ruskamp C., Pegg M. A., et al. (2020). Exit here: strategies for dealing with aging dams and reservoirs. Aquat. Sci. 82 (1), 2. doi: 10.1007/s00027-019-0679-3
Hanson C. A., Fuhrman J. A., Horner-Devine M. C., Martiny J. B. H. (2012). Beyond biogeographic patterns: processes shaping the microbial landscape. Nat. Rev. Microbiol. 10 (7), 497–506. doi: 10.1038/nrmicro2795
Han Q., Wang B. L., Liu C. Q., Wang F. S., Peng X., Liu X. L. (2018). Carbon biogeochemical cycle is enhanced by damming in a karst river. Sci. Total Environ. 616, 1181–1189. doi: 10.1016/j.scitotenv.2017.10.202
Harrison J. A., Bouwman A. F., Mayorga E., Seitzinger S. (2010). Magnitudes and sources of dissolved inorganic phosphorus inputs to surface fresh waters and the coastal zone: A new global model. Global Biogeochemical Cycles 24, GB1003. doi: 10.1029/2009gb003590
Harrison J. A., Maranger R. J., Alexander R. B., Giblin A. E., Jacinthe P. A., Mayorga E., et al. (2009). The regional and global significance of nitrogen removal in lakes and reservoirs. Biogeochemistry 93 (1-2), 143–157. doi: 10.1007/s10533-008-9272-x
Hartmann J., Levy J., Kempe S. (2011). Increasing dissolved silica trends in the Rhine river: an effect of recovery from high p loads? Limnology 12 (1), 63–73. doi: 10.1007/s10201-010-0322-4
Haygarth P. M., Jarvie H. P., Powers S. M., Sharpley A. N., Elser J. J., Shen J. B., et al. (2014). Sustainable phosphorus management and the need for a long-term perspective: The legacy hypothesis. Environ. Sci. Technol. 48 (15), 8417–8419. doi: 10.1021/es502852s
Hejzlar J., Anthony S., Arheimer B., Behrendt H., Bouraoui F., Grizzetti B., et al. (2009). Nitrogen and phosphorus retention in surface waters: an inter-comparison of predictions by catchment models of different complexity. J. Environ. Monit. 11 (3), 584–593. doi: 10.1039/b901207a
Hitt N. P., Eyler S., Wofford J. E. B. (2012). Dam removal increases American eel abundance in distant headwater streams. Trans. Am. Fisheries Soc. 141 (5), 1171–1179. doi: 10.1080/00028487.2012.675918
Horvath H., Matyas K., Sule G., Presing M. (2013). Contribution of nitrogen fixation to the external nitrogen load of a water quality control reservoir (Kis-balaton water protection system, Hungary). Hydrobiologia 702 (1), 255–265. doi: 10.1007/s10750-012-1329-0
Hou L. F., Zhou Q., Wu Q. P., Gu Q. H., Sun M., Zhang J. M. (2018). Spatiotemporal changes in bacterial community and microbial activity in a full-scale drinking water treatment plant. Sci. Total Environ. 625, 449–459. doi: 10.1016/j.scitotenv.2017.12.301
Howarth R. W., Marino R. (2006). Nitrogen as the limiting nutrient for eutrophication in coastal marine ecosystems: Evolving views over three decades. Limnology Oceanography 51 (1), 364–376. doi: 10.4319/lo.2006.51.1_part_2.0364
Huang L., Fang H. W., Reible D. (2015). Mathematical model for interactions and transport of phosphorus and sediment in the three gorges reservoir. Water Res. 85, 393–403. doi: 10.1016/j.watres.2015.08.049
Humborg C., Blomqvist S., Avsan E., Bergensund Y., Smedberg E., Brink J., et al. (2002). Hydrological alterations with river damming in northern Sweden: Implications for weathering and river biogeochemistry. Global Biogeochemical Cycles 16 (3), 1039. doi: 10.1029/2000gb001369
Inamdar S., Peipoch M., Gold A. J., Lewis E., Hripto J., Sherman M., et al. (2021). Ghosts of landuse past: legacy effects of milldams for riparian nitrogen (N) processing and water quality functions. Environ. Res. Lett. 16 (3), 035016. doi: 10.1088/1748-9326/abd9f5
Jankowski K., Schindler D. E., Holtgrieve G. W. (2012). Assessing nonpoint-source nitrogen loading and nitrogen fixation in lakes using delta n-15 and nutrient stoichiometry. Limnology Oceanography 57 (3), 671–683. doi: 10.4319/lo.2012.57.3.0671
Jansen N., Hartmann J., Lauerwald R., Durr H. H., Kempe S., Loos S., et al. (2010). Dissolved silica mobilization in the conterminous USA. Chem. Geology 270 (1-4), 90–109. doi: 10.1016/j.chemgeo.2009.11.008
Justic D., Rabalais N. N., Turner R. E. (2002). Modeling the impacts of decadal changes in riverine nutrient fluxes on coastal eutrophication near the Mississippi river delta. Ecol. Model. 152 (1), 33–46. doi: 10.1016/s0304-3800(01)00472-0
Keitel J., Zak D., Hupfer M. (2016). Water level fluctuations in a tropical reservoir: The impact of sediment drying, aquatic macrophyte dieback, and oxygen availability on phosphorus mobilization. Environ. Sci. Pollut. Res. 23 (7), 6883–6894. doi: 10.1007/s11356-015-5915-3
Kemenes A., Forsberg B. R., Melack J. M. (2011). CO2 emissions from a tropical hydroelectric reservoir (Balbina, Brazil). J. Geophysical Research-Biogeosciences 116, G03004. doi: 10.1029/2010jg001465
Kim C., Nishimura Y., Nagata T. (2006). Role of dissolved organic matter in hypolimnetic mineralization of carbon and nitrogen in a large, monomictic lake. Limnology Oceanography 51 (1), 70–78. doi: 10.4319/lo.2006.51.1.0070
Kirchherr J., Charles K. J. (2016). The social impacts of dams: A new framework for scholarly analysis. Environ. Impact Assess. Rev. 60, 99–114. doi: 10.1016/j.eiar.2016.02.005
Klausmeier C. A., Litchman E., Daufresne T., Levin S. A. (2008). Phytoplankton stoichiometry. Ecol. Res. 23 (3), 479–485. doi: 10.1007/s11284-008-0470-8
Koszelnik P., Tomaszek J. A. (2008). Dissolved silica retention and its impact on eutrophication in a complex of mountain reservoirs. Water Air Soil pollut. 189 (1-4), 189–198. doi: 10.1007/s11270-007-9567-x
Kreiling R. M., Bartsch L. A., Perner P. M., Hlavacek E. J., Christensen V. G. (2021). Riparian forest cover modulates phosphorus storage and nitrogen cycling in agricultural stream sediments. Environ. Manage. 68 (2), 279–293. doi: 10.1007/s00267-021-01484-9
Kumar A., Mishra S., Bakshi S., Upadhyay P., Thakur T. K. (2022). Response of eutrophication and water quality drivers on greenhouse gas emissions in lakes of China: A critical analysis. Ecohydrology e2483. doi: 10.1002/eco.2483
Kuypers M. M. M., Marchant H. K., Kartal B. (2018). The microbial nitrogen-cycling network. Nat. Rev. Microbiol. 16 (5), 263–276. doi: 10.1038/nrmicro.2018.9
Lane R. R., Day J. W., Justic D., Reyes E., Marx B., Day J. N., et al. (2004). Changes in stoichiometric Si, n and p ratios of Mississippi river water diverted through coastal wetlands to the gulf of Mexico. Estuar. Coast. Shelf Sci. 60 (1), 1–10. doi: 10.1016/j.ecss.2003.11.015
Lautz L., Fanelli R. (2008). Seasonal biogeochemical hotspots in the streambed around restoration structures. Biogeochemistry 91 (1), 85–104. doi: 10.1007/s10533-008-9235-2
Leisher C., Hess S., Dempsey K., Wynne M. L. P., Royte J. (2022). Measuring the social changes from river restoration and dam removal. Restor. Ecol. 30 (1), e13500. doi: 10.1111/rec.13500
Leuven R., van der Velde G., Baijens I., Snijders J., van der Zwart C., Lenders H. J. R., et al. (2009). The river Rhine: a global highway for dispersal of aquatic invasive species. Biol. Invasions 11 (9), 1989–2008. doi: 10.1007/s10530-009-9491-7
Liermann C. R., Nilsson C., Robertson J., Ng R. Y. (2012). Implications of dam obstruction for global freshwater fish diversity. Bioscience 62 (6), 539–548. doi: 10.1525/bio.2012.62.6.5
Liu M., Lei X., Zhou Y., Gao J., Zhou Y., Wang L., et al. (2022). Save reservoirs of humid subtropical cities from eutrophication threat. Environ. Sci. pollut. Res. 29 (1), 949–962. doi: 10.1007/s11356-021-15560-4
Liu L., Liu D. F., Johnson D. M., Yi Z. Q., Huang Y. L. (2012). Effects of vertical mixing on phytoplankton blooms in xiangxi bay of three gorges reservoir: Implications for management. Water Res. 46 (7), 2121–2130. doi: 10.1016/j.watres.2012.01.029
Liu M. D., Xie H., He Y. P., Zhang Q. R., Sun X. J., Yu C. H., et al. (2019). Sources and transport of methylmercury in the Yangtze river and the impact of the three gorges dam. Water Res. 166, 115042. doi: 10.1016/j.watres.2019.115042
Li S., Wang F. S., Zhou T., Cheng T. Y., Wang B. L. (2018). Carbon dioxide emissions from cascade hydropower reservoirs along the wujiang river, China. Inland Waters 8 (2), 157–166. doi: 10.1080/20442041.2018.1442040
Loucaides S., Van Cappellen P., Roubeix V., Moriceau B., Ragueneau O. (2012). Controls on the recycling and preservation of biogenic silica from biomineralization to burial. Silicon 4 (1), 7–22. doi: 10.1007/s12633-011-9092-9
Maavara T., Akbarzadeh Z., Van Cappellen P. (2020a). Global dam-driven changes to riverine N:P:Si ratios delivered to the coastal ocean. Geophysical Res. Lett. 47 (15), e2020GL088288. doi: 10.1029/2020gl088288
Maavara T., Chen Q. W., Van Meter K., Brown L. E., Zhang J. Y., Ni J. R., et al. (2020b). River dam impacts on biogeochemical cycling. Nat. Rev. Earth Environ. 1 (2), 103–116. doi: 10.1038/s43017-019-0019-0
Maavara T., Durr H. H., Van Cappellen P. (2014). Worldwide retention of nutrient silicon by river damming: From sparse data set to global estimate. Global Biogeochemical Cycles 28 (8), 842–855. doi: 10.1002/2014gb004875
Maavara T., Parsons C. T., Ridenour C., Stojanovic S., Duerr H. H., Powley H. R., et al. (2015). Global phosphorus retention by river damming. Proc. Natl. Acad. Sci. U.S.A. 112 (51), 15603–15608. doi: 10.1073/pnas.1511797112
Maavara T., Slowinski S., Rezanezhad F., Van Meter K., Van Cappellen P. (2018). The role of groundwater discharge fluxes on Si:P ratios in a major tributary to lake Erie. Sci. Total Environ. 622, 814–824. doi: 10.1016/j.scitotenv.2017.12.024
Ma J., Chen X., Huang B., Shi Y., Chi G. Y., Lu C. Y. (2015). Utilizing water characteristics and sediment nitrogen isotopic features to identify non-point nitrogen pollution sources at watershed scale in liaoning province, China. Environ. Sci. Pollut. Res. 22 (4), 2699–2707. doi: 10.1007/s11356-014-3540-1
Maranger R., Jones S. E., Cotner J. B. (2018). Stoichiometry of carbon, nitrogen, and phosphorus through the freshwater pipe. Limnology Oceanography Lett. 3 (3), 89–101. doi: 10.1002/lol2.10080
McPhillips L., Walter M. T. (2015). Hydrologic conditions drive denitrification and greenhouse gas emissions in stormwater detention basins. Ecol. Eng. 85, 67–75. doi: 10.1016/j.ecoleng.2015.10.018
Mebane C. A., Ray A. M., Marcarelli A. M. (2021). Nutrient limitation of algae and macrophytes in streams: Integrating laboratory bioassays, field experiments, and field data. PloS One 16 (6), e0252904. doi: 10.1371/journal.pone.0252904
Mendonca R., Kosten S., Sobek S., Barros N., Cole J. J., Tranvik L., et al. (2012). Hydroelectric carbon sequestration. Nat. Geosci. 5 (12), 838–840. doi: 10.1038/ngeo1653
Messager M. L., Lehner B., Grill G., Nedeva I., Schmitt O. (2016). Estimating the volume and age of water stored in global lakes using a geo-statistical approach. Nat. Commun. 7, 13603. doi: 10.1038/ncomms13603
Molisani M. M., Becker H., Barroso H. S., Hijo C. A. G., Monte T. M., Vasconcellos G. H., et al. (2013). The influence of castanhao reservoir on nutrient and suspended matter transport during rainy season in the ephemeral jaguaribe river (CE, Brazil). Braz. J. Biol. 73 (1), 115–123. doi: 10.1590/s1519-69842013000100013
Moran E. F., Lopez M. C., Moore N., Muller N., Hyndman D. W. (2018). Sustainable hydropower in the 21st century. Proc. Natl. Acad. Sci. U.S.A. 115, 47, 11891–11898. doi: 10.1073/pnas.1809426115
Mosseri J., Queguiner B., Rimmelin P., Leblond N., Guieu C. (2005). Silica fluxes in the northeast Atlantic frontal zone of mode water formation (38 degrees-45 degrees n, 16 degrees-22 degrees W) in 2001-2002. J. Geophysical Research-Oceans 110 (C7), C07S19. doi: 10.1029/2004jc002615
Moutinho F. H. M., Marafao G. A., Calijuri M. D., Moreira M. Z., Marcarelli A. M., Cunha D. G. F. (2021). Environmental factors and thresholds for nitrogen fixation by phytoplankton in tropical reservoirs. Int. Rev. Hydrobiology 106 (1), 5–17. doi: 10.1002/iroh.202002057
Mugidde R., Hecky R. E., Hendzel L. L., Taylor W. D. (2003). Pelagic nitrogen fixation in lake Victoria (East africa). J. Great Lakes Res. 29, 76–88. doi: 10.1016/s0380-1330(03)70540-1
Nair A. A., Yu F. (2020). Quantification of atmospheric ammonia concentrations: A review of its measurement and modeling. Atmosphere 11 (10), 1092. doi: 10.3390/atmos11101092
Nel J. L., Roux D. J., Maree G., Kleynhans C. J., Moolman J., Reyers B., et al. (2007). Rivers in peril inside and outside protected areas: a systematic approach to conservation assessment of river ecosystems. Diversity Distributions 13 (3), 341–352. doi: 10.1111/j.1472-4642.2007.00308.x
Noori R., Ansari E., Bhattarai R., Tang Q., Aradpour S., Maghrebi M., et al. (2021). Complex dynamics of water quality mixing in a warm mono-mictic reservoir. Sci. Total Environ. 777, 146097. doi: 10.1016/j.scitotenv.2021.146097
O'Connor J. E., Duda J. J., Grant G. E. (2015). 1000 dams down and counting. Science 348 (6234), 496–497. doi: 10.1126/science.aaa9204
Ouyang W., Hao F. H., Song K. Y., Zhang X. A. (2011). Cascade dam-induced hydrological disturbance and environmental impact in the upper stream of the yellow river. Water Resour. Manage. 25 (3), 913–927. doi: 10.1007/s11269-010-9733-6
Pacca S. (2007). Impacts from decommissioning of hydroelectric dams: a life cycle perspective. Climatic Change 84 (3-4), 281–294. doi: 10.1007/s10584-007-9261-4
Paerl H. W., Valdes L. M., Peierls B. L., Adolf J. E., Harding L. W. (2006). Anthropogenic and climatic influences on the eutrophication of large estuarine ecosystems. Limnology Oceanography 51 (1), 448–462. doi: 10.4319/lo.2006.51.1_part_2.0448
Paerl H. W., Xu H., Hall N. S., Zhu G. W., Qin B. Q., Wu Y. L., et al. (2014). Controlling cyanobacterial blooms in hypertrophic lake taihu, China: Will nitrogen reductions cause replacement of non-N-2 fixing by n-2 fixing taxa? PloS One 9 (11). doi: 10.1371/journal.pone.0113123
Paerl H. W., Xu H., McCarthy M. J., Zhu G. W., Qin B. Q., Li Y. P., et al. (2011). Controlling harmful cyanobacterial blooms in a hyper-eutrophic lake (Lake taihu, china): The need for a dual nutrient (N & p) management strategy. Water Res. 45 (5), 1973–1983. doi: 10.1016/j.watres.2010.09.018
Pan G., Krom M. D., Zhang M. Y., Zhang X. W., Wang L. J., Dai L. C., et al. (2013). Impact of suspended inorganic particles on phosphorus cycling in the yellow river (China). Environ. Sci. Technol. 47 (17), 9685–9692. doi: 10.1021/es4005619
Pess G. R., Quinn T. P., Gephard S. R., Saunders R. (2014). Re-colonization of Atlantic and pacific rivers by anadromous fishes: linkages between life history and the benefits of barrier removal. Rev. Fish Biol. Fisheries 24 (3), 881–900. doi: 10.1007/s11160-013-9339-1
Poff N. L., Olden J. D., Merritt D. M., Pepin D. M. (2007). Homogenization of regional river dynamics by dams and global biodiversity implications. Proc. Natl. Acad. Sci. United States America 104 (14), 5732–5737. doi: 10.1073/pnas.0609812104
Powers S. M., Bruulsema T. W., Burt T. P., Chan N. I., Elser J. J., Haygarth P. M., et al. (2016). Long-term accumulation and transport of anthropogenic phosphorus in three river basins. Nat. Geosci. 9 (5), 353–35+. doi: 10.1038/ngeo2693
Powers S. M., Tank J. L., Robertson D. M. (2015). Control of nitrogen and phosphorus transport by reservoirs in agricultural landscapes. Biogeochemistry 124 (1-3), 417–439. doi: 10.1007/s10533-015-0106-3
Prairie Y. T., Alm J., Beaulieu J., Barros N., Battin T., Cole J., et al. (2018). Greenhouse gas emissions from freshwater reservoirs: What does the atmosphere see? Ecosystems 21 (5), 1058–1071. doi: 10.1007/s10021-017-0198-9
Ramirez-Zierold J. A., Merino-Ibarra M., Monroy-Rios E., Olson M., Castillo F. S., Gallegos M. E., et al. (2010). Changing water, phosphorus and nitrogen budgets for Valle de bravo reservoir, water supply for Mexico city metropolitan area. Lake Reservoir Manage. 26 (1), 23–34. doi: 10.1080/07438140903539790
Ranalli A. J., Macalady D. L. (2010). The importance of the riparian zone and in-stream processes in nitrate attenuation in undisturbed and agricultural watersheds - a review of the scientific literature. J. Hydrology 389 (3-4), 406–415. doi: 10.1016/j.jhydrol.2010.05.045
Ran X. B., Yu Z. G., Yao Q. Z., Chen H. T., Guo H. B. (2013). Silica retention in the three gorges reservoir. Biogeochemistry 112 (1-3), 209–228. doi: 10.1007/s10533-012-9717-0
Ren Z., Wang F., Qu X. D., Elser J. J., Liu Y., Chu L. M. (2017). Taxonomic and functional differences between microbial communities in qinghai lake and its input streams. Front. Microbiol. 8. doi: 10.3389/fmicb.2017.02319
Ribeiro B. I. O., Braghin L. D. M., Lansac-Toha F. M., Bomfim F. F., Almeida B. A., Bonecker C. C., et al. (2022). Environmental heterogeneity increases dissimilarity in zooplankton functional traits along a large Neotropical river. Hydrobiologia 849 (14), 3135–3147. doi: 10.1007/s10750-022-04917-6
Rohlfs A. M., Mitrovic S. M., Williams S., Coleman D. (2016). Can tributary in-flows improve the recovery of the dissolved organic carbon regime in a snowmelt river regulated by a large reservoir? Mar. Freshw. Res. 67 (9), 1338–1345. doi: 10.1071/mf14230
Ryan Bellmore J., Duda J. J., Craig L. S., Greene S. L., Torgersen C. E., Collins M. J., et al. (2017). Status and trends of dam removal research in the united states. Wiley Interdiscip. Reviews-Water 4 (2), e1164. doi: 10.1002/wat2.1164
Saito L., Johnson B. M., Bartholow J., Hanna R. B. (2001). Assessing ecosystem effects of reservoir operations using food web-energy transfer and water quality models. Ecosystems 4 (2), 105–125. doi: 10.1007/s100210000062
Salvia-Castellvi M., Dohet A., Vander Borght P., Hoffmann L. (2001). Control of the eutrophication of the reservoir of esch-sur-Sure (Luxembourg): evaluation of the phosphorus removal by predams. Hydrobiologia 459, 61–71. doi: 10.1023/a:1012548006413
Sauer D., Saccone L., Conley D. J., Herrmann L., Sommer M. (2006). Review of methodologies for extracting plant-available and amorphous Si from soils and aquatic sediments. Biogeochemistry 80 (1), 89–108. doi: 10.1007/s10533-005-5879-3
Schaefer S. C., Alber M. (2007). Temperature controls a latitudinal gradient in the proportion of watershed nitrogen exported to coastal ecosystems. Biogeochemistry 85 (3), 333–346. doi: 10.1007/s10533-007-9144-9
Schindler D. W., Hecky R. E., Findlay D. L., Stainton M. P., Parker B. R., Paterson M. J., et al. (2008). Eutrophication of lakes cannot be controlled by reducing nitrogen input: Results of a 37-year whole-ecosystem experiment. Proc. Natl. Acad. Sci. United States America 105 (32), 11254–11258. doi: 10.1073/pnas.0805108105
Schmadel N. M., Harvey J. W., Alexander R. B., Schwarz G. E., Moore R. B., Eng K., et al. (2018). Thresholds of lake and reservoir connectivity in river networks control nitrogen removal. Nat. Commun. 9, 2779. doi: 10.1038/s41467-018-05156-x
Seitzinger S. P., Styles R. V., Boyer E. W., Alexander R. B., Billen G., Howarth R. W., et al. (2002). Nitrogen retention in rivers: model development and application to watersheds in the northeastern USA. Biogeochemistry 57 (1), 199–237. doi: 10.1023/a:1015745629794
Shade A., Read J. S., Youngblut N. D., Fierer N., Knight R., Kratz T. K., et al. (2012). Lake microbial communities are resilient after a whole-ecosystem disturbance. Isme J. 6 (12), 2153–2167. doi: 10.1038/ismej.2012.56
Shi W., Chen Q., Yi Q., Yu J., Ji Y., Hu L., et al. (2017). Carbon emission from cascade reservoirs: Spatial heterogeneity and mechanisms. Environ. Sci. Technol. 51 (21), 12175–12181. doi: 10.1021/acs.est.7b03590
Shi W. Q., Chen Q. W., Zhang J. Y., Liu D. S., Yi Q. T., Chen Y. C., et al. (2020a). Nitrous oxide emissions from cascade hydropower reservoirs in the upper Mekong river. Water Res. 173, 115582. doi: 10.1016/j.watres.2020.115582
Shi W. Q., Chen Q. W., Zhang J. Y., Zheng F. F., Liu D. S., Yi Q. T., et al. (2020b). Enhanced riparian denitrification in reservoirs following hydropower production. J. Hydrology 583, 124305. doi: 10.1016/j.jhydrol.2019.124305
Smith V. H., Schindler D. W. (2009). Eutrophication science: where do we go from here? Trends Ecol. Evol. 24 (4), 201–207. doi: 10.1016/j.tree.2008.11.009
Sohrt J., Uhlig D., Kaiser K., von Blanckenburg F., Siemens J., Seeger S., et al. (2019). Phosphorus fluxes in a temperate forested watershed: Canopy leaching, runoff sources, and in-stream transformation. Front. Forests Global Change 2. doi: 10.3389/ffgc.2019.00085
Song C. H., Gardner K. H., Klein S. J. W., Souza S. P., Mo W. W. (2018). Cradle-to-grave greenhouse gas emissions from dams in the united states of America. Renewable Sustain. Energy Rev. 90, 945–956. doi: 10.1016/j.rser.2018.04.014
Stanley E. H., Doyle M. W. (2003). Trading off: the ecological removal effects of dam removal. Front. Ecol. Environ. 1 (1), 15–22. doi: 10.1890/1540-9295(2003)001[0015:Toteeo]2.0.Co;2
Stimson A. G., Allott T. E. H., Boult S., Evans M. G. (2017). Reservoirs as hotspots of fluvial carbon cycling in peatland catchments. Sci. Total Environ. 580, 398–411. doi: 10.1016/j.scitotenv.2016.11.193
Struyf E., Smis A., Van Damme S., Garnier J., Govers G., Van Wesemael B., et al. (2010). Historical land use change has lowered terrestrial silica mobilization. Nat. Commun. 1, 129. doi: 10.1038/ncomms1128
Suchowolec T., Gorniak A. (2009). Riverine water transformation during retention in small lowland reservoirs. Oceanological Hydrobiological Stud. 38 (4), 103–108. doi: 10.2478/v10009-009-0047-z
Sugiyama Y., Anegawa A., Kumagai T., Harita Y., Hori T., Sugiyama M. (2004). Distribution of dissolved organic carbon in lakes of different trophic types. Limnology 5 (3), 165–176. doi: 10.1007/s10201-004-0128-3
Sun Y., Wang C., Chen X., Liu S., Lu X., Chen H. Y. H., et al. (2022). Phosphorus additions imbalance terrestrial ecosystem C:N:P stoichiometry. Global Change Biol. doi: 10.1111/gcb.16417
Syvitski J. P. M., Vorosmarty C. J., Kettner A. J., Green P. (2005). Impact of humans on the flux of terrestrial sediment to the global coastal ocean. Science 308 (5720), 376–380. doi: 10.1126/science.1109454
Tang X. Q., Wu M., Li R. (2018). Distribution, sedimentation, and bioavailability of particulate phosphorus in the mainstream of the three gorges reservoir. Water Res. 140, 44–55. doi: 10.1016/j.watres.2018.04.024
Tavernini S., Pierobon E., Viaroli P. (2011). Physical factors and dissolved reactive silica affect phytoplankton community structure and dynamics in a lowland eutrophic river (Po river, Italy). Hydrobiologia 669 (1), 213–225. doi: 10.1007/s10750-011-0688-2
Teixeira I. G., Arbones B., Frojan M., Nieto-Cid M., Alvarez-Salgado X. A., Castro C. G., et al. (2018). Response of phytoplankton to enhanced atmospheric and riverine nutrient inputs in a coastal upwelling embayment. Estuar. Coast. Shelf Sci. 210, 132–141. doi: 10.1016/j.ecss.2018.06.005
Teodoru C., Dimopoulos A., Wehrli B. (2006). Biogenic silica accumulation in the sediments of iron gate I reservoir on the Danube river. Aquat. Sci. 68 (4), 469–481. doi: 10.1007/s00027-006-0822-9
Teodoru C., Wehrli B. (2005). Retention of sediments and nutrients in the iron gate I reservoir on the Danube river. Biogeochemistry 76 (3), 539–565. doi: 10.1007/s10533-005-0230-6
Thomson J. R., Hart D. D., Charles D. F., Nightengale T. L., Winter D. M. (2005). Effects of removal of a small dam on downstream macroinvertebrate and algal assemblages in a Pennsylvania stream. J. North Am. Benthological Soc. 24 (1), 192–207. doi: 10.1899/0887-3593(2005)024<0192:Eoroas>2.0.Co;2
Thouvenot-Korppoo M., Billen G., Garnier J. (2009). Modelling benthic denitrification processes over a whole drainage network. J. Hydrology 379 (3-4), 239–250. doi: 10.1016/j.jhydrol.2009.10.005
Triplett L. D., Engstrom D. R., Conley D. J., Schellhaass S. M. (2008). Silica fluxes and trapping in two contrasting natural impoundments of the upper Mississippi river. Biogeochemistry 87 (3), 217–230. doi: 10.1007/s10533-008-9178-7
Turner P. A., Griffis T. J., Baker J. M., Lee X., Crawford J. T., Loken L. C., et al. (2016). Regional-scale controls on dissolved nitrous oxide in the upper Mississippi river. Geophysical Res. Lett. 43 (9), 4400–4407. doi: 10.1002/2016gl068710
Van Cappellen P., Maavara T. (2016). Rivers in the anthropocene: Global scale modifications of riverine nutrient fluxes by damming. Ecohydrology Hydrobiology 16 (2), 106–111. doi: 10.1016/j.ecohyd.2016.04.001
Van Nieuwenhuyse E. E. (2007). Response of summer chlorophyll concentration to reduced total phosphorus concentration in the Rhine river (Netherlands) and the Sacramento - San Joaquin delta (California, USA). Can. J. Fisheries Aquat. Sci. 64 (11), 1529–1542. doi: 10.1139/f07-121
Vanni M. J., Renwick W. H., Bowling A. M., Horgan M. J., Christian A. D. (2011). Nutrient stoichiometry of linked catchment-lake systems along a gradient of land use. Freshw. Biol. 56 (5), 791–811. doi: 10.1111/j.1365-2427.2010.02436.x
Vitousek P. M., Porder S., Houlton B. Z., Chadwick O. A. (2010). Terrestrial phosphorus limitation: mechanisms, implications, and nitrogen-phosphorus interactions. Ecol. Appl. 20 (1), 5–15. doi: 10.1890/08-0127.1
Wang Y., Shen Z. Y., Niu J. F., Liu R. M. (2009). Adsorption of phosphorus on sediments from the three-gorges reservoir (China) and the relation with sediment compositions. J. Hazardous Materials 162 (1), 92–98. doi: 10.1016/j.jhazmat.2008.05.013
Wang S., Wang W., Liu L., Zhuang L., Zhao S., Su Y., et al. (2018). Microbial nitrogen cycle hotspots in the plant-Bed/Ditch system of a constructed wetland with N2O mitigation. Environ. Sci. Technol. 52 (11), 6226–6236. doi: 10.1021/acs.est.7b04925
Wei R., Zhang W. C., Peng S. J. (2022). Energy and greenhouse gas footprints of China households during 1995-2019: A global perspective. Energy Policy 164, 112939. doi: 10.1016/j.enpol.2022.112939
Winemiller K. O., McIntyre P. B., Castello L., Fluet-Chouinard E., Giarrizzo T., Nam S., et al. (2016). Balancing hydropower and biodiversity in the Amazon, Congo, and Mekong. Science 351 (6269), 128–129. doi: 10.1126/science.aac7082
Winton R. S., Calamita E., Wehrli B. (2019). Reviews and syntheses: Dams, water quality and tropical reservoir stratification. Biogeosciences 16 (8), 1657–1671. doi: 10.5194/bg-16-1657-2019
Withers P. J. A., Jarvie H. P. (2008). Delivery and cycling of phosphorus in rivers: A review. Sci. Total Environ. 400 (1-3), 379–395. doi: 10.1016/j.scitotenv.2008.08.002
Wollheim W. M., Vorosmarty C. J., Bouwman A. F., Green P., Harrison J., Linder E., et al. (2008). Global n removal by freshwater aquatic systems using a spatially distributed, within-basin approach. Global Biogeochemical Cycles 22 (2), GB2026. doi: 10.1029/2007gb002963
Worman A., Kronnas V. (2005). Effect of pond shape and vegetation heterogeneity on flow and treatment performance of constructed wetlands. J. Hydrology 301 (1-4), 123–138. doi: 10.1016/j.jhydrol.2004.06.038
Wurtsbaugh W. A. (2020). Nutrients, eutrophication and harmful algal blooms along the freshwater to marine continuum (vol 6, e1373, 2019). Wiley Interdiscip. Reviews-Water 7 (4), e1453. doi: 10.1002/wat2.1453
Wu D., Zhang Z. M., Yu Z. D., Zhu L. (2018). Optimization of F/M ratio for stability of aerobic granular process via quantitative sludge discharge. Bioresource Technol. 252, 150–156. doi: 10.1016/j.biortech.2017.12.094
Xue Y. Y., Yu Z., Chen H. H., Yang J. R., Liu M., Liu L. M. A., et al. (2017). Cyanobacterial bloom significantly boosts hypolimnelic anammox bacterial abundance in a subtropical stratified reservoir. FEMS Microbiol. Ecol. 93 (10), fix118. doi: 10.1093/femsec/fix118
Yang X., Huang T. L., Zhang H. H. (2015). Effects of seasonal thermal stratification on the functional diversity and composition of the microbial community in a drinking water reservoir. Water 7 (10), 5525–5546. doi: 10.3390/w7105525
Yan X., Thieu V., Garnier J. (2021). Long-term assessment of nutrient budgets for the four reservoirs of the seine basin (France). Sci. Total Environ. 778, 146412. doi: 10.1016/j.scitotenv.2021.146412
Yi Q. T., Chen Q. W., Shi W. Q., Lin Y. Q., Hu L. M. (2017). Sieved transport and redistribution of bioavailable phosphorus from watershed with complex river networks to lake. Environ. Sci. Technol. 51 (18), 10379–10386. doi: 10.1021/acs.est.7b02710
Yu Z., Yang J., Amalfitano S., Yu X. Q., Liu L. M. (2014). Effects of water stratification and mixing on microbial community structure in a subtropical deep reservoir. Sci. Rep. 4, 5821. doi: 10.1038/srep05821
Zervoudaki S., Nielsen T. G., Carstensen J. (2009). Seasonal succession and composition of the zooplankton community along an eutrophication and salinity gradient exemplified by Danish waters. J. Plankton Res. 31 (12), 1475–1492. doi: 10.1093/plankt/fbp084
Zhang X., Fang C., Wang Y., Lou X., Su Y., Huang D. (2022). Review of effects of dam construction on the ecosystems of river estuary and nearby marine areas. Sustainability 14 (10), 5974. doi: 10.3390/su14105974
Zhang L. W., Xia X. H., Liu S. D., Zhang S. B., Li S. L., Wang J. F., et al. (2020). Significant methane ebullition from alpine permafrost rivers on the East qinghai-Tibet plateau. Nat. Geosci. 13 (5), 349–354. doi: 10.1038/s41561-020-0571-8
Zhang L. W., Zhang S. B., Xia X. H., Battin T. J., Liu S. D., Wang Q. R., et al. (2022). Unexpectedly minor nitrous oxide emissions from fluvial networks draining permafrost catchments of the East Qinghai-Tibet Plateau. Nat. Commun. 13 (1), 950. doi: 10.1038/s41467-022-28651-8
Zhang X., Fang C., Wang Y., Lou X., Su Y., Huang D., et al. (2022). Review of effects of dam construction on the ecosystems of river estuary and nearby marine areas. Sustainability 14 (10), 5974. doi: 10.3390/su14105974
Zhou J., Scherer L., van Bodegom P. M., Beusen A., Mogollon J. M. (2022). Regionalized nitrogen fate in freshwater systems on a global scale. J. Ind. Ecol. 26 (3), 907–922. doi: 10.1111/jiec.13227
Zhou S. L., Sun Y., Huang T. L., Cheng Y., Yang X., Zhou Z. Z., et al. (2020). Reservoir water stratification and mixing affects microbial community structure and functional community composition in a stratified drinking reservoir. J. Environ. Manage. 267, 110456. doi: 10.1016/j.jenvman.2020.110456
Zhou J. J., Zhang M., Lu P. Y. (2013). The effect of dams on phosphorus in the middle and lower Yangtze river. Water Resour. Res. 49 (6), 3659–3669. doi: 10.1002/wrcr.20283
Zhu X., Chang K., Cai W., Zhang A., Yue G., Zhao X. (2022). Response of runoff and nitrogen loadings to climate and land use changes in the middle fenhe river basin in northern China. J. Water Climate Change 13 (7), 2817–2836. doi: 10.2166/wcc.2022.121
Keywords: river damming, nutrient cycling, microbial community, hydraulic residence time, tributary habitat replacement
Citation: Wang X, Chen Y, Yuan Q, Xing X, Hu B, Gan J, Zheng Y and Liu Y (2022) Effect of river damming on nutrient transport and transformation and its countermeasures. Front. Mar. Sci. 9:1078216. doi: 10.3389/fmars.2022.1078216
Received: 24 October 2022; Accepted: 07 November 2022;
Published: 21 November 2022.
Edited by:
Haohan Yang, Yangzhou University, ChinaReviewed by:
Xin Zhao, Northeastern University, ChinaZhengjian Yang, China Three Gorges University, China
Copyright © 2022 Wang, Chen, Yuan, Xing, Hu, Gan, Zheng and Liu. This is an open-access article distributed under the terms of the Creative Commons Attribution License (CC BY). The use, distribution or reproduction in other forums is permitted, provided the original author(s) and the copyright owner(s) are credited and that the original publication in this journal is cited, in accordance with accepted academic practice. No use, distribution or reproduction is permitted which does not comply with these terms.
*Correspondence: Xun Wang, eHdhbmcyMDE0QGhodS5lZHUuY24=
†These authors have contributed equally to this work and share first authorship