- Cooperative Institute for Marine and Atmospheric Research, NOAA Pacific Islands Fisheries Science Center, Honolulu, HI, United States
Past research has demonstrated how local-scale human impacts—including reduced water quality, overfishing, and eutrophication—adversely affect coral reefs. More recently, global-scale shifts in ocean conditions arising from climate change have been shown to impact coral reefs. Here, we surveyed benthic reef communities at 34 U.S.-affiliated Pacific islands spanning a gradient of oceanic productivity, temperature, and human habitation. We re-evaluated patterns reported for these islands from the early 2000s in which uninhabited reefs were dominated by calcifiers (coral and crustose coralline algae) and thought to be more resilient to global change. Using contemporary data collected nearly two decades later, our analyses indicate this projection was not realized. Calcifiers are no longer the dominant benthic group at uninhabited islands. Calcifier coverage now averages 26.9% ± 3.9 SE on uninhabited islands (compared to 45.18% in the early 2000s). We then asked whether oceanic productivity, past sea surface temperatures (SST), or acute heat stress supersede the impacts of human habitation on benthic cover. Indeed, we found variation in benthic cover was best explained not by human population densities, but by remotely sensed metrics of chlorophyll-a, SST, and island-scale estimates of herbivorous fish biomass. Specifically, higher coral and CCA cover was observed in more productive waters with greater biomass of herbivores, while turf cover increased with daily SST variability and reduced herbivore biomass. Interestingly, coral cover was positively correlated with daily variation in SST but negatively correlated with monthly variation. Surprisingly, metrics of acute heat stress were not correlated with benthic cover. Our results reveal that human habitation is no longer a primary correlate of calcifier cover on central Pacific island reefs, and highlight the addition of oceanic productivity and high-frequency SST variability to the list of factors supporting reef builder abundance.
Introduction
Local-scale human-induced impacts like eutrophication, increasing sedimentation, and overfishing can compromise water quality, alter trophic functions, and reduce resilience that is essential to maintaining coral-dominated reef ecosystems (Mumby et al., 2006; Carilli et al., 2009). By contrast, remote coral reef systems hundreds of kilometers from humans provide a natural control group for exploring the relationship between human habitation and reef resilience. Using data from 56 islands and atolls in the U.S.-affiliated central tropical Pacific, Kiribati, and Phoenix Islands from 2002–2005, Smith et al. (2016) found reefs on uninhabited islands—presumably subject to minimal local human impacts—to be home to more reef-building taxa such as corals and crustose coralline algae (CCA) compared to inhabited islands. The authors posited that cumulative local human impacts at inhabited islands and atolls across the central Pacific led to a reduction in the abundance of reef builders resulting in island-scale shifts to algal dominance. Conversely, the uninhabited islands in the region remained dominated by reef builders, leading the authors to surmise these islands may be more resilient to global change (Smith et al., 2016).
However, climate stress is an increasingly important factor impacting coral reefs in the central and western tropical Pacific (Robinson et al., 2018). Whereas in the early 2000s benthic communities at uninhabited islands in this region were dominated by reef builders, since the early 2000s, acute heat stress has induced coral bleaching with varying severity within the region (Barkley et al., 2018; Fox et al., 2019; Vargas-Ángel et al., 2019, Winston et al., 2022). Given that increased global thermal stress events are impacting coral reefs worldwide (Hughes et al., 2018), we aim to revisit whether human presence is as clearly correlated with benthic structure on today’s central Pacific reefs as Smith et al. (2016) found at the start of this century. To address this aim, we were able to resurvey 34 of the 56 islands analyzed by Smith et al. (2016). We then consider whether large-scale climate change impacts are superseding local-scale impacts of human habitation on benthic cover, and if so, what metrics are now most strongly correlated with patterns in benthic cover?
The Pacific islands and atolls included in this dataset span a gradient in oceanographic regimes across latitude, including varying sea-surface temperature (SST), precipitation, interannual temperature variability, nutrient availability, and aragonite saturation state (Williams et al., 2015; Carilli et al., 2017; Barkley et al, in review). The increased spatial availability and resolution of remotely sensed data from satellites enables quantifying these gradients despite the geographic remoteness of many of the islands in our dataset. Hence, we can readily quantify the magnitude and duration of SST above a regional threshold (i.e., temperature anomalies). For example, a conventional metric for this type of cumulative heat stress in SST is Coral Reef Watch’s Degree Heating Weeks (DHW), where significant coral bleaching is predicted to occur when DHW values exceed 4°C·week and widespread bleaching mortality when values exceed 8°C·week (Skirving et al., 2020). Recent studies also suggest that variability in SST may affect coral’s response to heat stress, such that areas subject to high historical temperature variability or high daily variations in temperature range may have improved resilience to bleaching (Carilli et al., 2012; Safaie et al., 2018; Sully et al., 2019). Thus, historical variability in SST quantified over different temporal scales (i.e., variation in monthly means in addition to magnitude of the daily SST anomalies) may be important metrics to explain observed differences in coral cover across space. Primary production can also influence coral trophic ecology, potentially influencing coral persistence in relation to spatial gradients in nutrient availability (Fox et al., 2018). Greater nutrient availability can enhance heterotrophy (coral feeding) and confer higher resilience during periods of heat stress (Grottoli et al., 2006; Radice et al., 2019), but in excess may actually lower coral bleaching thresholds (Wooldridge, 2020). Satellite sensors can measure ocean color used to derive surface chlorophyll-a (chl-a) as proxy for primary production.
In this investigation, we re-evaluate the island-level patterns and hypotheses presented by Smith et al. (2016) using contemporary data from 34 U.S. and U.S.-affiliated islands and atolls in the central tropical Pacific. As most of these islands remain uninhabited, this dataset presents a unique opportunity to consider the influence of human habitation across a broad geographic range. We begin our analyses by repeating the approach used by Smith et al. (2016); asking whether benthic cover of coral, macroalgae, reef builders (coral + CCA), and fleshy algae (turf + macroalgae) varies by human habitation (inhabited vs. uninhabited) when controlling for latitude. Region was also included in these initial analyses to explore regional differences in benthic composition. Additionally, the availability of satellite-derived products allowed us to look beyond latitude and human presence/absence to consider what factors related to a changing ocean climate—such as productivity, SST, and acute heat stress—best explain the observed spatial variation in current benthic cover. While latitude is often a primary correlate of coral-reef ecosystem structure (Stuart-Smith et al., 2018), we asked what oceanic metrics co-vary with latitude and may provide a more ecological meaningful explanatory variable. Lastly, given the importance of herbivory in controlling algal prevalence in the region (Heenan and Williams, 2013), we also included herbivorous fish biomass as another known local driver of benthic composition.
Methods
Quantifying benthic cover
Benthic cover surveys were conducted from 2016-2019 as part of the Coral Reef Conservation Program’s National Coral Reef Monitoring Program (NCRMP). A total of 1,898 NCRMP forereef sites from 34 islands and atolls spanning a gradient of human habitation are included in this analysis and grouped into four regions (Figure 1, Table S1). Site-level percent cover of benthic functional groups (e.g., coral, CCA, turf, macroalgae, Halimeda, encrusting macroalgae) was assessed in CoralNet from 30 photoquadrats collected at each meter along a 30m transect tape (see Lozada-Misa et al., 2017 for detailed methodology). The number of random sites per island varied in proportion to the area of hard substrate in each of three depth strata: 0–6m, >6–18m, >18–30m. Site level data were averaged within strata, weighted by the proportional strata area for a given island, and then summed to generate an island-scale weighted mean used for all subsequent analyses. Survey year varied by region: Northwestern Hawaiian Islands (2016), Mariana Islands (2017), American Samoa (2018), Pacific Remote Island Area (2018), and main Hawaiian Islands (2019). The Northwestern Hawaiian Islands (NWHI) were grouped with the main Hawaiian Islands to facilitate comparison with findings of Smith et al. (2016). Likewise, scleractinian coral and CCA cover were summed as “reef builder” cover, macroalgae and turf cover were summed as “fleshy algae” cover, and Halimeda and calcareous crustose macroalgae (e.g. Peyssonnelia) were summed as “calcifying macroalgae” to align with benthic cover categories delineated by Smith et al. (2016).
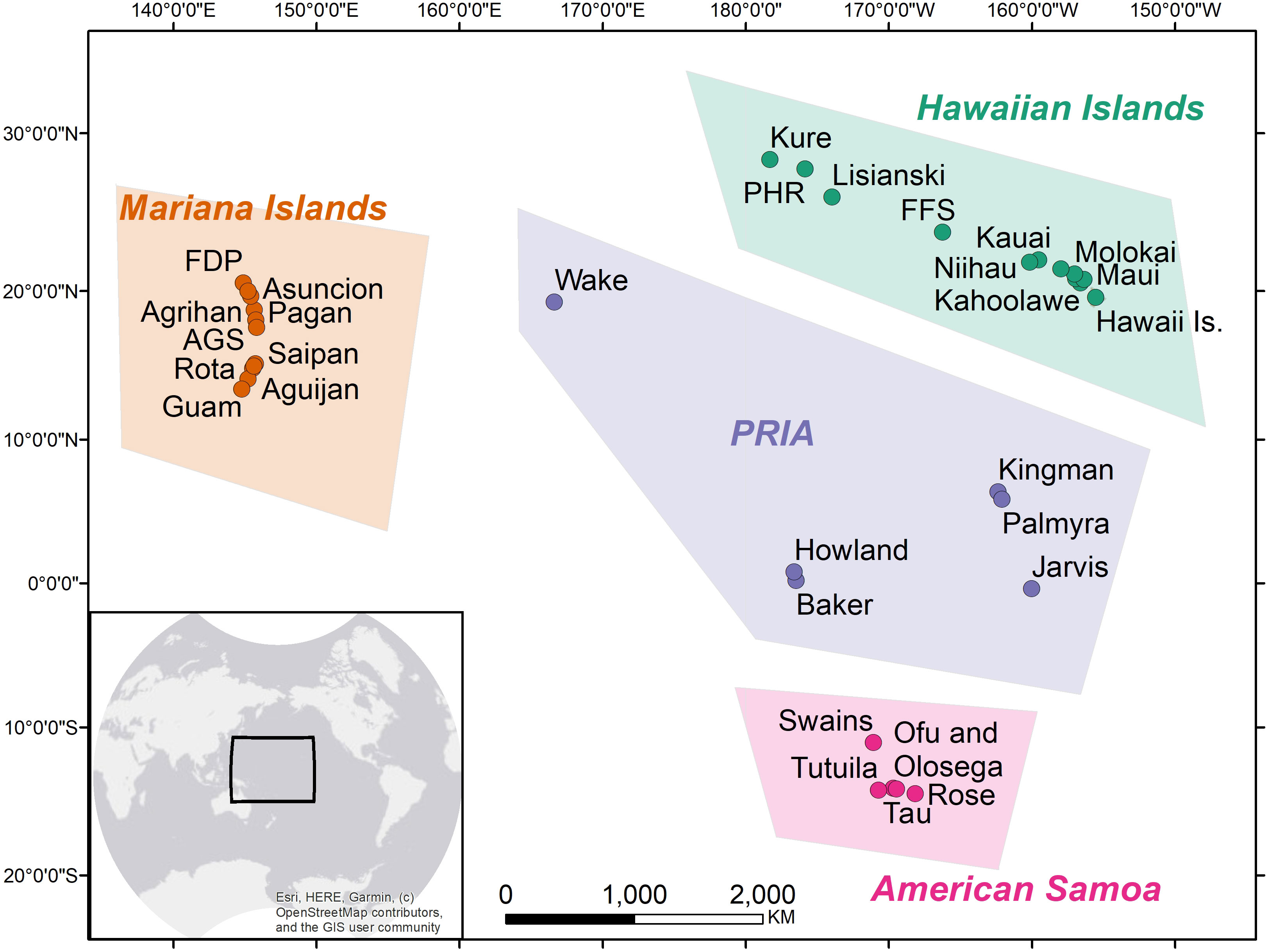
Figure 1 Map of the 34 islands and atolls included in the study grouped by region. Abbreviated island names are listed in Table S1.
Data analysis
We began conducting univariate and multivariate analyses to evaluate how benthic cover differs among islands with and without human presence. First, we assessed whether coral, macroalgal, reef builder, and fleshy algal cover varied by region and/or habitation (inhabited vs. uninhabited) using a two-way fixed effects analysis of covariance (ANCOVA) with absolute value of latitude as a covariate to account for the large spatial gradient in the dataset. Cover values were arcsine square root transformed and outliers removed prior to analysis to meet assumptions of ANCOVA (Table S2). Next, we analyzed benthic community composition using a two-factor permutational multivariate analysis of variance (PERMANOVA) on the Bray-Curtis dissimilarities of the square root transformed percent cover data (999 permutations) using the vegan package 2.5-7 in R (Oksanen et al., 2019). Region and habitation were again treated as fixed effects and absolute value of latitude was treated as a covariate. The PERMDISP2 procedure (Anderson, 2006)—a multivariate analogue of Levene’s test—was used to evaluate multivariate homogeneity assumptions for the PERMANOVA. Multivariate patterns in the data were visualized with nonmetric multidimensional scaling (NMDS) plots.
We expanded our analytical approach beyond the metrics of latitude, human habitation (as a binary response variable), and region to investigate how variability in island-level metrics of primary productivity, turbidity, past sea-surface temperature (SST), and heat stress correlate with shifts in benthic cover using distance based redundancy analysis (db-RDA). We also included herbivorous fish biomass as herbivory is an important correlate of benthic cover on reefs in American Samoa (Heenan and Williams, 2013). Biomass of herbivorous reef fishes was calculated at the island-scale from stationary point count surveys conducted alongside the previously described benthic surveys (see Ayotte et al., 2015 for detailed methods). Lastly, we calculated human population density—as a proxy for anthropogenic impacts on nearshore reef systems—scaled to island-level reef area as a more precise estimate of the gradient of human density amongst the islands in this study. Satellite-derived estimates of nearshore chl-a concentration (SNPP VIIRS MSL12, 750-m spatial resolution) and SST (Coral Reef Watch CoralTemp v3.1, 5-km spatial resolution) gridded datasets were accessed using the NOAA OceanWatch ERDAPP platform. All data were extracted from a 10km buffer around the perimeter of each island for an 8-year period preceding the benthic surveys. This time period was selected based on the previously documented timeline of 9–12 years for benthic recovery from climate-induced coral bleaching in the Indo-Pacific (Gilmour et al., 2013; Gouezo et al., 2019) such that any impact from heat stress within this time period will still manifest in the benthic cover data. As chl-a values from shallow waters are unreliable due to shallow-bottom reflectance (Maina et al., 2011), the buffer for extracting chl-a data was masked using the 30m depth contour around each island (Gove et al., 2013). Hence, chl-a values were used as a proxy for ‘ocean productivity’ (sensu Williams et al., 2015)—not nearshore or overall reef productivity—and monthly composites were used to generate a long-term mean, standard deviation (SD), and coefficient of variation [CV]. Past SST conditions were calculated as the maximum monthly mean (MMM) climatology; the mean, SD and CV of monthly SST to capture ‘low-frequency’ variation in SST; and the mean of the daily SST anomaly to capture ‘high-frequency’ variation. The magnitude of heat stress was represented by three metrics: the proportion of degree heating weeks (DHW) exceeding 8°C-weeks when severe bleaching and mortality is likely (Skirving et al., 2020), the maximum monthly SST value over the 8-year period, and maximum daily SST anomaly value. Collinearity amongst these 14 explanatory variables was reduced by excluding variables with correlation coefficients (pearson’s r) > 0.60 and retaining the more ecologically informative variable. For example, latitude was excluded due to positive correlation with CVSST, and negative correlations with CVchl-a. All variables including their data sources are listed in Table S3.
Eight variables were retained for the db-RDA (Table 1, data provided in Table S4). Cover data were square root transformed and the explanatory variables were transformed as needed to linearize the relationships with response variables and then z-score normalized. As variable order will influence significance in db-RDA, each term was evaluated as “type III” or marginal effects (i.e., significance when a term is added to the model after all other terms; Oksanen et al., 2019). Variance partitioning was used to divide the variation in benthic structure among explanatory variables. We then used beta regression to explore how significant variables identified by the db-RDA related to specific components of benthic cover using the betareg package in R with the logit link function (Cribari-Neto and Zeileis, 2010). Beta regression models are better suited for use with continuous proportional data bounded between [0, 1] than general linear models (Douma and Weedon, 2019; Geissinger et al., 2022). Outliers were excluded from beta regressions based on visual inspection of residual, Q-Q, and Cook’s distance plots and evaluated for improved fit by comparison of the precision parameter (φ). Outlier exclusion slightly changed model coefficients but resulted in no changes to which explanatory variables were significant. All data analyses were conducted in R v4.1.2 (R Core Team, 2020).
Results
Live coral cover averaged 14.1% ± 1.6 SE while reef builder cover (coral + CCA) averaged 24.9% ± 2.8 SE among the 34 islands and atolls. Habitation was not significantly correlated with coral, macroalgal, or reef builder cover when adjusting for latitude; however, fleshy algae was significantly higher on inhabited islands (61.7% ± 4.8 SE) compared to uninhabited (53.6% ± 4.6 SE) after adjusting for latitude (ANCOVA: Table 2, Figure 2). Turf drove much of this pattern in fleshy algae cover as macroalgae continued to be uniformly low (<4%) across all islands (Figure 2). For macroalgae, there was a significant interaction between habitation and region, and the data failed to meet assumptions of homogeneity of regression slopes between latitude and the grouped independent variables (Table 2). Hence, the effect of habitation was evaluated separately for each region using a single factor ANCOVA (factor: habitation, covariate: latitude). Habitation was only significant in the PRIA (p = 0.021), with Wake Island (inhabited) having greater macroalgal cover than all the other (uninhabited) islands in the PRIA. Macroalgal cover in the Marianas, American Samoa, and Hawaii was unrelated to habitation (single-factor ANCOVA, p > 0.05).
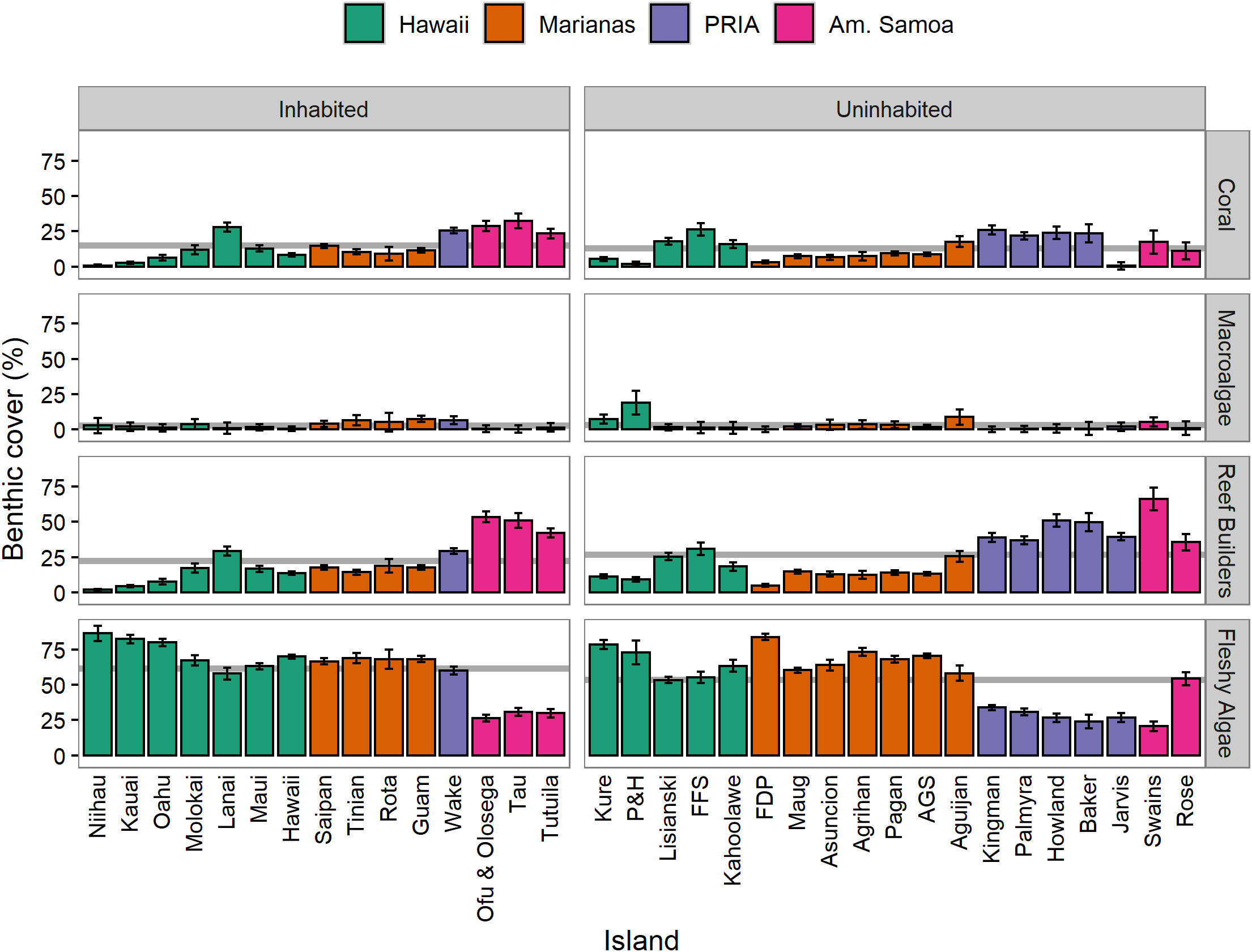
Figure 2 Island-scale weighted means (± SE) of coral, macroalgal, reef builder (coral + CCA) and fleshy algal (macroalgal + turf) percent cover grouped by habitation. Mean values for each benthic cover group by habitation are shown in gray horizontal lines. PRIA = Pacific Remote Islands Area. Abbreviated island names are listed in Table S1.
Benthic community composition did not vary between the inhabited and uninhabited islands (PERMANOVA, Table 3), though significant regional differences persisted between the higher latitude Marianas and Hawaiian archipelagos compared to lower latitude regions of American Samoa and the PRIA (Table 3; Figure 3). More reef builders were present in lower latitude regions of American Samoa and the PRIA, and more fleshy algae were present at the high latitudes of the Marianas and Hawaiian Archipelagos. Both region and habitation met assumptions of homogeneity of dispersion among factor levels (PERMDISP2; F3,30 = 0.92, p = 0.44 and F1,32 = 1.06, p = 0.31, respectively) indicating that variation in benthic community composition was similar between inhabited and uninhabited islands as well as between regions.

Table 3 PERMANOVA results (degrees of freedom, sum of squares, pseudo-F-ratio, and p-value based on permutation) for benthic cover (%) where region and habitation were treated as fixed effects, and latitude (absolute value) was treated as a covariate.
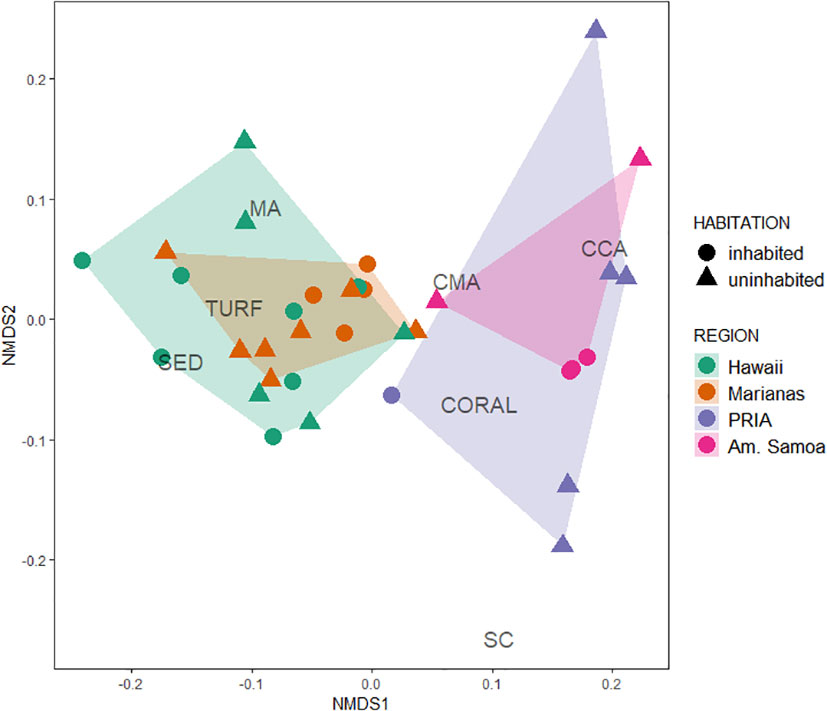
Figure 3 nMDS plot of benthic cover composition among the four regions. Inhabited islands symbolized by circles, uninhabited islands by triangles. CCA, crustose coralline algae; CMA, calcified macroalgae; MA, fleshy macroalgae; SED, sediment/sand; SC, soft coral. PRIA, Pacific Remote Island Area.
Expanding beyond the metrics of habitation, region, and latitude revealed four metrics relating to oceanic productivity, SST variability, and herbivore reef fish biomass as the strongest correlates of benthic community composition (db-RDA, Table 4). Mean chl-a, variation in past SST at both low- and high-frequency time scales (i.e., CVSST and SSTanom, respectively), and herbivore biomass collectively accounted for 25% of the variation (variance partitioning: conditional effects). Again, human habitation measured as population density was not a significant predictor of benthic cover composition. Population density together with metrics of acute heat stress (MaxSST and DHW > 8) and variation in oceanic productivity (CVchl-a) only explained 8% of variation (conditional effects) in benthic cover.
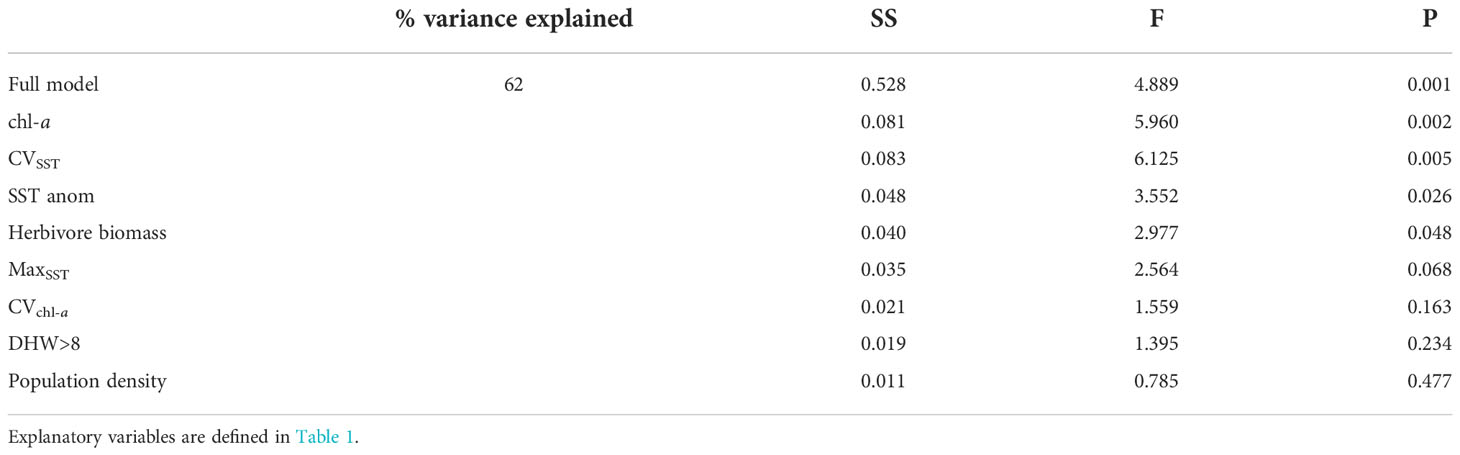
Table 4 db-RDA model outputs (sum of squares, F statistics, and p-value) for the full model including % of variance (adjusted R2) explained.
Beta regression models were then used to assess the direction and significance of the relationship between the variables identified by the db-RDA and specific components of benthic cover (Figure 4, Table S5). Oceanic productivity (chl-a) was a positive correlate of benthic calcifiers with higher coral and CCA cover observed in more productive waters. In contrast, both corals and CCA cover declined at islands that experienced more variability in SST monthly means (CVSST, i.e., low-frequency temperature variability)—though coral cover was positively correlated with the mean daily SST anomaly (SSTanom; i.e., high-frequency temperature variability). Both CCA and coral cover were positively correlated with biomass of herbivorous reef fish. Conversely, turf cover exhibited the opposite pattern. Waters with higher oceanic productivity had lower turf cover, while turf cover increased with greater variability in monthly SST. Lower turf cover was inversely related to both daily SST anomaly and herbivore biomass such that islands with greater swings in daily temperature and more herbivorous fish biomass were associated with less benthic turf cover. Macroalgae was negatively correlated only with herbivore biomass. These beta regression models for benthic cover explained a range of variability in the datasets; the adjusted R2 was greatest for turf (0.68), followed by CCA (0.54), coral (0.49), and lastly macroalgae (0.34).
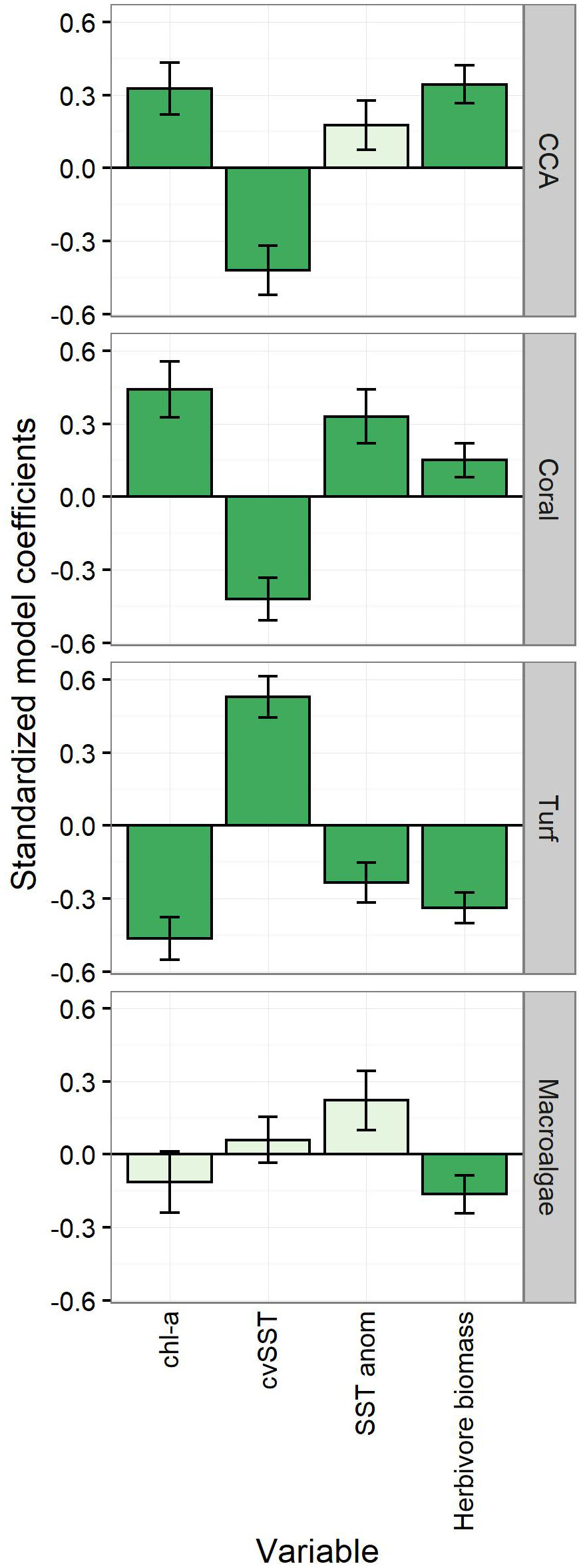
Figure 4 Explanatory variables of benthic cover functional groups and their standardized logit coefficients (± SE) from beta regression analysis. SSTanom captures high frequency (i.e., daily) variation in SST; cvSST captures low frequency variation (i.e., monthly) in SST. Dark green bars were statistically significant (α = 0.05). Outliers removed per benthic group: CCA - Swains; Coral - Jarvis, Pearl and Hermes; Turf - Swains; Macroalgae - Pearl and Hermes.
Discussion
Using data from 56 islands and atolls in the early 2000s, Smith et al. (2016) found that benthic communities at uninhabited islands and atolls in the central Pacific were dominated by reef builders (i.e. scleractinian corals and CCA) and therefore may be more resilient to future global change. Unfortunately, this prediction is not supported by NCRMP survey data collected more than a decade later at a subset of these islands. Reef builders were no more abundant on uninhabited reefs compared to their inhabited counterparts using contemporary data from the U.S.-affiliated islands in the central Pacific. Reef builder cover at uninhabited islands in our dataset averaged 26.9% ± 3.9 SE, compared to 45.18% reported from Smith et al. (2016) using a larger dataset collected nearly two decades prior. Interestingly, reef builder cover at inhabited islands was more comparable between these two studies as Smith reported values of 27.3% compared to our observations of 22.4%. The range in coral cover observed in our dataset (1–32%) was also narrower than the values found by Smith et al. (1–74%). A direct comparison of our dataset to that used by Smith was not undertaken due to differences in survey design over time. The dataset analyzed by Smith was limited by depth (5-15m), sample size (n = 452 sites), and haphazard site selection; in contrast our dataset reflects a more robust sampling effort spanning forereef habitats from 0-30m, with a larger sample size (n = 1,898 sites) collected using a stratified random study design. Furthermore, it is worth noting that not all the islands included in the Smith et al. study (n =56) were resurveyed here (n =34), including several uninhabited Line and Phoenix Islands where coral cover exceeded 60%. By extension, the absence of these coral-rich, uninhabited islands from this analysis may influence our finding of no difference in reef builder cover between inhabited and uninhabited islands. However, a recent study by Fox et al. (2021) reported mean coral cover of <20% in 2018 from three of these uninhabited Phoenix Islands present in the Smith et al. (2016) study. Thus, recent coral cover at uninhabited reefs of the Phoenix Islands seem to align with observed values reported here in the U.S.-affiliated islands and atolls in the central Pacific, suggesting that these reefs are not insulated from coral loss.
In contrast to reef builders, fleshy algae cover was found to be more abundant on inhabited islands in our study—albeit by a narrower margin (8.1% difference in cover) than reported by Smith et al. (2016) nearly two decades earlier (15.8% difference in cover),. Turf cover drives this pattern, greatly exceeding macroalgal cover on central Pacific reefs. Turf dominance has been previously described in the Line Islands and attributed to local human disruption of the reef ecosystem inducing competitive dominance of turfs over corals on inhabited islands (Barott et al., 2012). Yet, we now see evidence of greater turf abundance at uninhabited islands (50.2% cover) compared to values reported by Smith et al. (37.3% cover) in 2016. Though turf can rapidly occupy space immediately following disturbance, CCA appears to outcompete turf when herbivore biomass is high (Fox et al., 2019; Huntington et al., 2022). We did find strong inverse relationship between CCA and turf cover (r = -0.86) supporting this potential shift between CCA and turf; though caution should be applied here given the proportional nature of cover data such that inverse relationships are common due to the type of data, not ecology. Furthermore, these successional pathways may differ by latitude, as turf was more abundant on higher latitude reefs of the Hawaiian and Marianas Archipelagos in the early 2000s (Smith et al., 2016) and remains so in our contemporary dataset. Lastly, turf is a somewhat imprecise term that refers to highly cropped or filamentous algae as well as thick mats. The high abundances of turfs on central Pacific reefs underscore the importance of improving our taxonomic and functional resolution of turfs, as not all species and morphologies perform identical functions on a reef (Connell et al., 2014).
Smith et al. (2016) observed greater variation (i.e., multivariate dispersion) in benthic community composition on uninhabited islands compared to inhabited ones in the central Pacific, suggesting that human presence has a “consistent effect on the reef benthos” causing reef communities to become more similar on inhabited islands. However, we found no difference in multivariate dispersion of benthic cover communities between inhabited and uninhabited islands. We hypothesize that we may be seeing an example of the ‘protection paradox’ at play (sensu Bates et al., 2019) in which remote atolls and islands—where local stressors were presumably minimized—were home to relatively vulnerable benthic taxa or genotypes. In the face of increasing frequency of global stress events, these remote environments are predicted to lose more of the vulnerable taxa and undergo a homogenization towards stress-tolerant species that are more resistant (Côté and Darling, 2010; Darling et al., 2013). Exploring the temporal ‘homogenization’ of benthic reef communities at uninhabited islands over time in the central Pacific and the mechanisms behind such shifts merits further inquiry.
Local and global stressors combined have been shown to result in the decline of corals (Donovan et al., 2021). Hence, remote reefs isolated from local human disturbances are thought to be more resilient to the effects of global climate disturbance (Sandin et al., 2008). However, our results showed that neither coral nor calcifier cover was correlated with human presence, and abundance of individual benthic cover functional groups was unrelated to the density of local human inhabitants. This contrasts with the findings of Smith et al. (2016), where active reef builders were more abundant on uninhabited central Pacific islands and therefore presumably more resilient (though they found coral cover alone to be unrelated to human habitation). Recent studies have found similar results uncoupling benthic community composition—and by extension presumed coral reef resilience—from local human population density, concluding that remote reefs are just as susceptible to degradation caused by climate change as their inhabited counterparts (Bruno and Valdivia, 2016; Baumann et al., 2022). Yet in this study, herbivorous fish biomass (considered a proxy for local anthropogenic fishing pressure) was positively correlated with calcifier cover and negatively correlated with fleshy algal cover. This relationship between herbivore biomass and benthic cover has been well documented in the central Pacific (Heenan and Williams, 2013). This discrepancy suggests that human population density may not be an ideal proxy for local anthropogenic stressors such as reduced grazing pressure from overfishing or land-based pollution known to impact reef health. Or that such local stressors are spatially variable at sub-island scales (Donovan et al., 2021), and therefore poorly represented at broader geographic scales by the island-level human census data employed in this study. Admittedly, factors beyond local fishing pressure, such as reef rugosity, can modulate herbivore biomass (Foo et al., 2021). Yet, simulation tests evaluating the effects of global climate stress, local fishing pressure, land based sources of pollution on coral reef systems in the tropical western Pacific suggest that climate change, not local anthropogenic stressors, will have the largest overall effect on the ratio of calcifying to non-calcifying benthic groups by the middle of this century (Weijerman et al., 2015).
Our analyses add oceanic productivity and SST variability to the list of meaningful predictor of benthic community composition in the central Pacific. Reef calcifiers in the central Pacific respond poorly to greater variability in monthly SST as observed at higher latitude islands in the Hawaiian and Mariana Archipelagos (Figure S1). Corals in the Phoenix Islands have been shown to be highly acclimated to their local temperature regimes and consequently vulnerable to temperature variability associated with thermal stress events (Obura and Mangubhai, 2011). Similarly, Page et al. (2021) found CCA has a reduced ability to maintain photosynthetic rates during anomalous increases in temperature. In contrast, algal turf abundance was positively correlated with low frequency variability in monthly SST (i.e., CVSST). Turfs persist as the dominant benthic group in the northern archipelagos. Thus, corals and CCA may benefit from the relatively stable monthly SSTs that have characterized reef in the lower latitude PRIA and American Samoa islands, while turfs are competitively superior (or opportunistically occupy space) in the NWHI and Northern Mariana Islands where latitude-driven seasonal variability in SST limits calcifier development (Vroom and Braun, 2010). Yet, we also found that turf cover has increased at uninhabited, lower latitude islands of the PRIA and American Samoa—a region where modeling indicates that interannual SST variability in the central Pacific has increased and will continue to do so as climate change fuels greater variability in central Pacific El Niño (Liu et al., 2017). Continued monitoring of reef composition along with variation in SST will be key assessing if calcifier cover is on a sustained downward trajectory in this central Pacific region.
Interestingly, the frequency of temperature variation determined the impact on coral cover. High-frequency daily variation in SST (i.e., SSTanom) was positively associated with coral cover in our analyses. A similar finding was observed by Safaie et al. (2018) in a global analysis leading the authors to conclude that high-frequency temperature variability (i.e., daily temperature range) reduces risk of coral bleaching. Daily variation in SST is uncorrelated with latitude and may reflect oceanographic processes like internal waves or current-driven upwelling (Wyatt et al., 2020). Similar observations were made in the central Pacific where corals subject to higher frequency variations in temperature (e.g., CV of weekly SST) were better able to resist bleaching during a natural heat stress event (Donner and Carilli, 2019), indicating that acclimation to temperature variability is occurring in this region (Palumbi et al., 2014; Couch et al., 2017; Fox et al., 2021). Hence, the reefs of American Samoa, Lisianski and French Frigate Shoal in the NWHI, and Wake Atoll that experience high frequency temperature variability and possess high coral cover (Figure S1) may be more resistant to the threat of warming ocean temperatures and key to regional conservation.
In a changing climate, the persistence of reef builders may also be linked to patterns of oceanic primary production. In this study, higher coral and CCA cover was observed in more productive waters. Increasing evidence shows reef calcifiers (including corals and calcifying algae) are capable of capitalizing on higher productivity in oligotrophic waters—be it overall or in episodic pulses from deep-water upwelling or terrestrial subsidies—to increase their nutrition and enhance net calcification (Teichberg et al., 2013; Benkwitt et al., 2019; Kealoha et al., 2019; Radice et al., 2019). For mixotrophic corals, species-specific trophic strategies such as heterotrophic feeding can assist some taxa to meet nutritional needs under sustained ocean warming (Hughes et al., 2018; Radice et al., 2019). Fox et al. (2018) showed that one coral species common across our study regions, Pocillopora meandrina, can actually increase its reliance on heterotrophy when more food is available (based on satellite-derived estimates of chl-a). Feeding more in times of plenty may benefit survival during subsequent periods of heat stress. For example, corals with superior energy reserves (measured as tissue protein and lipid levels) are better equipped to resist thermal bleaching. Corals can achieve these superior energy reserves by possessing ‘optimal’ densities of zooxanthellae supported by higher seawater chl-a (Wooldridge, 2020). However, the relationship between productivity and coral resistance is not linear, but rather unimodal. Seawater chl-a monthly averages exceeding 0.45μg L-1 have been linked to zooxanthellae densities that exceed the optimal range and correlated with lower resistance to thermal stress (Wooldridge, 2020). The chl-a values reported in this study are well below this threshold such that greater chl-a around an island or atoll may enhance zooxanthellae densities within the coral and/or enhance heterotrophic feeding, enabling them to build tissue energy reserves that confer bleaching resistance in times of heat stress. While we did not explicitly analyze metrics of remotely-sensed turbidity in this study, more productive waters are often more turbid. Indeed, our metric of long-term mean chl-a was closely correlated with remotely-sensed estimates of light attenuation extracted over the same spatial and temporal extent (i.e. kd490; pearson’s r = 0.71). Hence, greater oceanic productivity may also influence turbidity, which has been shown to moderate coral bleaching by reducing light stress (Cacciapaglia and van Woesik, 2016). However, it is worth noting that for 32 of the 34 islands in our dataset, mean Kd490 values fall below the “moderating turbidity” range of 0.080 and 0.127 defined by Sully and van Woesik (2020), which may minimize the moderating effect (Table S6).
Unlike most other reef studies which have limited spatial sampling, this analysis leveraged a robust number of NCRMP survey sites (1,898) across 34 islands spanning a vast spatial gradient to investigate environmental drivers of benthic community composition. NCRMP’s stratified random dataset provides exceptional spatial comprehensiveness to characterize the shallow (0–30m) reef community at a given island. Our results show that, at island scales, human habitation is no longer a primary correlate of reef builder cover on central Pacific reefs. Rather, for contemporary reefs in this region, oceanic productivity, high-frequency variation in SST, and herbivore biomass support reef builder abundance. Neither metric of acute thermal heat stress (i.e., annual DHW >8, MaxSST) were correlated with coral cover at the island-scale. However, DHW is a problematic metric for accurately reflecting cumulative heat stress in the PRIA where ENSO events typically occur during December–January and therefore yield high inter-annual variability (Mollica et al., 2019). Furthermore, DHW derived from SST do not always accurately capture temperature at subsurface depths (Venegas et al., 2019), and therefore may not be a robust estimate of actual thermal stress experienced by corals at depth. Along the same lines, a recent study from American Samoa found that DHWs alone did not accurately predict bleaching; instead, bleaching was more accurately predicted by in situ-corrected DHW in combination with other in situ temperature metrics including daily variability (Smith et al., 2022). Admittedly, sub-island scale analyses may show more spatially nuanced responses to local factors, including in situ measures of heat stress. Yet, contemporary time series of SST and ocean productivity are more readily available and spatially comprehensive than datasets on local stressors such as sedimentation, fishing intensity, and pollution—giving us greater insight into global stressors than local ones (Bruno and Valdivia, 2016).
Stating that remote reefs are as susceptible to degradation caused by climate change as their inhabited counterparts does not equate to saying local human impacts are not important factors that influence reef health. Case in point, herbivore biomass remains a key correlate of all the benthic cover groups analyzed in this study. Hence, we are not advocating for abandoning management measures to reduce local stressors in the central Pacific. Indeed, management actions such as coral restoration that enhance reef rugosity or reduce commercial fishing levels can be effective in supporting higher herbivore biomass (Foo et al., 2021). Rather we are highlighting the relationships between gradients in oceanographic metrics and benthic reef community structure that have not explicitly been shown for this broad geographic region in the central Pacific. We are also emphasizing the need for spatially comprehensive, human impact metrics that move beyond census data and can be scaled to the same extent as remotely sensed metrics so that we can compare like-to-like. Lastly, this analysis focused solely on benthic cover—a widely used metric shown to respond to highlighting the relationships between gradients in oceanographic metrics that have not explicitly been shown for this broad geographic region in the central Pacific disturbances on the decadal timescale. Yet, benthic cover is coarse and does not provide much information about processes governing reef resistance and resilience. We encourage future analyses to incorporate increased taxonomic resolution in addition to colony density and demography to further our understanding how reef assembly in the central Pacific is proceeding in a changing ocean.
Data availability statement
Publicly available datasets were analyzed in this study. This data can be found here: doi.org/10.7289/v52v2dfw, doi.org/10.7289/v5js9nr4, doi.org/10.7289/v5154fbh, doi.org/10.7289/v57m0673 via the National Centers for Environmental Information.
Author contributions
BH and BV-A designed the work and conducted the analyses. CC and MA contributed data to the analyses. BH, BV-A, CC, HB wrote the manuscript. All authors contributed to the article and approved the submitted version.
Funding
This work was funded by NOAA’s Coral Reef Conservation Program, project # 31282.
Conflict of interest
The authors declare that the research was conducted in the absence of any commercial or financial relationships that could be construed as a potential conflict of interest.
Publisher’s note
All claims expressed in this article are solely those of the authors and do not necessarily represent those of their affiliated organizations, or those of the publisher, the editors and the reviewers. Any product that may be evaluated in this article, or claim that may be made by its manufacturer, is not guaranteed or endorsed by the publisher.
Supplementary material
The Supplementary Material for this article can be found online at: https://www.frontiersin.org/articles/10.3389/fmars.2022.1075972/full#supplementary-material
References
Anderson M. J. (2006). Distance-based tests for homogeneity of multivariate dispersions. Biometrics 62, 245–253. doi: 10.1111/j.1541-0420.2005.00440.x
Ayotte P., McCoy K., Heenan A., Williams I., Zamzow J. (2015). Coral reef ecosystem division standard operating procedures: data collection for rapid ecological assessment fish surveys. Honolulu, HI, USA. Pacific Islands Fisheries Science Center Administrative Report H-15-07 33.
Barkley H. C., Cohen A. L., Mollica N. R., Brainard R. E., Rivera H. E., DeCarlo T. M., et al. (2018). Repeat bleaching of a central pacific coral reef over the past six decades, (1960–2016). Commun. Biol. 1, 177. doi: 10.1038/s42003-018-0183-7
Barott K., Williams G., Vermeij M., Harris J., Smith J., Rohwer F., et al. (2012). Natural history of coral–algae competition across a gradient of human activity in the line islands. Mar. Ecol. Prog. Ser. 460, 1–12. doi: 10.3354/meps09874
Bates A. E., Cooke R. S. C., Duncan M. I., Edgar G. J., Bruno J. F., Benedetti-Cecchi L., et al. (2019). Climate resilience in marine protected areas and the ‘Protection paradox. Biol. Conserv. 236, 305–314. doi: 10.1016/j.biocon.2019.05.005
Baumann J. H., Zhao L. Z., Stier A. C., Bruno J. F. (2022). Remoteness does not enhance coral reef resilience. Glob. Change Biol. 28, 417–428. doi: 10.1111/gcb.15904
Benkwitt C. E., Wilson S. K., Graham N. A. J. (2019). Seabird nutrient subsidies alter patterns of algal abundance and fish biomass on coral reefs following a bleaching event. Glob. Change Biol. 25, 2619–2632. doi: 10.1111/gcb.14643
Bruno J. F., Valdivia A. (2016). Coral reef degradation is not correlated with local human population density. Sci. Rep. 6, 29778. doi: 10.1038/srep29778
Cacciapaglia C., van Woesik R. (2016). Climate-change refugia: shading reef corals by turbidity. Glob. Change Biol. 22, 1145–1154. doi: 10.1111/gcb.13166
Carilli J., Donner S. D., Hartmann A. C. (2012). Historical temperature variability affects coral response to heat stress. PloS One 7, e34418. doi: 10.1371/journal.pone.0034418
Carilli J. E., Hartmann A. C., Heron S. F., Pandolfi J. M., Cobb K., Sayani H., et al. (2017). Porites coral response to an oceanographic and human impact gradient in the line islands. Limnol. Oceanogr. 62, 2850–2863. doi: 10.1002/lno.10670
Carilli J. E., Norris R. D., Black B. A., Walsh S. M., McField M. (2009). Local stressors reduce coral resilience to bleaching. PloS One 4 (7), e6324. doi: 10.1371/journal.pone.0006324
Connell S., Foster M., Airoldi L. (2014). What are algal turfs? towards a better description of turfs. Mar. Ecol. Prog. Ser. 495, 299–307. doi: 10.3354/meps10513
Côté I. M., Darling E. S. (2010). Rethinking ecosystem resilience in the face of climate change. PloS Biol. 8, e1000438. doi: 10.1371/journal.pbio.1000438
Couch C. S., Burns J. H. R., Liu G., Steward K., Gutlay T. N., Kenyon J., et al. (2017). Mass coral bleaching due to unprecedented marine heatwave in papahānaumokuākea marine national monument (Northwestern Hawaiian islands). PloS One 12, e0185121. doi: 10.1371/journal.pone.0185121
Cribari-Neto F., Zeileis A. (2010). Beta regression in r. J. Stat. Softw. 34, 1–24. doi: 10.18637/jss.v034.i02
Darling E. S., McClanahan T. R., Côté I. M. (2013). Life histories predict coral community disassembly under multiple stressors. Glob. Change Biol. 19, 1930–1940. doi: 10.1111/gcb.12191
Donner S. D., Carilli J. (2019). Resilience of central pacific reefs subject to frequent heat stress and human disturbance. Sci. Rep. 9, 1–13. doi: 10.1038/s41598-019-40150-3
Donovan M. K., Burkepile D. E., Kratochwill C., Shlesinger T., Sully S., Oliver T. A., et al. (2021). Local conditions magnify coral loss after marine heatwaves. Science 372, 977–980. doi: 10.1126/science.abd9464
Douma J. C., Weedon J. T. (2019). Analysing continuous proportions in ecology and evolution: A practical introduction to beta and dirichlet regression. Methods Ecol. Evol. 10, 1412–1430. doi: 10.1111/2041-210X.13234
Foo S. A., Walsh W. J., Lecky J., Marcoux S., Asner G. P. (2021). Impacts of pollution, fishing pressure, and reef rugosity on resource fish biomass in West Hawaii. Ecol. Appl. 31 (1), e02213. doi: 10.1002/eap.2213
Fox M. D., Carter A. L., Edwards C. B., Takeshita Y., Johnson M. D., Petrovic V., et al. (2019). Limited coral mortality following acute thermal stress and widespread bleaching on Palmyra atoll, central pacific. Coral Reefs 38, 701–712. doi: 10.1007/s00338-019-01796-7
Fox M. D., Cohen A. L., Rotjan R. D., Mangubhai S., Sandin S. A., Smith J. E., et al. (2021). Increasing coral reef resilience through successive marine heatwaves. Geophys. Res. Lett. 48, e2021GL094128. doi: 10.1029/2021GL094128
Fox M. D., Williams G. J., Johnson M. D., Radice V. Z., Zgliczynski B. J., Kelly E. L. A., et al. (2018). Gradients in primary production predict trophic strategies of mixotrophic corals across spatial scales. Curr. Biol. 28, 3355–3363.e4. doi: 10.1016/j.cub.2018.08.057
Geissinger E. A., Khoo C. L. L., Richmond I. C., Faulkner S. J. M., Schneider D. C. (2022). A case for beta regression in the natural sciences. Ecosphere 13 (2), e3940. doi: 10.1002/ecs2.3940
Gilmour J. P., Smith L. D., Heyward A. J., Baird A. H., Pratchett M. S. (2013). Recovery of an isolated coral reef system following severe disturbance. Science 340, 69–71. doi: 10.1126/science.1232310
Gouezo M., Golbuu Y., Fabricius K., Olsudong D., Mereb G., Nestor V., et al. (2019). Drivers of recovery and reassembly of coral reef communities. Proc. R Soc. B Biol. Sci. 286, 20182908. doi: 10.1098/rspb.2018.2908
Gove J. M., Williams G. J., McManus M. A., Heron S. F., Sandin S. A., Vetter O. J., et al. (2013). Quantifying climatological ranges and anomalies for pacific coral reef ecosystems. PloS One 8 (4), e61974. doi: 10.1371/journal.pone.0061974
Grottoli A. G., Rodrigues L. J., Palardy J. E. (2006). Heterotrophic plasticity and resilience in bleached corals. Nature 440, 1186–1189. doi: 10.1038/nature04565
Heenan A., Williams I. D. (2013). Monitoring herbivorous fishes as indicators of coral reef resilience in American Samoa. PloS One 8 (11), e79604. doi: 10.1371/journal.pone.0079604
Hughes T. P., Kerry J. T., Baird A. H., Connolly S. R., Dietzel A., Eakin C. M., et al. (2018). Global warming transforms coral reef assemblages. Nature 556, 492–496. doi: 10.1038/s41586-018-0041-2
Huntington B., Weible R., Halperin A., Winston M., McCoy K., Amir C., et al. (2022). Early successional trajectory of benthic community in an uninhabited reef system three years after mass coral bleaching. Coral Reefs 41, 1087–1096. doi: 10.1007/s00338-022-02246-7
Kealoha A. K., Shamberger K. E. F., Reid E. C., Davis K. A., Lentz S. J., Brainard R. E., et al. (2019). Heterotrophy of oceanic particulate organic matter elevates net ecosystem calcification. Geophys. Res. Lett. 46, 9851–9860. doi: 10.1029/2019GL083726
Liu Y., Cobb K. M., Song H., Li Q., Li C.-Y., Nakatsuka T., et al. (2017). Recent enhancement of central pacific El niño variability relative to last eight centuries. Nat. Commun. 8, 15386. doi: 10.1038/ncomms15386
Lozada-Misa P., Schumacher B. D., Vargas-Ángel B. (2017). Analysis of benthic survey images via CoralNet: A summary of standard operating procedures and guidelines. Honolulu, HI, USA. Pacific Islands Fisheries Science Center Administrative Report H-17-02. 175.
Maina J., McClanahan T. R., Venus V., Ateweberhan M., Madin J. (2011). Global gradients of coral exposure to environmental stresses and implications for local management. PloS One 6 (8), e23064. doi: 10.1371/journal.pone.0023064
Mumby P. J., Dahlgren C. P., Harborne A. R., Kappel C. V., Micheli F., Brumbaugh D. R., et al. (2006). Fishing, trophic cascades, and the process of grazing on coral reefs. Science 311, 98. doi: 10.1126/science.1121129
Obura D., Mangubhai S. (2011). Coral mortality associated with thermal fluctuations in the phoenix islands 2002–2005. Coral Reefs 30, 607–619. doi: 10.1007/s00338-011-0741-7
Oksanen J., Blanchet F. G., Friendly M., Kindt R., Legendre P., McGlinn D., et al. (2019). Vegan: Community ecology package. (CRAN). Available at: https://CRAN.R-project.org/package=vegan.
Page T. M., Bergstrom E., Diaz-Pulido G. (2021). Acclimation history of elevated temperature reduces the tolerance of coralline algae to additional acute thermal stress. Front. Mar. Sci. 8, 660196. doi: 10.3389/fmars.2021.660196
Palumbi S. R., Barshis D. J., Traylor-Knowles N., Bay R. A. (2014). Mechanisms of reef coral resistance to future climate change. Science 344 (6186), 895–898. doi: 10.1126/science.1251336
Radice V. Z., Hoegh-Guldberg O., Fry B., Fox M. D., Dove S. G. (2019). Upwelling as the major source of nitrogen for shallow and deep reef-building corals across an oceanic atoll system. Funct. Ecol. 33, 1120–1134. doi: 10.1111/1365-2435.13314
R Core Team (2020). R: A language and environment for statistical computing (Vienna, Austria: R Foundation for Statistical Computing). Available at: https://www.R-project.org/.
Robinson J. P. W., Williams I. D., Yeager L. A., McPherson J. M., Clark J., Oliver T. A., et al. (2018). Environmental conditions and herbivore biomass determine coral reef benthic community composition: implications for quantitative baselines. Coral Reefs 37, 1157–1168. doi: 10.1007/s00338-018-01737-w
Safaie A., Silbiger N. J., McClanahan T. R., Pawlak G., Barshis D. J., Hench J. L., et al. (2018). High frequency temperature variability reduces the risk of coral bleaching. Nat. Commun. 9, 1671. doi: 10.1038/s41467-018-04074-2
Sandin S. A., Smith J. E., DeMartini E. E., Dinsdale E. A., Donner S. D., Friedlander A. M., et al. (2008). Baselines and degradation of coral reefs in the northern line islands. PloS One 3 (2), e1548. doi: 10.1371/journal.pone.0001548
Skirving W., Marsh B., de la Cour J., Liu G., Harris A., Maturi E., et al. (2020). CoralTemp and the coral reef watch coral bleaching heat stress product suite version 3.1. Remote Sens. 12, 3856. doi: 10.3390/rs12233856
Smith J. E., Brainard R., Carter A., Grillo S., Edwards C., Harris J., et al. (2016). Re-evaluating the health of coral reef communities: Baselines and evidence for human impacts across the central pacific. Proc. R Soc. B Biol. Sci. 283, 20151985. doi: 10.1098/rspb.2015.1985
Smith J., Halperin A., Barkley H. (2022). A “perfect storm” of cumulative and acute heat stress, and a warming trend, lead to bleaching events in tutuila, American Samoa. NOAA Tech. Memo. 129, 1–51. doi: 10.25923/yphg-pq04
Stuart-Smith R. D., Brown C. J., Ceccarelli D. M., Edgar G. J. (2018). Ecosystem restructuring along the great barrier reef following mass coral bleaching. Nature 560, 92–96. doi: 10.1038/s41586-018-0359-9
Sully S., Burkepile D. E., Donovan M. K., Hidgson G, van Woesik R. (2019). A global analysis of coral bleaching over the past two decades. Nat. Commun. 10, 1264. doi: 10.1038/s41467-019-09238-2
Sully S., van Woesik R. (2020). Turbid reefs moderate coral bleaching under climate-related temperature stress. Glob. Change Biol. 26, 1367–1373. doi: 10.1111/gcb.14948
Teichberg M., Fricke A., Bischof K. (2013). Increased physiological performance of the calcifying green macroalga Halimeda opuntia in response to experimental nutrient enrichment on a Caribbean coral reef. Aquat. Bot. 104, 25–33. doi: 10.1016/j.aquabot.2012.09.010
Vargas-Ángel B., Huntington B., Brainard R. E., Venegas R., Oliver T., Barkley H., et al. (2019). El Niño-associated catastrophic coral mortality at Jarvis island, central equatorial pacific. Coral Reefs 38, 731–741. doi: 10.1007/s00338-019-01838-0
Venegas R. M., Oliver T., Liu G., Heron S. F., Clark S. J., Pomeroy N., et al. (2019). The rarity of depth refugia from coral bleaching heat stress in the Western and central pacific islands. Sci. Rep. 9, 19710. doi: 10.1038/s41598-019-56232-1
Vroom P. S., Braun C. L. (2010). Benthic composition of a healthy subtropical reef: Baseline species-level cover, with an emphasis on algae, in the northwestern Hawaiian islands. PloS One 5 (3), e9733. doi: 10.1371/journal.pone.0009733
Weijerman M., Fulton E. A., Kaplan I. C., Gorton R., Leemans R., Mooij W. M., et al. (2015). An integrated coral reef ecosystem model to support resource management under a changing climate. PloS One 10, e0144165. doi: 10.1371/journal.pone.0144165
Williams I. D., Baum J. K., Heenan A., Hanson K. M., Nadon M. O., Brainard R. E. (2015). Human, oceanographic and habitat drivers of central and western pacific coral reef fish assemblages. PloS One 10, e0120516. doi: 10.1371/journal.pone.0120516
Winston M., Oliver T., Couch C., Donovan M. K., Asner G. P., Conklin E., et al. (2022). Coral taxonomy and local stressors drive bleaching prevalence across the Hawaiian Archipelago in 2019. PloS One 17 (9), e0269068. doi: 10.1371/journal.pone.0269068
Wooldridge S. A. (2020). Excess seawater nutrients, enlarged algal symbiont densities and bleaching sensitive reef locations: Identifying thresholds of concern for the great barrier reef, Australia. Mar. pollut. Bull. 152, 107667. doi: 10.1016/j.marpolbul.2016.04.054
Keywords: oceanography, climate change, reef monitoring, U.S. Pacific, global stressors, herbivore biomass, chlorophyll-a, population density
Citation: Huntington B, Vargas-Ángel B, Couch CS, Barkley HC and Abecassis M (2022) Oceanic productivity and high-frequency temperature variability—not human habitation—supports calcifier abundance on central Pacific coral reefs. Front. Mar. Sci. 9:1075972. doi: 10.3389/fmars.2022.1075972
Received: 21 October 2022; Accepted: 30 November 2022;
Published: 16 December 2022.
Edited by:
William F. Precht, Dial Cordy and Associates, Inc., United StatesReviewed by:
Courtney Cox, Barefoot Ocean, United StatesLauren T. Toth, United States Geological Survey (USGS), United States
Copyright © 2022 Huntington, Vargas-Ángel, Couch, Barkley and Abecassis. This is an open-access article distributed under the terms of the Creative Commons Attribution License (CC BY). The use, distribution or reproduction in other forums is permitted, provided the original author(s) and the copyright owner(s) are credited and that the original publication in this journal is cited, in accordance with accepted academic practice. No use, distribution or reproduction is permitted which does not comply with these terms.
*Correspondence: Brittany Huntington, QnJpdHRhbnkuaHVudGluZ3RvbkBub2FhLmdvdg==