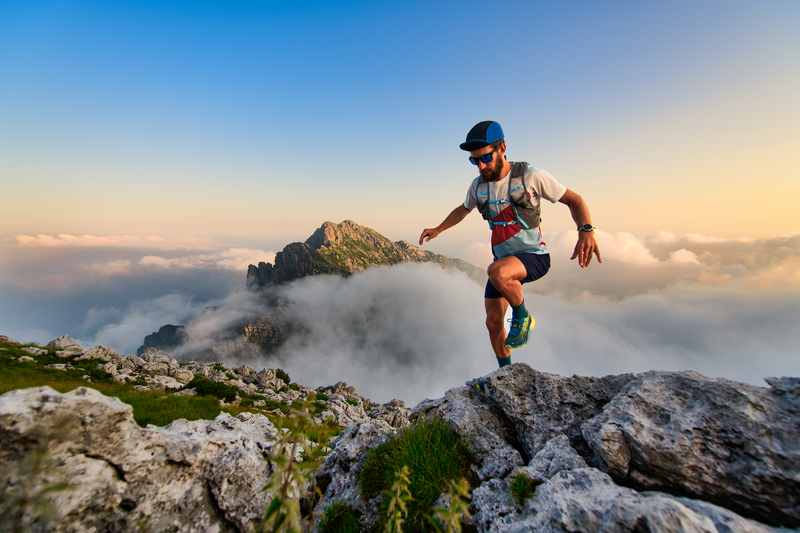
95% of researchers rate our articles as excellent or good
Learn more about the work of our research integrity team to safeguard the quality of each article we publish.
Find out more
ORIGINAL RESEARCH article
Front. Mar. Sci. , 15 December 2022
Sec. Marine Biology
Volume 9 - 2022 | https://doi.org/10.3389/fmars.2022.1070290
This article is part of the Research Topic Marine Invertebrates and Sound View all 12 articles
Anthropogenic noise is now a prominent pollutant increasing in both terrestrial and marine environments. In the ocean, proliferating offshore windfarms, a key renewable energy source, are a prominent noise concern, as their pile driving construction is among the most intense anthropogenic sound sources. Yet, across taxa, there is little information of pile driving noise impacts on organismal fine-scale movement despite its key link to individual fitness. Here, we experimentally quantified the swimming behavior of an abundant squid species (Doryteuthis pealeii) of vital commercial and ecological importance in response to in situ pile driving activity on multiple temporal and spatial scales (thus exposed to differing received levels, or noise-doses). Pile driving induced energetically costly alarm-jetting behaviors in most (69%) individuals at received sound levels (in zero to peak) of 112-123 dB re 1 µm s-2, levels similar to those measured at the kilometer scale from some wind farm construction areas. No responses were found at a comparison site with lower received sound levels. Persistence of swimming pattern changes during noise-induced alarm responses, a key metric addressing energetic effects, lasted up to 14 s and were significantly shorter in duration than similar movement changes caused by natural conspecific interactions. Despite observing dramatic behavioral changes in response to initial pile driving noise, there was no evidence of gait changes over an experiment day. These results demonstrate that pile driving disrupts squid fine-scale movements, but impacts are short-lived suggesting that offshore windfarm construction may minimally impact the energetics of this ecologically key taxon. However, further work is needed to assess potential behavioral and physiological impacts at higher noise levels.
There is a global investment in offshore wind (OSW) infrastructure as many countries increasingly prioritize renewable energies over fossil fuels (Gielen et al., 2019). The increased human presence in the ocean poses challenges to marine life since the pile driving noise emitted during OSW construction has been shown to cause physical damage (Halvorsen et al., 2012), sensory harm (Kastelein et al., 2016), and behavioral changes (Jones et al., 2020) to a myriad of marine taxa. Consequently, anthropogenic noise is recognized as a global pollutant of paramount concern (Halfwerk et al., 2011; Kunc et al., 2014; Duarte et al., 2021). Noise-induced behavioral changes can have direct fitness consequences, and the spatial extent is likely greater than that of noise-induced physical and physiological harm (Popper et al., 2022). However, movement responses are rarely quantified. Fine behavioral changes are difficult to measure in marine environments where animals are largely in accessible, leading to key knowledge gaps on the effects of noise on behaviors that can influence individual fitness.
Much of the existing research on noise-induced behavioral changes has focused upon large marine mammals, and to some extent fishes (Miller et al., 2000; Southall et al., 2007; Miller et al., 2012; Popper and Hawkins, 2019). There is scant data on marine invertebrates such as cephalopods. This is a surprising fact considering their central position in many ocean food webs (Clarke, 1996) and their high commercial value exceeding $1 billion USD per year worldwide (Hunsicker et al., 2010). Cephalopods have been shown to detect sounds within the same frequency range (<500 Hz) as pile driving noise, indicating a likely susceptibility to adverse effects of noise (Mooney et al., 2010; Mooney et al., 2020). Indeed, recent laboratory studies showed that solitary longfin squid (Doryteuthis pealeii), an important U.S. fishery taxon, exhibit alarm responses to pile driving playbacks (Jones et al., 2020; Jones et al., 2021). However these studies used solitary squid in tanks, which makes behavioral inferencing challenging since D. pealeii is an aggregating species and the acoustic field differed from field conditions (Birkett and Newton-Fisher, 2011; Jones et al., 2019). One field study examined caged squid (Sepioteuthis australis) behavioral responses to seismic air-gun surveys (Fewtrell and McCauley, 2012). The authors found that both the proportion of alarm responses (e.g., escape jetting) and swimming speed were positively correlated with received noise levels. Nonetheless, this preliminary study only assessed movement qualitatively, leading to important questions regarding the ecological consequences, energetics, and duration of the observed behavioral changes.
Most bioacoustic studies have not measured the duration of noise-induced behavioral changes (but see Miller et al., 2012) despite being a key consideration for policy makers (Finneran et al., 2017; Southall et al., 2021). Measuring the duration of noised-induced behavioral impacts is critical because it is inherently linked to impact severity and persistence of effect. For example, the energetic cost incurred from a transient increase in acceleration is less severe than a prolonged heightened acceleration state if an individual does not habituate or desensitize to a noise stimulus (Southall et al., 2007). The few studies measuring disturbance durations in aquatic animals have been restricted to large vertebrates capable of carrying motion sensor tags (Miller et al., 2012). For many marine species, quantifying individual movement is difficult, particularly over time scales comparable to pile driving operations; yet such data are needed to quantify behavioral changes and energetic costs. As a result, most studies on smaller and more abundant animals are conducted in tanks, providing key data but limiting the knowledge that can be applicable to actual noise exposures in field settings. New tools and methods are thus needed to accurately describe and quantify noise-induced behavioral changes, especially in more real-world conditions (Popper et al., 2022).
To date, there has been no field study quantifying the movement behavior of cephalopods, or any invertebrate, during real-time pile driving construction. Given that construction is imminent and considering the spatial overlap of cephalopod fisheries and planned OSW development (Figure 1), there is an urgent need to experimentally examine whether commercially-important cephalopods alter movement behaviors during pile-driving noise exposure, and if so, quantify how long those changes persist. In this context, our present aim was to quantitively examine the fine-scale swimming movements and kinematics of D. pealeii during field-based pile driving activities to assess potential ecological and energetic consequences of noise exposure. We utilized high-resolution movement sensors to measure individual-level swimming kinematics at sub-second to hourly temporal resolutions and at multiple spatial scales during the two main types of piling installation: continuous vibratory and impulsive impact hammering. Both installation methods are known to produce intense sounds, but the characteristics are vastly different (Amaral et al., 2020; Jézéquel et al., 2022). We then assessed the probability of squid changing their movement behavior associated with specific received noise levels, characterized the observed behavioral changes, and measured the durations of those alarm behaviors. These anthropogenically-induced alarm responses were then compared to natural swimming movements and gait disruptions observed throughout the course of quiet, control days to evaluate the potential biological and energetic implications of the noise-induced stress. To address these questions, we developed a new approach to quantify the movement of cephalopods that can be used to address similar questions for other species more broadly.
Figure 1 Future offshore windfarm construction largely overlaps with areas of high cephalopods harvest. The global map depicts individual OSW projects (dots) at four stages of development as well as the extend of cephalopod harvest within a country’s ocean governance area (The Wind Power (www.thewindpower.net), Food and Agriculture Organization).
Squid used in the present study were collected from Vineyard Sound, MA (41.22 N; 70.47 W). Animals were hand-selected and only animals without visible lesions and muscular damage were chosen for experimental use. Prior to the experiment, squid were held in multiple 1.2-m diameter cylindrical tanks constantly supplied with ambient, local seawater from the study area. Squid were fed mummichogs (Fundulus heteroclitus) and grass shrimps (Palaemonetes spp.) daily. Experimental squid were kept in holding tanks for no longer than three days before trials started, and new squid were used each experiment day. This study was carried out in accordance with the principles of the Basel Declaration and recommendations and approval of the Woods Hole Oceanographic Institution’s (WHOI’s) Institutional Animal Care and Use Committee scientific protocol to TAM.
Pile driving was conducted for 11 days in September 2021 off the WHOI’s dock (Figures 2A, B). At the start of each pile driving day a cylindrical steel pile (length: 10 m, diameter: 0.3 m, wall thickness: 0.02 m) was positioned into the sediment using a vibratory hammer (VH, weight: 212 kg, H&M model 135) at 1150 blows per minute. Squid were then introduced into cages (see below for details) and given 15 minutes to acclimate. Exposures began as (1) a steel impact hammer (IH, weight: 1500 kg) was dropped at 1.2 m height at a rate of 8 -12 strikes per minute until the bottom edge of the steel pile was approximately 5 m into the substrate, taking (mean ± standard deviation) 14.9 ± 0.47 min. (2) The VH was then used to pull the pile out of the substrate and to reposition the pile in an adjacent location for another round of impact hammering. This process was repeated five times per experiment day, which lasted for three to four hours.
Figure 2 The experimental setup including a (A) map of the two sites: near (2-8 m) and far (50 m). The yellow star denotes the pile driving location, while the shaded red regions are the position of squid cages. The northern and western boundaries around the pile driving were solid sea walls. There were no physical barriers between the noise source and squid cages apart from a series of 0.3 m diameter piles supporting the dock slips. (B) Drone images during both impact pile driving. (C, D) Video footage from an experiment showing a focal tagged squid schooling with conspecifics.
To assess potential dose-dependent responses, squid were monitored at two different distances from the pile (near site: within 8 m, far site: 50 m; received levels noted below). The exact distance from the noise source varied slightly because consecutive piles could not be driven in the exact same locations. Squid were placed in 1.5 m3 cages constructed using a polyvinyl chloride frame covered with 1.5 cm knotless polyester mesh netting (Figures 2C, D). Each cage contained 4-7 squid of mixed sexes to represent wild aggregations (Shashar and Hanlon, 2013). Two underwater cameras (GoPro Hero 7 Black, San Mateo, CA) were placed in the cages for visual observations. Cages were lowered roughly 5 m and hovered 0.5 m above a sandy substrate. The largest squid (male) in each cage was affixed with a modified ITAG, a biologging tag designed for soft-bodied animals (Mooney et al., 2015; Fannjiang et al., 2019; Cones et al., 2022). The ITAG was used to measure fine-scale swimming kinematics during noise exposure and control periods (see Section 2.3). The analysis focused on the swimming behavior of the tagged squid. Hence, a typical squid group consisted of one large, tagged male (dorsal mantle length (DML): 25.2 ± 2.6 cm) associated with smaller untagged squid (DML: 16.3 ± 2.5 cm).
Control experiments (n=7) were conducted using the same methods, but without pile driving noise exposure. To compare metrics between the two experiment types, noise exposure time periods from experiment days were randomly assigned to control experiments.
ITAGs were used to measure squid movement dynamics. The sensor package was small (length: 7 cm, width: 3 cm, height: 1 cm) and was affixed using surgical sutures (Mooney et al., 2015; Flaspohler et al., 2019; Cones et al., 2022). Additionally, ITAGs were neutrally buoyant, hydrodynamic, and focal tagged squid exhibited normal swimming and schooling behaviors with other conspecifics. ITAGs contain an inertial measurement unit (IMU) which measures acceleration, magnetic field strength, and angular velocity. These high-resolution (100 Hz sampling rate) accelerometers allowed for the estimation of overall dynamic body acceleration (ODBA), a widely used metric to quantify behavior (Zhang et al., 2018) and estimate energetic cost (Wilson et al., 2006; Halsey et al., 2009). The ITAG IMU was used to measure two swimming gaits: jet propulsion and finning.
Jet propulsion is pulsatile and entails the intake of water into the mantle cavity and its expulsion through a flexible funnel (Bartol et al., 2001). Intense jet propulsion events are high acceleration movements employed in response to predators or during conspecific interactions, but is also the common response of squid to recorded pile driving noise (Wells and O’Dor, 1991; Hanlon et al., 2002; Jones et al., 2020). The jetting gait was quantified using similar methods described in detail in previous studies (Flaspohler et al., 2019; Cones et al., 2022). In brevity, a movement was deemed a jetting event if ODBA exceeded 0.3 gravities (g).
Finning is a more continuous movement generated by fin-mediated thrust from waves propagating down the length of the squid mantle-fin. In contrast to intense jet propulsion events, finning is frequently used during low-speed swimming and maneuvering (Stewart et al., 2010; Bartol et al., 2016). To measure finning rates, two small cylindrical magnets (diameter: 3 mm, height: 1 mm) were placed dorso-ventrally on one fin and remained in position without any additional measures. The position of the fin and magnet were coupled, and movements distorted the ambient magnetic field measured by the ITAG magnetometer, resulting in fin position and magnetic field strength to be coupled. Concurrent video and tag data from a subset of six squid in preliminary lab control experiments revealed continuous fin-dominated swimming produced a sinusoidal curve with a frequency equivalent to fin rate (Supplementary Figure 1). First, a low-pass filter of 20 Hz was applied to the raw signal to smooth the high frequency noise. Then, a MATLAB (Mathworks, Natick, MA, USA) peak detector was used to enumerate crests in the signal which represented individual finning events. The technique was tested on 410 s of movement data from six squid. The algorithm had an average classification accuracy of 97.4%, and its worst segment performance was 95.8% correct detections.
The video data from the cages were used to corroborate and enumerate the number of intense jetting and startle alarm behaviors during noise exposure (defined in detail in Jones et al., 2020). For the impact hammer, only alarm behaviors coinciding with the impact hammer were considered. Alarm behaviors during agonistic encounters with conspecifics were not considered. Using kinematic data from the confirmed alarm behaviors, we created a custom MATLAB algorithm to identify similar movement patterns during the three noise treatment periods using the ITAG (control, vibratory hammer, impact hammer). If focal squid ODBA exceeded 0.3 g and had a concurrent two standard deviation change in finning rate, it was deemed a kinematic disturbance.
To assess if noise exposure impacted the overall swimming patterns, we applied the algorithm to all kinematic data (control and noise exposure sequences) to isolate all sequences, termed kinematic disturbances, during all noise treatments. For this analysis, noise exposure periods were treated as continuous, and all kinematic disturbances during impact and vibratory hammer periods were considered. This differs from the video analysis described above where only alarm behaviors coinciding with the hammer strike were considered.
Lastly, finning rates and ODBA were also used to measure the duration of a gait disruption. The disturbance duration was defined as the time required for the focal squid (1) to return within 25% of the mean finning rate for at least five consecutive finning events and (2) ODBA to decrease below 0.3 g. This method is analogous to Lowe (2002), which used tail-beat frequency as a metric to assess when captured sharks returned to baseline behavior after capture and handling.
Given cephalopods sensitivity to low frequency (< 1 kHz) underwater particle motion (Mooney et al., 2010), the sound field was quantified in particle acceleration using a calibrated PCB triaxial accelerometer (model W356B11; sensitivity: x = 10.26 mV m s-2, y = 10.38 mV m s-2, z = 10.62 mV m s-2) with a frequency sampling of 2 kHz. All acoustic measurements were taken during the behavioral experiments. The recording device was wired through a signal conditioner (Model 480B21, Piezotronics), which multiplied the recorded voltage by a factor of 10. The accelerometer signal was input to three analog filters (one per axis; Model FMB300B, Krohn-Hite), which each applied a bandpass filter between 0.06 and 2 kHz. Outputs of the filters were input to a data acquisition board (USB 6251, National Instruments), which was in turn connected to a laptop that ran a custom MATLAB script to record the audio files. Voltage values for each axis (x, y, and z) were calibrated to the sensitivity of the accelerometer and used to calculate the different following acoustic metrics. Recordings were taken at three distances from the pile (1, 8, and 50 m) during both IH and VH pile driving throughout the experimental period. For acoustic measurements, triaxial data were combined as the 3-D vector quantity.
For the IH, the pulse length (in ms) was measured as the time between 5% and 95% cumulative energy, and the rise time as the duration (in ms) from 5% of total energy to the peak acceleration of the signal (ISO standards 2017). The intensity was assessed by computing 0-peak accelerations (PALzpk; in dB re 1 µm s-2). Next single strike sound exposure levels (SELss; in dB re (1 µm s-2)2 * s) were calculated by integrating PALzpk over the pulse length containing 90% of the signal energy, and cumulative sound exposure levels (SELcum; in dB re (1 µm s-2)2 * s) were calculated using the following equation:
where N is the number of impulses.
Because VH signals were characterized as continuous (compared to transient IH signals), PAL was described in root mean square (PALrms; in dB re 1 µm s-2) in the 90% energy window and the 0-1 kHz frequency range, as well as SELss.
Finally, PALrms of the IH signals were calculated with identical methods as for VH signals. Based on PALrms datasets from both IH and VH, we estimated transmission losses (TL; in dB) by fitting nonlinear least-squared regressions using custom-made scripts in MATLAB (Ainslie, 2010). TL represents the loss of intensity due to the geometrical spreading of sounds in a physical medium (Ainslie, 2010), and was calculated as the slope of the logarithmic regression between PALrms and the distance from the noise source, which was expressed as:
where r is the distance between the piling and the accelerometer (in m), and alpha is the geometrical TL term.
The non-parametric Mann-Whitney U test was used to test for differences in the number of alarm behaviors at the near versus far site and between the IH versus VH. A two-sample t-test was used to test for differences in ODBA during alarm behaviors versus baseline schooling movements. Since our data fit normality assumptions, a one-way ANOVA was used to test for differences in finning rates during noise treatments and to test for differences in the frequency of kinematic disturbances during IH at the near site, far site, and control periods. Lastly, a two-sample Kolmogorov-Smirnov test was used to test if the duration of kinematic disturbances elicited during noise exposure and control periods had similar probability distributions.
A full summary of acoustic data is in Table 1. The IH and VH pile driving produced clear signals above background noise levels at both exposure sites, which allowed for isolation and analysis of all noise sequences (Figure 3A). Both rise time and pulse length increased with distance from the pile, with pulse length ranging from 190–990 ms and rise time increasing from 5.8 to 68 ms. PALzpk decreased from 122.96 dB re 1 µm s-2 at 1 m to 96.45 dB re 1 µm s-2 at 50 m. SELss for the IH ranged from 81.30 at 1 m to 68.28 dB re (1 µm s-2)2 * s at 50 m. In contrast, SELss for the continuous VH signals were greater, ranging between 137.76, 134.62, and 126.96 dB re (1 µm s-2)2 * s at 1, 8, and 50 m, respectively. SELcum for the IH was 102.04, 93.24, 88.32 dB re (1 µm s-2)2 * s at 1, 8 and 50 m. Interestingly, TL values were similar for both IH and VH signals (α = 12.9 and 11.8, respectively) despite greater PALrms for the IH (Figure 3B), which was consistent with acoustic propagation in shallow waters.
Table 1 Particle acceleration levels from the IH (black) and VH (red) at three different distances from the pile.
Figure 3 (A) PALrms propagation model labeled with the brackets denoting the distances of the experimental cages at the near and far sites. Particle acceleration was measured at multiple distances: 1, 8, and 50 m from the pile driving. The red line represents the empirically-based model fit, and the shaded region denotes the 95% confidence interval. (B) Power spectral density curves of the impact hammer and ambient noise measured at 1 m. The PSD curves were generated from a 1 min segment during both noise treatments, and the x (red), y (blue), and z (green) represent the three accelerometer axes during the impact hammer.
Over 11 experiment days, we tagged 20 squid and each animal was considered an individual noise exposure experiment. In total, 1101 and 416 minutes of kinematic and video data were collected during IH and VH pile driving, respectively. Thirteen of the 20 experiments were located at the near site, while seven experiments were conducted at the far site. Additionally, we conducted seven control experiments (409 minutes of kinematic data) with identical methods but with no pile driving noise exposure. There were significantly more noise-induced alarm behaviors at the near site [compared to the far site (near site = 17 alarm behaviors, far site = 0 alarm behaviors, Mann-Whitney U test, z = 2.19, p = 0.0284)]. Alarm behaviors were high acceleration jet propulsion events coinciding with the impact hammer or at the onset of the vibratory hammer (Figure 4). Kinematic data from the ITAG revealed that alarm responses resulted in a significant increase in ODBA (two-sample t test, t = 2.11, p = 0.0438; Figure 5). At the near site, nine of the 13 focal squid exhibited one or multiple alarm behaviors in response to the impact and vibratory hammer. Five squid elicited more than one alarm behavior. Of the squid eliciting an alarm response at noise onset, there were more alarm behaviors in response to the IH (16 alarm behaviors) compared to the onset of VH (1 alarm behavior). Eighty-two percent of the alarm responses occurred during the first or second impact or vibratory hammer sequences within a given exposure day, and a separate 82% of the alarm responses occurred within the first three impact hammer strikes or at the onset of vibratory hammer. No focal squid at the far site reacted to either pile driving noise type.
Figure 4 Squid elicit alarm behaviors in response to pile driving sound. (A) A schematic of the experimental setup with an overlaid example impact hammer signal. Black arrow highlights tagged large squid. (B) Focal tagged squid acceleration during a typical kinematic disturbance. Heightened acceleration occurs at the moment of the impact hammer strike. (C) Concurrent magnetic field strength data used to calculate finning rate. Magnetic field strength is a consistent sinusodial signal before impact hammer, but becomes irradic as the focal squid transitions to jet propulsion swimming.
Figure 5 ODBA averaged over the entire experiment periods and (left) across all 17 alarm behaviors in response to pile driving noise (right).
Although alarm behaviors occurred in response to the IH, there was no significant change in the number of kinematic disturbances over the course of an experiment vs. control day. Indeed, focal squid at the near (0.037 ± 0.034 kinematic disturbance min-1) and far (0.062 ± 0.048 kinematic disturbance min-1) sites had statistically similar kinematic disturbance frequencies compared to the quiet control periods (0.058 ± 0.058 min-1; One-way ANOVA, F2,26 = 0.88, p = 0.43, Figure 6).
Figure 6 The number of daily gait disturbances calculated from kinematic algorithms trained by confirmed reactions. Although squid reacted to pile driving noise, it did not significantly increase the number of total gait disturbances over an experimental day.
Alarm behaviors during IH sequences persisted for 4.2 ± 4.7 s. This was significantly shorter than kinematic disturbances measured during ‘quiet’ control periods 6.1 ± 4.2 s (two-sample Kolmogorov-Smirnov test, p < 0.001, Figure 7A). For each noise-induced disturbance, focal squid accelerated rapidly (i.e., high ODBA), but ODBA for each disturbance returned to similar baseline levels within ca. 4 seconds (Figure 7B). However, for some individuals, the finning gait continued to deviate from baseline or individuals reacted to consecutive hammer strikes, resulting in longer recover times, with a maximum recovery time of 14.7 s.
Figure 7 (A) Squid exhibited brief kinematic disturbances in response to pile driving noise, and these disturbances are similar in duration to natural kinematic changes during inter-individual interactions. The models compare the recovery time from both pile driving noise and naturally-induced kinematic changes. (B) ODBA during all 17 confirmed alarm responses to pile driving noise.
Although finning behavior changed at short time scales during kinematic disturbances, average finning rates during IH periods were not significantly different at the near site (1.563 ± 0.13 fin s-1), far site (1.624 ± 0.063 fin s-1), and during silent control periods (1.587 ± 0.11 fin s-1, One-way ANOVA, F2,39 = 0.63, p = 0.54, Figure 8A). Additionally, after combining all finning data across the two sites, there was no difference in average finning rates during noise exposure (IH: 1.584 ± 0.11 fin s-1; VH: 1.583 ± 0.11 fin s-1) and silent periods (1.587 ± 0.11 fin s-1; One-way ANOVA, F2,59 = 0.01, p = 0.99, Figure 8B).
Figure 8 Focal squid finning rates averaged during over the impact pile driving periods, separated by (A) near and far site and the control quiet periods. (B) Finning rates for both near and far site separated by noise treatment.
We present the first study quantifying the fine-scale movement behaviors of a marine invertebrate in response to an actual field-based anthropogenic noise source. We used high-resolution movement sensors to quantitively measure changes in swimming kinematics and measure how long gait disruptions persisted. Our results demonstrate that while field-conducted pile driving noise elicited clear alarm responses at high received levels, these were short-term evasions that persisted for only 4 s on average. Further, these escape behaviors were found only at a site of relatively high received sound levels, although the measured noise levels corresponded to roughly 1 km from actual windfarm construction pile driving (Sigray et al., 2022). Interestingly, alarm behaviors were shorter in duration than similar high acceleration movements during natural, intraspecific agonistic encounters observed during quiet control periods indicating that the animals quickly returned to sensory vigilance. Additionally, when considering overall jetting and finning gait behaviors throughout an exposure or control day, there was no detectable impact of pile driving noise on swimming behavior. Although, the experimental cage may have constrained certain swimming behaviors, particularly horizontal dispersion from the sound source.
This study used novel accelerometer-based particle acceleration measurements at multiple distances to create an acoustic propagation model and identify probabilities of movement behavior changes at specific received noise levels. Nine of 13 D. pealeii at the near site elicited at least one or more alarm movements in response to the IH between 122.96 and 112.32 PALzpk dB re 1 µm s-2, which are noise levels greater than 880 m from a one OSW construction site (Sigray et al., 2022). We know of no other sites in which we there are comparable, published, particle acceleration data. This suggests that behavioral disruption will likely occur at the kilometer scale and at a relatively substantial range, especially if we consider wind turbine pile spaces to be roughly 1 km apart and noise levels to stay consistent. More intense or persistent responses may occur within that 880 m range especially if larger pilings are used or if multiple platforms are constructed concurrently. Hence, the alarm responses described here may impact a significant majority of animals within the entire OSW development area, leading to potential regional impacts on squid populations. However, more information on noise-induced disruptions to group-level behaviors is needed to better assess impacts on populations.
Although there were clear alarm behaviors in response to pile driving noise, we found no significant difference in the number of kinematic disturbances measured from the ITAG between control and noise exposure periods (Figure 6). To be more representative of wild conditions, we used squid groups of mixed sexes in our experiments. D. pealeii are still reproductively active into September when our experiments took place (Stevenson, 1934), and squid are known to swim dynamically in breeding aggregations, and these movements were likely classified as kinematic disturbances in the present study (Shashar and Hanlon, 2013). This result provides more evidence that pile driving did not change long term swimming behaviors and it demonstrates the importance of considering the biology and group-level behaviors when quantifying noise-induced behavioral impacts. Future studies should avoid studying aggregating species in isolation because it may constrain individual behavior and limit interpretations.
Most alarm behaviors were associated with one or multiple rapid jet propulsion events; these jets resulted in elevated ODBA and a change in finning rate (Figure 4). An increase in ODBA and a transition to primarily jet propulsion indicates a higher energetic cost (Webber and O’Dor, 1986, Halsey et al., 2009). Squid are thought to operate at or near their metabolic limit (O’Dor and Webber, 1991), which suggests that an anthropogenically-induced high energy alarm behaviors may be detrimental to squid energy budgets. However, because the disruptions were transient and only elicited a maximum of three times per individual over 3-4 hours of pile driving, we suspect the impact to be non-substantial, especially considering squid frequently elicited similar dynamic kinematics during non-noise exposure periods. Additionally, free-ranging muscular squid naturally display high acceleration jet propulsion at rates, > 9 jets min-1 (Cones et al., 2022). Thus, the additional 0-3 jetting propulsion alarm responses over multiple hours of noise exposure are not likely detrimental to energetic expenditure.
No squid at the far site (with lower received levels) elicited alarm behaviors in response to either IH or VH pile driving noise despite noise levels occurring within D. pealeii sound detection abilities (Mooney et al., 2010). This result suggests there was either a dose-dependent response or there exists a minimum threshold that induces alarm behaviors, where animals detecting amplitudes 112-123 and 96 dB re 1 µm s-2 have a 69% and <1% probability of eliciting at least one alarm response, respectively. In fact, dose dependence behavioral responses were found in S. australis exposed to air gun noise (Fewtrell and McCauley, 2012). Squid elicited a higher proportion of alarm behaviors with increasing noise levels, implying the severity of noise impact on squid is related to the distance from the noise source.
Interestingly, 16 of the 17 alarm behaviors were observed during IH (7 alarm behaviors at the first hammer strike) pile driving, with only one instance of reaction to the onset of VH pile driving. This finding suggests that high amplitude and transient signals are more detrimental to squid swimming kinematics compared to low amplitude and continuous signals. Previous noise studies have largely focused upon IH noise impacts on marine life (Herbert-Read et al., 2017; Jones et al., 2020; van der Knaap et al., 2022), while fewer have directly compared noise impact with temporal variation (Neo et al., 2014; Shafiei Sabet et al., 2015). These studies also demonstrated that intermittent noises, rather than continuous, induced more severe behavioral changes including more alarm behaviors. Further research should seek impact severity comparisons between IH and VH techniques for a broader range of species. Considering some OSW farms have been successfully installed with only the VH, it could serve as an important mitigation technique in areas with suitable substrate type (OSPAR, 2014).
The duration of a behavioral disturbance is a key metric to address impacts to individual fitness, and it can inform models and evaluations of impacts by managers as they develop policy recommendations (Southall et al., 2007; Tyack et al., 2011; Ranaweerage et al., 2015; Finneran et al., 2017). Observed D. pealeii alarm responses were transient and had similar movements as anti-predator behaviors observed in other squid species (Mather, 2010). By resuming baseline swimming within only a few seconds, squid may be selecting to maximize other sensory systems or detection needs, particularly audition, to enable vigilance for predators. In late summer, coastal Massachusetts waters and the habitat of this squid are turbid. Such conditions likely renders auditory cues more useful than vision for long-term sensory perception. Low acceleration swimming could serve to decrease chaotic flow around sensory hair cells, which aid in predator detection (Mooney et al., 2010; York and Bartol, 2014; Higham et al., 2015). Another explanation for the short-term alarm responses was that D. pealeii experienced temporary or permanent shifts in hearing thresholds as seen in other species (Smith et al., 2004; Mooney et al., 2009). If so, squid may lack perception of the noise stimulus, explaining the rapid decline in alarm behaviors throughout exposure. Future studies should aim to measure hearing thresholds before and after noise exposure to determine whether D. pealeii desensitized to pile driving noise or experienced physiological impairments.
There was no significant difference in finning rates over noise treatment periods, which is more evidence suggesting pile driving noise does not alter longer term natural swimming patterns. To our knowledge, these are the first data on squid finning rates in semi-wild conditions. Most research on squid locomotion, especially in the field, has focused upon jet propulsion despite finning being integral to squid energetics and ecology (Anderson and DeMont, 2005; Bartol et al., 2016; Cones et al., 2022). Fin-dominated movements increase propulsive swimming efficiency at certain speeds and allow for versatile maneuvers which are thought to aid in squids’ ability to compete with fishes (Hoar et al., 1994; Bartol et al., 2016). Although we did not measure specific energetic costs throughout noise exposure, the finning detection method described here could be used in combination with other metrics (i.e., speed) in the future to estimate free-ranging squid energetics in response to real OSW constructions and more broadly (Anderson and DeMont, 2005; Bartol et al., 2008).
This work revealed that pile-driving noise induced clear but transient disruptions to squid swimming behavior. However, the scale of our experimental pile driving was much smaller than planned future pile driving associated with OSW development within the D. pealeii range in the U.S. eastern coast. The diameter of our steel pile was 0.3 m, while OSW turbines are using piles exceeding 8 m in diameter, perhaps approaching or exceeding 10 m diameter (Steelwind Nordenham, FHL Corporation). As a result, noise propagating from OSW constructions will likely be higher in amplitude and farther reaching, which would expand the volume of ocean where behavioral impacts may be elicited. It also indicates the alarm behaviors seen in our present study may be wide-spread or even more severe.
Consequently, this study represents a significant step toward understanding how an abundant and commercially important species will be impacted by current and planned offshore constructions. Our novel high-resolution movement and particle acceleration data allowed us to be the first study to document both the probability of behavioral change and its duration in multiple spatial scales and noise exposure contexts. Future studies should aim to assess if pile-driving causes horizontal displacement, which is of particular concern the management of commercial fisheries.
The raw data supporting the conclusions of this article will be made available by the authors, without undue reservation.
This study was carried out in accordance with the principles of the Basel Declaration and recommendations and approval of the Woods Hole Oceanographic Institution’s (WHOI’s) Institutional Animal Care and Use Committee scientific protocol to TM.
SC, YJ, and TAM designed research; SC, YJ, SF, and NA, performed research; SC and YJ analyzed the data; SC, YJ, SF, NA, and TAM wrote the paper. TAM acquired funding. All authors read and approved the last version of the manuscript.
This work was funded by the Bureau of Ocean Energy Management Cooperative Agreement #M20AC10009.
The authors thank the W.S. Schultz Co. for conducting the pile driving, especially Matt and Ben Karson. We thank Edward O’Brien and Kimberly Malkoski for facilitating SCUBA operations throughout the experiment. We also thank Rick Galat, Kerry Strom, Stephanie Madsen and other members of the WHOI Facilities team for coordinating dock space and vessel traffic. Lastly, we also express gratitude to Roger Hanlon for his discussions on our results and data interpretations.
The authors declare that the research was conducted in the absence of any commercial or financial relationships that could be construed as a potential conflict of interest.
All claims expressed in this article are solely those of the authors and do not necessarily represent those of their affiliated organizations, or those of the publisher, the editors and the reviewers. Any product that may be evaluated in this article, or claim that may be made by its manufacturer, is not guaranteed or endorsed by the publisher.
The Supplementary Material for this article can be found online at: https://www.frontiersin.org/articles/10.3389/fmars.2022.1070290/full#supplementary-material
Supplementary Figure 1 | Magnetic field strength as a method to measure fin rates. Fin and magnet position were linked, so propagating fin waves during swimming distorted the magnetic field at a frequency equivalent to the fin rate.
Ainslie M. (2010). Principles of sonar performance modelling. Princ. Sonar Perform. Model. doi: 10.1007/978-3-540-87662-5
Amaral J. L., Vigness-Raposa K., Miller J. H., Potty G. R., Newhall A., Lin Y.-T. (2020). The underwater sound from ooffshore wind farms. Acoust. Today 16, 13. doi: 10.1121/at.2020.16.2.13
Anderson E. J., DeMont M. E. (2005). The locomotory function of the fins in the squid Loligo pealei. Mar. Freshw. Behav. Physiol. 38, 169–189. doi: 10.1080/10236240500230765
Bartol I. K., Krueger P. S., Jastrebsky R. A., Williams S., Thompson J. T. (2016). Volumetric flow imaging reveals the importance of vortex ring formation in squid swimming tail-first and arms-first. J. Exp. Biol. 219, 392–403. doi: 10.1242/jeb.129254
Bartol I. K., Krueger P. S., Thompson J. T., Stewart W. J. (2008). Swimming dynamics and propulsive efficiency of squids throughout ontogeny. Integr. Comp. Biol. 48, 720–733. doi: 10.1093/icb/icn043
Bartol I. K., Patterson M. R., Mann R. (2001). Swimming mechanics and behavior of the shallow-water brief squid Lolliguncula brevis. J. Exp. Biol. 204, 3655–3682. doi: 10.1242/jeb.204.21.3655
Birkett L. P., Newton-Fisher N. E. (2011). How abnormal is the behaviour of captive, zoo-living chimpanzees? PloS One 6, e20101. doi: 10.1371/journal.pone.0020101
Clarke M. R. (1996). Cephalopods as prey. III. cetaceans. Philos. Trans. R. Soc B Biol. Sci. 351, 1053–1065. doi: 10.1098/rstb.1996.0093
Cones S., Zhang D., Shorter K., Katija K., Mann D., Jensen F., et al. (2022). Swimming behaviors during diel vertical migration in veined squid Loligo forbesii. Mar. Ecol. Prog. Ser. 691, 83–96. doi: 10.3354/meps14056
Duarte C. M., Chapuis L., Collin S. P., Costa D. P., Devassy R. P., Eguiluz V. M., et al. (2021). The soundscape of the anthropocene ocean. Science 80-, ). doi: 10.1126/science.aba4658
Fannjiang C., Aran Mooney T., Cones S., Mann D., Alex Shorter K., Katija K. (2019). Augmenting biologging with supervised machine learning to study in situ behavior of the medusa Chrysaora fuscescens. J. Exp. Biol. 222, jeb207654. doi: 10.1242/jeb.207654
Fewtrell J. L., McCauley R. D. (2012). Impact of air gun noise on the behaviour of marine fish and squid. Mar. pollut. Bull. 64, 984–993. doi: 10.1016/j.marpolbul.2012.02.009
Finneran J. J., Henderson E., Jenkins A. K., Houser D., Jenkins K., Kotecki S. E., et al. (2017). Criteria and thresholds for U. S. navy acoustic and explosive effects analysis. Space and Naval Warfare Systems Center Pacific, San Diego, CA
Flaspohler G. E., Caruso F., Aran Mooney T., Katija K., Fontes J., Afonso P., et al. (2019). Quantifying the swimming gaits of veined squid (Loligo forbesii) using bio-logging tags. J. Exp. Biol. 222, 1–13. doi: 10.1242/jeb.198226
Gielen D., Boshell F., Saygin D., Bazilian M. D., Wagner N., Gorini R. (2019). The role of renewable energy in the global energy transformation. Energy Strateg. Rev. 24, 38–50. doi: 10.1016/j.esr.2019.01.006
Halfwerk W., Holleman L. J. M., Lessells C. M., Slabbekoorn H. (2011). Negative impact of traffic noise on avian reproductive success. J. Appl. Ecol. 48, 210–219. doi: 10.1111/j.1365-2664.2010.01914.x
Halvorsen M. B., Casper B. M., Woodley C. M., Carlson T. J., Popper A. N. (2012). Threshold for onset of injury in chinook salmon from exposure to impulsive pile driving sounds. PLoS One 7, 2–12. doi: 10.1371/journal.pone.0038968
Hanlon R. T., Smale M. J., Sauer W. H. H. (2002). The mating system of the squid Loligo vulgaris reynaudii (Cephalopoda, Mollusca) off south Africa: Fighting, guarding, sneaking, mating and egg laying behavior. Bull. Mar. Sci. 71, 331–345.
Herbert-Read J. E., Kremer L., Bruintjes R., Radford A. N., Ioannou C. C. (2017). Anthropogenic noise pollution from pile-driving disrupts the structure and dynamics of fish shoals. Proc. R. Soc B Biol. Sci. 284, 20171627. doi: 10.1098/rspb.2017.1627
Higham T. E., Stewart W. J., Wainwright P. C. (2015). Turbulence, temperature, and turbidity: The ecomechanics of predator-prey interactions in fishes. Integr. Comp. Biol. 55, 6–20. doi: 10.1093/icb/icv052
Halsey L. G., Shepard E. L.C., Quintana F., Gomez Laich A., Green J. A., Wilson R. P. (2009). The relationship between oxygen consumption and body acceleration in a range of species. Comp. Biochem. Physiol. - A Mol. Integr. Physiol. 152, 197–202. doi: 10.1016/j.cbpa.2008.09.021.
Hoar J. A., Sim E., Webber D. M., O’Dor R. K. (1994). “The role of fins in the competition between squid and fish,” in Mechanics and physiology of animal swimming. Eds. Maddock L., Bone Q., Rayner J. Cambridge University Press, Cambridge UK, 27–43.
Hunsicker M. E., Essington T. E., Watson R., Sumaila U. R. (2010). The contribution of cephalopods to global marine fisheries: can we have our squid and eat them too? Fish Fish. 11, 421–438. doi: 10.1111/j.1467-2979.2010.00369.x
Jézéquel Y., Cones S., Jensen F. H., Brewer H., Collins J., Mooney T. A. (2022). Pile driving repeatedly impacts the giant scallop (Placopecten magellanicus). Sci. Rep., 1–11. doi: 10.1038/s41598-022-19838-6
Jones I. T., Stanley J. A., Bonnel J., Mooney T. A. (2019). Complexities of tank acoustics warrant direct, careful measurement of particle motion and pressure for bioacoustic studies. 2019 Int. Congr. Ultrason. 38, 010005. doi: 10.1121/2.0001073
Jones I. T., Stanley J. A., Mooney T. A. (2020). Impulsive pile driving noise elicits alarm responses in squid (Doryteuthis pealeii). Mar. pollut. Bull. 150, 110792. doi: 10.1016/j.marpolbul.2019.110792
Jones I. T., Peyla J. F., Clark H., Song Z., Stanley J. A., Mooney T. A. (2021). Changes in feeding behavior of longfin squid (Doryteuthis pealeii) during laboratory exposure to pile driving noise. Mar. Environ. Res. 165, 105250. doi: 10.1016/j.marenvres.2020.105250.
Kastelein R. A., Helder-Hoek L., Covi J., Gransier R. (2016). Pile driving playback sounds and temporary threshold shift in harbor porpoises (Phocoena phocoena): Effect of exposure duration. J. Acoust. Soc Am. 139, 2842–2851. doi: 10.1121/1.4948571
Kunc H. P., Lyons G. N., Sigwart J. D., McLaughlin K. E., Houghton J. D. R. (2014). Anthropogenic noise affects behavior across sensory modalities. Am. Nat. 184, E93–E100. doi: 10.1086/677545
Lowe C. G. (2002). Bioenergetics of free-ranging juvenile scalloped s. lewini. J. Exp. Mar. Bio. Ecol. 278, 141–156.
Mather J. A. (2010). Vigilance and antipredator responses of Caribbean reef squid. Mar. Freshw. Behav. Physiol. 43, 357–370. doi: 10.1080/10236244.2010.526760
Miller P. J. O., Biassoni N., Samuels A., Tyack P. L. (2000). Whale songs lengthen in response to sonar. Nature 405, 903. doi: 10.1038/35016148
Miller P. J. O., Kvadsheim P. H., Lam F. P. A., Wensveen P. J., Antunes R., Alves A. C., et al. (2012). The severity of behavioral changes observed during experimental exposures of killer (Orcinus orca), long-finned pilot (Globicephala melas), and sperm (Physeter macrocephalus) whales to naval sonar. Aquat. Mamm. 38, 362–401. doi: 10.1578/AM.38.4.2012.362
Mooney T. A., Andersson M. H., Stanley J. A. (2020). Acoustic impacts of offshore wind energy on fishery resources. Oceanography 33(4), 82–95. doi: 10.5670/oceanog.2020.408
Mooney T. A., Hanlon R. T., Christensen-Dalsgaard J., Madsen P. T., Ketten D. R., Nachtigall P. E. (2010). Sound detection by the longfin squid (Loligo pealeii) studied with auditory evoked potentials: Sensitivity to low-frequency particle motion and not pressure. J. Exp. Biol. 213, 3748–3759. doi: 10.1242/jeb.048348
Mooney T. A., Katija K., Shorter K. A., Hurst T., Fontes J., Afonso P. (2015). ITAG: An eco-sensor for fine-scale behavioral measurements of soft-bodied marine invertebrates. Anim. Biotelemetry 3, 1–14. doi: 10.1186/s40317-015-0076-1
Mooney T. A., Nachtigall P. E., Vlachos S. (2009). Sonar-induced temporary hearing loss in dolphins. Biol. Lett. 5, 565–567. doi: 10.1098/rsbl.2009.0099
Neo Y. Y., Seitz J., Kastelein R. A., Winter H. V., ten Cate C., Slabbekoorn H. (2014). Temporal structure of sound affects behavioural recovery from noise impact in European seabass. Biol. Conserv. 178, 65–73. doi: 10.1016/j.biocon.2014.07.012
O’Dor R. K., Webber D. M. (1991). Invertebrate Athletes: Trade-Offs between Transport Efficiency and Power Density in Cephalopod Evolution. J. Exp. Biol. 160, 93–112. doi: 10.1242/jeb.160.1.93.
OSPAR (2014). OSPAR inventory of measures to mitigate the emission and environmental impact of underwater noise biodiversity series 41.
Popper A. N., Hawkins A. D. (2019). An overview of fish bioacoustics and the impacts of anthropogenic sounds on fishes. J. Fish Biol. 94, 692–713. doi: 10.1111/jfb.13948
Popper A. N., Hice-Dunton L., Jenkins E., Higgs D. M., Krebs J., Mooney T. A., et al. (2022). Offshore wind energy development: Research priorities for sound and vibration effects on fishes and aquatic invertebrates. J. Acoust. Soc Am. 151, 205–215. doi: 10.1121/10.0009237
Ranaweerage E., Ranjeewa A. D. G., Sugimoto K. (2015). Tourism-induced disturbance of wildlife in protected areas: A case study of free ranging elephants in Sri Lanka. Glob. Ecol. Conserv. 4, 625–631. doi: 10.1016/j.gecco.2015.10.013
Shafiei Sabet S., Neo Y. Y., Slabbekoorn H. (2015). The effect of temporal variation in sound exposure on swimming and foraging behaviour of captive zebrafish. Anim. Behav. 107, 49–60. doi: 10.1016/j.anbehav.2015.05.022
Shashar N., Hanlon R. T. (2013). Spawning behavior dynamics at communal egg beds in the squid Doryteuthis (Loligo) pealeii. J. Exp. Mar. Bio. Ecol. 447, 65–74. doi: 10.1016/j.jembe.2013.02.011
Sigray P., Linné M., Andersson M. H., Nöjd A., Persson L. K. G., Gill A. B., et al. (2022). Particle motion observed during offshore wind turbine piling operation. Mar. pollut. Bull. 180, 113734. doi: 10.1016/j.marpolbul.2022.113734
Smith M. E., Kane A. S., Popper A. N. (2004). Noise-induced stress response and hearing loss in goldfish (Carassius auratus). J. Exp. Biol. 207, 427–435. doi: 10.1242/jeb.00755
Southall B. L., Bowles A. E., Ellison W. T., Finneran J. J., Gentry R. L., Greene C. R. Jr, et al. (2007). Marine mammal noise exposure criteria: Initial scientific recommendations. Aquat. Mamm. 33, 446–473. doi: 10.1578/AM.33.4.2007.411
Southall B. L., Nowacek D. P., Bowles A. E., Senigaglia V., Bejder L., Tyack P. L. (2021). Evolutions in marine mammal noise exposure criteria. Aquat. Mamm. 47, 421–464. doi: 10.1121/at.2021.17.2.52
Stevenson J. A. (1934). On the behavior of the long-finned squid Loligo pealei (LeSueur). Can. F. Nat. 48, 4–7.
Stewart W. J., Bartol I. K., Krueger P. S. (2010). Hydrodynamic fin function of brief squid, Lolliguncula brevis. J. Exp. Biol. 213, 2009–2024. doi: 10.1242/jeb.039057
Tyack P. L., Zimmer W. M. X., Moretti D., Southall B. L., Claridge D. E., Durban J. W., et al. (2011). Beaked whales respond to simulated and actual navy sonar. PloS One 6, e17009. doi: 10.1371/journal.pone.0017009
van der Knaap I., Slabbekoorn H., Moens T., Van den Eynde D., Reubens J. (2022). Effects of pile driving sound on local movement of free-ranging Atlantic cod in the Belgian north Sea auteurs. Environ. pollut. 300, 118913. doi: 10.1016/j.envpol.2022.118913
Webber D. M., O’Dor R. K. (1986). Monitoring the Metabolic Rate and Activity of Free-Swimming squid With Telemetered Jet Pressure. J. Exp. Biol. 126, 205–224. doi: 10.1242/jeb.126.1.205
Wells M. J., O’Dor R. K. (1991). Jet propulsion and the evolution of the cephalopods. Bull. Mar. Sci. 49, 419–432.
Wilson R. P., White C. R., Quintana F., Halsey L. G., Liebsch N., Martin G. R., et al. (2006). Moving towards acceleration for estimates of activity-specific metabolic rate in free-living animals: The case of the cormorant. J. Anim. Ecol. 75, 1081–1090. doi: 10.1111/j.1365-2656.2006.01127.x
York C. A., Bartol I. K. (2014). Lateral line analogue aids vision in successful predator evasion for the brief squid, Lolliguncula brevis. J. Exp. Biol. 217, 2437–2439. doi: 10.1242/jeb.102871
Keywords: noise, energetics, gait, jet propulsion, finning
Citation: Cones SF, Jézéquel Y, Ferguson S, Aoki N and Mooney TA (2022) Pile driving noise induces transient gait disruptions in the longfin squid (Doryteuthis pealeii). Front. Mar. Sci. 9:1070290. doi: 10.3389/fmars.2022.1070290
Received: 14 October 2022; Accepted: 23 November 2022;
Published: 15 December 2022.
Edited by:
Marta Solé, Universitat Politècnica de Catalunya, BarcelonaTech (UPC), SpainReviewed by:
Michael L. Fine, Virginia Commonwealth University, United StatesCopyright © 2022 Cones, Jézéquel, Ferguson, Aoki and Mooney. This is an open-access article distributed under the terms of the Creative Commons Attribution License (CC BY). The use, distribution or reproduction in other forums is permitted, provided the original author(s) and the copyright owner(s) are credited and that the original publication in this journal is cited, in accordance with accepted academic practice. No use, distribution or reproduction is permitted which does not comply with these terms.
*Correspondence: Seth F. Cones, c2V0aGZjb25lc0BnbWFpbC5jb20=
Disclaimer: All claims expressed in this article are solely those of the authors and do not necessarily represent those of their affiliated organizations, or those of the publisher, the editors and the reviewers. Any product that may be evaluated in this article or claim that may be made by its manufacturer is not guaranteed or endorsed by the publisher.
Research integrity at Frontiers
Learn more about the work of our research integrity team to safeguard the quality of each article we publish.