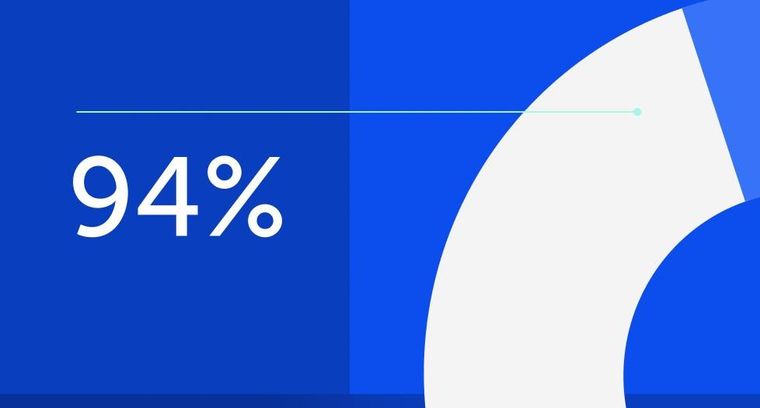
94% of researchers rate our articles as excellent or good
Learn more about the work of our research integrity team to safeguard the quality of each article we publish.
Find out more
BRIEF RESEARCH REPORT article
Front. Mar. Sci., 16 January 2023
Sec. Global Change and the Future Ocean
Volume 9 - 2022 | https://doi.org/10.3389/fmars.2022.1068967
Offshore wind farms (OWFs) can increase the transfer and stock of organic carbon (OC) in the surrounding sediments during their operational phase, while their construction and decommissioning release carbon. To answer the question whether sediments of OWFs trap more OC than they release, we estimate the net carbon effect over the entire life cycle (construction, operational and decommissioning phases) of OWFs in the Southern North Sea. Based on existing studies we compare the increased OC flux due to the colonization of organisms at the foundations of wind turbines and the OC loss due to sediment-disturbing activities during construction and decommissioning. Our results show that the areal intensity of carbon release in the disturbed areas is about 43.5 times higher than that of carbon trapping in the entire area of the OWFs. However, since the disturbed areas only account for about 0.50 ± 0.06% of the total area of the OWFs, in absolute terms about 4.6 ± 1.4 times more carbon is trapped in the sediment of the OWFs than is released. Due to limited data availability and the resulting need for extensive assumptions, our estimates only represent orders of magnitude. We therefore provide sensitivity estimates that define the limits of our calculations in terms of disturbance depth, remineralisation ratio, scour protection measures and heterogenous OC contents. In addition, we identify shortcomings of our extrapolation. Further research, especially more advanced impact assessments of construction and decommissioning processes must follow to improve the understanding of impacts of OWFs on sedimentary OC.
The global solar and wind energy sectors, including offshore wind, are booming (Mangi, 2013; Szulecki et al., 2016; Voormolen et al., 2016), as the expansion of these renewable energy sources is necessary to ensure compliance with the Paris Climate Agreement (United Nations, 2015). In the European Union (EU), the number of offshore wind farms (OWFs) is expected to increase 40-fold by 2030 (Global Wind Energy Council, 2015), with most of these OWFs built on the sediments of the continental shelf of the North Sea. Continental shelf sediments are globally significant CO2 storage areas and potentially provide an important, yet largely overlooked, climate regulation service via the Earth’s carbon cycle (Diesing et al., 2017; Smeaton et al., 2021). Hence, it is crucial to understand how OWFs impact shelf sediments such as those of the North Sea and whether they increase or decrease sedimentary carbon stocks.
There is growing evidence that OWFs affect the physical properties of the water column (Broström, 2008; Christiansen et al., 2022) and the sediment (Hiscock et al., 2002; Ivanov et al., 2021), as well as the benthic fauna (Coates et al., 2014; Degraer et al., 2020) in their immediate surroundings, potentially altering the natural carbon stocks in the sediment. For instance, the presence of turbine foundations introduces artificial hard substrate into areas of natural soft bottom, creating a new type of habitat (Krone et al., 2013). This new habitat, the foundations, is rapidly colonized by epifaunal communities consisting mainly of suspension feeders, in the North Sea most notably the blue mussel Mytilus edulis, which accounts for up to 90% of the biomass of epistructures at some sites (Slavik et al., 2019), and the amphipod crustacean Jassa herdmani (Mavraki et al., 2022). This new epifaunal colonization leads to a significant transfer of organic carbon (OC) in the form of fecal pellets and biomass sinking from the structures onto the sediments surrounding the wind turbines (Krone et al., 2013; De Borger et al., 2021; Mavraki et al., 2022). Using a 3D hydrodynamic model developed by Ivanov et al. (2021); De Borger et al. (2021) found that this leads to a 10-11% increase in OC accumulation in the upper 10 cm of the sediment and an increase in anoxic mineralization processes in the vicinity of the OWFs. As a result, the OWFs installed on the sediments of the Belgian Part of the North Sea became local carbon sinks compared to the baseline scenario (areas without OWFs) and have increased the total amount of OC in the upper 10 cm of the sediment by 28,715 tons (De Borger et al., 2021). Other colonizing organisms also contribute to the increase of OC stocks in the sediment of OWFs. For example, J. herdmani contribute with an additional flux of 255 to 547 tons of carbon per year into the sediment of OWFs in the waters of Belgium, the Netherlands and Germany (Mavraki et al., 2022).
However, this is not the entire story of the impact of OWFs on sediment carbon stocks (Figure 1). First, the construction of OWFs is expected to release carbon accumulated in the sediment, as this work involves a range of sediment-disturbing activities (OSPAR Commission, 2008) that resuspends sedimentary carbon. Second, after an expected wind turbine lifetime of ~ 20 years (Nghiem and Pineda, 2017), the concession holder must restore the concession area to its original conditions (Kruse et al., 2019). De Borger et al. (2021) already described that during decommissioning, sediment-disturbing activities can cause the release of accumulated carbon into the water column, where it can be remineralised and potentially escapes to the atmosphere. About 50% of the currently installed capacity of wind turbines (including many OWFs) will reach the end of their operational life by 2030 (Nghiem and Pineda, 2017). Hence, the entire life cycle (construction, operation, decommissioning) of OWFs needs to be considered to assess the impact of these anthropogenic constructions on natural carbon stocks. However, so far, no attempt has been made to quantify the impact of construction and decommissioning on carbon stocks in shelf sediments.
Figure 1 Impacts of different life cycles (construction phase, operational phase, decommissioning phase) of OWFs on the sediment and the resulting trapped and released organic carbon (OC) in the upper 1 m of the sediment. *The period refers only to the duration of sediment-disturbing activities of individual elements of OWFs, such as individual cables and individual turbines, and not to time needed to install the entire wind farm or to the duration of all processes involved in construction or decommissioning, i.e. the time for installation of the rotor blades is not considered, for example.
Therefore, we provide a first estimate of the magnitude of carbon release from the sediment as a result of construction and decommissioning processes for OWFs located in the Southern North Sea (SNS). Combined with the values of trapped carbon during the operational period described by De Borger et al. (2021) and Mavraki et al. (2022), we estimate the net effect (per area and turbine) on the sedimentary OC stock after an entire life cycle of OWFs (20 years) and upscale these calculations for the OWF areas in the whole SNS.
We focus on the SNS (Figure 2) as 63% of OWFs in EU waters are located in this area and it is expected to remain a hotspot for further OWF development in the EU (Slavik et al., 2019).
Figure 2 Study area in the SNS. OWFs are divided into three categories based on their construction and planning status as of 2022. Category I includes fully commissioned, pre-construction and under construction wind farms (green), category II includes consent application submitted, consent authorized and concept/early planning wind farms (orange) and category III includes development zones (blue) (edited map from 4C offshore (2022)). For our calculations, we focus on OWFs of category I and II.
We retrieve all data about the OWFs of the SNS (e.g. size, number of turbines, capacity, status) from 4C offshore (2022), a company specialized in marine energy consultancy. We divide the OWFs into three categories based on their status as of 2022: category I (including fully commissioned, under construction, pre-construction), category II (including consent application submitted, consent authorized, concept/early planning) and category III (development zones) (Figure 2). As the completeness of information varies greatly between these categories (see Supplementary 1, T1-T4), we focus our calculations on categories I and II and only address category III briefly given the high degree of incomplete data and uncertainties of category III OWFs.
Over an area of currently installed OWFs of 238 km² and 399 turbines, the additional amount of OC fixed in the top 10 cm of sediment in the Belgian part of the North Sea amounts to 28,715 tons due to blue mussels (De Borger et al., 2021) and 700 to 1,500 t (35 to 75 t/year for the Belgian turbines in the North Sea) due to J. herdmani (Mavraki et al., 2022). Extrapolating this result, an average of 74.7 ± 1,1 tons per turbine (29,800 ± 400 tons divided by 399 turbines) of additional OC could be captured by OWFs in the SNS. Multiplying the amount of additional carbon per turbine with the estimated number of turbines for OWFs of categories I and II, we obtain the amount of OC that is trapped in the OWFs in the SNS after the operational phase of 20 years.
Since very little qualitative let alone quantitative information are available on the construction and especially the decommissioning processes, we have to simplify the complexity of the involved processes. We do not distinguish between the different sediment-disturbing activities (such as disposal of spoil, explosive, drilling, the legs of jack-up barges and anchorage of construction vessels) during construction and decommissioning, as their impacts are likely to be similar (Hiscock et al., 2002; OSPAR Commission, 2008). In addition, we only use the areas affected during drilling of foundation holes and cable laying. For other activities, the extent to which they affect the sediment is even less known. Spielmann et al. (2021) described that the foundation structure will be cut off 1 m below the seabed during decommissioning. Therefore, we only include the first meter of sediment in our calculations. Although deeper layers are likely also affected, most studies on organic carbon distribution in the sediment only consider the first 10 cm of sediment, so data on the properties of the deeper sediment layers, e.g. OC content, are very limited.
For calculating the effect of OWF construction and decommissioning on the OC stocks, we first quantify the number of turbines and the lengths of inner and export cables as well as the associated disturbed sediment areas.
We focus on monopiles foundations, since these are by far the most used foundations for offshore wind turbines in the North Sea and are expected to be primarily used in the future (Grow, 2022). In some of the category II OWFs, we are missing turbine numbers. In these cases, we calculate the average capacity per turbine using already complete information (available: expected capacity of OWFs, number of turbines) and divide the total capacity of the category II OWFs with the average capacity per turbine. The diameter of the central drill used for monopile foundations ranges from 5.1 to 8.5 m (Acteon, 2022). Since monopiles will become even larger in the future (XXL monopiles) and turbines construction and decommissioning affect sediments beyond their diameter, we use a diameter of 9 m for our calculations, which results in a disturbed area of 63.62 m² per foundation, both for construction and decommissioning. Scour protection measures installed around turbines, when removed, increase the disturbed sediment area. Since the exact decommissioning of these structures is still unclear and leaving them in place is a quite realistic option, as Spielmann et al. (2021) shows in 4 out of 5 of their decommissioning scenarios, we calculate without these structures. However, we perform additional sensitivity estimates including the area of scour protection in the “Discussion”.
Cables are buried in trenches approximately 2 m wide (OSPAR Commission, 2008). Based on data collected from the Amrumbank West wind farm (RWE, 2022), 3.33 km inner cables are used per square kilometer and 1.25 km per turbine. To calculate the absolute area of inner cabling for different OWFs, we multiply 3.33 km km-2 and 1.25 km per turbine by the total area of the OWFs and the number of turbines respectively and average the resulting two values, as this is closest to the reference area of the Amrumbank West wind farm (Supplementary 1, T6).
Since there is a large information gap regarding the onshore export cables and the additional offshore platforms, we have followed the report by the Bundesamt für Seeschiffahrt und Hydrographie (2017), which, however, only refers to German OWFs. Summing the reported values of export cables (4.18 km2), additional offshore platforms (600 m2 per platform) and so-called crossing structures (900 m2 per structure), the export cable area amounts to 4.55 km2 for a total of 31 German offshore wind farms. Based on this calculation, we estimated the export cable area per OWF, allowing us to extrapolate the total export cable area for all OWFs in the SNS. Even though the extrapolation is subject to many uncertainties, it is necessary to include the export cables in the calculations, since they account for a considerable part (22.86%) of the disturbed area (Supplementary 1, T9).
After estimating the disturbed areas, we calculate the standing stock of particulate organic carbon (POC) in the sediment of the OWFs that is resuspended due to sediment-disturbances and remineralized in the water column. In the absence of data on the effect of construction or decommissioning on resuspension and remineralisation processes and thus on carbon release, we used data on the effects of bottom trawling on carbon release from Luisetti et al. (2019), as this process potentially disturbs sediment in a similar way. Following Luisetti et al. (2019), we do not include changes in benthic remineralisation processes for simplicity. The general equation for calculating carbon release in kgC m-2 from bottom trawling according to Luisetti et al. (2019) is (Eq. 1):
where the POC stock (POCStock) in kgC m-3 is the product of the POC content (POCC) in % and the dry bulk density (DBD) kg m-3 and the fraction of this stock that is remineralised is determined with the inverse of the resuspended POC (POCresusp) in %. To obtain the amount of remineralized OC for the construction phase, we use POCC and DBD data from Diesing et al. (2017) and a POCresusp value given by Luisetti et al. (2019). Due to the spatial non-uniformity of sediment types with different POCC, we resolve mud, sand and gravel within the OWF sediments using sediment information provided by Wilson (2017) and the coordinates of category I and II OWFs, which we determine with the European Atlas of the Seas (European Union, 2022) (Supplementary I, T26 and Supplementary III). Based on the sediment distribution within rectangles around these coordinates (latitude and longitude ± 0.3° for larger and ± 0.1° for smaller OWFs), we calculate the percentages of mud, sand and gravel across all OWFs (summary in Supplementary 2). With these percentages, we calculate the average POCC and DBD for all OWFs. Although the resolution is still coarse and the rectangles are a simplification, we consider our method acceptable because we are interested in orders of magnitude, and changes in the size of the rectangles do not significantly alter the result of the percentages. For the construction phase, we calculate the release of carbon as follows (Eq. 2):
and for the decommissioning phase with the additional OC trapped in the upper 10 cm according to De Borger et al. (2021) (Eq. 3):
We subsequently multiply the carbon release per area with the estimated disturbed sediment areas to determine the absolute amount of OC that is lost after construction and decommissioning.
The following estimates refer to OWFs within categories I and II. In total, these OWFs cover an area of 12,010 km², including 6,434 wind turbines. The total area affected by sediment-disturbing activities amounts to 60 ± 7 km². The area of foundations accounts for 0.68 ± 0.08% (0.4 km²), that of inner cabling for 76 ± 8% (46 ± 7 km²) and that of export cables and offshore platforms for 22.9 ± 2.4% (14 km²) of the total area of disturbed sediments.
The areal intensity of carbon release within disturbed areas (1,700 ± 500 t km-2) is 43.5 times more intense than the areal intensity of carbon trapping within OWFs (40.0 ± 0.6 t km-2). However, since only 0.50 ± 0.06% of the total area of the OWFs are affected by sediment-disturbing activities, while the operational phase increases the OC content in the whole area, multiplying the area-specific values by the affected areas results in 481,000 ± 7,000 t OC being trapped in the sediments of OWFs at the end of the operational phase (after 20 years), while only 100,000 ± 40,000 t carbon is released into the atmosphere during the construction and decommissioning phases (Table 1; Figure 3). This means that in absolute terms 4.6 ± 1.4 times more carbon is trapped than is released after an entire life cycle.
Table 1 Carbon released and trapped during the different phases of the life cycle of OWFs in the SNS.
Figure 3 Impacts of different life cycles (construction phase, operational phase, decommissioning phase) of OWFs on the sediment and the resulting trapped and released organic carbon (OC) in the upper 1 m of the sediment. The area affected during the different life cycles is highlighted (light brown). The absolute values apply to the entire area of the OWFs in the Southern North Sea (SNS). For the construction and decommissioning phases, we round the values to one figure after the first significant figure to illustrate their minor differences.*The period refers only to the duration of sediment-disturbing activities of individual elements of OWFs, such as individual cables and individual turbines, and not to time needed to install the entire wind farm or to the duration of all processes involved in construction or decommissioning, i.e. the time for installation of the rotor blades is not considered, for example.
Our estimation of the net carbon effect of OWFs over their entire life cycle indicates that the sediments within the area of the OWFs trap more OC than they release. This outcome can be explained by the varying temporal and spatial scales of the different phases and processes of the life cycle of the OWFs in our calculation. About 0.50 ± 0.06% of the area where the OC accumulates during the operational phase (total area of the OWFs) is affected by sediment-disturbing activities during the construction and decommissioning phases (Figure 3). Additionally, OWFs are operated for at least two decades, while sediment-disturbing activities only have an effect for days to weeks at individual spots. Therefore, the additional OC entering the sediments during 20 years of operational phase (De Borger et al., 2021) is not released again, since most of the area of the OWFs remains untouched during decommissioning. In other words, to shift the net effect in OWF areas from sink to source, the area affected by sediment-disturbing activities would have to increase by 4.6 (category 1) to 2.0 (category 2) (Supplementary I, T25). Despite the uncertainties in the calculations of the inner and export cables and scour protection measures, we consider it unlikely that the area we have neglected takes up this order of magnitude.
One of the largest uncertainties in our calculations is the depth to which disturbances are exerted. According to Hiscock et al. (2002), the steel piles of the foundations are driven 10 - 20 m deep into the seabed, and the cables are laid at a depth of 3 m. Keeping all other variables constant and increasing the depth to 20 m for the foundations and 3 m for the cables, our calculation results in about 3.1 times the amount of carbon per km² entering the water column and being remineralized compared to the original calculation (Supplementary 1, T15). The calculated deviation describes an upper and unrealistically high limit, as the OC content of the sediment decreases with depth, yet we consider it constant here. Despite the overestimation, 1.50 ± 0.11 times more OC would be trapped than released with increased disturbance depths.
To account for potentially higher OC levels in the sediment due to different sediment types, we assess how a very high homogeneous POCC would change our results. If the sediment consisted only of gravelly mud (0.91% POCC) and all other conditions remained constant, the carbon release per km² would be about 2.4 times higher than in our original calculation but the sediments of the OWFs would still trap 1.92 ± 0.15 more carbon than they release (Supplementary 1, T17). Again, this sensitivity calculation presents an upper limit, as the uniformly high POCC is unrealistic (Diesing et al., 2017).
Given the significant uncertainties in estimating the carbon release of sediment-disturbing activities, it is possible that more sediment would be resuspended and more carbon than the assumed 22.5% of the resuspended sediment would be remineralized (Lovelock et al., 2017). Luisetti et al. (2019) speculated that up to 100% could be remineralized during bottom-trawling. Using 100% remineralization would result in a 4.4 times higher carbon release per km² when compared to the original calculation. Under these extreme remineralization conditions, the sediments would trap about 1.0 ± 0.4 more OC than they would release, which is close to a net zero balance. Since the sediment is only strongly disturbed for a comparatively short time, it is very unlikely that 100% of the resuspended material is remineralized (Black et al., 2022).
According to Coates et al. (2014), 2.174 times more OC is trapped in a 15 m radius around the foundation compared to the remaining OWF area (Supplementary 1, T7) during the operational phase. This could potentially influence our result, especially if the removal of scour protection measures increases the disturbance area around turbines. Adjusting the increase of the OC content from an average of 10% (De Borger et al., 2021) to 21.69% in a 15 m radius around turbines and 9.98% in the rest (Supplementary I, T7) alone shows no significant change in the ratio of stored to released carbon (Supplementary 1, T10-T13). Therefore, we also increase the disturbed area by the area of potential scour protection measures (Supplementary 1, T10-T13). The protection measures differ in material and size (25 - 40 m diameter) (OSPAR Commission, 2008; Maar et al., 2009). We assume a width of 15 m (30 m diameter) around the turbine. The inclusion of scour protection measures would increase the disturbed foundation area by about 18 times during decommissioning. Overall, however, this corresponds to only about 11.4% of the total disturbed area. The combination of horizontally variable OC content and larger disturbed foundation area would still trap 4.1 ± 1.2 times more carbon than it would release. Since it is still debated if and how scour protection measures will be removed, as mentioned before, this result also presents an upper limit.
Combinations of the above-mentioned changed variables lead to negative net effects in each case (Supplementary 1, T20-T24). This is plausible, as they all represent mainly unrealistic upper limits. Nevertheless, we cannot eliminate the possibility that there are combinations of the discussed variables that no longer lead to a positive balance, but are realistic nonetheless. More research on the horizontal and vertical sedimentary carbon distribution around OWFs and the impacts of construction and decommissioning processes on sedimentary carbon is therefore necessary for a concluding evaluation of the conditions under which our result holds.
Apart from the variables mentioned above, the sign of the net balance stands and falls with the amount of OC that is trapped in the sediment by the sinking of fecal pellets and detritus. Unfortunately, this amount is highly variable and poorly determined on larger scales such as the SNS, as very heterogeneous factors determine the OC downward flux. For example, OC flux into sediment depends on factors such as local to regional hydrodynamics (Ivanov et al., 2020), water depth (Ivanov et al., 2021), temperature (Mavraki et al., 2022), primary productivity (Bayne et al., 1993), seasonality of organisms (Krone et al., 2013), biomass density and habitable turbine surface areas (Mavraki et al., 2022) and the duration of the operational phase, which are unique to each OWF. Extrapolating the results of De Borger et al. (2021) and Mavraki et al. (2022) for the Belgian OWFs to the whole SNS is therefore our major shortcoming, as we implicitly assume that the conditions in the Belgian part of the North Sea are applicable to the whole SNS. This is certainly not the case, so the actual OC sediment flux is likely to differ from our estimate. For example, fecal pellet production could be lower at colder temperatures due to decreased metabolic rates (Mavraki et al., 2022) in the northern part of the SNS or in winter, or it could be reduced due to lower primary productivity as a result of decreasing nutrients (De Borger et al., 2021). Our extrapolation does not account for this heterogeneity. However, since our estimated net balance would only be zero if inputs were reduced by 4.6 in total, and since we focus on orders of magnitude and especially the sign of the net balance, we consider our result sufficient for the time being. However, future research should further focus on smaller scales and add up the results of different OWFs to get the whole picture. The equations and assumptions we have made can be adapted to smaller scales and may help.
A final comment needs to be made about an even larger scale. Our results only apply to the OWF areas of the SNS and do not represent the overall impact of the OWFs on the whole SNS, as the effects outside the OWF can be quite different and may cancel or amplify each other. De Borger et al. (2021) have already demonstrated that mineralization rates and carbon storage decrease in sediments outside OWF areas. In addition, OWFs can also affect oceanographic, e.g. oxygen levels (Daewel et al., 2022), and biological processes, e.g. primary production (Slavik et al., 2019; Daewel et al., 2022), even in adjacent areas. The consequences for carbon stocks in these areas are currently unknown. Therefore, it is necessary to consider the whole ecosystem and additional variables beyond the OWF areas to rule out possible reallocations in the carbon pools and to answer the question whether there is indeed additional trapping of atmospheric carbon due to OWFs in the whole SNS.
Given the demand of many EU countries for an independent and renewable energy source such as wind energy and the resulting rapidly growing offshore wind industry, there is an urgent need for specific studies on the impacts of OWF construction and decommissioning on biogeochemical and ecological processes and on sediments, as well as on the resulting carbon release. We provide a first assessment of the net impact of OWFs on sediment OC stocks over the entire life cycle of OWFs, indicating that sediments of the OWFs trap more OC than they release.
To determine the net carbon balance under broader conditions and to account for the heterogeneity of the SNS, the relevant parameters need to be better constrained and quantified, especially at smaller scales. Our results show that, in addition to their proven mitigation potential as renewable energy, OWFs can promote carbon storage and increase carbon stocks in OWF sediments.
The original contributions presented in the study are included in the article/Supplementary Material. Further inquiries can be directed to the corresponding author.
KH conceived/designed the study, performed research, analysed data, contributed new methods and wrote the paper. MS conceived/designed study, contributed new methods and wrote the paper. All authors contributed to the article and approved the submitted version.
Our work is funded by the project APOC “Anthropogenic impacts on particulate organic carbon cycling in the North Sea”, 03F0874D, financed through the German Federal Ministry of Education and Research (BMBF).
We thank Jana Hinners and Josephine Herrford for their valuable feedback on our first manuscript. In addition, we thank the reviewers for their critical and extremely helpful comments
The authors declare that the research was conducted in the absence of any commercial or financial relationships that could be construed as a potential conflict of interest.
All claims expressed in this article are solely those of the authors and do not necessarily represent those of their affiliated organizations, or those of the publisher, the editors and the reviewers. Any product that may be evaluated in this article, or claim that may be made by its manufacturer, is not guaranteed or endorsed by the publisher.
The Supplementary Material for this article can be found online at: https://www.frontiersin.org/articles/10.3389/fmars.2022.1068967/full#supplementary-material
4C offshore (2022) Global offshore windfarms. Available at: https://www.4coffshore.com/offshorewind/.
Acteon (2022). Marine energy and infrastructure services. Available at: https://acteon.com/.
Bayne B. L., Iglesias J. I. P., Hawkins A. J. S., Navarro E., Heral M., Deslous-Paoli J. M. (1993). Feeding behaviour of the mussel, mytilus edulis : responses to variations in quantity and organic content of the seston. J. Mar. Biol. Ass. 73 (4), 813–829. doi: 10.1017/S0025315400034743
Black K. E., Smeaton C., Turrell W. R., Austin W. E.N. (2022). Assessing the potential vulnerability of sedimentary carbon stores to bottom trawling disturbance within the UK EEZ. Front. Mar. Sci. 9. doi: 10.3389/fmars.2022.892892
Broström Göran (2008). On the influence of large wind farms on the upper ocean circulation. J. Mar. Syst. 74 (1-2), 585–591. doi: 10.1016/j.jmarsys.2008.05.001
Bundesamt für Seeschiffahrt und Hydrographie (2017). Bundesfachplan offshore für die deutsche ausschließliche wirtschaftszone der nordsee 2016/2017 und umweltbericht. Hamburg. Available at: https://www.bsh.de/DE/PUBLIKATIONEN/_Anlagen/Downloads/Offshore/Bundesfachplan-Nordsee/Bundesfachplan-Offshore-Nordsee-2016-2017.html.
Christiansen N., Daewel U., Djath B., Schrum C. (2022). Emergence of Large-scale hydrodynamic structures due to atmospheric offshore wind farm wakes. Front. Mar. Sci. 9. doi: 10.3389/fmars.2022.818501
Coates D. A., Deschutter Y., Vincx M., Vanaverbeke J. (2014). Enrichment and shifts in macrobenthic assemblages in an offshore wind farm area in the Belgian part of the north Sea. Mar. Environ. Res. 95, 1–12. doi: 10.1016/j.marenvres.2013.12.008
Daewel U., Akhtar N., Christiansen N., Schrum C. (2022). Offshore wind farms are projected to impact primary production and bottom water deoxygenation in the north Sea. Commun. Earth Environ. 3 (1), 1–8. doi: 10.1038/s43247-022-00625-0
De Borger E., Ivanov E., Capet A., Braeckman U., Vanaverbeke J., Grégoire M., et al. (2021). Offshore windfarm footprint of sediment organic matter mineralization processes. Front. Mar. Sci. 8. doi: 10.3389/fmars.2021.632243
Degraer S., Carey D. A., Coolen J. W.P., Hutchison ZoëL., Kerckhof F., Rumes B., et al. (2020). Offshore wind farm artificial reefs affect ecosystem structure and functioning. oceanog 33 (4), 48–57. doi: 10.5670/oceanog.2020.405
Diesing M., Kröger S., Parker R., Jenkins C., Mason C., Weston K. (2017). Predicting the standing stock of organic carbon in surface sediments of the north–West European continental shelf. Biogeochemistry 135 (1), 183–200. doi: 10.1007/s10533-017-0310-4
European Union (2022) European Atlas of the seas. Available at: https://ec.europa.eu/maritimeaffairs/atlas/maritime_atlas/#lang=EN;p=w;bkgd=5;theme=913:0.75;c=299811.1455360078,7181638.012176704.
Global Wind Energy Council (2015). Global Wind Report. Annual Market. Available at: https://gwec.net/global-wind-report-2015/.
Grow (2022) Grow offshore wind project simox. Available at: https://www.grow-offshorewind.nl/project/simox.
Hiscock K., Tyler-Walters H., Jones H. (2002). High level environmental screening study for offshore wind farm developments–marine habitats and species project (Marine Biological Association to The Department of Trade and Industry New & Renewable Energy Programme). (AEA Technology, Environment Contract: W/35/00632/00/00.). Available at: https://tethys.pnnl.gov/sites/default/files/publications/Hiscock_et_al._2002.pdf.
Ivanov E., Capet A., Barth A., Delhez E. J. M., Soetaert K., Grégoire M. (2020). Hydrodynamic variability in the southern bight of the north Sea in response to typical atmospheric and tidal regimes. benefit of using a high resolution model. Ocean Model. 154, 101682. doi: 10.1016/j.ocemod.2020.101682
Ivanov E., Capet A., Borger E. de, Degraer S., Delhez E. J.M., Soetaert K., et al. (2021). Offshore wind farm footprint on organic and mineral particle flux to the bottom. Front. Mar. Sci. 8. doi: 10.3389/fmars.2021.631799
Krone R., Gutow L., Joschko T. J., Schröder A. (2013). Epifauna dynamics at an offshore foundation–implications of future wind power farming in the north Sea. Mar. Environ. Res. 85, 1–12. doi: 10.1016/j.marenvres.2012.12.004
Kruse M., Lindaas J. C., Olivares A., Korporaal H., Keijzer D., Ring H. (2019) Market analysis decom tools 2019. Available at: https://northsearegion.eu/media/11753/market-analysis_decomtools.pdf.
Lovelock C. E., Fourqurean J. W., Morris J. T. (2017). Modeled CO2 emissions from coastal wetland transitions to other land uses: Tidal marshes, mangrove forests, and seagrass beds. Front. Mar. Sci. 4. doi: 10.3389/fmars.2017.00143
Luisetti T., Turner R.K., Andrews J. E., Jickells T. D., Kröger S., Diesing M., et al. (2019). Quantifying and valuing carbon flows and stores in coastal and shelf ecosystems in the UK. Ecosystem Serv. 35, 67–76. doi: 10.1016/j.ecoser.2018.10.013
Maar M., Bolding K., Petersen J. K., Hansen JørgenL. S., Timmermann K. (2009). Local effects of blue mussels around turbine foundations in an ecosystem model of nysted off-shore wind farm, Denmark. J. Sea Res. 62 (2-3), 159–174. doi: 10.1016/j.seares.2009.01.008
Mangi S. C. (2013). The impact of offshore wind farms on marine ecosystems: A review taking an ecosystem services perspective. Proc. IEEE 101 (4), 999–1009. doi: 10.1109/JPROC.2012.2232251
Mavraki N., Coolen J. W.P., Kapasakali D.-A., Degraer S., Vanaverbeke J., Beermann J. (2022). Small suspension-feeding amphipods play a pivotal role in carbon dynamics around offshore man-made structures. Mar. Environ. Res. 178, 105664. doi: 10.1016/j.marenvres.2022.105664
Nghiem A., Pineda Iván (2017). Wind energy in Europe: Scenarios for 2030. Ed. Pierre T. (Wind EUROPE). Available at: https://windeurope.org/wp-content/uploads/files/about-wind/reports/Wind-energy-in-Europe-Scenarios-for-2030.pdf.
OSPAR Commission (2008). Assessment of the environmental impact of offshore wind-farms. In OSPAR Biodiversity Series. Available at: https://qsr2010.ospar.org/media/assessments/p00385_Wind-farms_assessment_final.pdf.
RWE (2022) Der konzern. Available at: https://www.rwe.com/der-konzern/laender-und-standorte/windpark-amrumbank.
Slavik K., Lemmen C., Zhang W., Kerimoglu O., Klingbeil K., Wirtz K. W. (2019). The large-scale impact of offshore wind farm structures on pelagic primary productivity in the southern north Sea. Hydrobiologia 845 (1), 35–53. doi: 10.1007/s10750-018-3653-5
Smeaton C., Hunt C. A., Turrell W. R., Austin W. E.N. (2021). Marine sedimentary carbon stocks of the united kingdom’s exclusive economic zone. Front. Earth Sci. 9. doi: 10.3389/feart.2021.593324
Spielmann V. B. T., Dannheim J., Vajhøj J., Ebojie M., Klein J., Eckardt S. (2021). Integration of sustainability, stakeholder and process approaches for sustainable offshore wind farm decommissioning. Renewable Sustain. Energy Rev. 147, 111222. doi: 10.1016/j.rser.2021.111222
Szulecki K., Fischer S., Gullberg A. T., Sartor O. (2016). Shaping the ‘Energy union’: Between national positions and governance innovation in EU energy and climate policy. Climate Policy 16 (5), 548–567. doi: 10.1080/14693062.2015.1135100
United Nations (2015). “Adoption of the Paris agreement,” in Proposal by the President, Draft decision in Conference of the Parties. Twenty-First Session, New York, NY. Available at: https://unfccc.int/resource/docs/2015/cop21/eng/l09r01.pdf.
Voormolen J. A., Junginger H. M., van Sark W.G.J.H.M. (2016). Unravelling historical cost developments of offshore wind energy in Europe. Energy Policy 88, 435–444. doi: 10.1016/j.enpol.2015.10.047
Wilson R. (2017) A synthetic map of the northwest European shelf sedimentary environment for applications in marine science. With assistance of Robert Wilson, Michael heath, Douglas speirs, alessandro sabatino. Available at: https://pureportal.strath.ac.uk/en/datasets/data-for-a-synthetic-map-of-the-northwest-european-shelf-sediment.
Keywords: construction phase, decommissioning, operational phase, wind park, offshore wind turbine (OWT) monopile, sediment disturbance, enviromental impacts
Citation: Heinatz K and Scheffold MIE (2023) A first estimate of the effect of offshore wind farms on sedimentary organic carbon stocks in the Southern North Sea. Front. Mar. Sci. 9:1068967. doi: 10.3389/fmars.2022.1068967
Received: 13 October 2022; Accepted: 30 December 2022;
Published: 16 January 2023.
Edited by:
Nathan John Waltham, James Cook University, AustraliaReviewed by:
Rene Friedland, Leibniz Institute for Baltic Sea Research (LG), GermanyCopyright © 2023 Heinatz and Scheffold. This is an open-access article distributed under the terms of the Creative Commons Attribution License (CC BY). The use, distribution or reproduction in other forums is permitted, provided the original author(s) and the copyright owner(s) are credited and that the original publication in this journal is cited, in accordance with accepted academic practice. No use, distribution or reproduction is permitted which does not comply with these terms.
*Correspondence: Knut Heinatz, a251dC5oZWluYXR6QHN0dWRpdW0udW5pLWhhbWJ1cmcuZGU=
Disclaimer: All claims expressed in this article are solely those of the authors and do not necessarily represent those of their affiliated organizations, or those of the publisher, the editors and the reviewers. Any product that may be evaluated in this article or claim that may be made by its manufacturer is not guaranteed or endorsed by the publisher.
Research integrity at Frontiers
Learn more about the work of our research integrity team to safeguard the quality of each article we publish.