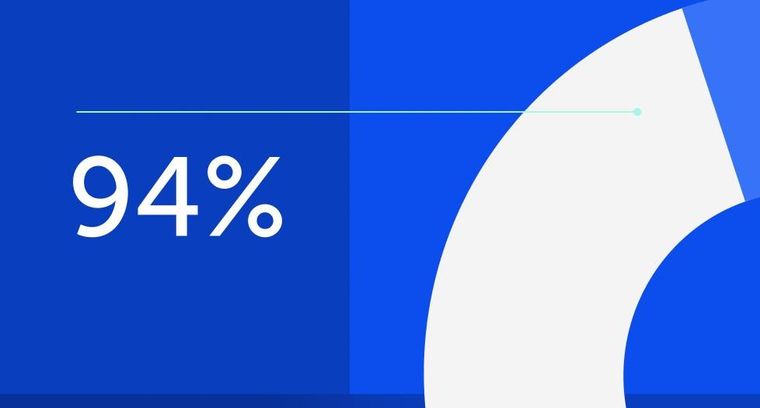
94% of researchers rate our articles as excellent or good
Learn more about the work of our research integrity team to safeguard the quality of each article we publish.
Find out more
ORIGINAL RESEARCH article
Front. Mar. Sci., 21 December 2022
Sec. Marine Biology
Volume 9 - 2022 | https://doi.org/10.3389/fmars.2022.1055404
This article is part of the Research TopicBiological Invasions in Marine and Estuarine EcosystemsView all 9 articles
One of the consequences of climate change and globalization is the recent proliferation of the invasive blue crab Callinectes sapidus in the Mediterranean Sea. In this study, C. sapidus thermal tolerance was investigated through experiments based on species metabolic response (measuring respiration rates) to a wide temperature range. Based on metabolic rates, Thermal Habitat Suitability (THS) maps were performed on current and futures temperature conditions in the Mediterranean Sea. Thermal Performance Curve showed a CTmax at 40°C and an optimum at 24°C. Respiration rate increased between 12°C and 24°C and decreased until 30°C. At the highest temperatures (> to 30°C) a pointed increase in the respiration rate values was observed from 32°C before the organisms’ death at 40°C. Predictive maps showed that the whole basin shows suitable conditions for population maintenances of C. sapidus in all used warming scenarios. The future scenarios show a mean increase of +0.2 of the THS over the year. The present study increases the understanding of the ecological performance and potential distribution of C. sapidus. This information will contribute to the design and implementation of risk assessment and management plans of this impactful crustacean in the Mediterranean Sea.
The expansion of marine species is a consequence of climate change and human activities (Ramos, 2010; Gallardo et al., 2017; Ramos et al., 2018; Seebens et al., 2021). The global rise in ocean temperatures drive and affect organismal performance, population dynamics and ecosystem structure (Yao and Somero, 2014; Ramos et al., 2018) producing an expansion of organisms towards the poles and temperate zones as a response of their adaptiveness (Sarà et al., 2013b; 2018). Changes in temperature regimes, surface currents, ice cover, and other key processes contribute to changes in natural or anthropogenic dispersal of species, promoting the survival and establishment of some species in previously inappropriate locations (Occhipinti-Ambrogi, 2007; Hellmann et al., 2008; Bellard et al., 2018; Chan et al., 2019).Temperature plays a key role in driving biochemical and metabolic reactions of organisms determining thermal tolerance limits, phenology and their ultimate distribution (Walter and Graf, 2002; Helmuth, 2009; Freitas et al., 2010; Yao and Somero, 2014). Differences in environmental exposure may underlie the wide range of physiological responses observed, highlighting the importance of quantifying the effects of climate change across most coastal systems (Cooley et al., 2009).
One of the consequences of the increase in temperature and human stressors, at local level is the spread and recruitment of non-indigenous species (NIS) affecting coastal economies worldwide (Sarà et al., 2018). New introductions of NIS are intensified in recent decades due to rapid globalization and the increasing trends in human activities (shipping, aquaculture, fisheries, tourism, etc.) (Boudouresque and Verlaque, 2002; Katsanevakis et al., 2014). Some authors have considered the NIS spread around the world (Poloczanska et al., 2013; Cheung et al., 2019) as the largest source of biological pollution, significantly affecting recipient ecosystems (Albano et al., 2018; Mangano et al., 2019; Giangrande et al., 2020; Ardura et al., 2021; Seebens et al., 2021). When some of these species become invasive, they often entail strong ecological and socio-economic impacts such as habitat modification, biodiversity loss and a consequent impairment of ecosystem functioning (e.g. trophic interactions) (Gallardo et al., 2016). As a direct consequence of these, NIS are able to modify the capacity of ecosystems to provide ecosystem services and this is often the major cause of net socio-economic losses of the ecosystem-based human activities (e.g. fisheries, industrial activities and tourism) (Katsanevakis et al., 2014; Gallardo et al., 2016; Tsiamis et al., 2018).
The recent proliferation of the invasive blue crab Callinectes sapidus (Rathbun, 1896) in the Mediterranean Sea. Native from the Atlantic American coasts, C. sapidus appeared in Mediterranean Sea in 1949, Black Sea and in the Sea of Azov in 1967 (Nehring, 2011). This species shows a large metabolic plasticity allowing it to settle in a great diversity of habitats (Azra et al., 2020). By benefiting from climate change (Nehring, 2011), the number of new records entering the Basin continues to increase in recent years and this species is now found almost everywhere (Mancinelli et al., 2021) above all in variable environments such as marine and brackish shallow lagoon systems, estuarine and coastal ecosystems (Nehring, 2011; Mancinelli et al., 2017a). The species is considered a strong competitor and predator of other species with the ability to modify the benthic habitats, negatively interacting with the local biodiversity (2017b; Nehring, 2011; Mancinelli et al., 2016). The fast and widespread distribution of C. sapidus across the Mediterranean combined with the relative lack of information regarding the impacts of current and future warming conditions on the species performance, makes pressing to get a credible assessment of the effect of climatic disturbance on the species functional traits.
One tangible measure of how environmental change can affect the niche extent of one species is represented by rates of change of the metabolic machinery that, according to first thermodynamic principles, is driven by external temperature above all in ectotherms/poikilotherms (Gillooly et al., 2001; Gaitán-Espitia et al., 2017). Oxygen consumption rate is the most recognized and effective proxy of metabolic machinery (Pörtner et al., 2017) which generally has a bell-shaped relationship with temperature, as tested if many species (2019; Harley et al., 2017; Flanagan et al., 2018) such as crustaceans (Leffler, 1972; Breteler, 1975; Eriksson and Edlund, 1977; Gutermuth and Armstrong, 1989; Booth and McMahon, 1992) and has been linked to the high ventilation rates observed in this taxon (Frederich and Pörtner, 2000).
The blue crab C. sapidus has a complex life history living both in oceanic and estuarine habitats, and using all salinity regimes to complete the life cycle (Millikin, 1984). Low salinity environments are used for mating and as nursery habitat, while the larval growth is observed in sea-level salinities (Hines et al., 2010). The global warming of the water surface temperature induced by climate change is evident in the Mediterranean Sea, especially in the Sicily strait (Bonino et al., 2022; Juza et al., 2022), and may have positively affected the expansion and the invasive success of the C. sapidus as well as their maturation rate, brood production, and fitness (Mancinelli et al., 2017a; 2021).
In this study, the thermal performance of the adult stage of C. sapidus was investigated using the metabolic response as expressed by the relationship with the oxygen consumption. Also, this study spatially-contextualizes the thermal niche of the species under current and predicted future climate conditions in the Mediterranean Sea.
To define the thermal window of C. sapidus to be investigated in the experiments, the worldwide geographic distribution of the species records was extracted from the Global Biodiversity Information Facility (https://www.gbif.org/) through the R function “rgbif”. Such points were intersected with the in-situ temperature (minimum, maximum, mean) downloaded from Copernicus project website (http://marine.copernicus.eu/; daily means from 2017 to 2019). The density of occurrences was calculated and afterwards plotted as a function of temperature using R Studio software (version 2021.09.0).
To investigate the thermal tolerance of C. sapidus, individual respiration rate (RR, mgO2 h-1 gWW-1) was estimated as a proxy of the metabolism (Angilletta et al., 2010; Sokolova et al., 2012; Gould et al., 2021) at 13 different temperatures: 12°C, 16°C, 20°C, 22°C, 24°C, 26°C, 28°C, 30°C, 32°C, 34°C, 36°C, 38°C and 40°C. The lowest temperature at 12°C corresponded to the thermal limits of the Mediterranean Sea surface temperature which was not below 12°C in the Sicily seas (Reyes Suarez et al., 2019).
In May 2022 (period when the population has completed the growth; G. Marchessaux, unpublished data), 80 specimens (mean wet weight: 105 ± 39 g; mean carapace width: 10.7 ± 1.4 cm) of blue crabs C. sapidus were collected using hand nets in the saltmarshes (named “Salina Grande”; 37°57’13.7”N, 12°29’51.3”E) in the “Saline di Trapani e Paceco” Nature Reserve, Sicily (Italy). During the transport, organisms were placed in different coolers (Volume: 50 L, 6 organisms per each cooler) and oxygenated with an air pump. Organisms were brought back to the Laboratory of Ecology in Palermo (Sicily, Italy) within 2 h, and acclimated to the aquarium conditions (60 L; temperature: 22°C; salinity: 35 psu) for 48 hours.
After having made sure of their good morphological conditions (whole organisms, no missing organs [claws, legs, etc.]), individuals were randomly divided in 13 groups (n=6) according to the number of experimental temperatures. The thermal tolerance experiments were performed starting from the sampling water temperature (22°C), the temperature in each group was increased/decreased to the following level at a rate of 1°C per hour (2022; Prusina et al., 2014; Bosch-Belmar et al., 2021). Individuals were acclimatized for 24 h after reaching the 13 experimental temperatures.
After the acclimatization to the experimental temperature, oxygen consumption measurements as a function of different temperatures were performed. The six individuals belonged to every temperature set were individually placed within a respirometric chamber (2 300 ml) containing filtered (Whatman GF/C 0.45 μm) air-saturated, 35 psu seawater. Two other respirometric chambers were filled only with filtered seawater and used as controls. To ensure the constant mixing of the water, each chamber was stirred with a magnet bar and an individual stirring device (2022; Bosch-Belmar et al., 2021). The respirometric chambers were then randomly distributed in two temperature-controlled circulated stable water baths (Grant Optima TX150). The controlled chiller system and the thermostatic heater were used to cool/heat the water and maintain the experimental temperatures. Temperature was recorded throughout the recording period through the HOBO Pendant® loggers (mod. MX2201, ± 0.5°C accuracy). The dissolved oxygen concentration was measured simultaneously with 3 optical oxygen meters (Pyro Science Firesting O2) each equipped with four optodes (12 optodes in total). Oxygen consumption was measured in continuum for 1 hour.
Respiration rate was calculated according to Sarà et al. (2013a): RR=(Ct0− Ct1) Volr 60 (t1−t0)−1 where “Ct0” is oxygen concentration at the beginning of the measurement, “Ct1” is the oxygen concentration at the end of the measurement, and “Volr” is the volume of water in the respirometric chamber.
After respiration rate measurements, biometric parameters of carapace width (CW, as the widest point behind the posterior anterolateral spines, in cm), carapace length (CL, as the distance between the center of the anterior interorbital margin, and the center of the posterior margin, in cm), and wet weight (WW, in g) of all organisms were recorded. The respiration rate was reported to the individuals’ wet weight to control the effect of the body mass on the respiration rate.
To identify the thermal tolerance of C. sapidus Thermal Performance Curve (TPC) model regression was performed using the “rTPC” R package (Padfield and O’Sullivan, 2020) launching and comparing a total of 24 different non-linear least-squares TPC models (Supplementary Figure 1) (Padfield and O’Sullivan, 2020). The “best” fitting model was identify based of the lowest AIC scores (Padfield and O’Sullivan, 2020; Bosch-Belmar et al., 2022). The TPC was drawn, and then the bootstrapping was performed to calculate 95% prediction limits for the selected TPC model and confidence intervals around its temperature optimum. The respiration rates related to the shift from the optimal to the pejus temperature range (e.g. thermal stress; > 30°C in our study) (Sokolova et al., 2012; Prusina et al., 2014) were removed for the TPC (Thermal Performance Curve) analysis since they only represent a stress and not an inhibition of the metabolism linked to high temperatures (Jost et al., 2012; Sokolova et al., 2012; Prusina et al., 2014; Bosch-Belmar et al., 2022).
To test the differences in respiration rates between temperatures between the optimum obtained in the model and the other temperatures tested, an ANOVA and a Bonferroni post-hoc test (significant difference = p< 0.05) were performed using R Studio (version 2021.09.0).
The model parameters were used to predict the current and future potential occurrence of C. sapidus based on the adaptation of respiration rate to the future increase of temperature (Sakallı, 2017; Di Biagio et al., 2020). Parameters related to the maximum respiration rate (rmax), the optimal temperature (Topt) and the full curve width (a) were extracted from the TPC model and used in the thermal habitat suitability maps. The equation curve of the “best TPC model” was applied to predict the potential occurrence of the blue crab C. sapidus in the present-day climatic conditions (year 2019, data extracted from Copernicus project website (http://marine.copernicus.eu/).), and in two 2050 future scenarios of greenhouse gas (GHG) emissions (RCP (Representative Concentration Pathway), RCP4.5 and RCP8.5; outputs from Bio-ORACLE V2.0 (Assis et al., 2018, www.bio-oracle.org/). To convert the TPC in thermal habitat suitability (THS) maps, data on experimentally measured respiration rate was converted into probability of occurrence (between 0 and 1) at different in situ temperatures. To predict the Thermal Habitat Suitability (THS) the different oxygen consumption values were scaled to the RR value at the temperature optimum predicted by the model, using the following formula according to Bosch-Belmar et al. (2022): THS= RRt/RROpt where THS was the occurrence probability, RRt the respiration rate value at temperature t, and RROpt the value of respiration rate at the optimum temperature predicted by the best TPC model chosen. The THS, comprised between 0 and 1, were calculated at pixel level (1 km2) using the current and future environmental values (Bosch-Belmar et al., 2022) for the first 40 m depth, corresponding to the depth range of C. sapidus (Mancinelli et al., 2017a). The obtained maps were exported in raster format. To identify the monthly difference between the current and future scenarios, subtractions between future and present raster layers were performed and the results were presented in histograms using R Studio (version 2021.09.0).
The occurrence of Callinectes sapidus was observed over a wide range of temperatures (Figure 1) from 7°C to 32°C (number of records: 52 323). The highest densities were observed at 12°C (the minimum temperature), at 23°C (average temperature) and at 32°C (the maximum temperature). The thermal window of C. sapidus was large with a possible optimum around 22-23°C based on the world scale occurrences (Figure 1).
Figure 1 Density of Callinectes sapidus occurrences as a function of temperature (minimum, maximum, mean). Number of = 52 323 records.
Temperature significantly affected the respiratory rates (Figure 2). Respiration rate (mean ± standard deviation) measured in acclimated crabs increased from 0.04 ± 0.01 mgO2 h-1 gWW-1 at 12°C to 0.15 ± 0.03 mgO2 h-1 gWW-1 at 24°C. Between 24°C and 30°C, the oxygen consumption decreased from 0.13 ± 0.03 mgO2 h-1 gWW-1 (26°C) to 0.10 ± 0.02 mgO2 h-1 gWW-1 (30°C). At the highest tested temperatures (> to 30°C) a pointed increase in the respiration rate values was observed from 32°C (0.19 ± 0.05 mgO2 h-1 gWW-1) to 38°C (0.26 ± 0.03 mgO2 h-1 gWW-1) before the organisms’ death at 40°C (100% of mortality) (Figure 2). Such a response between 32 and 38°C may be related to the shift from the optimal to the pejus temperature range.
Figure 2 Respiration rate (RR; mgO2 h–1 gWW–1; WW: wet weight) of Callinectes sapidus as a function of the temperature measured experimentally. The blue line represents the mean thermal performance curve (TPC) regression. The red indications explain the trends and the metabolic responses to the different temperatures.
Among other, Flinn regression (Flinn, 1991) realized the “best fitting” model presenting the lowest AIC score (see Supplementary Figure 2; Supplementary Tables 2, 3 for details). Thus, the thermal performance curve (TPC) obtained from our laboratory experimental measurements of the fitted Flinn model and estimated in this specific Mediterranean population, showed a thermal optimum (Topt) for C. sapidus at 24°C (Figure 3A); respiration rate significantly different (Supplementary Table 4) with the other temperatures (12°C-30°C).
Figure 3 (A) Thermal Performance Curve (TPC) according the Flinn model based on the respiration rates (mgO2 h–1 gWW–1). The blue band represent the 95% confidence interval; (B) Thermal habitat suitability (THS) scale within the TPC of Callinectes sapidus ranging from low (0) to high (1) species thermal suitability probability. Different colors (representing the species THS) would correspond to: optimum temperature range (green colored), lower and upper pejus ranges (light green and yellow colored), pessimum (orange colored), and lethal ranges (red colored) (Sokolova et al., 2012).
Based on this estimated experimentally metabolic rate range (Figure 3B), predictive metabolic maps showed that the Thermal Habitat Suitability (THS) of C. sapidus in the Mediterranean Sea may seasonally vary among different basin sections (Figure 4). For example, the autumn and winter temperatures (between October and March) were only suitable along the southeastern coasts of the Mediterranean from the Gabès Gulf (Tunisia) to the Levantine Basin (Thermal Habitat Suitability, THS between 0.4 and 0.6). Instead, the spring (from April to June) was optimal for C. sapidus along the south coast and in the Aegean Sea (THS > 0.6); in June and July, temperatures were particularly optimal for the species in the whole Mediterranean Sea (THS > 0.8). The THS were mostly optimal (THS > 0.8) along the Northern coasts (from Spain to Turkey) of the Mediterranean between August and October. Finally, our analysis showed a decrease of THS in the northern part of the Mediterranean Sea in November and December in contrast to the southern coasts where high THS (> 0.8) were observed until December.
Figure 4 Current predicted Thermal Habitat Suitability (THS) of Callinectes sapidus in the Mediterranean Sea. The graph below represents the Thermal habitat suitability (THS) scale within the TPC of Callinectes sapidus ranging from low (0) to high (1) species thermal suitability probability. Different colors (representing the THS scale) would correspond to: optimum temperature range (green colored), lower and upper pejus ranges (light green and yellow colored), pessimum (orange colored), and lethal ranges (red colored).
Maps illustrating the distributional predictions under the RCP 4.5 and 8.5 (2050) scenarios showed a spatial increase of monthly THS for C. sapidus (Figures 5, 6). Akin trends as in the current scenario were observed in the southern and northern Mediterranean for both futures scenarios. The main difference was an increase of the THS (from 0.2-0.4 to 0.6) in the Gulf of Gabès (Tunisia), the Adriatic Sea, the Tyrrhenian and Sicilian Seas during the winter periods under the future warming scenarios. The summer optimal periods were longer than in the current scenario with THS > 0.6 from July to November. For both future scenarios, the monthly THS increased by an average of +0.2 (Supplementary Figures 3, 4).
Figure 5 Future 2050 RCP 4.5 predicted Thermal Habitat Suitability (THS) of Callinectes sapidus in the Mediterranean Sea. The graph below represents the Thermal habitat suitability (THS) scale within the TPC of Callinectes sapidus ranging from low (0) to high (1) species thermal suitability probability. Different colors (representing the THS scale) would correspond to: optimum temperature range (green colored), lower and upper pejus ranges (light green and yellow colored), pessimum (orange colored), and lethal ranges (red colored).
Figure 6 Future 2050 RCP 8.5 predicted Thermal Habitat Suitability (THS) of Callinectes sapidus in the Mediterranean Sea. The graph below represents the Thermal habitat suitability (THS) scale within the TPC of Callinectes sapidus ranging from low (0) to high (1) species thermal suitability probability. Different colors (representing the THS scale) would correspond to: optimum temperature range (green colored), lower and upper pejus ranges (light green and yellow colored), pessimum (orange colored), and lethal ranges (red colored).
The invasive success of the blue crab Callinectes sapidus in the Mediterranean Sea is due to the high physiological plasticity and the capacity to benefit of local and changing conditions due to global increasing of temperature (Edgell and Hollander, 2011; Nehring, 2011). Our study permitted to investigate of the Thermal Performance Curve (TPC) and the identification of thermal thresholds for C. sapidus.
The respiration rates measured in this study were in the same ranges with the limited datasets available on the effects of temperature on the oxygen consumption rate of C. sapidus in its native areas (Leffler, 1972; Laird and Haefner, 1976; Booth and McMahon, 1992; Brylawski and Miller, 2003). Indeed, to date there are no studies presenting the thermal tolerance of C. sapidus in its native range and in areas where the species is introduced. For example, the populations studied in the literature were mostly from estuarine waters of the North Atlantic (native range), while the population studied in our study came from Mediterranean coastal environments where C. sapidus was introduced only some decades ago. Across the current the literature, there is no reported experimentally clear optimum for the native areas, although there is evidence of respiration rates falling in the same ranges of those measured in the Mediterranean. Overall, apart from the extreme values observed by Leffler (1972), respiration rates were similar between studies (previous published literature and our study), indicating a predictable increase in oxygen consumption rate up to high temperature levels with two different optimum depending on population geographic location: (i) one thermal optimum at 34°C estimated in native areas based on literature data extracted during a meta-analysis (Brylawski & Miller, 2003), and a new one (measured experimentally) at 24°C in the introduced area (Mediterranean Sea, present study). Further research is needed on the adaptation mechanisms responsible to the discrepancy between what was obtained in the native range and data coming from our experiments with individuals collected in our study area. Nonetheless, due to our experimental evidence produced in this study, we are forced to trait with skepticism literature data indicating an optimum at 34°C in the native range, seen such an observation was done in the native range whose mean temperature is much colder than what is usually observed in our Mediterranean study areas. Moreover, our experimental optimum (24°C) identified for Mediterranean populations coincides with the thermal optimum (23-24°C) obtained from the performed density plot based on species GBIF occurrence records (Figure 1). Several authors agree on the correlation between species occurrence (and the relative putative abundance) and the environmental suitability, which fixes that a species exhibit greater aggregations where the environment is more suitable (Weber et al., 2017; de la Fuente et al., 2021). This further corroborates our data showing the thermal optimum of the species around 24°C. Thus, we are confident in saying that our experimental thermal data fully confirmed what we derived from the occurrence density around of the Mediterranean Sea. Indeed, the positive effect of temperature on the respiration rates of the blue crab C. sapidus is now established and provides major precision in getting metabolic estimates, which is a good proxy of invasive capabilities in the Mediterranean. This is crucial when assessing how temperature influences metabolism and to infer on how species is able to maintain itself and colonize new areas. In fact, the levels of temperature treatment in this study were chosen based on the potential realized niche ranges observed for C. sapidus, as well as including high environmental temperatures representatives of the future changes expected especially in the summer period in coastal environments and more globally in the Mediterranean Sea. The Flinn thermal performance curve model identified the critical maximum temperature (CTmax) for Mediterranean C. sapidus individuals at 40°C, in agreement with previous published data reporting lethal temperatures for the species at 39-40°C (Brylawski and Miller, 2003) and survival data coming from the current work (crabs mortality was recorded at 40°C). The large window of thermal tolerance for blue crabs C. sapidus may be explained by the adaptation to temperatures of the Western Sicily salt ponds (the sites from where crabs flourish and where individuals for present experiments were collected) that due to the shallowness of ponds can go over 30°C in August (Bellino et al., 2019; G. Marchessaux personal data) or even higher (33°C) during multiple extreme climatic events (Sarà et al., 2021).
Lagoon and estuarine systems are particularly affected by increasing temperature due to global change. There, temperature varies rapidly on short spatial and temporal scales (both seasonal and daily) (Brito et al., 2012). Thus, physiological plasticity and tolerance to wide temperature ranges are advantageous for species such as blue crabs living in these habitats. For example, during migrations from the sea to lagoon and estuarine systems, juvenile blue crabs are exposed to highly changing environmental conditions (Etherington and Eggleston, 2003). Variability in physiological variables such as oxygen consumption rate and broad thermal tolerance may therefore help crabs to survive this migration and could contribute to population success in the face of a changing climate. Callinectes sapidus species can (i) survive and thrive as the climate continues to change in the future (Azra et al., 2018) and (ii) spread in new non-natives environments as observed in the Mediterranean Sea. Based on the thermal suitability mapped at the Mediterranean Sea scale it appeared that the current thermal ranges offered by this region are suitable for C. sapidus. The probable increase of temperature in a close future could increase the presence probability of this species all the year in the areas where the species is.
Invasive species are of great concern, especially in marine ecosystems, as they are considered a global threat to local biodiversity (Bax et al., 2003; Costello et al., 2015). Previous studies have shown that heat tolerance plays an important role in the successful invasion of an aquatic invertebrate (Iftikar et al., 2010; Bates et al., 2013; Kelley, 2014; Azra et al., 2020; Bosch-Belmar et al., 2022). The data from this study, combined with previous work on the effects of temperature increase on adult blue crabs in a Mediterranean lagoon, provides a window into the overall effect of environmental change on the performance and potential distribution of this highly invasive species in the Mediterranean Sea. Understanding the metabolic linked aspects as expressed by a simple functional trait such as the oxygen consumption rate, allows for a better understanding of the habitats favorable to the establishment of C. sapidus populations.
Increasing our understanding of the ecological role of an invasive species in affecting the ecosystem functioning is essential for the future management measures of biodiversity, especially at cross-border scale. The use of trait-based information, such that used here, to improve management tools for ecosystem conservation and management of ecosystem-based economic activities has recently increased (Anton et al., 2010; Truchy et al., 2015). The creation of basal layers based on species functional traits following mechanistic approaches and its integration within traditional management tools (such as correlative species distribution models) (2022; Talluto et al., 2016; Gamliel et al., 2020; Bosch-Belmar et al., 2021), may generate more feasible and ecologically informed management measures increasing our ability to predict ecosystems responses to climate change (Mangano et al., 2019)
Temperature is a key environmental factor influencing the ectotherms metabolism. The thermal suitability maps of this study can be an effective visual tool to assist conservation managers and practitioners in addressing biodiversity conservation measures and for detecting current and future specific areas of vulnerability. Mapping the suitable thermal habitats will enable effective preventive and mitigation measures such as, the removal of individuals and/or to implement Early Detection Response (EDR) frameworks supporting practitioners in assessing changes in species composition and evaluating the status of vulnerable and ecosystems (Butchart et al., 2010; McGeoch et al., 2010). Visualizing the metabolic distribution through maps helps to identify the emergence of new conditions conducive to the introduction of NIS, and to address the risks associated with policy making and administration at national and European levels (Hulme et al., 2008). Furthermore, these results can be easily used to predict thermal suitable habitats and predict the invasion probability. Baseline information on species thermal tolerance and thresholds is of vital importance to improve our ability to predict effects and consequences of future changing environmental conditions on organisms’ performance, phenology and distributions.
The original contributions presented in the study are included in the article/Supplementary Material. Further inquiries can be directed to the corresponding author.
GM, MB-B, MCM, and GS contributed to the conception of the study. GM, MB-B, LC, and GS designed the study. GM, NM, and NL performed the experiments. GM, MB-B, and GS contributed to the data analyses. GM and MB-B developed the metabolic suitability prediction maps. GM wrote the first version of the manuscript. All authors contributed to manuscript revision, read, and approved the submitted version.
This study was founded by the BLEU-ADAPT project supported by Interreg Italy-Tunisia Program 2014-2020, Ref. n° IS_3.1_021. M.C.M.'s research activity was supported by the European Union's Horizon 2020 Research and Innovation programme under the Marie Skłodowska-Curie Action (Grant agreement no. 835589, MIRROR Project).
The authors declare that the research was conducted in the absence of any commercial or financial relationships that could be construed as a potential conflict of interest.
All claims expressed in this article are solely those of the authors and do not necessarily represent those of their affiliated organizations, or those of the publisher, the editors and the reviewers. Any product that may be evaluated in this article, or claim that may be made by its manufacturer, is not guaranteed or endorsed by the publisher.
The Supplementary Material for this article can be found online at: https://www.frontiersin.org/articles/10.3389/fmars.2022.1055404/full#supplementary-material
Albano P. G., Gallmetzer I., Haselmair A., Tomašovỳch A., Stachowitsch M., Zuschin M. (2018). Historical ecology of a biological invasion: the interplay of eutrophication and pollution determines time lags in establishment and detection. Biol. Invasion. 20, 1417–1430. doi: 10.1007/s10530-017-1634-7
Angilletta M. J., Cooper B. S., Schuler M. S., Boyles J. G. (2010). The evolution of thermal physiology in endotherms. Front. Biosci.-Elite 2, 861–881. doi: 10.2741/e148
Anton C., Young J., Harrison P. A., Musche M., Bela G., Feld C. K., et al. (2010). Research needs for incorporating the ecosystem service approach into EU biodiversity conservation policy. Biodivers. Conserv. 19, 2979–2994. doi: 10.1007/s10531-010-9853-6
Ardura A., Fernandez S., Haguenauer A., Planes S., Garcia-Vazquez E. (2021). Ship-driven biopollution: How aliens transform the local ecosystem diversity in pacific islands. Mar. pollut. Bull. 166, 112251. doi: 10.1016/j.marpolbul.2021.112251
Assis J., Tyberghein L., Bosch S., Verbruggen H., Serrão E. A., De Clerck O. (2018). Bio-ORACLE v2. 0: Extending marine data layers for bioclimatic modelling. Glob. Ecol. Biogeogr. 27, 277–284. doi: 10.1111/geb.12693
Azra M. N., Aaqillah-Amr M. A., Ikhwanuddin M., Ma H., Waiho K., Ostrensky A., et al. (2020). Effects of climate-induced water temperature changes on the life history of brachyuran crabs. Rev. Aquac. 12, 1211–1216. doi: 10.1111/raq.12380
Azra M. N., Chen J.-C., Ikhwanuddin M., Abol-Munafi A. B. (2018). Thermal tolerance and locomotor activity of blue swimmer crab portunus pelagicus instar reared at different temperatures. J. Therm. Biol. 74, 234–240. doi: 10.1016/j.jtherbio.2018.04.002
Bates A. E., McKelvie C. M., Sorte C. J., Morley S. A., Jones N. A., Mondon J. A., et al. (2013). Geographical range, heat tolerance and invasion success in aquatic species. Proc. R. Soc B. Biol. Sci. 280, 20131958. doi: 10.1098/rspb.2013.1958
Bax N., Williamson A., Aguero M., Gonzalez E., Geeves W. (2003). Marine invasive alien species: a threat to global biodiversity. Mar. Policy 27, 313–323. doi: 10.1016/S0308-597X(03)00041-1
Bellard C., Jeschke J. M., Leroy B., Mace G. M. (2018). Insights from modeling studies on how climate change affects invasive alien species geography. Ecol. Evol. 8, 5688–5700. doi: 10.1002/ece3.4098
Bellino A., Mangano M. C., Baldantoni D., Russell B. D., Mannino A. M., Mazzola A., et al. (2019). Seasonal patterns of biodiversity in Mediterranean coastal lagoons. Divers. Distrib. 25, 1512–1526. doi: 10.1111/ddi.12942
Bonino G., Masina S., Galimberti G., Moretti M. (2022). Southern Europe and Western Asia marine heat waves (SEWA-MHWs): a dataset based on macro events. Earth Syst. Sci. Data Discuss. 1–19. doi: 10.5194/essd-2022-343
Booth C. E., McMahon B. R. (1992). Aerobic capacity of the blue crab, callinectes sapidus. Physiol. Zool. 65, 1074–1091. doi: 10.1086/physzool.65.6.30158269
Bosch-Belmar M., Giommi C., Milisenda G., Abbruzzo A., Sarà G. (2021). Integrating functional traits into correlative species distribution models to investigate the vulnerability of marine human activities to climate change. Sci. Total Environ. 799, 149351. doi: 10.1016/j.scitotenv.2021.149351
Bosch-Belmar M., Piraino S., Sarà G. (2022). Predictive metabolic suitability maps for the thermophilic invasive hydroid pennaria disticha under future warming Mediterranean Sea scenarios. Front. Mar. Sci. 9. doi: 10.3389/fmars.2022.810555
Boudouresque C. F., Verlaque M. (2002). Biological pollution in the Mediterranean Sea: invasive versus introduced macrophytes. Mar. pollut. Bull. 44, 32–38. doi: 10.1016/S0025-326X(01)00150-3
Breteler W. K. (1975). Laboratory experiments on the influence of environmental factors on the frequency of moulting and the increase in size at moulting of juvenile shore crabs, carcinus maenas. Neth. J. Sea Res. 9, 100–120. doi: 10.1016/0077-7579(75)90025-3
Brito A. C., Newton A., Tett P., Fernandes T. F. (2012). How will shallow coastal lagoons respond to climate change? a modelling investigation. Estuar. Coast. Shelf Sci. 112, 98–104. doi: 10.1016/j.ecss.2011.09.002
Brylawski B. J., Miller T. J. (2003). Bioenergetic modeling of the blue crab (Callinectes sapidus) using the fish bioenergetics (3.0) computer program. Bull. Mar. Sci. 72, 491–504.
Butchart S. H., Walpole M., Collen B., Van Strien A., Scharlemann J. P., Almond R. E., et al. (2010). Global biodiversity: indicators of recent declines. Science 328, 1164–1168. doi: 10.1126/science.1187512
Chan F. T., Stanislawczyk K., Sneekes A. C., Dvoretsky A., Gollasch S., Minchin D., et al. (2019). Climate change opens new frontiers for marine species in the Arctic: Current trends and future invasion risks. Glob. Change Biol. 25, 25–38. doi: 10.1111/gcb.14469
Cheung W., Ota Y., Cisneros-Montemayor A. (2019). Predicting future oceans: Sustainability of ocean and human systems amidst global environmental change (Elsevier).
Cooley S. R., Kite-Powell H. L., Doney S. C. (2009). Ocean acidification’s potential to alter global marine ecosystem services. Oceanography 22, 172–181. doi: 10.5670/oceanog.2009.106
Costello M. J., Claus S., Dekeyzer S., Vandepitte L., Tuama E. O., Lear D., et al. (2015). Biological and ecological traits of marine species. PeerJ 3, e1201. doi: 10.7717/peerj.1201
de la Fuente A., Hirsch B. T., Cernusak L. A., Williams S. E. (2021). Predicting species abundance by implementing the ecological niche theory. Ecography 44, 1723–1730. doi: 10.1111/ecog.05776
Di Biagio V., Cossarini G., Salon S., Solidoro C. (2020). Extreme event waves in marine ecosystems: an application to Mediterranean Sea surface chlorophyll. Biogeosciences 17, 5967–5988. doi: 10.5194/bg-17-5967-2020
Edgell T. C., Hollander J. (2011). “The evolutionary ecology of European green crab, carcinus maenas, in north America,” in In the wrong place-alien marine crustaceans: Distribution, biology and impacts (Dordrecht: Springer), 641–659.
Eriksson S., Edlund A.-M. (1977). On the ecological energetics of 0-group carcinus maenas (L.) from a shallow sandy bottom in gullmar fjord, Sweden. J. Exp. Mar. Biol. Ecol. 30, 233–248. doi: 10.1016/0022-0981(77)90033-8
Etherington L. L., Eggleston D. B. (2003). Spatial dynamics of large-scale, multistage crab (Callinectes sapidus) dispersal: determinants and consequences for recruitment. Can. J. Fish. Aquat. Sci. 60, 873–887. doi: 10.1139/f03-072
Flanagan S. P., Forester B. R., Latch E. K., Aitken S. N., Hoban S. (2018). Guidelines for planning genomic assessment and monitoring of locally adaptive variation to inform species conservation. Evol. Appl. 11, 1035–1052. doi: 10.1111/eva.12569
Flanagan P. H., Jensen O. P., Morley J. W., Pinsky M. L. (2019). Response of marine communities to local temperature changes. Ecography 42, 214–224. doi: 10.1111/ecog.03961
Flinn P. W. (1991). Temperature-dependent functional response of the parasitoid cephalonomia waterstoni (Gahan)(Hymenoptera: Bethylidae) attacking rusty grain beetle larvae (Coleoptera: Cucujidae). Environ. Entomol. 20, 872–876. doi: 10.1093/ee/20.3.872
Frederich M., Pörtner H. O. (2000). Oxygen limitation of thermal tolerance defined by cardiac and ventilatory performance in spider crab, maja squinado. Am. J. Physiol.-Regul. Integr. Comp. Physiol. 279, R1531–R1538. doi: 10.1152/ajpregu.2000.279.5.R1531
Freitas V., Cardoso J. F., Lika K., Peck M. A., Campos J., Kooijman S. A., et al. (2010). Temperature tolerance and energetics: a dynamic energy budget-based comparison of north Atlantic marine species. Philos. Trans. R. Soc B. Biol. Sci. 365, 3553–3565. doi: 10.1098/rstb.2010.0049
Gaitán-Espitia J. D., Bacigalupe L. D., Opitz T., Lagos N. A., Osores S., Lardies M. A. (2017). Exploring physiological plasticity and local thermal adaptation in an intertidal crab along a latitudinal cline. J. Therm. Biol. 68, 14–20. doi: 10.1016/j.jtherbio.2017.02.011
Gallardo B., Aldridge D. C., González-Moreno P., Pergl J., Pizarro M., Pyšek P., et al. (2017). Protected areas offer refuge from invasive species spreading under climate change. Glob. Change Biol. 23, 5331–5343. doi: 10.1111/gcb.13798
Gallardo B., Clavero M., Sánchez M. I., Vilà M. (2016). Global ecological impacts of invasive species in aquatic ecosystems. Glob. Change Biol. 22, 151–163. doi: 10.1111/gcb.13004
Gamliel I., Buba Y., Guy-Haim T., Garval T., Willette D., Rilov G., et al. (2020). Incorporating physiology into species distribution models moderates the projected impact of warming on selected Mediterranean marine species. Ecography 43, 1090–1106. doi: 10.1111/ecog.04423
Giangrande A., Pierri C., Del Pasqua M., Gravili C., Gambi M. C., Gravina M. F. (2020). The Mediterranean in check: Biological invasions in a changing sea. Mar. Ecol. 41, e12583. doi: 10.1111/maec.12583
Gillooly J. F., Brown J. H., West G. B., Savage V. M., Charnov E. L. (2001). Effects of size and temperature on metabolic rate. Science 293, 2248–2251. doi: 10.1126/science.1061967
Gould K., Bruno J. F., Ju R., Goobdoby-Gringley G. (2021). Upper-mesophotic and shallow reef corals exhibit similar thermal tolerance, sensitivity and optima. Coral Reefs 40, 907–920. doi: 10.1007/s00338-021-02095-w
Gutermuth F. B., Armstrong D. A. (1989). Temperature-dependent metabolic response of juvenile dungeness crab cancer magister Dana: ecological implications for estuarine and coastal populations. J. Exp. Mar. Biol. Ecol. 126, 135–144. doi: 10.1016/0022-0981(89)90085-3
Harley M. D., Turner I. L., Kinsela M. A., Middleton J. H., Mumford P. J., Splinter K. D., et al. (2017). Extreme coastal erosion enhanced by anomalous extratropical storm wave direction. Sci. Rep. 7, 1–9. doi: 10.1038/s41598-017-05792-1
Hellmann J. J., Byers J. E., Bierwagen B. G., Dukes J. S. (2008). Five potential consequences of climate change for invasive species. Conserv. Biol. 22, 534–543. doi: 10.1111/j.1523-1739.2008.00951.x
Helmuth B. (2009). From cells to coastlines: how can we use physiology to forecast the impacts of climate change? J. Exp. Biol. 212, 753–760. doi: 10.1242/jeb.023861
Hines A. H., Johnson E. G., Darnell M. Z., Rittschof D., Miller T. J., Bauer L. J., et al. (2010). “Predicting effects of climate change on blue crabs in Chesapeake bay,” in Biol. manag. exploit. crab popul. clim. change (Fairbanks: Alsk. Sea Grant Univ. Alsk. Fairbanks), 109–127.
Hulme P. E., Bacher S., Kenis M., Klotz S., Kühn I., Minchin D., et al. (2008). Grasping at the routes of biological invasions: a framework for integrating pathways into policy. J. Appl. Ecol. 45, 403–414. doi: 10.1111/j.1365-2664.2007.01442.x
Iftikar F. I., MacDonald J., Hickey A. J. (2010). Thermal limits of portunid crab heart mitochondria: Could more thermo-stable mitochondria advantage invasive species? J. Exp. Mar. Biol. Ecol. 395, 232–239. doi: 10.1016/j.jembe.2010.09.005
Jost J. A., Podolski S. M., Frederich M. (2012). Enhancing thermal tolerance by eliminating the pejus range: a comparative study with three decapod crustaceans. Mar. Ecol. Prog. Ser. 444, 263–274. doi: 10.3354/meps09379
Juza M., Fernández-Mora À., Tintoré J. (2022). Sub-Regional marine heat waves in the Mediterranean Sea from observations: Long-term surface changes, Sub-surface and coastal responses. Front. Mar. Sci. 9, 785771. doi: 10.3389/fmars.2022.785771
Katsanevakis S., Coll M., Piroddi C., Steenbeek J., Ben Rais Lasram F., Zenetos A., et al. (2014). Invading the Mediterranean Sea: biodiversity patterns shaped by human activities. Front. Mar. Sci. 1, 32. doi: 10.3389/fmars.2014.00032
Kelley A. L. (2014). The role thermal physiology plays in species invasion. Conserv. Physiol. 2, 1–14. doi: 10.1093/conphys/cou045
Laird C. E., Haefner P. A. Jr. (1976). Effects of intrinsic and environmental factors on oxygen consumption in the blue crab, callinectes sapidus rathbun. J. Exp. Mar. Biol. Ecol. 22, 171–178. doi: 10.1016/0022-0981(76)90093-9
Leffler C. (1972). Some effects of temperature on the growth and metabolic rate of juvenile blue crabs, callinectes sapidus, in the laboratory. Mar. Biol. 14, 104–110. doi: 10.1007/BF00373209
Mancinelli G., Bardelli R., Zenetos A. (2021). A global occurrence database of the Atlantic blue crab callinectes sapidus. Sci. Data 8, 1–10. doi: 10.1038/s41597-021-00888-w
Mancinelli G., Chainho P., Cilenti L., Falco S., Kapiris K., Katselis G., et al. (2017a). On the Atlantic blue crab (Callinectes sapidus rathbun 1896) in southern European coastal waters: Time to turn a threat into a resource? Fish. Res. 194, 1–8. doi: 10.1016/j.fishres.2017.05.002
Mancinelli G., Glamuzina B., Petrić M., Carrozzo L., Glamuzina L., Zotti M., et al. (2016). The trophic position of the Atlantic blue crab callinectes sapidus rathbun 1896 in the food web of parila lagoon (South Eastern Adriatic, croatia): a first assessment using stable isotopes. Mediterr. Mar. Sci. 17, 634–643. doi: 10.12681/mms.1724
Mancinelli G., Guerra M., Alujevic K., Raho D., Zotti M., Vizzini S. (2017b). Trophic flexibility of the Atlantic blue crab callinectes sapidus in invaded coastal systems of the apulia region (SE italy): A stable isotope analysis. Estuar. Coast. Shelf Sci. 198, 421–431. doi: 10.1016/j.ecss.2017.03.013
Mangano M. C., Ape F., Mirto S. (2019). The role of two non-indigenous serpulid tube worms in shaping artificial hard substrata communities: case study of a fish farm in the central Mediterranean Sea. Aquac. Environ. Interact. 11, 41–51. doi: 10.3354/aei00291
McGeoch M. A., Butchart S. H., Spear D., Marais E., Kleynhans E. J., Symes A., et al. (2010). Global indicators of biological invasion: species numbers, biodiversity impact and policy responses. Divers. Distrib. 16, 95–108. doi: 10.1111/j.1472-4642.2009.00633.x
Millikin M. R. (1984). Synopsis of biological data on the blue crab, callinectes sapidus rathbun (National Oceanic and Atmospheric Administration, National Marine Fisheries).
Nehring S. (2011). “Invasion history and success of the American blue crab callinectes sapidus in European and adjacent waters,” in In the wrong place-alien marine crustaceans: distribution, biology and impacts. (Dordrecht: Springer) 607–624.
Occhipinti-Ambrogi A. (2007). Global change and marine communities: alien species and climate change. Mar. pollut. Bull. 55, 342–352. doi: 10.1016/j.marpolbul.2006.11.014
Poloczanska E. S., Brown C. J., Sydeman W. J., Kiessling W., Schoeman D. S., Moore P. J., et al. (2013). Global imprint of climate change on marine life. Nat. Clim. Change 3, 919–925. doi: 10.1038/nclimate1958
Pörtner H.-O., Bock C., Mark F. C. (2017). Oxygen-and capacity-limited thermal tolerance: bridging ecology and physiology. J. Exp. Biol. 220, 2685–2696. doi: 10.1242/jeb.134585
Prusina I., Sarà G., De Pirro M., Dong Y.-W., Han G.-D., Glamuzina B., et al. (2014). Variations in physiological responses to thermal stress in congeneric limpets in the Mediterranean Sea. J. Exp. Mar. Biol. Ecol. 456, 34–40. doi: 10.1016/j.jembe.2014.03.011
Ramos J. E., Pecl G. T., Moltschaniwskyj N. A., Semmens J. M., Souza C. A., Strugnell J. M. (2018). Population genetic signatures of a climate change driven marine range extension. Sci. Rep. 8, 1–12. doi: 10.1038/s41598-018-27351-y
Reyes Suarez N. C., Cook M. S., Gačić M., Paduan J. D., Drago A., Cardin V. (2019). Sea Surface circulation structures in the malta-sicily channel from remote sensing data. Water 11, 1589. doi: 10.3390/w11081589
Sakallı A. (2017). Sea Surface temperature change in the Mediterranean Sea under climate change: a linear model for simulation of the sea surface temperature up to 2100. Appl. Ecol. Environ. Res. 15, 707–716. doi: 10.15666/aeer/1501_707716
Sarà G., Giommi C., Giacoletti A., Conti E., Mulder C., Mangano M. C. (2021). Multiple climate-driven cascading ecosystem effects after the loss of a foundation species. Sci. Total Environ. 770, 144749. doi: 10.1016/j.scitotenv.2020.144749
Sarà G., Palmeri V., Montalto V., Rinaldi A., Widdows J. (2013a). Parameterisation of bivalve functional traits for mechanistic eco-physiological dynamic energy budget (DEB) models. Mar. Ecol. Prog. Ser. 480, 99–117. doi: 10.3354/meps10195
Sarà G., Palmeri V., Rinaldi A., Montalto V., Helmuth B. (2013b). Predicting biological invasions in marine habitats through eco-physiological mechanistic models: a case study with the bivalve b rachidontes pharaonis. Divers. Distrib. 19, 1235–1247. doi: 10.1111/ddi.12074
Sarà G., Porporato E. M., Mangano M. C., Mieszkowska N. (2018). Multiple stressors facilitate the spread of a non-indigenous bivalve in the Mediterranean Sea. J. Biogeogr. 45, 1090–1103. doi: 10.1111/jbi.13184
Seebens H., Bacher S., Blackburn T. M., Capinha C., Dawson W., Dullinger S., et al. (2021). Projecting the continental accumulation of alien species through to 2050. Glob. Change Biol. 27, 970–982. doi: 10.1111/gcb.15333
Sokolova I. M., Frederich M., Bagwe R., Lannig G., Sukhotin A. A. (2012). Energy homeostasis as an integrative tool for assessing limits of environmental stress tolerance in aquatic invertebrates. Mar. Environ. Res. 79, 1–15. doi: 10.1016/j.marenvres.2012.04.003
Talluto M. V., Boulangeat I., Ameztegui A., Aubin I., Berteaux D., Butler A., et al. (2016). Cross-scale integration of knowledge for predicting species ranges: a metamodelling framework. Glob. Ecol. Biogeogr. 25, 238–249. doi: 10.1111/geb.12395
Truchy A., Angeler D. G., Sponseller R. A., Johnson R. K., McKie B. G. (2015). “Linking biodiversity, ecosystem functioning and services, and ecological resilience: towards an integrative framework for improved management,” in Advances in ecological research (Elsevier), 55–96.
Tsiamis K., Zenetos A., Deriu I., Gervasini E., Cardoso A. C. (2018). The native distribution range of the European marine non-indigenous species. Aquat. Invasion. 13 (2), 187–198. doi: 10.3391/ai.2018.13.2.01
Walter K., Graf H.-F. (2002). On the changing nature of the regional connection between the north Atlantic oscillation and sea surface temperature. J. Geophys. Res. Atmos. 107, ACL–AC7. doi: 10.1029/2001JD000850
Weber M. M., Stevens R. D., Diniz-Filho J. A. F., Grelle C. E. V. (2017). Is there a correlation between abundance and environmental suitability derived from ecological niche modelling? a meta-analysis. Ecography 40, 817–828. doi: 10.1111/ecog.02125
Keywords: thermal tolerance, respiration rate, ecological performance, climate change, mapped metabolism
Citation: Marchessaux G, Bosch-Belmar M, Cilenti L, Lago N, Mangano MC, Marsiglia N and Sarà G (2022) The invasive blue crab Callinectes sapidus thermal response: Predicting metabolic suitability maps under future warming Mediterranean scenarios. Front. Mar. Sci. 9:1055404. doi: 10.3389/fmars.2022.1055404
Received: 27 September 2022; Accepted: 06 December 2022;
Published: 21 December 2022.
Edited by:
Pedro Morais, Florida International University, United StatesReviewed by:
Eudrianoo Costa, University of the Azores, PortugalCopyright © 2022 Marchessaux, Bosch-Belmar, Cilenti, Lago, Mangano, Marsiglia and Sarà. This is an open-access article distributed under the terms of the Creative Commons Attribution License (CC BY). The use, distribution or reproduction in other forums is permitted, provided the original author(s) and the copyright owner(s) are credited and that the original publication in this journal is cited, in accordance with accepted academic practice. No use, distribution or reproduction is permitted which does not comply with these terms.
*Correspondence: Guillaume Marchessaux, Z3VpbGxhdW1lLmdtYXJjaGVzc2F1eEBnbWFpbC5jb20=
Disclaimer: All claims expressed in this article are solely those of the authors and do not necessarily represent those of their affiliated organizations, or those of the publisher, the editors and the reviewers. Any product that may be evaluated in this article or claim that may be made by its manufacturer is not guaranteed or endorsed by the publisher.
Research integrity at Frontiers
Learn more about the work of our research integrity team to safeguard the quality of each article we publish.