- 1Ecology Division, Faculty of Biosciences and Aquaculture, Nord University, Bodø, Norway
- 2Shoals Marine Laboratory, University of New Hampshire, Durham, NH, United States
- 3Department of Biological Sciences, University of Bergen, Bergen, Norway
- 4Algal and Microbial Biotechnology Division, Faculty of Biosciences and Aquaculture, Nord University, Bodø, Norway
The genus Fucus dominates the intertidal and shallow subtidal rocky reefs of the North Atlantic and also is commonly found in the intertidal of the North Pacific. It likely diversified 12.2-2.7 mya into two genetically distinct lineages: Lineage 1 with one species in the North Pacific and two in the North Atlantic; and Lineage 2 found only in the North Atlantic (one species recently introduced into the North Pacific). With 10 accepted species, Fucus spp. (and the Fucales) are unique among algae in having a diplontic life cycle, whereby the only haploid stage is the single-celled gamete. Further, Fucus spp. produce eight eggs in each oogonium; have hermaphroditic and dioecious species in each lineage; display sperm:egg ratios differing by more than one order of magnitude; have synchronized and predictable release of gametes; are capable of self- and/or cross- fertilization and asexual (fragmentation via adventitious branching) reproduction; readily hybridize in culture, as well as the field; and form ecads (free-living individuals with morphological variability linked to habitat) by hybridization or polyploidy. Consequently, the genus is an excellent model for a variety of studies in reproductive biology, employing laboratory and field manipulations as well as detailed genetic studies using the molecular ‘omics’. We review here the relevant literature in order to fully understand and appreciate the unique opportunities that Fucus spp. provide as model organisms for future studies of reproduction.
Introduction
The brown algal genus Fucus (Ochrophyta, Fucales) is an important ecosystem engineer on sheltered and moderately exposed rocky shores of the North Pacific and the North Atlantic. As providers of an important foundational habitat, the species collectively provide shelter and food for a plethora of invertebrate and fish species, as well as a substrate for attachment of epibionts (Lüning, 1990; Chapman, 1995).
Fucus also is important as a food source for large land herbivores (both wild and domestic), as well as human cultures. For example, reindeer venture into the intertidal in high Arctic regions to forage on Fucus when snow cover is too deep and/or rain-on-snow icing makes it difficult to obtain their normal diet of lichens (Hansen et al., 2019). Elsewhere in northwestern Europe, seaweeds (including Fucus spp.) have been consumed by, and fed to, sheep, cattle, and pigs, both historically and presently (see references in Blanz et al., 2020). Sheep that escaped the annual round-up in Iceland and Norway often survived the harsh winters by foraging on intertidal Fucus (Landsborough, 1857). The feral and protected North Ronaldsay or Orkney sheep on North Ronaldsay (northernmost island of Orkney) have modified their gut biome, as a result of confinement to the intertidal by humans, to subsist almost entirely on seaweed, including Fucus (Balasse et al., 2005; Ruggeri, 2015). With respect to humans, Fucus spp. have been a food source, processed to a form of ‘soda ash’ for soap and glass making, used for centuries as fertilizer in maritime areas that could not support livestock for manure (Pereira & Cotas, 2019), and had/have a variety of traditional medicinal uses, albeit of suspect effectiveness (https://medlineplus.gov/druginfo/natural/726.html; but see Catarino et al., 2018).
Students and researchers began studying coastal intertidal regions as soon as marine laboratories were established along the shores of the North Atlantic, beginning in the mid-1800s (e.g., Station Biologique de Roscoff, 1859; Woods Hole, USA, 1871; Kristineberg Marine Research Station, 1877; Laboratory of the Marine Biological Association at Plymouth, 1885; Marine Biological Laboratory (USA), 1888; University of Oslo Drøebak, 1894) and a prominent subject for these early investigations was the readily available Fucus. However, perhaps the first published description of Fucus was over 300 years ago, in a 1711 paper by René-Antoine Réaumur that included detailed illustrations of what appears to be a combination of Fucus vesiculosus and F. serratus (Figure 1).
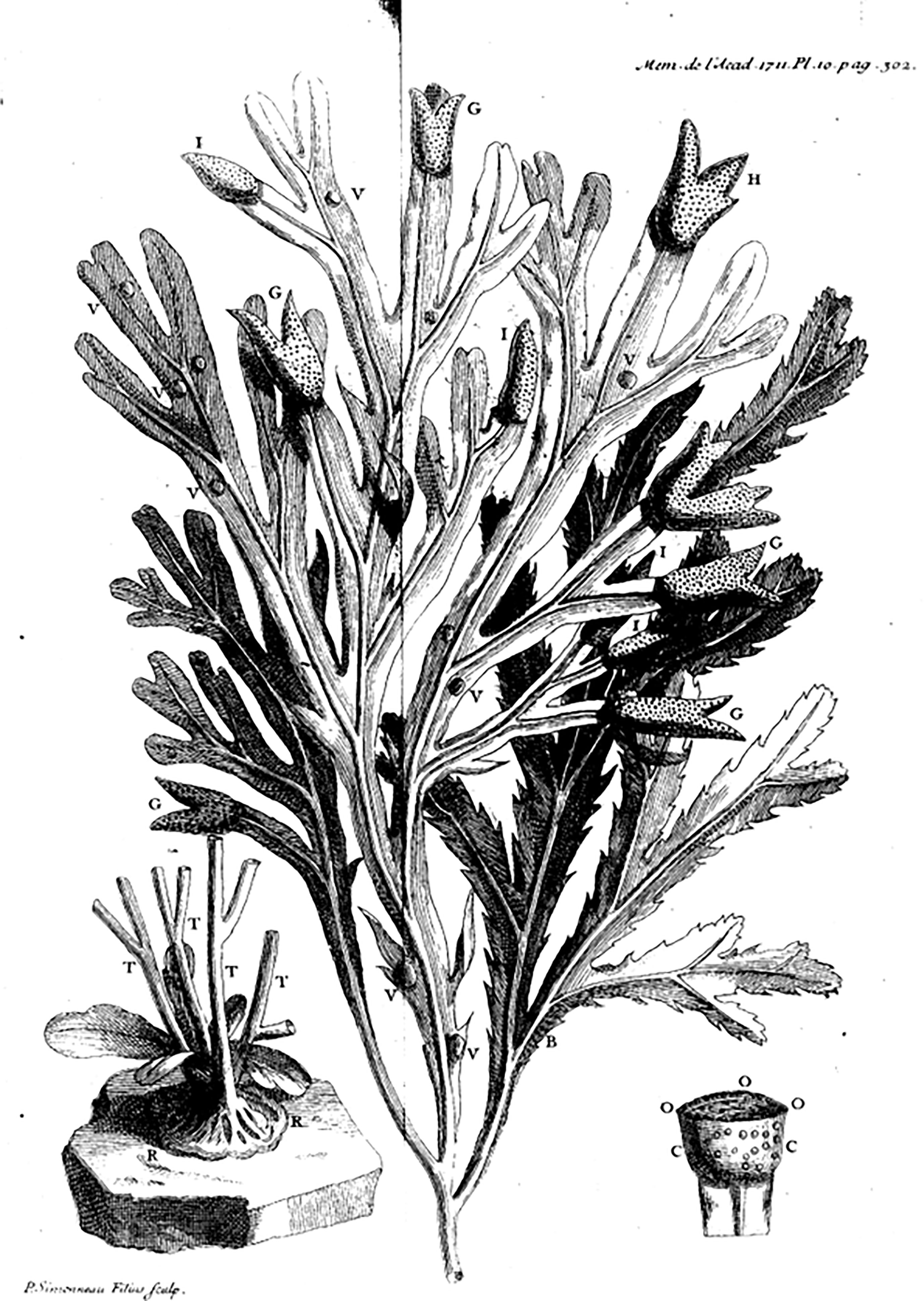
Figure 1 Illustration of Fucus serratus from Réaumur (1711). Note that one thallus appears to have receptacles and vesicles characteristic of F. vesiculosus, perhaps mistakenly interpreted as stemming from the same holdfast, when in fact, it may be two independent attachments, one on the other (see insert). Permission to reuse this image was confirmed by Service des Archives et du Patrimoine historique under the free use of public archive documents.
Coyer et al. (2006a) first proposed a North Pacific origin of Fucus based on a phylogeny derived from both a variable and conserved region of the mitochondrion that revealed: 1) sister-taxa to Fucus are found only in the North Pacific, and 2) high haplotype and nucleotide diversity in the variable mt region was present in the North Pacific, whereas only a single haplotype (shared with the North Pacific) was found in the North Atlantic. A later phylogenetic study using 13 protein-coding genes also supported a North Pacific origin of Fucales dating to 19.5-7 mya with divergence of the genus Fucus at 12.5-2.7 mya (Cánovas et al., 2011), agreeing with an earlier estimate of Fucus divergence at 2.3-5.5 mya using single-strand conformation polymorphisms (SSCP) of a mtDNA spacer region (Hoarau et al., 2007). However, the most recent Fucus phylogeny, with more extensive sampling throughout the Arctic and Subarctic and using 21 mtDNA-IGS haplotypes, placed the ancestor of the F. distichus complex (likely near the ancestral member of the genus) in the low Arctic/Subarctic (Laughinghouse et al., 2015). Sequence analysis of nuDNA and mtDA also revealed two distinct lineages within the genus, Lineage 1 with two accepted species and Lineage 2 with eight accepted species (see https://www.algaebase.org/search/genus/detail/?genus_id=71; Almeida et al., 2022).
Recent studies have illustrated the dynamic nature of speciation in Fucus. For example, microsatellite markers suggest that F. radicans diverged from F. vesiculosus the northern Baltic within the last 400 years (Pereyra et al., 2009). On the other hand, however, is extinction; the glacial relict populations of F. virsoides along the Slovenian coast declined significantly by 2010 and disappeared entirely by 2016 (Battelli, 2016).
Excellent reviews on the broad subjects of phylogeny (Cánovas et al., 2011); physiology (Chapman, 1995; Colvard et al., 2014; Colvard & Helmuth, 2017); gamete release and settlement/recruitment (Chapman, 1995; Brawley et al., 1999); and ecology (Chapman & Johnson, 1990; Chapman, 1995; Wahl et al., 2011) have been written and publications on various aspects of Fucus have increased dramatically, closely tracking key technological advancements (Figure 2). This review will address and update aspects of reproduction in Fucus.
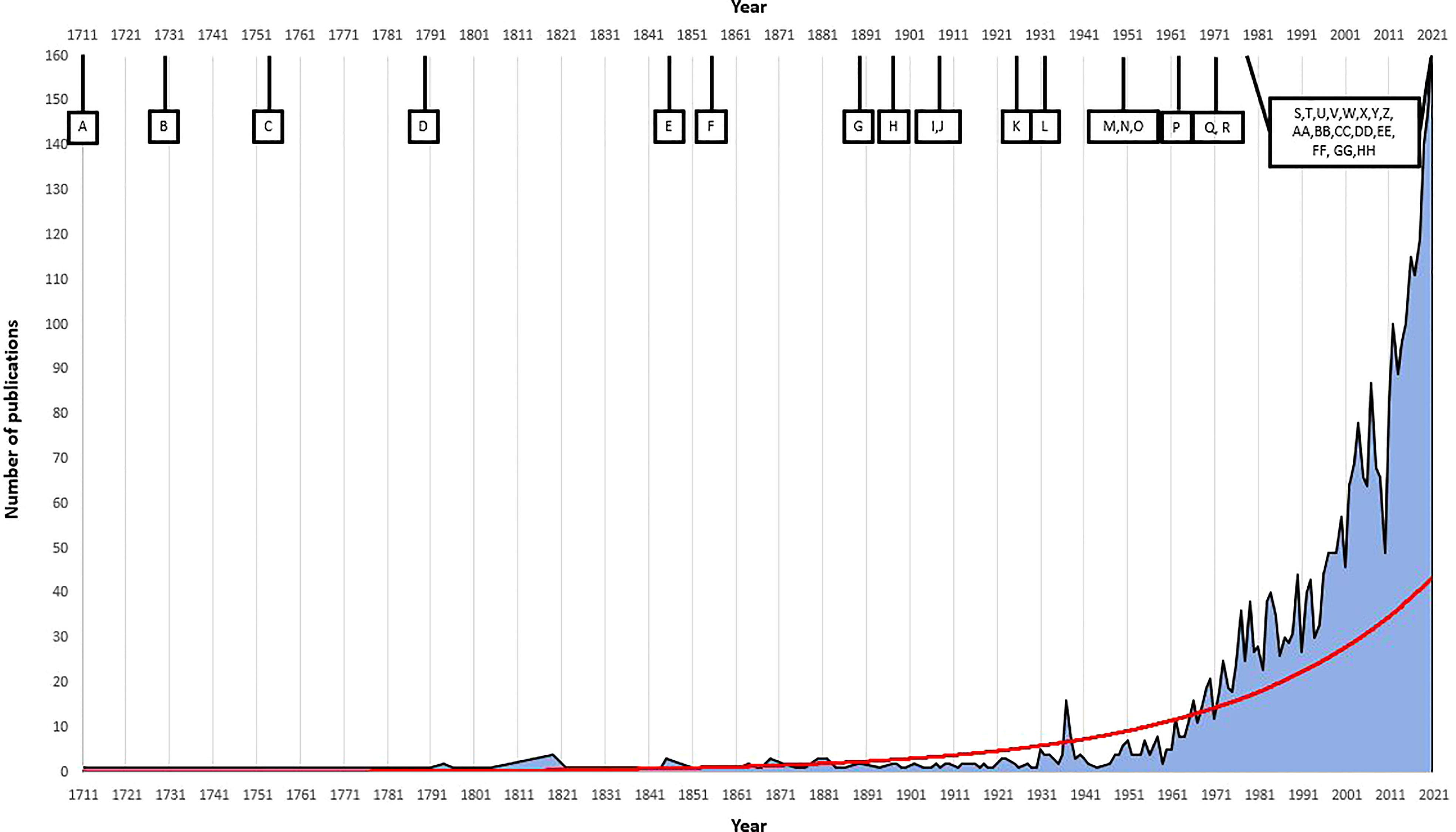
Figure 2 Correlation of number of Fucus publications and key advances in Fucus reproduction/research and general technological advancements over time. We used the software Dimensions (Digital Science; https://app.dimensions.ai/discover/publication) to search for ‘Fucus’ in titles and abstracts of all journals and all dates. Red line is the exponential trendline based on the number of publications released. (A) 1711, first publication on Fucus reproduction (Réaumur, 1711); (B) 1730, advancement in microscope technology (Tromp, 2015), (C) 1753, first taxonomic description of Fucus Linnaeus (Linneí and Salvius, 1753); (D) 1790-1900, early chemical, physiological and biological descriptions (Woodward, 1791; Stackhouse, 1801; Kniep, 1925); (E) 1845, initial research on life history, reproductive methods and taxonomy reassignment (Genus Fucus) (Decaisne & Thuret, 1845); (F) 1854, first description of fertilisation in Fucus and first cross-experiments (Thuret, 1855); (G) 1890, beginning of laboratory cultures (Campbell, 1889); (H) 1897, first cytological study of Fucus (Farmer & Williams, 1897); (I) 1909, first observation of fertalisation and total number of chromosomes (Yamanouchi, 1909); (J) 1909, early ecological studies (Baker, 1909; Baker, 1910); (K) 1925, first successful cross experiments (Kniep, 1925); (L) 1931, invention of the electron microscope (Freundlich, 1963); (M) 1950, first electron microscope image of Fucus (Manton & Clarke, 1950); (N) 1950, beginning of autocological research in Fucus (Knight & Parke, 1950; Burrows & Lodge, 1951); (O) 1951, natural hybridization experiment (Burrows & Lodge, 1951); (P) 1962, further studies on Fucus chromosome number (Evans, 1962); (Q) 1970, detailed experimentation on gamete release and gamete physiological structure and characteristics for Fucus (Pollock, 1970); (R) 1970-1995, in-depth research on individual, population and community ecology in Fucus (Chapman, 1995); (S) 1977, first generation sequencing (Heather & Chain, 2016); (T) 1979, detailed investigation on natural morphological variation and phenotic plasticity in Fucus (Scott & Hardy, 1994); (U) 1980, formal demographic analysis on Fucus species (Gunnill, 1980); (V) 1970-1990, development of PCR and microsatellites (Kaunitz, 2015; Saeed et al., 2016); (W) 1985, discovery of pheromonal gamete attraction in Fucus (Müller & Gassmann, 1985); (X) 1980-1991, comprehensive studies on egg production in Fucus (Vernet & Harper, 1980; Robertson, 1987; Ang, 1991); (Y) ~1990, detailed research in rates of reproduction, settlement, recruitment and population modelling in Fucus begun (Chapman, 1995); (Z) 1997-1999, rDNA and nrDNA sequencing of internal transcribed spacer region in Fucus and SSU and LSU sequences (Leclerc et al., 1998; Rousseau et al., 1997; Rousseau & de Reviers, 1999; Serrão et al., 1999a); (AA) 2002-2003, design of polymorphic microsatellite markers for Fucus (Coyer et al., 2002c; Engel et al., 2003); (BB) 2005, next generation sequencing begun (Heather & Chain, 2016); (CC) 2006, RNA extraction method designed for Fucus and tested with RT-PCR, RNA-labelling and Northern analysis methods (Pearson et al., 2006), (DD) 2006, complete mitochondrial genome for F. vesiculosus and mtDNA-based phylogeny showing the two Fucus lineages (Oudot-Le Secq et al., 2006; Coyer et al., 2006a); (EE) 2011, third generation sequencing (Heather and Chain, 2016); (FF) 2013, first investigation in sex-biased gene expression in F. vesiculosus (Martins et al., 2013); (GG) 2013-present, effects of climate change on Fucus distribution (Jueterbock et al., 2014; Rothäusler et al., 2018; Rugiu et al., 2018; Rothäusler et al., 2019); (HH) 2020-to present, complete annotated genome and transcriptome using illumina and nanopore platforms (https://phaeoexplorer.sb-roscoff.fr/home/).
General Characteristics
Sexual dimorphism (when reproductive) and the realization of dioecy and hermaphroditism in Fucus species were determined in the 1800s. Reproductive individuals produce numerous receptacles at the apical tips of branches, each of which contain many conceptacles. In dioecious species, all conceptacles on an individual contain either antheridia or oogonia (Figure 3); hermaphroditic species have conceptacles containing both antheridia and oogonia (see Engel et al., 2005). There are no monoecious species of Fucus (e.g., one individual with receptacles containing conceptacles with either antheridia or oogonia). Fucus spp. exhibit a diplontic life cycle with male heterogamy (XX/XY) (Heesch et al., 2021) and gametes are the only haploid cells. Sex of dioecious species often can be determined by eye in the field when reproductive: male receptacles appear red/orange-colored because of carotenoids in eyespots for the negatively phototaxic sperm contained within antheridia of the conceptacles (Decaisne & Thuret, 1845), whereas female conceptacles are green or brown because of chloroplasts in the eggs (Whitaker, 1931). The egg begins dividing ~24 hrs after fertilization (Thuret, 1855).
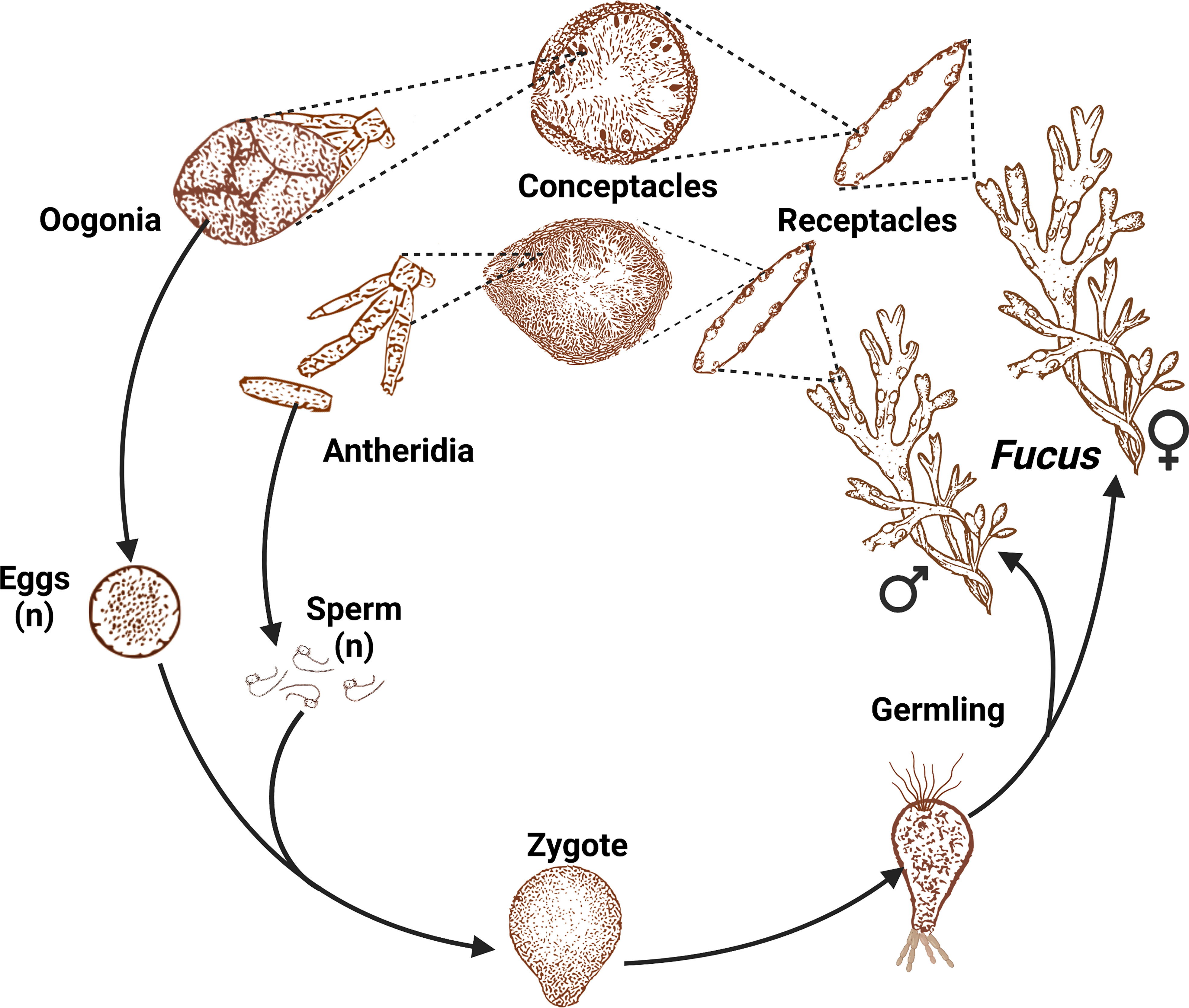
Figure 3 Dioecious life cycle of the genus Fucus. A representative illustration, not to scale, adapted from Müller (1991) footage, provided by Technische Informationsbibliothek (TIB) and created with BioRender.com. Receptacles of a hermaphroditic species contain both antheridia and oogonia, whereas in dioecious species (pictured), an individual is either male (all receptacles contain antheridia) or female (all receptacles contain oogonia).
All species of Fucus reproduce sexually, but sexual reproduction is difficult in brackish conditions. In F. vesiculosus, for example, the low salinity (<5 PSU) typical of the Baltic Sea: 1) drastically reduces the release of gametes; 2) diminishes longevity and motility of released gametes; 3) reduces fertilization rates; and 4) fosters polyspermy, which is lethal in Fucus and increases dramatically in brackish conditions (Brawley, 1991; Brawley, 1992; Serrão et al., 1996; Serrão et al., 1999a). Consequently, asexual reproduction (fragmentation via adventitious branching) is common among populations existing in marginal environments, including ecads (discussed below) and F. radicans in the Baltic (Tatarenkov et al., 2005; Pereyra et al., 2009; Johannesson et al., 2011). In the northern Baltic, F. radicans recruits both sexually and asexually, varying from complete sexual to >90% monoclonal with phenotypic variation significantly lower in monoclonal stands than in multi-clonal groups (Johannesson et al., 2012). Furthermore, a shift from sexual to asexual reproduction has occurred in a few absolute marginal populations of F. vesiculosus in the northern Baltic Sea (Tatarenkov et al., 2005).
It is important to realize that asexual reproduction does not necessarily reduce fitness, (Preston et al., 2022 and references therein). For example, F. vesiculosus in the Baltic Sea can exist in two forms: the most common being the epilithic form (attached, sexual and rarely asexual) and less commonly, the benthopleustophytic form (free-living and asexual) found on any substrate within the photic zone (Preston et al., 2022 and references therein). However, each form likely will respond differently to future environmental changes. Additionally, asexual reproduction may conserve existing genotypes by preventing recombination of new genotypes, unlike selfing (see below) (Ardehed et al., 2015).
In Fucus, adventitious branches can form from both the thallus and holdfast, with regeneration most rapid from the midrib region of the thallus (Fulcher & McCully, 1969; Fulcher & McCully, 1971; McLachan et al., 1971; Van Alstyne, 1989). Branches can be induced by herbivory or in response to no obvious physical stimulus (Van Alstyne, 1989). The epidermal cells grow outward forming distinct ‘embryos’ instead of lateral branches, which are indistinguishable from early sexually produced embryos (Fulcher and McCully, 1969; McCook & Chapman, 1993).
Detached adventitious branches may reattach to the substratum via rhizoids and develop into apparently functional male and female thalli (asexual recruitment), which subsequently can form large clones with skewed sex ratios (Tatarenkov et al., 2005; Johannesson et al., 2011; Ardehed et al., 2015). For example, a large F. radicans female clone in the northern Baltic was distributed over 550 km and a large male clone over 100 km; both were likely to be a few thousand years old (Johannesson et al., 2011; Ardehed et al., 2015). Populations in more southerly Baltic locations, however, almost exclusively displayed sexual recruitment (Johannesson et al., 2011; Ardehed et al., 2015).
More recently, experiments with F. radicans demonstrated that temperature and light interactively resulted in the highest success of re-attachment of adventitious branches (Schagerström & Salo, 2019). Light level alone had no effects on success in cooler water temperature while success in high water temperature under low light levels was very low. Their results further suggested that rhizoid formation (re-attachment success) depends on the net primary production (metabolic balance) of the adventitious branches.
The notion that sexual reproduction in Fucus is impeded in areas of low salinity has been challenged by recent studies detailing a much more complex picture. Ardehed et al. (2016) found high levels of sexual reproduction in some F. radicans populations inhabiting the extreme low salinity (2-3 PSU) Gulf of Finland. Kinnby et al. (2019) showed that low salinity, temperature, and oxygen (i.e., high stress) were associated with high number of adventitious branches in F. vesiculosus and F. radicans populations within the Baltic, but outside the Baltic, high salinity, high phosphate, and low turbidity were positively correlated with adventitious branching. Kinnby et al. (2019) hypothesized that variation in patterns of adventitious branching between populations was due either to genetic differences arising from local adaptation unrelated to the physical factors that were measured or from stochastic effects of population separation, and emphasized the need to identify additional environmental factors that may explain the predominance of asexual reproduction in the Baltic Sea. These studies clearly demonstrate that the recently formed postglacial Baltic Sea (8000 yrs; Björk, 1995), with steep gradients in physical characteristics from south to north (this and all further directional references are poleward), is a challenging and marginal environment for the closely related F. radicans and F. vesiculosus in terms of allocating resources to sexual or asexual reproduction.
Fucus spp. in Lineage 2 exhibit a general spring-summer reproduction period in most studies (Table 1), but in the low salinity Baltic Sea, F. vesiculosus displayed two peaks of reproduction: early summer (May-June) and late autumn (September-October) (Table 1; Berger et al., 2001). Summer-reproducing individuals initiated receptacle development and produced more, but smaller eggs in response to short-day laboratory conditions, whereas receptacle development was independent of daylight in autumn-reproducing individuals, suggesting the presence of two distinct genotypes (Berger et al., 2001). In southern Europe, mature oogonia are present all year in F. vesiculosus and F. spiralis (Table 1).
Maximum reproductive peaks for F. serratus on open coasts of the NE Atlantic occurred in both the spring and the autumn (March and September) and in the spring-autumn in the NW Atlantic, but in the Baltic Sea, mainland Swedish populations were reproductive in the autumn (October-November) and on the coast of Öland in the summer (June-July) (Malm et al., 2001). Fertilization success in F. serratus decreased markedly as salinity decreases, more so than for F. vesiculosus, but unlike F. vesiculosus, asexual reproduction was not observed in the Baltic (Malm et al., 2001). Non-overlapping reproductive periods also have been observed among F. distichus populations off the New England coast in the US (Sideman & Mathieson, 1983a; Sideman & Mathieson, 1983b; Pearson & Brawley, 1996). In general, reproductive seasons vary within and among species of Fucus (Table 1) and clearly, continuation of non-overlapping reproductive periods within populations of a species could increase the probability of eventual speciation.
Gamete structure, release, and fertilization
Studies of reproductive structures in Fucus began soon after microscopes were developed. Decaisne & Thuret (1845) published one of the first microscopic descriptions of Fucus antheridia and ‘spores’, stating that the “transparent corpuscles (are) nearly pear-shaped, each one inclosing a single red globule; each one of these corpuscles is furnished with two very thin cilia, by means of which it moves with extreme vivacity”. Conceptacles were described by Bower (1880), followed by descriptions of mitosis, meiosis, physiology of Fucus spermatozoids, conceptacle development, and egg development (e.g., Farmer & Williams, 1897; Farmer & Williams, 1898; Yamanouchi, 1909; Robbins, 1916; Roe, 1916; see also reviews by Whitaker, 1931; Fritsch, 1945). The first electron micrographs of F. serratus sperm revealed that the base of the anterior flagellum was enveloped by a flexible membrane of unknown function and the flagellum was covered with ‘hairy’ appendages; both were absent in the posterior flagellum. (Manton & Clarke, 1950). For further microscopic descriptions of gamete structure and fertilization in Fucus, see: Pollock, 1970; Brawley et al., 1976; Callow et al., 1978; Motomura, 1994. Recent advances in electron microscopy (high pressure freezing, microinjection of fluorescent dyes) examining ultrastructure, distribution, and de novo formation of plasmodesmata in F. distichus promise to advance studies of receptors and cell-to-cell communication (Nagasato et al., 2015).
In Fucus, there are eight eggs per conceptacle (Serrão et al., 1999b; Coyer et al., 2002b), however the number of eggs per receptacle can be species-specific; for example, F. vesiculosus produces 10x more eggs than F. serratus (Malm & Kautsky, 2003) and hermaphroditic species produce significantly fewer sperms/egg (40:1) than dioecious species (400:1) (Vernet & Harper, 1980). Additionally, sperm in the hermaphrodite F. spiralis are much smaller (0.7 1 < 0.46 μm, > 0.21) than in the dioecious F. vesiculosus and F. serratus (1.58 < 1.25 μm > 0.92), suggesting that the smaller hermaphroditic sperm have fewer energy reserves for swimming in search of eggs (Vernet & Harper, 1980). Experiments have indicated, however, that sperm numbers do not limit fertilization (Berndt et al., 2002). Egg volume varies widely among Fucus species, ranging from 235 x 103 μm3 in F. spiralis to 68 x 103 and 181 x 103 μm3 in F. vesiculosus and F. serratus, respectively (Vernet & Harper, 1980).
As soon as gametes were observed with the early microscopes, it was realized that Fucus provided a favorable system for the study of fertilization (Thuret, 1855); later observations revealed that Fucus sperm was attracted to the eggs (Robbins, 1916; see also references in Müller & Seferiadis, 1977). The extremely small amounts of attractant were below detection by instruments until the early 1970s when the pheromone fucoserratene was isolated and identified from eggs of F. serratus (Müller & Jaenicke, 1973). Maier and Müller (1986) reviewed extraction methods, identified sexual pheromones in several species of brown algae, including Fucus, and detailed how the passage of Fucus sperm through a critical concentration level induces a phobic return to the source of pheromone. Given the natural and complex chemical ‘noise’ that exists in the marine environment, it is perhaps not surprising that introduction of anthropomorphic pollution has the potential to increase the level of ‘noise’ and affect fertilization in Fucus (Steele, 1977).
To achieve maximum fertilization success in Fucus, gametes must be synchronously released from nearby individuals and under optimal environmental conditions. While mature oogonia were found all year in receptacles of F. vesiculosus, F. spiralis and F. guiryi (now F. limitaneus, Almeida et al., 2022) in a study from North-Portugal, release of eggs (measured as egg settlement) was mainly observed during late spring and summer, with very low settlement observed during the rest of the year (Monteiro et al., 2016). Andersson et al. (1994) measured egg release of F. vesiculosus from the Baltic Sea, and observed peaks that occurred in a semilunar pattern. In addition, the highest egg release took place during the evening, between 18:00 and 22:00. Serrão et al. (1996) demonstrated that photosynthesis was necessary for gamete release during calm conditions when extremely high levels of fertilization success, mostly >90%, were achieved (see also Pearson and Brawley, 1996, Pearson et al., 1998). Higher levels of fertilization success (100%) were noted for F. vesiculosus in calm conditions within a one-hour interval of a 6-7 hr high tide, 2-3 hrs after being covered by the rising tide (Berndt et al., 2002). Experiments demonstrated that release of gametes was correlated with depletion of dissolved inorganic carbon (DIC) in isolated tide pools during increased light conditions and that sensitivity of gamete release to high water motion was DIC dependent. Specifically, the boundary layer surrounding the receptacle becomes thicker in calm conditions and DIC becomes limiting to photosynthesis, acting as an initial signal on a pathway leading to gamete release (Pearson et al., 1998). In conclusion, gamete release in Fucus requires a sunny day, calm conditions, and high tide immersion (Brawley, 1990; Brawley, 1992; Pearson et al., 1998; Berndt et al., 2002; Monteiro et al., 2016).
Low-tide release of gametes, however, is possible under certain conditions. Berndt et al. (2002) documented gamete release in F. vesiculosus following several hours of submergence by a rising tide as photosynthesis is required to prepare receptacles for gamete release. They noted that release at low tide occurred after several days of stormy weather and postulated that the normal high tide release was subsequently inhibited and too many mature gametangia were present in the conceptacles leading to release at low tide.
On the other hand, time of day, tidal height, and wave exposure also influenced egg release and settlement patterns, which further differed according to mating type. Ladah et al. (2008) found that dioecious F. vesiculosus released more eggs later in the day and at a lower tide than the hermaphroditic F. spiralis which released few eggs throughout the day and at all tides. Thus, the importance of low tide or high tide in gamete release is equivocal.
Dispersal of released gametes is very limited. According to a model, small propagules released one meter from the substratum in turbulent water motion will be transported to the substratum within 2-25 seconds or during 1-6 waves (Denny & Shibata, 1989) and only a few meters laterally during this time frame. Furthermore, Fucus sperm are too short-lived to be effective agents of dispersal and are attracted to egg-produced pheromones at only μm to mm distances. Additionally, eggs are fertilized quickly after release, are subjected to lethal polyspermy, and the negatively buoyant zygotes secrete a sticky substance for rapid adherence to the substrate (Kropf, 1992; Serrão et al., 1996; Muhlin et al., 2008). Thus, viable Fucus gametes and zygotes are likely to disperse only a few meters from the parent (Arrontes, 1993; Serrão et al., 1997; Engel et al., 2005; Muhlin et al., 2008). Strong genetic structuring (isolation-by-distance) revealed by microsatellites among some populations of F. serratus implied limited dispersal due to salinity gradients (e.g., Coyer et al., 2003; Coyer et al., 2011a); whereas the lack of genetic structuring among nearby populations of F. vesiculosus may be due to high gene flow, inbreeding depression, microscopic forms persisting from previous generations, and/or inappropriateness of using neutral genetic markers to detect the presence of sub-populations (Zardi et al., 2013; Teixeira et al., 2016).
Additionally, long-distance dispersal via rafting of fertile Fucus that interbreed with attached individuals may increase connectivity among populations (Muhlin et al., 2008). For example, F. vesiculosus possesses air bladders on the vegetative thalli allowing rafting to distant locales when detached and free-floating (Coleman & Brawley, 2005; Tatarenkov et al., 2007; Muhlin et al., 2008; Rothäusler et al., 2015) or when attached to floating objects such as buoyant seaweed, natural wood, and anthropogenic debris (Thiel et al., 2011). Rafting may be particularly important in dispersal of hermaphroditic species as only one fertile individual is necessary for successful colonization via a stepping-stone dispersal over longer distances. Indeed, genetic analysis strongly infers the success of rafting for hermaphroditic Fucus species (Coleman & Brawley, 2005; Coyer et al., 2011b). Long-distance dispersal of clones via detached adventitious branches (asexual reproduction) also occurs. For example, Ardehed et al. (2015) found single thalli of F. radicans genetically assigned to clones from distant sites rather than from the population in which they were found and reported finding single and vital thalli 18 and 50 km from the nearest population. Such dispersal is important because in a new area, a unisexual population (=clone) may evolve into a bisexual population and initiate sexual reproduction (Ardehed et al., 2015).
Selfing
Simultaneous hermaphroditism can lead to self-fertilization (selfing), an important aspect of evolutionary biology. Hermaphroditism has been reported for 10 animal phyla and ~5% of animal species, a percentage that increases substantially if the highly specious insects are excluded (Jarne & Auld, 2006). In flowering plants, the transition from outcrossing to selfing occurs in many independent lineages (10-15% of seed plants) and may be a driver of speciation (Wright et al., 2013). Within the genus Fucus, in vitro fertilization experiments have demonstrated selfing in F. spiralis (Pollock, 1970; Vernet & Harper, 1980; Müller & Gassmann, 1985) and genetic investigations have supported selfing in F. spiralis, F. guiryi, and F. distichus (Billard et al., 2005; Coleman & Brawley, 2005; Engel et al., 2005; Coyer et al., 2007; Perrin et al., 2007; Billard et al., 2007; Coyer et al., 2011c; Almeida et al., 2017; Whitaker et al., 2017).
Selfing usually is considered to be deleterious (see Wells, 1979, and references therein). First, it increases the probability that recessive maladaptive genes will become homozygous and subsequently decrease adaptation and fitness (e.g., ‘dead end’ of Stebbins, 1974). Secondly, genetic recombination is limited and further reduces genetic potential to enhance survival and reproduction in a changing environment. Thirdly, effective population size may be reduced.
However, the notion of selfing being a ‘dead end’ over the long term is unclear and the evolutionary and ecological mechanisms need further investigation (Wright, et al., 2013). Some advantages to selfing exist (see Wells, 1979, and references therein). In angiosperms, for example, it is possible that environmental conditions will inhibit pollen dispersal, thereby leading to extinction unless selfing is employed. Additionally, when pollinators and/or mates are rare, or when sperm is limiting (or only self-sperm is available for fusion with eggs due to phrenological incompatibility; Engel et al., 2005) and outcrossing is uncertain, selfing offers reproductive assurance (see references in Vernet & Harper, 1980; Wright, et al., 2013, and Perrin et al., 2007). Selfing also allows transmission of a whole genome through both the male and female functions to the next generation (Fisher, 1941). Furthermore, selfing is a viable means of colonization requiring only one fertile individual and can be an advantage in a mixed population of two species that produce sterile hybrids. Prolonged selfing also can lead to purging of deleterious homozygotes and reduce inbreeding depression (Schoen, 2005; Igić et al., 2006) in unchanging environments. And finally, high-fitness selfed individuals with low rates of recombination at adaptive loci may facilitate colonization by locally-adapted genotypes (Eriksson and Rafajlović, 2021)
Selfing may be advantageous in the upper shore F. spiralis as the severe desiccation stress may maintain favorable co-adapted gene combinations by reducing recombination (shuffling of genetic material between male and female chromosomes during meiosis) (Stebbins, 1950; Engel et al., 2005). Additionally, selfing may be important in the closely related species F. spiralis and F. macroguiryi (formerly F. guiryi Almeida et al., 2022) that are sympatric along a vertical exposure gradient in the intertidal regions of northern Portugal and southern France. The two species are separated by a meter or so on the shore, and although extensive gene flow occurs between the species in sympatry (only F. macroguiryi is present in southern Iberia), experiments suggest that strong selection on physiological traits across the intertidal gradient maintains the distinct genetic and morphological species within their preferred vertical distribution (Zardi et al., 2011). The prevalence and importance of selfing (relative to outcrossing) in these species in sympatry remains to be determined.
The most recent common ancestor of Fucus probably occurred in the Atlantic/Arctic Ocean Basin, where subsequent diversification occurred after opening of the Bering Strait 5.5-5.4 mya (Cánovas et al., 2011). In this area, selfing may be an important and overlooked aspect underlying the diverse morphological forms exhibited by the hermaphroditic F. distichus complex throughout its range in the low- and sub-Arctic. Sequence analysis of a variable intergenic spacer and a conserved portion of the 23S subunit in the mitochondrion was unable to differentiate the several species/subspecies of F. distichus (e.g., F. evanescens, F. gardneri, F. anceps; Coyer et al., 2006a; Cánovas et al., 2011; Laughinghouse et al., 2015). Laughinghouse et al. (2015) found a distinct Arctic haplotype, clearly showing the ancestor of the F. distichus complex to be centered in the low Arctic/Subarctic and invoked glacial cycles in maintaining the various morphs.They postulated that during an interglacial period, the central Arctic becomes a mixing bowl, from which populations expand further into the northern regions of the Atlantic/Arctic. As ice advances southward during the following glacial period, these populations disperse south to widely separated suitable habitats and subsequently adapt to local conditions. During the next interglacial, the locally-adapted populations again expand to the central Arctic Ocean and admixture again occurs, thereby diluting the previously evolved local adaptations (Laughinghouse et al., 2015). The opportunity for differential importance of selfing may exist, with greater selfing occurring in the expanded populations during glacial advance than in the admixture during glacial retreat.
Mating system
The evolution of reproductive strategies has been extensively studied in plants as they exhibit a range of mating systems from hermaphroditism to monoecy to dioecy (Geber et al., 1999). In angiosperms, dioecy appears to be the derived state based on theoretical, empirical, and phylogenetic studies (Charlesworth, 2002; Charlesworth, 2006) and in Fucus, dioecy is thought to have evolved from ancestral hermaphroditism (Billard et al., 2005; Billard et al., 2007).
The hypothesis was supported by a recent study of ancestral states in the family Fucales. Using 13 protein-coding genes, Cánovas et al. (2011) established hermaphroditism as ancestral in the family (in accordance with plants), but switching from derived dioecy back to hermaphroditism in one species in each of the two Fucus lineages. The switch to the diploid sex-determination system from the haploid UV (via a hermaphroditic intermediate) occurred in several families of Fucales ~17.5 mya and the transition toward hermaphroditism within diploid lineages has occurred independently in several genera of the Fucaceae (Heesch et al., 2021).
It also may be significant that hermaphroditic species of Fucus frequently occupy exposed or higher shores, whereas dioecious species are found in the more frequently submerged lower or shallow subtidal regions (Vernet & Harper, 1980). Furthermore, multi-gene phylogenies of Fucus suggested that switching from dioecy to hermaphroditism has coincided with colonization of more extreme environments (Billard et al., 2010; Cánovas et al., 2011). Two hypotheses may explain the pattern. First, selfing may be favored among species in the higher shores because more frequent desiccation renders cross-fertilization more hazardous, and secondly, repeated exposure to the same physical conditions of the high shore does not ‘penalize’ the lack of recombination and genetic diversity in hermaphrodites, whereas evolving and high biological diversity/interactions in the more physically stable lower/submerged shores favors genetic diversity provided by dioecy and recombination (Vernet & Harper, 1980; Billard et al., 2010).
A recent and detailed phylogenetic analysis of the low latitude hermaphroditic clade in the Iberian Peninsula and Northern Africa (F. macroguiryi, F. spiralis, F. limitaneus) by Almeida et al. (2022) suggested that the strong metapopulation structure within southern F. vesiculosus (dioecious) in restricted habitats favored parapatric speciation of an ancestral hermaphrodite lineage. A single ancestral hermaphrodite diversification event ~0.54 mya led to the F. macroguiryi and F. limitaneus/F. spiralis clades which differ in vertical ranges on rocky shores, with the morphologically and ecologically similar F. limitaneus and F. spiralis diverging more recently (~0.34 mya). Thus, the evolution of selfing lineages from outcrossing progenitors, a feature that is common among higher plants and some animals, has occurred several times in Fucus (Almeida et al., 2022).
Hybridization
Morphological variability within and between Fucus spp. is legendary (e.g., Sideman and Mathieson, 1985; Rice & Chapman, 1985; Rice et al., 1985; Munda & Kremer, 1997; Kalvas and Kautsky, 1998; Anderson & Scott, 1998). The ability to detect differences in DNA sequences, either indirectly or directly, has provided additional taxonomic characters with which to examine morphological differences and has proved to be especially important in unraveling some of the taxonomic confusion in Fucus, a genus consisting of 717 described names, of which 10 are currently accepted as species (https://www.algaebase.org/search/genus/detail/?genus_id=71).
Some species are more related than others. Using sequences of the internal transcribed region (ITS-1, 5.8S, ITS2) of nuclear ribosomal DNA, Serrão et al. (1999a) established the presence of two lineages (or clades) within the genus: Lineage 1 and Lineage 2 (see Table 1 for species within each Lineage), subsequently supported with the 23S subunit and intergenic spacer regions of mitochondria (Coyer et al., 2006a). Additionally, each Lineage contains hermaphroditic and dioecious species with reciprocal habitat specialist species: (F. spiralis=high intertidal; F. serratus low intertidal/subtidal and generalist species F. vesiculosus = high to low intertidal; F. distichus = high intertidal pools to low intertidal) (Coyer et al., 2006a). More recently, Cánovas et al. (2011) used 13 protein-coding genes to further clarify the two lineages and indicated that species in Lineage 1 are characterized by being northern in distribution, adapted to cold water and stress-receptible, whereas species in Lineage 2 are more southern in distribution and generally stress-tolerable. As discussed below, hybridization in Fucus occurs among species within a Lineage, rarely between, and always involves a parental species pair in which one parent is a hermaphrodite (e.g., F. spiralis, F. distichus) and the other is dioecious (e.g., F. vesiculosus, F. serratus) (Coyer et al., 2007).
A more complicated dynamic is evident in the low latitude hermaphroditic F. spiralis complex. Initially, two genetically and morphologically distinct entities, F spiralis-High and F. spiralis-Low, corresponded to F. spiralis var spiralis and F. spiralis var platycarpus, respectively, in addition to a third entity named F. spiralis-South (Billard et al., 2007; Billard et al., 2010; Coyer et al., 2011c). Using microsatellites in tandem with expressed sequence tags for partial sequencing of 14 protein-coding genes, F. spiralis var platycarpus (F. spiralis-Low) was synonymized with F. spiralis-South as F. guiryi (Zardi et al., 2011), a distinct entity in southern Iberia (allopatry), but as it was also distributed across F. vesiculosus and F. spiralis clades in Northern Portugal (sympatry), extensive hybridization and introgression is possible (Zardi et al., 2011).
A new study using a phylo-transcriptomic approach based on short read transcriptomic data (RNA-seq) of warm affinity Lineage 2 populations existing in sympatry (Britain, NW Iberia) and allopatry (SW Iberia, Morocco) further clarified the complex patterns of cryptic speciation (Almeida et al., 2022). Specifically, F. spiralis-Low diverged earlier than the others, necessitating removal of F. spiralis-Low from F. guiryi and designation as new species F. macroguiryi that is genetically, morphologically, and physiologically distinct from all others and is introgressed F. guiryi (Cánovas et al., 2011; Almeida et al., 2022). F. guiryi on the Canary Islands has thus reverted to the previously described F. limitaneus (Almeida et al., 2022).
Reports of field individuals with an intermediate morphology between two co-existing Fucus species have occurred several times in the past century (Gard, 1910; Gard, 1915; Powell, 1963; see also references in Scott & Hardy, 1994). Some viewed these forms as ecotypes, but others (e.g., McLachan et al., 1971; Scott & Hardy, 1994) stated strongly that hybridization most likely was the root of the considerable morphological variation in Fucus. At that time, verification of hybridization required difficult and labor-intensive crossing studies, although several attempts were made (Thuret, 1855; Williams, 1899; Sauvageau, 1909; Kniep, 1925; Burrows and Lodge, 1951; Burrows and Lodge, 1953; Bolwell et al., 1977; Scott & Hardy, 1994; Kim et al., 1997). Few zygotes resulting from the laboratory crosses between various species of Fucus, particularly between Lineage 1 and 2 species and F. vesiculosus x Ascophyllum nodosum (Kim et al., 1997), survived beyond a few days (e.g., Scott & Hardy, 1994).
The advent of genetic techniques made detection of hybridization much easier and more robust. For example, nuclear and cytoplasmic DNA reveal relatedness among individuals at the population level and above, whereas plastid DNA (mitochondria, chloroplasts) are maternally inherited. Microsatellites in particular have been invaluable for much more detailed studies of hybridization and introgression in many plants and animals, including the genus Fucus, as the bi-parentally inherited markers clearly delineate heterozygosity and parentage using both plastid and nuclear DNA.
The first molecular-based studies of hybridization in Fucus used the nuclear rDNA-ITS1 sequence, the Rubiso spacer in chloroplasts, and nad11gene in mitochondria; demonstrating that the plastids are maternally inherited in Fucus (Coyer et al., 2002a; Coyer et al., 2002b), although some ‘paternal leakage’ has been observed (Coyer et al., 2004; Hoarau et al., 2009). Results confirmed hybridization and introgression between Lineage 1 species F. evanescens (=distichus, see Coyer et al., 2007) and F. serratus in both field specimens and laboratory crosses, and further revealed that: 1) hybridization was asymmetrical, with the dioecious F. serratus contributing sperm and the hermaphroditic F. evanescens the eggs, and 2) hybridization was restricted to sympatric, rather than allopatric stands (Coyer et al., 2002a; Coyer et al., 2002b). Using 10 microsatellite loci of a mixed population consisting of native F. serratus and introduced F. evanescens in Denmark that had existed for 60-100 yrs, revealed: 1) nearly 13% of the individuals were F1 hybrids (also asymmetrical as described above), 2) ca. 1.5% of genes were introgressed into each parental species, and 3) F1 hybrids displayed lower survivorship (Coyer et al., 2007).
Several subsequent studies have employed microsatellites to examine hybridization and introgression in Lineage 2 Fucus spp. Engel et al. (2005) used five loci and found a higher proportion of genetically intermediate individuals (F. vesiculosus x F. spiralis hybrids) in two sympatric populations (12.5 and 14.2%) than in parapatric populations (5.6 and 9.0%). As for F. serratus x F. distichus (Coyer et al., 2002a), F. spiralis (hermaphroditic) eggs were fertilized by F. vesiculosus (dioecious) sperm (Billard et al., 2007). Hybridization was far more common in mixed populations, although did occur in separate distributions. In some cases, F. spiralis x F. vesiculosus F1s were fertile (Billard et al., 2007; Billard et al., 2010). More recently, microsatellite analysis was combined with network analysis to reliably determine the occurrence of present-day hybridization between F. spiralis and F. vesiculosus (Moalic et al., 2011).
Hybridization also has been demonstrated among hermaphroditic species of Lineage 2. Short read transcriptomic data (RNA-seq) consistently indicated gene flow between F. macroguiryi and F. limitaneus, exceeding that between F. macroguiryi and F. spiralis. The pattern is best explained by assuming that F. macroguiryi was present further south than F. spiralis during glacial stages and farther from the ice limits, with extensive gene flow between F. macroguiryi and F. limitaneus in the south during relatively lengthy glacial periods contributing to the observed introgression signal (Almeida et al., 2022).
Clearly, hybrid fitness will determine the fate of a hybrid zone, and three scenarios are possible: 1) if there is no selection against the hybrids and introgression is extensive, all individuals become hybrids; 2) if introgressed individuals become established and/or are adapted for new habitats, new lineages can evolve; and 3) if hybrids are less fit, pre-zygotic isolating barriers can evolve to strengthen selection against formation of hybrids (=reinforcement), as less fit hybrids can be viewed as energetically expensive ‘mistakes’ (summarized in Hoarau et al., 2015). Two lines of evidence suggest that in Fucus, scenario 3 is most likely. First, F. serratus x F. evanescens hybrids have lower survivorship and reduced fertility than either parent (Coyer et al., 2007; Hoarau et al., 2015).
Secondly, reinforcement of pre-zygotic isolation appears to have evolved in older contact zones of F. serratus and F. distichus, but not in younger contact zones. Hoarau et al. (2015) examined hybridization and introgression of the two species in contact zones: 1) near Denmark where F. distichus was introduced ~150 yrs ago, 2) in Iceland where F. serratus was introduced ~150 yrs ago, and 3) northern Norway where the two species have co-existed since the end of the Last Glacial Maximum ~10,000 yrs ago. Both Danish and Icelandic populations revealed a high proportion of hybrids (13-24%) and several F1 individuals, whereas the Norwegian populations displayed a low proportion of hybrids (2-3%) and an absence of F1 individuals (Hoarau et al., 2015). Additionally, the success rate of interspecific laboratory crosses to one-week old embryos was significantly lower in the older contact zones of Norway than in the younger contact zones of Denmark and Iceland, again suggesting selection against hybridization and for pre-zygotic isolation (Hoarau et al., 2015).
Similarly, the introgression signal from F. macroguiryi (hermaphroditic) into F. vesiculosus (dioecious) in secondary contact has decayed, but is still detectable (Almeida et al., 2022). The decay may be due to steady reinforcement of species boundaries in the sympatric range, as observed for F. distichus and F. serratus in Norway (Hoarau et al., 2015), or a consequence of reduced contact time during range expansion (Almeida et al., 2022).
If gamete release in Fucus is delayed by environmental conditions such as high-water motion, mature gametes accumulate in the conceptacles. Several studies have demonstrated that when ripe receptacles are stored in the laboratory for several days before releasing gametes, species-specific barriers are diminished and hybrids can be produced (Bolwell et al., 1977; Edwards et al., 1997; Edwards, 1999). Additionally, fertilization success was significantly reduced when eggs were retained in the receptacles for ~ 3 weeks due to unfavorable environmental conditions for release (Serrão et al., 1999a).
Despite the wide occurrence of hybridization among Fucus spp., pre-zygotic mechanisms, such as asynchronous release of gametes, have evolved to significantly reduce hybridization. Monteiro et al. (2012, 2016) studied gamete release among four sympatric species of Fucus in northern Portugal. Dioecious F. vesiculosus and F. serratus released gametes during daytime neap tides, while hermaphroditic F. guiryi and F. spiralis released gametes during night-time high tides during the same phase of the semilunar cycle, effectively reducing the potential for hybridization with the dioecious F. vesiculosus. As the divergence between hermaphroditic and dioecious species may be > 1 mya (Cánovas et al., 2011), the shift in periods of gamete release is remarkably rapid.
Ecads
An especially interesting aspect of Fucales is the existence of ecads and the role of hybridization and polyploidy in their existence. Ecads are free-living individuals with morphological variability linked to habitat (Clements, 1905); in the case of Fucus, to the low-energy muddy shorelines of estuaries and high-intertidal salt marshes, and presumably arise from attached ‘parental’ species. Fucoid salt marsh ecads have been known for over 100 years for F. vesiculosus and F. spiralis in the North Atlantic (Cotton, 1912; Baker & Bohling, 1916), and more recently F. gardneri (part of F. distichus complex; see Coyer et al., 2006a; Cánovas et al., 2011) in the North Pacific (Ruiz et al., 2000; Kucera & Saunders, 2008). Ecads also are known in the closely related fucoid Ascophyllum nodosum (Chock and Mathieson, 1976; Chock and Mathieson, 1979; Mathieson & Dawes, 2001; Mathieson et al., 2001). All fucoid ecads are characterized by the absence of a holdfast; a dwarf morphology; asexual reproduction; and curled, proliferating thalli (see also references in Wallace et al., 2004) and may be a major source of biomass and productivity in these habitats (Tyrrell et al., 2012; Tyrrell et al., 2015).
In one of the first uses of microsatellites to examine hybridization in Fucus, Wallace et al. (2004) concluded that: 1) the muscoides-like Fucus ecad in Maine (USA) salt marshes consisted mainly of F. vesiculosus x F. spiralis F1 hybrids; 2) another ecad (F. vesiculosus ecad volubilis) may have arisen through introgression between fertile hybrids and F. vesiculosus; and 3) introgression had likely occurred between F. vesiculosus and F. spiralis. A later study using microsatellites and mtDNA analysis showed that muscoides-like Fucus ecads in Iceland were consistent with asymmetrical hybridization between the dioecious F. vesiculosus sperm and hermaphroditic F. spiralis eggs, whereas similar ecads in Ireland were the result of polyploidy (Coyer et al., 2006b).
Sjøtun et al. (2017) examined the complexity of Fucus ecads at three sites in western Ireland. In one location, a morphological cline existed with small Fucus individuals lacking bladders in the upper intertidal salt marshes ranging to F. vesiculosus in mid-intertidal; nuclear DNA content ranged from 1-1.8 pg, suggesting polyploidy in some individuals. At Locality 2, microsatellite analysis revealed salt marsh individuals were derived mainly from F. vesiculosus, whereas at Locality 3, salt marsh individuals were F. vesiculosus x F. spiralis hybrids with greatest affiliation to F. spiralis. DNA content of the small individuals from Locality 2 (ca. 4 pg) suggested they were octoploids, whereas the individuals from Locality 3 formed two groups based on DNA content: one with 3.9-4.6 pg and the other with 1.5-2.8 pg. Furthermore, DNA content of individuals in Locality 3 varied between 1.1-2.8 pg in F. vesiculosus and 2-3.5 pg in F. spiralis, demonstrating a somewhat stepwise increase in both species consistent with polyploidy. The authors hypothesized that the small salt marsh Fucus originated from genome size changes in the parents.
Neiva et al. (2012) used microsatellite loci to examine Fucus ecads from Oregon (US) in the North Pacific and Ireland and concluded that they were more related to F. gardnerii, and F. spiralis, respectively. Additionally, they suggested that fucoid ecads are evolutionarily independent populations stemming from hybrid or polyploid origins that confer a fitness advantage over their parental species in a marginal and/or stressful habitat.
An interesting ecad is F. vesiculosus growing on intertidal mussel beds in the Wadden Sea and along the North Sea coast, first described by Nienburg (1925, 1927) and later by others (Wohlenberg, 1937; Nienhuis, 1970; van den Hoek et al., 1979). The F. vesiculosus ecad lacks the species’ characteristic gas bladders; reproduces vegetatively; and does not have a holdfast, being attached to the muddy substratum via the mussel’s byssal threads (Albrecht & Reise, 1994). The association is mutual: the ecad prevents mussels from sinking into the mud, whereas the mussels anchor the ecads and allows steady growth (Nienburg, 1925; Nienburg, 1927). It is unknown if F. spiralis co-occurs on mussels, so derivation of the ecad by hybridization or polyploidy remains unknown.
Recently, a study of attached (epilithic) and free-living (benthopleustophytic) forms of F. vesiculosus in the Baltic Sea revealed the presence of polyploidy (likely through autopolyploidy) throughout the majority of populations regardless of form with important implications in population structure (Preston et al., 2022).There is no direct evidence of sexual reproduction in the free-living form, which probably originated asexually via detached pieces of thalli aggregating in sheltered locations (Preston et al., 2022), and presumably without a functioning holdfast. Thus, the free-living form is at least ‘ecad like’. Although the free-living form was less genetically diverse than the attached, genetic diversity was still within expected limits for both forms and frequent asexual reproduction in the free-living form did not reduce the overall genetic variation in F. vesiculosus. Gene flow within and among the forms differed at various spatial scales, but the free-living populations were judged to be more unstable and at increased risk of local extinction (Preston et al., 2022).
Evolution of diplontic life cycle and sex-biased genes
In all orders of brown algae, sex is determined in the haploid stage of the gametophyte generation except for the Fucales, where sex is determined via haploid gametes during the diploid state (Coelho et al., 2019). Fucales also are of interest because of the relatively recent transition from haploid to diploid sex determination (Silberfeld et al., 2014), switching from ancestral hermaphroditism to dioecy and in some species, back to hermaphroditism (Billard et al., 2005; Billard et al., 2007; Cánovas et al., 2011). The switching of reproductive method coincides with a dramatic rise in sea levels ca. 75 mya and could have opened new ecological niches for Fucales (Heesch et al., 2021). It also is important to note that hermaphroditic lineages are better colonizers of marginal habitats via increased reproductive assurance and the maintenance of locally adaptive traits (Cánova et al., 2011).
Genes that are differentially expressed in males and females (sex-biased genes) have been well documented across a wide number of animals, plants, and brown algae (see references in Hatchett et al., 2021). A comparative transcriptomic study of vegetative and gender-specific reproductive tissue in F. vesiculosus revealed striking differences (Martins et al., 2013). For example, cell cycle and meiotic pathways were over-expressed in male (not female) reproductive tissue relative to vegetative tissue, as well as genetic information processing pathways associated with sperm production. Further, the number of sex-biased genes were ~3-fold higher in male relative to female tissue and the average expression level of male-biased genes was greater than female-biased genes. Candidate sex-biased genes in females were limited to those with likely roles in cell wall/matrix modification, whereas a variety of male-biased genes were related to development; signaling and signal perception; and potential flagella-localized proteins.
Hatchett et al. (2021) examined the evolution of sex-biased genes in vegetative and reproductive tissue of male and female F. serratus and F. vesiculosus with RNAseq and de novo reference transcriptome assembly. While very few genes were differentially expressed between male and female vegetative tissue (8-9% in each species), thousands of genes were differentially expressed in the reproductive tissues. A similar proportion of the genome displayed tissue-biased expression between receptacle and non-receptacle tissue, demonstrating that the majority of tissue- and sex-biased expression was allocated to the reproductive structures.
The authors also found that male-biased genes were highly conserved between the two species, with clustering of male reproductive samples by sex rather than by species. Furthermore, overexpression of male-biased genes was >3-fold the number of female-biased genes and conserved male-biased genes were enriched in functions related to gamete production, sperm competition, and flagellar proteins. The increase in male biased gene expression of the transcriptome also suggested that males may experience relaxed purification selection or stronger selection than females, a trait found in many other species of eukaryotes (Hatchett et al., 2021 and references therein). Female-biased genes were uniformly and highly expressed throughout the female and male tissues, thus sexual conflict over gene expression in Fucus may be resolved by down-regulating expression of pleiotropic female genes in male receptacles and restricting expression of male-biased genes to the male reproductive tissue resulting in an increase of male biased gene expression.
Climate change and Fucus reproduction
Several studies employing ecological niche modeling or other species distribution models (SDMs) comparing present-day vs. projected future distributions have been performed for Fucus under contrasting IPCC (Intergovernmental Panel on Climate Change) climate change scenarios (e.g., Nicastro et al., 2013; Assis et al., 2014; Jueterbock et al., 2014; Jueterbock et al., 2016). In general, all have demonstrated a northward shift into expanding suitable habitat and decline or extinction in the southern edge populations due to rising temperatures. For example, experiments with F. serratus confirmed that thermal extremes will regularly reach physiologically stressful levels in Brittany (France) and further south by the end of the 22nd century (Jueterbock et al., 2014).
On the other hand, expansion into northern habitats will require adaptations to cooler water and 24 hr light/dark months. Recent studies in Fucus species have focused on how climate change influences average seawater temperature, salinity and pCO2 and how these changes affect reproductive success. Predicted future changes in seawater conditions for F. radicans in the Northern Baltic Sea, showed a high tolerance in photosynthesis and growth, but decreased survival and cessation of sexual reproduction (Rothäusler et al., 2018; Rugiu et al., 2018). In the southwest Baltic Sea (Kiel Fjord), F. vesiculosus was unaffected by elevated pCO2 and/or warming, but matured earlier with a subsequent earlier gamete release (Graiff et al., 2017). Furthermore, southern edge populations of F. vesiculosus are exposed to higher sea and air temperatures (a proxy for climate change) have significantly lower biomass of reproductive tissue and smaller number of receptacles per individual (Ferreira et al., 2015).
Additionally, increased glacial melting may decrease salinity in some areas. For example, F. vesiculosus along the Finnish coast currently tolerates 5.8 PSU, but this is projected to reach 2.5 PSU by the end of the century (Meier et al., 2012). At 2.5 PSU egg release was reduced and at 3.5 PSU, sperm cells began to swell, drastically reducing reproductive success (Rothäusler et al., 2019). As discussed above, low salinities also increase the probability of lethal polyspermy (see ‘General Characteristics, above).
Future directions
The continuing and rapid development of the ‘omics’ (genomics, transcriptomics, proteomics, metabolomics), as well as CRISPR/Cas9 techniques, provide invaluable tools to address even more questions in Fucus reproduction (as well as evolutionary history), especially with the future release of full genome data for several species (Table 2), and the ease of laboratory culturing and subsequent manipulation of gene lines. There also, however, remains the need for basic investigations in reproductive ecology, particularly among the lesser studied Lineage 1 species. Taking a larger view, investigating the various modes of reproduction (e.g., hermaphroditism, dioecy, hybridization, selfing, asexual reproduction, ecads) in Fucus may well lead to new insights in the study of reproduction in other organisms with a diplontic life cycle, consequently, Fucus can be a useful model organism. We offer below a small subset of questions to stimulate thoughts of future research.
1. Why is Lineage 2 much more specious than Lineage 1 and how can whole genome analysis (and genome-wide markers such as SNPs) resolve the numerous and controversial number of species in Lineage 2? Although the genus evolved in, and radiated from, the central Arctic (Cánovas et al., 2011; Laughinghouse et al., 2015), far more species have emerged in the relatively younger North Atlantic than in the older North Pacific. Is it simply that there was less competition from other species of algae in the North Atlantic or did the diplontic life cycle of reproduction favor Fucus spp. in a younger habitat?
2. How/why is F. vesiculosus so successful in such diverse habitats (e.g., sheltered to moderately exposed, rocky shores to salt marshes, degree of immersion/emersion, brackish to marine salinity)? Comparative genomics/transcriptomics may reveal the presence/absence of genes, differential levels of gene expression, and/or an important role of epigenetics (heritable and reversible changes in transgenerational phenotypes without corresponding changes in DNA sequence) that may be unique to F. vesiculosus.
3. The existence of ecads leads to many ecological and genomic questions. For example, what are the longevity and growth patterns of ecads resulting from hybridization relative to those arising from polyploidy and how are both influenced by the local environment? From a genomic perspective: 1) what is the genetic basis for the hybridization or polyploidy dichotomy and is it reversible; 2) is the genome size divergence (e.g., Sjøtun et al., 2017) due to non-coding elements or gene duplication, 3) how do the mechanisms of inducement differ in dioecious (F. vesiculosus) and hermaphroditic species (F. distichus); and 4) is epigenetics a key factor or are different regions/genes in the genome important? Finally, is the F. vesiculosus ecad on mussels in the Wadden Sea a result of hybridization or polyploidy?
4. How does rate of selfing in hermaphroditic species vary with changing environments?
5. How does frequency of hybridization vary as a function of stochastic and dynamic environmental conditions (field and laboratory cultures)?
6. Detailed field studies of gamete release and fertilization success of Lineage 1 species are needed for comparison to the much more studied F. spiralis and F. vesiculosus in Lineage 2. For example, hermaphroditic F. distichus shares shore position with the dioecious F. vesiculosus and F. serratus is mostly subtidal with no equivalent in Lineage 1. How do aspects of reproduction in Lineage 1 vary as a function of tidal cycle, light, and water motion? Given the different shore positions, how do selfing rates and success differ when comparing F. spiralis and F. distichus?
7. What is the genetic basis for the morphological differences (particularly receptacles) among the various morphs of F. distichus (F. anceps, F. evanescens, F. edentatus, F. gardneri, etc.) and F. vesiculosus (with and without vesicles)? Is epigenetics present in Fucus spp.?
8. Does the metabolome (see Parrot et al., 2019) on Fucus fronds differ from that on receptacles and if so, why, and how do the differences influence gamete release?
9. What is the genomic/transcriptomic basis for non-overlapping reproductive seasons of F. vesiculosus observed in the Baltic Sea?
10. What is the genetic basis for reproductive isolation and the connection between prezygotic barriers to fertilization and within-species sexual selection?
11. How can whole genome sequencing identify sex chromosomes and molecules involved in sperm/egg receptors?
12. How will global changes (e.g., temperature, salinity, dissolved CO2) affect aspects of Fucus reproduction (development time, gamete output/size/dispersal, fertilization, dispersal of fertilized eggs, etc.)?
Author contributions
WH: Original concept, drafting and editing of the manuscript, producing/providing figures, and tables. JC: Drafting and editing of the manuscript, design of figures. KS: Table and editing of the manuscript. AJ: Editing of the manuscript. GH: Editing of the manuscript. All authors contributed to the article and approved the submitted version.
Funding
This research was funded by Nord University.
Acknowledgments
Dedicated to the late Dr. Art Mathieson.
Conflict of interest
The authors declare that the research was conducted in the absence of any commercial or financial relationships that could be construed as a potential conflict of interest.
Publisher’s note
All claims expressed in this article are solely those of the authors and do not necessarily represent those of their affiliated organizations, or those of the publisher, the editors and the reviewers. Any product that may be evaluated in this article, or claim that may be made by its manufacturer, is not guaranteed or endorsed by the publisher.
Supplementary material
The Supplementary Material for this article can be found online at: https://www.frontiersin.org/articles/10.3389/fmars.2022.1051838/full#supplementary-material
References
Albrecht A., Reise K. (1994). Effects of Fucus vesiculosus covering intertidal mussel beds in the wadden Sea. Helgoländer wissenschaftliche Meeresuntersuchungen. 48, 243–256. doi: 10.1007/BF02367039
Almeida S. C., Neiva J., Sousa F., Martins N., Cox C. J., Melo-Ferreira J., et al. (2022). A low-latitude species pump: peripheral isolation, parapatric speciation and mating-system evolution converge in a marine radiation. Mol. Ecol 31, 4797–4817. doi: 10.1111/mec.16623
Almeida S. C., Nicastro K. R., Zardi G. I., Pearson G. A., Valero M., Serrão E. A. (2017). Reproductive strategies and population genetic structure of Fucus spp. across a northeast Atlantic biogeographic transition. Aquat. Living Resources. 30, 16. doi: 10.1051/alr/2017012
Anderson C. I. H., Scott G. W. (1998). The occurrence of distinct morphotypes within a population of Fucus spiralis. J. Mar. Biol. Association UK. 78, 1003–1006. doi: 10.1017/S0025315400044933
Andersson A., Kautsky L., Kalvas A. (1994). Circadian and lunar gamete release in Fucus vesiculosus in the atidal Baltic Sea. Mar. Ecol. Prog. Series. 110, 195–201. doi: 10.3354/meps110195
Ang P. O. (1991). Natural dynamics of a Fucus distichus (Phaeophyceae, Fucales) population: reproduction and recruitment. Mar. Ecol. Prog. Series. 78, 71–85. doi: 10.3354/meps078071
Ardehed A., Johansson D., Schagerström E., Kautsky L., Johannesson K., Pereyra R. T. (2015). Complex spatial clonal structure in the macroalgae Fucus radicans with both sexual and asexual recruitment. Ecol. Evolution. 5, 4233–4245. doi: 10.1002/ece3.1629
Ardehed A., Johansson D., Sundqvist L., Schagerström E., Zagrodzka Z., Kovaltchouk N. A., et al. (2016). Divergence within and among seaweed siblings (Fucus vesiculosus and F. radicans) in the Baltic Sea. PloS One 11 (8), e0161266. doi: 10.1371/journal.pone.0161266
Arrontes J. (1993). The nature of the distributional boundary of Fucus serratus on the north shore of Spain. Mar. Ecol. Prog. Series. 93, 183–193. doi: 10.3354/meps093183
Assis J., Serrão E. R., Claro B., Perrin C., Pearson G. A. (2014). Climate-driven range shifts explain the distribution of extant gene pools and predict future loss of unique lineages in a marine brown alga. Mol. Ecology. 23, 2797–2810. doi: 10.1111/mec.12772
Bäck S., Collins J. C., Russell G. (1991). Aspects of the reproductive biology of Fucus vesiculosus from the coast of SW Finland. Ophelia 34, 129–141. doi: 10.1080/00785326.1991.10429701
Baker S. M. (1909). On the causes of the zoning of brown seaweeds on the seashore. New Phytol. 8, 196–202. doi: 10.1111/j.1469-8137.1909.tb05523.x
Baker S. M. (1910). On the causes of the zoning of brown seaweeds on the seashore. part II.-the effect of periodic exposure on the expulsion of gametes and on the germination of the oospore. New Phytol. 9, 54–67. doi: 10.1111/j.1469-8137.1910.tb05553.x
Baker S. M., Bohling M. H. (1916). On the brown seaweeds of the salt marsh. part II. their systematic relationships, morphology, and ecology. J. Linn. Society. Botany. 43, 325–380. doi: 10.1111/j.1095-8339.1916.tb00609.x
Balasse M., Tresset A., Dobney K., Ambrose S. H. (2005). The use of isotope ratios to test for seaweed eating in sheep. J. Zoology. 266, 283–291. doi: 10.1017/S0952836905006916
Battelli C. (2016). Disappearance of Fucus virsoides J. agardh from the Slovenian coast (Gulf of Trieste, northern Adriatic). Ann. Istrian Mediterr. Stud. Ser. Hist. Nat. 26, 1–13. doi: 10.19233/ASHN.2016.1
Berger R., Malm T., Kautsky L. (2001). Two reproductive strategies in Baltic Fucus vesiculosus (Phaeophyceae). Eur. J. Phycology. 36, 265–273. doi: 10.1080/09670260110001735418
Berndt M. L., Callow J. A., Brawley S. H. (2002). Gamete concentrations and timing and success of fertilization in a rocky shore seaweed. Mar. Ecol. Prog. Series. 226, 273–285. doi: 10.3354/meps226273
Billard E., Serrão E., Neiva J., Pearson G., Destome C., Valero M. (2007). A mosaic of hybrids between species with a contrasting reproductive system at the micro-spatial-scale of the shore: phenotypic and genetic analyses (PhD Dissertation Universidade do Algarve and Universite Paris VI), 87–129.
Billard E., Serrão E., Pearson G., Destombe C., Valero M. (2010). Fucus vesiculosus and spiralis species complex: a nested model of local adaptation at the shore level. Mar. Ecol. Prog. Ser. 405, 163–174. doi: 10.3354/meps08517
Billard E., Serrão E., Pearson G. A., Engel C. R., Destombe C., Valero M. (2005). Analysis of sexual penotype and prezygotic fertility in natural populations of F. spiralis, F. vesiculosus (Fucaceae, Phaeophyceae) and their putative hybrids. Eur. J. Phycology. 40, 397–407. doi: 10.1080/09670260500334354
Björk S. (1995). A review of the history of the Baltic Sea, 13.0-8.0 kaBP. Q. Int. 27, 19–40. doi: 10.1016/1040-6182(94)00057-C
Blanz M., Mainland I., Richards M., Balasse M., Ascough P., Wolfhagen J., et al. (2020). Identifying seaweed consumption by sheep using isotope analysis of their bones and teeth: Modern reference δ13C and δ15N values and their archaeological implications. J. Archaeological Science. 118, 1–25. doi: 10.1016/j.jas.2020.105140
Bolwell G. P., Callow J. A., Callow M. E., Evans L. V. (1977). Cross-fertilization in fucoid seaweeds. Nature 268, 626–627. doi: 10.1038/268626a0
Bower F. O. (1880). On the development of the conceptacle in the Fucaceae. Q. J. Microscopical Science. 20, 36–49. doi: 10.1086/331762
Brawley S. H. (1990). “Polyspermy blocks in fucoid algae and the occurrence of polyspermy in nature,” in Mechanism of fertilization, vol. H45 . Ed. Dale B. (Verlag, Berlin: Springer), 419–431. doi: 10.1007/978-3-642-83965-8_28
Brawley S. H. (1991). The fast block against polyspermy in fucoid algae is an electrical block. Dev. Biol. 144, 94–106. doi: 10.1016/0012-1606(91)90482-i
Brawley S. H. (1992). Fertilization in natural population of the dioecious brown alga Fucus ceranoides and the importance of the polyspermy block. Mar. Biol. 113, 145–157. doi: 10.1007/BF00367648
Brawley S. H., Johnson L. E., Pearson G. A., Speransky V., Rui L., Serrao E. (1999). Gamete release at low tide in fucoid algae: maladaptive or advantageous. Am. Zoologist. 39, 218–229. doi: 10.1093/icb/39.2.218
Brawley S. H., Wetherbee R., Quatrano R. S. (1976). Fine structural studies of the gametes and embryo of Fucus vesiculosus L. (Phaeophyta). J. Cell Science. 20, 233–254. doi: 10.1242/jcs.20.2.233
Burrows E. M., Lodge S. M. (1951). Autecology and the species problem in Fucus. J. Mar. Biol. Assoc. UK. 30, 161–175. doi: 10.1017/S0025315400012650
Burrows E. M., Lodge S. M. (1953). Culture of Fucus hybrids. Nat. (London). 172, 1009–1010. doi: 10.1038/1721009a0
Callow M. E., Evans L. V., Bolwell G. P., Callow J. A. (1978). Fertilization in brown algae. i. SEM and other observations on Fucus serratus. J. Cell Science. 32, 45–54. doi: 10.1242/jcs.32.1.45
Campbell D. H. (1889). The study of Fucus in inland laboratories. Botanical Gazette 14, 182–182. doi: 10.1086/326432
Cánovas F. G., Mota C. F., Serrão E. A., Pearson G. A. (2011). Driving south: a multi-gene phylogeny of the brown algal family Fucaceae reveals relationships and recent drivers of a marine radiation. BMC Evolutionary Biol. 11, 371. doi: 10.1186/1471-2148-11-371
Carlson L. (1991). Seasonal variation in growth, reproduction and nitrogen content of Fucus vesiculosus L. in the Öresund, southern Sweden. Botanica Marina 34 (5), 447–54. doi: 10.1515/botm.1991.34.5.447
Catarino M. D., Siva A. M. S., Cardoso S. M. (2018). Phycochemical constituents and biological activities of Fucus spp. Mar. Drugs 16 (249), 1–34. doi: 10.3390/md16080249
Chapman A. R. O. (1995). Functional ecology of fucoid algae: twenty-three years of progress. Phycologia 34, 1–32. doi: 10.2216/i0031-8884-34-1-1.1
Chapman A. R. O., Johnson C. R. (1990). Disturbance and organization of macroalgal assemblages in the Northwest Atlantic. Hydrobiologia 192, 77–121. doi: 10.1007/BF00005687
Charlesworth D. (2002). Plant sex determination and sex chromosomes. Heredity 88, 94–101. doi: 10.1038/sj.hdy.6800016
Charlesworth D. (2006). Evolution of plant breeding systems. Curr. Biol. 16, R726–R735. doi: 10.1016/j.cub.2006.07.068
Chock J. S., Mathieson A. C. (1976). Ecological studies of the saltmarsh ecad scorpioides (Hornemann) of Asophyllum nodosum (L). Le Jolis. J. Exp. Mar. Biol. Ecology. 23, 171–190. doi: 10.1016/0022-0981(76)90140-4
Chock J. S., Mathieson A. C. (1979). Physiological ecology of Ascophyllum nodosum ((L). Le Jolis and its detached ecad scorpioides (Hornemann) Hauck) Fucales, Phaeophyta. Botanica Marina XXII, 21–26. doi: 10.1515/botm.1979.22.1.21
Clements F. E. (1905). Research methods in ecology (Lincoln, NE: Lincoln University Publishing Company). doi: 10.1126/science.22.550.45
Coelho S. M., Mignerot L., Cock J. M. (2019). Origin and evolution of sex-determination systems in the brown algae. New Phytologist. 222, 1751–1756. doi: 10.1111/nph.15694
Coelho S., Rijstenbil J. W., Sousa-Pinto I., Brown M. T. (2001). Cellular responses to elevated light levels in Fucus spiralis embryos during the first days after fertilization. Plant Cell Environ. 24 (8), 801–810. doi: 10.1046/j.0016-8025.2001.00731.x
Coleman M. A., Brawley S. H. (2005). Are life history characteristics good predictors of genetic diversity and structure? a case study of the intertidal alga Fucus spiralis (Heterokontophyta; Phaeophyceae). J. Phycology. 41, 753–762. doi: 10.1111/j.1529-8817.2005.00102.x
Colvard N. B., Carrington E., Helmuth B. (2014). Temperature-dependent photosynthesis in the intertidal alga Fucus gardneri and sensitivity to ongoing climate change. J. Exp. Mar. Biol. Ecology. 465, 6–12. doi: 10.1016/j.jembe.2014.05.001
Colvard N. B., Helmuth B. (2017). Nutrients influence the thermal ecophysiology of an intertidal macroalga: multiple stressors or multiple drivers? Ecol. Applications. 27, 669–681. doi: 10.1002/eap.1475
Cotton A. D. (1912). Clare Island survey., Part 15: marine algae. Proc. Roy. Irish Acad 31, 178. doi: 10.5962/bhl.title.64352
Coyer J. A., Hoarau, Costa J. F., Hogerdijk B., Serrão E. A., Billard E., et al. (2011c). Evolution and diversification within the intertidal brown macroalgae Fucus spiralis/F. vesiculosus species complex in the north Atlantic. Mol. Phylogenet. Evolution. 58, 283–296. doi: 10.1016/j.ympev.2010.11.015
Coyer J. A., Hoarau G., Oudot-le Secq M.-P., Stam W. T., Olsen J. L. (2006a). A mtDNA-based phylogeny of the brown algal genus Fucus (Heterokontophyta; phaeophyta). Mol. Phylogenet. Evolution. 39, 209–222. doi: 10.1016/j.ympev.2006.01.019
Coyer J. A., Hoarau G., Pearson G., Mota C., Jüterbock A., Alpermann T., et al. (2011a). Genomic scans detect signatures of selection along a salinity gradient in populations of the intertidal seaweed Fucus serratus on a 12 km scale. Mar. Genomics 4, 41–49. doi: 10.1016/j.margen.2010.12.003
Coyer J. A., Hoarau G., Pearson G. A., Serrão E. A., Stam W. T., Olsen J. L. (2006b). Convergent adaptation to a marginal habitat by homoploid hybrids and polyploidy ecads in the seaweed genus, Fucus. Biol. Letters. 2, 405–408. doi: 10.1098/rsbl.2006.0489
Coyer J. A., Hoarau G., Stam W. T., Olsen J. L. (2004). Geographically-specific heteroplasmy of mitochondrial DNA in the seaweed, Fucus serratus (Heterokontophyta: Phaeophyceae, Fucales). Mol. Ecology. 13, 1323–1326. doi: 10.1111/j.1365-294X.2004.02128.x
Coyer J. A., Hoarau G., Stam W., Olsen J. (2007). Hybridization and introgression in a mixed population of the intertidal seaweeds Fucus evanescens and F. serratus. J. Evolutionary Biol. 20, 2322–2333. doi: 10.1111/j.1420-9101.2007.01411.x
Coyer J., Hoarau G., van Schaik J., Luijckx P., Olsen J. (2011b). Trans-pacific and trans-Arctic pathways of the intertidal macroalga Fucus distichus L. reveal multiple glacial refugia and colonizations from the north pacific to the north Atlantic. J. Biogeography. 38, 756–771. doi: 10.1111/j.1365-2699.2010.02437.x
Coyer J. A., Peters A. F., Hoarau G., Stam W. T., Olsen J. L. (2002a). Inheritance patterns of ITS1, chloroplasts and mitochondria in artificial hybrids of the seaweeds Fucus serratus and F. evanescens (Phaeophyceae). Eur. J. Phycology. 37, 173–178. doi: 10.1017/s0967026202003682
Coyer J. A., Peters A. F., Hoarau G., Stam W. T., Olsen J. L. (2002b). Hybridization of the marine seaweeds, Fucus serratus and F. evanescens (Heterokontophyta; Phaeophyceae) in a century-old zone of secondary contact. Proc. R. Soc. London. B. 269, 1829–1834. doi: 10.1098/rspb.2002.2093
Coyer J. A., Peters A. F., Stam W. T., Olsen J. L. (2003). Post-ice age recolonization and differentiation of Fucus serratus L. (Phaeophyceae; Fucaceae) population in northern Europe. Mol. Ecology. 12, 1817–1829. doi: 10.1046/j.1365-294x.2003.01850.x
Coyer J. A., Veldsink J. H., Jones K., Stam W. T., Olsen J. L. (2002c). Characterization of microsatellite loci in the marine seaweeds, Fucus serratus and F. evanescens (Heterokontophyta; Fucaceae). Mol. Ecol. Notes 2 (1), 35–37. doi: 10.1046/j.1471-8286.2002.00138.x
d’Avack E. A. S., Marshall C. (2015). “Fucus serratus, sponges and ascidians on tide-swept lower eulittoral rock,” in Marine life information network: Biology and sensitivity key information reviews. Eds. Tyler-Walters H., Hiscock K. Sensitivity Key Information Reviews Plymouth: Marine Biological Association of the United Kingdom. doi: 10.17031/marlinhab.42.1
Decaisne M. M. J., Thuret G. (1845). LVII. on the antheridia and spores of some species of Fucus. Ann. Magazine Natural History: Ser. 1 14 (94), 480–481. doi: 10.1080/037454809495230
Denny M. W., Shibata M. F. (1989). Consequences of surf-zone turbulence for settlement and external fertilization. Am. Naturalist. 134, 859–889. doi: 10.1086/285018
Edelstein T., McLachlan J. (1975). Autecology of Fucus distichus ssp. distichus (Phaeophyceae: Fucales) in Nova Scotia, Canada. Mar. Biol. 30, 305–324. doi: 10.1007/BF00390636
Edwards G. O. (1999). Cross-fertilisation in Fucus: a molecular approach (PhD Thesis, University of Birmingham).
Edwards G. O., Callow J. A., Brownlee C. (1997). Cross-fertilization in Fucus: a molecular approach. Phycologia 36 (Abstract), 28. doi: 10.2216/i0031-8884-36-4S-1.1
Engel C. R., Brawley S. H., Edwards K. J., Serrão E. (2003). Isolation and cross-species amplification of microsatellite loci from the fucoid seaweeds Fucus vesiculosus, F. serratus and Ascophyllum nodosum (Heterokontophyta, Fucaceae). Molular Ecol. Notes 3, 180–182. doi: 10.1046/j.1471-8286.2003.00390.x
Engel C. R., Daguin C., Serrão E. (2005). Genetic entities and mating system in hermaphroditic Fucus spiralis and its close dioecious relative F. vesiculosus (Fucaceae, Phaeophyceae). Mol. Ecology. 14, 2033–2046. doi: 10.1111/j.1365-294X.2005.02558.x
Eriksson M., Rafajlović M. (2021). The effect of the recombination rate between adaptive loci on the capacity of a population to expand its range. Am. Naturalist. 197, 526–542. doi: 10.1086/713669
Evans L. V. (1962). Cytological studies in the genus Fucus. Ann. Botany. 26, 345–346. doi: 10.1093/oxfordjournals.aob.a083799
Falace A., Tamburello L., Guarnieri G., Kaleb S., Papa L., Fraschetti S. (2018). Effects of a glyphosate-based herbicide on Fucus virsoides (Fucales, Ochrophyta) photosynthetic efficiency. Environ. Pollution. 243, 912–918. doi: 10.1016/j.envpol.2018.08.053
Farmer J. B., Williams J. L. (1897). On fertilisation, and the segmentation of the spore, in Fucus. Proc. R. Soc. London 60, 188–195. doi: 10.1098/rspl.1896.0036
Farmer J. B., Williams J. L. (1898). X. Contributions to our knowledge of the Fucaceae: their life-history and cytology. Philosopical Trans. Biol. 190, 623–645. doi: 10.1098/rstb.1898.0010
Ferreira J. G., Hawkins S. J., Jenkins S. R. (2015). Patterns of reproductive traits of fucoid species in core and marginal populations. Eur. J. Phycology. 50, 457–468. doi: 10.1080/09670262.2015.1066036
Fisher R. A. (1941). Average excess and average effect of a gene substitution. Ann. Eugenics 11, 53–63. doi: 10.1111/j.1469-1809.1941.tb02272.x
Forslund H., Kautsky L. (2012). Reproduction and reproductive isolation in Fucus radicans (Phaeophyceae). Mar. Biol. Res. 9, 321–326. doi: 10.1080/17451000.2012.731694
Fredriksen S. (1985). En fenologisk undersøkelse av utvalgte benthiske arter (Universitetet i Bergen, Bergen: Hovedfagsoppgave i Marinbiologi).
Fredriksen S. (1990). Fenologiske undersøkelser av tangarter fra sør-norge og nord-norge. Blyttia 48, 3–7.
Freundlich M. M. (1963). Origin of the electron microscope. Sci. (1979) 142, 185–188. doi: 10.1126/science.142.3589.185
Fritsch F. E. (1945). “Fucales,” in The structure and reproduction of the algae, vol. II. (Cambridge, London), 322–389.
Fulcher R. G., McCully M. E. (1969). Histological studies on the genus Fucus IV. regeneration and adventive embryony. Can. J. Botany. 47, 1643–1649. doi: 10.1139/b69-239
Fulcher R. G., McCully M. E. (1971). Histological studies on the genus Fucus. v. an autoradiographic and electron microscopic study of the early stages of regeneration. Can. J. Botany. 49, 161–165. doi: 10.1139/b71-027
Gard M. (1910). Sur un hybride de Fucus platycarpus et Fucus ceranoides. Comptes rendus l'Académie Des. Science. III. 151, 888–890. doi: 10.1007/BF01785747
Gard M. (1915). Sur un hybride des Fucus ceranoides L. et F. vesiculosus L. Comptes rendus l'Académie Des. Science. 160, 323–325.
Geber M. A., Dawson T. E., Delph L. F. (1999). Gender and sexual dimorphism in flowering plants (Berlin: Springer). doi: 10.1007/978-3-662-03908-3
Gómez Garreta (2000). Flora Phycologica Iberica. Vol. 1. Fucales. (Murcia: Servicio de Publicaciones, Universidad de Murcia), 192 pp.
Graiff A., Dankworth M., Wahl M., Karsten U., Bartsch I. (2017). Seasonal variations of Fucus vesiculosus fertility under ocean acidification and warming in the western Baltic Sea. Botanica Marina 60, 239–55. doi: 10.1515/bot-2016-0081
Gunnill F. C. (1980). Demography of the intertidal brown alga Pelvetia fastigiata in southern California, USA. Mar. Biol. 59, 169–179. doi: 10.1007/BF00396865
Hansen B. B., Lorentzen J. R., Welker J. M., Varpe Ø., Aanes R., Beumer L. T., et al. (2019). Reindeer turning maritime: Ice-locked tundra triggers changes in dietary niche utilization. Ecosphere 10 (4), e02672. doi: 10.1002/ecs2.2672
Hatchett W. J., Jueterbock A., Kopp M., Coyer J. A., Coelho S. M., Hoarau G., et al. (2021). Evolutionary dynamics of sex-biased gene expression in a young XY system: insights from brown algae. bioRiv. doi: 10.1101/2021.08.12.455804
Heather J. M., Chain B. (2016). The sequence of sequencers: The history of sequencing DNA. Genomics 107, 1–8. doi: 10.1016/j.ygeno.2015.11.003
Heesch S., Serrano-Serrano M., Barrera-Redondo J., Luthringer R., Peters A. F., Destombe C., et al. (2021). Evolution of life cycles and reproductive traits: insights from the brown algae. J. Evolutionary Biol. 34, 992–1009. doi: 10.1111/jeb.13880
Hoarau G., Coyer J. A., Giesbers M. C. W. G., Jueterbock. A., Olsen J. L. (2015). Pre-zygotic isolation in the macroalgal genus Fucus from four contact zones spanning 100–10 000 years: a tale of reinforcement? R. Soc. Open Science. 2, 140538. doi: 10.1098/rsos.140538
Hoarau G., Coyer J. A., Olsen J. L. (2009). Paternal leakage of mitochondrial DNA in a Fucus (Phaeophyceae) hybrid zone. J. Phycology. 45, 621–624. doi: 10.1111/j.1529-8817.2009.00679.x
Hoarau G., Coyer J. A., Veldsink J. H., Stam W. T., Olsen J. L. (2007). Glacial refugia and recolonization pathways in the brown seaweed Fucus serratus. Mol. Ecology. 16, 3606–3616. doi: 10.1111/j.1365-294X.2007.03408.x
van den Hoek C., Admiraal W., Colijn F., de Jonge V. N. (1979). “The role of algae and seagrasses in the ecosystem of the wadden Sea,” in Flora and vegetation of the wadden Sea. Ed. Wolff W. J. (Balkema. Rotterdam), 9–118.
Hughey J. R., Gabrielson P. W. (2017). The complete mitogenome of the rockweed Fucus distichus (Fucaceae, Phaeophyceae). Mitochondrial DNA Part B 2 (1), 203–204. doi: 10.1080/23802359.2017.1310604
Igić B., Bohs L., Kohn J. R. (2006). Ancient polymorphism reveals unidirectional breeding system shifts. Proc. Natl. Acad. Science. 103, 1359–1363. doi: 10.1073/pnas.0506283103
Jarne P., Auld J. R. (2006). Animals mix it up too: the distribution of self-fertilization among hermaphroditic animals. Evolution 60, 1816–1824. doi: 10.1111/j.0014-3820.2006.tb00525.x
Johannesson K., Forslund H., Capetilo Å., Kautsky L., Johansson D., Pereyra R. T., et al. (2012). Phenotypic variation in sexually and asexually recruited individuals of the Baltic Sea endemic macroalga Fucus radicans: in the field and after growth in a common-garden. BMC Ecology. 12, 2. doi: 10.1186/1472-6785-12-2
Johannesson K., Johansson D., Larsson K. H., Huenchuñir C. J., Perus J., Forslund H., et al. (2011). Frequent clonality in fucoids (Fucus radicans and Fucus vesiculosus; Fucales Phaeophyceae) in the Baltic Sea. J. Phycology. 47, 990–998. doi: 10.1111/j.1529-8817.2011.01032.x
Johnson L. E., Brawley S. H., Adey W. H. (2012). Secondary spread of invasive species: historic patterns and underlying mechanisms of the continuing invasion of the European rockweed Fucus serratus in eastern north America. Biol. Invasions 14 (1), 79–97. doi: 10.1007/s10530-011-9976-z
Jueterbock A., Kollias S., Smolina I., Fernandes J. M. O., Coyer J. A., Olsen J. L., et al. (2014). Thermal stress resistance of the brown alga Fucus serratus along the north-Atlantic coast: Acclimatization potential to climate change. Mar. Genomics 13, 27–36. doi: 10.1016/j.margen.2013.12.008
Jueterbock A., Smolina I., Coyer. J. A., Hoarau G. (2016). The fate of the Arctic seaweed Fucus distichus under climate change: an ecological niche modeling approach. Ecol. Evol. doi: 10.1002/ece3.2001
Kalvas A., Kautsky L. (1998). Morphological variation in Fucus vesiculosus populations along temperature and salinity gradients in Iceland. J. Mar. Biol. Ass. U.K. 78, 985–1001. doi: 10.1017/S0025315400044921
Kashutin A. N., Klimova A. V., Klochkova N. G. (2019). The seasonal growth dynamics of Fucus distichus subsp. evanescens (C. agardh) H.T. Powell 1957 (Phaeophyceae: Fucales) in the avacha bay (Southeastern kamchatka). Russian J. Mar. Biol. 45 (4), 275–282. doi: 10.1134/S1063074019040096
Kaunitz J. D. (2015). The discovery of PCR: ProCuRement of divine power. Dig Dis. Sci. 60, 2230–2231. doi: 10.1007/s10620-015-3747-0
Kim K. Y., O’Leary S. J., Garbary D. J. (1997). Artificial hybridization between Ascophyllum nodosum and Fucus vesiculosus (Phaeophyceae) from Nova Scotia, Canada. Can. J. Botany. 75, 1133–1136. doi: 10.1139/b97-124
Kinnby A., Pereyra R. T., Havenhand J. N., De Wit P., Jonsson P. R., Pavia H., et al. (2019). Factors affecting formation of adventitious branches in the seaweeds Fucus vesiculosus and F. radicans. BMC Ecology. 19, 22. doi: 10.1186/s12898-019-0239-7
Kniep H. (1925). Über Fucus-bastarde. Flora oder Allgemeine Botanische Zeitung 118–119, 331–338. doi: 10.1016/S0367-1615(17)32895-1
Knight M., Parke M. (1950). A biological study of Fucus vesiculosus L. and F. serratus l. J. Mar. Biol. Assoc. United Kingdom 29, 439–514. doi: 10.1017/S0025315400055454
Kropf D. L. (1992). Establishment and expression of cellular polarity in fucoid zygotes. Microbiological Rev. 56, 316–339. doi: 10.1128/mr.56.2.316-339.1992
Kucera H., Saunders G. W. (2008). Assigning morphological variants of Fucus (Fucales, Phaeophyceae) in Canadian waters to recognized species using DNA barcoding. Botany 86, 1065–1079. doi: 10.1139/B08-056
Ladah L., Bermudez R., Pearson G., Serrão E. (2003). Fertilization success and recruitment of dioecious and hermaphroditic fucoid seaweeds with contrasting distributions near their southern limit. Mar. Ecol. Prog. Ser. 262, 173–183. doi: 10.3354/meps262173
Ladah L. B., Feddersen F., Pearson G. A., Serrão E. A. (2008). Egg release and settlement patterns of dioecious and hermaphroditic fucoid algae during the tidal cycle. Mar. Biol. 155, 583–591. doi: 10.1007/s00227-008-1054-4
Landsborough D. (1857). A popular history of British Sea weeds. 3rd Ed (London: John Edward Taylor). doi: 10.5962/bhl.title.115594
Laughinghouse H. D. IV., Müller K. M., Adey W. H., Lara Y., Young R., Johnson G. (2015). Evolution of the northern rockweed, Fucus distichus, in a regime of glacial cycling: Implications for benthic algal phylogenetics. PloS One 10, e0143795. doi: 10.1371/journal.pone.0143795
le Corguillé G., Pearson G., Valente M., Viegas C., Gschloessl B., Corre E., et al. (2009). Plastid genomes of two brown algae, Ectocarpus siliculosus and Fucus vesiculosus: further insights on the evolution of red-algal derived plastids. BMC Evolutionary Biol. 9 (1), 253. doi: 10.1186/1471-2148-9-253
Leclerc M. C., Barriel V., Lecointre G., de Reviers B.. (1998). Low divergence in rDNA ITS sequences among five species of Fucus (Phaeophyceae) suggests a very recent radiation. J. Mol. Evol. 46 (1), 115–120. doi: 10.1007/PL00006278
Lein T. E. (1984). Distribution, reproduction, and ecology of Fucus ceranoides L. (Phaeophyceae) in Norway. Sarsia 69, 75–81. doi: 10.1080/00364827.1984.10420592
Linneí C.V., Salvius L. (1753). “Caroli linnaei. species plantarum :exhibentes plantas rite cognitas, ad genera relatas, cum differentiis specificis, nominibus trivialibus, synonymis selectis, locis natalibus, secundum systema sexuale digestas,” in Holmiae: Impensis laurentii salvii (Stockholm: Laurentius Salvius). doi: 10.5962/bhl.title.669
Lüning K. (1990). Seaweeds: their environment, biogeography and ecophysiology (New York: John Wiley).
Mačič V. (2006). Distribution of seaweed Fucus virsoides J.Agardh in boka kotorska bay (South Adriatic Sea). Annales Ser. Hist. Naturalis. 16, 1.
Maier I., Müller D. G. (1986). Sexual pheromones in algae. Biol. Bulletin. 170, 145–175. doi: 10.2307/1541801
Malm T., Kautsky L. (2003). Differences in life-history characteristics are consistent with the vertical distribution pattern of Fucus serratus and Fucus vesiculosus (Fucales, Phaeophyceae) in the central Baltic Sea. J. Phycology. 39, 880–887. doi: 10.1046/j.1529-8817.2003.02115.x
Malm T., Kautsky L., Engkvist R. (2001). Reproduction, recruitment and geographical distribution of Fucus serratus L. in the Baltic Sea. Botanica Marina. 44, 101–108. doi: 10.1515/BOT.2001.014
Manton I., Clarke B. (1950). Electron microscope observations on the spermatozoid of Fucus. Nature 166, 973–974. doi: 10.1038/166973a0
Martins M. J., Mota C. F., Pearson G. A. (2013). Sex-biased gene expression in the brown algal Fucus vesiculosus. BMC Genomics 14, 294. doi: 10.1186/1471-2164-14-294
Mathieson A. C., Dawes C. J. (2001). A muscoides-like Fucus from a Maine salt marsh: its origin, ecology, and taxonomic implications. Rhodora 103, 172–201. doi: 10.2307/23313470
Mathieson A. C., Dawes C. J., Anderson M. J., Hehre E. J. (2001). Seaweeds of the Brave Boat Harbor salt marsh and adjacent open coast of southern Maine. Rhodora 103, 1–46. doi: 10.2307/23313431
Mathieson A. C., Hehre E. J. (1982). The composition, seasonal occurrence and reproductive periodicity of the Phaeophyceae (brown algae) in New Hampshire. Rhodora 84, 411–437. doi: 10.2307/23310991
McCook L. I., Chapman A. R. O. (1993). Community succession following massive ice-scour on a rocky intertidal shore: recruitment, competition and predation during early, primary succession. Mar. Biol. 115, 565–575. doi: 10.1007/BF00349363
McLachan J., Chen L. C.-M., Edelstein T. (1971). The culture of four species of Fucus under laboratory conditions. Can. J. Botany. 49, 1463–1469. doi: 10.1139/b71-206
Meier H. E., Andersson H. C., Arheimer B., Blenckner T. (2012). Comparing reconstructed past variations and future projections of the Baltic Sea ecosystem-first results from multi-model ensemble simulations. Environ. Res. Lett. 7, 034005. doi: 10.1088/1748-9326/7/3/034005
Moalic Y., Arnauld-Haond S., Perrin C., Pearson G. A., Serrão E. A. (2011). Travelling in time with networks: revealing present day hybridization versus ancestral polymorphism between two species of brown algae, Fucus vesiculosus and F. spiralis. BMC Evolutionary Biol. 11, 33. doi: 10.1186/1471-2148-11-33
Monteiro C. A., Paulino C., Jacinto R., Serrão E. A., Pearson G. A. (2016). Temporal windows of reproductive opportunity reinforce species barriers in a marine broadcast spawning assemblage. Sci. Rep. 6, 29198. doi: 10.1038/srep29198
Monteiro C. A., Serrão E. A., Pearson G. A. (2012). Prezygotic barriers to hybridization in marine broadcast spawners: reproductive timing and mating system variation. PloS One 7 (4), e35978. doi: 10.1371/journal.pone.0035978
Motomura T. (1994). Electron and immunofluorescence microscopy on the fertilization of Fucus distichus (Fucales, Phaeophyceae). Protoplasma 178, 97–110. doi: 10.1007/BF01545960
Muhlin J. F., Engel C. R., Stessel R., Weatherbee R. A., Brawley S. H. (2008). The influence of coastal topography, circulation patterns, and rafting in structuring populations of an intertidal alga. Mol. Ecology. 17, 1198–1210. doi: 10.1111/j.1365-294X.2007.03624.x
Müller D. G. (1991). Fertilization biology in Fucus (Phaeophyceae) - dioecism and moecism. IWF (Göttingen). doi: 10.3203/IWF/C-1747eng
Müller D. G., Gassmann G. (1985). Sexual reproduction and the role of sperm attractants in monoecious species of the brown algae order Fucales (Fucus, Hesperophycus, Pelvetia, and Pelvetiopsis). J. Plant Physiol. 118, 401–408. doi: 10.1016/S0176-1617(85)80200-5
Müller D. G., Jaenicke L. (1973). Fucoserratene, the female sex attractant of Fucus serratus L. (Phaeophyta). FEBS Letters. 30, 137–139. doi: 10.1016/0014-5793(73)80636-2
Müller D. G., Seferiadis K. (1977). Specificity of sexual chemotaxis in Fucus serratus and Fucus vesiculosus (Phaeophyceae). Z. für Pflanzenphysiologie. 84, 85–94. doi: 10.1016/S0044-328X(77)80126-8
Munda I. M., Kremer B. P. (1997). Morphological variation and population structure of Fucus spp. (Phaeophyta) from helgoland. Nova Hedwigia. 64, 67–86. doi: 10.1127/nova.hedwigia/64/1997/67
Nagasato C., Terauchi M., Tanaka A., Motomura T. (2015). Development and function of plasmodesmata in zygotes of Fucus distichus. Botanica Marina 58, 229–238. doi: 10.1515/bot-2014-0082
Neiva J., Pearson G. A., Valero M., Serrão E. A. (2012). Fine-scale genetic breaks driven by historical range dynamics and ongoing density-barrier effects in the estuarine seaweed Fucus ceranoides l. BMC Evolutionary Biol. 12, 78. doi: 10.1186/1471-2148-12-78
Neto A. I. (2000). Observations on the biology and ecology of selected macroalgae from the littoral of são Miguel (Azores). Botanica Marina 43 (5), 483–498. doi: 10.1515/BOT.2000.049
Nicastro K. R., Zardi G. I., Teixeira S., Neiva J., Serrão E. R., Pearson G. A. (2013). Shift happens: trailing edge contraction associated with recent warming trends threatens a distinct genetic lineage in the marine macroalga Fucus vesiculosus. BMC Biol. 11, 6. doi: 10.1186/1741-7007-11-6
Niemeck R. A., Mathieson A. C. (1976). An ecological study of Fucus spiralis l. J. Exp. Mar. Biol. Ecol. 24, 33–48. doi: 10.1016/0022-0981(76)90041-1
Nienburg W. (1925). Einne eigenatige lebensgemeinschaft zwischen Fucus und Mytilus. Berichte der Deutschen Botanischen Gesellschaft. 43, 292–298.
Nienburg W. (1927). Zur Ökologie dt flora des Wateeenmeeres. 1. der Königshafen bei list auf Sylt. Helgoländer wissenschaftliche Meeresuntersuchungen 20, 146–196.
Nienhuis P. H. (1970). The benthic algal communities of flats and salt marshes in the grevelingen, a sea arm in the south-western Netherlands. Netherlands J. Sea Res. 5, 20–49. doi: 10.1016/0077-7579(70)90003-7
Oudot-Le-Secq M.-P., Goër S. L., Stam W. T., Olsen J. L. (2006). Complete mitochondrial genomes of the three brown algae (Heterokonta: Phaeophyceae) Dictyota dichotoma, Fucus vesiculosus and Desmarestia viridis. Curr. Genet. 49 (1), 47–58. doi: 10.1007/s00294-005-0031-4
Parrot D., Blumel M., Utermann C., Chianese G., Krause S., Kovalev A., et al. (2019). Mapping the surface microbiome and metabolome of brown seaweed Fucus vesiculosus by amplicon sequencing integrated metabolomics and imaging techniques. Sci. Rep. 9, 1061. doi: 10.1038/s41598-018-37914-8
Pearson G., Brawley S. (1996). Reproductive ecology of Fucus distichus (Phaeophyceae): An intertidal alga with successful external fertilization. Mar. Ecol. Prog. Series. 143, 211–223. doi: 10.3354/meps143211
Pearson G. A., Serrão E. A., Brawley S. (1998). Control of gamete release in fucoid algae: sensing hydrodynamic conditions via carbon acquisition. Ecology 79, 1725–1739. doi: 10.1890/0012-9658(1998)079[1725:COGRIF]2.0.CO;2
Pearson G., Lago-Leston A., Valente M., Serrão E. (2006). Simple and rapid RNA extraction from freeze-dried tissue of brown algae and seagrasses. Eur. J. Phycology. 41, 97–104. doi: 10.1080/09670260500505011
Pearson G., Serrão E. A., Brawley S. H. (1998). Control of gamete release in fucoid algae: sensing hydrodynamic conditions via carbon acquisition. Ecology 79, 1725–1739. doi: 10.1890/0012-9658(1998)079[1725:COGRIF]2.0.CO;2
Pereira L., Cotas J. (2019). “Historical uses of seaweed as agricultural fertilizer in the European Atlantic area. chap. 1., 1-23,” in Seaweeds as plant fertilizer, agricultural biostimulants and animal fodder. Eds. Pereira L., Bahcevandziev K., Joshi N. H. (CRC Press, Taylor & Francis Group).
Pereyra R., Bergström L., Kautsky L., Johannesson K. (2009). Rapid speciation in a Newly opened postglacial marine environment, the Baltic Sea. BMC Evolutionary Biol. 9, 1–9. doi: 10.1186/1471-2148-9-70
Perrin C., Daguin C., Van De Vliet M., Engel C., Pearson G., Serrão E. (2007). Implications of mating system for genetic diversity of sister algal species Fucus spiralis and Fucus vesiculosus (Heterokontophyta, Phaeophyceae). Eur. J. Phycology. 42, 219–230. doi: 10.1080/09670260701336554
Perry F., Hill J. M. (2015). “Fucus distichus and Fucus spiralis F. nana on extremely exposed upper shore rock,” in Marine life information network: Biology and sensitivity key information reviews. Eds. Tyler-Walters H., Hiscock K. (Plymouth: Marine Biological Association of the United Kingdom). Available at: https://www.marlin.ac.uk/habitat/detail/234/Fucus_distichus_and_Fucus_spiralis_f_nana_on_extremely_exposed_upper_shore_rock.
Powell H. T. (1963). Speciation in the genus Fucus L. and related genera. In Harding J. P., Tebble N. Eds Speciation in the Sea. (London: Systematics Association Publication) 5, 63–77.
Preston R., Blomster J., Schagerström E., Seppä P. (2022). Clonality, polyploidy and spatial population structure in Baltic Sea Fucus vesiculosus. Ecol. Evolution., 12e9336. doi: 10.1002/ece3.9336
Réaumur R.A. (1711). “Description des fleurs et des graines de divers Fucus et quelques autres obser-vations physiques sur ces mêmes plantes,” in Mémoires de mathématique et de physique de l’Académie royale des sciences (Académie royale des sciences).
Rice E. L., Chapman A. R. O. (1985). A numerical taxonomic study of Fucus distichus (Phaeophyta). J. Mar. Biol. Association UK. 65, 433–459. doi: 10.1017/S0025315400050530
Rice E. L., Kenchington T. J., Chapman A. R. O. (1985). Intraspecific geographic-morphological variation patterns in Fucus distichus and F. evanescens. Mar. Biol. 88, 207–215. doi: 10.1007/BF00397168
Robbins W. J. (1916). Notes on the physiology of Fucus spermatozooids. Biol. Bulletin. 30, 125–130. doi: 10.2307/1536278
Robertson B. L. (1987). Reproductive ecology and canopy structure of Fucus spiralis l. Botanica Marina 30, 475–482. doi: 10.1515/botm.1987.30.6.475
Roe M. (1916). The development of the conceptacle in Fucus. Botanical Gazette. 61, 231–251. doi: 10.1086/331762
Rousseau F., Leclerc M. -C., de Reviers B. (1997). Molecular phylogeny of European Fucales (Phaeophyceae) based on partial large-subunit rDNA sequence comparisons. Phycologia. 36 (6), 438–446. doi: 10.2216/i0031-8884-36-6-438.1
Rousseau F., de Reviers B. (1999). Phylogenetic relationships within the Fucales (Phaeophyceae) based on combined partial SSU + LSU rDNA sequence data. European Journal of Phycology 34 (1), 53–64. doi: 10.1080/09670269910001736082
Rothäusler E., Corell H., Jormalainen V. (2015). Abundance and dispersal trajectories of floating Fucus vesiculosus in the northern Baltic Sea. Limnology Oceanography. 60, 2173–2184. doi: 10.1002/lno.10195
Rothäusler E., Rugiu L., Jormalainen V. (2018). Forecast climate change conditions sustain growth and physiology but hamper reproduction in range-margin populations of a foundation rockweed species. Mar. Environ. Res. 141, 205–213. doi: 10.1016/j.marenvres.2018.09.014
Rothäusler E., Uebermuth C., Haavisto F., Jormalainen V. (2019). Living on the edge: Gamete release and subsequent fertilisation in Fucus vesiculosus (Phaeophyceae) are weakened by climate change–forced hyposaline conditions. Phycologia 58, 111–114. doi: 10.1080/00318884.2018.1524246
Ruggeri A. (2015). North ronaldsay sheep eat seaweed and little else (BBC). Available at: https://web.archive.org/web/20170213201859/http://www.bbc.co.uk/earth/story/20150924-north-ronaldsay-sheep-eat-seaweed-and-little-else.
Rugiu L., Manninen I., Rothäusler E., Jormalainen V. (2018). Tolerance to climate change of the clonally reproducing endemic Baltic seaweed, Fucus radicans: is phenotypic plasticity enough? J. Phycolology. 54, 888–898. doi: 10.1111/jpy.12796
Ruiz G. M., Fofonoff P. W., Carlton J. T., Wonham M. J., Hines A. H. (2000). Invasion of coastal marine communities in north America: apparent patterns, processes, and biases. Annu. Rev. Ecol. Systematics. 31, 481–531. doi: 10.1146/annurev.ecolsys.31.1.481
Saeed A. F., Wang R., Wang S. (2016). Microsatellites in pursuit of microbial genome evolution. Front. Microbiol. 6. doi: 10.3389/fmicb.2015.01462
Sánchez de Pedro R., Nieves Fernández A., Jesús García-Sánchez M., Flores-Moya A., Bañares-España E. (2019). “When the microclimate does matter: differences in the demographic, morphometric and reproductive variables of Fucus guiryi from two nearby populations,” in Conference paper, II International Conference of Young Marine Researchers. Available at: http://jisdelmar.uma.es/ficheros-contribuciones/1562710791/1562710791.pdf
Sauvageau C. (1909). Sur l’hybride des Fucus vesiculosus et Fucus serratus. Comptes rendus des seances de la societe de biologie et de ses filiales. Paris 67, 832–833.
Schagerström E. (2013). Fucus radicans: Reproduction, adaptation and distribution patterns (Stockholm: Department of Ecology, Environment and Plant Sciences, Stockholm University), ISSN 1651-9248; 2013/2, p. 22.
Schagerström E., Kautsky L. (2016). Despite marine traits, the endemic Fucus radicans (Phaeophyceae) is restricted to the brackish Baltic Sea. Eur. J. Phycology 51 (4), 378–386. doi: 10.1080/09670262.2016.1183234
Schagerström E., Salo T. (2019). Interactive effects of temperature and light on reattachment success in the brown alga Fucus radicans. Botanica Marina. 62, 43–50. doi: 10.1515/bot-2018-0011
Schiel D. R. (1988). Algal interactions on shallow subtidal reefs in northern New Zealand: A review. N Z J. Mar. Freshw. Res. 22, 481–489. doi: 10.1080/00288330.1988.9516317
Schoen D. J. (2005). Deleterious mutation in related species of the plant genus Amsinckia with contrasting mating systems. Evolution 59, 2370–2377. doi: 10.1111/j.0014-3820.2005.tb00947.x
Schueller G. H., Peters A. F. (1994). Arrival of Fucus evanescens in the Kiel bight (Western Baltic). Botanica Marina 37, 471–477. doi: 10.1515/botm.1994.37.5.471
Scott G. W., Hardy F. G. (1994). Observations of the occurrence of hybrids between two sympatric species of fucoid algae. Cryptogamie Algologie. 15, 297–305.
Serrão E. A., Alice L. A., Brawley S. H. (1999a). Evolution of the Fucaceae (Phaeophyceae) inferred from nrDNA-ITS. J. Phycology. 35, 382–394. doi: 10.1046/j.1529-8817.1999.3520382.x
Serrão E. A., Brawley S. H., Hedman J., Kautsky L., Samuelsson G. (1999b). Reproductive success in Fucus vesiculosus (Phaeophyceae) in the Baltic Sea. J. Phycology. 35, 254–269. doi: 10.1046/j.1529-8817.1999.3520254.x
Serrão E. A., Kautsky L., Brawley S. H. (1996). Distributional success of the marine seaweed Fucus vesiculosus L. in the brackish Baltic Sea correlates with osmotic capabilities of Baltic gametes. Oecologia 107, 1–12. doi: 10.1007/BF00582229
Serrão E. A., Kautsky L., Lifvergren T., Brawley S. (1997). Gamete dispersal and pre-recruitment mortality in Baltic Fucus vesiculosus. Phycologia Suppl. 36, 101–102. doi: 10.2216/i0031-8884-36-4S-1.1
Sideman E. J., Mathieson A. C. (1983a). The growth, reproductive phenology and longevity of non-tide pool Fucus distichus (L.) Powell in New England. J. Exp. Mar. Biol. Ecology. 68, 111–117. doi: 10.1016/0022-0981(83)90154-5
Sideman E. J., Mathieson A. C. (1983b). Ecological and genealogical distinction of a high intertidal, dwarf form of Fucus distichus (L.) Powell. J. Exp. Mar. Biol. Ecology. 72, 171–188. doi: 10.1016/0022-0981(83)90142-9
Sideman E. J., Mathieson A. C. (1985). Morphological variation within and between natural populations of non-tide pool Fucus distichus (Phaeophyta) in New England. J. Phycology. 21, 250–257. doi: 10.1111/j.0022-3646.1985.00250.x
Silberfeld T., Rousseau F., de Reviers B. (2014). An updated classification of brown algae (Ochrophyta, Phaeophyceae). Cryptogamie Algologie 35, 117–156. doi: 10.7872/crya.v35.iss2.2014.117
Sjøtun K., Heesch S., Lluch J. R., Martin R. M., Garreta A. M., Brysting A. K., et al. (2017). Unravelling the complexity of salt marsh ‘Fucus cottonii’ forms (Phaepophyceae, Fucales). Eur. J. Phycology. 52, 360–370. doi: 10.1080/09670262.2017.1309688
Stackhouse J. (1801). Nereis britannica: containing all the species of fuci, natives of the British coasts: with a description in English and Latin, and plates coloured from nature (Creative Media Partners, LLC).
Stebbins G. L. (1950). Variation and evolution in plants (London, UK: Oxford University Press). doi: 10.7312/steb94536
Stebbins G. L. (1974). Flowering plants: evolution above the species level (Cambridge, MA: Harvard University Press). doi: 10.4159/harvard.9780674864856
Steele R. L. (1977). “Effects of certain petroleum products on reproduction and growth of zygotes and juvenile stages of the alga Fucus edentatus de la pyl. (Phaeophyceae: Fucales),” in Fate and effects of petroleum hydrocarbons in marine ecosystems and organisms. Ed. Wolfe D. A. (Pergamon Press), 138–142. doi: 10.1016/B978-0-08-021613-3.50017-6
Steen H., Rueness J. (2004). Comparison of survival and growth in germlings of six fucoid species (Fucales, Phaeophyceae) at two different temperature and nutrient levels. Sarsia 89, 175–183. doi: 10.1080/00364820410005818
Subrahmanyan R. (1960). Ecological studies on the fucales ii. Fucus spiralis L.*. J. Indian Bot. Soc 39 (4), 614–630.
Sundene O. (1953). The algal vegetation of Oslofjord Vol. 2 Oslo: Det Norske videnskaps-akademi i Oslo. Skrifter-Nat. Naturv Kl 2, 1–244.
Tatarenkov A., Bergström L., Jönsson R. B., Serrão E. A., Kautsky L., Johannesson K. (2005). Intriguing asexual life in marginal populations of the brown seaweed Fucus vesiculosus. Mol. Ecol. 14, 647–651. doi: 10.1111/j.1365-294X.2005.02425.x
Tatarenkov A., Jönsson R. B., Kautsky L., Johannesson K. (2007). Genetic structure in populations of Fucus vesiculosus (Phaeophyceae) over spatial scales from 10 m to 800 km. J. Phycology. 43, 675–685. doi: 10.1111/j.1529-8817.2007.00369.x
Teixeira S., Pearson G. A., Candeias R., Maderira C., Valero M., Serrão E. A. (2016). Lack of fine-scale genetic structure and distant mating in natural populations of Fucus vesiculosus. Mar. Ecol. Prog. Series. 544, 131–142. doi: 10.3354/meps11607
Thiel M., Hinojosa I. A., Joschko T., Gutow. L. (2011). Spatial-temporal distribution of floating objects in the German bight (North Sea). J. Sea Res. 65, 368–379. doi: 10.1016/j.seares.2011.03.002
Thuret G. (1855). Recherches sur la fécundation des fucacées. Ann. Des. Sci. Naturelles Botanique. Série 4 2, 197–214.
Tromp R. M. (2015). An adjustable electron achromat for cathode lens microscopy. Ultramicroscopy 159, 497–502. doi: 10.1016/j.ultramic.2015.03.001
Tyrrell M. C., Dionne M., Eberhardt S. A. (2012). Salt marsh fucoid algae: overlooked ecosystem engineers of north temperate salt marshes. Estuaries Coasts. 35, 754–762. doi: 10.1007/s12237-011-9472-9
Tyrrell M. C., Thornber C. S., Burkhardt J. A., Congretel M. (2015). The influence of salt marsh fucoid algae (ecads) on sediment dynamics of northwest Atlantic marshes. Estuaries Coasts. 38, 1262–1273. doi: 10.1007/s12237-014-9919-x
Van Alstyne K. L. (1989). Adventitious branching as a herbivore-induced defense in the intertidal brown alga Fucus. Mar. Ecol. Prog. Ser. 56, 169–176.
Vernet P., Harper J. L. (1980). The cost of sex in seaweeds. Biol. J. Linn. Society. 13, 129–138. doi: 10.1111/j.1095-8312.1980.tb00076.x
Viana I. G., Bode A., Fernández C. (2015). Ecology of Fucus vesiculosus (Phaeophyceae) at its southern distributional limit: growth and production of early developmental stages. Eur. J. Phycology 50, 247–259. doi: 10.1080/09670262.2015.1013159
Wahl M., Jormalainen V., Eriksson B. E., Coyer J. A., Molis M., Schubert H., et al. (2011). “Stress ecology in Fucus: abiotic, biotic, and genetic interactions,” in Advances in marine biology, vol. 59 . Ed. Lesser M. (London: Academic Press, Elsevier), 39–105. doi: 10.1016/B978-0-12-385536-7.00002-9
Wahl M., Shahnaz L., Dobretsov S., Saha M., Symanowski F., David K., et al. (2010). Ecology of antifouling resistance in the bladder wrack Fucus vesiculosus: patterns of microfouling and antimicrobial protection. Mar. Ecol. Prog. Ser. 411, 33–48. doi: 10.3354/meps08644
Wallace A., Klein A. S., Mathieson A. C. (2004). Determining the affinities of salt marsh fucoids using microsatellite markers: evidence of hybridization and introgression between two species of Fucus (Phaeophyta) in a Maine estuary. J. Phycology. 40, 1013–1027. doi: 10.1111/j.1529-8817.2004.04085.x
Wells H. (1979). Self-fertilization: advantageous or deleterious? Evolution 33, 252–255. doi: 10.2307/2407381
Whitaker D. M. (1931). Some observations on the eggs of Fucus and upon their mutual influence in the determination of the developmental axis. Biol. Bulletin. 61, 294–308. doi: 10.2307/1536949
Whitaker S. G., Fong D. R., Neiva J., Serrão E. A., Anderson L. M., Raimondi P. T. (2017). Distribution and genetic structure of Fucus distichus Linnaeus 1953 (formerly F. gardneri) within central San Francisco bay. San Francisco Estuary Watershed Science. 15 (3), 1–15. doi: 10.15447/sfews.2017v15iss3art4
Wright S. I., Kalisz S., Slotte T. (2013). Evolutionary consequences of self-fertilization in plants. Proc. R. Soc. B. 280, 20120122. doi: 10.1098/rspb.2013.0133
Williams L. (1899). New Fucus hybrids. Ann. Botany. 13, 187–188. doi: 10.1093/oxfordjournals.aob.a088726
Williams G. A. (1996). “Seasonal variation in a low shore Fucus serratus (Fucales, phaeophyta) population and its epiphytic fauna,” in Lindstrom S. C., Chapman D. J. Eds Fifteenth international seaweed symposium (Springer Netherlands: Kluwer Academic Publishers), 191–197. doi: 10.1007/978-94-009-1659-3_26
Woodward T. (1791). XIII. The history and description of a New species of Fucus. Trans. Linn. Soc. London 1, 131–134. doi: 10.1111/j.1096-3642.1791.tb00393.x
Wohlenberg E. (1937). Die Wattenmeer-Lebensgemeinschaft im Königshafen von Sylt. Helgoländer wissenschaftliche Meeresuntersuchungen 1, 1–92. doi: 10.1007/BF02285337
Wright S. I., Kalisz S., Slotte T. (2013). Evolutionary consequences of self-fertilization in plants. Proc. R. Society. B. 280, 20130133. doi: 10.1098/rspb.2013.0133
Yates J. L., Peckol P. (1993). Effects of nutrient availability and herbivory on polyphenolics in the seaweed Fucus vesiculosus. Ecology 74 (6), 1757–1766. doi: 10.2307/1939934
Zardi G. I., Nicastro K. R., Cánovas F., Costa J. F., Serrão E. A., Pearson G. (2011). Adaptive traits are maintained on steep selective gradients despite gene flow and hybridization in the intertidal zone. PloS One 6, e19402. doi: 10.1371/journal.pone.0019402
Zardi G. I., Nicastro K. R., Costa J. F., Serrão E. A., Pearson G. (2013). Broad scale agreement between intertidal habitats and adaptive traits on a basis of contrasting population genetic structure. Estuarine Coast. Shelf Science. 131, 140–148. doi: 10.1016/j.ecss.2013.08.016
Zardi G. I., Nicastro K. R., Serrão E. A., Jacinto R., Monteiro C. A., Pearson G. A. (2015). Closer to the rear edge: ecology and genetic diversity down the core-edge gradient of a marine macroalga. Ecosphere 6 (2), art23. doi: 10.1890/ES14-00460.1
Keywords: Fucus, reproduction, review, diplontic life cycle, selfing, hybridization, fertilization, ecads
Citation: Hatchett WJ, Coyer JA, Sjøtun K, Jueterbock A and Hoarau G (2022) A review of reproduction in the seaweed genus Fucus (Ochrophyta, Fucales): Background for renewed consideration as a model organism. Front. Mar. Sci. 9:1051838. doi: 10.3389/fmars.2022.1051838
Received: 23 September 2022; Accepted: 24 October 2022;
Published: 16 November 2022.
Edited by:
Georgina Valentine Wood, University of Western Australia, AustraliaReviewed by:
Nahlah Abdullah Alsuwaiyan, University of Western Australia, AustraliaKai Xu, Jimei University, China
Copyright © 2022 Hatchett, Coyer, Sjøtun, Jueterbock and Hoarau. This is an open-access article distributed under the terms of the Creative Commons Attribution License (CC BY). The use, distribution or reproduction in other forums is permitted, provided the original author(s) and the copyright owner(s) are credited and that the original publication in this journal is cited, in accordance with accepted academic practice. No use, distribution or reproduction is permitted which does not comply with these terms.
*Correspondence: William J. Hatchett, d2lsbGlhbS5oYXRjaGV0dEBub3JkLm5v