- 1Center for Biomedical Optics and Photonics (CBOP) & College of Physics and Optoelectronic Engineering, Key Lab of Optoelectronics Devices and systems of Ministry of Education/Guangdong Province, Shenzhen University, Shenzhen, China
- 2CAS Key Laboratory of Coastal Environmental Processes and Ecological Remediation, Yantai Institute of Coastal Zone Research (YIC), Chinese Academy of Sciences (CAS), Shandong Key Laboratory of Coastal Environmental Processes, YICCAS, Yantai, China
Introduction: Cadmium (Cd) is one of the most dominant heavy metals in the Bohai Sea. Our previous study proved that Cd could induce gill mitochondrial toxicity in marine animals. Herein, we aimed to elucidate the toxicity mechanism of Cd on liver mitochondria, as liver is the main metabolic and detoxification organ and generally rich in mitochondria.
Methods: The mitochondrial responses induced by Cd (5 and 50 μg/L) were characterized by observing mitochondrial morphology, measuring mitochondrial membrane potential (MMP), and proteomic and metabolomic analysis in juvenile olive flounder Paralichthys olivaceus livers.
Results: After water-bonre exposure for 14 days, two Cd treatments decreased MMPs significantly and caused ultrastructural-damaged mitochondria in flounder livers. NMR-based metabolomics revealed that Cd exposure mainly altered the abundances of metabolites (ATP, AMP, phosphocholine, lactate and succinate) related to energy metabolism in flounder livers. iTRAQ-based mitochondrial proteomics indicated that 27 differentially expressed proteins (DEPs) were screened out from liver mitochondria after Cd treatments. These proteins were mainly associated with energy metabolism (oxidative phosphorylation (OXPHOS) and tricarboxylic acid (TCA) cycle) and apoptosis.
Discussion: These results indicated that Cd disrupted mitochondrial morphology, energy homeostasis and apoptosis in liver mitochondria in flounder P. olivaceus. This work revealed a comprehensive view on Cd-induced mitochondrial responses in the liver tissues of flounder using an integrated proteomic and metabolomic approach.
1. Introduction
Cadmium (Cd) is a typical metal contaminant in the Bohai Sea owing to rapidly developed industry and unavoidable discharges (Li et al., 2018; Lu and Wang, 2018). As a nearly non-essential metal, Cd could be readily bio-accumulated in aquatic organisms and poses a great threat to marine organisms and the human body by bioaccumulation and biomagnification (Lane and Morel, 2000; Liu et al., 2019). Cd even at low levels could influence various physiological processes, such as growth, reproduction, osmoregulation, immunity and apoptosis in marine animals (Bertin and Averbeck, 2006; Lu et al., 2019). Furthermore, numerous researches have shown that Cd exhibits toxic effects on energy metabolism, especially on mitochondrial bioenergetics (Sokolova et al., 2005a; Charan-Dixon et al., 2017). Mitochondria are the main sites producing energy, and energy metabolism is believed to be the basis for many key biological processes. Therefore, it is necessary to pay more attention to the Cd-induced mitochondrial toxicity in marine organisms.
In general, mitochondria are the major energy source in eukaryotes and related to various biological processes, such as material and energy metabolism, calcium homeostasis, endocrine regulation, and apoptosis. Mitochondria are susceptible to environmental toxicants and probably act as one of the main targets (Shaughnessy et al., 2010; Meyer et al., 2013). Numerous researches have proved that Cd showed toxicological effects on mitochondria, such as damaged ultrastructures, inhibited ATP production, and decreased mitochondrial membrane potential (MMP) (Kurochkin et al., 2011; Yang et al., 2015). Moreover, our previous studies demonstrated that mitochondrion was important target of Cd toxicity to gill tissues of marine animals, and Cd-induced responses of mitochondrial endpoints were related to energy disturbance, apoptosis and stress resistance in gill tissues of clam Ruditapes philippinarum (Ji et al., 2019) and flounder Paralichthys olivaceus (Lu et al., 2020). However, to our knowledge, there is a lack of global evaluation of Cd-induced mitochondrial responses in liver tissues of marine animals, even though liver is the main metabolic organ and generally rich in mitochondria.
As the rapid development of omics-based techniques, proteomics, metabolomics, genomics and transcriptomics have been widely introduced in biological and ecological studies (Yu et al., 2016). Among them, mitochondrial proteomics is an extensive research of mitochondrial proteins which encoded by mitochondrial and nuclear genomes in organisms. It could shed more light on mitochondrial function and has been extensively applied to toxicological studies and mitochondrial diseases (Leahy et al., 2018; Li et al., 2019). Metabolomics generally targets on a full set of low molecular weight (less than 1000 Da) metabolites, which are final products of organs, tissues, or even entire organisms (Wu and Wang, 2015). Mitochondrial proteomics and metabolomics could directly elucidate the perturbations of metabolic pathways which associated with mitochondria, so the integration of these two omics techniques may provide new insights into stressor-induced mitochondrial responses (Ji et al., 2019).
The olive flounder P. olivaceus is an economically important species in the Bohai Sea, and also a common experimental animal widely used in marine ecotoxicology (Cui et al., 2020). Cd is a known heavy metal causing acute toxicity and to flounders (Zhu et al., 2006), and it also showed mitochondrial toxicity in flounder gills according to our previous research (Lu et al., 2020). Unlike gills, the liver does not directly contact the external aquatic environment, and it is the main metabolic and detoxification organ of fish. Therefore, the global mitochondrial response in flounder livers should be considered to better understand the Cd-induced mitochondrial toxicity. In this study, iTRAQ-based mitochondrial proteomics and NMR-based metabolomics were combined to characterize the mitochondrial toxicity and mechanism of Cd on flounder livers after two environment-related sublethal concentrations (5 and 50 μg/L) (Gao et al., 2014) of Cd exposure for 14 days.
2. Material and method
2.1. Animals and Cd exposure
One hundred and eighty juvenile flounders (body weight: ~20.8 g, total body length: ~13.5 cm) were acquired from a farm (Yantai, China) and raised as described in our previous study (Lu et al., 2020). After acclimatization for 7 days, flounders were randomized into 3 groups (two Cd treatments and one control). The flounders in control group were kept in the normal seawater. Two environment-related sublethal concentrations (5 and 50 μg/L) of Cd (in CdCl2, 99.99%, Sigma-Aldrich) were used for water-borne exposure. Each group contained two replicates in two individual tanks with thirty flounders in each tank. During the acclimation and exposure periods, all flounders were kept in aerated filtered seawater (32.5 psu, 20 ± 0.5°C) under a photoperiod of L12: D12, and fed with pellet feed at a ratio of 2% of tissue dry weight every day. After feeding for 1 h, seawater was replaced with 50% fresh seawater daily. No mortality was found during whole experimental period. After exposure for 14 days, all the flounders were anesthetized with 0.15% ethyl 3-aminobenzoate methanesulfonate (98%, Sigma-Aldrich) and immediately dissected for liver tissues. These fresh liver tissues from each group were separated into four parts for mitochondrial observation, mitochondrial isolation, metabolite extraction, and Cd determination. Liver samples for metabolite extraction and Cd determination were quick-frozen and stored at -80 °C.
2.2. Cd determination
For each group, about 100 mg flounder liver samples (n = 5) were dried, digested and measured same as our previous research (Lu et al., 2020). Cd contents in flounder livers were defined as μg/g dry weight. The recovery of Cd was restricted within 94.8-101.4%.
2.3. Isolation of liver mitochondria
Isolation of liver mitochondria was operated according to our previous study with minor modifications (Lu et al., 2020). In brief, to exclude the cytosolic proteins, fresh livers were homogenized in pre-cooled lysis buffer and then centrifuged. The pellet was disrupted completely with disruption buffer. Then the obtained lysate (Fraction 1) was centrifuged, and the pellet (Fraction 2) containing unbroken cells, nuclei and cell debris was removed. The supernatant was then centrifuged, and the crude mitochondria pellet (Fraction 3) was reserved and re-suspended with mitochondria storage buffer for subsequent MMP measurement. A density gradient centrifugation was carried out on the basis of crude mitochondria to obtain high-purity mitochondria which were suitable for further mitochondrial proteomic analysis. For different fractions (Fraction 1-3), the activities of cytochrome c oxidase (complex IV) were measured to evaluate the mitochondrial purity.
2.4. Observation of mitochondrial morphology
To assess the Cd-induced morphological changes of liver mitochondria, ultrastructures were observed using transmission electron microscope (TEM, JEM 1200, Japan). Two fresh liver samples (~ 1 mm3) for each group were used to prepare ultraslices (70 nm), and three microscopic fields for each sample were observed with TEM. Detailed operations were available in our previous research (Lu et al., 2020). Mitochondrial area index and mitochondrial density were analyzed using ImageJ software. The mitochondrial area index represents total mitochondrial area in one microscopic field, mitochondrial density refers to mitochondria number in one microscopic field.
2.5. Measurement of MMP
MMP is essential for mitochondrial function maintenance and ATP production (Zorova et al., 2018). In this study, MMP alterations in liver mitochondria (n = 4) were detected as described previously (Lu et al., 2020). The mitochondrial potential-dependent fluorescent probe 5,5’,6,6’-tetrachloro-1,1’,3,3’-tetraethylbenzimidazolylcarbocyanine iodide (JC-1) (Beyotime, China) was used to measure MMP. In brief, crude liver mitochondria (0.1 mL) were fully mixed with JC-1 working solution (0.9 mL). Then the mixtures were immediately detected at 488 nm excitation on Accuri C6 Plus flow cytometer (BD, USA). JC-1 aggregates and monomers were measured respectively in the PE channel (565-605 nm) and FITC channel (515-545 nm). The visual histograms of PE versus FITC were plotted, and MMP of stained mitochondria was indicated by the ratio of aggregates (P1) to monomers (P2).
2.6. NMR-based metabolomic analysis
Polar metabolites in flounder livers were extracted with a modified method (Wu and Wang, 2015). In brief, metabolite extracts of liver tissues (about 100 mg, n = 10) were homogenized, extracted and determined on a spectrometer (AV 500 NMR, Bruker, Germany). NMR spectrum was analyzed using TopSpin 2.1 (Bruker, Germany), and ProMetab 7.0 in MATLAB (The MathsWorks, Natick, USA) (Viant et al., 2003). More detailed information was available in the Supporting Information.
2.7. iTRAQ-based mitochondrial proteomics
iTRAQ-based mitochondrial proteomics was applied to characterize the comprehensive mitochondrial responses in flounder livers exposed to Cd (Lu et al., 2020). The high-purity mitochondria of flounder livers were dissolved and digested. Then eight samples (3 parallels for each Cd treatment and 2 parallels for control) were labeled with the iTRAQ 8-plex Reagent Kit (SCIEX, USA). The labeled peptides were purified and fractionated, and the collected fractions were then analyzed on mass spectrometer combined with HPLC system (EASY-nLC 1200, Thermo, USA). The identification and relative quantification of proteins were performed on Proteome Discoverer (Thermo, USA) with Mascot (MatrixScience, UK) by searching P. olivaceus protein database downloaded from NCBI. Protein ratio with false discovery rate ≤ 0.01 and P < 0.05 was assigned as positive protein. Proteins with P < 0.05 and foldchange > 1.2 or < 0.83 were assigned as differentially expressed proteins (DEPs). In this study, DEPs function was annotated and analyzed based on the GO and KEGG databases. More information was described in the Supporting Information.
2.8. Statistical analysis
These parameters, such as mitochondrial area indexes, mitochondrial densities, MMP alterations, and Cd contents in liver samples were expressed as mean ± standard deviation (SD.), and data between the control and Cd treatments tested by one-way analysis of variance (ANOVA) with Student’s t-test was performed on SPSS (version 20, USA). P values < 0.05 represented statistical significance.
3. Result
3.1. Cd accumulations
After exposure for 14 days, Cd contents in flounder livers were measured in control and Cd treatments. With the increased concentrations for exposure, Cd contents in liver samples were significantly (P < 0.01) increased (Figure 1). Average contents in 5 and 50 μg/L Cd treatments were 3.02 and 4.32 μg/g dry weight respectively, which were approximately twice and triple as much as that in control group (1.50 ± 0.39 μg/g dry weight).
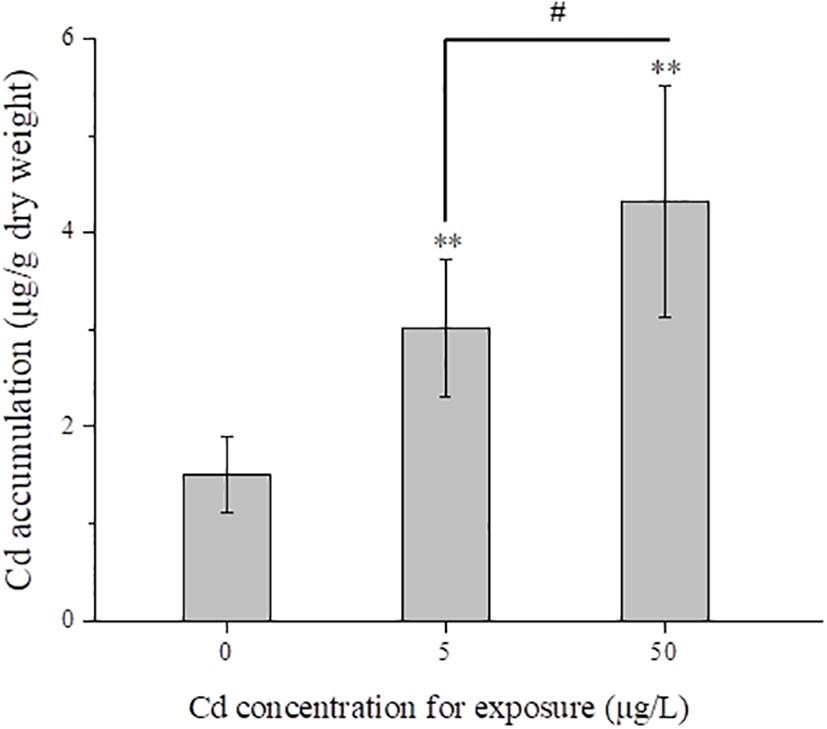
Figure 1 Mean total Cd concentrations (μg/g dry weight) in liver tissues (n = 5) from control and Cd treatments after exposure for 14 days. # represents 7P < 0.05, and ** represents P < 0.01.
3.2. Purity of isolated mitochondria
As shown in Figure S1, Fraction 1 and Fraction 3 showed higher activities of complex IV than Fraction 2, showing that we obtained significantly enriched liver mitochondria. High-purity mitochondria increased the reliability of subsequent measurements (MMP detection and proteomic analysis).
3.3. Mitochondrial morphology changes
Flounder livers were rich in mitochondria which were distributed along the lipid droplets (Figure 2). The normal mitochondria with homogeneous shape and size, integrated membrane structure and densely arranged cristae were observed in liver tissues from the control group (Figure 2A). However, Cd treatments induced ultrastructural damages in liver mitochondria, such as irregular shedding of mitochondrial inner membrane (MIM) or mitochondrial outer membrane (MOM), loosely arranged or fractured cristae (Figure 2B, C). In addition, both mitochondrial area index and mitochondrial density were increased after Cd treatments, but there was only a significant difference (P < 0.05) between 50 μg/L Cd-treated group and control group (Figure 2D).
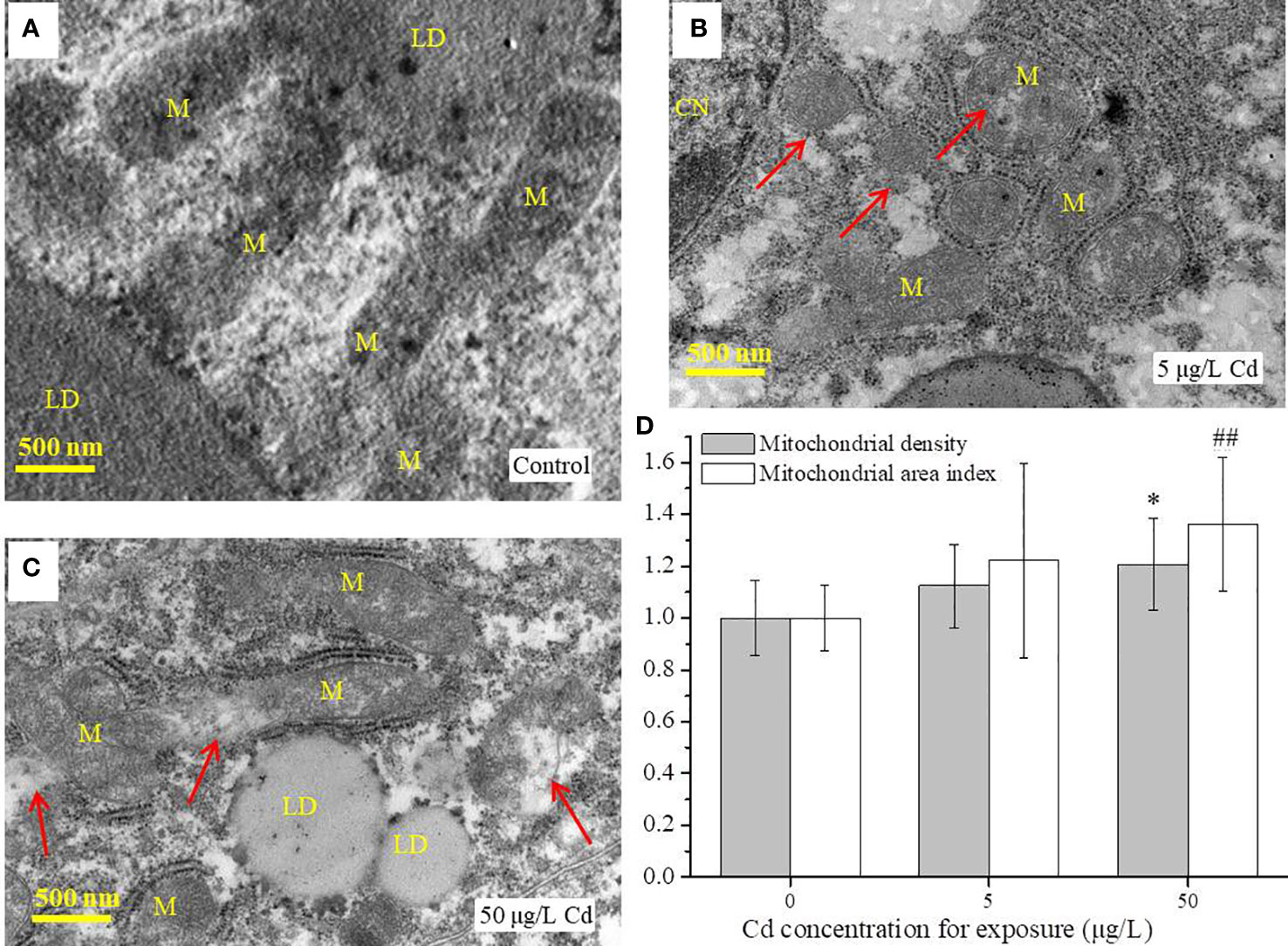
Figure 2 TEM analysis of liver tissues (n = 3) from control and Cd treatments after exposure for 14 days. (A–C) images of mitochondria in flounder livers; (D) the mitochondrial area index and mitochondrial density of liver samples (n = 3 × 3) from each group. Scale = 500 nm. Red arrows indicate the damaged mitochondria. Values are normalized by setting the control value as 1. ## represents P < 0.01, and * represents P < 0.05. M, mitochondria; LD, lipid droplet; CN, cell nucleus.
3.4. MMP alterations
As shown in Figure 3, Cd exposures decreased the MMPs of liver mitochondria. In details, compared with the control group (2.78 ± 0.45), MMPs in 5 (1.57 ± 0.49) and 50 μg/L (1.69 ± 0.19) Cd-treated groups were significantly (P < 0.01) decreased (Figure 3). No significant difference between both Cd groups.
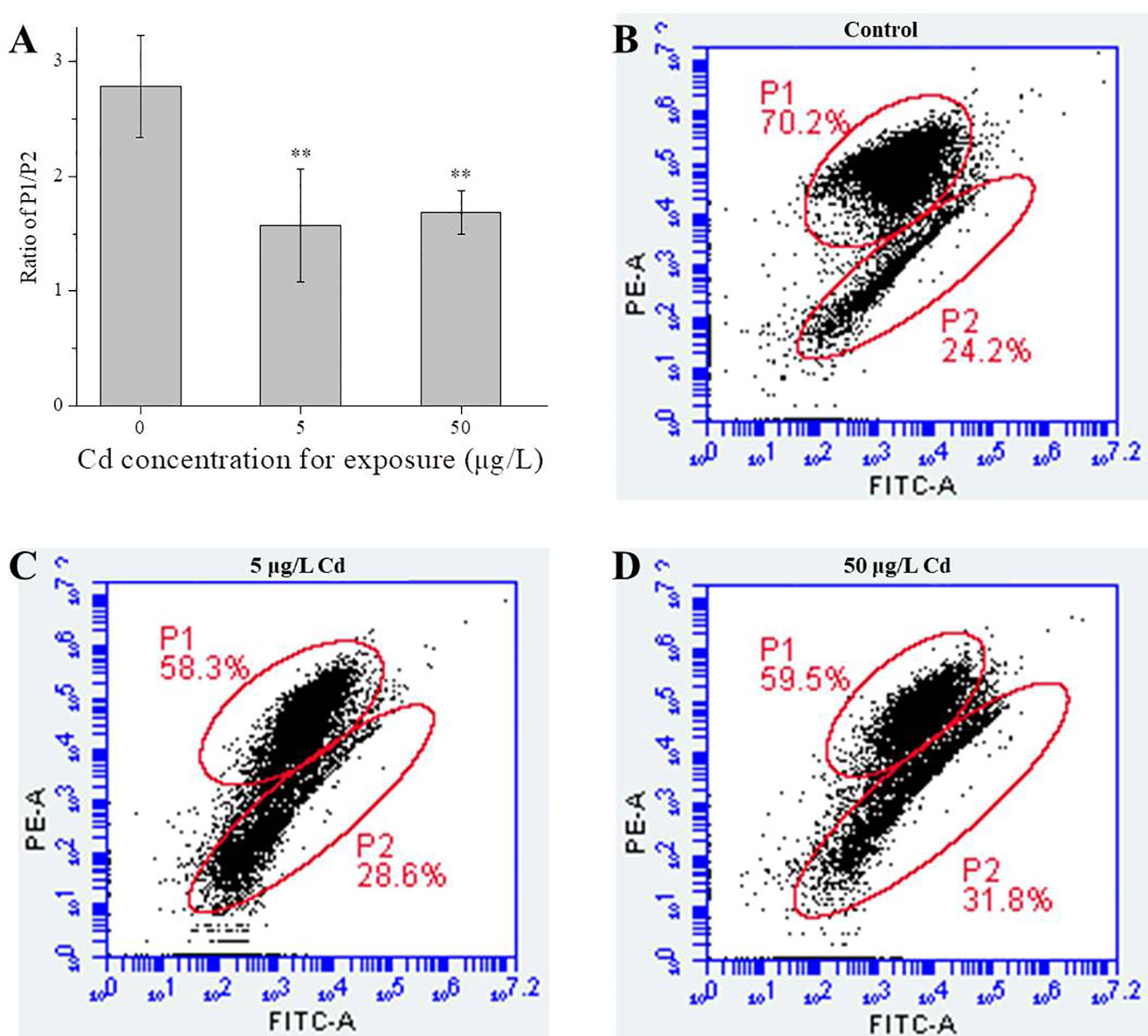
Figure 3 MMP changes of liver mitochondria from control and Cd treatments. (A), ratio of P1/P2 for liver mitochondria (n = 4). (B–D) typical scatter plots of MMPs detected by FCM. ** represents P < 0.01.
3.5. Overview of liver metabolome
For metabolomic analysis, NMR spectral data were analyzed by orthogonal partial least squares discrimination analysis (OPLS-DA). Robust classifications were observed between the control and two Cd treatments, with reliable Q2 values of 0.726 and 0.877, respectively. Significant differences for metabolites between control and Cd treatments were reflected by their corresponding loading plots (Figures 4C, D).
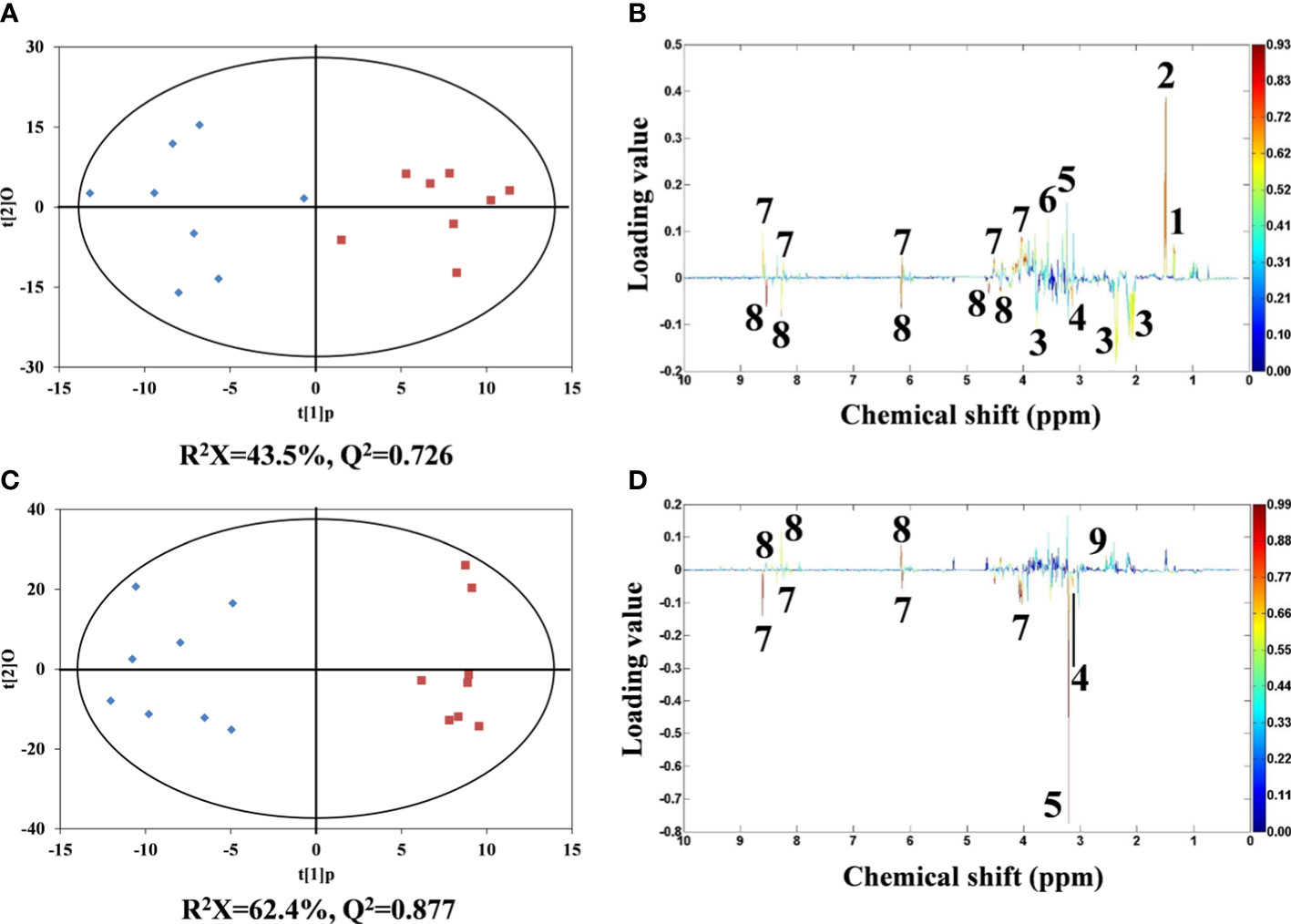
Figure 4 OPLS-DA scores derived from 1H NMR spectra of flounder liver extracts from control (♦) and Cd treatments (▪). (A) 5 and (C) 50 µg/L of Cd treatments and corresponding coefficient plots (B) and (D). The color map expresses the significance of metabolite variations between two classes (control and Cd treatments). Peaks in the positive and negative direction indicate metabolites that are more abundant in the Cd treatments and control group, respectively. Keys: (1) lactate, (2) alanine, (3) glutamate, (4) malonate, (5) phosphocholine, (6) glycine, (7) AMP, (8) ATP and (9) succinate.
Compared with the metabolic profiles of flounder livers in control group, the liver samples in 5 μg/L Cd treatment showed increased alanine, lactate, glycine, phosphocholine and AMP, and decreased glutamate, ATP and malonate (Figure 4B). And 50 μg/L Cd-treated flounder livers had elevated succinate and ATP, and the depleted malonate, phosphocholine and AMP (Figure 4D). Interestingly, the alterations of ATP, AMP and phosphocholine showed opposite trends in two Cd treatments.
3.6. Overview of mitochondrial proteome
The differentially expressed mitochondrial proteins were analyzed by iTRAQ-based proteomics to further clarify the Cd-induced mitochondrial responses. There were 3381 identified proteins, of which 280 proteins were significantly affected by Cd exposure. Among them, only 27 proteins were known mitochondrial proteins. In details, 14 DEPs were identified in 5 μg/L Cd treatment, containing 13 down-regulated DEPs (P < 0.05, foldchange < 0.83) and 1 up-regulated DEP (foldchange > 1.2, P < 0.05). Moreover, 19 out of 27 DEPs were identified in 50 μg/L Cd treatment, including 16 down-regulated and 3 up-regulated DEPs (Figure 5A). Two Cd treatments shared six (22.2%) common DEPs (Figure 5B). Apparently, most DEPs were down-regulated in liver mitochondria induced by Cd treatments. As shown in Table S1, these mitochondrial DEPs were classified based on their functions to reveal the Cd-induced mitochondrial toxicity in flounder livers (Figures 5C–H).
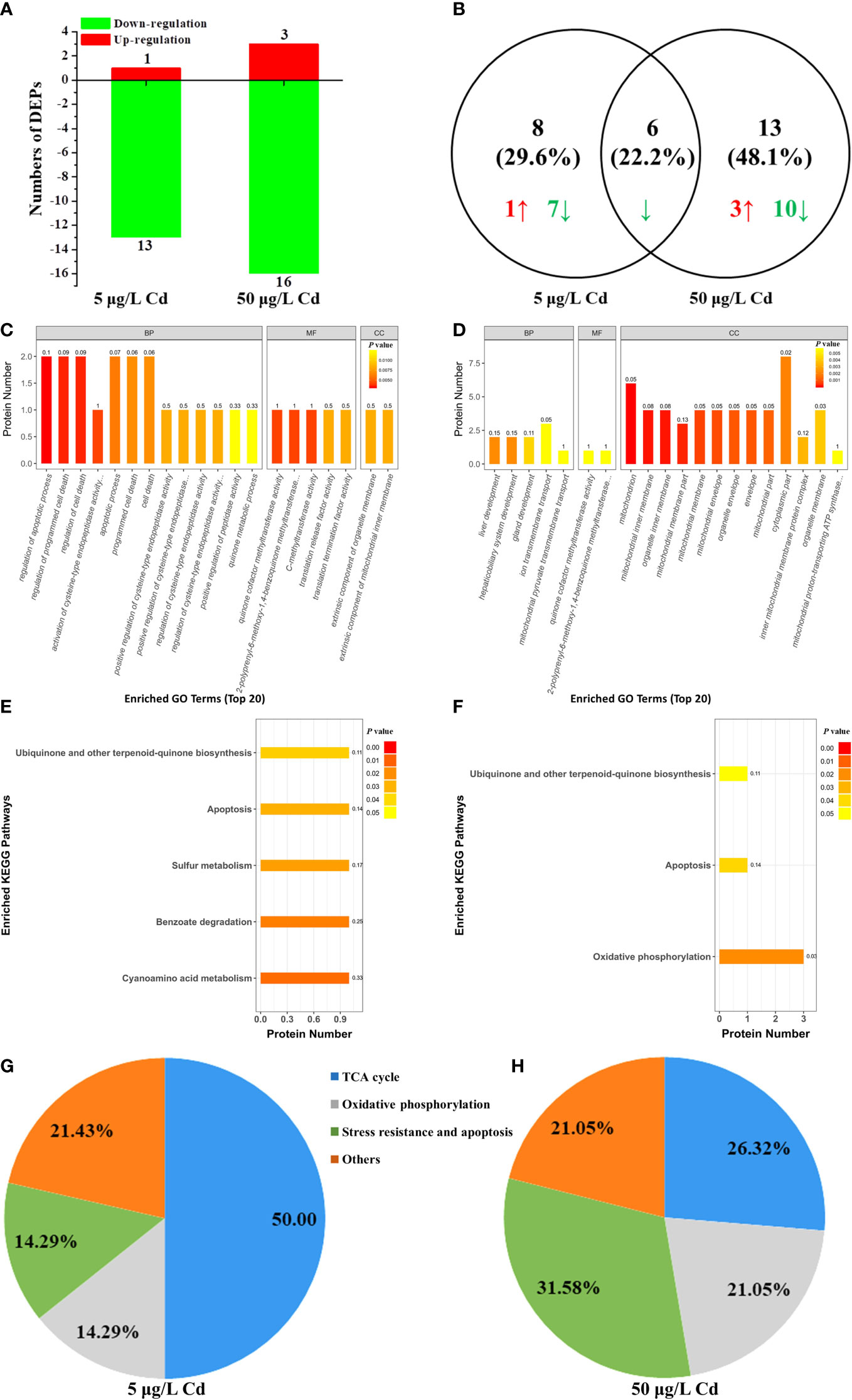
Figure 5 iTRAQ-based proteomic analysis of mitochondrial proteins from control, 5 μg/L and 50 μg/L Cd treatments. (A) DEPs numbers in each group. Down- and up-regulated proteins are exhibited in green and red, respectively. (B) venn diagrams of DEPs between 5 and 50 μg/L Cd treatments. Green and red arrows showed the down- and up-regulated DEPs, respectively. (C, D) the 20 most significantly (P < 0.05) enriched GO terms based on mitochondrial DEPs of flounder livers in 5 μg/L (C) and 50 μg/L (D) Cd treatments. (E, F) the significantly (P < 0.05) enriched KEGG pathways based on mitochondrial DEPs of flounder livers in 5 μg/L (E) and 50 μg/L (F) Cd treatments. (G, H) functional classification of liver mitochondrial DEPs in 5 μg/L (G) and 50 μg/L (H) Cd treatments.
4. Discussion
4.1. Effects of Cd on mitochondrial morphology and import
Morphology and number of mitochondria are closely linked to the cell viability and energy status (Karbowski and Youle, 2003). In this study, mitochondrial density was increased after Cd treatments (Figure 2D), indicating that Cd caused more mitochondria in flounder livers. Consistently, similar increases of mitochondrial number in oysters Crassostrea virginica and flounder P. olivaceus gills were observed (Sokolova et al., 2005b; Lu et al., 2020), which was thought to be the compensatory mechanism for respiratory depression due to the Cd toxicity. In addition, Cd induced mitochondrial ultrastructural damages and large numbers of lipid droplets within the liver cells. Similarly, Thophon et al. (2010) found ultrastructural alterations (mitochondrial condensation, swelling, and lysis) and abundant stored glycogen and numerous lipid droplets in white sea bass Lates calcarifer livers after Cd treatments. Cd could bind to biomolecules, accumulate or deposit in lipid droplets and liposomes, further disturb the metabolic pathways (Deb and Fukushima, 1999). These findings indicated that Cd disturbed the normal physiological and metabolic functions of mitochondria in flounder livers by altering the mitochondrial structure, number and morphology.
Proteomic analysis showed that there were no DEPs related to mitochondrial morphology, but only one DEP was associated with mitochondrial import (Table S1). The mitochondrial inner membrane translocase subunit Tim8A (Tim8A) could promote the import of mitochondrial inner membrane substrates (such as Tim23) (Karin et al., 2004). The down-regulation of Tim8A indicated the disturbed mitochondrial protein import in flounder livers induced by 50 μg/L Cd treatment, which may be connected with the ultrastructural damages of liver mitochondrial membranes observed by TEM (Figure 2). In our previous study, fourteen DEPs associated with mitochondrial morphology and import were discovered in gill mitochondria under the same exposure conditions, which demonstrated that gill mitochondria mobilized more relevant proteins to meet the Cd challenges compared with liver mitochondria.
4.2. Effects of Cd on mitochondrial-dependent energy metabolism
Apart from the main organ for accumulation and detoxification of pollutants in fish, liver is also an important metabolic organ involved in the metabolism of carbohydrate, fat, protein, vitamins, hormones, and other components (Tseng and Hwang, 2008). In this study, oxidative phosphorylation (OXPHOS) and the tricarboxylic acid (TCA) cycle were affected in flounder livers by Cd exposure (Figure 6).
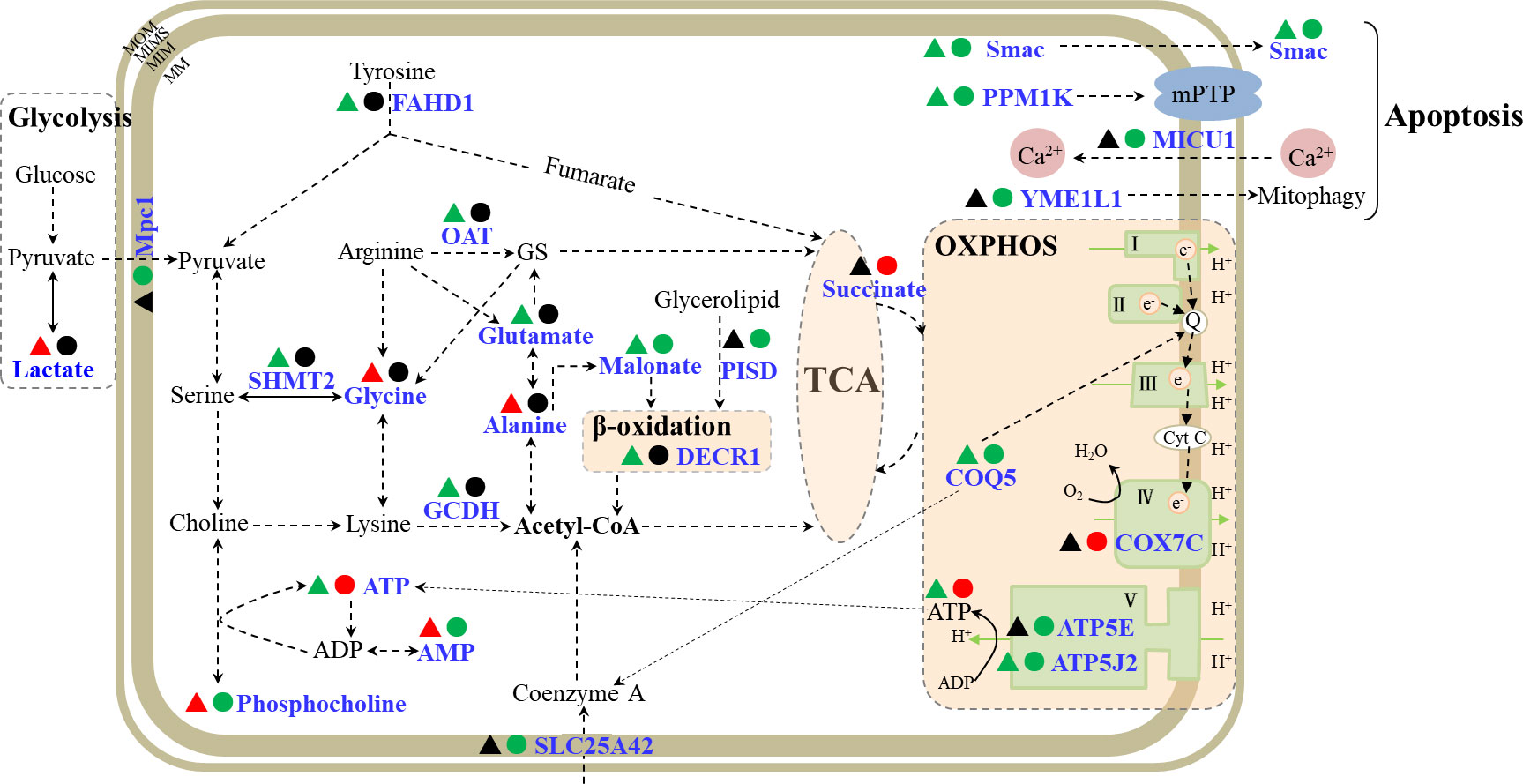
Figure 6 A schematic diagram of mitochondrial responses in flounder livers induced by Cd exposures. The metabolites and proteins involved in OXPHOS, TCA cycle and apoptosis were showed in the schematic. The responsive metabolites or proteins were marked in blue, and the down- and up- regulated proteins or metabolites in 5 μg/L (▴) and 50 μg/L (●) Cd treatments were labeled in green and red. MIM, mitochondrial inner membrane; MOM, mitochondrial inner membrane; MIMS, mitochondrial intermembrane space; MS, mitochondrial matrix; OXPHOS, oxidative phosphorylation; TCA, tricarboxylic acid cycle; MPTP, mitochondrial permeability transition pore; GS, L-glutamate-5-semaldehyde; FAHD1, acylpyruvase; ATP5J2, ATP synthase subunit f; COQ5, 2-methoxy-6-polyprenyl-1,4-benzoquinol methylase; COX7C, cytochrome c oxidase subunit 7C; ATP5E, ATP synthase subunit epsilon; Mpc1, mitochondrial pyruvate carrier 1; SLC25A42, mitochondrial coenzyme A transporter; PISD, phosphatidylserine decarboxylase proenzyme; DECR1, 2,4-dienoyl-CoA reductase; GCDH, glutaryl-CoA dehydrogenase; OAT, ornithine aminotransferase; SHMT2, serine hydroxymethyltransferase; Smac, second mitochondria-derived activator of caspase homolog; PPM1K, protein phosphatase 1K; MICU1, calcium uptake protein 1; YME1L1, ATP-dependent zinc metalloprotease.
4.2.1. TCA cycle
The TCA cycle is the central hub of carbon metabolism and coordinates various metabolic processes, such as glycometabolism, lipid metabolism and protein metabolism in aerobic organisms. There were 10 DEPs and 6 differentially expressed metabolites (DEMs) involved in TCA cycle (Figure 6; Table S1). Under anaerobic conditions, glucose could be converted into pyruvate and lactate through glycolysis. Pyruvate is not only the product of glycolysis, but also the initial substrate of the TCA cycle. Mitochondrial pyruvate carrier 1 (Mpc1) mediates the uptake of pyruvate into mitochondria (Bricker et al., 2012), and acylpyruvase (FAHD1) contributes to the production of pyruvate (Petit et al., 2017). Mpc1 showed down-regulation in 50 μg/L Cd treatment, while lactate was elevated and FAHD1 showed down-regulation in 5 μg/L Cd treatment, suggesting the enhanced anaerobic metabolism and inhibited contribution of glucose metabolism to TCA cycle in flounder livers induced by Cd treatments. On the other side, hydroxyacid-oxoacid transhydrogenase (ADHFE1) could catalyze the reduction process of α-ketoglutarate which serves as a bridge between amino acid and carbohydrate metabolism and is also a precursor of glutamine (Struys et al., 2005). ADHFE1 showed a down-regulated expression trend in both Cd treatments, indicating decreased reductive consumption of α-ketoglutarate, which might indirectly promote the production of glutamine in liver mitochondria. These proteins (Mpc1, FAHD1 and ADHFE1) and metabolite (lactate) showed that the glucose metabolism was inhibited and the contributions of glutamine metabolism to TCA cycle were strengthened in liver mitochondria from Cd-treated flounders.
Moreover, the contribution of acetyl-CoA conversion to the TCA cycle was affected through regulation of coenzyme A transport, amino acid metabolism and fatty acid metabolism in liver mitochondria after Cd treatments (Figure 6; Table S1). Mitochondrial coenzyme A transporter SLC25A42-like (SLC25A42) showed down-regulation in 50 μg/L Cd treatment, suggesting that the transport of coenzyme A and its conversion to acetyl-CoA were inhibited by 50 μg/L Cd. Glutaryl-CoA dehydrogenase (GCDH) catalyzes the oxidative decarboxylation during the degradation of amino acids and promotes the production of acetyl-CoA (Stefan et al., 2006). In 5 μg/L Cd treatment, the down-regulated GCDH manifested the reduced acetyl-CoA production by the inhibited amino acid catabolism. Besides, acetyl-CoA could also be generated during the β-oxidation of fatty acids (Houten and Wanders, 2010). Phosphatidylserine decarboxylase proenzyme (PISD) promotes the production of fatty acids (Schuiki and Daum, 2010), and 2,4-dienoyl-CoA reductase (DECR1) is a coenzyme in the β-oxidation process of polyunsaturated fatty acid (Miinalainen et al., 2009). DECR1 and PISD exhibited down-regulation in 5 and 50 μg/L Cd treatments respectively, indicating decreased acetyl-CoA production due to the inhibited production of fatty acids and β-oxidation. These four significantly down-regulated mitochondrial proteins (SLC25A42, GCDH, DECR1 and PISD) showed that the contributions of acetyl-CoA conversion to TCA cycle were suppressed in liver mitochondria of Cd-treated flounders.
In addition, succinate generates from TCA cycle and acts as the substrate for complex II. According to the metabolomic profile, succinate was uniquely increased in 50 μg/L Cd treatment, which might compensate for inhibited contributions from glycolysis, amino acid metabolism and β-oxidation.
These results showed inhibited contribution of glucose metabolism and acetyl-CoA conversion to TCA cycle, and promoted contributions of glutamine metabolism to TCA cycle in liver mitochondria of flounders induced by both Cd treatments. Interestingly, an opposite pattern in gill mitochondria was observed in our previous research (Lu et al., 2020), implying the tissue-specific effects on the TCA cycle induced by Cd.
4.2.2. Oxidative phosphorylation
Most of the energy for maintaining normal cell functions is generated from OXPHOS (Senior, 1988). OXPHOS generally consists of five complexes, covering ATP synthase (complex V), NADH dehydrogenase (complex I), cytochrome c reductase (complex III), complex IV, and succinate dehydrogenase (complex II). In our previous study, many proteins associated with all five complexes showed up-regulated trends in gill mitochondria of Cd-treated flounders (Lu et al., 2020). However, in this work, only four proteins related to OXPHOS, including ATP synthase subunit epsilon (ATP5E), cytochrome c oxidase subunit 7C (COX7C), 2-methoxy-6-polyprenyl-1,4-benzoquinol methylase (COQ5), ATP synthase subunit f (ATP5J2), were altered in liver mitochondria of flounder after Cd treatments (Figure 6, Table S1). Among these proteins, COQ5 and ATP5J2 were inhibited in both Cd-treated groups, while ATP5E was down-regulated and COX7C was up-regulated in 50 μg/L Cd treatment. COQ5 could catalyze the only C-methylation in coenzyme Q biosynthesis (ubiquinone or Q) (Nguyen et al., 2014), and Q is responsible for transferring electrons from complex II and complex I to complex III. Both Cd treatments induced significant down-regulation of COQ5 in flounders, implying depressed electron transportation in flounder livers induced by Cd exposures. Complex IV could catalyze the electron transport from cytochrome c to molecular oxygen (Valnot et al., 2000). As an important subunit of complex IV, the up-regulation of COX7C might induce increased oxygen consumption in 50 μg/L Cd-treated flounders. Besides, complex V could generate ATP from ADP using the proton-motive force (Junge et al., 1997). The down-regulated ATP5J2 in two Cd treatments, and down-regulation of ATP5E in 50 μg/L Cd treatment, indicated inhibition of ATP production induced by Cd treatments, which was in accordance with the decreased ATP in 5 μg/L Cd treatment (Figure 4). Conversely, metabolomic analysis of 50 μg/L Cd-treated samples showed increased ATP and decreased phosphocholine and AMP. Phosphocholine could react with ADP to convert into ATP and choline (Pomfret et al., 1989). Thus, the significant increase of ATP in 50 μg/L Cd-treated flounder livers may result from the biotransformation of phosphocholine. Overall, these altered DEPs (COQ5, COX7C, ATP5J2 and ATP5E) and energy metabolites (ATP, AMP and phosphocholine) probably implied the decreased electron transportation, increased oxygen consumption and unbalanced ATP production in flounder livers induced by Cd treatments.
4.3. Effects of Cd on mitochondrial-dependent apoptosis
In this work, six proteins involved in apoptosis were identified after Cd treatments (Figure 6, Table S1). Among these DEPs, second mitochondria-derived activator of caspase homolog (Smac) and protein phosphatase 1K (PPM1K) commonly showed down-regulation in both Cd treatments. The other four DEPs, ATP-dependent RNA helicase (SUPV3L1), calcium uptake protein 1 (MICU1), ATP-dependent RNA helicase (DDX3X), and ATP-dependent zinc metalloprotease (YME1L1), were uniquely changed in 50 μg/L Cd treatment, suggesting stronger effects on apoptosis induced by 50 μg/L Cd. PPM1K regulates mitochondrial permeability transition pore, its knockdown or deficiency could cause the loss of MMP and apoptosis (Lu et al., 2007). In this study, the down-regulated PPM1K was identified, consistent with the decreased MMPs (Figure 3) in both Cd treatments. MICU1 governs the uptake of Ca2+ by mitochondria and relieves mitochondrial damage and apoptosis caused by Ca2+ overload (Madesh et al., 2012). The significantly down-regulated PPM1K and MICU1 suggested that Cd exposure caused the decrease of MMP and Ca2+ overload in flounder livers, and may further induce cell apoptosis. Additional four DEPs, including Smac, DDX3X, SUPV3L1 and YME1L1, were regulatory proteins of apoptosis. When released from mitochondria, Smac could specifically bind to apoptosis inhibitors and activate the cytochrome c/Apaf-1/caspase-9 pathway to promote apoptosis (Du et al., 2000); DDX3X and SUPV3L1 participate in RNA regulation and exert anti-apoptotic protein functions (Szczesny et al., 2007; Li et al., 2014); and YME1L1 is essential for apoptosis resistance, cristae morphogenesis, and cell proliferation (Stiburek, 2012). Smac showed down-regulation in both Cd treatments, SUPV3L1 and YME1L1 were down-regulated and DDX3X was up-regulated in 50 μg/L Cd treatment, implying that Cd treatments interfered with the mitochondrial-dependent apoptosis pathway in flounder livers.
5. Conclusion
In this study, a comprehensive view of mitochondrial response of liver mitochondria, including mitochondrial morphology, energy metabolism and apoptosis, were substantially changed by Cd treatments. In addition, more mitochondria with decreased MMPs were identified in flounder livers after Cd exposure. Our results further confirmed that mitochondria were main targets of Cd toxicity, and dynamic regulation of mitochondrial morphology, apoptosis and energy homeostasis in liver mitochondria may play essential roles in coping with Cd exposure for flounders. This work presented a global evaluation on Cd-induced mitochondrial toxicity in flounder livers, offering novel insight into the toxicity mechanism of Cd in marine animals.
Data availability statement
The original contributions presented in the study are included in the article/Supplementary Material. Further inquiries can be directed to the corresponding author.
Ethics statement
The animal study was reviewed and approved by the Ethics Committee of Yantai Institute of Coastal Zone Research, Chinese Academy of Sciences (CAS).
Author contributions
CRediT authorship contribution statement ZL: Formal analysis, Writing - original draft, Project administration, Funding acquisition. ZX: Data analysis, Writing - review & editing. SW: Data analysis, Writing - review & editing. JS: Supervision, Project administration, Writing - review & editing. XP: Supervision, Funding acquisition, Writing - review & editing, Project administration. All authors contributed to the article and approved the submitted version.
Funding
This work was supported by the National Natural Science Foundation of China (grant numbers 31771584); the Guangdong Basic and Applied Basic Research Foundation (grant number 2022A1515011845); the China Postdoctoral Science Foundation (grant number 2020M682858).
Acknowledgments
We thank Prof. Mark Viant for the use of ProMetab software.
Conflict of interest
The authors declare that the research was conducted in the absence of any commercial or financial relationships that could be construed as a potential conflict of interest.
Publisher’s note
All claims expressed in this article are solely those of the authors and do not necessarily represent those of their affiliated organizations, or those of the publisher, the editors and the reviewers. Any product that may be evaluated in this article, or claim that may be made by its manufacturer, is not guaranteed or endorsed by the publisher.
Supplementary material
The Supplementary Material for this article can be found online at: https://www.frontiersin.org/articles/10.3389/fmars.2022.1041705/full#supplementary-material
References
Bertin G., Averbeck D. (2006). Cadmium: cellular effects, modifications of biomolecules, modulation of DNA repair and genotoxic consequences (a review). Biochimie 88 (11), 1 549–1 559. doi: 10.1016/j.biochi.2006.10.001
Bricker D. K., Taylor E. B., Schell J. C., Orsak T., Boutron A., Chen Y. C., et al. (2012). A mitochondrial pyruvate carrier required for pyruvate uptake in yeast. Drosophila Humans Sci. 337 (6090), 96–100. doi: 10.1126/science.1218099
Charan-Dixon H., Gaw S., Goldstien S. J., Glover C. N. (2017). Effects of waterborne cadmium on energy metabolism in the tropical sea cucumber, Stichopus horrens, and a comparison of tissue-specific cadmium accumulation with the temperate sea cucumber Australostichopus mollis. Ecotoxicol. Environ. Saf. 141, 1–8. doi: 10.1016/j.ecoenv.2017.03.001
Cui W., Cao L., Liu J., Ren Z., Zhao B., Dou S. (2020). Effects of seawater acidification and cadmium on the antioxidant defense of flounder. Paralichthys Olivaceus Larvae Sci. Total Environ. 718, 137234. doi: 10.1016/j.scitotenv.2020.137234
Deb S. C., Fukushima T. (1999). Metals in aquatic ecosystems: mechanisms of uptake, accumulation and release - ecotoxicological perspectives. Int. J. Environ. Stud. 56 (3), 385–417. doi: 10.1080/00207239908711212
Du C., Fang M., Li Y., Li L., Wang X. (2000). Smac, a mitochondrial protein that promotes cytochrome c-dependent caspase activation by eliminating IAP inhibition. Cell 102 (1), 33–42. doi: 10.1016/S0092-8674(00)00008-8
Gao X., Zhou F., Chen C. T. A. (2014). Pollution status of the bohai Sea: An overview of the environmental quality assessment related trace metals. Environ. Int. 62, 12–30. doi: 10.1016/j.envint.2013.09.019
Houten S. M., Wanders R. J. A. (2010). A general introduction to the biochemistry of mitochondrial fatty acid β-oxidation. J. Inherited Metab. Dis. 33 (5), 469–477. doi: 10.1007/s10545-010-9061-2
Ji C., Lu Z., Xu L., Li F., Cong M., Shan X., et al. (2019). Evaluation of mitochondrial toxicity of cadmium in clam Ruditapes philippinarum using iTRAQ-based proteomics. Environ. pollut. 251, 802–810. doi: 10.1016/j.envpol.2019.05.046
Junge W., Lill H., Engelbrecht S. (1997). ATP synthase: an electrochemical transducer with rotatory mechanics. Trends Biochem. Sci. 22 (11), 420–423. doi: 10.1016/S0968-0004(97)01129-8
Karbowski M., Youle R. J. (2003). Dynamics of mitochondrial morphology in healthy cells and during apoptosis. Cell Death Diff. 10 (8), 870–880. doi: 10.1038/sj.cdd.4401260
Karin R., Hynds P. J., Renee V., Lisbeth T., Koehler C. M. (2004). The calcium-binding aspartate/glutamate carriers, citrin and aralar1, are new substrates for the DDP1/TIMM8a-TIMM13 complex. Hum. Mol. Genet. 13 (18), 2 101–2 111. doi: 10.1093/hmg/ddh217
Kurochkin I. O., Etzkorn M., Buchwalter D., Leamy L., Sokolova I. M. (2011). Top-down control analysis of the cadmium effects on molluscan mitochondria and the mechanisms of cadmium-induced mitochondrial dysfunction. Am. J. Physiol.-Regul. Integr. Comp. Physiol. 300 (1), R21–R31. doi: 10.1152/ajpregu.00279.2010
Lane T. W., Morel F. M. M. (2000). A biological function for cadmium in marine diatoms. Proc. Natl. Acad. Sci. United States America 97 (9), 4 627–4 631. doi: 10.1073/pnas.090091397
Leahy J., Spahis S., Bonneil E., Garofalo C., Grimard G., Morel S., et al. (2018). Insight from mitochondrial functions and proteomics to understand cardiometabolic disorders in survivors of acute lymphoblastic leukemia. Metabolism 85, 151–160. doi: 10.1016/j.metabol.2018.03.011
Li H., Gao X., Gu Y., Wang R., Xie P., Liang M., et al. (2018). Comprehensive large-scale investigation and assessment of trace metal in the coastal sediments of bohai Sea. Mar. pollut. Bull. 129 (1), 126–134. doi: 10.1016/j.marpolbul.2018.02.022
Li H., Zhang W., Zhang H., Xie Y., Sun C., Di C., et al. (2019). Mitochondrial proteomics reveals the mechanism of spermatogenic cells apoptosis induced by carbon ion radiation in zebrafish. J. Cell. Physiol. 234 (12), 22 439–22 449. doi: 10.1002/jcp.28808
Li Q., Zhang P., Zhang C., Wang Y., Wan R., Yang Y., et al. (2014). DDX3X regulates cell survival and cell cycle during mouse early embryonic development. J. Biomed. Res. 28 (4), 282–291. doi: 10.7555/JBR.27.20130047
Liu J., Cao L., Dou S. (2019). Trophic transfer, biomagnification and risk assessments of four common heavy metals in the food web of laizhou bay, the bohai Sea. Sci. Total Environ. 670, 508–522. doi: 10.1016/j.scitotenv.2019.03.140
Lu G., Ren S., Korge P., Choi J., Yuan D., James W., et al. (2007). A novel mitochondrial matrix serine/threonine protein phosphatase regulates the mitochondria permeability transition pore and is essential for cellular survival and development. Genes Dev. 21 (7), 784–796. doi: 10.1101/gad.1499107
Lu G., Wang W. (2018). Trace metals and macroelements in mussels from Chinese coastal waters: National spatial patterns and normalization. Sci. Total Environ. 626, 307–318. doi: 10.1016/j.scitotenv.2018.01.018
Lu Z., Wang S., Ji C., Li F., Cong M., Shan X., et al. (2020). iTRAQ-based proteomic analysis on the mitochondrial responses in gill tissues of juvenile olive flounder Paralichthys olivaceus exposed to cadmium. Environ. pollut. 257, 113591. doi: 10.1016/j.envpol.2019.113591
Lu Z., Wang S., Shan X., Ji C., Wu H. (2019). Differential biological effects in two pedigrees of clam Ruditapes philippinarum exposed to cadmium using iTRAQ-based proteomics. Environ. Toxicol. Pharmacol. 65, 66–72. doi: 10.1016/j.etap.2018.12.002
Madesh M., Mallilankaraman K., Doonan P., Cardenas C., Chandramoorthy H. C., Muller M., et al. (2012). MICU1 is an essential gatekeeper for MCU-mediated mitochondrial Ca2+ uptake that regulates cell survival. Cell 151, 630–644. doi: 10.1016/j.cell.2012.10.011
Meyer J. N., Leung M. C. K., Rooney J. P., Sendoel A., Hengartner M. O., Kisby G. E., et al. (2013). Mitochondria as a target of environmental toxicants. Toxicol. Sci. 134 (1), 1–17. doi: 10.1093/toxsci/kft102
Miinalainen I. J., Werner S., Anne H., Autio K. J., Raija S., Emiel V. L. V. T., et al. (2009). Mitochondrial 2,4-dienoyl-CoA reductase deficiency in mice results in severe hypoglycemia with stress intolerance and unimpaired ketogenesis. PloS Genet. 5 (7), e1000543. doi: 10.1371/journal.pgen.1000543
Nguyen T. P. T., Casarin A., Desbats M. A., Doimo M., Trevisson E., Santos-Ocaña C., et al. (2014). Molecular characterization of the human COQ5 c-methyltransferase in coenzyme q 10 biosynthesis. Biochim. Biophys. Acta 1841 (11), 1 628–1 638. doi: 10.1016/j.bbalip.2014.08.007
Petit M., Koziel R., Etemad S., Pircher H., Jansen-Dürr P. (2017). Depletion of oxaloacetate decarboxylase FAHD1 inhibits mitochondrial electron transport and induces cellular senescence in human endothelial cells. Exp. Gerontol. 92, 7–12. doi: 10.1016/j.exger.2017.03.004
Pomfret E. A., daCosta K.-A., Schurman L. L., Zeisel S. H. (1989). Measurement of choline and choline metabolite concentrations using high-pressure liquid chromatography and gas chromatography-mass spectrometry. Analytical Biochem. 180 (1), 85–90. doi: 10.1016/0003-2697(89)90091-2
Schuiki I., Daum G. (2010). Phosphatidylserine decarboxylases, key enzymes of lipid metabolism. Int. Union Biochem. Mol. Biol. Life 61 (2), 151–162. doi: 10.1002/iub.159
Senior A. E. (1988). ATP synthesis by oxidative phosphorylation. Physiol. Rev. 68 (1), 177–231. doi: 10.1152/physrev.1988.68.1.177
Shaughnessy D. T., Jr. W. L., Lawler C. P., McAllister K. A., Longley M. J., Copeland W. C. (2010). Meeting report: Identification of biomarkers for early detection of mitochondrial dysfunction. Mitochondrion 10 (5), 579–581. doi: 10.1016/j.mito.2010.02.001
Sokolova I. M., Ringwood A. H., Johnson C. (2005b). Tissue-specific accumulation of cadmium in subcellular compartments of eastern oysters. Crassostrea Virginica Gmelin (Bivalvia: Ostreidae) Aquat. Toxicol. 74 (3), 218–228. doi: 10.1016/j.aquatox.2005.05.012
Sokolova I. M., Sokolov E. P., Ponnappa K. M. (2005a). Cadmium exposure affects mitochondrial bioenergetics and gene expression of key mitochondrial proteins in the eastern oyster. Crassostrea Virginica Gmelin (Bivalvia: Ostreidae) Aquat. Toxicol. 73 (3), 242–255. doi: 10.1016/j.aquatox.2005.03.016
Stefan K. L., Garbade S. F., Greenberg C. R., Leonard J. V., Jean-Marie S., Antonia R., et al. (2006). Natural history, outcome, and treatment efficacy in children and adults with glutaryl-CoA dehydrogenase deficiency. Pediatr. Res. 59 (6), 840–847. doi: 10.1203/01.pdr.0000219387.79887.86
Stiburek L. (2012). YME1L controls the accumulation of respiratory chain subunits and is required for apoptotic resistance, cristae morphogenesis, and cell proliferation. Mol. Biol. Cell 23 (6), 1 010–1 023. doi: 10.1091/mbc.e11-08-0674
Struys E. A., Verhoeven N. M., Ten Brink H. J., Wickenhagen W. V., Gibson K. M., Jakobs C. (2005). Kinetic characterization of human hydroxyacid–oxoacid transhydrogenase: Relevance toD-2-hydroxyglutaric and γ-hydroxybutyric acidurias. J. Inherited Metab. Dis. 28 (6), 921–930. doi: 10.1007/s10545-005-0114-x
Szczesny R. J., Obriot H., Paczkowska A., Jedrzejczak R., Dmochowska A., Bartnik E., et al. (2007). Down-regulation of human RNA/DNA helicase SUV3 induces apoptosis by a caspase- and AIF-dependent pathway. Biol. Cell 99 (6), 323–332. doi: 10.1042/BC20060108
Thophon S., Pokethitiyook P., Chalermwat K., Upatham E. S., Sahaphong S. (2010). Ultrastructural alterations in the liver and kidney of white sea bass, Lates calcarifer, in acute and subchronic cadmium exposure. Environ. Toxicol. Chem. 19 (1), 11–19. doi: 10.1002/tox.10146
Tseng Y. C., Hwang P. P. (2008). Some insights into energy metabolism for osmoregulation in fish. Toxicol. Pharmacol. 148 (4), 419–429. doi: 10.1016/j.cbpc.2008.04.009
Valnot I., Osmond S., Gigarel N., Mehaye B., Amiel J., Cormierdaire V., et al. (2000). Mutations of the SCO1 gene in mitochondrial cytochrome c oxidase deficiency with neonatal-onset hepatic failure and encephalopathy. Am. J. Hum. Genet. 67 (5), 1 104–1 109. doi: 10.1016/S0002-9297(07)62940-1
Viant M. R., Rosenblum E. S., Tieerdema R. S. (2003). NMR-based metabolomics: a powerful approach for characterizing the effects of environmental stressors on organism health. Environ. Sci. Technol. 37 (21), 4 982–4 989. doi: 10.1021/es034281x
Wu H., Wang W. X. (2015). Tissue-specific toxicological effects of cadmium in green mussels (Perna viridis): nuclear magnetic resonance-based metabolomics study. Environ. Toxicol. Chem. 30 (4), 806–812. doi: 10.1002/etc.446
Yang J., Liu D., He Y., Wang L. (2015). Mitochondrial energy metabolism in the hepatopancreas of freshwater crabs (Sinopotamon henanense) after cadmium exposure. Environ. Sci.: Processes Impacts 17 (1), 156–165. doi: 10.1039/C4EM00453A
Yu D., Ji C., Zhao J., et al. (2016). Proteomic and metabolomic analysis on the toxicological effects of as (III) and as (V) in juvenile mussel Mytilus galloprovincialis. Chemosphere 150 (3), 194–201. doi: 10.1016/j.chemosphere.2016.01.113
Zhu J. Y., Huang H. Q., Bao X. D., Lin Q., Cai Z., et al. (2006). Acute toxicity profile of cadmium revealed by proteomics in brain tissue of Paralichthys olivaceus: Potential role of transferrin in cadmium toxicity. Aquat. Toxicol. 78 (2), 127–135. doi: 10.1016/j.aquatox.2006.02.010
Keywords: cadmium, mitochondrial toxicity, mitochondrial proteomics, metabolomics, Paralichthys olivaceus
Citation: Lu Z, Xiao Z, Wu S, Song J and Peng X (2022) Proteomic and metabolomic analysis on cadmium-induced mitochondrial toxicity in liver tissues of juvenile olive flounder Paralichthys olivaceus. Front. Mar. Sci. 9:1041705. doi: 10.3389/fmars.2022.1041705
Received: 28 September 2022; Accepted: 08 November 2022;
Published: 25 November 2022.
Edited by:
Yafei Duan, South China Sea Fisheries Research Institute, ChinaReviewed by:
Gianfranco Santovito, University of Padua, ItalyLinbao Zhang, South China Sea Fisheries Research Institute, Chinese Academy of Fishery Sciences (CAFS), China
Copyright © 2022 Lu, Xiao, Wu, Song and Peng. This is an open-access article distributed under the terms of the Creative Commons Attribution License (CC BY). The use, distribution or reproduction in other forums is permitted, provided the original author(s) and the copyright owner(s) are credited and that the original publication in this journal is cited, in accordance with accepted academic practice. No use, distribution or reproduction is permitted which does not comply with these terms.
*Correspondence: Xiao Peng, cGVuZ3hpYW9fcHhAc3p1LmVkdS5jbg==