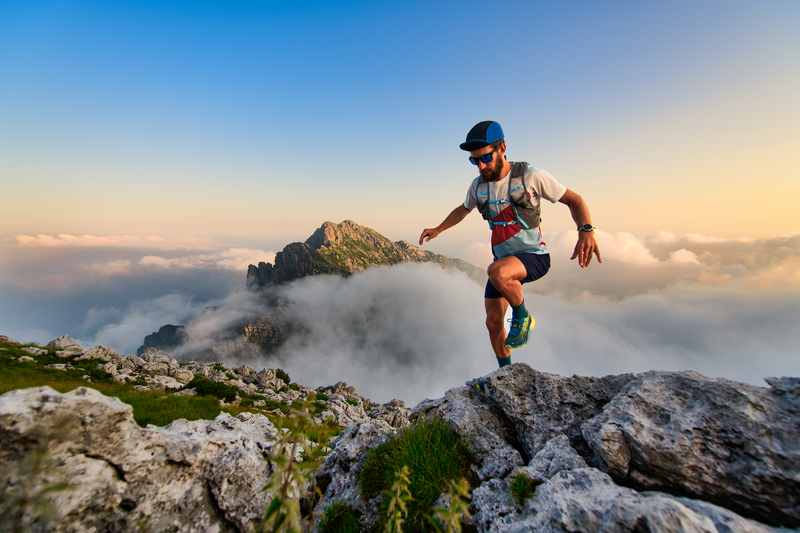
95% of researchers rate our articles as excellent or good
Learn more about the work of our research integrity team to safeguard the quality of each article we publish.
Find out more
ORIGINAL RESEARCH article
Front. Mar. Sci. , 10 November 2022
Sec. Coral Reef Research
Volume 9 - 2022 | https://doi.org/10.3389/fmars.2022.1026321
This article is part of the Research Topic ICYMARE - Early Career Researchers in Marine Science View all 32 articles
Hard corals are in decline as a result of the simultaneous occurrence of global (e.g., ocean warming) and local (e.g., inorganic eutrophication) factors, facilitating phase shifts towards soft coral dominated reefs. Yet, related knowledge about soft coral responses to anthropogenic factors remains scarce. We thus investigated the ecophysiological response of the pulsating soft coral Xenia umbellata to individual and combined effects of phosphate enrichment (1, 2, and 8 μM) and ocean warming (26 to 32°C) over 35 days. Throughout the experiment, we assessed pulsation, mortality, Symbiodiniaceae density, and cellular chlorophyll a content. Simulated ocean warming up to 30°C led to a significant increase in polyp pulsation and by the end of the experiment to a significant increase in Symbiodiniaceae density, whereas cellular chlorophyll a content significantly decreased with warming, regardless of the phosphate treatment. The combination of phosphate enrichment and simulated ocean warming increased pulsation significantly by 41 – 44%. Warming alone and phosphate enrichment alone did not affect any of the investigated response parameters. Overall, X. umbellata displayed a high resilience towards ocean warming with no mortality in all treatments. Phosphate enrichment enabled soft corals to significantly increase their pulsation under increasing temperatures which may enhance their resilience towards ocean warming. This, in turn, could further facilitate their dominance over hard corals on future reefs.
Tropical coral reefs provide essential ecosystem services including food provision, carbon sequestration, and coastal protection (Connell, 1978; Hoegh-Guldberg, 1999; Moberg and Folke, 1999). Yet, the simultaneous occurrence of global and local factors has contributed to the decline of live coral cover and the capacity of coral reefs to provide ecosystem services by half since the 1950s (Eddy et al., 2021). Specifically, the simultaneous occurrence of global ocean warming and local eutrophication exacerbates this alarming trend (Hall et al., 2018; Zhao et al., 2021).
Both ocean warming and eutrophication have been linked to bleaching in hard corals (Pogoreutz et al., 2017; Cziesielski et al., 2019; Fernandes de Barros Marangoni et al., 2020). In most cases, extensive coral bleaching has been attributed to elevated ocean temperatures (Brown, 1997). Following bleaching, hard corals can return to pre-bleaching states if the stressful conditions are relatively mild and short-term (Fitt et al., 1993; Brown, 1997). Nevertheless, mass coral bleaching events have increased in magnitude and frequency in response to steadily warming ocean temperatures, leaving reef-building corals with insufficient time to recover (Hughes et al., 2017; Hughes et al., 2018). In addition, reduced water quality can interact with ocean warming and exacerbate the thermal-induced bleaching response in hard corals (Koop et al., 2001; Fabricius et al., 2013; Wiedenmann et al., 2013; Fernandes de Barros Marangoni et al., 2020). Inorganic eutrophication, especially in form of nitrate enrichment, can cause an increase in density in algal symbionts of the family Symbiodiniaceae in host corals, thereby possibly shifting Symbiodiniaceae from a nitrogen limited to a phosphate starved state (Wiedenmann et al., 2013; Rädecker et al., 2015). Eutrophication can also shift the mutualism between host corals and Symbiodiniaceae towards parasitism or increase oxidative stress in corals (Baker et al., 2018; Fernandes de Barros Marangoni et al., 2020). Subsequently, corals are more susceptible to bleaching due to the destabilization of the coral-algal symbiosis (Rädecker et al., 2021).
Together, global and local anthropogenic factors lead to hard coral decline and thus facilitate phase shifts on coral reefs towards new, rather opportunistic benthic organisms (Wild et al., 2011; Naumann et al., 2015; Baum et al., 2016). These are often associated with shifts from hard coral dominated to macroalgae dominated communities, while shifts towards other benthic communities following a disturbance are also possible outcomes (Done, 1992; McCook, 1999; McManus and Polsenberg, 2004; Norström et al., 2009). Several studies observed an increase in soft coral abundances or reefs shifting from a previously hard coral dominated to a soft coral dominated state (Robinson, 1971; Bell, 1992; Fox et al., 2003; Stobart et al., 2005; Tilot et al., 2008; Inoue et al., 2013). Norström et al. (2009) hypothesized that shifts from hard to soft coral dominated reefs are often related to changes in the dynamics of bottom-up factors such as eutrophication. Although soft corals are important constituents in coral reef ecosystems, influencing ecological and biochemical processes on coral reefs (Changyun et al., 2008; Jeng et al., 2011; Epstein and Kingsford, 2019; Steinberg et al., 2020; El-Khaled et al., 2021), they do not provide the diverse ecosystem services of hard corals (Inoue et al., 2013; Bednarz et al., 2015; Hall-Spencer and Harvey, 2019).
Soft corals are successful colonizers due to fast growth rates, high fecundity, multiple dispersal modes, and strong chemical defense mechanisms (Benayahu and Loya, 1985; Fox et al., 2003; Changyun et al., 2008). These features help soft corals to dominate space, pre-empty settlement room, inhibit hard coral recruitment, or imped hard coral growth on reefs (Fox et al., 2003; Norström et al., 2009). This makes them strong competitors against hard corals, specifically under environmental pressures. Soft corals display a considerable resilience towards global factors such as ocean warming and acidification (Inoue et al., 2013; Sammarco and Strychar, 2013; Lopes et al., 2018). In addition, some soft coral species benefit from active heterotrophy by helping them to sustain their metabolism requirements during thermal stress (Fabricius and Dommisse, 2000; Baum et al., 2016). Soft corals generally rely more on heterotrophic feeding because of their lower photosynthetic activity compared to hard corals (Fabricius and Klumpp, 1995; Bednarz et al., 2012). Indeed, reefs dominated by zooxanthellate soft corals extract large quantities of suspended particulate matter from the water column due to high levels of heterotrophy (Fabricius and Dommisse, 2000). In particular, pulsating soft corals seem to cope better under ocean warming and eutrophication (Vollstedt et al., 2020). Recently, Vollstedt et al. (2020) observed that the pulsating soft coral Xenia umbellata benefits from organic eutrophication (in form of glucose), resulting in an increased resilience to warming. In contrast, inorganic eutrophication (in form of nitrate) decreased the resilience of X. umbellata to warming (Thobor et al., 2022) supporting the finding of Wiedenmann et al. (2013): high N:P ratios can decrease the resilience of corals to warming, possibly due to a destabilized symbiosis between the coral host and its Symbiodiniaceae following phosphate starvation. Phosphate generally plays an important role during thermal stress in corals by maintaining Symbiodiniaceae density and photosynthetic rates (Ezzat et al., 2016).
In the last decades, the body of literature concerning multiple stressor effects on coral reefs is growing (Harborne et al., 2016; Ellis et al., 2019; Cannon et al., 2021). Yet, there are notable gaps in the literature with respect to numerous interaction pairs including temperature as a global factor and eutrophication as a local factor (Ban et al., 2014). Additionally, previous studies concentrated on threats to hard corals with less attention on soft corals, despite their potentially important ecological roles and increasing occurrence on many coral reefs (Norström et al., 2009; Epstein and Kingsford, 2019; Steinberg et al., 2020). Hence, understanding how the ecophysiology of soft corals is affected by anthropogenic factors and what drives shifts on coral reefs towards a soft coral dominated state is fundamental for predicting future scenarios and implementing management actions.
To improve our understanding of the combined effects of inorganic eutrophication and warming on soft corals, this study aims to answer the following research questions: (1) How does phosphate enrichment affect X. umbellata? and (2) How does phosphate enrichment affect the response of X. umbellata to warming? The pulsating soft coral X. umbellata was used as a study organism because it is a common species in the Indo-Pacific region (Benayahu, 1990; Fabricius and Klumpp, 1995; Fox et al., 2003; Janes, 2013). We hypothesize that phosphate, in contrast to nitrate, will increase the resilience of X. umbellata to warming because its Symbiodiniaceae will not suffer from phosphate starvation (Wiedenmann et al., 2013; Rädecker et al., 2015; Thobor et al., 2022). We tested our hypothesis in a five-week laboratory experiment with two phases. First, corals were exposed to phosphate enrichment (1, 2, 8 µM PO43- addition) under ambient temperature (26°C) for two weeks. Second, ocean warming was simulated by a stepwise increase in water temperature from 26 to 32°C over the course of three weeks. To assess coral health in response to phosphate enrichment and/or warming, we measured ecophysiological parameters such as pulsation, mortality, Symbiodiniaceae density and their cellular chlorophyll a (Chl. a) content. By answering the research questions, our study aims to fill the knowledge gaps regarding the interactive effects of global and local factors on the eco-physiology of soft corals. Addressing these knowledge gaps will help to better understand phase shift dynamics and to predict the resilience of coral reefs in the future of the Anthropocene.
The study was conducted in the Marine Ecology Laboratory at the University of Bremen, Germany, in 2020. Xenia umbellata specimens used for the present study were collected in the northern Red Sea in 2017 and kept in a maintenance aquarium under controlled conditions. Prior to the experiment, clonal mother colonies were fragmented into 276 smaller colonies using a scalpel and attached to calcium carbonate plugs (AF Plug Rocks, Aquaforest, Poland) with rubber bands. After coral fragments healed and were firmly attached to the plugs, the rubber bands were removed. Colonies were transferred from the maintenance aquarium to a tower system, which consisted of 12 individual tanks (60 L). 23 colonies were placed onto grid plateaus in each tank. Three of the colonies per tank were allocated for the observational parameter pulsation. Three random colonies per tank were used to assess Symbiodiniaceae density and cellular Chl. a concentration by freezing one colony per tank at -20°C, pending further analysis, at three time points (day 0, 14, and 35). All colonies were used to observe mortality.
The 12 individual tanks were arranged in a three-level tower system with four tanks per level. Each tank contained ~ 40 L artificial seawater prepared from demineralized (DM) water and artificial sea salt (Zoo Mix, Tropic Marin, Switzerland). The tanks were separated into a technical part and an experimental part. The technical part contained a thermostat (3613 aquarium heater. 75 W220–240 V; EHEIM GmbH and Co. KG, Germany), temperature controller (Schego Thermostat TRD 1000 Art.-Nr. 112), recirculation pump (EHEIM CompactOn 1000 pump; EHEIM GmbH and Co. KG, Germany), and a temperature logger (HOBO pendant temp/light, Onset, USA). The experimental part contained X. umbellata colonies on polycarbonate grid plateaus and a calcium carbonate sand layer of approx. 10 cm. The reef sand (grain size 0.5 – 1.2 mm) (Orbit-Aquaristik, Germany) was transferred into the tanks 5 weeks before the start of the experiment, so that a microcosm with microbial activity could develop. Two light emitting diode (LED) lights (Royal Blue—matrix module and Ultra Blue White 1:1—matrix module, WALTRON daytime® LED light, Germany) were adjusted above each tank. LED lights operated with a light intensity of 109.8 ± 12.1 µmol quanta m-2 s-1, which was measured in photosynthetically active radiation (PAR) using a LI-1400 Data Logger (LI-COR Biosciences, Germany), in a day-night rhythm of 12-12 h (Table 1).
Table 1 Mean values (± SD) of water quality parameters maintained in the tower system throughout the experiment (excluding PO43- concentrations and temperature).
During an acclimation phase of 14 days, experimental tanks stayed connected to the maintenance aquarium (~ 24°C). Afterwards, the tower system was disconnected from the main system and tanks were separated at the start of the experiment (day 0) to ensure equal starting water conditions across treatments. Further, the temperature in each tank was increased to ~ 26°C (1°C per day) to commensurate experimental conditions of previous studies (Vollstedt et al., 2020; Thobor et al., 2022). 10% of the artificial seawater was replaced daily to maintain a high water renewal rate in each tank. Temperature, salinity, pH, and dissolved oxygen were measured daily with a digital multimeter (Hach HQ40D portable multi meter, United States) and chemical water quality parameters were monitored twice a week (Table 1, Supplementary Table S1). Phosphate (PO43-) concentrations were measured daily using a photometer (Turner Designs Trilogy Laboratory Fluorometer). To this end, we adjusted the protocol of a commercially available phosphate test kit for salt water (TESTLAB MARIN, JBL, Germany) by quantifying weights and volumes of reagents and by creating a calibration curve (R2 = 0.97). Phosphate concentrations were manually adjusted using a 1 M stock solution of disodium hydrogen phosphate (purity ≥ 98%, Sigma-Aldrich 71645). Coral colonies were fed ~ 0.1 g dried marine plankton (150 – 200 µM) (Reef-Roids, Polyp Lab, USA) per tank two times per week to sustain previous maintenance conditions and avoid stress due to starvation. Tanks and grid plateaus were cleaned once a week.
Xenia umbellata was exposed to three different phosphate treatments. Three tanks served as controls in which colonies were exposed to very low phosphate concentrations (< 0.2 µM), simulating natural phosphate loads in oligotrophic reefs (Crossland et al., 1984; Koop et al., 2001; Rosset et al., 2017). Further, three different phosphate enrichment treatments were maintained in three tanks each by daily additions of disodium hydrogen phosphate (purity ≥ 98%, Sigma-Aldrich 71645). Phosphate treatments were adjusted to low (1 µM), medium (2 µM), and high (8 µM) concentrations which are comparable to previous phosphate experiments with corals and human-induced in situ conditions in coastal waters (Ferrier-Pagès et al., 2000; Koop et al., 2001; Cruz-Piñón et al., 2003; Renegar and Riegl, 2005; Dunn et al., 2012; Baum et al., 2016; van der Wulp et al., 2016; Al-Farawati et al., 2019).
Phosphate concentrations were measured daily in all tanks to maintain the different phosphate treatments as described before. A photometric o-phosphate determination method was applied by using JBL ProAqua Phosphat Test-Kits and the resulting change in coloration was measured with a calibrated photometer (Turner Designs Trilogy Laboratory Fluorometer). Subsequently, the amount of phosphate needed to be added to maintain phosphate treatments was adjusted. The first phosphate addition was carried out after the recording of baseline values (day 0). Afterwards, phosphate addition was administered every afternoon.
During the first experimental phase (day 1 – 14), the water temperature was kept stable at 26°C in all tanks. During the second experimental phase (day 15 – 35), the temperature was increased stepwise by 2°C over three days (1°C per day) and then kept stable for four days. This procedure was repeated until the final water temperature of 32°C was reached. The selected temperature treatment was based on the experimental design of previous studies investigating the combined effect of organic/inorganic eutrophication and ocean warming on X. umbellata (Vollstedt et al., 2020; Thobor et al., 2022).
Pulsation and mortality of X. umbellata colonies were monitored once a week throughout the experiment. Pulsations of three randomly selected polyps from three marked colonies per experimental tank were counted following the method developed by Vollstedt et al. (2020). Polyp pulsations were assessed in the morning to avoid the effects of circadian rhythms and possible disturbances due to other measurements and before the addition of coral food. Pulsations were counted for 30 sec. and then standardized to one minute as a comparative unit (beats min-1). Only whole tentacle contractions of a polyp (open – fully closed – open) passed as a pulsation and were counted. Mean rates were calculated for each colony and subsequently for each tank, resulting in three true replicates per treatment.
Mortality was assessed for all X. umbellata colonies by counting the number of colonies alive or dead in each tank. In case of uncertainty, colonies were observed for polyp pulsation or tissue necrosis. If no reaction of polyps in combination with tissue necrosis was observed, colonies were determined to be dead and removed from the tank.
Sample processing and normalization metrics for Symbiodiniaceae analysis in soft corals followed the methods recommended by Pupier et al. (2018). One colony per tank was randomly selected at the start of the experiment (day 0) and the end of the first and second experimental phase (day 14, day 35), resulting in three temporal samples and replicates per treatment. Colonies were removed from their coral plugs, rinsed with DM water, and frozen at -20°C until further analysis.
At the end of the five-week experiment, all samples were freeze-dried at -60°C for 24 h and stored under dry and dark conditions. The dry weight (DW) of the colonies was measured and used as a normalization metric. All samples were then homogenized in 10 mL DM water by using a hand tissue grinder (10 mL glass Potter-Elvehjem). Afterwards, two subsamples were transferred into 2 mL Eppendorf cups to analyze Symbiodiniaceae densities and Chl. a concentrations within 24 h. Subsamples were centrifuged for 10 min, supernatants were discarded, and pellets were re-suspended in DM water. Subsamples were centrifuged again for 10 min and supernatants were discarded.
Pellets of the subsamples for the Symbiodiniaceae density counts were re-suspended in 2 mL DM water. After samples were thoroughly mixed, 10 µL of the sample was transferred onto two hemocytometers (Improved Neubauer counting chamber, depth 0.1 mm), allowing for two replicate counts per sample. To obtain Symbiodiniaceae densities, the hemocytometer counting method as described by LeGresley and McDermott (2010) was applied. Consequently, counts were adjusted to the total initial sample volume of 10 mL to calculate the algal cell density of the colony. The mean algal cell density per tank, obtained from the two replicate counts, was then normalized to host DW. In total, three replicates per treatment were obtained.
Pellets of the Chl. a subsamples were re-suspended in 2 mL 100% acetone to extract chlorophyll from Symbiodiniaceae, and stored in darkness for 24 h at 4°C. Following this, samples were centrifuged for 5 min and then transferred into two quartz cuvettes, allowing for two replicate readings per sample. The determination of Chl. a concentrations followed the method described by Jeffrey and Humphrey (1975) which is used for the spectrophotometric determination of Chl. a in dinoflagellates. Chl. a concentrations were measured at two fixed wavelengths (663 nm and 630 nm) using a UV-Spectrophotometer (GENESYS 150, Fisher Scientific, Germany). Resulting concentrations were standardized to host DW and subsequently to Symbiodiniaceae density to calculate the mean cellular Chl. a concentration per algal cell. In total, three replicates per treatment were obtained. All Chl. a analysis steps were conducted under minimal light exposure.
The statistical analysis was carried out in R version 3.6.1 (R Core Team, 2019) using the packages tidyverse, ggplot2, ggpubr, and rstatix (Wickham, 2016; Wickham et al., 2019; Kassambara, 2020; Kassambara, 2021). Parametric data obtained from repeated measurements (i.e., pulsation) was analyzed by using a 2-way mixed ANOVA to test for significant differences between “days” as within-subject factors and “treatments” as between-subject factor. To check assumptions, normality was tested with the Shapiro-Wilk test, homogeneity of variance was confirmed by the Levene´s test, homogeneity of covariance was tested with the Box´s M-test, and sphericity was assessed by the Mauchly´s test. Randomly collected parametric data (Symbiodiniaceae density counts and cellular Chl. a concentrations) was analyzed by using a two-way ANOVA. To check assumptions, normality was tested with the Shapiro-Wilk test and homogeneity of variance was confirmed by the Levene´s test. In order to normalize the cellular Chl. a data, a log10 transformation was applied. No outliers were identified using the rstatix package. For post-hoc analysis, pairwise comparisons (pwc) with Bonferroni adjustment were applied. A pairwise t-test was used for parametric data. Results in text, figures, and tables were represented as means ± standard error of the mean (SEM) and differences were considered as statistically significant at p < 0.05.
During the first experimental phase, the two week exposure to phosphate enrichment did not significantly affect any of the investigated ecophysiological parameters of X. umbellata. Colonies exposed to different phosphate treatments did not display significant differences in pulsation (two-way mixed ANOVA, F (3, 8) = 3.71, p = 0.06) (Figure 1, Supplementary Table S2). Yet, regardless of the phosphate treatment, average pulsation significantly increased from 36 ± 1.3 on day 0 (baseline value) to 43 ± 2.0 beats min -1 on day 14. At the end of the first experimental phase, no mortality of X. umbellata colonies was observed. Further, there were no statistically significant differences in Symbiodiniaceae densities (two-way ANOVA, F (3, 24) = 0.860, p = 0.48) (Figure 2, Supplementary Table S3) and cellular Chl. a concentrations in X. umbellata colonies (two-way ANOVA, F (3, 24) = 0.770, p = 0.52) (Figure 3, Supplementary Table S4).
Figure 1 Pulsation rates ± SEM of Xenia umbellata colonies (n = 3) kept at different phosphate concentrations (control < 0.2 μM; low ~ 1 μM; medium ~ 2 μm; high ~ 8 μM) and exposed to an artificially induced water temperature increase. Bars represent the mean of three replicates. Different capital letters above bars indicate significant differences between days and different letters within bars show significant differences within treatments over time (pwc, Bonferroni adjustment, pairwise t-test, p < 0.05).
Figure 2 Symbiodiniaceae density ± SEM of Xenia umbellata colonies (n = 3) kept at different phosphate concentrations (control < 0.2 μM; low ~ 1 μM; medium ~ 2 μm; high ~ 8 μM) and exposed to an artificially induced water temperature increase. Bars represent the mean of three replicates. Different capital letters above bars indicate significant differences between days and different letters within bars show significant differences within treatments over time (pwc, Bonferroni adjustment, t-test, p < 0.01).
Figure 3 Cellular chlorophyll a ± SEM of Xenia umbellata colonies (n = 3) kept at different phosphate concentrations (control < 0.2 μM; low ~ 1 μM; medium ~ 2 μm; high ~ 8 μM) and exposed to an artificially induced water temperature increase. Bars represent the mean of three replicates. Different capital letters above bars indicate significant differences between days and different letters within bars show significant differences within treatments over time (pwc, Bonferroni adjustment, pairwise t-test, p < 0.05).
Warming significantly affected pulsation of X. umbellata colonies (two-way mixed ANOVA, F (5, 10) = 49.464, p < 0.001), regardless of the phosphate treatment, by increasing average pulsation up to 30°C from day 0 to day 28 by 33% (pwc, Bonferroni adjustment, pairwise t-test, p < 0.001) (Figure 1, Supplementary Table S2). Temperatures exceeding 30°C led to a general significant decrease in pulsation in all treatments from day 28 to day 35 of 15% (pwc, Bonferroni adjustment, pairwise t-test, p < 0.001). Despite slight necrosis in some colonies (single polyp mortality), at the end of the experiment, all colonies survived. Warming caused a significant increase in average Symbiodiniaceae densities (two-way ANOVA, F (2, 24) = 7.996, p < 0.01), regardless of the phosphate treatment (Figure 2, Supplementary Table S3). Yet, the effect of warming alone was not significant in control colonies which showed an increase in Symbiodiniaceae densities by ~ 63%, possibly due to the low number of replicates (n = 3). Warming led to a general decrease in cellular Chl. a concentrations (two-way ANOVA, F (2, 24) = 12.927, p < 0.001) within all treatments (Figure 3, Supplementary Table S4). Nevertheless, at the end of the experiment, only Chl. a concentrations in control colonies exposed to warming alone significantly decreased by ~ 80% compared to baseline values (pwc, Bonferroni adjustment, t-test, p < 0.01).
Xenia umbellata colonies in the medium and high phosphate treatment significantly increased their pulsation rates when exposed to additional warming (Figure 1, Supplementary Table S2). The colonies exposed to medium phosphate enrichment and warming showed a significant increase in their pulsation by ~ 10% from day 7 to day 21 and ~ 44% from day 0 to day 28 (pwc, Bonferroni adjustment, pairwise t-test, p < 0.05). The colonies exposed to high phosphate enrichment and warming significantly increased their pulsation by ~ 18% from day 7 to day 28 (pwc, Bonferroni adjustment, pairwise t-test, p < 0.05). Further, these colonies showed a significant increase in pulsation of ~ 41% from day 0 to day 28 (pwc, Bonferroni adjustment, pairwise t-test, p < 0.01). Survival was not affected by the combined effect of phosphate enrichment and simulated ocean warming over a short period of three weeks. The Symbiodiniaceae density of colonies exposed to medium phosphate enrichment and warming was significantly increased by ~ 195% (pwc, Bonferroni adjustment, t-test, p < 0.01) (Figure 2, Supplementary Table S3). In contrast, warming from 26°C to 32°C caused an increase in Symbiodiniaceae densities of colonies exposed to low or high phosphate enrichment by ~ 109%, and ~ 31%, respectively. At the end of the experiment, Chl. a concentrations of colonies exposed to the combined factors of low, medium, and high phosphate enrichment and warming decreased by ~ 65%, ~ 55%, and ~ 30%, respectively, compared to baseline values (Figure 3, Supplementary Table S4).
Inorganic phosphate enrichment alone did not affect polyp pulsation in X. umbellata. Similar, Vollstedt et al. (2020) found that organic eutrophication in form of glucose had no effect on pulsation in the same species. However, a significant increase in pulsation was observed during the first week of the experiment in the present study. While baseline values were measured shortly after the tower system was disconnected from the maintenance aquarium (~ 24°C), the temperature in the tower system was increased to ~ 26°C to commensurate experimental conditions of previous studies (Vollstedt et al., 2020; Thobor et al., 2022), possibly leading to the increase in pulsation. Pulsation in this study was comparable to observations in another laboratory experiment with X. umbellata at ambient temperatures of 26 – 28°C (Vollstedt et al., 2020) and were similar to in situ pulsation rates of Heteroxenia fucscescens, another pulsating soft coral in the family Xeniidae, in the Red Sea (Cohen et al., 1977; Kremien et al., 2013). While inorganic phosphate enrichment does not suggest a disturbance of pulsation in X. umbellata, other studies discovered that exposure to excess concentrations of inorganic ions (potassium, sodium, magnesium, calcium), crude oil, or a chemical dispersant caused reduced pulsation or paralysis in xeniid soft corals (Horridge, 1956; Cohen et al., 1977; Studivan et al., 2015). Horridge (1956) suggested that the excess of certain inorganic ions caused alterations of the polarization of excitable membranes in Heteroxenia affecting pulsation. Studivan et al. (2015), in comparison, hypothesized that reduced pulsation in Xenia elongata after the exposure to a chemical dispersant may be related to reduced photosynthetic rates of Symbiodiniaceae or increased coral respiration. This, in turn, alleviates the necessity of pulsation behavior to facilitate rapid oxygen diffusion on the coral surface.
Soft coral colonies did not show any signs of mortality in the present study due to inorganic phosphate enrichment at ambient temperatures. In contrast, phosphate enrichment can cause increased mortality (Walker and Ormond, 1982), reduced coral growth (Kinsey and Davies, 1979; Renegar and Riegl, 2005), and altered skeletogenesis (Simkiss, 1964; Koop et al., 2001) in hard corals as a result of reduced light availability due to nutrient-stimulated phytoplankton growth, increased competition with macroalgae, and inhibited calcification. Yet, phosphate enrichment can also lead to enhanced hard coral growth at the cost of a significant reduction in skeletal density and an increased porosity, increasing the susceptibility of hard corals to breakage by natural events or colonization by internal bioeroders (Risk et al., 1995; Koop et al., 2001; Dunn et al., 2012). While soft corals lack a calcium carbonate skeleton, they do produce internal sclerites of calcium carbonate for the structural support of colonies and protection against predation (Van Alstyne et al., 1992; West, 1997). So far, only a single study by McCauley and Goulet (2019) investigated the effect of phosphate enrichment (4 µM) on sclerites in two gorgonians, Pseudoplexaura porosa and Eunicea tourneforti, and found that sclerite content and their isotopic signatures were not affected. Considering that the effect of phosphate enrichment seems to be highly species specific in hard corals, it is sensible that future studies investigate the effect of phosphate enrichment on sclerites in pulsating Xeniidae and other soft coral species.
After 14 days, phosphate enrichment alone did not cause significant differences in Symbiodiniaceae density and cellular Chl. a concentration, suggesting no disruption of the symbiotic relationship between Symbiodiniaceae and X. umbellata. In contrast, Bednarz et al. (2012) observed that Chl. a tissue content in Xenia sp. significantly increased after a 4 week exposure to phosphate enrichment (2 µM). Similar observations have been made in hard coral studies, where phosphate enrichment over time periods of 4 to 58 weeks resulted in significantly increased Symbiodiniaceae densities and Chl. a tissue contents (Steven and Broadbent, 1997; Bucher and Harrison, 2002; Godinot et al., 2011a). In comparison to the present study, these corals were exposed to phosphate enrichment over longer periods which could explain the different results, suggesting that phosphate may not have an immediate effect on Symbiodiniaceae. In addition, these studies measured Chl. a tissue content, not cellular Chl. a concentrations as in the present study. Another possibility could be that Symbiodiniaceae in the present study stored inorganic phosphate as a precaution measure in case of reduced heterotrophy rather than using it for population density growth or increasing cellular Chl. a concentrations. This is supported by previous studies that found Symbiodiniaceae acting as a sink of inorganic nutrients within the symbiotic association and actively controlling uptake rates depending on the organic and inorganic feeding history of the coral host (Grover et al., 2002; Grover et al., 2003; Godinot et al., 2009). For instance, phosphate uptake rates by Symbiodiniaceae were significantly increased in starved corals and anemones (Muller-Parker et al., 1990; Grover et al., 2002; Godinot et al., 2009).
Across all three phosphate treatments and controls, pulsation increased with warming up to a temperature of 30°C. Specifically, colonies exposed to medium and high phosphate enrichment under warming significantly increased their pulsation by 44% and 41%, respectively, compared to their baseline values. However, temperatures exceeding 30°C led to an overall decrease in pulsation. These findings suggest that phosphate enrichment may support X. umbellata to increase its pulsation under warming to a certain thermal threshold. The continuous pulsation can benefit the coral in several ways by promoting water movement around the colony, fast removal of excess oxygen at the coral surface, and preventing the refiltration of water by neighboring polyps (Kremien et al., 2013; Wild and Naumann, 2013). This, in turn, enhances the coral´s photosynthesis and food supply with particulate and dissolved organic matter (Kremien et al., 2013). The significantly increased pulsation observed in colonies exposed to phosphate enrichment under warming may enhance the heterotrophic feeding ability of X. umbellata and thus may support retaining necessary energy levels to withstand thermal stress. Further, increased pulsation rates may help to mitigate high water temperatures at the coral surface and may reduce their thermal bleaching susceptibility. In comparison, colonies exposed to organic eutrophication maintained but did not increase their pulsation under warming (Vollstedt et al., 2020). Overall, pulsating xeniid soft corals could be considered bioindicators by using pulsation rate as an easily detectable, non-invasive, and inexpensive early warning indicator for changes in water quality and ocean warming (sensu Vollstedt et al., 2020).
Throughout the experiment, no mortality was observed in colonies exposed to phosphate enrichment and/or warming. Hence, X. umbellata generally displayed a high resistance towards ocean warming. However, previous work predicted Xenia spp. to be more susceptible to rising ocean temperatures than other Octocorallia in the Great Barrier Reef (Strychar et al., 2005; Sammarco and Strychar, 2013). One possible explanation for the high thermal tolerance of X. umbellata in our study could be their origination from the northern Red Sea. Studies discovered that hard corals are generally more thermal tolerant in the northern Red Sea (Fine et al., 2013; Osman et al., 2018), which may also apply to soft coral species. Another explanation could be the short exposure time (three weeks) of colonies to simulated ocean warming and thus may not be reflective of true thermal stress leading to colony mortality in this species. Yet, Vollstedt et al. (2020) observed a 30% mortality rate of colonies with increasing temperatures up to 32°C after three weeks that were not exposed to glucose enrichment (low (10 mg/L), medium (20 mg/L), high (40 mg/L)). As our study used a similar design to simulate ocean warming from 26 to 32°C over the course of three weeks as Vollstedt et al. (2020), the finding of Vollstedt et al. (2020) is contradictory to the observation in the present phosphate enrichment experiment, where warming alone did not result in colony mortality. An explanation could be that corals exposed to warming alone in the present experiment were able to maintain survival due to supplemental feed as an additional energy resource. Vollstedt et al. (2020) hypothesized that X. umbellata can switch from photoautotrophy to mixotrophy, if necessary. Further, Hughes and Grottoli (2013) hypothesized that heterotrophic compensation in corals could be a sign of resilience towards ocean warming. Heterotrophy could supply the coral holobiont with additional energy resources and may help the coral host to maintain functions, especially when experiencing autotrophic disruption (Borell and Bischof, 2008; Wooldridge, 2013). However, Ezzat et al. (2019) points out that a shift towards greater heterotrophy under thermal stress requires a minimum of autotrophically acquired inorganic nutrients to be functional.
Warming significantly increased Symbiodiniaceae densities while cellular Chl. a contents were significantly decreased, regardless of the phosphate treatment. Increases in Symbiodiniaceae densities can be observed when Symbiodiniaceae are no longer nutrient restricted, especially when they are not nitrogen limited (Muscatine et al., 1989; Marubini and Davies, 1996; Ezzat et al., 2015). While Symbiodiniaceae were not significantly affected by phosphate enrichment in the present study, higher Symbiodiniaceae densities could have been caused by increased dinitrogen fixation due to higher diazotroph activity because of warming (Rädecker et al., 2015; Pogoreutz et al., 2017; Tilstra et al., 2019). Lesser et al. (2007) observed that Symbiodiniaceae are the primary users of the dinitrogen fixation products of diazotrophs, implying that this process is important for primary production. However, Rädecker et al. (2021) observed that heat stress reduces the contribution of diazotrophs to coral holobiont nitrogen cycling. They found that in the coral Stylophora pistillata, even though dinitrogen fixation rates were increased under warming, the additional fixed nitrogen was not assimilated by the coral tissue or the Symbiodiniaceae.
Phosphate plays a central role in the bleaching susceptibility of corals. Phosphate uptake rates can significantly increase while nitrate uptake rates significantly decrease in corals exposed to thermal stress (Godinot et al., 2011b; Ezzat et al., 2016). Corals required inorganic phosphate rather than nitrate during thermal stress to maintain their Symbiodiniaceae density and photosynthetic rate, as well as to enhance the translocation and retention of carbon within the host tissue (Ezzat et al., 2016). Therefore, phosphate starvation can destabilize the coral-algal symbioses, resulting in an increased bleaching susceptibility of corals (Wiedenmann et al., 2013; Rosset et al., 2017). Wiedenmann et al. (2013), for instance, observed that corals previously incubated at high N:P ratios (< 5 weeks) displayed signs of bleaching within 10 days when exposed to a stepwise increase in heat (up to 30°C) and light (up to 160 μmol m¬2 s¬1) stress due to phosphate starvation. Supporting results were observed by Thobor et al. (2022), who found a higher susceptibility of X. umbellata to ocean warming when previously exposed to nitrate enrichment (37 µM) for two weeks followed by a combined treatment of nitrate enrichment and a stepwise temperature increase from 26 to 32°C in three weeks. However, X. umbellata colonies in the present study were not exposed to high N:P ratios and thus should not suffer from phosphate starvation.
The increase in Symbiodiniaceae density was accompanied by a reduction in cellular Chl. a content. Similar results have been reported by Hoegh-Guldberg and Smith (1989), who observed that cellular Chl. a concentrations in Symbiodiniaceae of the coral Seriatopora hystrix decreased while Symbiodiniaceae population densities increased. They hypothesized that this was the result of reduced inorganic nitrogen availability to Symbiodiniaceae at high population densities. Reduced chlorophyll content due to reduced inorganic nitrogen availability has also previously been observed for marine algae (Falkowski and Owens, 1980; Dawes et al., 1984; Granéli and Sundbäck, 1985). In addition, Béraud et al. (2013) showed that heat stress in combination with reduced nitrogen availability resulted in reduced cellular Chl. a concentrations in the scleractinian coral Turbinaria reniformis. Other studies observed that the chlorophyll content of the sea anemone Aiptasia pallida decreased as the host was starved (Cook et al., 1988). Hence, reduced cellular Chl. a contents in the present study could be explained by either reduced inorganic nitrogen availability per algal cell, reduced heterotrophy, thermal stress of Symbiodiniaceae, or a combination of all these factors.
Overall, X. umbellata displayed a high resilience towards phosphate enrichment and/or ocean warming. This, in turn, could further increase their dominance over hard corals on future reefs. Soft corals, in general, have several competitive advantages against hard corals due to their high fecundity, multiple dispersal modes, chemical defense mechanisms, and their higher resistance to ocean warming and acidification (Benayahu and Loya, 1985; Fox et al., 2003; Inoue et al., 2013; Wild and Naumann, 2013). Shifts from hard to soft coral dominated reefs will have major implications for ecosystem functions as soft corals provide less structural complexity than hard corals, even though soft corals may still provide a suitable habitat for many fish species (Epstein and Kingsford, 2019; Hall-Spencer and Harvey, 2019). Xeniids, for instance, were already associated with shifts from hard to soft coral dominated reefs after blast fishing events in the Komodo National Park in Indonesia and an outbreak of the corallivore Acanthaster planci in the South Sinai region of the Red Sea (Fox et al., 2003; Tilot et al., 2008). In addition, Xenia displayed higher abundances at sites exposed to sedimentation and wastewater discharges compared to unimpacted sites in the Red Sea (Ziegler et al., 2016).
Our findings suggest that inorganic phosphate enrichment enhanced the resilience of the pulsating soft coral X. umbellata towards ocean warming by enabling them to increase their pulsation. Although X. umbellata may be more resistant towards phosphate enrichment in a warming ocean compared to hard corals, they are still restricted in their capability to deal with eutrophication. Inorganic nitrate enrichment, for instance, reduced the resilience of X. umbellata towards ocean warming, causing reduced pulsation, darkening of colonies, and high rates of partial as well as whole-colony mortality (Thobor et al., 2022). Considering that human activities will likely further increase nutrient levels in coastal ecosystems, a continued degradation of hard and soft coral communities under ocean warming is likely, shifting tropical reefs towards a macro- and turf algae dominated state, which often benefit from eutrophication (Naumann et al., 2015; Adam et al., 2020; Karcher et al., 2020). Thus, our findings support the conservation strategy of strengthening coral resilience towards climate change by addressing local factors such as inorganic eutrophication through regional management measures (Kuffner and Paul, 2001; Wooldridge and Done, 2009; D’Angelo and Wiedenmann, 2014). It is of great importance to not only control nutrient inputs into coastal systems, but also to consider managing N:P ratios directly as imbalanced ratios have been found to be especially harmful to corals (Wiedenmann et al., 2013; Ezzat et al., 2016; Rosset et al., 2017). A starting point to reach favorable N:P ratios is to focus on local nutrient profiles, adjusting agriculture and tertiary wastewater-treatment practices, and to evaluate whether the reduction of nitrate or phosphate or both nutrients is the most effective to promote reef resilience (Conley et al., 2009; Wiedenmann et al., 2013; D’Angelo and Wiedenmann, 2014).
The original contributions presented in the study are included in the article/Supplementary Material. Further inquiries can be directed to the corresponding author.
AK, SM, AT, BT, and CW conceptualized the study. AK and SM conducted the experiment. AK analyzed the samples and the data and wrote the manuscript. CW acquired the funding and supervised the study. All authors revised drafts of the manuscript. All authors contributed to the article and approved the submitted version.
The study was funded by resources of the Marine Ecology Department of University of Bremen, Germany, and the DFG grant Wi 2677/16-1.
We want to thank Daisy Ruhlmann for supporting us in daily marine aquaria maintenance activities during the experiment.
The authors declare that the research was conducted in the absence of any commercial or financial relationships that could be construed as a potential conflict of interest.
All claims expressed in this article are solely those of the authors and do not necessarily represent those of their affiliated organizations, or those of the publisher, the editors and the reviewers. Any product that may be evaluated in this article, or claim that may be made by its manufacturer, is not guaranteed or endorsed by the publisher.
The Supplementary Material for this article can be found online at: https://www.frontiersin.org/articles/10.3389/fmars.2022.1026321/full#supplementary-material
Adam T., Burkepile D., Holbrook S., Carpenter R., Claudet J., Loiseau C., et al. (2020). Landscape-scale patterns of nutrient enrichment in a coral reef ecosystem: implications for coral to algae phase shifts. Ecol. applications: Publ. Ecol. Soc. America 31, e2227. doi: 10.1002/eap.2227
Al-Farawati R., El Sayed M. A. K., Rasul N. M. A. (2019). Nitrogen, phosphorus and organic carbon in the Saudi Arabian red Sea coastal waters: Behaviour and human impact. oceanographic and biological aspects of the red Sea springer oceanography. Eds. Rasul N. M. A., Stewart I. C. F. (Cham: Springer International Publishing), 89–104. doi: 10.1007/978-3-319-99417-8_5
Baker D., Freeman C., Wong J. C. Y., Fogel M., Knowlton N. (2018). Climate change promotes parasitism in a coral symbiosis. ISME J. 12, 921–930. doi: 10.1038/s41396-018-0046-8
Ban S. S., Graham N. A. J., Connolly S. R. (2014). Evidence for multiple stressor interactions and effects on coral reefs. Global Change Biol. 20, 681–697. doi: 10.1111/gcb.12453
Baum G., Januar I., Ferse S. C. A., Wild C., Kunzmann A. (2016). Abundance and physiology of dominant soft corals linked to water quality in Jakarta bay, Indonesia. PeerJ 4, e2625. doi: 10.7717/peerj.2625
Bednarz V. N., Cardini U., van Hoytema N., Al-Rshaidat M. M. D., Wild C. (2015). Seasonal variation in dinitrogen fixation and oxygen fluxes associated with two dominant zooxanthellate soft corals from the northern red Sea. Mar. Ecol. Prog. Ser. 519, 141–152. doi: 10.3354/meps11091
Bednarz V. N., Naumann M. S., Niggl W., Wild C. (2012). Inorganic nutrient availability affects organic matter fluxes and metabolic activity in the soft coral genus. Xenia J. Exp. Biol. 215 (20), 3672–3679. doi: 10.1242/jeb.072884
Bell P. R. F. (1992). Eutrophication and coral reefs–some examples in the great barrier reef lagoon. Water Res. 26, 553–568. doi: 10.1016/0043-1354(92)90228-V
Benayahu Y. (1990). Xeniidae (Cnidaria: Octocorallia) from the red Sea, with the description of a new species. Zoologische mededelingen 64, 113–120.
Benayahu Y., Loya Y. (1985). Settlement and recruitment of a soft coral: why is Xenia macrospiculata a successful colonizer? Bull. Mar. Sci. 36, 177–188.
Béraud E., Gevaert F., Rottier C., Ferrier-Pagès C. (2013). The response of the scleractinian coral Turbinaria reniformis to thermal stress depends on the nitrogen status of the coral holobiont. J. Exp. Biol. 216, 2665–2674. doi: 10.1242/jeb.085183
Borell E. M., Bischof K. (2008). Feeding sustains photosynthetic quantum yield of a scleractinian coral during thermal stress. Oecologia 157, 593–601. doi: 10.1007/s00442-008-1102-2
Brown B. E. (1997). Coral bleaching: causes and consequences. Coral Reefs 16, S129–S138. doi: 10.1007/s003380050249
Bucher D. J., Harrison P. L. (2002). “Growth response of the reef coral Acropora longicyathus to elevated inorganic nutrients: do responses to nutrients vary among coral taxa,” in In Proceedings of the 9th International Coral Reef Symposium, Bali, (Indonesia: Ministry of Environment) 1, 443–448.
Cannon S. E., Aram E., Beiateuea T., Kiareti A., Peter M., Donner S. D. (2021). Coral reefs in the Gilbert islands of Kiribati: Resistance, resilience, and recovery after more than a decade of multiple stressors. PloS One 16, e0255304. doi: 10.1371/journal.pone.0255304
Changyun W., Haiyan L., Changlun S., Yanan W., Liang L., Huashi G. (2008). Chemical defensive substances of soft corals and gorgonians. Acta Ecologica Sin. 28, 2320–2328. doi: 10.1016/S1872-2032(08)60048-7
Cohen Y., Nissenbaum A., Eisler R. (1977). “Effects of Iranian crude oil on the red Sea octocoral heteroxenia fuscescens,” in Environmental pollution, vol. 12. (London: United Kingdom).
Conley D. J., Paerl H. W., Howarth R. W., Boesch D. F., Seitzinger S. P., Havens K. E., et al. (2009). Controlling eutrophication: Nitrogen and phosphorus. Science 323, 1014–1015. doi: 10.1126/science.1167755
Connell J. H. (1978). Diversity in tropical rain forests and coral reefs. Science 199, 1302–1310. doi: 10.1126/science.199.4335.1302
Cook C. B., D’Elia C. F., Muller-Parker G. (1988). Host feeding and nutrient sufficiency for zooxanthellae in the sea anemone Aiptasia pallida. Mar. Biol. 98, 253–262. doi: 10.1007/BF00391203
Crossland C., Hatcher B., Atkinson M., Smith S. (1984). Dissolved nutrients of a high-latitude coral reef, houtman abrolhos islands, Western Australia. Mar. Ecol. Prog. Ser. 14, 159–163. doi: 10.3354/meps014159
Cruz-Piñón G., Carricart-Ganivet J. P., Espinoza-Avalos J. (2003). Monthly skeletal extension rates of the hermatypic corals Montastraea annularis and Montastraea faveolata: biological and environmental controls. Mar. Biol. 143, 491–500. doi: 10.1007/s00227-003-1127-3
Cziesielski M. J., Schmidt-Roach S., Aranda M. (2019). The past, present, and future of coral heat stress studies. Ecol. Evol. 9, 10055–10066. doi: 10.1002/ece3.5576
D’Angelo C., Wiedenmann J. (2014). Impacts of nutrient enrichment on coral reefs: new perspectives and implications for coastal management and reef survival. Curr. Opin. Environ. Sustainability 7, 82–93. doi: 10.1016/j.cosust.2013.11.029
Dawes C., Chen c.-P., Jewett-Smith J., Marsh A., Watts S. (1984). Effect of phosphate and ammonium levels on photosynthetic and respiratory responses of the red alga Gracilaria verrucosa. Mar. Biol. 78, 325–328. doi: 10.1007/BF00393018
Done T. J. (1992). Phase shifts in coral reef communities and their ecological significance. Hydrobiologia 247, 121–132. doi: 10.1007/BF00008211
Dunn J. G., Sammarco P. W., LaFleur G. (2012). Effects of phosphate on growth and skeletal density in the scleractinian coral Acropora muricata: A controlled experimental approach. J. Exp. Mar. Biol. Ecol. 411, 34–44. doi: 10.1016/j.jembe.2011.10.013
Eddy T. D., Lam V. W. Y., Reygondeau G., Cisneros-Montemayor A. M., Greer K., Palomares M. L. D., et al. (2021). Global decline in capacity of coral reefs to provide ecosystem services. One Earth 4, 1278–1285. doi: 10.1016/j.oneear.2021.08.016
El-Khaled Y. C., Roth F., Rädecker N., Tilstra A., Karcher D. B., Kürten B., et al. (2021). Nitrogen fixation and denitrification activity differ between coral- and algae-dominated red Sea reefs. Sci. Rep. 11, 11820. doi: 10.1038/s41598-021-90204-8
Ellis J. I., Jamil T., Anlauf H., Coker D. J., Curdia J., Hewitt J., et al. (2019). Multiple stressor effects on coral reef ecosystems. Global Change Biol. 25, 4131–4146. doi: 10.1111/gcb.14819
Epstein H. E., Kingsford M. J. (2019). Are soft coral habitats unfavourable? a closer look at the association between reef fishes and their habitat. Environ. Biol. Fishes 102, 479–497. doi: 10.1007/s10641-019-0845-4
Ezzat L., Maguer J.-F., Grover R., Ferrier-Pagès C. (2015). New insights into carbon acquisition and exchanges within the coral–dinoflagellate symbiosis under NH4+ and NO3– supply. Proc. R. Soc. London B. Biol. Sci. 282, 20150610. doi: 10.1098/rspb.2015.0610
Ezzat L., Maguer J.-F., Grover R., Ferrier-Pagès C. (2016). Limited phosphorus availability is the Achilles heel of tropical reef corals in a warming ocean. Sci. Rep. 6, 31768. doi: 10.1038/srep31768
Ezzat L., Maguer J.-F., Grover R., Rottier C., Tremblay P., Ferrier-Pagès C. (2019). Nutrient starvation impairs the trophic plasticity of reef-building corals under ocean warming. Funct. Ecol. 33, 643–653. doi: 10.1111/1365-2435.13285
Fabricius K. E., Cséke S., Humphrey C., De’ath G. (2013). Does trophic status enhance or reduce the thermal tolerance of scleractinian corals? a review, experiment and conceptual framework. PLoS One 8, e54399. doi: 10.1371/journal.pone.0054399
Fabricius K., Dommisse M. (2000). Depletion of suspended particulate matter over coastal reef communities dominated by zooxanthellate soft corals. Mar. Ecol. Prog. Ser. 196, 157–167. doi: 10.3354/meps196157
Fabricius K., Klumpp D. (1995). Widespread mixotrophy in reef-inhabiting soft corals: the influence of depth, and colony expansion and contraction on photosynthesis. Mar. Ecol. Prog. Ser. 125, 195–204. doi: 10.3354/meps125195
Falkowski P. G., Owens T. G. (1980). Light–shade adaptation 1. Plant Physiol. 66, 592–595. doi: 10.1104/pp.66.4.592
Fernandes de Barros Marangoni L., Ferrier-Pagès C., Rottier C., Bianchini A., Grover R. (2020). Unravelling the different causes of nitrate and ammonium effects on coral bleaching. Sci. Rep. 10, 11975. doi: 10.1038/s41598-020-68916-0
Ferrier-Pagès C., Gattuso J.-P., Dallot S., Jaubert J. (2000). Effect of nutrient enrichment on growth and photosynthesis of the zooxanthellate coral Stylophora pistillata. Coral Reefs 19, 103–113. doi: 10.1007/s003380000078
Fine M., Gildor H., Genin A. (2013). A coral reef refuge in the red Sea. Global Change Biol. 19, 3640–3647. doi: 10.1111/gcb.12356
Fitt W. K., Spero H. J., Halas J., White M. W., Porter J. W. (1993). Recovery of the coral Montastrea annularis in the Florida keys after the 1987 Caribbean “bleaching event”. Coral Reefs 12, 57–64. doi: 10.1007/BF00302102
Fox H. E., Pet J. S., Dahuri R., Caldwell R. L. (2003). Recovery in rubble fields: long-term impacts of blast fishing. Mar. pollut. Bull. 46, 1024–1031. doi: 10.1016/S0025-326X(03)00246-7
Godinot C., Ferrier-Pagés C., Grover R. (2009). Control of phosphate uptake by zooxanthellae and host cells in the scleractinian coral Stylophora pistillata. Limnol. Oceanogr. 54, 1627–1633. doi: 10.4319/lo.2009.54.5.1627
Godinot C., Ferrier-Pagès C., Montagna P., Grover R. (2011a). Tissue and skeletal changes in the scleractinian coral Stylophora pistillata esper 1797 under phosphate enrichment. J. Exp. Mar. Biol. Ecol. 409, 200–207. doi: 10.1016/j.jembe.2011.08.022
Godinot C., Houlbrèque F., Grover R., Ferrier-Pagès C. (2011b). Coral uptake of inorganic phosphorus and nitrogen negatively affected by simultaneous changes in temperature and pH. PLoS One 6, e25024. doi: 10.1371/journal.pone.0025024
Granéli E., Sundbäck K. (1985). The response of planktonic and microbenthic algal assemblages to nutrient enrichment in shallow coastal waters, southwest Sweden. J. Exp. Mar. Biol. Ecol. 85 (3), 253–268. doi: 10.1016/0022-0981(85)90161-3
Grover R., Maguer J.-F., Allemand D., Ferrier-Pagés C. (2003). Nitrate uptake in the scleractinian coral Stylophora pistillata. Limnol. Oceanogr. 48, 2266–2274. doi: 10.4319/lo.2003.48.6.2266
Grover R., Maguer J.-F., Reynaud-Vaganay S., Ferrier-Pagès C. (2002). Uptake of ammonium by the scleractinian coral Stylophora pistillata: Effect of feeding, light, and ammonium concentrations. Limnol. Oceanogr. 47, 782–790. doi: 10.4319/lo.2002.47.3.0782
Hall E. R., Muller E. M., Goulet T., Bellworthy J., Ritchie K. B., Fine M. (2018). Eutrophication may compromise the resilience of the red Sea coral Stylophora pistillata to global change. Mar. pollut. Bull. 131, 701–711. doi: 10.1016/j.marpolbul.2018.04.067
Hall-Spencer J. M., Harvey B. P. (2019). Ocean acidification impacts on coastal ecosystem services due to habitat degradation. Emerging Topics Life Sci. 3, 197–206. doi: 10.1042/ETLS20180117
Harborne A., Rogers A., Bozec Y.-M., Mumby P. (2016). Multiple stressors and the functioning of coral reefs. Annu. Rev. Mar. Sci. 9, 445–468. doi: 10.1146/annurev-marine-010816-060551
Hoegh-Guldberg O. (1999). Climate change, coral bleaching and the future of the world’s coral reefs. Mar. Freshw. Ressearch 50, 839–866. doi: 10.1071/MF99078
Hoegh-Guldberg O., Smith G. J. (1989). Influence of the population density of zooxanthellae and supply of ammonium on the biomass and metabolic characteristics of the reef corals Seriatopora hystrix and Stylophora pistillata. Mar. Ecol. Prog. Ser. 57, 173–186. doi: 10.3354/meps057173
Horridge G. A. (1956). The responses of Heteroxenia (Alcyonaria) to stimulation and to some inorganic ions. J. Exp. Biol 33, 604–614. doi: 10.1242/jeb.33.3.604
Hughes T. P., Anderson K. D., Connolly S. R., Heron S. F., Kerry J. T., Lough J. M., et al. (2018). Spatial and temporal patterns of mass bleaching of corals in the anthropocene. Science 359, 80–83. doi: 10.1126/science.aan8048
Hughes A. D., Grottoli A. G. (2013). Heterotrophic compensation: A possible mechanism for resilience of coral reefs to global warming or a sign of prolonged stress? PLoS One 8, e81172. doi: 10.1371/journal.pone.0081172
Hughes T. P., Kerry J. T., Álvarez-Noriega M., Álvarez-Romero J. G., Anderson K. D., Baird A. H., et al. (2017). Global warming and recurrent mass bleaching of corals. Nature 543, 373–377. doi: 10.1038/nature21707
Inoue S., Kayanne H., Yamamoto S., Kurihara H. (2013). Spatial community shift from hard to soft corals in acidified water. Nat. Climate Change 3, 683–687. doi: 10.1038/nclimate1855
Janes M. P. (2013). Distribution and diversity of the soft coral family xeniidae (Coelenterata: Octocorallia) in lembeh strait, Indonesia. Galaxea J. Coral Reef Stud. 15, 195–200. doi: 10.3755/galaxea.15.195
Jeffrey S. W., Humphrey G. F. (1975). New spectrophotometric equations for determining chlorophylls a, b, c1 and c2 in higher plants, algae and natural phytoplankton. Biochemie und Physiologie der Pflanzen 167, 191–194. doi: 10.1016/S0015-3796(17)30778-3
Jeng M.-S., Huang H. D., Dai C.-F., Hsiao Y.-C., Benayahu Y. (2011). Sclerite calcification and reef-building in the fleshy octocoral genus sinularia (Octocorallia: Alcyonacea). Coral Reefs 30, 925–933. doi: 10.1007/s00338-011-0765-z
Karcher D. B., Roth F., Carvalho S., El-Khaled Y. C., Tilstra A., Kürten B., et al. (2020). Nitrogen eutrophication particularly promotes turf algae in coral reefs of the central red Sea. PeerJ 8, e8737. doi: 10.7717/peerj.8737
Kassambara A. (2020) 'ggplot2' based publication ready plots. Available at: http://rpkgs.datanovia.com/ggpubr/index.html (Accessed August 15,2022).
Kassambara A. (2021) Rstatix package - r documentation. Available at: https://www.rdocumentation.org/packages/rstatix/versions/0.7.0 (Accessed August 15,2022).
Kinsey D. W., Davies P. J. (1979). Effects of elevated nitrogen and phosphorus on coral reef growth 1. Limnol. Oceanogr. 24, 935–940. doi: 10.4319/lo.1979.24.5.0935
Koop K., Booth D., Broadbent A., Brodie J., Bucher D., Capone D., et al. (2001). ENCORE: The effect of nutrient enrichment on coral reefs. synthesis of results and conclusions. Mar. pollut. Bull. 42, 91–120. doi: 10.1016/S0025-326X(00)00181-8
Kremien M., Shavit U., Mass T., Genin A. (2013). Benefit of pulsation in soft corals. Proc. Natl. Acad. Sci. 110, 8978–8983. doi: 10.1073/pnas.1301826110
Kuffner I. B., Paul V. J. (2001). Effects of nitrate, phosphate and iron on the growth of macroalgae and benthic cyanobacteria from Cocos lagoon, Guam. Mar. Ecol. Prog. Ser. 222, 63–72. doi: 10.3354/meps222063
LeGresley M., McDermott G. (2010). Counting chamber methods for quantitative phytoplankton analysis - haemocytometer, PalmerMaloney cell and Sedgewick-Rafter cell. UNESCO (IOC manuals and guides) 25–30.
Lesser M., Falcón L., Rodríguez-Román A., Enríquez S., Hoegh-Guldberg O., Iglesias-Prieto R, et al (2007). Nitrogen fixation by symbiotic cyanobacteria provides a source of nitrogen for the scleractinian coral Montastraea cavernosa. Mar. Ecol. Prog. Ser 346, 143–152. doi: 10.3354/meps07008
Lopes A. R., Faleiro F., Rosa I. C., Pimentel M. S., Trubenbach K., Repolho T., et al. (2018). Physiological resilience of a temperate soft coral to ocean warming and acidification. Cell Stress Chaperones 23, 1093–1100. doi: 10.1007/s12192-018-0919-9
Marubini F., Davies P. S. (1996). Nitrate increases zooxanthellae population density and reduces skeletogenesis in corals. Mar. Biol. 127, 319–328. doi: 10.1007/BF00942117
McCauley M., Goulet T. L. (2019). Caribbean Gorgonian octocorals cope with nutrient enrichment. Mar. pollut. Bull. 141, 621–628. doi: 10.1016/j.marpolbul.2019.02.067
McCook L. J. (1999). Macroalgae, nutrients and phase shifts on coral reefs: scientific issues and management consequences for the great barrier reef. Coral Reefs 18, 357–367. doi: 10.1007/s003380050213
McManus J. W., Polsenberg J. F. (2004). Coral–algal phase shifts on coral reefs: Ecological and environmental aspects. Prog. Oceanogr. 60, 263–279. doi: 10.1016/j.pocean.2004.02.014
Moberg F., Folke C. (1999). Ecological goods and services of coral reef ecosystems. Ecol. Economics 29, 215–233. doi: 10.1016/S0921-8009(99)00009-9
Muller-Parker G., Cook C., D’Elia C. (1990). Feeding affects phosphate fluxes in the symbiotic sea anemone Aiptasia pallida. Mar. Ecol. Prog. Ser. 60, 283–290. doi: 10.3354/meps060283
Muscatine L., Falkowski P. G., Dubinsky Z., Cook P. A., McCloskey L. R., Smith D. C. (1989). The effect of external nutrient resources on the population dynamics of zooxanthellae in a reef coral. Proc. R. Soc. London B. Biol. Sci. 236, 311–324. doi: 10.1098/rspb.1989.0025
Naumann M. S., Bednarz V. N., Ferse S. C. A., Niggl W., Wild C. (2015). Monitoring of coastal coral reefs near dahab (Gulf of aqaba, red Sea) indicates local eutrophication as potential cause for change in benthic communities. Environ. Monit. Assess. 187, 44. doi: 10.1007/s10661-014-4257-9
Norström A., Nyström M., Lokrantz J., Folke C. (2009). Alternative states on coral reefs: beyond coral–macroalgal phase shifts. Mar. Ecol. Prog. Ser. 376, 295–306. doi: 10.3354/meps07815
Osman E. O., Smith D. J., Ziegler M., Kürten B., Conrad C., El-Haddad K. M., et al. (2018). Thermal refugia against coral bleaching throughout the northern red Sea. Global Change Biol. 24, e474–e484. doi: 10.1111/gcb.13895
Pogoreutz C., Rädecker N., Cárdenas A., Gärdes A., Voolstra C. R., Wild C. (2017). Sugar enrichment provides evidence for a role of nitrogen fixation in coral bleaching. Global Change Biol. 23, 3838–3848. doi: 10.1111/gcb.13695
Pupier C. A., Bednarz V. N., Ferrier-Pagès C. (2018). Studies with soft corals – recommendations on sample processing and normalization metrics. Fronties Mar. Sci. 5, 348. doi: 10.3389/fmars.2018.00348
Rädecker N., Pogoreutz C., Gegner H. M., Cárdenas A., Roth F., Bougoure J., et al. (2021). Heat stress destabilizes symbiotic nutrient cycling in corals. PNAS 118, e2022653118. doi: 10.1073/pnas.2022653118
Rädecker N., Pogoreutz C., Voolstra C. R., Wiedenmann J., Wild C. (2015). Nitrogen cycling in corals: the key to understanding holobiont functioning? Trends Microbiol. 23, 490–497. doi: 10.1016/j.tim.2015.03.008
R Core Team (2019). R: A language and environment for statistical computing (Vienna, Austria: R Foundation for Statistical Computing). Available at: https://www.R-project.org/.
Renegar D. A., Riegl B. M. (2005). Effect of nutrient enrichment and elevated CO2 partial pressure on growth rate of Atlantic scleractinian coral Acropora cervicornis. Mar. Ecol. Prog. Ser. 293, 69–76. doi: 10.3354/meps293069
Risk M. J., Sammarco P. W., Edinger E. N. (1995). Bioerosion in Acropora across the continental shelf of the great barrier reef. Coral Reefs 14, 79–86. doi: 10.1007/BF00303427
Robinson D. E. (1971). Observations on Fijian coral reefs and the crown of thorns starfish. J. R. Soc. New Z. 1, 99–112. doi: 10.1080/03036758.1971.10419344
Rosset S., Wiedenmann J., Reed A. J., D’Angelo C. (2017). Phosphate deficiency promotes coral bleaching and is reflected by the ultrastructure of symbiotic dinoflagellates. Mar. pollut. Bull. 118, 180–187. doi: 10.1016/j.marpolbul.2017.02.044
Sammarco P. W., Strychar K. B. (2013). Responses to high seawater temperatures in zooxanthellate octocorals. PloS One 8, e54989. doi: 10.1371/journal.pone.0054989
Simkiss K. (1964). Phosphates as crystal poisons of calcification. Biol. Rev. 39, 487–504. doi: 10.1111/j.1469-185X.1964.tb01166.x
Steinberg R. K., Dafforn K. A., Ainsworth T., Johnston E. L. (2020). Know thy anemone: A review of threats to octocorals and anemones and opportunities for their restoration. Front. Mar. Sci. 7. doi: 10.3389/fmars.2020.00590
Steven A. D. L., Broadbent A. D. (1997). “Growth and metabolic responses on Acropora palifera to long term nutrient enrichment,” in In Proceedings of the 8th International Coral Reef Symposium (Panama: University of Panama and the Smithsonian Tropical Research Institute) 1, 867–872.
Stobart B., Teleki K., Buckley R., Downing N., Callow M. (2005). Coral recovery at aldabra atoll, Seychelles: five years after the 1998 bleaching event. Philos. Trans. R. Soc. A 363, 251–255. doi: 10.1098/rsta.2004.1490
Strychar K., Coates M., Sammarco P., Piva T., Scott P. (2005). Loss of symbiodinium from bleached soft corals Sarcophyton ehrenbergi, Sinularia sp. and Xenia sp. J. Exp. Mar. Biol. Ecol. 320, 159–177. doi: 10.1016/j.jembe.2004.12.039
Studivan M. S., Hatch W. I., Mitchelmore C. L. (2015). Responses of the soft coral Xenia elongata following acute exposure to a chemical dispersant. SpringerPlus 4, 80. doi: 10.1186/s40064-015-0844-7
Thobor B., Tilstra A., Bourne D. G., Springer K., Mezger S. D., Struck U., et al. (2022). The pulsating soft coral Xenia umbellata shows high resistance to warming when nitrate concentrations are low. Sci. Rep. 12, 16788. doi: 10.1038/s41598-022-21110-w
Tilot V., Leujak W., Ormond R. F. G., Ashworth J. A., Mabrouk A. (2008). Monitoring of south Sinai coral reefs: influence of natural and anthropogenic factors. Aquat. Conservation: Mar. Freshw. Ecosyst. 18 (7), 1109–1126. doi: 10.1002/aqc.942
Tilstra A., Pogoreutz C., Rädecker N., Ziegler M., Wild C., Voolstra C. (2019). Relative diazotroph abundance in symbiotic red Sea corals decreases with water depth. Front. Mar. Sci. 6. doi: 10.3389/fmars.2019.00372
Van Alstyne K. L., Wylie C. R., Paul V. J., Meyer K. (1992). Antipredator defenses in tropical pacific soft corals (Coelenterata: Alcyonacea). i. sclerites as defenses against generalist carnivorous fishes. Biol. Bull. 182, 231–240. doi: 10.2307/1542116
van der Wulp S. A., Damar A., Ladwig N., Hesse K.-J. (2016). Numerical simulations of river discharges, nutrient flux and nutrient dispersal in Jakarta bay, Indonesia. Mar. pollut. Bull. 110, 675–685. doi: 10.1016/j.marpolbul.2016.05.015
Vollstedt S., Xiang N., Simancas-Giraldo S. M., Wild C. (2020). Organic eutrophication increases resistance of the pulsating soft coral Xenia umbellata to warming. PeerJ 8, e9182. doi: 10.7717/peerj.9182
Walker D. I., Ormond R. F. G. (1982). Coral death from sewage and phosphate pollution at aqaba, red Sea. Mar. pollut. Bull. 13, 21–25. doi: 10.1016/0025-326X(82)90492-1
West J. M. (1997). Plasticity in the sclerites of a gorgonian coral: Tests of water motion, light level, and damage cues. Biol. Bull. 192, 279–289. doi: 10.2307/1542721
Wickham H. (2016) ggplot2: Elegant graphics for data analysis (Springer-Verlag New York). Available at: https://ggplot2.tidyverse.org/ (Accessed August 15,2022).
Wickham H., Averick M., Bryan J., Chang W., McGowan L. D., François R., et al. (2019). Welcome to the tidyverse. J. Open Source Software 4, 1686. doi: 10.21105/joss.01686
Wiedenmann J., D’Angelo C., Smith E. G., Hunt A. N., Legiret F.-E., Postle A. D., et al. (2013). Nutrient enrichment can increase the susceptibility of reef corals to bleaching. Nat. Climate Change 3, 160–164. doi: 10.1038/nclimate1661
Wild C., Hoegh-Guldberg O., Naumann M. S., Colombo-Pallotta M. F., Ateweberhan M., Fitt W. K., et al. (2011). Climate change impedes scleractinian corals as primary reef ecosystem engineers. Mar. Freshw. Res. 62, 205. doi: 10.1071/MF10254
Wild C., Naumann M. S. (2013). Effect of active water movement on energy and nutrient acquisition in coral reef-associated benthic organisms. Proc. Natl. Acad. Sci. 110, 8767–8768. doi: 10.1073/pnas.1306839110
Wooldridge S. A., Done T. J. (2009). Improved water quality can ameliorate effects of climate change on corals. Ecol. Appl. 19 (6), 1492–1499. doi: 10.1890/08-0963.1
Wooldridge S. A (2013). Breakdown of the coral-algae symbiosis: towards formalising a linkage between warm-water bleaching thresholds and the growth rate of the intracellular zooxanthellae. Biogeosciences 10, 1647–1658. doi: 10.5194/bg-10-1647-2013
Zhao H., Yuan M., Strokal M., Wu H. C., Liu X., Murk A., et al. (2021). Impacts of nitrogen pollution on corals in the context of global climate change and potential strategies to conserve coral reefs. Sci. Total Environ. 774, 145017. doi: 10.1016/j.scitotenv.2021.145017
Keywords: multiple stressors, soft coral, global factor, local factor, ocean warming, eutrophication, phosphate, pulsation
Citation: Klinke A, Mezger SD, Thobor B, Tilstra A, El-Khaled YC and Wild C (2022) Phosphate enrichment increases the resilience of the pulsating soft coral Xenia umbellata to warming. Front. Mar. Sci. 9:1026321. doi: 10.3389/fmars.2022.1026321
Received: 23 August 2022; Accepted: 26 October 2022;
Published: 10 November 2022.
Edited by:
Viola Liebich, Bremen Society for Natural Sciences, GermanyReviewed by:
Stephane Roberty, University of Liège, BelgiumCopyright © 2022 Klinke, Mezger, Thobor, Tilstra, El-Khaled and Wild. This is an open-access article distributed under the terms of the Creative Commons Attribution License (CC BY). The use, distribution or reproduction in other forums is permitted, provided the original author(s) and the copyright owner(s) are credited and that the original publication in this journal is cited, in accordance with accepted academic practice. No use, distribution or reproduction is permitted which does not comply with these terms.
*Correspondence: Annabell Klinke, a2xpbmtlYW5uYWJlbGxAZ21haWwuY29t
Disclaimer: All claims expressed in this article are solely those of the authors and do not necessarily represent those of their affiliated organizations, or those of the publisher, the editors and the reviewers. Any product that may be evaluated in this article or claim that may be made by its manufacturer is not guaranteed or endorsed by the publisher.
Research integrity at Frontiers
Learn more about the work of our research integrity team to safeguard the quality of each article we publish.