- 1Center for Global Discovery and Conservation Science, Arizona State University, Hilo, HI, United States
- 2Department of Biology, University of Louisiana at Lafayette, Lafayette, LA, United States
- 3Division of Aquatic Resources, Department of Land and Natural Resources, Kailua-Kona, HI, United States
- 4Marine Science Department, University of Hawai’i at Hilo, Hilo, HI, United States
The recently described crustose calcifying red algal species Ramicrusta hawaiiensis, known only from mesophotic depths off Lehua Island, west of Kaua’i Island, was found in shallow benthic reef habitats (3-18 m deep) along the western coast of Hawai’i Island. Molecular and microscopy techniques were used for genetic confirmation and for detailed morphological and anatomical examination. Two independent benthic cover survey datasets collected from west Hawai’i Island were used to investigate temporal and geographic distribution of Ramicrusta. In both datasets, we report Ramicrusta at approximately 60% of the sites surveyed. Benthic cover for this alga varies among sites and among years and its presence in west Hawai’i is evident since at least 2003. These findings help to document Hawaiian coral reef ecosystem change and benthic community composition reshuffling. This study also emphasizes the critical importance of taxonomy and proper identification of macroalgal species to understand the potential for phase-shifts of dominant taxa in coral reef ecosystems after environmental disturbances and fluctuations in abiotic factors. In the last decade, members of the red algal order Peyssonneliales have increased in abundance and overgrown other benthic species in reef ecosystems in the Caribbean and tropical Pacific. The novel aspect of finding abundant Ramicrusta in much shallower water than originally described, the decadal presence of Ramicrusta, and its potential for competition with other benthic organisms make this research valuable to coral reef ecology and justify further investigation of Ramicrusta ecology and biology in the Hawaiian Islands and globally.
Introduction
The interaction between corals and macroalgae is integral to reef ecology (Littler and Littler, 1984; McCook et al., 2001; McManus and Polsenberg, 2004; Bruno et al., 2009; Bramanti et al., 2017). Changes in ecological relationships, such as nutrient levels (Stimson, 2015; Carlson et al., 2019), herbivory (Donovan et al., 2018), coral diseases (Maynard et al., 2015), and climate factors (Foo et al., 2022) have been observed to make the critical difference in mixed algal-coral reef communities by influencing competitive advantage (McCook et al., 2001; McManus and Polsenberg, 2004). With increases in global climatic events, such as storms, heatwaves, and El Niño, the balance in abundance between corals and macroalgae is likely to change (Norström et al., 2009; Arif et al., 2022), but rarely are long-term benthic survey data available to document changing patterns in community composition.
Members of the order Peyssonneliales (Rhodophyta) (Krayesky et al., 2009) are mostly crustose calcifying red algae (CCRA) (Deinhart et al., 2022) that can coexist with corals or dominate subtidal reef habitats (Ballantine and Ruiz, 2011; Edmunds et al., 2019). One genus, Ramicrusta, comprises 19 species with a global distribution (Guiry and Guiry, 2022). Ramicrusta species have been described in marine environments in the Caribbean (Pueschel and Saunders, 2009; Ballantine et al., 2016; Bramanti et al., 2017), Gulf of Mexico (Fredericq et al. 2014); Brazil (Pestana et al., 2020), Guam (Mills and Schils, 2021), Australia (Dixon and Saunders, 2013; Dixon, 2018), Vanuatu (Dixon and Saunders, 2013), New Guinea (Dixon and Saunders, 2013), South China Sea (Nieder et al., 2019), Tunisia (Manghisi et al., 2019), and recently Hawai‘i (Sherwood et al., 2021c).
The ability of Ramicrusta species to directly overgrow living corals and to rapidly expand into new areas has been documented on reefs in the Caribbean (Eckrich et al., 2011; Ruiz, 2015; Smith et al., 2019; Hollister et al., 2021), Guam (Mills and Schils, 2021), and Donsha Atoll (Nieder et al., 2019). Eckrich and Engel (2013) reported that 45.8% of live coral colonies on reefs in Bonaire showed evidence of overgrowth by Ramicrusta thalli. This aggressive macroalga directly overgrew and killed individual coral colonies within 18 months (Eckrich and Engel, 2013). In benthic surveys, Williams and García-Sais (2020) recorded a dramatic increase in the abundance of Ramicrusta in Puerto Rico from 2016 - 2018. Ramicrusta thalli were absent at monitored sites prior 2016, but were observed at 76% of survey locations two years later, with benthic cover as high as 63% at some sites (Williams and García-Sais, 2020). In the Caribbean, Ramicrusta species have been documented overgrowing gorgonians, sponges, hydrocoral, crustose coralline algae, and the green calcified alga, Halimeda (Eckrich et al., 2011). Although Edmunds et al. (2019) and Williams and Edmunds (2021) observed decreases in percent cover of peyssonnelid algal crusts (PAC) on reefs after strong hurricanes in St. John, US Virgin Islands, shortly thereafter, PAC growth increased and PAC benthic cover returned to pre-disturbance levels, implying that PAC, including Ramicrusta, have the ability to be resilient and competitive in the community after natural catastrophic events.
In the last decade, many crustose genera in the Peyssonneliales and Gigartinales have been collected from the shallow intertidal to mesophotic depths in the Hawaiian Islands (Sherwood et al., 2010; Sherwood et al., 2020b; Sherwood et al., 2021a; Sherwood et al., 2021b; Sherwood et al., 2021c). These red algal crusts are often overlooked in reef ecosystems, lumped together in surveys as crustose calcifying red algae (CCRA) without determining genus or species identities, and their ecology remains little known. While many species of Peyssonneliales were observed in previous biological inventories, such as Sherwood et al. (2010), no Ramicrusta specimens were reported. Sherwood et al. (2021c) described two new species of Ramicrusta in the Hawaiian Islands: Ramicrusta hawaiiensis and Ramicrusta lehuensis. These two new algae represent the first of their genus to be described in Hawai‘i and have been reported only off Lehua Island, a 115 hectare uninhabited island 1.1 km north of Ni‘ihau, due west of Kaua‘i (22.02103°N, 160.10246°W). The potential for Hawaiian Ramicrusta species to be similar to their Caribbean or western Pacific relatives makes early geographic identification and regular monitoring of Hawaiian Ramicrusta crucial to understanding their role in the reef communities in the Hawaiian Islands (Eckrich et al., 2011; Eckrich and Engel, 2013; Ruiz, 2015; Smith et al., 2019; Hollister et al., 2021; Mills and Schils, 2021).
In recent years, a CCRA was frequently observed on survey transects on reefs along the west coast of Hawai‘i Island, Hawai‘i USA. With the recent decline of coral cover in this region as a result of coral bleaching events (Kramer et al., 2016), this unidentified alga may have become more obvious to reef survey teams. We noticed this CCRA actively overgrowing live coral colonies and crustose coralline algae in west Hawai‘i during surveys in 2020. The objective of this study was to confirm the identity of this unidentified CCRA along the western coast of Hawai‘i Island using DNA sequence data, describe the anatomy of the species using scanning electron microscopy, and document temporal and geographic patterns of this macroalga from 2003 to 2020 using benthic survey data. Our description and assessment of trends in multi-year long-term ecological data provide useful information foundational for marine monitoring and management of this macroalgal species.
Methods
DNA extraction and PCR protocols
The macroalgal sample was collected on May 18, 2021, at approximately 8 m deep just north of Kealakekua Bay at Keopuka DAR-WHAP site 18, HI, USA (19.48314°N, 155.9458°W) and stored in silica gel desiccant for scanning electron microscopy and molecular analyses. The material was divided in half to allow it to be deposited in the UL Lafayette herbarium (LAF) and Bernice Pauahi Museum Herbarium in Honolulu, HI (BISH). Total genomic DNA was extracted from the algal sample using the Quick-DNA Plant/Seed Kit (Zymo Research). DNA was extracted from the same specimen used for morphological analysis.
A portion of the mitochondrion-encoded cytochrome oxidase subunit I (COI) was amplified using the GazF1 & GazR1 primers (Saunders, 2005). The chloroplast-encoded ribulose-1,5,-bisphosphate carboxylase/oxygenase large subunit (rbcL) marker was amplified using multiple primer pairs: F7-R753, F15-R916, F57-R753, F577-R1150, F645-R1381, and F993-RStart (Freshwater and Rueness, 1994; Lin et al., 2001; Gavio and Fredericq, 2002; Krayesky et al., 2009; Kim et al., 2010). Multiple overlapping reads were generated to ensure quality chromatograms were obtained for the full length of the consensus read.
PCR for COI was conducted according to the methods in Saunders (2005) with an initial denaturation at 94°C for 3 min followed by 40 cycles at 94°C for 1 min (denaturation), 45°C for 1 min (primer annealing), and 72°C (extension) for 1 min followed by a final extension at 72°C for 5 min. PCR for rbcL was conducted using established methods (Freshwater and Rueness, 1994; Lin et al., 2001; Gavio and Fredericq, 2002) with an initial denature of 94°C for 4 min followed by 2 cycles at 94°C for 1 min (denaturation), 40°C for 1 min (primer annealing), and 72°C (extension) for 2 min then 40 cycles at 94°C for 1 min (denaturation), 42°C for 1 min (primer annealing), and 72°C (extension) for 2 min followed by a final extension at 72°C for 5 min.
The resulting PCR products were purified with ExoCIP Rapid PCR Cleanup kit (New England Biolabs). Purified PCR products were subsequently cycle sequenced using the BigDye Terminator v. 3.1. kit (Life Technologies). The resulting cycle sequence reactions were purified with ETOH/EDTA precipitation and were sequenced in-house at the UL Lafayette campus on an ABI Model 3130xl Genetic Analyzer. The resulting chromatograms were assembled using Sequencher v. 5.1 (Gene Codes Corp., Ann Arbor, MI, USA) and exported as individual “.fasta” files.
Multi-gene alignment
The COI and rbcL sequences were aligned with the CLUSTAL W (Thompson et al., 1994) algorithm in the MEGA X software (Kumar et al., 2018), and then edited manually for a more precise alignment with other available sequences of Ramicrusta and related taxa from NCBI GenBank as in Sherwood et al. (2021c) (Supplementary Table 1). A concatenated gene dataset for COI and rbcL was assembled using the application Sequence Matrix v. 1.8 (Vaidya et al., 2011) and exported as a “.phy” format. DNA sequences from the peyssonnelid genus Incendia were used as the outgroup for phylogenetic analyses.
Phylogenetic analysis
The concatenated dataset was imported into the CIPRES gateway (Miller et al., 2010) and analyzed in PartitionFinder2 (Lanfear et al., 2017) to determine the best partition scheme and model(s) of evolution that can be implemented by RAxML. For the two datasets, a three-codon position partitioning scheme, each evolving with a GTR+I+G model was selected on the basis of the Akaike Information Criterion (AIC), the corrected Akaike Information Criterion (AICc), and the Bayesian Information Criterion (BIC). The alignment with these models and partitioning scheme was then analyzed for maximum likelihood (ML) with RAxML-HPC2 on XSEDE v. 8.2.12 (Stamatakis, 2014) via the CIPRES gateway (Miller et al., 2010) with 1,000 bootstrap replicates.
Sequence divergence analyses
The COI and rbcL sequences were aligned with the CLUSTAL W algorithm in the MEGA X software, but truncated close to the primer sites to produce base pair lengths with no missing data. Sequence divergences values were calculated as the number of pairwise base pair differences in MEGA X (COI = Supplemental Tables 2A, rbcL = Supplemental Table 2B).
Scanning electron microscopy methods
Scanning electron microscopy methods were adapted from Richards et al. (2014) as follows: portions of the thallus from silica gel-dried specimens were removed using a razor blade and forceps and sectioned by performing vertical fractures. Specimens were sectioned manually using a new single-edge razor blade for each fracture and were mounted using liquid graphite conductive adhesive (Electron Microscopy Sciences) on a 32 mm x 6 mm-sized aluminum mount (Electron Microscopy Sciences). An 18 nm gold coating was applied to the sample using the EMS 550X sputter coating device. Specimens were viewed using a Scios 2 Dual Beam Focused Ion Beam scanning electron microscope (FIB-SEM) at a voltage of 15 kV, housed in the Microscopy Center at the University of Louisiana at Lafayette following manufacturer’s instructions.
Morphological comparisons of vegetative Ramicrusta hawaiiensis tissue used the characteristics delineated in Sherwood et al. (2021c) and Mills and Schils (2021) to distinguish Ramicrusta species that lack reproductive structures, including thallus thickness, epithallial cells, perithallial cells, presence of pit connections, hypobasal cells, and rhizoid cells.
Study region
We assessed the distribution of a previously unidentified CCRA along the leeward or west coast of Hawai‘i Island, HI, USA (Figure 1). This coastline has a range of nearshore marine habitats that consist of calcareous reefs, basalt outcroppings, and large sand flats. The West Hawai‘i Fish and Habitat Monitoring and Assessment project of the Department of Land and Natural Resources (DLNR)’s Division of Aquatic Resources (DAR) has collected benthic photographic data at 26 permanent sites established for the West Hawai‘i Aquarium Project (WHAP). Benthic photo-quadrat images were collected at 23 sites beginning in 2003; two additional sites were added in 2007 and one more site was added in 2014 for a total of 26 sites with imagery. The 26 DAR fixed monitoring sites span the west coast of Hawai‘i Island from Manukā in the south to Lapakahi in the north, along 140 km of coastline, and represent mid-depth (9-15m), coral-rich habitat (Tissot et al., 2004). The photo-quadrat imagery from these surveys represents a robust, long-term temporal benthic dataset. While the DAR-WHAP benthic surveys are temporally extensive, the sites were established to represent primarily mid-depth, coral-rich habitat in west Hawai‘i and to assess the efficacy of a marine reserve system (Tissot et al., 2004). In addition to these data, we incorporated benthic data from the 2020 Arizona State University South Kona Intensive (SKI) reef surveys to broaden the scope of habitats analyzed. These SKI photographic data are representative of only a single time point, but were designed to capture the variation that exists among marine nearshore habitats at 2-18 m depths along 60 km of coastline in the district of South Kona (Asner et al., 2021). These two datasets (WHAP and SKI) and their field sites, while in the same region and occasionally in the same vicinity, were collected independently. We used the DAR-WHAP data to explore temporal trends in benthic cover of the unidentified CCRA, and we used the SKI data to assess the most recent geographical distribution of the unidentified CCRA in the South Kona district from Keauhou to Manukā. These surveys only represent shallow reef environments (< 20 m deep). No geographically extensive or long term mesophotic surveys have been conducted in west Hawai‘i to-date.
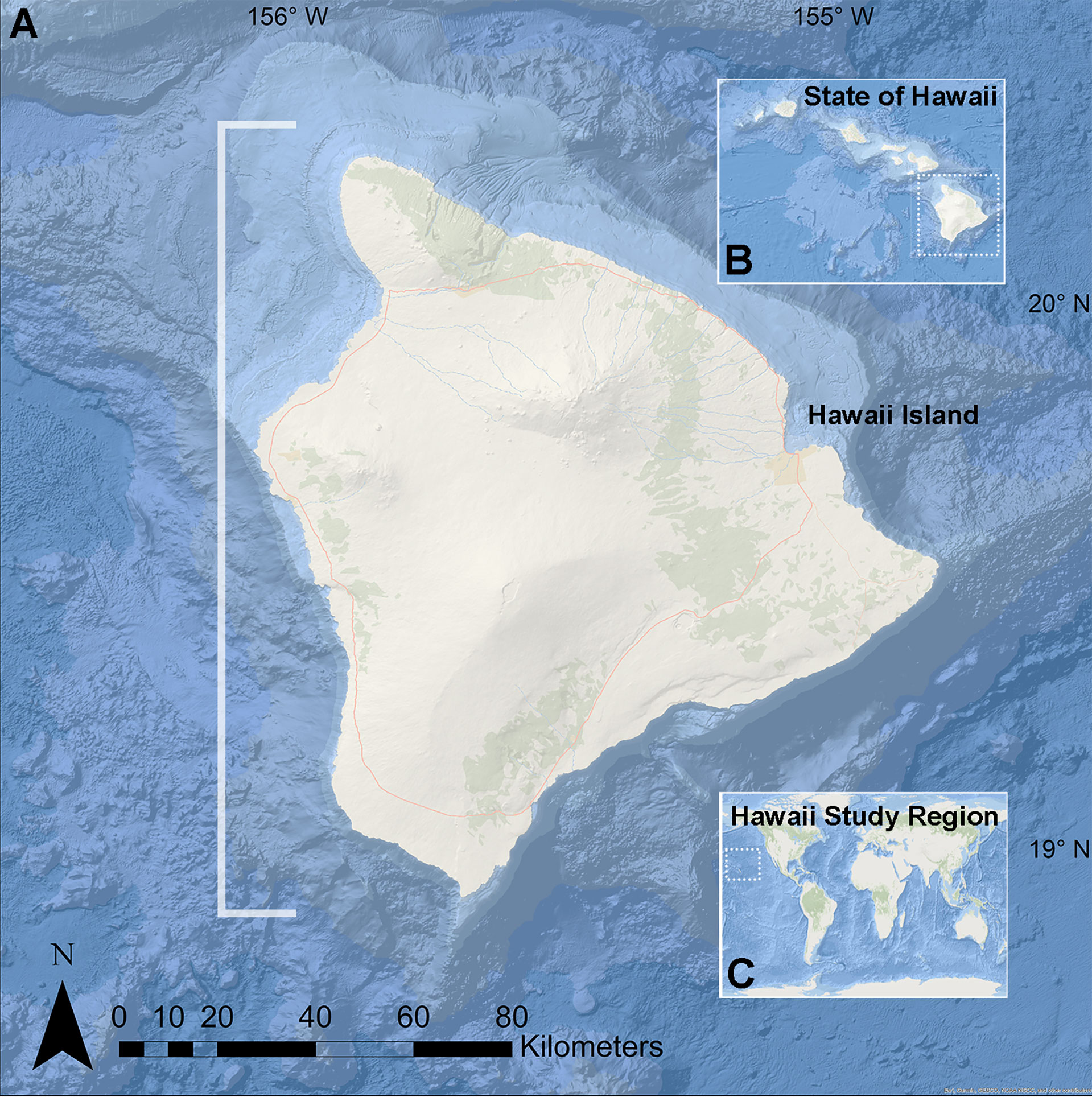
Figure 1 Map of study region on Hawai’i island, Hawai’i USA. (A) Hawai’i Island. (B) Location of Hawai’i Island, the southernmost of the Hawaiian Islands. (C) Location of the Hawaiian Archipelago in the north Pacific Ocean. White bracket indicates the west Hawai’i region in which survey data and specimens were collected.
Temporal and geographic benthic data collection and analysis
In 2021 and 2022, we analyzed two photographic benthic survey datasets from west Hawai‘i to determine the temporal and geographic patterns of the CCRA suspected to be a Ramicrusta species. DAR-WHAP photo-quadrat images were collected during respective survey years and annotated in 2021 to calculate a percent cover of Ramicrusta distinct from other algal crusts with which it was grouped during photo analyses in previous years. The photo-quadrat surveys at the WHAP sites were conducted in the years: 2003, 2007, 2011, 2014, 2016, 2017, and 2020. For each of the surveyed years, images were collected usually in less than one month between May and November. Four permanent 25-meter transects are located at each site. Transects are approximately 10 meters apart, with two north-facing transects parallel to each other, and two south-facing transects parallel to each other. Images were taken at 1 m intervals along each transect starting at the 0 m mark and ending at the 25 m mark to produce 26 images of a 0.3 m2 area of the benthic habitat. A 75 cm monopod was secured to the camera housing and placed perpendicular to the reef substratum to ensure that images were taken from a standard height. Individual images were uploaded to CoralNet (v1.0 https://coralnet.ucsd.edu; Beijbom et al., 2015; Williams et al., 2019) for image annotation and benthic taxa identification. Twenty random points were placed on each photo and the underlying benthic taxa at each point were identified to the lowest taxon possible. CCRA were identified to the genus Ramicrusta by looking for the characteristic dark maroon-red and rusty orange color of the algal crust and the occasional raised edges or free margins. Percent cover was calculated by pooling all points within a given transect and dividing the number of points of a specific taxon by the total points included in that transect. Points identified as heavily shaded, artifact (survey tape or camera pole), or mobile species (fish, urchins, etc.) were removed before the percent cover was calculated. Because the four transects are so close to each other, data are presented as the mean of these four averages with standard error representing the variance among transects.
The 112 SKI sites from 2020 were chosen based on a stratified random sampling design and were surveyed between June and August of 2020; methods for the stratification and site selection can be found in Asner et al. (2021). The percent cover of the unidentified CCRA at each site was determined using a 10 m x 10 m Structure from Motion (SfM) survey with a Canon 5D Mark IV (Canon U.S.A., Inc. Melville, New York, USA). SfM photogrammetry is an image processing technique that allows the reconstruction of accurate 3D models from overlapping successive photographs taken from cameras at various angles with or without ground control points (GCPs). Images were processed using Agisoft Metashape software (v1.6.2, Agisoft LLC., St. Petersburg, Russia) using procedures described in Burns et al. (2015) and Bayley and Mogg (2020). Spatially rectified orthomosaics were clipped into 10 m x 10 m squares. Prior to any further analysis, all clipped orthomosaics were visually inspected for the presence of the unidentified CCRA. The CCRA were identified to the genus Ramicrusta by looking for the characteristic dark maroon-red and rusty orange color of the algal crust and the occasional raised edges. Orthomosaics in which the alga’s presence was evident, were then assessed for the CCRA percent cover by randomly placing ten 0.25 x 0.25 m quadrats throughout the orthomosaic. Orthomosaics in which no presence of the alga was evident given a CCRA percent cover of 0 and were kept in the dataset for analysis. Metadata for all SKI sites, such as depth, were taken at the mosaic level, but not the individual quadrat level. Individual quadrats were clipped from each mosaic and were uploaded into CoralNet (v1.0 https://coralnet.ucsd.edu Beijbom et al., 2015; Williams and García-Sais, 2020). Fifty random points were generated in each quadrat photo to estimate the average percent cover of the unidentified CCRA for each quadrat. Quadrat points from a single mosaic were summarized to the site level to estimate mean CCRA benthic cover. Maps and clipped orthomosaics were processed using ArcMap version 10.7.1.
Environmental datasets were collected to investigate any temporal and geographic trends in relation to the unidentified CCRA percent cover. Sea Surface Temperature Anomaly (SSTA) data were collected using National Oceanic and Atmospheric Administration (NOAA) Coral Reef Watch program (CRW) at the site level for both DAR-WHAP and SKI sites (https://coralreefwatch.noaa.gov/product/5km/index.php#data_access: NOAA Coral Reef Watch, 2018). All NOAA CRW data were gathered at 5 km resolution GeoTIFFs for the entire coast of west Hawai‘i. Each pixel represents the relative temperature compared to the mean temperature of the past seven years for that particular time point. Positive SSTA values represent warmer than average sea surface temperature (SST) in the given location for that date; whereas, negative SSTA values represent cooler than average SST for the location for that date. These SSTA data were collected on the first of every month at each site beginning one year prior to the benthic surveys being conducted to allow a lag time for benthic environments to respond to any changes in SSTA (Doty, 1971; Glenn et al., 1990; Edmunds and Lasker, 2016; Donovan et al., 2021; Foo et al., 2022). These monthly data were averaged by year and site to determine specific SSTA for each location with a one-year-lagged temporal timestamp.
Degree heating week (DHW) data were collected using NOAA CRW at the site level for the 2015 and 2019 coral bleaching events (Winston et al., 2022). These data were created by using the DHW from the first of each month, from August to December, and then calculating the average DHW for each of the 2020 SKI site locations during each of the two bleaching events. The DHW variables were assessed only at the SKI locations to assess any effect they may have had on the most recent benthic cover of the unidentified CCRA.
Using the DAR-WHAP dataset, we conducted a Two-way Analysis of Variance (ANOVA) to investigate differences in percent cover among years at individual sites. A natural log transformation was used for percent cover data to unbound the benthic cover data so that values are not restricted to 0 - 100 and to shift these left-skewed data to a normal distribution. To assess trends and correlative relationships with environmental data, we used a Linear Mixed Effects Regression (LMER) as an exploratory analysis. This model used each site’s year as a random intercept variable in the model to account for year level variation. In this temporal analysis, we used SSTA, latitude, and the interaction of the two as predictor variables. Prior to the analysis, we scaled predictor variables to a mean of zero and a standard deviation of one. Due to discrepancies or lack of sampling consistency, for this analysis, we used only 23 of the 26 DAR-WHAP sites available. Sites 96, 97, and 98 were removed prior to analysis because these sites were not sampled in all years. Data from sites 96, 97, and 98 are still plotted in preceding figures, but are not included in any figures that present statistical results.
We used an Ordinary Least Squares (OLS) multiple regression to determine correlative relationships between the percent cover of the unidentified CCRA, depth, SSTA, and average DHW from both the 2015 and 2019 bleaching events in the 2020 SKI data. All statistics were calculated in R Studio version 3.6.2.
Results
Phylogenetic analyses and sequence divergence analyses
The phylogenetic analyses from the concatenated COI and rbcL revealed that the voucher specimen LAF 7678 aligns with Ramicrusta hawaiiensis (Figure 2), thus expanding the geographic and depth range of the species. The COI and rbcL barcode sequences of voucher LAF 7678 were very similar to Ramicrusta hawaiiensis voucher from Lehua Island ARS 09600 (1.8% and 0.04% intraspecific sequence divergence, respectively). Ramicrusta hawaiiensis LAF 7678 was distinct from R. lehuensis based on the sequence divergence values of 9.1% and 7.1% for COI5-P and rbcL, respectively (Supplemental Tables 2A, 2B). Ramicrusta hawaiiensis LAF 7678 interspecific sequence divergence ranges from 6.94% (R. aranea) to 12.5% (R. australica) for COI-5P and 1.98% (R. aranea) to 10.8% (R. australica) for rbcL. Comparing interspecific divergence values of the outgroup taxa Incendia glabra and I. regularis ranged from 14.6% and 15.78% for COI5-P and rbcL, respectively (Supplemental Tables 2A, B). All interspecific sequence divergence values are in the range consistent with previous studies in the Peyssonneliales order (Mills and Schils, 2021; Sherwood et al., 2021a; Sherwood et al., 2021b; Sherwood et al., 2021c).
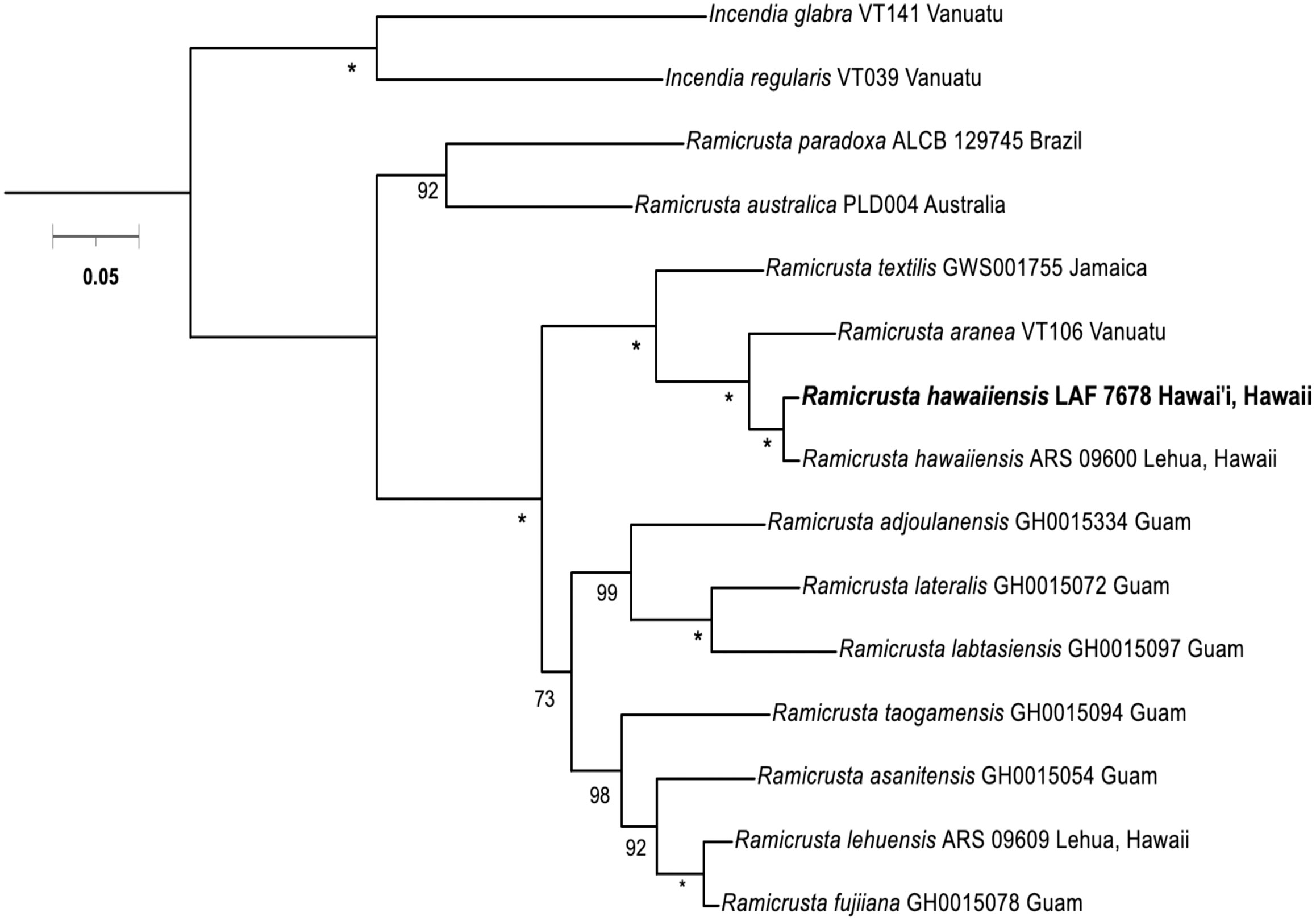
Figure 2 Concatenated COI and rbcL maximum-likelihood tree (2,035 bp) for Ramicrusta hawaiiensis and closely related species. Numbers along branches indicate nodal bootstrap support. Nodes with full support are indicated with an asterisk. Bootstrap support values less than 50 were omitted. Scale bar = substitutions per site.
Morphological and anatomical description
The morphology of R. hawaiiensis LAF 7678 generally agrees with the description of the one specimen of R. hawaiiensis collected by Sherwood et al. (2021c), but discrepancies may be explained by the difference in habitats (Table 1). The thallus surface of LAF 7678 is dark red to pink color with orange bumps and moderate calcification (Figure 3), approximately 550 µm thick, and forms tightly adherent crusts with occasional free margins on reef structures (Figure 4A). Epithallial cells are composed of several stacked rows of small cells, around 7-10 µm in diameter by 4-8 µm in height in the uppermost rows (Figures 4B, 3C, E). Perithallial cells are slightly elongated, 12-21 µm in diameter and 20-27 µm in height; they get smaller and more regular in shape toward the epithallus (Figures 4B–E). Primary pit connections and cell fusions are present in some perithallial cells. Hypobasal cells are globose, around 4-8 µm in diameter and 17-20 µm in height (Figure 4F). Rhizoids are common, 50-130 µm long and 12-21 µm in diameter (Figures 4G, H). Reproductive structures were not observed.
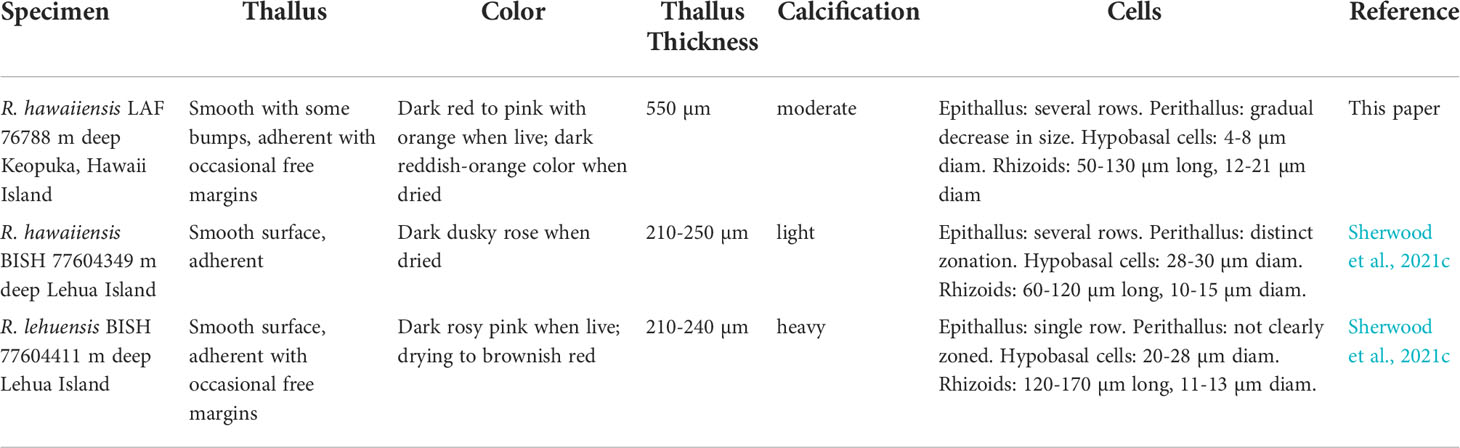
Table 1 Morphological comparisons of Hawaiian specimens of Ramicrusta hawaiiensis and R. lehuensis, including thallus thickness, epithelial cells, perithallial cells, presence of pit connections, hypobasal cells, and rhizoid cells.
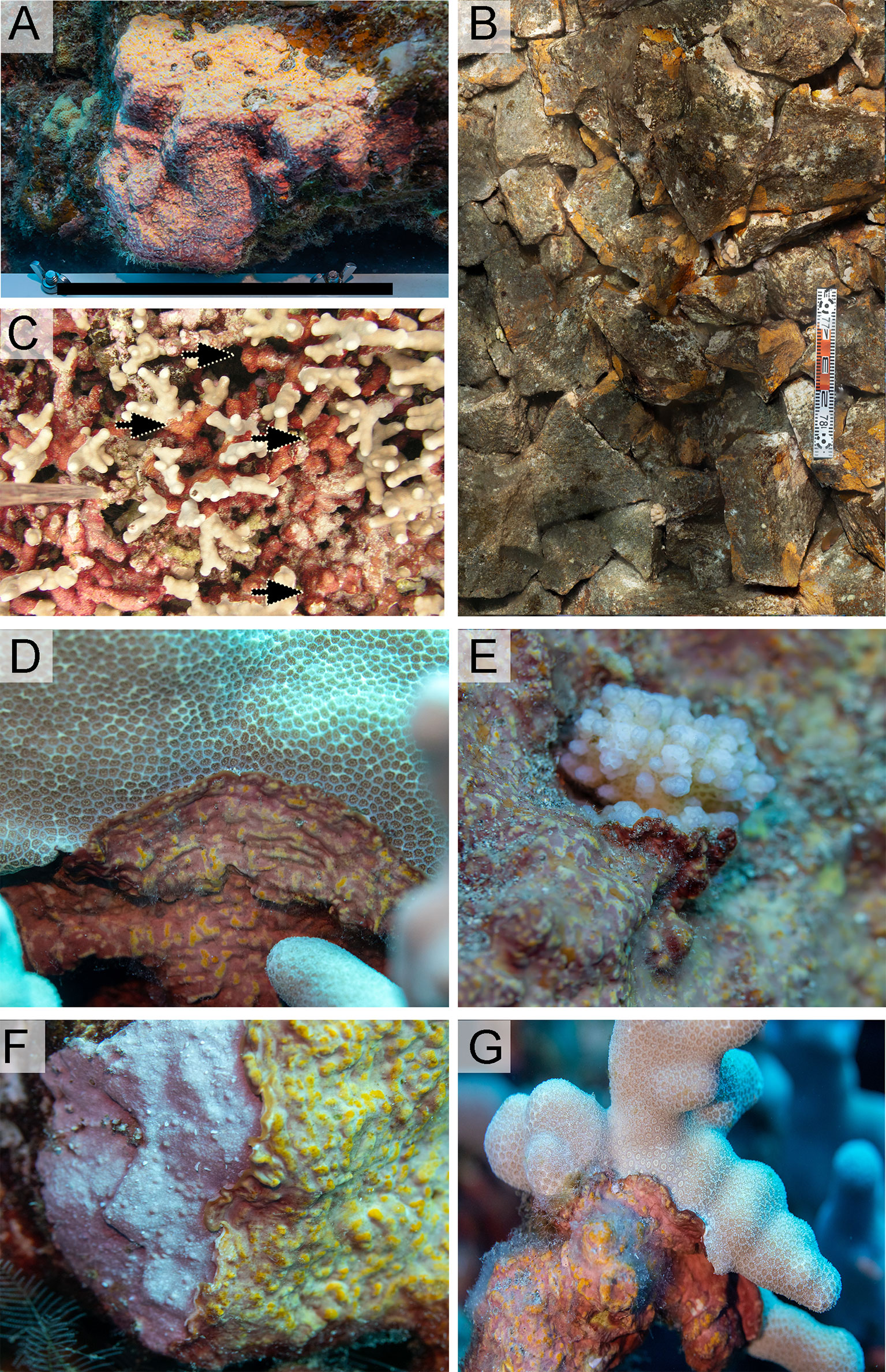
Figure 3 In-situ images of the morphology of Ramicrusta hawaiiensis overgrowing a basalt boulder on South Kona coral reefs in 2021. (A) Note the distinct color shift on the specimen with the upper lighter orange section and the lower red ventral section of the specimen, black line bar = 23 cm. Field observations have noted that surface-facing sections of this alga tend to be rusted orange while shaded sections of specimens have rusted red or pink hues. (B) Image from SfM orthomosaic where Ramicrusta hawaiiensis is prominent with orange encrusting morphology on basalt slabs, scale bar = 50 cm. (C) Field observations from 2003 DAR-WHAP photo-quadrat image surveys with Ramicrusta specimens noted by the black arrow. Images (D–G) show active overgrowth by Ramicrusta hawaiiensis on (D) Porites lobata, E. Pocillopora spp., (F) Crustose Coralline Algae (CCA), and (G) Porites compressa.
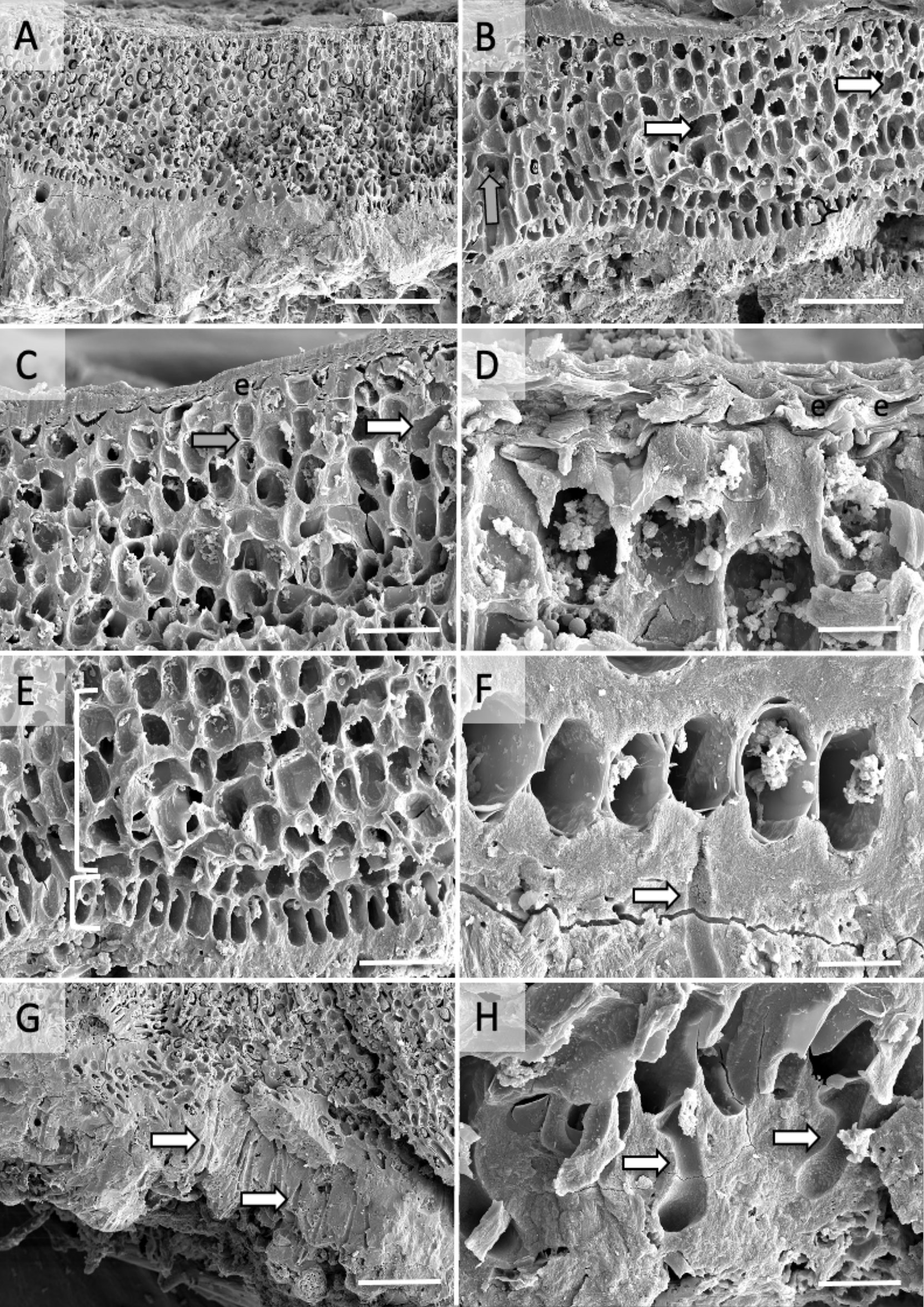
Figure 4 Scanning Electron Microscopy images of Ramicrusta hawaiiensis voucher LAF7678. (A) Section view of thallus construction, scale bar = 200 µm. (B) Section showing cell fusions (arrows), epithallial cells (e), and hypobasal cells (bracket), scale bar = 100 µm. (C) Section showing epithallial cells (e), primary pit connections (gray arrow), and cell fusions (white arrows), scale bar = 50 µm. (D) Zoomed in view of layered epithallial construction (e), scale bar = 10 µm. (E) Section showing perithallial cells with cell fusions (top bracket) and a single row of hypobasal cells (bottom bracket), scale bar = 50 µm. (F) Enlarged view of single row of hypobasal cells with rhizoid (arrow), scale bar = 20 µm. (G) Section showing multiple rhizoids, common (arrows), scale bar = 100 µm. (H) Zoomed in view of rhizoids (arrows), scale bar = 20 µm.
The identification of Hawaiian Ramicrusta to the species level requires vouchers and microscopic examination of a cross-section of the crust or DNA analysis. Vouchers were only available for benthic surveys in 2021. However, the CCRA in photographs from 2003-2020 were confidently identified to the genus Ramicrusta by the characteristic dark maroon-red and rusty orange color of the encrusting alga and the occasional upturned or raised edges.
Temporal and geographic trends of Ramicrusta on west Hawai’i island
Of the 23 DAR-WHAP sites assessed from 2003-2020, 16 of them (69.6%) had a detectable amount of Ramicrusta present during at least one survey year. Of these 16 sites,14 sites had Ramicrusta present as early as 2003 (Figure 5; Table 2). Among the 16 sites where Ramicrusta was present during at least one survey year, the greatest percent changes in cover were observed between survey years 2007 and 2011, and between 2017 and 2020 (Table 2). Geographically, southern sites within the DAR-WHAP dataset, such as those in South Kona, had higher benthic cover of Ramicrusta (Figure 6). From 2003-2020, average percent cover of Ramicrusta at DAR-WHAP sites showed an abrupt increase at sites south of 19.60°N latitude (Figure 6). Results from a Two-way ANOVA showed differences in the percent cover of Ramicrusta among multiple DAR-WHAP sites (F(1)=89.104, p < 0.001) and among years 2017 and 2020 (F(2)=2.828, p < 0.012) (Figures 5 and 7). In our exploratory analysis, we found long-term geographic patterns for the benthic cover of Ramicrusta and the environmental variable of SSTA. Results from a Linear Mixed Effect Regression (Table 3) showed that SSTA data had a positive effect with the percent cover data of Ramicrusta (β-Est = 0.0.0846, t value = 2.773, p = 0.016), while increased latitude (sites farther north) had a negative effect (β-Est = -0.228, t value = -7.829, p = < 0.001). Additionally, the interaction of the two parameters (SSTA*latitude) showed a negative effect (β-Est = -0.120, t value = -3.573, p < 0.001) (Table 3).
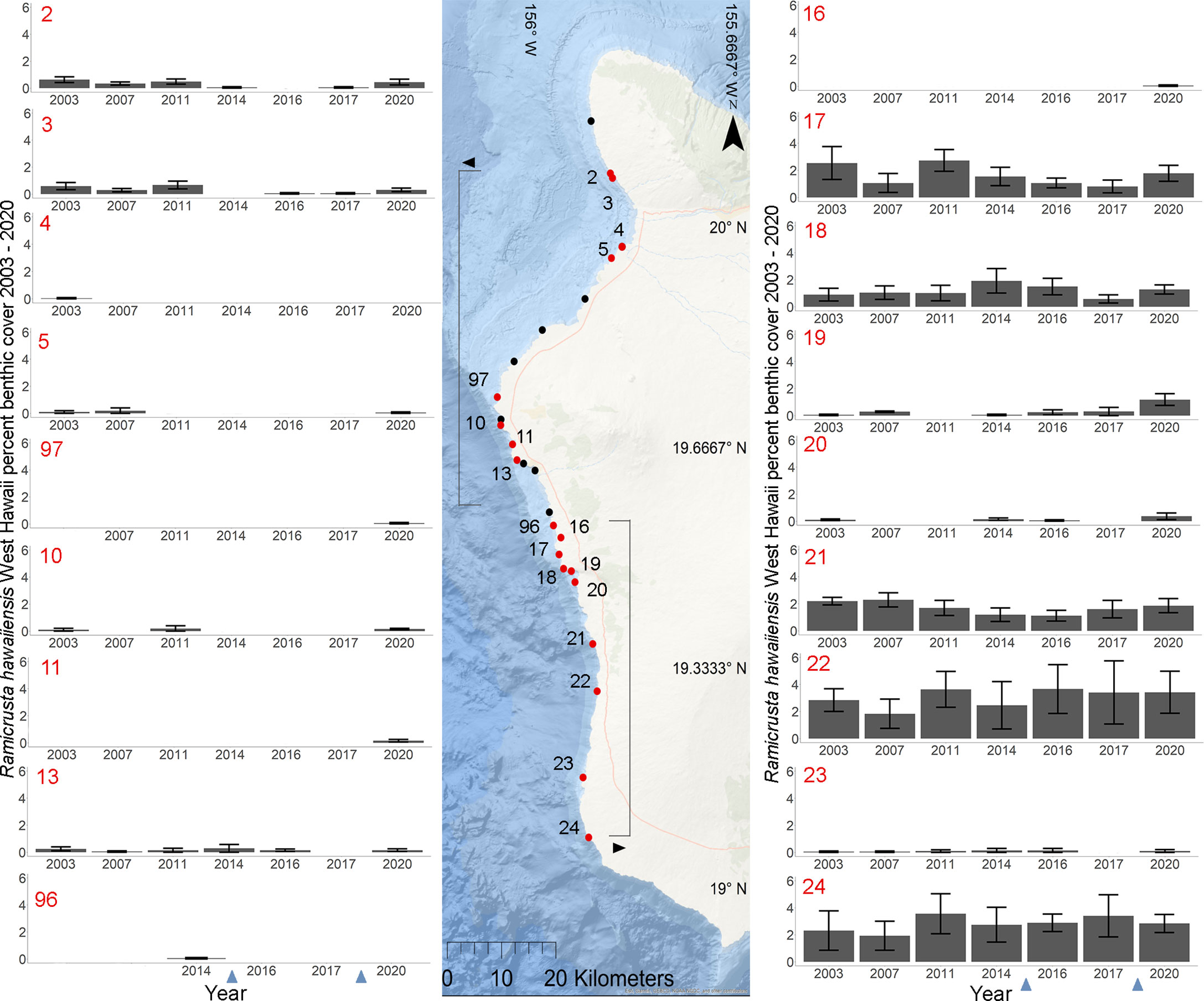
Figure 5 Geographic and temporal distribution of percent benthic cover of Ramicrusta. Map of the 26 DAR-WHAP sites in west Hawai’i (center), and 18 graphs of temporal benthic cover at sites where Ramicrusta was present (left and right). Ramicrusta was present at sites shown in red, but absent at sites shown in black. Individual site percent cover values for Ramicrusta are ordered by latitude with standard error bars with northern (bracketed with arrow pointing left) and southern (bracketed with arrow pointing right) sites. Only sites that had detectable Ramicrusta were plotted. The absence of bars indicates that no Ramicrusta was observed at that site in that year. Note sites 97 and 96 are plotted in this figure but not included in statistical analysis due to missing survey years. The absence of years in the x axis indicates that no benthic surveys were conducted at that site in that year. Blue arrows on the x axis indicate years with marine heat waves.
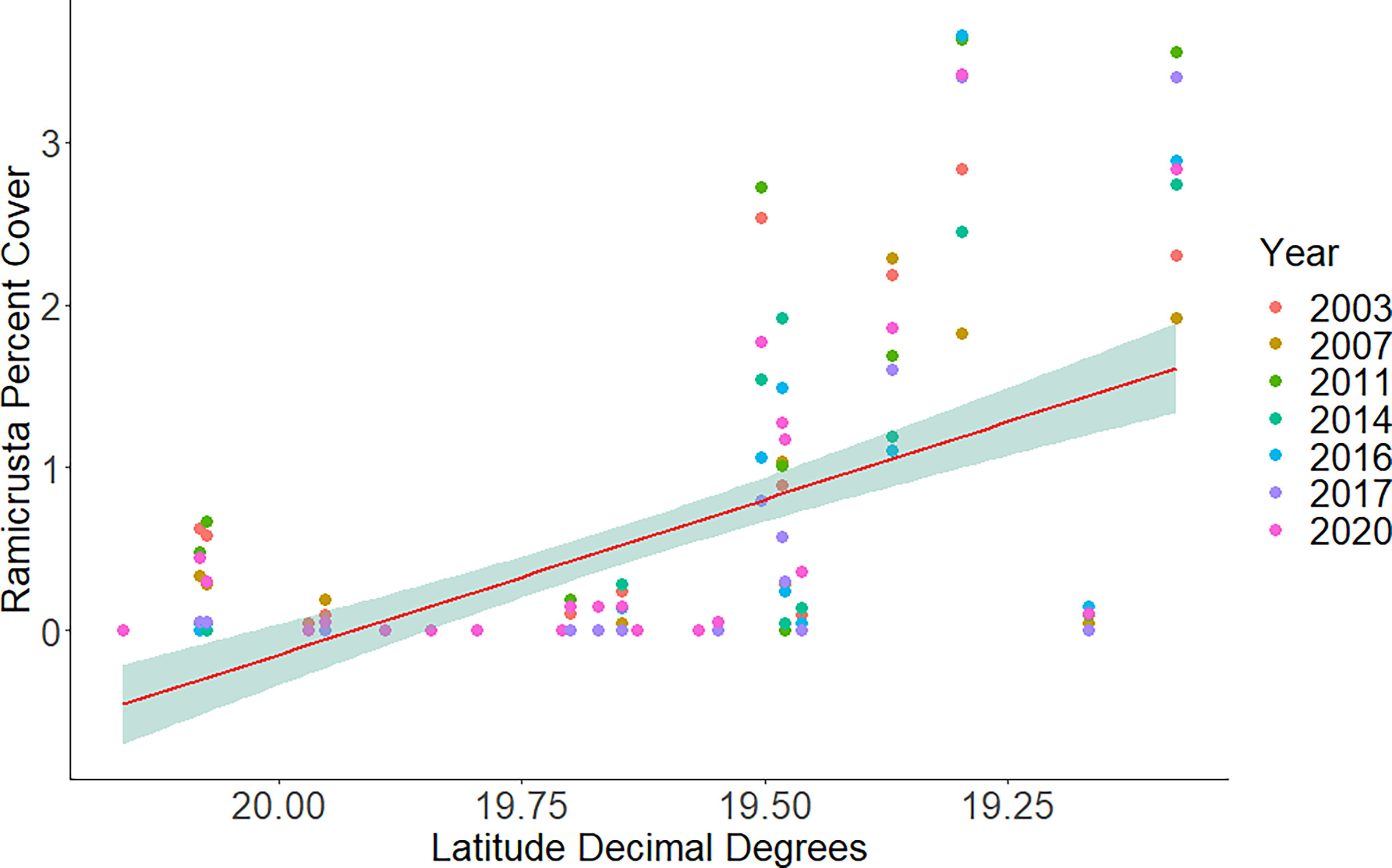
Figure 6 Average percent cover of Ramicrusta at DAR-WHAP sites from north to south in each year of surveying. Ramicrusta percent cover shows an abrupt increase at sites south of 19.60 latitude. Red line indicates the best fit line with the shaded region showing the standard error.
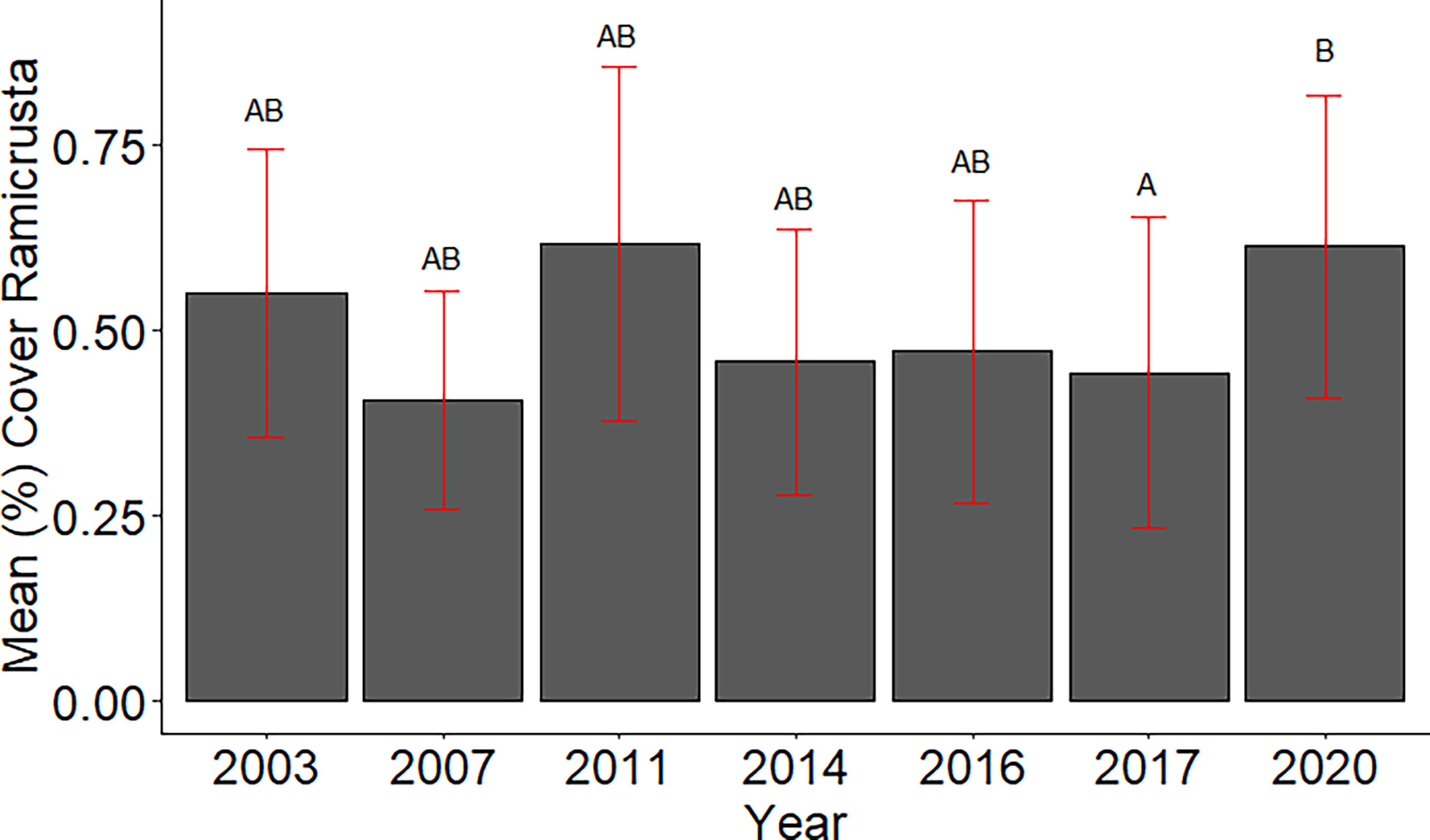
Figure 7 Mean percent cover of Ramicrusta in DAR-WHAP surveys 2003 - 2020. Bars represent standard error of the mean. The DAR-WHAP data indicate significantly higher abundance of Ramicrusta in 2020 than in 2017. Shared letters indicate annual means that are not significantly different based on a Tukey post-hoc comparison test.

Table 3 Coefficient estimates of Sea Surface Temperature Anomalies (SSTA), latitude, and their interaction on the percent cover of Ramicrusta from a Linear Mixed Effect Model accounting for site as a random effect at DAR-WHAP sites from 2003 - 2020.
In 2020, Ramicrusta was present at 71 (63%) of the 112 SKI survey sites. The average South Kona regional percent cover in 2020 was 2.22% ± 0.57 SE (Figure 8). As expected, because of the wide range of habitats surveyed, averages varied considerably among sites with a median percent cover of 1.2%. The most southern site surveyed (site 178) had the highest Ramicrusta benthic cover of 37.4% ± 9.17 SE. In 2020, average percent cover of Ramicrusta at DAR-WHAP and SKI sites showed higher abundance at the more southern survey sites (Figure 9). In the OLS multiple regression, a positive effect between SSTA and Ramicrusta benthic cover (p = 0.019, β-Est = 0.14) was evident, similar to the analysis of the DAR-WHAP temporal data. However, this effect is very weak and explains little overall data variance; an independent OLS regression showed that these SSTA data captured a very small proportion of the overall variance within the Ramicrusta SKI data (p = 0.024, Adjusted R-squared = 0.03). Predictor variables of depth (p = 0.671, β-Est = -0.026) and average degree heating weeks (DHW) for the 2019 (p = 0.608, β-Est = -0.234) or the 2015 (p = 0.504, β-Est = 0.304) marine heat waves events did not show any significant trends relative to the Ramicrusta benthic data.
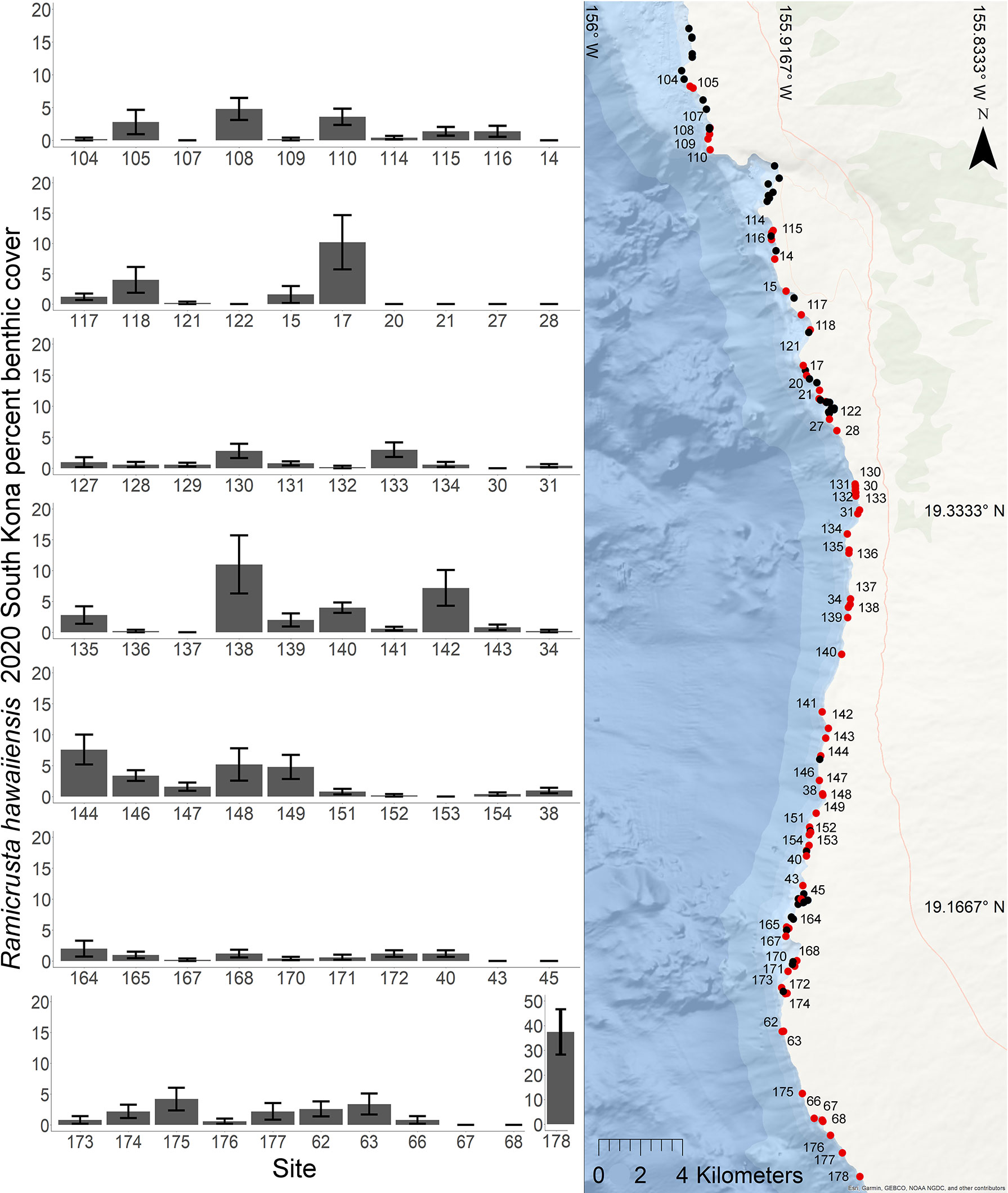
Figure 8 Geographic distribution and percent benthic cover of Ramicrusta at South Kona sites in 2020. Map of the 112 South Kona Intensive (SKI) sites in South Kona (right). Ramicrusta was present at 71 sites shown in red, but absent at 41 sites shown in black. Average percent cover values with standard error bars for Ramicrusta are ordered by latitude from north to south at the sites (red) where Ramicrusta was observed in the surveys. Note that the scale of the y axis is 0-20% (unlike 0-5% in Figure 4) for all sites, except site 178 (0-50%).
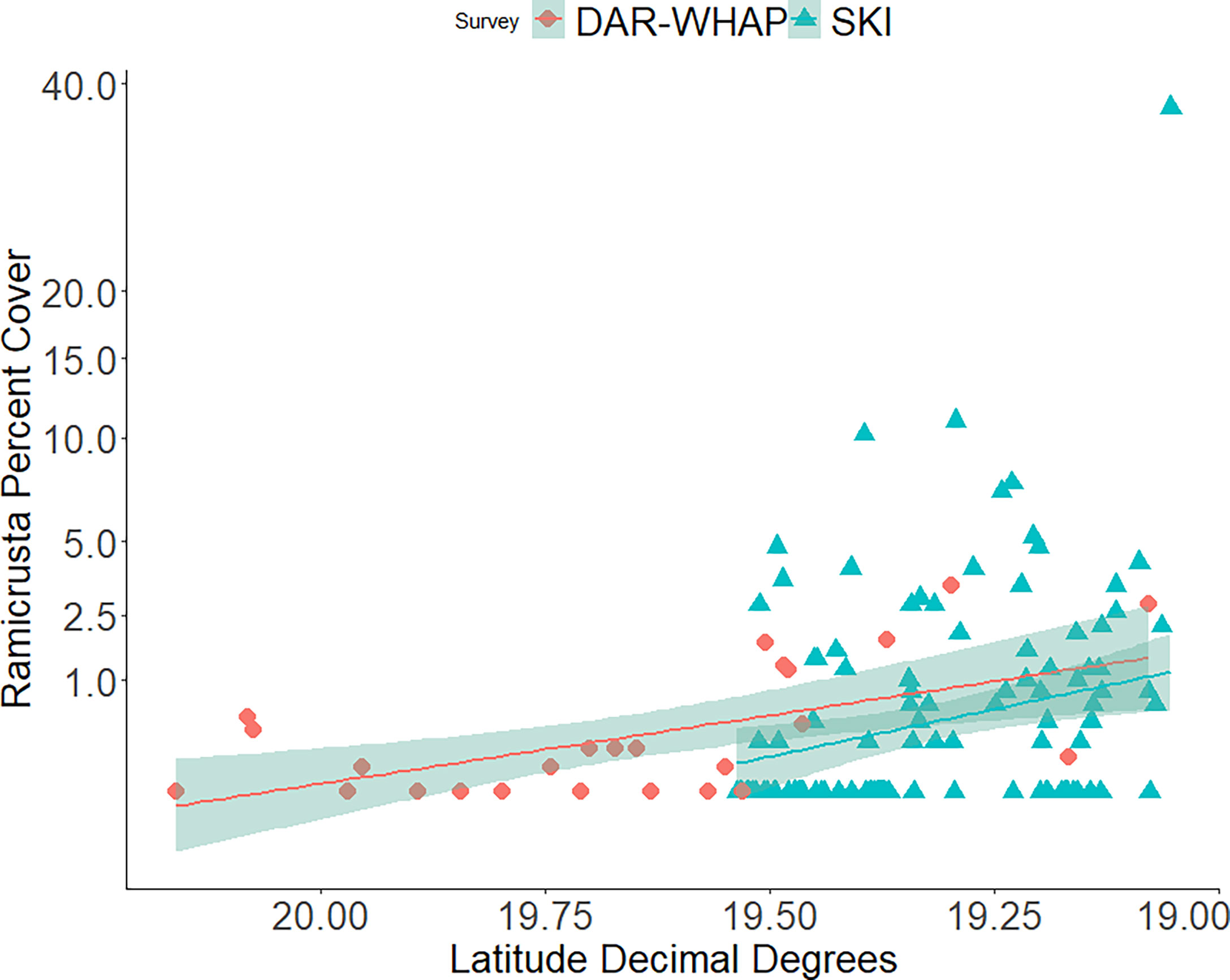
Figure 9 Average percent cover of Ramicrusta at DAR-WHAP sites and SKI sites from north to south in 2020. Ramicrusta percent cover shows higher abundance at several of the southern survey sites at 19.50 latitude and farther south. Trend lines indicate the best fit line with the shaded regions showing the standard error.
Discussion
Temporal, geographic, and habitat characteristics of Ramicrusta in west Hawai’i
Crustose calcifying red algae (CCRA) in the order Peyssonneliales are gaining more ecological attention because of their presence and growth patterns in reef ecosystems around the world. Despite the challenges of accurate taxonomy of CCRA because of their crustose morphology, frequent lack of reproductive structures, cryptic habit on reefs, and plasticity of growth forms in different habitats, these macroalgae must not be overlooked or misidentified in coral-algal studies, especially as climate change continues to alter basic environmental conditions. In the Hawaiian Islands, in the last decade, many crustose genera in the Peyssonneliales and Gigartinales have been collected (Sherwood et al., 2010; Sherwood et al., 2020b; Sherwood et al., 2021a; Sherwood et al., 2021b; Sherwood et al., 2021c) and two new species, Ramicrusta hawaiiensis and R. lehuensis, were described in 2021: R. hawaiiensis from mesophotic depths (> 30 m deep) and R. lehuensis from 11 m deep in the northern portion of the archipelago. However, this study documents that on the west coast of Hawai‘i Island, the red, crustose, calcifying Ramicrusta is present, abundant, and clearly visible in shallower depths (3 -18 m deep), and showed the propensity to overgrown live coral colonies and crustose coralline algae.
This study shows that the unidentified CCRA observed along the west coast of Hawai‘i Island in reef surveys DAR-WHAP site 18 are Ramicrusta hawaiiensis. Although assemblages of Ramicrusta at the other sites surveyed were visually attributed to R. hawaiiensis, additional collections are required for molecular analysis to verify that they are, in fact, R. hawaiiensis. Differences in the percent cover of Ramicrusta among sites (Figures 5, 6, 8, and 9) and among years (Figures 5 and 7) suggest that there may be environmental (substratum, wave exposure, currents), ecological (recruitment success), or biological controls (urchin and fish abundance) influencing the growth and spread of this macroalga. Future studies should investigate the influence of the many variables on the ecology of Ramicrusta, as well as sequence additional samples from this region to verify the full range of Ramicrusta species and to assess if other closely related taxa are present in the region. Previous biological inventories focusing on Hawaiian algal species such as Sherwood et al. (2010) provided baseline data however did not find evidence of Ramicrusta. Given the abundance of Ramicrusta presented in these data, additional biological inventories focusing specifically on CCRA on Hawaiian reefs may describe further species and an even greater geographic extent of Ramicrusta species.
The examination of temporal photographic datasets suggests a long-standing presence of Ramicrusta on Hawai‘i Island for at least the past 20 years that has been overlooked or identified as “crust” or misidentified as a CCA in monitoring. In comprehensive taxonomic surveys of Hawaiian red algae from 2006 to 2010, no species of Ramicrusta were reported in the shallow intertidal zone to 60 m deep using LSU, COI, and UPA genes (Sherwood et al., 2010). Abbott (1999) did not include any Ramicrusta species in the marine flora or the Hawaiian Islands, but did note that Hawaiian members of the Peyssonneliales are commonly found without reproductive structures which poses an additional challenge for identification and phylogenetic determination of these cryptic species. The previously undocumented presence of Ramicrusta in Hawai‘i over the past two decades highlights the need for accurate taxonomic and phylogenetic assessments to be incorporated into marine monitoring. As climate change continues to affect coral reefs, reef communities may respond and shift—additional macroalgal species, which are rare or cryptic and were previously overlooked, may become more abundant. For example, the fleshy red alga, Chondria tumulosa may have been a sparse and cryptic species at Pearl and Hermes Atoll in the Northwestern Hawaiian Islands that increased in abundance and spread rapidly after changes in ecosystem conditions (Sherwood et al., 2020a). Changes in nutrient concentrations (Stimson, 2015), water temperature (Boudouresque and Verlaque, 2010; Dijkstra et al., 2019), and greenhouse gas concentrations (Fernández de la Hoz et al., 2019) have been linked to coral-macroalgal phase shifts on reefs, range shifts in macroalgal distribution, and changes in macroalgal abundances.
The distance between our west Hawai‘i study area and Lehua Island where R. hawaiiensis was first described (Sherwood et al., 2021c) is over 500 km and this vast distance suggests a unique and likely incomplete geographical pattern with a yet-to-be-determined distribution vector. Ramicrusta hawaiiensis was described at Lehua Island at 49 – 50 m depths in the mesophotic zone (Sherwood et al., 2021c); however, DAR-WHAP and SKI surveys in west Hawai‘i documented Ramicrusta growing at depths of 3 – 18 m. The habitats investigated in west Hawai‘i represent strikingly different habitat types than those described by Sherwood et al. (2021c) at Lehua Island. Different environmental and oceanographic characteristics, such as irradiance and water temperature might impact the growth rate, pigmentation (Figure 3), morphology, and abundance of this alga. The slight genetic differences among specimens from the mesophotic depths at Lehua Island and the shallow subtidal zone of west Hawai‘i Island suggest that Ramicrusta has affinities with multiple ancestral populations.The expanded distributional data presented in this paper make clear that further geographic inquiry at an archipelagic scale is needed to determine this alga’s overall distribution. This is particularly relevant since the presence of Ramicrusta was readily detectable in previously acquired benthic imagery. Ramicrusta might be present but overlooked or misidentified in other areas of the Hawaiian Archipelago, perhaps even the Northwestern Hawaiian Islands, but voucher specimens are needed.
Nondescript macroalgal thalli that are cryptic, both in situ and taxonomically, pose a challenge to marine scientists. Although SfM photogrammetry is a powerful survey tool that provides detailed information about structural habitat complexity, the accuracy of identification of macroalgal community composition to the species level is low unless the SfM surveys are conducted in concert with voucher collections. Researchers adding annotations to reef mosaic or photo-quadrat images cannot confidently assign algal species names and in many cases, not even genus names in some cases, to crustose, filamentous, and foliose algae without examining anatomical and genetic traits. Macroalgal members of the reef community, even those with similar morphologies, may differ in life history, phenology, and physiology, emphasizing the importance of identification and monitoring of individual species in order to fully understand reef ecology (Stuercke & McDermid, 2004). For instance, in mesophotic depths in the Hawaiian Archipelago, accurate identification of frondose green macroalgae with similar, simple morphologies required genetic analyses to distinguish the four species belonging to two separate genera, Ulva and Umbraulva (Spalding et al., 2016). Future studies focused on DNA sequencing of additional shallow water and mesophotic specimens of Ramicrusta should be performed to verify the distribution and range of Ramicrusta species in the Hawaiian Archipelago to corroborate the ecological findings presented in this study. Ramicrusta specimens in west Hawai‘i did not show evidence of cystocarps or sporangia, enhancing the taxonomic enigma of a cryptic species, and thus, additional studies are needed to document the reproductive structures and strategies of R. hawaiiensis.
Ramicrusta species occupy a diverse range of habitats globally. Atlantic and Caribbean specimens have been observed at depths similar to those reported in west Hawai‘i: 4.5 – 15.5 m (Hollister et al., 2021), < 9 m depth (Edmunds et al., 2019), 5 – 30 m (Williams and García-Sais, 2020). The overall vertical distribution across reef landscapes of the Ramicrusta species in Guam is broad—from intertidal areas to lower mesophotic zones (Mills and Schils, 2021). The new depth distribution of R. hawaiiensis described here (mesophotic to shallow subtidal) corroborates this pattern of broad vertical distribution. Field observations made in 2020 showed that Ramicrusta presence was more notable at sites with higher basalt cover and in exposed areas as opposed to major bays. Some morphological and anatomical traits of Ramicrusta, e.g., the raised margins and thick calcification may give this species a competitive edge in terms of growth, overgrowth of corals, and resistance to herbivory (Steneck, 1985; Steneck et al., 1991). The rebound of Ramicrusta after strong hurricanes in the Virgin Islands (Williams and Edmunds, 2021) suggests that small patches survived and grew quickly. In addition, four newly described species of Ramicrusta in Guam were reported in reef environments that have previously experienced stressor events, such as coral bleaching, or that were exposed to rapid changes in salinity, temperature, nutrients, and terrestrial run-off (Mills and Schils, 2021). In Puerto Rico, large patches (>100 m2) of Ramicrusta textilis have been observed overgrowing reef substrata (Ballantine et al., 2011). These patches may be similar to those observed in west Hawai‘i, especially at site 178 in the 2020 SKI dataset (Figure 10). Larger unconsolidated patches (100 cm2) were observed in several areas, particularly in the southern section of west Hawai‘i during 2020 SKI field observations, and may represent areas of significant overgrowth of the reef substrata and live coral by R. hawaiiensis. Perhaps some Ramicrusta species are ideal competitors with wide tolerances to variations in depth, light, temperature, and water movement, and when given an ecological window of opportunity after a disturbance, the crusts may exhibit a competitive edge.
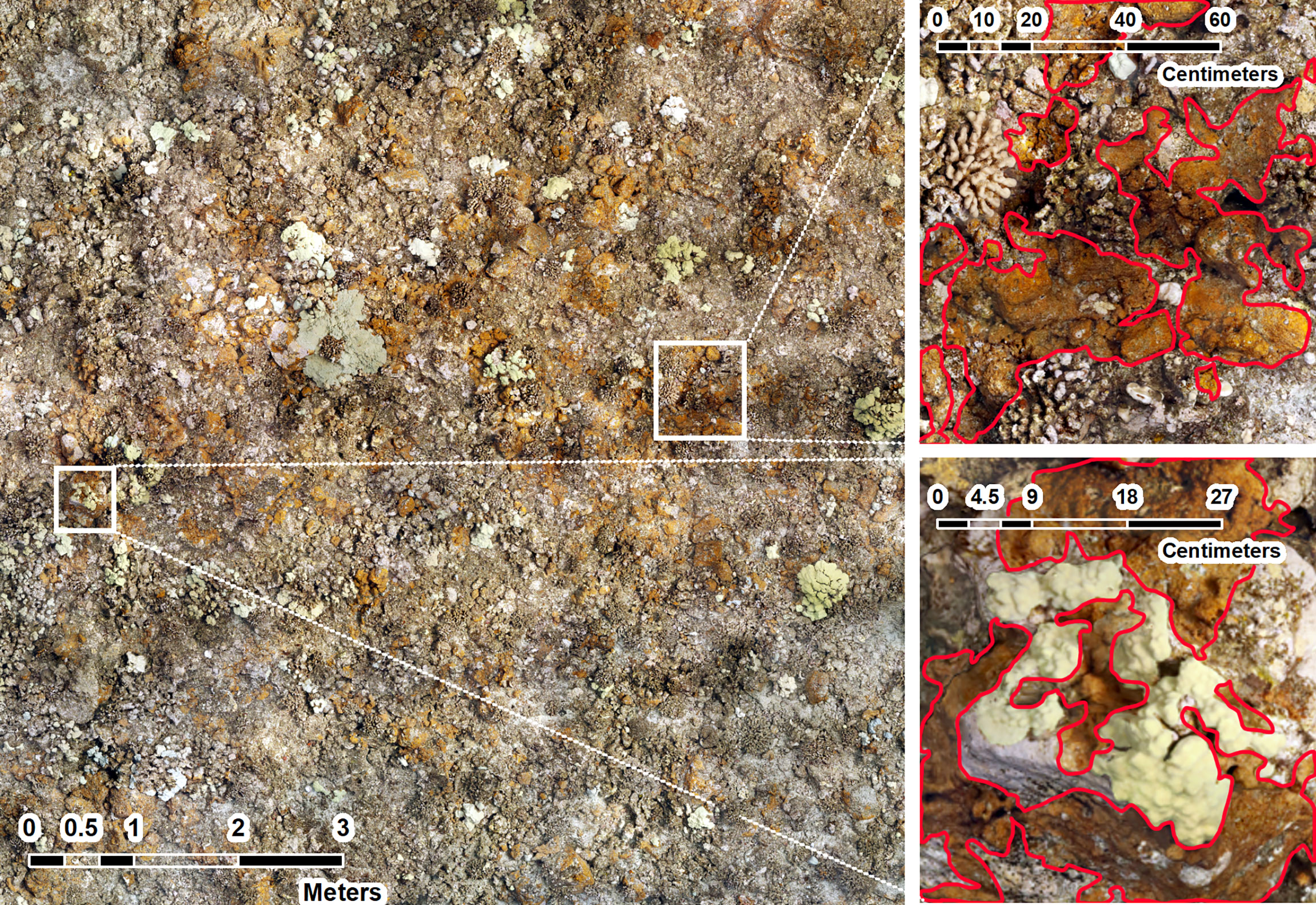
Figure 10 Image of 10 m x 10 m orthomosaic in 2020 at SKI site 178 (Left). This site had the highest observed Ramicrusta benthic cover = 37.4% ± 9.17 SE. Ramicrusta is distributed across this SfM mosaic as the rusty-orange encrusting algae throughout the plot (Left) and outlined in red in the images on the right. Ramicrusta can be seen overgrowing a Porites coral in the image on the bottom right.
The expanded geographic distribution of this algal species reported in this study, and the ability of Ramicrusta hawaiiensis to overgrow living coral (Figure 3), highlight the need for continued monitoring and investigation of potential management strategies of the Ramicrusta-coral interaction. Herbivory and nutrients can affect colonization of crustose coralline algae, and thus shape the benthic community structure (Belliveau and Paul, 2002), perhaps these factors also affect peyssonnelid crust recruitment and growth. Flat, hard, crustose morphology may confound some grazers, and crust anatomy with meristematic regions buried under layers of cells may provide some protection from herbivory (Littler, 1976; Littler et al., 1983a, Littler et al., 1983b). However, herbivory has been suggested as a potential key in managing Ramicrusta and PAC outbreaks on coral reefs (Williams and García-Sais, 2020). Herbivory on Ramicrusta and PAC by the sea urchin, Diadema antillarum has been documented in the Caribbean (Ruiz, 2015; Stockton and Edmunds, 2021). Diadema antillarum has been found to be the only consumer of Ramicrusta and PAC on Caribbean reefs, but more information is still needed on the predation of Ramicrusta by other herbivores (Williams, 2022). Previous studies have suggested that a unique microbiome on Ramicrusta specimens may deter coral settlement and cause the macroalga to be unpalatable to herbivorous fishes and urchin species (Wilson et al., 2020; Williams, 2022). No notable herbivore predation events on Ramicrusta were observed during the recent 2020 WHAP or SKI field surveys, and no evidence of scraping or abrasion typical of parrotfish (Scaridae) predation was found in the photo imagery. However, further investigation into predation by local urchin and fish species in west Hawai‘i is merited.
Long-term dynamics of Ramicrusta in west Hawai’i
The Ramicrusta patches photographed in 2003 were lumped together with other crustose species in surveys by the long-term monitoring biologists; however, the divers’ decades-long ecological data stored as photos, mosaic images, and vouchers are rare treasures for phycologists and marine ecologists working to understand ecosystem dynamics. Ramicrusta hawaiiensis may have been in Hawai‘i before 2003 as cryptic and unnoticed crusts; however, as ocean conditions changed and especially after the mass bleaching event in 2015 (Kramer et al., 2016), Hawaiian Ramicrusta may have gained a competitive advantage to expand its distribution, increase its abundance, or gain enhanced visibility to divers.
Temporal trends suggest that in west Hawai‘i, there is a correlation between warmer years (with positive SSTA twelve months prior to surveying) and higher cover of Ramicrusta. Antecedent temperature regimens may affect the Ramicrusta population as has been documented for other algal species in the Hawaiian Islands (Doty, 1971; Glenn et al., 1990). In a recent study of intertidal communities in the Hawaiian Islands, a significant positive relationship was found between SST and the abundance of three dominant algal taxa: turf algae, brown crustose algae, and a fleshy brown, Turbinaria ornata (Phaeophyceae, Fucales) (Ward, 2022). Increased intertidal community diversity in response to SST was best explained by SST conditions in the ten months preceding sampling (Ward, 2022). A more detailed analysis of prior environmental conditions at various time intervals are needed to better understand the influence of multiple factors, i.e., temperature, solar irradiance, water motion, and nutrient levels, on the benthic community along the coast of west Hawai‘i. In addition, trends in the interaction variable (SSTA*latitude) suggest that there is a negative correlation between Ramicrusta and SSTA together particularly in the northern region of west Hawai‘i Island. Laboratory-based experiments would help to reveal the effects of temperature on the growth and reproduction of R. hawaiiensis.
This study documents Ramicrusta far south of the location of its original description in the Hawaiian Archipelago. Its full geographic range and vertical distribution remain unknown. As coral reef communities in the Hawaiian Islands shift and evolve, accurate taxonomic identification of macroalgae to the species level, not just as “crusts” or ambiguous group names, e.g., CCA, PAC, and CCRA, will be crucial to scientific advances in the understanding of reef community dynamics. Now with the recognition of R. hawaiiensis and a better understanding of its ecology, future assessments can track Ramicrusta in the Hawaiian Islands and support efforts to preserve and protect coral reef resources.
Data availability statement
The datasets presented in this study can be found in online repositories. The names of the repository/repositories and accession number(s) can be found in the article/Supplementary Material.
Author contributions
KM with the help of BG and RK conceived and developed this study; BG, ML, and AP collected the samples; RK and JR conducted the laboratory work; BG, RK, AP, and QA performed the data analyses. KM, BG, and RK wrote the manuscript with contributions from AP, ML, SF, and JR. All authors edited the manuscript before submission. All authors contributed to the article and approved the submitted version.
Funding
The monitoring surveys for the WHAP data were funded in part by the Division of Aquatic Resources, the Research Corporation of the University of Hawai‘i, and the NOAA Coral Reef Conservation Program grant awards NA19NOS4820051 and NA21NOS4820017. Base SfM mosaic imagery from the South Kona Intensive Reef Survey used in the study was supported by the Lenfest Ocean Program and the Dorrance Family Foundation. This study was partially funded by NSF grant DEB-1754504. RK is grateful to the UL Lafayette Graduate Student Organization for partially funding the cost of research supplies.
Acknowledgments
We thank everyone who helped combine the diverse methods and data sources used in this project: ʻAʻohe hana nui ke alu ʻia. No task is too great when working together. We acknowledge Dr. Tom Pesacreta at the University of Louisiana at Lafayette Microscopy Center for the continuous support and use of the Scios 2 Dual Beam Focused Ion Beam scanning electron microscope, and Dr. Sophie Plouviez for overseeing the use and maintenance of the ABI Model 3130xl Genetic Analyzer at the University of Louisiana at Lafayette. We deeply appreciate the support from NSF research grant DEB-1754504. We thank Rebecca Ostertag (UH Hilo), John Stimson (UH Mānoa), and DAR reviewers (Chris Teague and Ryan Okano) for reading and improving our manuscript. We dedicate our paper to Professor Bernabé Santelices, a phycologist, marine ecologist, taxonomist, and friend extraordinario, who received his PhD from the University of Hawai’i, and taught for many years at the Pontificia Universidad Católica de Chile. His insight and body of work inspire us all to be better marine scientists.
Conflict of interest
The authors declare that the research was conducted in the absence of any commercial or financial relationships that could be construed as a potential conflict of interest.
Publisher’s note
All claims expressed in this article are solely those of the authors and do not necessarily represent those of their affiliated organizations, or those of the publisher, the editors and the reviewers. Any product that may be evaluated in this article, or claim that may be made by its manufacturer, is not guaranteed or endorsed by the publisher.
Supplementary material
The Supplementary Material for this article can be found online at: https://www.frontiersin.org/articles/10.3389/fmars.2022.1009471/full#supplementary-material
Supplementary Table 1 | List of taxa names, voucher numbers, localities, GenBank numbers and reference information for newly generated sequences and sequences downloaded from GenBank of taxa included in phylogenetic analyses. Newly generated sequences are shown in bold.
Supplementary Table 2 | Sequence divergence values (as a proportion of the number of base pair differences divided by the alignment length) for A) cytochrome oxidase I (COI) (664 bp) and B) ribulose-bisphosphate carboxylase (rbcL) (1,371 bp).
References
Arif S., Graham N. A., Wilson S., MacNeil M. A. (2022). Causal drivers of climate-mediated coral reef regime shifts. Ecosphere 13, e3956. doi: 10.1002/ecs2.3956
Asner G. P., Vaughn N., Grady B. W., Foo S. A., Anand H., Carlson R. R., et al. (2021). Regional reef fish survey design and scaling using high-resolution mapping and analysis. Front. Mar. Sci. 8. doi: 10.3389/fmars.2021.683184
Ballantine D. L., Athanasiadis A., Ruiz H. (2011). Notes on the benthic marine algae of Puerto Rico. X. Additions to the flora. Bot. Mar. 54, 293–302. doi: 10.1515/bot.2011.039
Ballantine D., Ruiz H. (2011). Metapeyssonnelia milleporoides, a new species of coral-killing red alga (Peyssonneliaceae) from Puerto Rico, Caribbean Sea. Bot. Mar. 54, 47–51. doi: 10.1515/bot.2011.003
Ballantine D. L., Ruiz H., Lozada-Troche C., Norris J. N. (2016). The genus Ramicrusta (Peyssonneliales, Rhodophyta) in the Caribbean Sea, including Ramicrusta bonairensis sp. nov. and Ramicrusta monensis sp. nov. Bot. Mar. 56, 417–431. doi: 10.1515/bot-2016-0086
Bayley D. T., Mogg A. O. (2020). A protocol for the large-scale analysis of reefs using structure from motion photogrammetry. Methods Ecol. Evol. 11, 1410–1420. doi: 10.1111/2041-210X.13476
Beijbom O., Edmunds P. J., Roelfsema C., Smith J., Kline D. I., Neal B. P., et al. (2015). Towards automated annotation of benthic survey images: Variability of human experts and operational modes of automation. PloS One 10 (7), e0130312. doi: 10.1371/journal.pone.0130312
Belliveau S. A., Paul V. J. (2002). Effects of herbivory and nutrients on the early colonization of crustose coralline and fleshy algae. Mar. Ecol. Prog. Ser. 232, 105–114. doi: 10.3354/meps232105
Boudouresque C. F., Verlaque M. J. (2010). “Is global warming involved in the success of seaweed introductions in the Mediterranean Sea?,” in Seaweeds and their role in globally changing environments. Eds. Israel A., Einav R., Seckbach J. (Dordrecht: Springer), 31–50.
Bramanti L., Lasker H., Edmunds P. J. (2017). An encrusting peyssonnelid preempts vacant space and overgrows corals in St. John, US Virgin Islands. Reef Encounter 32, 68–70.
Bruno J. F., Sweatman H., Precht W. F., Selig E. R., Schutte V. G. W. (2009). Assessing evidence of phase shifts from coral to macroalgal dominance on coral reefs. Ecology 90, 1478–1484. doi: 10.1890/08-1781.1
Burns J. H. R., Delparte D., Gates R. D., Takabayashi M. (2015). Integrating structure-from-motion photogrammetry with geospatial software as a novel technique for quantifying 3D ecological characteristics of coral reefs. PeerJ 3, e1077. doi: 10.7717/peerj.1077
Carlson R. R., Foo S. A., Asner G. P. (2019). Land use impacts on coral reef health: A ridge-to-reef perspective. Front. Mar. Sci. 6. doi: 10.3389/fmars.2019.00562
Deinhart M. E., Mills M. S., Schils T. (2022). Community assessment of crustose calcifying red algae as coral recruitment substrates. PLoS ONE 17 (7), e0271438. doi: 10.1371/journal.pone.0271438
Dijkstra J. A., Litterer A., Mello K., O’Brien B. S., Rzhanov Y. (2019). Temperature, phenology, and turf macroalgae drive seascape change: Connections to mid-trophic level species. Ecosphere 10 (11), e02923. doi: 10.1002/ecs2.2923
Dixon K. R. (2018). “Peyssonneliales,” in Algae of Australia: Marine benthic algae of north-western Australia. 2. Red lgae. Ed. Huisman J. M. (Canberra & Melbourne: ABRS & CSIRO Publishing), 208–244.
Dixon K., Saunders G. (2013). DNA barcoding and phylogenetics of Ramicrusta and Incendia gen. nov., two early diverging lineages of the Peyssonneliaceae (Rhodophyta). Phycologia 52, 82–108. doi: 10.2216/12-62.1
Donovan M. K., Burkepile D. E., Kratochwill C., Shlesinger T., Sully S., Oliver T. A., et al. (2021). Local conditions magnify coral loss after marine heatwaves. Science 372, 977–980. doi: 10.1126/science.abd9464
Donovan M. K., Friedlander A. M., Lecky J., Jouffray J. B., Williams G. J., Wedding L. M., et al. (2018). Combining fish and benthic communities into multiple regimes reveals complex reef dynamics. Sci. Rep. 8, 1–11. doi: 10.1038/s41598-018-35057-4
Doty M. (1971). Antecedent event influence on benthic marine algal standing crops in Hawaii. J. Exp. Mar. Biol. Ecol. 6, 161–166. doi: 10.1016/0022-0981(71)90015-3
Eckrich C. E., Engel M. S. (2013). Coral overgrowth by an encrusting red alga (Ramicrusta sp.): A threat to Caribbean reefs? Coral Reefs 32, 81–84. doi: 10.1007/s00338-012-0961-5
Eckrich C. E., Engel M. S., Peachey R. B. J. (2011). Crustose, calcareous algal bloom (Ramicrusta sp.) overgrowing scleractinian corals, gorgonians, a hydrocoral, sponges, and other algae in Lac Bay, Bonaire, Dutch Caribbean. Coral Reefs 30, 131–131. doi: 10.1007/s00338-010-0683-5
Edmunds P. J., Lasker H. R. (2016). Cryptic regime shift in benthic community structure on shallow reefs in St. John, US Virgin Islands. Mar. Ecol. Prog. Ser. 559, 1–12. doi: 10.3354/meps11900
Edmunds P. J., Zimmermann S. A., Bramanti L. (2019). A spatially aggressive peyssonnelid algal crust (PAC) threatens shallow coral reefs in St. John, US Virgin Islands. Coral Reefs 38, 1329–1341. doi: 10.1007/s00338-019-01846-0
Fernández de la Hoz C., Ramos E., Puente A., Juanes J. A. (2019). Climate change induced range shifts in seaweeds distributions in Europe. Mar. Envir. Res. 148, 1–11. doi: 10.1016/j.marenvres.2019.04.012
Foo S. A., Teague C. H., Asner G. P. (2022). Warming alters the relationship between benthic cover and herbivores on Hawaiian reefs. Front. Mar. Sci. 9. doi: 10.3389/fmars.2022.787314
Fredericq S., Arakaki N., Camacho O., Gabriel D., Krayesky D., Self-Krayesky O., et al (2014). A dynamic approach to the study of rhodoliths: A case study for the northwestern Gulf of Mexico. Cryptog., Algol. 35, 77–92.
Freshwater D. W., Rueness J. (1994). Phylogenetic relationships of some European Gelidium (Gelidiales, Rhodophyta) species, based on rbcL nucleotide sequence analysis. Phycologia 33, 187–194. doi: 10.2216/i0031-8884-33-3-187.1
Gavio B., Fredericq S. (2002). Grateloupia turuturu (Halymeniaceae, Rhodophyta) is the correct name of the non-native species in the Atlantic known as Grateloupia doryphora. Eur. J. Phycol. 37, 349–359. doi: 10.1017/S0967026202003839
Glenn E. P., Smith C. M., Doty M. S. (1990). Influence of antecedent water temperatures on standing crop of a Sargassum spp.-dominated reef flat in Hawaii. Mar. Biol. 105, 323–328. doi: 10.1007/BF01344302
Guiry M. D., Guiry G. M. (2022). AlgaeBase (National University of Ireland, Galway: World-wide electronic publication). Available at: https://www.algaebase.org.
Hollister K. J., Ennis R. S., Spalding H. L., Gabrielson P. W., Smith T. B. (2021). Caribbean corals exhibit species-specific differences in competitive abilities with an aggressive encrusting alga, Ramicrusta textilis. Coral Reefs 40, 1729–1740. doi: 10.1007/s00338-021-02172-0
Kim M. S., Kim S. Y., Nelson W. (2010). Symphyocladia lithophila sp. nov. (Rhodomelaceae, Ceramiales), a new Korean red algal species based on morphology and rbcL sequences. Bot. Mar. 53, 233–241. doi: 10.1515/bot.2010.031
Kramer K., Cotton S., Lamson M., Walsh W. (2016). Bleaching and catastrophic mortality ofreef-building corals along west Hawai‘i Island: Findings and future directions. Proceedings of the 13th international coral reef symposium, Honolulu: 219–230.
Krayesky D. M., Norris J. N., Gabrielson P. W., Gabriel D., Fredericq S. (2009). A new order of red algae based on the Peyssonneliaceae, with an evaluation of the ordinal classification of the Florideophyceae (Rhodophyta). Proc. Biol. Soc Wash. 122, 364–391. doi: 10.2988/08-43.1
Kumar S., Stecher G., Li M., Knyaz C., Tamura K. (2018). MEGA X: Molecular evolutionary genetics analysis across computing platforms. Mol. Biol. Evol. 35, 1547–1549. doi: 10.1093/molbev/msy096
Lanfear R., Frandsen P. B., Wright A. M., Senfeld T., Calcott B. (2017). PartitionFinder 2: New methods for selecting partitioned models of evolution for molecular and morphological phylogenetic analyses. Mol. Biol. Evol. 34, 772–773. doi: 10.1093/molbev/msw260
Lin S. M., Fredericq S., Hommersand M. H. (2001). Systematics of the Delesseriaceae (Ceramiales, Rhodophyta) based on large subunit rDNA and rbcL sequences, including the Phycodryoideae, subfam. nov. J. Phycol. 37, 881–899. doi: 10.1046/j.1529-8817.2001.01012.x
Littler M. M., Littler D. S. (1984). Models of tropical reef biogenesis: The contribution of algae. Prog. Phycological Res. 3, 323–364.
Littler M. M., Littler D. S., Taylor P. R. (1983a). Evolutionary strategies in a tropical barrier reef system: Functional-form groups of marine macroalgae. J. Phycol 19, 229–237. doi: 10.1111/j.0022-3646.1983.00229.x
Littler M. M., Taylor P. R., Littler D. S. (1983b). Algal resistance to herbivory on a Caribbean barrier reef. Coral Reefs 2, 111–118. doi: 10.1007/BF02395281
Manghisi A., Miladi R., Minicante S. A., Genovese G., Le Gall L., Abdelkafi S., et al. (2019). DNA barcoding sheds light on novel records in the Tunisian red algal flora. Cryptogam. Algol. 40, 5–27. doi: 10.5252/cryptogamie-algologie2019v40a3
Maynard J., Van Hooidonk R., Eakin C. M., Puotinen M., Garren M., Williams G., et al. (2015). Projections of climate conditions that increase coral disease susceptibility and pathogen abundance and virulence. Nat. Clim. Change 5, 688–694. doi: 10.1038/nclimate2625
McCook L., Jompa J., Diaz-Pulido G. (2001). Competition between corals and algae on coral reefs: A review of evidence and mechanisms. Coral Reefs 19, 400–417. doi: 10.1007/s003380000129
McManus J. W., Polsenberg J. F. (2004). Coral–algal phase shifts on coral reefs: Ecological and environmental aspects. Prog. Oceanogr. 60, 263–279. doi: 10.1016/j.pocean.2004.02.014
Miller M. A., Pfeiffer W., Schwartz T. (2010). Creating the CIPRES science gateway for inference of large phylogenetic trees. In Proceedings of the Gateway Computing Environments Workshop (GCE). Institute of Electrical and Electronics Engineers, Piscataway, NJ. p. 11572–8. doi: 10.1109/GCE.2010.5676129
Mills M. S., Schils T. (2021). The habitat-modifying red alga Ramicrusta on Pacific reefs: A new generic record for the tropical northwestern Pacific and the description of four new species from Guam. PloS One 16, e0259336. doi: 10.1371/journal.pone.0259336
Nieder C., Chen P. C., Allen Chen C., Liu S. L. (2019). New record of the encrusting alga Ramicrusta textilis overgrowing corals in the lagoon of Dongsha Atoll, South China Sea. Bull. Mar. Sci. 95, 459–462. doi: 10.5343/bms.2019.0010
NOAA Coral Reef Watch (2018)Updated daily. In: NOAA Coral reef watch version 3.1 daily global 5km satellite coral bleaching degree heating week product, Jun. 3, 2013-Jun. 2, 2014 (College Park, Maryland, USA: NOAA Coral Reef Watch) (Accessed 2020-09-01).
Norström A. V., Nyström M., Lokrantz J., Folke C. (2009). Alternative states on coral reefs: Beyond coral–macroalgal phase shifts. Mar. Ecol. Prog. Ser. 376, 295–306. doi: 10.3354/meps07815
Paiano M. O., Huisman J. M., Cabrera F. P., Spalding H. L., Kosaki R. K., Sherwood A. R. (2020). Haraldiophyllum hawaiiense sp. nov. (Delesseriaceae, Rhodophyta): A new mesophotic genus record for the Hawaiian islands. Algae 35, 337–347. doi: 10.4490/algae.2020.35.11.5
Parsons M. L., Walsh W. J., Settlemier C. J., White D. J., Ballauer J. M., Ayotte P. M., et al. (2008). A multivariate assessment of the coral ecosystem health of two embayments on the lee of the island of Hawai‘i. Mar. Poll. Bull. 56, 1138–1149. doi: 10.1016/j.marpolbul.2008.03.004
Pestana E. M. S., Lyra G. M., Santos G. N., Santos C. C., Cassano V., Nunes J. M. C. (2020). Integrative approach reveals underestimated Peyssonneliales diversity in Brazil: Registering the first occurrence of Ramicrusta and Incendia, with the description of three new species. Phytotaxa 439, 39–55. doi: 10.11646/phytotaxa.439.1.2
Pueschel C. M., Saunders G. W. (2009). Ramicrusta textilis sp. nov. (Peyssonneliaceae, Rhodophyta), an anatomically complex Caribbean alga that overgrows corals. Phycologia 48, 480–491. doi: 10.2216/09-04.1
Richards J. L., Gabrielson P. W., Fredericq S. (2014). New insights into the genus Lithophyllum (Lithophylloideae, Corallinaceae, Corallinales) from offshore the NW gulf of mexico. Phytotaxa 190, 162–175. doi: 10.11646/phytotaxa.190.1.11
Ruiz H. (2015). “The ecology of the red algae Ramicrusta textilis, its dynamic with corals and the evaluation of possible management strategies to minimize its threat to coral reefs around Puerto Rico,” in Final report to Puerto Rico department of natural and environmental resources.
Saunders G. W. (2005). Applying DNA barcoding to red macroalgae: A preliminary appraisal holds promise for future applications. Philos. Trans. R. Soc Lond. B Biol. Sci. 360, 1879–1888. doi: 10.1098/rstb.2005.1719
Sherwood A. R., Cabrera F. P., Spalding H. L., Alvarado E. A., Smith C. M., Hauk B. B., et al. (2021a). Biodiversity of Hawaiian peyssonneliales (Peyssonneliaceae, Rhodophyta): New species in the genera Incendia and Seiria. Phytotaxa 524, 14–26. doi: 10.11646/phytotaxa.524.1.2
Sherwood A. R., Huisman J. M., Paiano M. O., Williams T. M., Kosaki R. K., Smith C. M., et al. (2020a). Taxonomic determination of the cryptogenic red alga, Chondria tumulosa sp. nov., (Rhodomelaceae, Rhodophyta) from Papahānaumokuākea Marine National Monument, Hawai‘i, USA: A new species displaying invasive characteristics. PloS One 15 (7), e0234358. doi: 10.1371/journal.pone.0234358
Sherwood A. R., Kurihara A., Conklin K. Y., Sauvage T., Presting G. G. (2010). The Hawaiian Rhodophyta biodiversity survey, (2006-2010): A summary of principal findings. BMC Plant Biol. 10 (1), 1–29. doi: 10.1186/1471-2229-10-258
Sherwood A. R., Paiano M. O., Cabrera F. P., Spalding H. L., Hauk B. B., Kosaki R. K. (2021b). Ethelia hawaiiensis (Etheliaceae, Rhodophyta), a new mesophotic marine alga from Manawai (Pearl and Hermes Atoll), Papahānaumokuākea Marine National Monument, Hawai'i. Pac. Sci. 75, 237–246. doi: 10.2984/75.2.6
Sherwood A. R., Paiano M. O., Spalding H. L., Kosaki R. K. (2020b). Biodiversity of Hawaiian Peyssonneliales (Rhodophyta): Sonderophycus copusii sp. nov., a new species from the Northwestern Hawaiian Islands. Algae 35, 145–155. doi: 10.4490/algae.2020.35.5.20
Sherwood A. R., Paiano M. O., Wade R. M., Cabrera F. C., Spalding H. L., Kosaki R. K. (2021c). Biodiversity of Hawaiian Peyssonneliales (Rhodophyta). 1. Two new species in the genus Ramicrusta from Lehua Island. Pac. Sci. 75, 185–195. doi: 10.2984/75.2.2
Smith T. B., Brandt M. E., Brandtneris V. W., Ennis R. S., Groves S. H., Habtes S., et al. (2019). “The United States Virgin Islands,” in Mesophotic coral ecosystems. Ccoral reefs of the world, vol. 12 . Eds. Loya Y., Puglise K., Bridge T. (Cham: Springer). doi: 10.1007/978-3-319-92735-0_8
Spalding H. L., Conklin K. Y., Smith C. M., O'Kelly C. J., Sherwood A. R. (2016). New Ulvaceae (Ulvophyceae, Chlorophyta) from mesophotic ecosystems across the Hawaiian Archipelago. J. Phycology 52 (1), 40–53. doi: 10.1111/jpy.12375
Stamatakis A. (2014). RAxML version 8: A tool for phylogenetic analysis and post-analysis of large phylogenies. Bioinformatics 30, 1312–1313. doi: 10.1093/bioinformatics/btu033
Steneck R. S. (1985). “Adaptations of crustose coralline algae to herbivory: Patterns in space and time,” in Paleoalgology. Eds. Toomey D. F., Nitecki M. H. (Berlin, Heidelberg: Springer), 352–366. doi: 10.1007/978-3-642-70355-3_29
Steneck R. S., Hacker S. D., Dethier M. D. (1991). Mechanisms of competitive dominance between crustose coralline algae: An herbivore-mediated competitive reversal. Ecology 72 (3), 938–950. http://links.jstor.org/sici?sici=0012-9658%28199106%2972%3A3%3C938%3AMOCDBC%3E2.0.
Stimson J. (2015). Long-term record of nutrient concentrations in Kaneohe Bay, Oahu, Hawaii, and its relevance to onset and end of a phase shift involving an indigenous alga, Dictyosphaeria cavernosa. Pac. Sci. 69, 319–339.
Stockton L., Edmunds P. J. (2021). Spatially aggressive peyssonnelid algal crusts (PAC) constrain coral recruitment to Diadema grazing halos on a shallow Caribbean reef. J. Exp. Mar. Biol. Ecol. 541, 151569. doi: 10.1016/j.jembe.2021.151569
Stuercke B., McDermid K. J. (2004). Variation in algal turf species composition and abundance on two Hawaiian shallow subtidal reefs. Cryptogamie Algologie 25 (4), 353–365. doi: 10.1111/j.0022-3646.2003.03906001_158.x
Thompson J. D., Higgins D. G., Gibson T. J. (1994). CLUSTAL W: improving the sensitivity of progressive multiple sequence alignment through sequence weighting, position-specific gap penalties and weight matrix choice. Nucleic Acids Res. 22, 4673–4680. doi: 10.1093/nar/22.22.4673
Tissot B. N., Walsh W., Hallacher L. E. (2004). Evaluating the effectiveness of a marine reserve network in Hawaii to increase the productivity of an aquarium fishery. Pac. Sci. 58 (2), 175–188. doi: 10.1353/psc.2004.0024
Vaidya G., Lohman D. J., Meier R. (2011). SequenceMatrix: Concatenation software for the fast assembly of multi-gene datasets with character set and codon information. Cladistics 27, 171–180. doi: 10.1111/j.1096-0031.2010.00329.x
Ward R. (2022). Spatial variation in sea surface temperature (SST) and antecedent SST conditions influence community structure in the Hawaiian intertidal (Hilo: Master’s Thesis, Univ. of Hawaii), 47.
Williams S. M. (2022). The reduction of harmful algae on Caribbean coral reefs through the reintroduction of a keystone herbivore, the long-spined sea urchin Diadema antillarum. Restor. Ecol. 30, e13475. doi: 10.1111/rec.13475
Williams I. D., Couch C. S., Beijbom O., Oliver T. A., Vargas-Angel B., Schumacher B. D., et al. (2019). Leveraging automated image analysis tools to transform our capacity to assess status and trends of coral reefs. Front. Mar. Sci. 6. doi: 10.3389/fmars.2019.00222
Williams M. K., Edmunds P. J. (2021). Reconciling slow linear growth and equivocal competitive ability with rapid spread of peyssonnelid algae in the Caribbean. Coral Reefs 40, 473–483. doi: 10.1007/s00338-021-02052-7
Williams S. M., García-Sais J. R. (2020). A potential new threat on the coral reefs of Puerto Rico: The recent emergence of Ramicrusta spp. Mar. Ecol. 41, e12592. doi: 10.1111/maec.12592
Wilson B., Fan C. M., Edmunds P. J. (2020). An unusual microbiome characterises a spatially-aggressive crustose algae rapidly overgrowing shallow Caribbean reefs. Sci. Rep. 10, 1–13. doi: 10.1038/s41598-020-76204-0
Keywords: coral reef community, competition, crustose calcifying red algae (CCRA), phylogenetics, Peyssonneliaceae, peyssonnelid algal crusts (PAC), scanning electron microscopy, sea surface temperature (SST)
Citation: Grady BW, Kittle RP III, Pugh A, Lamson MR, Richards JL, Fredericq S, McDermid KJ, Allen Q and Asner GP (2022) Long-term ecological monitoring of reefs on Hawai’i Island (2003-2020): Characterization of a common cryptic crust, Ramicrusta hawaiiensis (Peyssonneliales, Rhodophyta). Front. Mar. Sci. 9:1009471. doi: 10.3389/fmars.2022.1009471
Received: 01 August 2022; Accepted: 10 October 2022;
Published: 31 October 2022.
Edited by:
Iva Popovic, The University of Queensland, AustraliaReviewed by:
Alexandra Ordonez Alvarez, Southern Cross University, AustraliaRachael Wade, University of British Columbia, Canada
Alison Sherwood, University of Hawaii at Manoa, United States
Copyright © 2022 Grady, Kittle, Pugh, Lamson, Richards, Fredericq, McDermid, Allen and Asner. This is an open-access article distributed under the terms of the Creative Commons Attribution License (CC BY). The use, distribution or reproduction in other forums is permitted, provided the original author(s) and the copyright owner(s) are credited and that the original publication in this journal is cited, in accordance with accepted academic practice. No use, distribution or reproduction is permitted which does not comply with these terms.
*Correspondence: Karla J. McDermid, bWNkZXJtaWRAaGF3YWlpLmVkdQ==
†Present address: Bryant W. Grady, The Nature Conservancy Hawai’i Island Marine Program, Kailua-Kona, HI, United States