- 1Scottish Association for Marine Science, Oban, United Kingdom
- 2Physics Department, University of Strathclyde, Glasgow, United Kingdom
- 3Department of Arctic and Marine Biology UiT - The Arctic University of Norway, Tromsø, Norway
- 4Akvaplan-niva, Tromsø, Norway
- 5Arctic Biology Department, University Centre in Svalbard, Tromsø, Norway
- 6Ecosystem Processes Group, Institute of Marine Research, Tromsø, Norway
On Arctic shelves, where primary production occurs in both the pelagic and sympagic (ice-associated) habitats, sympagic organic material (OM) can constitute a disproportionate fraction of benthic diets due to higher sinking rates and lower grazing pressure than pelagic OM. Less documented is how sympagic OM assimilation across feeding guilds varies seasonally and in relation to sea ice duation. We therefore investigated the relative abundance of sympagic vs pelagic OM in Barents Sea shelf megabenthos in the summer and winter of 2018 and 2019, from 10 stations where sea ice duration ranged from 0 to 245 days per year. We use highly branched isoprenoids, which are lipid biomarkers produced with distinct molecular structures by diatoms in sea ice and the water column, to determine the ratio of sympagic-to-pelagic OM assimilated by benthic organisms. From 114 samples of 25 taxa analysed, we found that the proportion of sympagic OM assimilated ranged from 0.4% to 95.8% and correlated strongly (r2 = 0.754) with the duration of sea ice cover. The effect of sea ice duration was more evident in fauna collected during summer than winter, indicating that sympagic signals are more evident in the summer than in the winter at higher latitudes. Our data show that sympagic production can supply a high fraction of carbon for Barents Sea benthos, although this is highly variable and likely dependent on availability and patchiness of sympagic OM deposition. These results are comparable to similar studies conducted on benthos in the Pacific Arctic and highlight the variable importance of sympagic OM in the seasonal ice zone of Arctic inflow shelves, which are the Arctic regions with highest rates of sea ice loss.
1 Introduction
Benthic food webs on offshore Arctic shelves are primarily fuelled by organic matter produced in the upper water column (Ambrose and Renaud, 1995). In sea ice covered waters far from terrestrial inputs, this production can be either ice-associated (sympagic) or pelagic, with sympagic production accounting for 3-57% of annual production (Gosselin et al., 1997). Significant declines in the extent, thickness, and persistence of sea ice are expected to impact the timing and magnitude of both sympagic and pelagic primary production (Wassmann et al., 2020), but there is little agreement on what these impacts will be. In the Barents Sea, the most productive Arctic shelf sea, rates of sea ice loss are the largest observed for the entire Arctic (Wassmann and Reigstad, 2011; Onarheim and Årthun, 2017). This marginal Arctic Sea is perennially ice-free in the south and seasonally covered by ice in the north (Jørgensen et al., 2015). This seasonal ice zone (SIZ) has a strong controlling effect on the primary production in the northern Barents Sea, which in turn affects the whole ecosystem, including the benthos (Cochrane et al., 2009). Understanding the contribution of both pelagic and sympagic primary production to benthic consumers is therefore central to improving our understanding of the ecosystem impacts of sea ice loss.
The benthos plays an important role in carbon flow through Arctic food webs, due to the tight sympagic-pelagic-benthic coupling on Arctic shelves (Grebmeier and Barry, 1991; Grebmeier et al., 2015). Carbon flow to the benthos is mostly driven by large pulses of spring production resulting in sedimentation of organic matter (OM) (Wassmann and Reigstad, 2011). Sympagic blooms inside the brine channel system of sea ice generally occur before pelagic blooms, and much of this production reaches the seafloor un-grazed due to rapid sinking and limited zooplankton grazing in early spring (Ambrose et al., 2005). This early season pulse of organic matter is readily utilised by the benthos and has been suggested to help fuel reproduction (Ambrose and Renaud, 1997; Renaud et al., 2007). The pelagic bloom occurs soon after the ice retreats and is associated with large sedimentation events, but the bloom is more susceptible to grazing by zooplankton and degradation in the water column, therefore reducing the quantity and quality of pelagic OM reaching the benthos (Olli et al., 2002; Reigstad et al., 2008).
The strong signal of sympagic OM detected in the benthos of the Pacific Arctic and Baffin Bay suggests a high contribution of this food source to benthic food webs (Koch et al., 2020a; Yunda-Guarin et al., 2020). However, in the Barents Sea, there is only sparse knowledge regarding the contribution of sympagic OM to benthic food webs: while sympagic OM has been detected in benthic organisms (McMahon et al., 2006; Søreide et al., 2013), little is known about the geographical and seasonal changes in sympagic and pelagic OM utilisation by the benthos. The contribution of sympagic OM to other consumers in the European Arctic is variable, but sometimes high, as inferred from trophic markers in zooplankton and sympagic fauna (Kohlbach et al., 2021a), polar cod (Kohlbach et al., 2017), and certain pinnipeds (Kunisch et al., 2021). This variable importance across food web components warrants a better understanding of the utilisation of sympagic OM by the Barents Sea benthos as an important food web component in overall energy flow (Sivel et al., 2021).
Trophic biomarkers provide a time-integrated signal of consumer diets and have been widely used to effectively track sympagic and pelagic carbon in the Arctic system (Tamelander et al., 2006; Søreide et al., 2013; Kohlbach et al., 2016). While stable isotope and fatty acid analysis are two commonly used biomarkers for this purpose, highly branched isoprenoids (HBIs) are a relatively novel biomarker which have the advantage of high source-specificity for sympagic and pelagic OM in the Arctic (Brown et al., 2014a; Brown et al., 2014b). HBIs are 25-carbon molecules produced by diatoms in both the sympagic and pelagic realms, and notably have different levels of saturation depending on the synthesising species (Belt, 2018). A small number of pan-Arctic sympagic diatoms belonging to the genera Haslea and Pleurosigma have been identified as synthesisers of the monounsaturated HBI IP25 (Ice Proxy with 25 carbons), which is only known to be produced by these ice algae (Brown et al., 2014c). Due to its high source specificity, IP25 has been used for tracking sympagic OM through Arctic ecosystems (Brown et al., 2014b; Limoges et al., 2018). Conveniently, the di-unsaturated HBI II is also only produced by sympagic diatoms, whereas the tri-unsaturated HBI III is produced by pelagic diatoms (see Figure S1 for structures of these molecules) (Belt, 2018). HBIs are stable molecules that are well-conserved though the food chain and have even been observed at the highest trophic level (e.g., in polar bears) (Brown et al., 2018).
There is no evidence of bioaccumulation of HBIs, but their turnover rates in Arctic consumers remain unclear. Depuration rates of HBIs are about one month, as experimentally suggested for invertebrates (Koch et al., 2020a), and — consistent with this finding — environmental observations suggest that HBI levels in organisms reflect seasonal cycles (Brown et al., 2014b). This makes them particularly appropriate for the challenge of tracking sympagic and pelagic OM in Arctic food webs (Brown et al., 2017a; Brown et al., 2018; Koch et al., 2020a; Yunda-Guarin et al., 2020). The ratio of sympagic HBIs (IP25 and HBI II) to a pelagic HBI (HBI III) in a consumer’s tissue – known as the H-print – has previously been used to estimate the carbon source partitioning in an organism’s diet (Brown and Belt, 2017). However, HBIs are produced by taxa which typically only constitute 1-5% of algal communities in terms of abundance (Brown et al., 2014c), and the target HBIs are sometimes either not present or at concentrations below the detection limit (Leu et al., 2020). In such cases, a reliable H-print cannot be calculated, and those samples cannot be further analysed, as in Yunda-Guarin et al. (2020), where around one third of the samples had to be discarded from analyses. Regardless, in both the shallow Pacific Arctic inflow shelves (Koch et al., 2020a) and deep Baffin Bay (Yunda-Guarin et al., 2020), HBI ratios have revealed a high proportion of sympagic OM assimilation in benthos under heavy sea ice cover.
This study aims to use HBI-based analysis to assess how much of the carbon assimilated by Barents Sea megabenthos originated from sympagic production. Results are evaluated over a spatial gradient of sea ice duration and during the summer and winter seasons. Feeding guilds, rather than individual taxa, are used to give an idea of how community function changes over this gradient. The natural gradient of sea ice duration in the Barents Sea is useful to test how ice cover can influence sympagic and pelagic OM utilisation by the benthos, while minimizing the influence of other environmental variables. To date, there are few studies of seasonal change in Arctic benthic food webs, especially in the winter period and in areas where sympagic production is high (Renaud et al., 2011; Kędra et al., 2012; Bridier et al., 2021). Investigating the seasonal change in sympagic OM utilisation will provide additional insights into its role in benthic food webs during the non-productive winter season. The research questions addressed in this paper are: (1) how does sympagic OM utilisation by the benthos vary spatially with different degrees of sea ice cover, (2) do organisms with different feeding strategies utilise sympagic and pelagic OM in different proportions, and (3) how does sympagic OM utilisation by the benthos change from the summer to winter season?
2 Methods
2.1 Sample collection and preparation
Samples were collected in January 2018 aboard the R/V Helmer Hanssen, in June 2018 aboard the RRS James Clark Ross, and in August 2018 and 2019 and December 2019 aboard the R/V Kronprins Haakon. Samples were collected in the central and northern Barents Sea in open water and within the seasonal ice zone using trawls and box cores, targeting megafaunal epibenthos (see Figure 1 and Table 1). Organisms were selected to cover a wide range of taxa and feeding guilds, and frozen for subsequent identification and analysis. For further information regarding seafloor composition, please refer to Freitas et al., 2020, De Freitas et al., 2022a and De Freitas et al., 2022b.
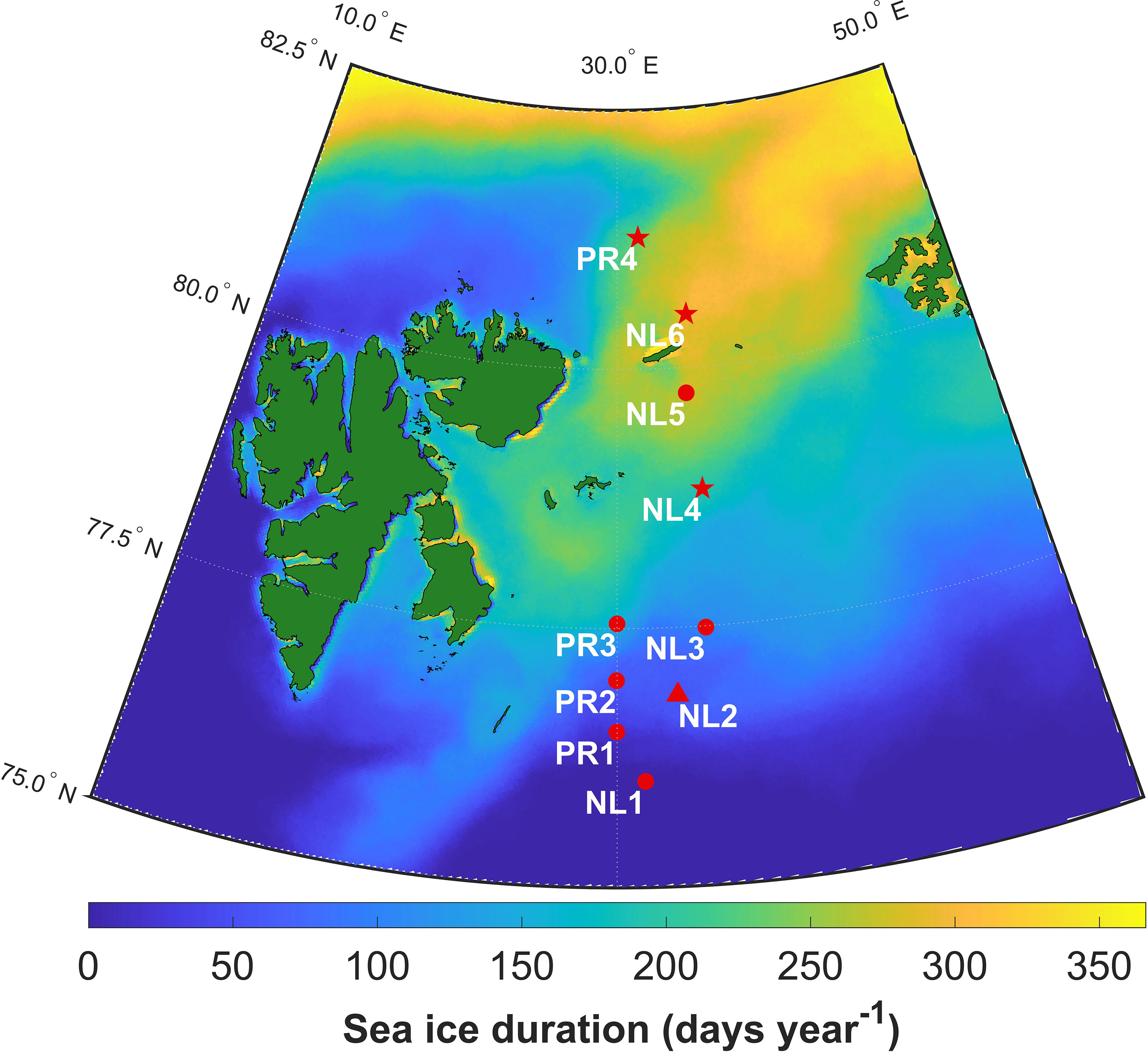
Figure 1 Map of sampling stations in the Barents Sea, with duration of sea ice cover from June 2017 to June 2018. Symbols represent the seasons during which stations were sampled: star – summer, triangle – winter, circle – both seasons.
In the laboratory, each organism was identified to the lowest practical taxonomic level (usually to species for most individuals) with taxon names standardized to the World Register of Marine Species (WoRMS Editorial Board, 2022) and assigned a feeding guild based on the Arctic Traits Database (Degen and Faulwetter, 2019). Five feeding guilds were used: surface deposit feeders, subsurface deposit feeder, suspension feeders, facultative surface deposit/suspension feeders, and carnivores/scavengers. Mollusc shells, polychaete tubes, and, where possible, stomach contents were removed prior to analysis. In the case of larger organisms, specific tissues were selected for further analysis (e.g., muscle in certain decapods and gastropods). Samples were then freeze dried and homogenised using a mortar and pestle. At least 0.2 g of freeze-dried material per sample was required for HBI analysis: in the case of small organisms, multiple individuals were pooled together to reach this mass (see Table S1 for details).
2.2 Lipid extraction and purification
Extraction of lipids from dried organisms was carried out following (Brown et al. 2017b) methodology. After addition of an internal standard (9-octylheptadec-8-ene [9-OHD]; 10 μL; 2 μg mL−1), samples were saponified in 4 ml methanolic potassium hydroxide (H2O: MeOH, 1: 9; 20% KOH) at 70°C for 60 minutes. After addition of hexane (2 mL) each sample was vortexed for 1 min and centrifuged (2500 rpm: 2 minutes). The supernatant – containing hexane and non-saponifiable lipids (NSL) – was transferred to a new vial. Three aliquots of NSL-containing supernatant were extracted this way and extracts transferred to the same vial. The NSL-containing hexane was then dried under a gentle stream of N2 gas, removing any remaining water and methanol. Hexane (1 mL) was added to the dried NSL, and the polar and non-polar fractions were separated using open column chromatography. Samples were run through a SiO2 column (0.5 g), which was then flushed with hexane (5 mL). Polar lipids were retained on the column, whereas nonpolar lipids (e.g., HBIs) passed through, into a new vial. These were dried under an N2 stream and resuspended in 50 µL of hexane for GC-MS analysis.
2.3 HBI analysis
Nonpolar lipid fractions were analysed with a Shimadzu QP2020 GC-MS system using a 30 m Rxi-5Sil column (0.25 mm i.d., 0.25 µm film), operating according to Belt et al. (2012). Analysis was run in selective ion monitoring mode to increase sensitivity, and the ions targeted represented the three HBIs of interest (mass-to-charge (m/z) ratios of selected ions were m/z 350.3: IP25, m/z 348.3: HBI II, m/z 346.3: HBI III). The mass spectral intensities of these HBIs were used to calculate the H-print (Equation 1), a ratio of pelagic-to-total HBIs (Brown et al., 2014c). A high H-print (>50%) indicates more pelagic HBIs, while a low H-print indicates more sympagic HBIs (Yunda-Guarin et al., 2020).
Using a previous H-print calibration, it was then possible to estimate the proportion of organic material from sympagic sources in the tissues of organisms, with Equation 2 (Brown and Belt, 2017).
2.4 Sea ice cover duration
Sea ice cover duration (SID) was calculated using gridded (resolution: 3.125 km) Advanced Microwave Scanning Radiometer 2 (AMSR 2) satellite data downloaded from https://seaice.uni-bremen.de/sea-ice-concentration/ (Spreen et al., 2008). Using MATLAB, SID was calculated as the number of days in a 365-day period where ice concentration was more than 15% in a grid cell. The commonly used 15% concentration threshold allowed for comparison with a similar study in the Pacific Arctic (Koch et al., 2020a). For each sampling event, SID was calculated for the grid cell containing the station, using the 365-day period preceding the sampling event. Figure 1 shows SID for the sampling area in the year preceding the first sampling event.
2.5 Numerical analysis
Numerical analyses were conducted in R (version 3.6.1). After examination, the data were transformed using the logit function (Equation 3), as they represent proportions (of sympagic OM). Regression models were used to examine the relationship between logit-transformed assimilated sympagic OM and SID. Due to the uneven distribution of sampling effort among seasons (see Section 3.3), with summer sampling being much more extensive, two analyses were conducted: (a) a model with only summer samples, to discern any effect of SID and feeding guild on sympagic OM assimilation across the whole study area and (b) a model restricted to stations south of (and including) NL4, but including both seasons to determine the effect of SID, season and feeding guild on sympagic OM assimilation. If a covariate was found to have no significant effect (significance level p<0.05) on sympagic OM assimilation, it was removed from the model, to have the simplest explanatory model for each analysis. In such cases, an ANOVA was used to compare the original and simplified models to confirm there was no significant difference between them.
3 Results
3.1 Overall summary
Overall, 126 samples from 10 stations were analysed, and 12 were discarded due to a lack of detection of at least one HBI of interest, thus preventing H-print calculation. The proportion of OM from sympagic sources in these samples was highly variable, with values ranging from 0.4-95.8%. The proportion of sympagic OM was generally higher in the northern half (north of 78.5°N) of the transect but remained very variable: mean ± standard deviation of 61.5 ± 21.7% in the northern half, versus 8.9 ± 13.4% in the southern half, with ranges of 5.8-95.8% and 0.4-65.4%, respectively. Sampling effort was unequal among taxa and feeding guilds: out of 25 taxa, 16 only had one to two samples, while individual feeding guilds had between 6 and 41 samples (Table S1; Figure S2).
3.2 Effect of sea ice duration on sympagic OM assimilation
Sea ice duration at the 10 stations lasted between 0-245 days (Table 1) with mean and median SID at 110 and 123 days, respectively. The station with the lowest SID was NL1 (0 days in 2018, and 20 days in 2019) and the station with the longest SID was NL6 in winter 2019 (245 days in 2019). Regression models showed a significant effect of SID on proportion of sympagic OM assimilated in the summer, but no effect of feeding guild (Figure 2; Table 2). Figures 2B–F shows the similar positive relationship with SID for all feeding guilds, except suspension feeders (though this is likely due to low sample size for this feeding guild). While there is a strong effect of SID on proportion of assimilated sympagic carbon, it is important to note the large variation, even at similar SID (Figure 2A). A similar regression analysis was conducted with Ctenodiscus crispatus samples, the taxon with the most samples (23 in summer), which covered most of the study area (Figure 3). Within this taxon, there was a similarly strong relationship between SID and proportion of sympagic OM, again with large variability at similar SID.
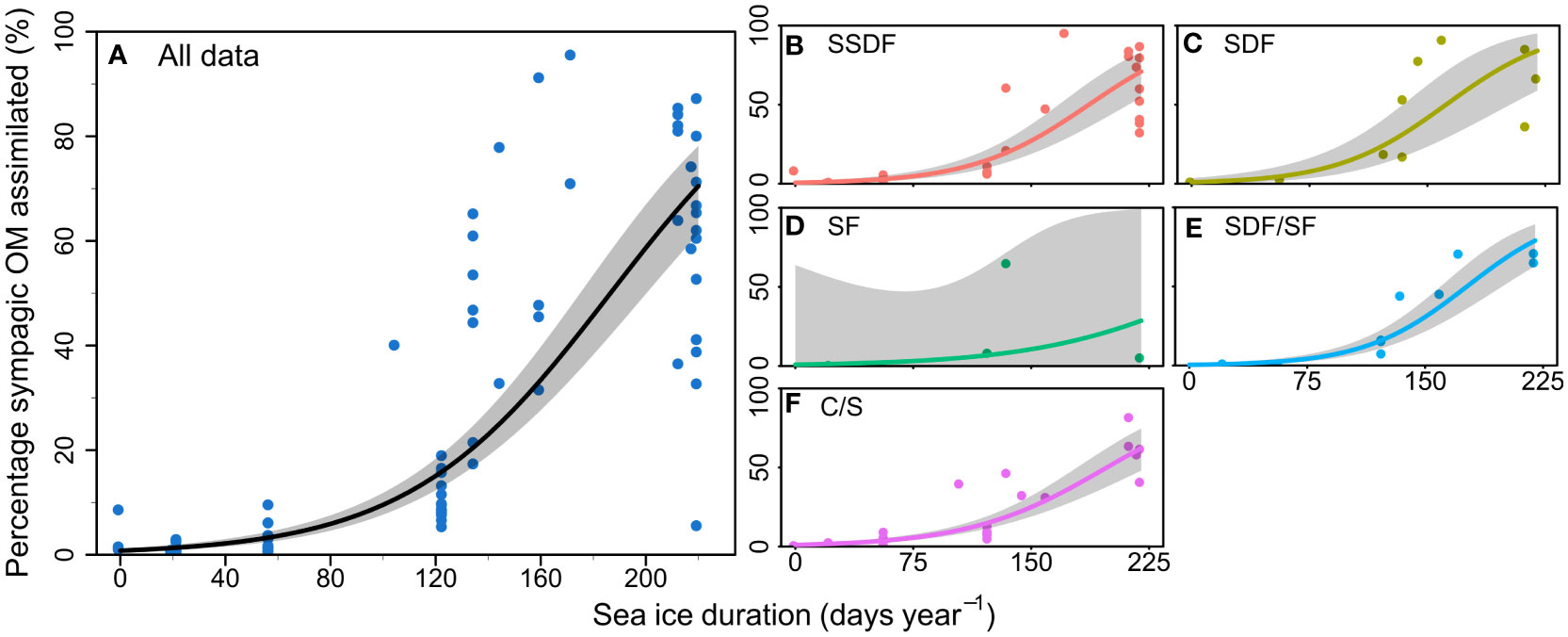
Figure 2 Proportion of sympagic organic matter (OM) assimilated based on the H-print approach against sea ice duration at each station during sampling period for (A) all samples analysed in the summer, and separated by feeding guilds: (B) subsurface deposit feeders; (C) surface deposit feeders; (D) suspension feeders; (E) surface deposit/suspension feeders; (F) carnivores/scavengers. Lines and shaded areas respectively represent regressions on logit-transformed data and 95% confidence intervals for each feeding guild. Each point represents one sample.
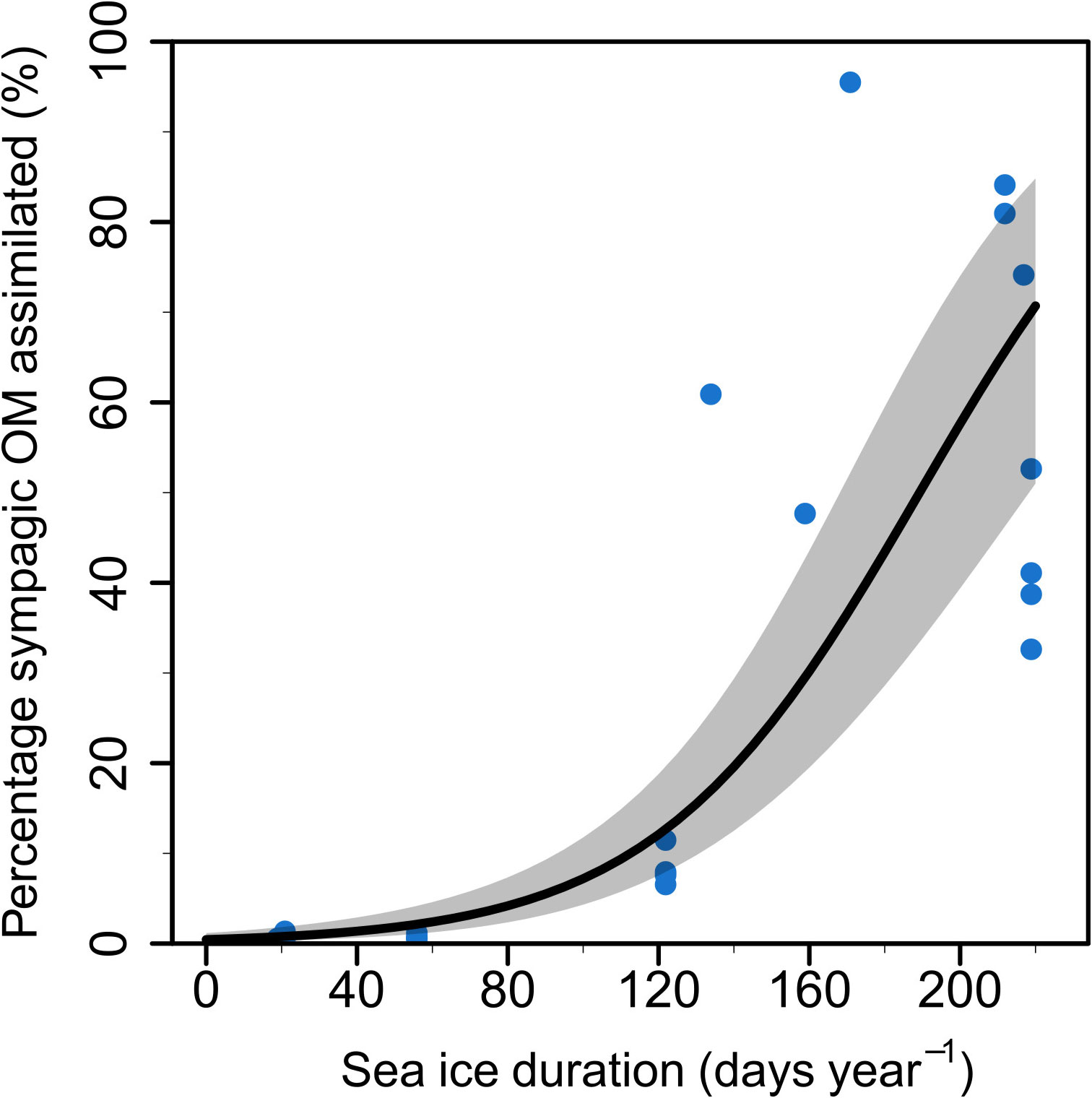
Figure 3 Proportion of sympagic organic matter (OM) assimilated based on the H-print approach against sea ice duration at each station for Ctenodiscus crispatus samples. The line represents a regression on logit-transformed data with 95% confidence intervals as shaded grey area. Each point represents one sample.
3.3 Seasonal differences in assimilation of sympagic OM
Winter sampling was complicated by ice presence, and there were therefore more summer samples than winter samples (87 and 27 samples, respectively). Importantly, while the summer samples were from the whole study area, winter samples were mostly restricted to the southern half of the transect, and seasonal analysis was therefore restricted to this part of the transect. This included stations south of 79°N: NL1-NL4, and PR1-PR3, with SID from 0-160 days. There were 64 summer samples and 26 winter samples used for seasonal analysis. Regression models showed a significant effect of SID, season, and their interaction on proportion of assimilated sympagic OM, but no significant effect of feeding guild (Figure 4; Table 3). However, note that both feeding guild as a covariate, and its interaction with SID had non-significant but low p-values (p=0.089 and p=0.070, respectively): this was largely driven by surface deposit feeders, which had higher proportions of sympagic OM at higher SID in summer than winter, and to some degree by suspension feeders, which had a low sample size and a different response to SID than other feeding guilds (Figure 2D). The regression presented does not include the feeding guild covariate, as an ANOVA reported no significant difference between the models with and without feeding guild (Table 3). In summer, the proportion of sympagic OM increased more rapidly with SID than in winter, due to higher values at higher SID (more than 100 days). However, in stations where SID was between 0-57 days, the proportions of assimilated sympagic OM remained similar in both seasons.
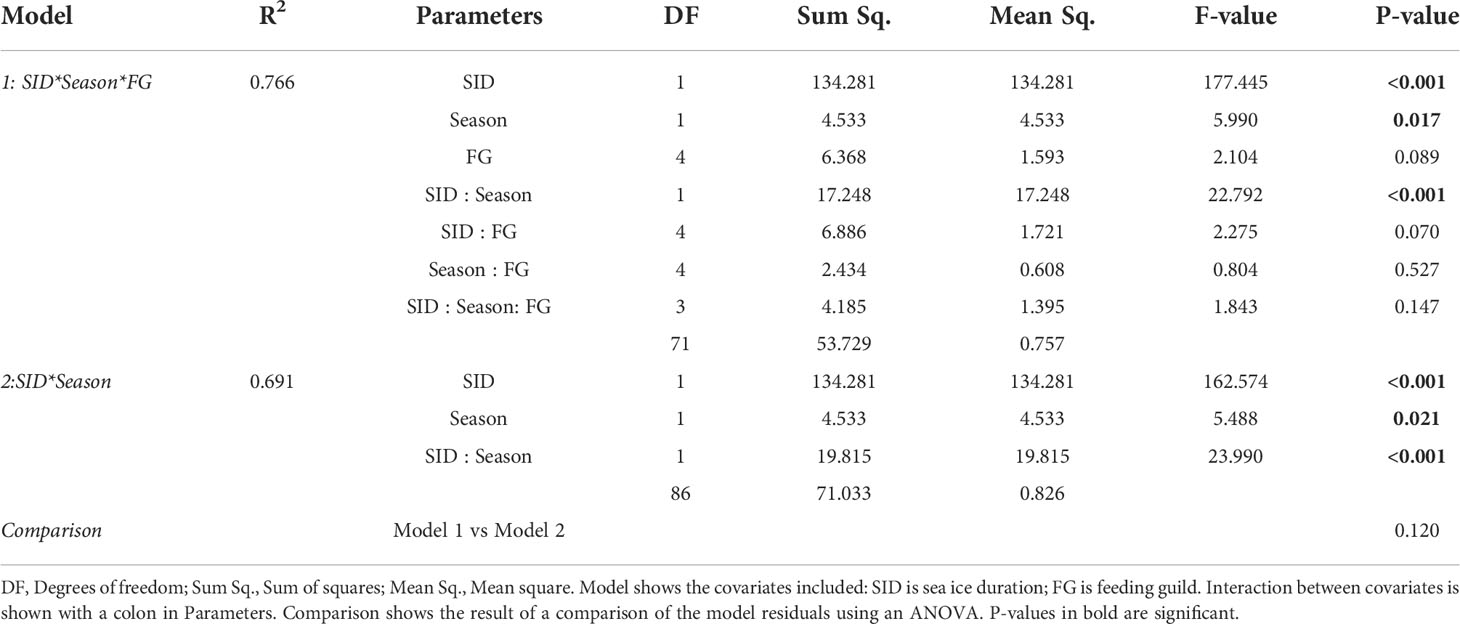
Table 3 Model diagnostics for regression models conducted on logit-transformed data from stations south of 79°N.
NL4 only had summer samples and was located much further North than other stations used for seasonal analysis (Figure 1) but had a similar SID to some of them (e.g., 160 days for NL4, 156 for PR3 – Table 1). It was therefore included in the seasonal analysis, to extend the dataset. A regression without NL4 (i.e., only stations south of 78°N) also showed a significant effect of season and confirmed that the presence of this northern station was not driving the seasonal difference (inset in Figure 4; Table S2). There was one winter sample from a station with higher SID, and it had a high proportion of assimilated sympagic OM (C. crispatus from P4: SID – 245 days year-1; proportion of assimilated sympagic OM – 73.8%). However, it was not included in analyses as it did change the general seasonal response, and would have led to an unbalanced number of summer samples from higher SID (Table S3; Figure S3).
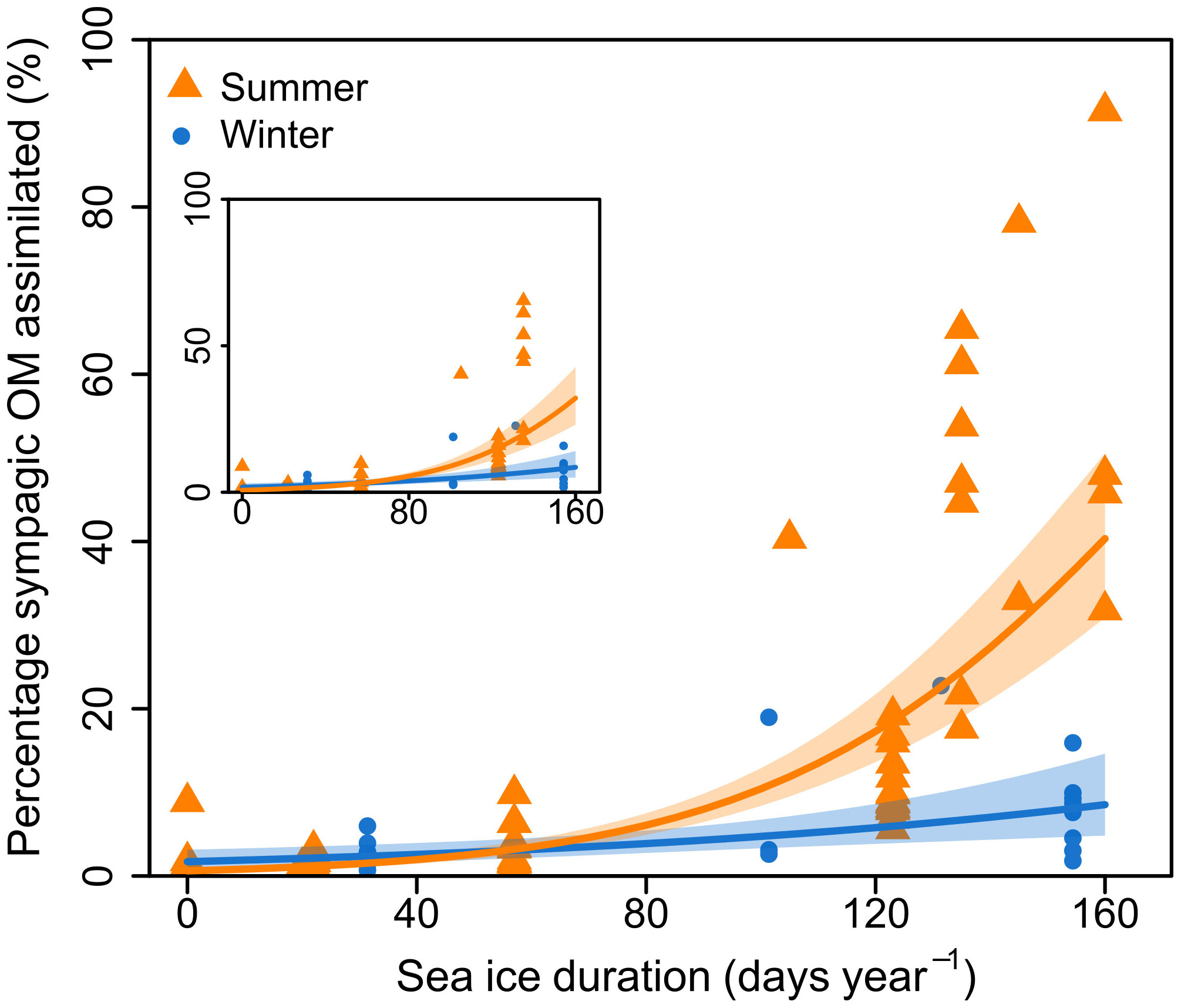
Figure 4 Proportion of sympagic organic matter (OM) assimilated based on the H-print approach against sea ice duration for stations south of 79°N. Samples are separated according to season, with orange triangles representing summer, and blue circles representing winter. Lines and shaded areas respectively represent regressions on logit-transformed data and 95% confidence intervals for each season. Inset shows the same graph, but with only stations south of 78°N.
4 Discussion
There was a strong increase in the contribution of sympagic OM to the diets of the benthos with increasing ice duration in the northern Barents Sea. Sympagic OM constituted a smaller proportion (~8.9%) of assimilated carbon in the southern part of the transect than in the northern part, where it contributed to over half (~61.5%) of the assimilated carbon. This north-south difference in assimilation of sympagic carbon was observed across most feeding guilds, indicating sympagic production was a major source for benthic food webs in the northern Barents Sea. However, variability in proportion of sympagic OM assimilated by different feeding guilds was very high, even at similar SID. This utilisation of sympagic OM was also seasonally variable, as reflected in a decrease from summer to winter. This highlights the spatiotemporal variation in the relative contributions of sympagic and pelagic OM, further improving our understanding of food web dynamics in the Barents Sea SIZ.
4.1 Effect of sea ice duration on assimilated sympagic OM
The spatial distribution of sea ice duration followed a south-to-north gradient, generally increasing with latitude (Figure 1). Previous studies have established a link between degree of ice cover and the input of sympagic material to the ecosystem. Studies on primary production found that sympagic production contributes a larger proportion of annual primary production where there is increased ice presence (Gosselin et al., 1997; Wassmann and Reigstad, 2011), while palaeontological studies have connected stronger levels of ice algae signals with higher sea ice concentrations (Stein et al., 2017). In this study, there was a significant increase in the contribution of sympagic OM to benthic diets with increasing duration of sea ice. This strong relationship indicates that sea ice cover plays an important role in the availability and uptake of sympagic versus pelagic OM by Barents Sea megabenthos.
The overall relationship of increasing proportion of sympagic OM assimilated with sea ice duration was observed across all feeding guilds (except in suspension feeders—see below). However, there is notably high variability in the assimilation of sympagic OM, even within the same feeding guild at locations with similar ice durations. While this could be due to the taxonomic differences between organisms within the same feeding guild, the well-sampled Ctenodiscus crispatus also show this variability within-species (Figure 3), indicating there are other processes leading to this variability. One such process may be a patchy input of sympagic material greatly increasing the sympagic OM available to certain organisms.
Areas of particularly high sympagic OM sedimentation can be very localised due to the inhomogeneous deposition of algal aggregates (Boetius et al., 2013), leading to higher proportions of sympagic OM assimilated in organisms at these locations. Ice algal production and biomass in turn is very patchy because snow and ice thickness and sediment load strongly modulate production and themselves vary on small scales (e.g., Lee et al., 2008; Gradinger, 2009). Even ice-associated fauna can show highly variable uptake of sympagic OM depending on the availability of both sympagic and pelagic OM (Brown et al., 2017b; Kohlbach et al., 2022). Patchiness of OM deposits may also be caused (or further enhanced) by differences in bathymetry – such as depressions which concentrate OM – or near bottom currents redistributing sinking OM (Lovvorn et al., 2020).
In addition, individual organism responses to sympagic and pelagic OM input may further increase variability. The Arctic benthos exhibits high dietary plasticity and employs many strategies to adapt to variable food quality and quantity. These strategies include omnivory, switching sources of OM, and changing feeding behaviour by searching for fresh OM or selectively feeding on OM from specific sources (Sun et al., 2009; Boetius et al., 2013; Morata et al., 2015; Kędra et al., 2019). Benthic organisms also utilise different types of fatty acids from ice algae and phytoplankton, adapting their assimilation strategies to meet energetic requirements (Sun et al., 2007). Certain deposit-feeding organisms may have selectively fed on either sympagic or pelagic OM depending on the quality of OM (Sun et al., 2009), whereas mobile deposit feeders (e.g., Pontaster tenuispinus, Strongylocentrotus spp.) may have responded to concentrated deposits of fresh OM, such as ice algae aggregates (Boetius et al., 2013). As the sea ice extent in the Barents Sea has large interannual variability (Herbaut et al., 2015), flexibility in responding to variable inputs of different types of OM may be crucial for the megabenthos in this region, by allowing them to adapt their feeding and assimilation methods to make use of the OM reaching the seafloor. Therefore, while the predicted retreat of sea ice from the Barents Sea (Onarheim and Årthun, 2017) may result in less sympagic OM availability to the benthos, reduced ice cover often leads to higher pelagic primary productivity due to higher light availability (Arrigo & van Dijken, 2015). In the Barents Sea, the southwestern, ice-free areas are associated with higher primary production, generally have higher infaunal abundance (Cochrane et al., 2009) and higher megafaunal abundance and biomass (Jørgensen et al., 2015), indicating that benthic communities may benefit from reduced ice cover.
Suspension feeders were the only feeding guild which did not show a strong increase in proportion of assimilated sympagic OM with sea ice duration (Figure 2D). This trend is based on a very small number of samples: only four suspension feeders were analysed for the analysis of the effect of sea ice duration. With more samples, there most likely would have been a stronger increase in the proportion of sympagic carbon with longer sea ice duration – but possibly not as strong as other feeding guilds, reflecting a lower uptake of sympagic OM by suspension feeders. Previous studies have found that suspension feeders generally assimilated little sympagic OM compared to other benthic consumers, which these authors attributed to the short residence time of this organic material in the water column (McMahon et al., 2006; Schollmeier et al., 2018; Koch et al., 2020a). The higher uptake of sympagic material by Arctic deposit feeders, in contrast, is well-documented and attributed to the large, early season sedimentation pulse of this fast-sinking organic material to the seafloor at a time when zooplankton grazing pressure is low (McMahon et al., 2006; Roy et al., 2015; Schollmeier et al., 2018; Kędra et al., 2019). Carnivore/scavengers had the same trend as most other feeding guilds, indicating that their H-print was similar to the community of organisms they preyed upon. This suggests that the ratio of pelagic and sympagic HBIs remains largely unchanged as they move through the benthic food web.
Interestingly, surface deposit feeders and subsurface deposit feeders had similar proportions of assimilated sympagic OM, indicating mixing of OM to deeper layers of sediment. Arctic organisms respond rapidly to inputs of fresh organic material through increased oxygen demand and bioturbation activity (Morata et al., 2015). Bioturbation activity can rapidly mix organic matter into the sediment, for example freshly deposited detritus was mixed to a depth of 10 cm within nine days in the North Atlantic deep-sea (Graf, 1989). If mixing occurs on similar timescales in the Barents Sea, freshly deposited OM would rapidly be available to both surface deposit and subsurface deposit feeding organisms, leading to similar HBI ratios. Current knowledge suggests that HBI signals likely reflect recent (1-2 months) carbon inputs, as supported by HBI depuration rates [around 30 days in whole molluscs (Koch et al., 2020a)] indicating relatively rapid turnover, and HBI signals detecting seasonal differences in OM assimilation (see next section). Therefore, similarities observed between feeding guilds are likely due to ecological processes, rather than long-term stability of HBIs in the food web or environment.
4.2 Effect of season on assimilated sympagic OM
Season affected the relationship between sea ice duration and proportion of sympagic OM: at longer sea ice durations, a higher proportion of sympagic OM was assimilated in summer than in winter. This is presumably due to a higher availability and more recent deposition of sympagic OM in the summer. However, the single winter data point from longer ice duration suggests that there can also be a high proportion of assimilated sympagic OM in this season. Sympagic production contributes to a higher proportion of total annual primary production at higher latitudes (Hegseth, 1998), and therefore the input and signal of sympagic OM likely remains in the system for longer. However, due to the variability observed in the summer, it is difficult to know how representative this single point is of the winter sympagic OM assimilation in the northern SIZ.
At shorter sea ice durations, the proportion of assimilated sympagic OM did not change seasonally, because there were low proportions in both seasons, presumably due to little sympagic production occurring in these areas. The higher summer values at longer sea ice durations indicate that sympagic OM can contribute to a large part of benthic diets in the summer in the ice-covered part of the Barents Sea shelf. Similarly high summer values have also been observed in the Chukchi sea, with both studies together jointly confirming the importance of sympagic OM to benthic food webs at this time on Arctic inflow shelves (Koch et al., 2020a). In contrast, sympagic OM contributed little to late summer pelagic food webs, even during an ice-heavy year in the Barents Sea (Søreide et al., 2006; Kohlbach et al., 2021a). This difference between benthic and pelagic food webs suggests that sympagic OM is present mostly in sediments in the late summer Barents Sea, and likely originated from the ice-algal spring bloom.
The lower winter contribution of sympagic OM to the benthos at higher sea ice durations is presumably due to a proportionally larger input of pelagic OM after the ice algal bloom [and increasingly from fall blooms (Ardyna et al., 2020)], as well as the summer consumption of sympagic OM (see Figure 5). For example, the stronger response of sympagic OM assimilation to SID in summer in surface deposit feeders suggests that these mobile deposit feeders responded to areas of higher sympagic OM deposition present only during the summer. Sea ice formation in the study area occurs in the autumn or winter, and there is therefore very little to no sympagic production which occurs outside of the spring bloom period. A seasonal difference in sympagic OM utilisation from summer to winter was not observed in zooplankton in the study area during the same period, because they generally did not feed on sympagic OM in the late summer (Kohlbach et al., 2021b). The presence (albeit low) of sympagic OM in the benthos in winter indicates that a repository of sympagic material remains in this season, although in low amounts. This is consistent with findings in the Chukchi sea, where sympagic material was found in surface sediments throughout the winter period (Koch et al., 2020b), and the sympagic OM assimilated by benthic invertebrates decreased from summer to winter, as was observed in this study.
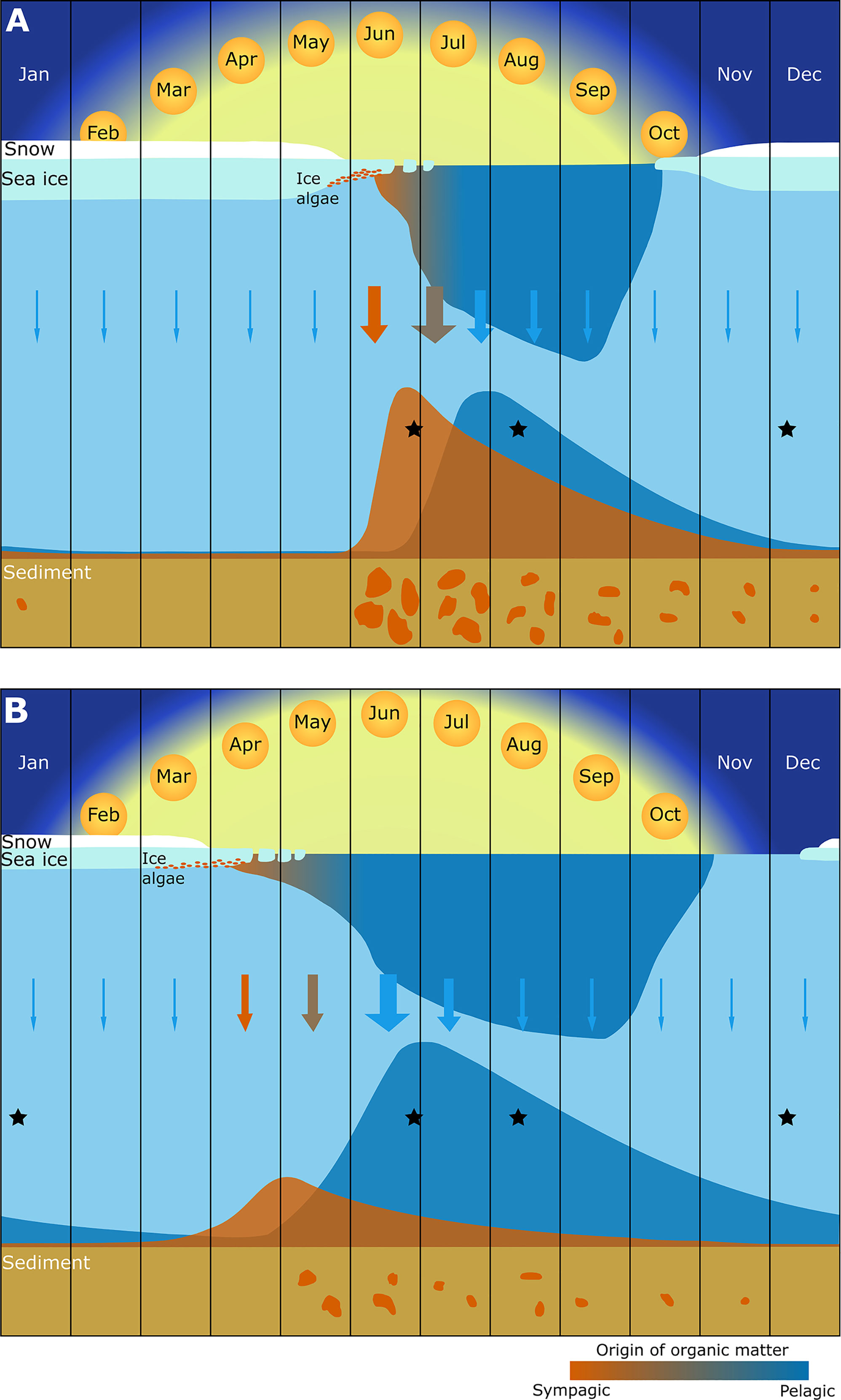
Figure 5 Schematic representation of the seasonal distribution, sinking and benthic assimilation of sympagic (orange) and pelagic (blue) organic matter in both the (A) northern (around 80°N) and (B) southern (around 77.5°N) sections of the study area. Surface primary production is dominated by the transition from a sympagic to pelagic bloom, followed by lower productivity in the late summer, autumn, and winter. The shaded area in the water column indicates the seasonal distribution of suspended organic matter. Arrows indicate sinking organic matter, with size representing magnitude and colour the dominance of pelagic or sympagic organic material. Estimates of the magnitude of assimilation of sympagic and pelagic organic matter are represented as plots at the seabed. Orange patches on the sediment represent patchiness of sympagic organic matter, which decreases in availability from spring to winter. Black stars show the timing of sampling events for this study. This figure is adapted from Wassmann and Reigstad, 2011 (their Figure 2) to include representation of the fate of organic matter reaching the seabed, based on our data and other published knowledge.
As sympagic OM input has been shown to affect benthic food web structure by shifting ecological niche space of organisms and modifying feeding of higher trophic levels (Yunda-Guarin et al., 2020), the seasonal difference observed in sympagic OM assimilation may also cause similar seasonal changes in the benthic food web structure of the Barents Sea. Previous food web studies have, however, reported little seasonal change in Arctic benthic food web structure due to the dietary flexibility of benthic consumers (Renaud et al., 2011; Kędra et al., 2012; Bridier et al., 2021). However, these studies were conducted in areas with very little sympagic production, such as Young Sound (Greenland) and Kongsfjorden (Svalbard) and may have therefore not fully captured the impact of sympagic OM on benthic consumers. The large seasonal difference in assimilated sympagic OM observed here suggests there may a seasonal change in food web structure of the Barents Sea benthos where sympagic OM contributes to a large part of the diet in summer.
4.3 Is sympagic OM overestimated in consumers?
The ratio of sympagic-to-pelagic OM in benthic consumers was high relative to the contribution of sympagic production to total annual production in the Barents Sea SIZ. Sympagic production accounts for 16-22% of annual production (5.3 g C m-2) in the Barents Sea SIZ (Hegseth, 1998), and 27% of daily primary production (11 mg C m-2 d-1) north of Svalbard (Ehrlich et al., 2021). In the northern half of our transect, the proportion of OM from sympagic sources assimilated by benthic consumers was 61.5%. While these numbers are not directly comparable due to what they represent (rates versus amount assimilated), the large discrepancy suggests a possible mismatch between supply and demand when extrapolating to total benthic demand. Another biomarker method also showed high contributions of ice-derived matter to benthic organisms in ice-covered waters, but the sympagic signal may have included feeding on refractory material (Søreide et al., 2013). Although there is less sympagic than pelagic production in the Barents Sea SIZ, phytoplankton blooms generally occur after the ascent of grazing copepods, leading to less sinking pelagic production reaching the benthos (Reigstad et al., 2008; Tamelander et al., 2008). Benthic utilisation of the OM that reaches the seafloor may also favour the uptake of sympagic OM through selective feeding or assimilation (e.g., through incorporation of the polyunsaturated fatty acids in ice algae) (McMahon et al., 2006). These processes would lead to higher-than-expected proportions of sympagic OM in benthic consumers when considering the proportion of annual primary production which occurs in sea ice. However, the high proportions of sympagic OM in the northern part of this study may also be due to the time of sampling, where ice retreat in the northern Barents Sea SIZ occurs in July to early August, with concurrent release of ice algae (Ji et al., 2013; Dong et al., 2020). We suggest therefore, that our sampling probably coincided with the period of maximum availability and uptake of sympagic OM by benthic consumers. This is supported by high levels of sympagic HBIs in flux-feeding zooplankton in the summer, indicating recent sympagic matter sedimentation (Kohlbach et al., 2021a). Sympagic OM values for the northern half of the transect may therefore reflect a short period of high uptake of sympagic OM by the benthos (see Figure 5).
The high proportion of sympagic OM observed in benthic consumers may also be an overestimation due to the HBI-based method used. HBIs are diatom-specific biomarkers and, while sea ice algae communities are usually dominated by diatoms, phytoplankton communities can also include a wide variety of other taxa which are not accounted for in HBI analysis (Wassmann et al., 1999; Hegseth and von Quillfeldt, 2022). In 2018, diatoms dominated the spring phytoplankton bloom (up to 270 µg C L-1), but the community shifted to a mix of diatoms and nanoflagellates (mostly Dinobryon sp. and Phaeocystis pouchetii) in summer (maximum diatom biomass: 163 µg C L-1, maximum nanoflagellate biomass: 86 µg C L-1) (K. Davidson, SAMS, unpubl. data). In the summer of 2019, taxonomic and fatty acid analysis pointed to a mix of diatoms and flagellates in the phytoplankton community (Kohlbach et al., 2021a). While nanoflagellates were present in both study years, their contribution to benthic diets would not have been represented by HBI levels in benthic consumers. However, some of these small-celled producers are less likely to sink to the benthos than heavy, silicified diatoms, and therefore are more susceptible to grazing and disintegration in the water column (Heiskanen and Kononen, 1994; Fahnenstiel et al., 1995; Kiørboe et al., 1996). Still, it is documented that flagellates do contribute to benthic invertebrate diets (Napolitano et al., 1997; Paar et al., 2019), and the HBI analysis would therefore have overlooked this pelagic contribution to benthic diets.
Increased degradation of pelagic HBIs relative to sympagic HBIs could also have led to overestimation of the proportion of sympagic OM in benthic consumers. HBIs in senescent algal cells are susceptible to photooxidation, and the presumed longer residence time of pelagic OM in the water column may result in higher degradation of pelagic HBIs compared to sympagic HBIs (Rontani et al., 2016; Rontani et al., 2019). With these methodological limitations in mind, it is suggested that a multiple trophic marker approach may be the most effective in future studies (such as used by Kohlbach et al., 2016; Yunda-Guarin et al., 2020; Kohlbach et al., 2021a) when investigating the role of sympagic OM in the Arctic system (see Leu et al., 2020 for an in-depth investigation of various sympagic trophic markers). In addition, analysis of sympagic and pelagic particulate organic matter would improve confidence in the results obtained. As HBIs are produced by certain diatoms which represent only a small proportion of total algal abundance (Brown et al., 2014c) this would provide information on the presence of HBI-producing taxa during the study period, as well as give baseline values of the HBIs produced. The inclusion of more sympagic and pelagic HBIs in the H-print calculation on a study-by-study basis could make results even more robust, though it would also require adapting the calculation used to estimate sympagic OM in organisms (Equation 2).
While the absolute values presented here may represent an overestimation of the contribution of sympagic OM to benthic consumers, the spatial and seasonal data provide new insights into the role of sympagic production for Barents Sea benthic food webs and are consistent with results from the Chukchi sea and with sea ice patterns (Koch et al., 2020a). Based on our results and the literature, we present a figure illustrating the contribution of sympagic and pelagic OM to Arctic benthic food webs under short and long ice cover (Figure 5). Our data do not reflect the magnitude of OM available to the system, but previous studies indicate a much lower feeding rates and activity during the winter season than in other seasons, due to less labile OM in the sediments (Renaud et al., 2007; Bourgeois et al., 2017). Even though current data do not cover the whole range of seasons, especially at higher latitudes, this conceptual figure represents a step toward a better understanding of how pelagic and sympagic production fuel benthic ecosystems.
5 Conclusion
We quantify for the first time for Barents Sea benthos the relationship of sympagic OM assimilation to sea ice duration, and its variation by season. Establishing this relationship showed sympagic OM contributions upwards of 50% in the Barents Sea SIZ when ice is present for over 130 days. Where sea ice duration is longer, we find a higher assimilation of sympagic OM by benthos in the summer than in the winter. The apparent assimilation is observed in almost all feeding guilds, highlighting the high relevance of sympagic OM across the benthic food web. We hypothesise that substantial variability of sympagic OM assimilation, even within areas of similarly long ice cover, was likely driven by patchiness in sympagic OM deposition due to patchy distribution of sympagic primary production (e.g. Lee et al., 2008), as well as by bathymetric and hydrographic redistribution of OM (e.g. Lovvorn et al., 2020). The spatiotemporal variation in the utilisation of pelagic and sympagic OM by benthic organisms largely follows the variation in sympagic and pelagic production (see Figure 5). High assimilation of pelagic OM by the benthos in areas of shorter ice duration suggests a dietary adaptability to feed on whichever OM is more available. Therefore, while sympagic OM can represent a large proportion of OM assimilated by the benthos, the predicted declines in sea ice cover with Climate Change – and therefore sympagic production – will not necessarily be detrimental to benthic organisms. In fact, sea ice decline is a major factor expected to increase pelagic primary productivity on Arctic inflow shelves (Ardyna and Arrigo, 2020), which may result in higher organic matter deposition in the Barents Sea. Organic matter deposition during the productive spring and summer seasons is an important source of energy for the Arctic benthos and has been linked to higher faunal densities and abundances (Ambrose and Renaud, 1995; Lovvorn et al., 2005). A better understanding of the energetic and nutritive roles of these sources of OM, as well as how their magnitude and delivery to the benthos will change with reduced ice cover, will increase our understanding of the impacts of decreasing ice on the Barents Sea benthos. As the Barents Sea is rapidly losing sea ice, the data in this study provides a baseline for understanding the implications of future changes in the proportion of these sources of organic matter.
Data availability statement
The original contributions presented in the study are included in the article/Supplementary Material. Further inquiries can be directed to the corresponding author.
Author contributions
IC is a PhD student supervised by all co-authors bar AZ. All authors contributed to the study. Samples were collected by BN and BB. IC and AZ processed the samples, and IC conducted HBI analysis. IC conducted data analysis and led the writing of the manuscript. All authors contributed to the article and approved the submitted version.
Funding
This work was conducted as part of the Arctic PRIZE (PRoductivity in the seasonal Ice ZonE) project (grant no. NE/P006302/1) funded by the UK Natural Environment Research Council (NERC) Changing Arctic Ocean program. Samples were obtained from cruises conducted for this project, as well as from cruises for the ‘The Nansen Legacy’ project (RCN#276730) funded by the Research Council of Norway. The sample collection was successful thanks to the invaluable help of M. Foley, R. Descoteaux, L. Jørgensen, A. McIvor and E. Åström. We thank J. Hovinen, C. Ruiz-González and A. Rock for their assistance in the laboratory. We thank the captains and crews of the R/V Helmer Hanssen, RRS James Clark Ross and R/V Kronprins Haakon, as well as collaborators from Arctic PRIZE and Nansen Legacy for their support. Thank you to M. Burrows for the invaluable help with statistical analyses, and to the three reviewers for their comments which greatly improved this manuscript.
Conflict of interest
The authors declare that the research was conducted in the absence of any commercial or financial relationships that could be construed as a potential conflict of interest.
Publisher’s note
All claims expressed in this article are solely those of the authors and do not necessarily represent those of their affiliated organizations, or those of the publisher, the editors and the reviewers. Any product that may be evaluated in this article, or claim that may be made by its manufacturer, is not guaranteed or endorsed by the publisher.
Supplementary material
The Supplementary Material for this article can be found online at: https://www.frontiersin.org/articles/10.3389/fmars.2022.1009303/full#supplementary-material
References
Ambrose W. G., Renaud P.E. (1995). Benthic response to water column productivity patterns: Evidence for benthic-pelagic coupling in the Northeast Water Polynya. J. Geophys. Res. 100, 4411–4421. doi: 10.1029/94jc01982
Ambrose W. G., Renaud P.E. (1997). Does a pulsed food supply to the benthos affect polychaete recruitment patterns in the Northeast Water Polynya? J. Mar. Syst. 10, 483–495. doi: 10.1016/S0924-7963(96)00053-X
Ambrose W. G., von Quillfeldt C., Clough L. M., Tilney P. V. R., Tucker T. (2005). The sub-ice algal community in the Chukchi sea: Large- and small-scale patterns of abundance based on images from a remotely operated vehicle. Polar Biol. 28, 784–795. doi: 10.1007/s00300-005-0002-8
Ardyna M., Arrigo K. R. (2020). Phytoplankton dynamics in a changing Arctic Ocean. Nat. Climate Change 10, 892–903. doi: 10.1038/s41558-020-0905-y
Ardyna M., Mundy C. J., Mills M. M., Oziel L., Grondin P. L., Lacour L., et al. (2020). Environmental drivers of under-ice phytoplankton bloom dynamics in the Arctic Ocean. Elementa Sci. Anthropocene 8, 30. doi: 10.1525/elementa.430
Arrigo K. R., van Dijken G. L. (2015). Continued increases in Arctic Ocean primary production. Prog. Oceanog. 136, 60–70. doi: 10.1016/j.pocean.2015.05.002
Belt S. T. (2018). Source-specific biomarkers as proxies for Arctic and Antarctic sea ice. Org. Geochem. 125, 277–298. doi: 10.1016/j.orggeochem.2018.10.002
Belt S. T., Brown T., Rodriguez A. N., Sanz P. C., Tonkin A., Ingle R. (2012). A reproducible method for the extraction, identification and quantification of the Arctic Sea ice proxy IP25 from marine sediments. Anal. Methods 4, 705–713. doi: 10.1039/c2ay05728j
Boetius A., Albrecht S., Bakker K., Bienhold C., Felden J. (2013). Export of algal biomass from the melting Arctic sea ice. Sci. (1979) 339, 1430–1432. doi: 10.1126/science.1231346
Bourgeois S., Archambault P., Witte U. (2017). Organic matter remineralization in marine sediments: A pan-Arctic synthesis. Global Biogeochem. Cycles 31 (1), 190–213. doi: 10.1002/2016GB005378
Bridier G., Olivier F., Chauvaud L., Sejr M. K., Grall J. (2021). Food source diversity, trophic plasticity, and omnivory enhance the stability of a shallow benthic food web from a high-Arctic fjord exposed to freshwater inputs. Limnol. Oceanog. 66, S259–S272. doi: 10.1002/lno.11688
Brown T. A., Alexander C., Yurkowski D. J., Ferguson S. H., Belt S.T. (2014a). Identifying variable sea ice carbon contributions to the Arctic ecosystem: A case study using highly branched isoprenoid lipid biomarkers in Cumberland Sound ringed seals. Limnol. Oceanog. 59, 1581–1589. doi: 10.4319/lo.2014.59.5.1581
Brown T. A., Assmy P., Hop H., Wold A., Belt S.T. (2017a). Transfer of ice algae carbon to ice-associated amphipods in the high-Arctic pack ice environment. J. Plankton Res. 39, 664–674. doi: 10.1093/plankt/fbx030
Brown T. A., Belt S. T. (2017). Biomarker-based H-print quantifies the composition of mixed sympagic and pelagic algae consumed by Artemia sp. J. Exp. Mar. Biol. Ecol. 488, 32–37. doi: 10.1016/j.jembe.2016.12.007
Brown T. A., Belt S. T., Tatarek A., Mundy C.J. (2014b). Source identification of the Arctic Sea ice proxy IP25. Nat. Commun. 5, 1–7. doi: 10.1038/ncomms5197
Brown T. A., Chrystal E., Ferguson S. H., Yurkowski D. J., Watt C., Hussey N. E., et al. (2017b). Coupled changes between the H-print biomarker and δ15N indicates a variable sea ice carbon contribution to the diet of Cumberland Sound beluga whales. Limnol. Oceanog. 62, 1606–1619. doi: 10.1002/lno.10520
Brown T. A., Galicia M. P., Thiemann G. W., Belt S. T., Yurkowski D. J., Dyck M. G. (2018). High contributions of sea ice derived carbon in polar bear (Ursus maritimus) tissue. PloS One 13, e0191631. doi: 10.1371/journal.pone.0191631
Brown T. A., Yurkowski D. J., Ferguson S. H., Alexander C., Belt S. T. (2014c). H-print: a new chemical fingerprinting approach for distinguishing primary production sources in Arctic ecosystems. Environ. Chem. Lett. 12, 387–392. doi: 10.1007/s10311-014-0459-1
Cochrane S. K. J., Denisenko S. G., Renaud P. E., Emblow C. S., Ambrose W. G., Ellingsen I. H., et al. (2009). Benthic macrofauna and productivity regimes in the Barents Sea - ecological implications in a changing Arctic. J. Sea Res. 61, 222–233. doi: 10.1016/j.seares.2009.01.003
De Freitas T. R., Hess S., Alve E., Bluhm B. A., Molina E. J., Reiss H., et al (2022a). Seabed sediment data (upper 6 cm) on water content, total nitrogen, total carbon, total organic carbon, total inorganic carbon, carbon and nitrogen isotopic composition from the Nansen Legacy seasonal cruise 2019706. doi: 10.21335/NMDC-490057692
De Freitas T. R., Hess S., Alve E., Bluhm B. A., Molina E. J., Reiss H., et al (2022b). Seabed sediment data (upper 6 cm) on water content, total nitrogen, total carbon, total organic carbon, total inorganic carbon, carbon and nitrogen isotopic composition from the Nansen Legacy seasonal cruise 2019711. doi: 10.21335/NMDC-799257283
Degen R., Faulwetter S. (2019). The Arctic traits database – a repository of Arctic benthic invertebrate traits. Earth System Sci. Data 11, 301–322. doi: 10.5194/essd-11-301-2019
Dong K., Kvile K.Ø., Stenseth N. C., Stige L. C. (2020). Associations among temperature, sea ice and phytoplankton bloom dynamics in the Barents Sea. Mar. Ecol. Prog. Ser. 635, 25–36. doi: 10.3354/meps13218
Ehrlich J., Bluhm B. A., Peeken I., Massicotte P., Schaafsma F. L., Castellani G., et al. (2021). Sea-ice associated carbon flux in Arctic spring. Elementa 9, 1. doi: 10.1525/elementa.2020.00169
Fahnenstiel G. L., McCormick M. J., Lang G. A., Redalje D. G., Lohrenz S. E., Markowitz M., et al. (1995). Taxon-specific growth and loss rates for dominant phytoplankton populations from the northern Gulf of Mexico. Mar. Ecol. Prog. Ser. 117, 229–239. doi: 10.3354/meps117229
Freitas F. S., Hendry K. R., Henley S. F., Faust J. C., Tessin A. C., Stevenson M. A., et al. (2020). Benthic-pelagic coupling in the Barents Sea: an integrated data-model framework. Philos. Trans. R. Soc. A: Math. Phys. Eng. Sci. 378, 20190359. doi: 10.1098/rsta.2019.0359
Gosselin M., Levasseur M., Wheeler P. A., Horner R. A., Booth B. C. (1997). New measurements of phytoplankton and ice algal production in the Arctic Ocean. Deep-Sea Res. Part II: Topical Stud. Oceanog. 44, 1623–1644. doi: 10.1016/S0967-0645(97)00054-4
Gradinger R. (2009). Sea-ice algae: Major contributors to primary production and algal biomass in the Chukchi and Beaufort Seas during May/June 2002. Deep-Sea Res. Part II: Topical Stud. Oceanog. 56, 1201–1212. doi: 10.1016/j.dsr2.2008.10.016
Graf G. (1989). Benthic-pelagic coupling in a deep-sea benthic community. Nature 341, 437–439. doi: 10.1038/341437a0
Grebmeier J. M., Barry J. P. (1991). The influence of oceanographic processes on pelagic-benthic coupling in polar regions: A benthic perspective. J. Mar. Syst. 2, 495–518. doi: 10.1016/0924-7963(91)90049-Z
Grebmeier J. M., Bluhm B. A., Cooper L. W., Danielson S. L., Arrigo K. R., Blanchard A. L., et al. (2015). Ecosystem characteristics and processes facilitating persistent macrobenthic biomass hotspots and associated benthivory in the Pacific Arctic. Prog. Oceanog. 136, 92–114. doi: 10.1016/j.pocean.2015.05.006
Hegseth E. N. (1998). Primary production of the northern Barents Sea. Polar Res. 17 (2), 113–123. doi: 10.3402/polar.v17i2.6611
Hegseth E. N., von Quillfeldt C. (2022). The Sub-ice algal communities of the Barents Sea pack ice: Temporal and spatial distribution of biomass and species. J. Mar. Sci. Eng. 10, 164. doi: 10.3390/jmse10020164
Heiskanen A. S., Kononen K. (1994). Sedimentation of vernal and late summer phytoplankton communities in the coastal Baltic Sea. Archiv für Hydrobiologie 131, 175–198. doi: 10.1127/archiv-hydrobiol/131/1994/175
Herbaut C., Houssais M. N., Close S., Blaizot A. C. (2015). Two wind-driven modes of winter sea ice variability in the Barents Sea. Deep-Sea Res. Part I: Oceanog. Res. Papers 106, 97–115. doi: 10.1016/j.dsr.2015.10.005
Jørgensen L. L., Ljubin P., Skjoldal H. R., Ingvaldsen R. B., Anisimova N., Manushin I. (2015). Distribution of benthic megafauna in the Barents Sea: baseline for an ecosystem approach to management. ICES J. Mar. Sci. 72, 595–613. doi: 10.1093/icesjms/fsu106
Ji R., Jin M., Varpe Ø. (2013). Sea Ice phenology and timing of primary production pulses in the Arctic Ocean. Global Change Biol. 19, 734–741. doi: 10.1111/gcb.12074
Kędra M., Cooper L. W., Zhang M., Biasatti D., Grebmeier J. M. (2019). Benthic trophic sensitivity to on-going changes in Pacific Arctic seasonal sea ice cover – insights from the nitrogen isotopic composition of amino acids. Deep-Sea Res. Part II: Topical Stud. Oceanog. 162, 137–151. doi: 10.1016/j.dsr2.2019.01.002
Kędra M., Kuliński K., Walkusz W., Legezyńska J. (2012). The shallow benthic food web structure in the high Arctic does not follow seasonal changes in the surrounding environment. Estuarine Coast. Shelf Sci. 114, 183–191. doi: 10.1016/j.ecss.2012.08.015
Kiørboe T., Hansen J. L. S., Alldredge A. L., Jackson G. A., Passow U., Dam H. G., et al. (1996). Sedimentation of phytoplankton during a diatom bloom: Rates and mechanisms. J. Mar. Res. 54, 1123–1148. doi: 10.1357/0022240963213754
Koch C. W., Cooper L. W., Grebmeier J. M., Frey K., Brown T. A. (2020a). Ice algae resource utilization by benthic macro- and megafaunal communities on the Pacific Arctic shelf determined through lipid biomarker analysis. Mar. Ecol. Prog. Ser. 651, 23–43. doi: 10.3354/meps13476
Koch C. W., Cooper L. W., Lalande C., Brown T. A., Frey K. E., Grebmeier J. M. (2020b). Seasonal and latitudinal variations in sea ice algae deposition in the northern Bering and Chukchi seas determined by algal biomarkers. PloS One 15, e0231178. doi: 10.1371/journal.pone.0231178
Kohlbach D., Graeve M., A. Lange B., David C., Peeken I., Flores H. (2016). The importance of ice algae-produced carbon in the central Arctic Ocean ecosystem: Food web relationships revealed by lipid and stable isotope analyses. Limnol. Oceanog. 61, 2027–2044. doi: 10.1002/lno.10351
Kohlbach D., Hop H., Wold A., Schmidt K., Smik L., Belt S. T., et al. (2021a). Multiple trophic markers trace dietary carbon sources in Barents Sea zooplankton during late summer. Front. Mar. Sci. 7. doi: 10.3389/fmars.2020.610248
Kohlbach D., Schaafsma F. L., Graeve M., Lebreton B., Lange B. A., David C., et al. (2017). Strong linkage of polar cod (Boreogadus saida) to sea ice algae-produced carbon: Evidence from stomach content, fatty acid and stable isotope analyses. Prog. Oceanog. 152, 62–74. doi: 10.1016/j.pocean.2017.02.003
Kohlbach D., Schmidt K., Hop H., Wold A., Al-Habahbeh A. K., Belt S. T., et al. (2021b). Winter carnivory and diapause counteract the reliance on ice algae by Barents Sea zooplankton. Front. Mar. Sci. 8. doi: 10.3389/fmars.2021.640050
Kohlbach D., Smik L., Belt S. T., Hop H., Wold A., Graeve M., et al. (2022). A multi-trophic marker approach reveals high feeding plasticity in Barents Sea under-ice fauna. Prog. Oceanog. 208, 102895. doi: 10.1016/j.pocean.2022.102895
Kunisch E. H., Graeve M., Gradinger R., Haug T., Kovacs K. M., Lydersen C., et al. (2021). Ice-algal carbon supports harp and ringed seal diets in the European Arctic: Evidence from fatty acid and stable isotope markers. Mar. Ecol. Prog. Ser. 675, 181–197. doi: 10.3354/meps13834
Lee S. H., Whitledge T. E., Kang S.-H. (2008). Carbon uptake rates of Sea ice algae and phytoplankton under different light intensities in a landfast sea ice zone, Barrow, Alaska. Source: Arctic 61, 281–291. doi: 10.14430/arctic25
Leu E., Brown T. A., Graeve M., Wiktor J., Hoppe C. J. M., Chierici M., et al. (2020). Spatial and temporal variability of ice algal trophic markers–with recommendations about their application. J. Mar. Sci. Eng. 8, 1–24. doi: 10.3390/jmse8090676
Limoges A., Massé G., Weckström K., Poulin M., Ellegaard M., Heikkilä M., et al. (2018). Spring succession and vertical export of diatoms and IP25 in a seasonally ice-covered high Arctic fjord. Front. Earth Sci. 6. doi: 10.3389/feart.2018.00226
Lovvorn J. R., Cooper L. W., Brooks M. L., de Ruyck C. C., Bump J. K., Grebmeier J. M. (2005). Organic matter pathways to zooplankton and benthos under pack ice in late winter and open water in late summer in the north-central Bering Sea. Mar. Ecol. Prog. Ser. 291, 135–150. doi: 10.3354/meps291135
Lovvorn J. R., Rocha A. R., Danielson S. L., Cooper L. W., Grebmeier J. M., Hedstrom K. S. (2020). Predicting sediment organic carbon and related food web types from a physical oceanographic model on a subarctic shelf. Mar. Ecol. Prog. Ser. 633, 37–54. doi: 10.3354/meps13163
McMahon K. W., Ambrose W. G., Johnson B. J., Sun M., Lopez G. R., Clough L. M., et al. (2006). Benthic community response to ice algae and phytoplankton in Ny-Ålesund, Svalbard. Mar. Ecol. Prog. Ser. 310, 1–14. doi: 10.3354/meps310001
Morata N., Michaud E., Włodarska-Kowalczuk M. (2015). Impact of early food input on the Arctic benthos activities during the polar night. Polar Biol. 38, 99–114. doi: 10.1007/s00300-013-1414-5
Napolitano G. E., Pollero R. J., Gayoso A. M., MacDonald B. A., Thompson R. J. (1997). Fatty acids as trophic markers of phytoplankton blooms in the bahia blanca estuary (Buenos Aires, Argentina) and in Trinity Bay (Newfoundland, Canada). Biochem. Syst. Ecol. 25 (8), 739–755. doi: 10.1016/S0305-1978(97)00053-7
Olli K., Wexels Riser C., Wassmann P., Ratkova T., Arashkevich E., Pasternak A. (2002). Seasonal variation in vertical flux of biogenic matter in the marginal ice zone and the central Barents Sea. J. Mar. Syst. 38, 189–204. doi: 10.1016/S0924-7963(02)00177-X
Onarheim I. H., Årthun M. (2017). Toward an ice-free Barents Sea. Geophys. Res. Lett. 44, 8387–8395. doi: 10.1002/2017GL074304
Paar M., Lebreton B., Graeve M., Greenacre M., Asmus R., Asmus H. (2019). Food sources of macrozoobenthos in an Arctic kelp belt: Trophic relationships revealed by stable isotope and fatty acid analyses. Mar. Ecol. Prog. Ser. 615, 31–49. doi: 10.3354/meps12923
Reigstad M., Wexels Riser C., Wassmann P., Ratkova T. (2008). Vertical export of particulate organic carbon: Attenuation, composition and loss rates in the northern Barents Sea. Deep-Sea Res. Part II: Topical Stud. Oceanog. 55, 2308–2319. doi: 10.1016/j.dsr2.2008.05.007
Renaud P. E., Riedel A., Michel C., Morata N., Gosselin M., Juul-Pedersen T., et al. (2007). Seasonal variation in benthic community oxygen demand: A response to an ice algal bloom in the Beaufort Sea, Canadian Arctic? J. Mar. Syst. 67, 1–12. doi: 10.1016/j.jmarsys.2006.07.006
Renaud P. E., Tessmann M., Evenset A., Christensen G. N. (2011). Benthic food-web structure of an Arctic fjord (Kongsfjorden, Svalbard). Mar. Biol. Res. 7, 13–26. doi: 10.1080/17451001003671597
Rontani J. F., Belt S. T., Brown T. A., Amiraux R., Gosselin M., Vaultier F., et al. (2016). Monitoring abiotic degradation in sinking versus suspended Arctic Sea ice algae during a spring ice melt using specific lipid oxidation tracers. Org. Geochem. 98, 82–97. doi: 10.1016/j.orggeochem.2016.05.016
Rontani J. F., Smik L., Belt S. T., Vaultier F., Armbrecht L., Leventer A., et al. (2019). Abiotic degradation of highly branched isoprenoid alkenes and other lipids in the water column off East Antarctica. Mar. Chem. 210, 34–47. doi: 10.1016/j.marchem.2019.02.004
Roy V., Iken K., Gosselin M., Tremblay J.É., Bélanger S., Archambault P. (2015). Benthic faunal assimilation pathways and depth-related changes in food-web structure across the Canadian Arctic. Deep-Sea Res. Part I: Oceanog. Res. Papers 102, 55–71. doi: 10.1016/j.dsr.2015.04.009
Søreide J. E., Carroll M. L., Hop H., Ambrose W. G., Hegseth E. N., Falk-Petersen S. (2013). Sympagic-pelagic-benthic coupling in Arctic and Atlantic waters around Svalbard revealed by stable isotopic and fatty acid tracers. Mar. Biol. Res. 9, 831–850. doi: 10.1080/17451000.2013.775457
Søreide J. E., Hop H., Carroll M. L., Falk-Petersen S., Hegseth E. N. (2006). Seasonal food web structures and sympagic-pelagic coupling in the European Arctic revealed by stable isotopes and a two-source food web model. Prog. Oceanog. 71, 59–87. doi: 10.1016/j.pocean.2006.06.001
Schollmeier T., Oliveira A. C. M., Wooller M. J., Iken K. (2018). Tracing sea ice algae into various benthic feeding types on the Chukchi sea shelf. Polar Biol. 41, 207–224. doi: 10.1007/s00300-017-2182-4
Sivel E., Planque B., Lindstrøm U., Yoccoz N. G. (2021). Multiple configurations and fluctuating trophic control in the Barents Sea food-web. PloS One 16, e0254015. doi: 10.1371/journal.pone.0254015
Spreen G., Kaleschke L., Heygster G. (2008). Sea ice remote sensing using AMSR-E 89-GHz channels. Journal of Geophysical Research 113, C02S03. doi: 10.1029/2005JC003384
Stein R., Fahl K., Gierz P., Niessen F., Lohmann G. (2017). Arctic Ocean sea ice cover during the penultimate glacial and the last interglacial. Nat. Commun. 8, 1–13. doi: 10.1038/s41467-017-00552-1
Sun M. Y., Carroll M. L., Ambrose W. G., Clough L. M., Zou L., Lopez G. R. (2007). Rapid consumption of phytoplankton and ice algae by arctic soft-sediment benthic communities: Evidence using natural and 13C-labeled food materials. J. Mar. Res. 65, 561–588. doi: 10.1357/002224007782689094
Sun M. Y., Clough L. M., Carroll M. L., Dai J., Ambrose W. G., Lopez G. R. (2009). Different responses of two common Arctic macrobenthic species (Macoma balthica and monoporeia affinis) to phytoplankton and ice algae: Will climate change impacts be species specific? J. Exp. Mar. Biol. Ecol. 376, 110–121. doi: 10.1016/j.jembe.2009.06.018
Tamelander T., Reigstad M., Hop H., Carroll M. L., Wassmann P. (2008). Pelagic and sympagic contribution of organic matter to zooplankton and vertical export in the Barents Sea marginal ice zone. Deep-Sea Res. Part II: Topical Stud. Oceanog. 55, 2330–2339. doi: 10.1016/j.dsr2.2008.05.019
Tamelander T., Renaud P., Hop H., Carroll M., Ambrose W. G., Hobson K. A. (2006). Trophic relationships and pelagic–benthic coupling during summer in the Barents Sea marginal ice zone, revealed by stable carbon and nitrogen isotope measurements. Mar. Ecol. Prog. Ser. 310, 33–46. doi: 10.3354/meps310033
Wassmann P., Carmack E. C., Bluhm B. A., Duarte C. M., Berge J., Brown K., et al. (2020). Towards a unifying pan-arctic perspective: A conceptual modelling toolkit. Prog. Oceanog. 189, 102455. doi: 10.1016/j.pocean.2020.102455
Wassmann P., Ratkova T., Andreassen I., Vernet M., Pedersen G., Rey F. (1999). Spring bloom development in the marginal ice zone and the central Barents Sea. Mar. Ecol. 20, 321–346. doi: 10.1046/j.1439-0485.1999.2034081.x
Wassmann P., Reigstad M. (2011). Future Arctic Ocean seasonal ice zones and implications for pelagic-benthic coupling. Oceanography 24, 220–231. doi: 10.5670/oceanog.2011.74
WoRMS Editorial Board (2022). World register of marine species. World Register of Marine Species (Accessed 2022-08-01) doi: 10.14284/170
Yunda-Guarin G., Brown T. A., Michel L. N., Saint-Béat B., Amiraux R., Nozais C., et al. (2020). Reliance of deep-sea benthic macrofauna on ice-derived organic matter highlighted by multiple trophic markers during spring in Baffin Bay, Canadian Arctic. Elementa: Sci. Anthropocene 8. doi: 10.1525/elementa.047
Keywords: highly branched isoprenoids (HBI), sympagic-benthic coupling, biomarker, organic matter, seasonal ice cover, Arctic megabenthos
Citation: Cautain IJ, Last KS, McKee D, Bluhm BA, Renaud PE, Ziegler AF and Narayanaswamy BE (2022) Uptake of sympagic organic carbon by the Barents Sea benthos linked to sea ice seasonality. Front. Mar. Sci. 9:1009303. doi: 10.3389/fmars.2022.1009303
Received: 01 August 2022; Accepted: 11 October 2022;
Published: 11 November 2022.
Edited by:
Eleonora Puccinelli, Université de Bretagne Occidentale, FranceReviewed by:
Baptiste Le Bourg, Station de Biologie Marine et Marinarium de Concarneau, FranceGuillaume Bridier, Université du Québec à Rimouski, Canada
James Lovvorn, Southern Illinois University Carbondale, United States
Copyright © 2022 Cautain, Last, McKee, Bluhm, Renaud, Ziegler and Narayanaswamy. This is an open-access article distributed under the terms of the Creative Commons Attribution License (CC BY). The use, distribution or reproduction in other forums is permitted, provided the original author(s) and the copyright owner(s) are credited and that the original publication in this journal is cited, in accordance with accepted academic practice. No use, distribution or reproduction is permitted which does not comply with these terms.
*Correspondence: Ivan J. Cautain, SXZhbi5DYXV0YWluQHNhbXMuYWMudWs=