- Key Laboratory of Integrated Rice-Fish Farming Ecology, Ministry of Agriculture and Rural Affairs, Freshwater Fisheries Research Center, Chinese Academy of Fishery Sciences, Wuxi, China
In intensive inland fish farming, discharge of untreated effluents adversely affects adjacent water bodies and causes water pollution. Thus, it is highly necessary to treat the effluents from inland fish farm. In this study, we built a commercial-scale integrated constructed wetland (CW) system with vertical subsurface flow, and monitored the purifying effect. During fish farming and discharge of effluents periods, the water samples were collected to detected the total nitrogen (TN), total phosphorus (TP), ammonia nitrogen (NH4+-N), nitrate nitrogen (NO3--N), nitrite nitrogen (NO2--N) and chemical oxygen demand (CODMn). Results showed that the system was stable and significantly improved water quality from fish pond. During the fish farming period, the removal efficiency for TN, TP, NH4+-N, NO3--N, NO2--N, and COD was 24.93–43.72%, 61.92–72.18%, 56.29–68.63%, 56.66–64.81%, 56.42–64.19% and 28.37–42.79%, respectively. Similarly, these parameters were also markedly decreased by the integrated CW system during sewage discharge period, and the average total removal rate for TN, TP, NH4+-N, NO3--N, NO2--N, and COD was 50.24%, 64.48%, 61.36%, 62.65%, 56.16% and 37.32%, respectively. It was worth noting that three key parameters for effluents detection TN, TP and COD values were below the threshold values of water quality of Class II in freshwater sewage discharge standard of China (SCT9101-2007). In conclusion, this study evidently demonstrated that application of CW system was an environmental sustainable sewage treatment strategy in intensive inland fish farming.
Introduction
Aquaculture products are key protein source in the global food production, which are increasing constantly in recent decades (FAO, 2020). They are mainly from marine water culture, inland freshwater culture and capture fisheries. In China, inland freshwater culture is the most important farming model, with a total area of 5,116.32 thousands ha and production of 30.14 million tons in 2019 (Yu et al., 2020). In the intensive inland freshwater farming, high-quality freshwater inflow was required. In turn, discharge of effluents can contaminate adjacent water bodies, which causes many environmental issues.
Like livestock farming and husbandry, large amounts of waste in aquaculture are generated, such as uneaten feed, feces, and dissolved material, which may be released into surface water and cause water pollution (Sindilariu et al., 2009). The pollution problem has aroused widespread concern as it exerts an adverse effect to aquatic ecological integrity (Turcios and Papenbrock, 2014). Excessive input nitrogen (N) and phosphorus (P) in aquatic ecosystems can promote the growth of algae and other phytoplankton, and subsequently cause dissolved oxygen (DO) depletion, water quality deterioration, and even death of aquatic animals (Bhateria and Jain, 2016; Wang et al., 2021). In China, the discharge standard of aquaculture effluents has been issued to prevent the harmful environmental effect (SCT9101-2007) (Ministry of Agriculture and Rural Affairs, 2007). However, existing methods for the treatment of aquaculture effluents, such as bio-filter and physical filter, require high investment and increase the cost of farming, which is beyond the reach of most of small producers. Therefore, it is highly necessary to seek effective method with a relatively low cost to address sustainability issues of inland fish farming.
Constructed wetland (CW) is one of the most promising methods for dealing with aquaculture effluents as it possesses some advantages, such as low cost, ecological benefit and ease of operation and maintenance (Tepe, 2018; Hang Pham et al., 2021). When integrated with special matrix and aquatic plants, CW can effectively remove many types of contaminants from wastewater, including organic matter, N, P, suspended solids, heavy metals and pathogenic microorganism through the coordinated action of biological, physical and chemical processes (Almuktar et al., 2018; Wang et al., 2021). Meanwhile, substrate microorganisms (e.g. nitrifying bacteria and denitrifying bacteria) play a pivotal role in conversion of nutrients, especially N, in wastewater (Liang et al., 2003; Li et al., 2018).
In aquaculture, CW has been applied to treat the discharged effluents from farms (Schulz et al., 2003; Sindilariu et al., 2007), or as a water filter unit to connect with recirculating aquaculture system (Zhang et al., 2010; Shi et al., 2011). According to surface area, the CW is classified into lab-scale CW (< 10 m2) (Saeed and Sun, 2013; Jesus et al., 2017), pilot-scale CW (10-100m2) (Papaevangelou et al., 2016; Uggetti et al., 2016) and commercial-scale CW (> 100 m2) (Tilley et al., 2002; Shi et al., 2011) in research or farming practice regarding aquaculture. Early study reported that a hybrid free water surface-horizontal subsurface flow CW (surface area of 5 m2) could remove 95% of total inorganic N and 32-71% of P in effluents from milkfish (Chanos chanos) pond (Lin et al., 2002). Horizontal subsurface flow CW (28 m2) effectively reduced the total suspended solids (TSS), chemical oxygen demand (COD), total nitrogen (TN) and total phosphorus (TP) concentrations in effluents form the flow-through trout fish farm (Chazarenc et al., 2007). Vertical flow CW (80 m2) for treatment of aquaculture ponds water proved to be very effective and the reduction amounted to 61.5% of ammonia nitrogen ( -N), 68% of nitrate nitrogen ( -N) and 20% of P (Li et al., 2007). Shi et al. (2011) built a combination CW (221.0 m2) to purify brackish effluent from commercial recirculating and super-intensive shrimp farming systems, and it exhibited good removal of TN (67%), TAN (71%) and TSS (66%). It is apparent that the most of these studies were conducted at lab-scale and pilot-scale systems. However, there was relatively little published research regarding practical application of a commercial-scale CW in aquaculture of China, and thus, its treatment performance needs to be further evaluated.
Based on the issues addressed above, we built a commercial-scale integrated CW system (2,400 m2) with vertical subsurface flow, which connected with fish ponds with a total surface area of 19,200 m2 to form a recirculating pond system. The objective of this study was to evaluate the performance of the CW system to manage the pond water quality with no water exchange during the farming period. Further, we examine whether the system improved the quality of effluents to comply with the discharge standard at the end of farming.
Materials and methods
Aquaculture system and constructed wetland design
The experiment was conducted in Wujiang modern agriculture industrial park (Suzhou, China), where we built an integrated CW system with a vertical subsurface flow. The CW system was connected with 7 fish ponds with a total surface area of 19,200 m2 to form a recirculating pond system (Figure S1).
The CW system (total surface area 2,400 m2) was consisted of sedimentation pond (250 m2), aeration pond (250 m2), constructed wetland (1,800 m2) and stabilize water pond (100 m2) (Figure S1). The wetland was filled with three layers of media. The bottom was filled with 30 cm of cobblestone (diameter 8-10 cm), the middle layer was filled with 25 cm of pelelith (diameter 4-6 cm) and the top was filled 25 cm of pelelith (diameter 2-4 cm). Three types of macrophytes Thalia dealbata, Iris germanica and Canna indica were selected to plant into the wetland due to stronger uptake capacity of N, P and metal. The planning density was one plant/m2. The mean hydraulic loading rate (HLR) was 0.6 m3/m2 d and hydraulic retention time (HRT) was 0.83 d. The water in CW-fish pond recirculating system was not renewed during the experiment. The system ran from early Jun to late November 2020.
Fish culture
The largemouth bass (Micropterus salmoides) were provided by Suzhou Tongli Modern Agriculture Development Co. LTD (Suzhou, China). The stocking density was 30,000 fish/hm2 with an average initial weight of 20 ± 2 g/fish. The fish were fed on a commercial pellet diet containing 47% crude protein, 5% crude lipids, 18.0% crude ash and 3.0% crude fiber (Changzhou Haida Biological Feed Co., Ltd, Changzhou, China). During the farming period, the fish were fed 3–4 times daily and feeding amount was approximately 2–4% of the fish weight. The management of fish farming was operated according to the method reported by Sun (Sun, 2020). The use of fish was approved by the Freshwater Fisheries Research Centre (FFRC) of the Chinese Academy of Fishery Sciences, Wuxi, China.
Water sampling and parameters detection
During the fish farming period, water samples were collected from the fish pond, sedimentation pond, aeration pond (inlet of wetland) and outlet of wetland once per 24-25 days from July 10 to October 20, 2020. During the sewage discharge period, water samples were collected from the fish pond, sedimentation pond, aeration pond (inlet of wetland) and outlet of wetland once per 9 days from November 1 to November 21, 2020. In each sampling site, we randomly collected the water samples via the five-point sampling method (Jiang et al., 2017).
The temperature and DO were detected using an YSI-DO 200 (YSI Inc., Ohio, USA), and the pH was measured using PHS-3CT acidometer (Shanghai Huyueming Scientific Instrument Co., LTD, China).The water quality parameters including CODMn, TN, -N, -N, -N and TP were measured using standard methods (Wei, 2002). The COD content was measured using potassium permanganate as an oxidant. The TN concentration was detected according to alkaline potassium persulfate digestion method. The TP concentration was determined by ammonium molybdate spectrophotometric method. The -N concentration was tested with UV spectrophotometry method. The -N was examined using N-(1-naphthalene) –ethylenediamine as a chromogenic agent. The -N concentration was analyzed by nesslers reagent spectrophotometry method. Removal efficiency (%) of the CW for each water quality parameter was calculated using following equation.
Where C0 and C1 is the concentration of the target parameter in outflow and inflow of wetland, respectively.
Statistical analysis
Data are analyzed using SPSS 24.0 and values are expressed as the mean ± standard error of the mean (SEM). Normal distribution was assessed by Kolmogorov–Smirnov test, and homogeneity of variance was examined by Levene teste. Difference among different groups were analyzed by One-way analysis of variance (ANOVA) with LSD post hoc test. P value less than 0.05 were considered to be statistically significant.
Results
Running parameters of the integrated CW system
During the experiment period, there was a similar change of temperature in fish pond, sedimentation pond, aeration pond, and CW outlet, ranging from 20.7°C to 32.2°C. Average pH value was 7.31 ± 0.15, 7.29 ± 0.19, 7.17 ± 0.34 and 7. 09 ± 0.28 in fish pond, sedimentation pond, aeration pond and CW outlet, respectively. The average DO value was 5.82 ± 0.51 mg/L, 5.07 ± 0.37 mg/L, 6.38 ± 0.58 mg/L and 2.07 ± 0.29 mg/L in fish pond, sedimentation pond, aeration pond and CW outlet, respectively. The DO reduction showed an oxygen depletion in the CW, which may be related to the aerobic respiration of heterotrophic microorganisms (Hang Pham et al., 2021). During the experiment period, the integrated CW system operated stably.
Changes of water quality parameters during fish farming period
During the fish farming period, the TN concentration successively decreased when the water flowed through sedimentation pond, aeration pond and CW, and significant difference was observed between CW inlet and CW outlet (p< 0.05; Figure 1A). The average TN concentration was 8.65 mg/L, 8.25 mg/L, 7.82 mg/L and 5.33 mg/L in fish pond, sedimentation pond, aeration pond (CW inlet) and CW outlet, respectively. Total TN removal rate of the integrated CW system ranged from 24.93 to 43.73% during the monitoring period (Figure 1B).
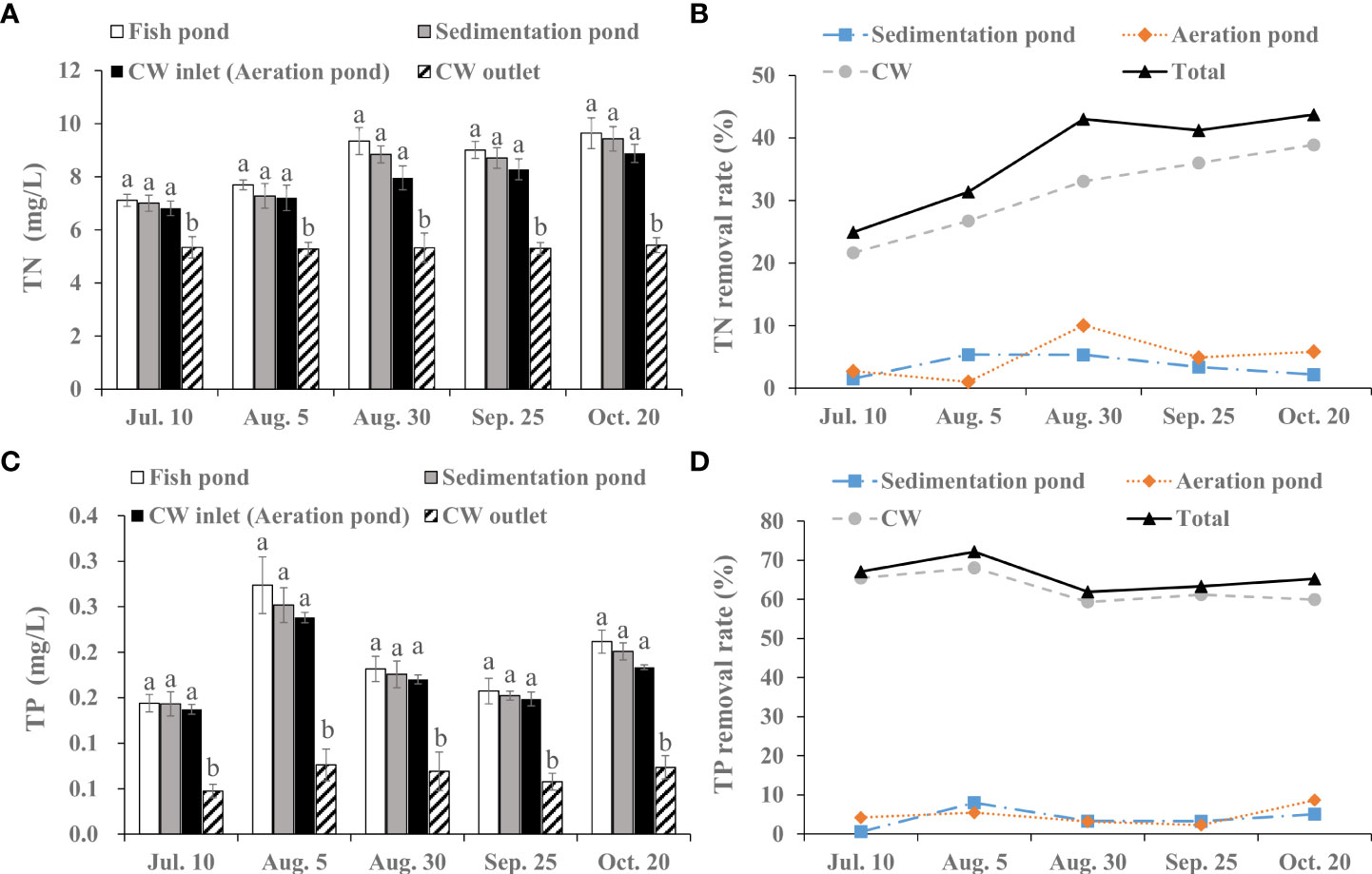
Figure 1 Removal efficiency of integrated CW system on TN and TP during the fish farming period. (A) TN value in the fish pond, sedimentation pond, CW inlet (aeration pond) and CW outlet. (B) TN removal rate of the sedimentation pond, aeration pond and CW. (C) TP value in the fish pond, sedimentation pond, CW inlet (aeration pond) and CW outlet. (D) TP removal rate of the sedimentation pond, aeration pond and CW. The value is expressed as mean ± SE (n = 5). Different small letters in bar graph indicate significant difference (p< 0.05) among fish pond, sedimentation pond, CW inlet (aeration pond) and CW outlet.
The integrated CW system significantly removed TP concentration in effluents from fish pond, and TP value at the CW inlet was clearly higher than that at the CW outlet (p< 0.05; Figure 1C). Meanwhile, there was a stable total removal rate on TP ranging from 61.92 to 72.19%. Average removal rate was 4.02%, 4.76% and 62.79% in sedimentation pond, aeration pond and CW, respectively (Figure 1D).
As shown in Figure 2A, average -N concentration in water generally decreased from fish pond to CW outlet, and the decrease exhibited significant difference between the CW inlet and CW outlet during fish farming period (p< 0.05). Average reduction rate of -N was 2.75%, 2.82% and 60.81% in the sedimentation pond, aeration pond and CW, respectively (Figure 2B).
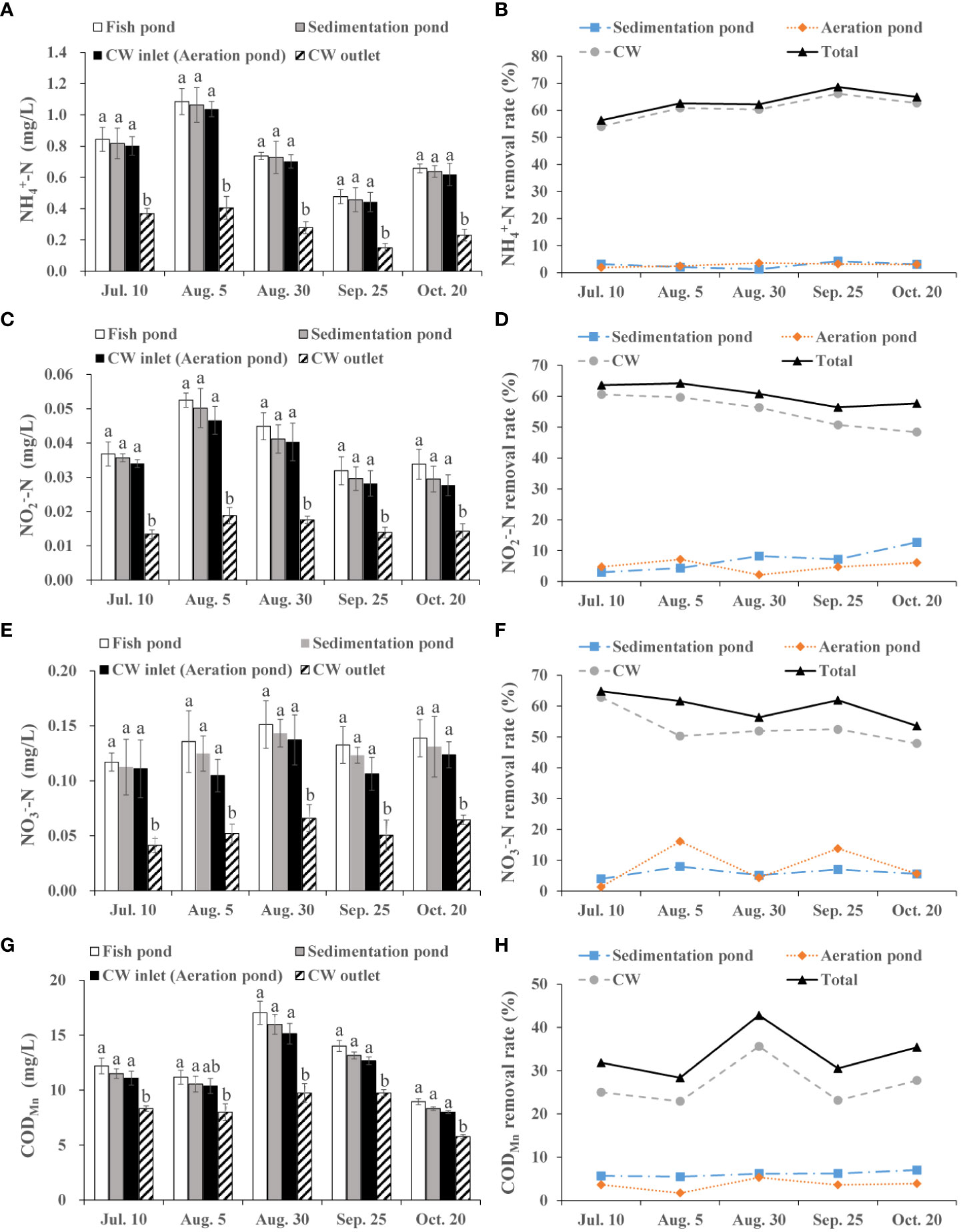
Figure 2 Removal efficiency of integrated CW system on NH4+-N, NO3--N, NO2--N and CODMn during the fish farming period. (A) NH4+-N value in the fish pond, sedimentation pond, CW inlet (aeration pond) and CW outlet. (B) NH4+-N removal rate of the sedimentation pond, aeration pond and CW. (C) NO2--N value in the fish pond, sedimentation pond, CW inlet (aeration pond) and CW outlet. (D) NO2--N removal rate of the sedimentation pond, aeration pond and CW. (E) NO3--N value in the fish pond, sedimentation pond, CW inlet (aeration pond) and CW outlet. (F) NO3--N removal rate of the sedimentation pond, aeration pond and CW. (G) CODMn value in the fish pond, sedimentation pond, CW inlet (aeration pond) and CW outlet. (H) CODMn removal rate of the sedimentation pond, aeration pond and CW. The value is expressed as mean ± SE (n = 5). Different small letters in bar graph indicate significant difference (p< 0.05) among fish pond, sedimentation pond, CW inlet (aeration pond) and CW outlet.
The variation of -N level followed a similar trend to -N, decreasing from 0.04 mg/L (mean) at the fish pond to 0.016 mg/L (mean) at the CW outlet when the water flowed through sedimentation pond, aeration pond and CW (Figure 2C). Specifically, -N level was obviously reduced at the CW outlet compared with the CW inlet during the July 10–October 20 (p< 0.05), and average total reduction rate of -N was 60.54% (Figure 2D).
The integrated CW system showed a high removal effect for -N, with an average total removal rate of 59.66% (Figure 2F). In addition, average -N concentration was 0.135 mg/L, 0.127 mg/L, 0.117 mg/L and 0.055 mg/L in fish pond, sedimentation pond, aeration pond (CW inlet) and CW outlet, respectively, and there was a significant difference between the CW inlet and CW outlet (p< 0.05; Figure 2E).
The CODMn is frequently used to evaluate COD content in aquaculture effluents. Concentration of CODMn displayed a nonlinear decrease in different sampling time when the water flowed through sedimentation pond, aeration pond and CW (Figure 2G). Notably, CODMn value at CW inlet was higher than that at CW outlet (p< 0.05). Meanwhile, this system showed a stable removal efficiency for CODMn with 30.51–42.79% removal rate (Figure 2H).
Changes of water quality parameters during the effluents discharge period
During the effluents discharge period, average TN concentration decreased from 9.89 mg/L to 4.92 mg/L when the water from fish pond flowed through the CW system (Figure 3A). Compared with CW inlet, TN value was clearly reduced at CW outlet (p< 0.05), with an average reduction rate of 43.17% (Figure 3B).
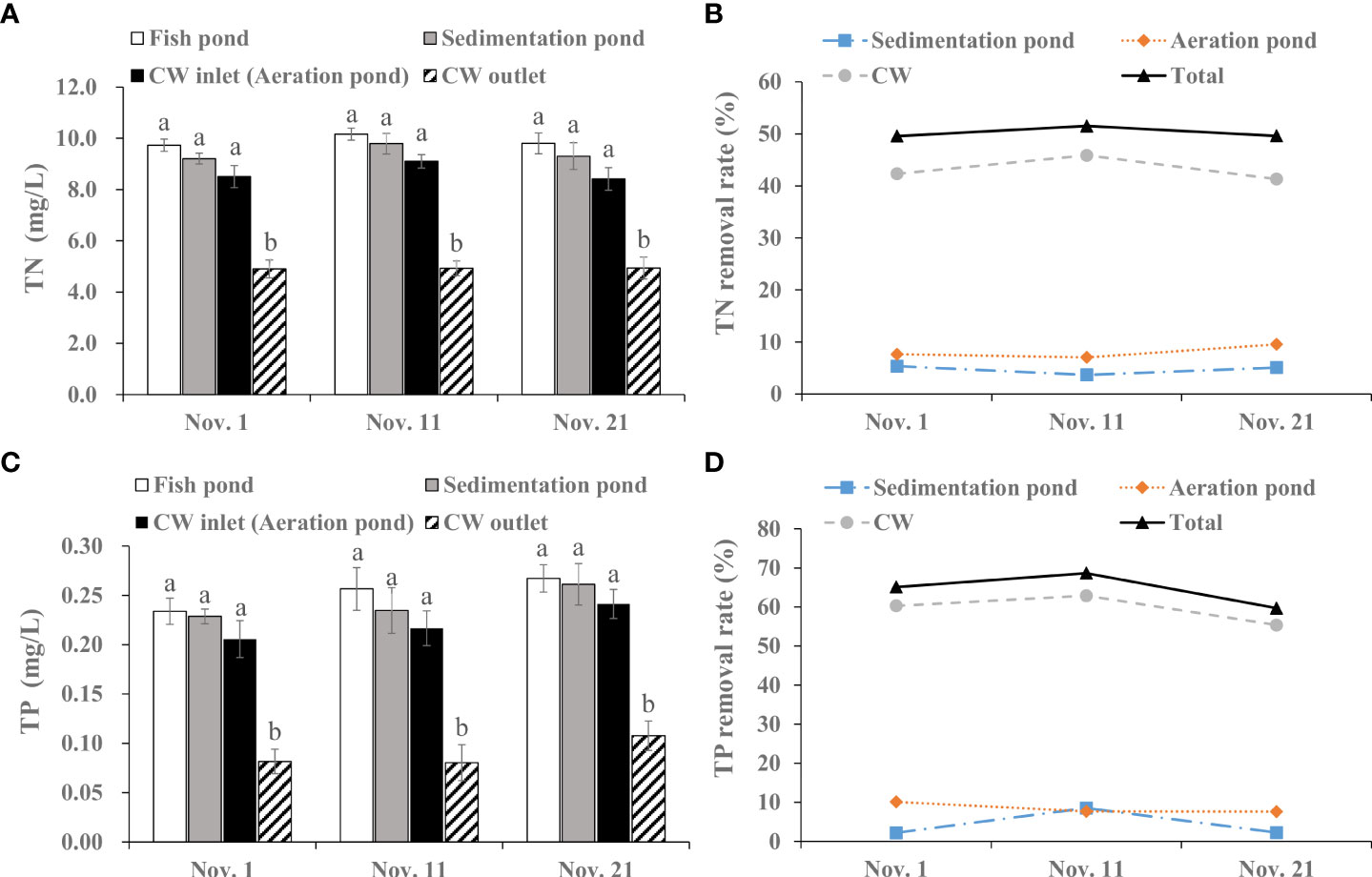
Figure 3 Removal efficiency of integrated CW system on TN and TP during the effluents discharge period. (A) TN value in the fish pond, sedimentation pond, CW inlet (aeration pond) and CW outlet. (B) TN removal rate of the sedimentation pond, aeration pond and CW. (C) TP value in the fish pond, sedimentation pond, CW inlet (aeration pond) and CW outlet. (D) TP removal rate of the sedimentation pond, aeration pond and CW. The value is expressed as mean ± SE (n = 5). Different small letters in bar graph indicate significant difference (p< 0.05) among the fish pond, sedimentation pond, CW inlet (aeration pond) and CW outlet.
Similarly, The TP concentration was effectively reduced by the CW system. The TP concentration was prominently lowered from 0.25 mg/L at fish pond to 0.09 mg/L at CW outlet, with a relatively stable average removal rate of 64.48% (p< 0.05; Figure 3C). The removal rate was 2.18–8.49% in sedimentation pond, 7.16–10.10% in aeration pond and 55.37–62.89% in CW (Figure 3D).
As shown in Figure 4A, The -N concentration showed a decreasing tendency in water from fish pond to CW outlet, and there was a significant difference between the CW inlet and CW outlet (p< 0.05). The average removal rate was 5.73%, 9.81% and 54.57% in the sedimentation pond, aeration pond and CW, respectively (Figure 4B).
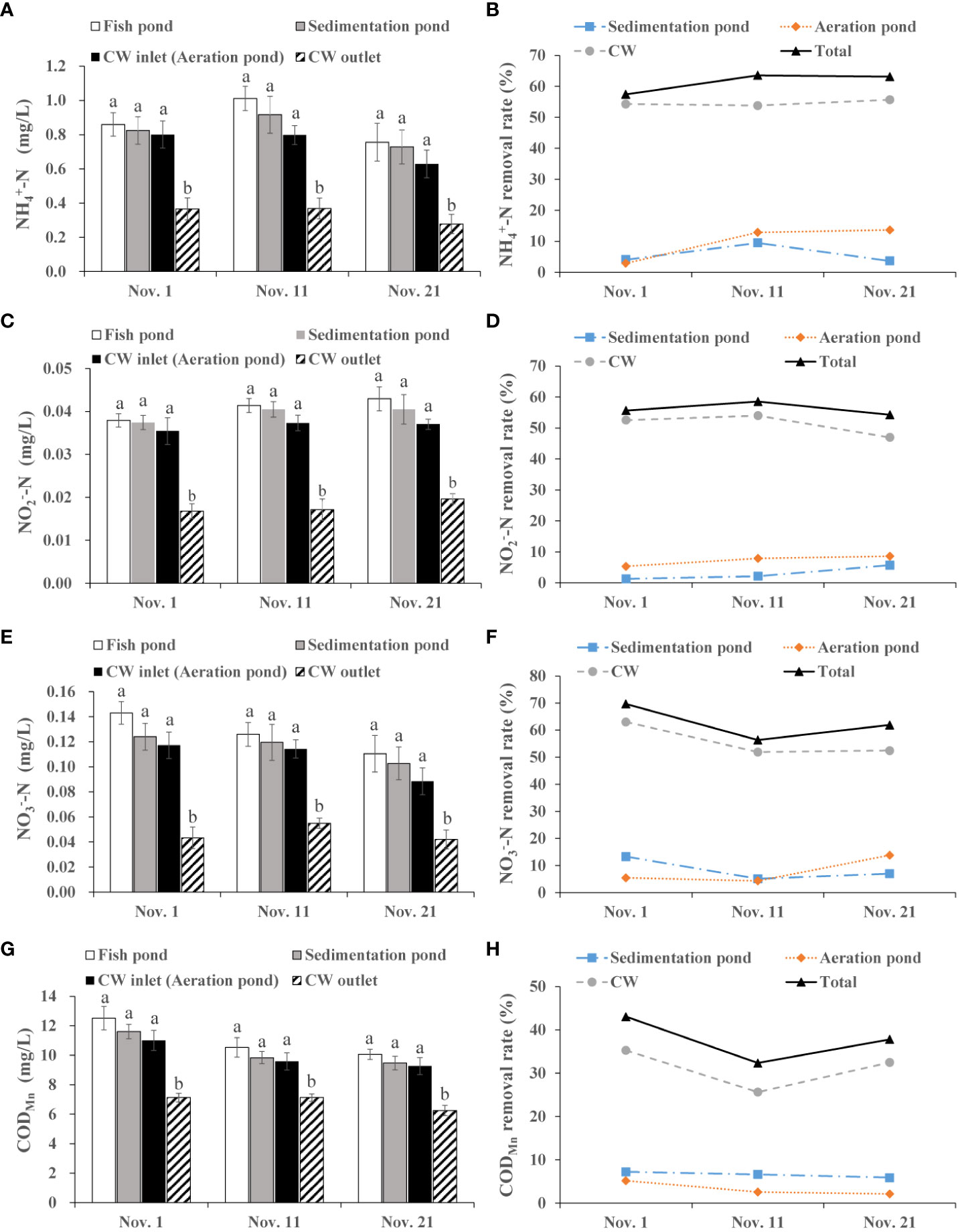
Figure 4 Removal efficiency of integrated CW system on NH4+-N, NO3-N, NO2--N and CODMn during the effluents discharge period. (A) NH4+-N value in the fish pond, sedimentation pond, CW inlet (aeration pond) and CW outlet. (B) NH4+-N removal rate of the sedimentation pond, aeration pond and CW. (C) NO2--N value in the fish pond, sedimentation pond, CW inlet (aeration pond) and CW outlet. (D) NO2--N removal rate of the sedimentation pond, aeration pond and CW. (E) NO3--N value in the fish pond, sedimentation pond, CW inlet (aeration pond) and CW outlet. (F) NO3--N removal rate of the sedimentation pond, aeration pond and CW. (G) CODMn value in the fish pond, sedimentation pond, CW inlet (aeration pond) and CW out. (H) CODMn removal rate of the sedimentation pond, aeration pond and CW. The value is expressed as mean ± SE (n = 5). Different small letters in bar graph indicate significant difference (p< 0.05) among fish pond, sedimentation pond, CW inlet (aeration pond) and CW outlet.
The -N concentration was successively decreased in fish pond, sedimentation pond, aeration pond (CW inlet) and CW outlet, and the decrease showed significant difference between the CW inlet and CW outlet (p< 0.05; Figure 4C). The integrated CW system has a stable removal rate ranging 54.27% to 58.55% during the effluents discharge period (Figure 4D).
Variation of the -N level followed a similar trend to -N. The average -N level was 0.126 mg/L, 0.115 mg/L, 0.107 mg/L and 0.047 mg/L in the fish pond, sedimentation pond, aeration pond (CW inlet) and CW outlet, respectively (Figure 4E). The integrated CW system strongly reduced -N level in the aquaculture effluents with a total average removal rate 62.65% (Figure 4F).
During the effluents discharge period, the average CODMn concentration was 11.03 mg/L, 10.30 mg/L, 9.95 mg/L and 6.84 mg/L in the fish pond, sedimentation pond, aeration pond and CW outlet, respectively (Figure 4G), and the value at CW outlet was apparently lower than that at other sampling sites (p< 0.05). Meanwhile, the integrated CW system has a distinct impact on CODMn reduction, and removal rate ranged from 32.33% to 43.04% (Figure 4H).
Discussion
In aquaculture effluents, inorganic N is a key pollutant, primarily generated from residual feeds and feces (Piedrahita, 2003). Overloading N can cause eutrophication, algal blooms and water anoxia, which may further lead to abnormal changes in physiology and behavior of aquatic animals (Banerjee et al., 2021). Thus TN level is a common parameter used to assess water quality in aquaculture. A CW (9.5 m2) was used to treat effluents from a recirculating aquaculture system farmed Oreochromis niloticus, and there was a 95.5% removal rate for TN (Behrends et al., 1999). Similarly, Lin et al. (2002) built a hybrid CW (5 m2) to treat effluents of Chanos chanos farming, and TN concentration was significantly removed by the CW system. Zhang et al. (2010) used a farm-scale CW (320 m2) to improve water quality in Ictalurus punctatus farming, and the TN removal rate was 48%. In addition, TN removal rate was related to types of CW systems, such as, 12.4% in floating-bed CW, 64.7% in horizontal subsurface flow CW, and 23.0% in surface flow CW (Bai et al., 2020). In the present study, the TN removal rate was 36.85% during the fish farming period and 50.24% during the effluents discharge period, indicating the integrated CW system has a high removal rate for inorganic N. In addition, the average TN value was 4.92 mg/L at CW outlet during the effluents discharge period, which met the water quality of Class II in the freshwater sewage discharge standard of China (SCT9101-2007).
In aquaculture practice, -N, -N and -N are ubiquitous pollutants, and have received the most attention due to high toxicity on aquatic animals (Molayemraftar et al., 2022). High levels of -N and -N have been reported to inhibit growth performance, induce tissue damage or even death in aquatic animals (Ip and Chew, 2010; Kroupova et al., 2018). Chronic -N exposure reduces growth, increases susceptibility to hypoxia, disrupts endocrine system and affects the health status in fish (Monsees et al., 2017; Kellock et al., 2018; Isaza et al., 2021). It has been reported that CW was an efficient and cost-effective management practice to maintain reasonable concentrations of -N, -N and -N (Lin et al., 2010). Mahmood et al. (2016) structured a CW-commercial ponds of Macrobrachium rosenbergii system, and found that the 43.8% -N, 25.7% -N and 14.3% -N were removed. In a lab-scale closed recirculation aquaculture system, horizontal subsurface flow CW removed 99% -N and 82–99% -N, but had lower removal rate for -N (10%) (Hang Pham et al., 2021). In this work, the The CW system exhibited a high and stable removal rate for -N (62.93%), -N (59.66%) and -N (60.54%), and improved substantially water quality form the fish pond during the fish farming period. It is worth noting that the average -N concentration at CW outlet was below 0.5 mg/L during the effluents discharge period, meeting environmental quality standard of Class II for surface water in China (GB3838-2002). The data also indicated that the integrated CW system had a high removal efficiency for inorganic N in aquaculture effluents, which reduced aquaculture-included environmental contamination.
In CW system, N removal is a complex process, which may be related to sedimentation, uptake of aquatic plant, adsorption of media and biodegradation (Liu et al., 2019; Lu et al., 2020). N as an essential nutrient can be absorbed by aquatic plants, thus some aquatic plants are planted in CW to remove N. CW planted Thalia dealbata and Pontederia cordata displayed 66.44% and 68.56% TN reduction rate and 54.09% and 77.39% -N reduction rate (Liu, 2011). In a CW planted Canna indica, the removal rate for -N was 45.3-84.5% (Zhu et al., 2004). In this study, the Thalia dealbata, Iris germanica and Canna indica were planted into the CW to clean the effluents from fish pond, which reflected that the inorganic N removal was related to uptake of the aquatic plants. However, some researchers suggested that CW removed N primarily through ammonification, nitrification and denitrification, but not aquatic plants uptake (Uusheimo et al., 2018; Liu et al., 2019; Lu et al., 2020). In addition, the substrates of CW, such as pebbles and pelelith, have been reported to have strong adsorption on N (Wang et al., 2018). Previous study suggested that, in commercial-scale CW system, TN removal rate was often around 40%, of which limiting factors were organic carbon concentration and low DO level (Xie et al., 2018; Lu et al., 2020). In this study, we speculated the major limiting factor for N removal was the DO level (2.07 ± 0.29 mg/L in CW outlet), because heterotrophic microorganisms played an important role in N removal process (Friedland, 2004).
Apart from N, P level is another important parameter evaluating water quality. P can promote algal growth and has a significant impact on microcystins production by Microcystis aeruginosa in aquatic environment (Dai et al., 2016). High concentration of P deteriorated not only water quality (Smith et al., 1999), but caused microcystins accumulation though enhancing Microcystis biomass (Wang et al., 2010). CW has the potential to treat P in aquaculture effluents. For example, CW as a recirculation filter in large-scale shrimp aquaculture was effective in removal of TP (65%) (Tilley et al., 2002); a laboratory-scale CW markedly removed the TP (average reduction rate 31%) from Litopenaeus vannamei farming water (Hang Pham et al., 2021). In line with previous studies, our data showed that the integrated CW system effectively removed TP with an average removal rate of 65.95% during the fish farming period. Interestingly, our data also displayed that the average TP concentration was 0.09 mg/L at CW outlet, which lower than the threshold value (0.5 mg/L) in the freshwater effluents discharge standard Class II of China (SCT9101-2007). P removal in CW is a manifold process including physical, chemical and biological forces (Sindilariu et al., 2007). It also depends on the ecological situations, type of CW and planted macrophytes (Kumar and Dutta, 2019). P as an essential mineral can be absorbed by macrophytes in CW system. Various macrophytes possesses different uptake capacity of P, such as 48.1% for Canna indica and 76% for Thalia dealbata (BU et al., 2010; Yang et al., 2021). In addition, the P removal was related to adherence capability of a range of filter media in CW (Vohla et al., 2011; Wu et al., 2015).
Like TN and TP, COD concentration is a key monitoring parameter during aquaculture effluents discharge period. It is also used to evaluate organic pollutant in aquatic environment. Numerous studies suggested that CW had a strongly positive impact on the removal of organic matter with 59.7–89.0% COD reduction rate (Tuszyńska and Obarska-Pempkowiak, 2008). In the current study, the COD concentration was also clearly reduced by CW system, with 28.37–42.79% reduction rate. Meanwhile, the average COD concentration was 6.84 mg/L, below the threshold value (15 mg/L) in freshwater effluents discharge standard of China (SCT9101-2007). In CW, the COD can be removed by sedimentation and filtration suspended solids (Hang Pham et al., 2021). However, our data showed that the COD removal rate of sedimentation was 5.88-7.33% which was much lower than that of CW, reflecting the COD may be removed mainly via filtration of CW media. On the other hand, biodegradation by aerobic and anaerobic microorganisms was also an important removal mechanism of organic matter (Kumar and Dutta, 2019). In the aerobic biodegradation process, oxygen transfer may be a limiting factor for COD removal. Thus, the low COD reduction in this study may be related to DO level (2.07 ± 0.29 mg/L at CW outlet).
Conclusion
In the study, we built a commercial-scale vertical subsurface flow CW connected with fish ponds, which run stably during the fish farming and effluents discharge periods. During the fish farming period, the integrated CW system had high and stable removal efficiency for TN of 24.93–43.72%, TP of 61.92–72.18%, -N of 56.29–68.63%, -N of 56.66–64.81% and -N of 56.42–64.19%. During the effluents discharge period, average value of TN, TP and COD, three key parameters for effluents detection, was 4.92 mg/L, 0.09 mg/L and 6.84 mg/L, respectively, which met the water quality of Class II in freshwater effluents discharge standard of China (SCT9101-2007). Finally, this study evidently demonstrated that application of CW was an environmental sustainable sewage treatment strategy in intensive fish culture system.
Data availability statement
The original contributions presented in the study are included in the article/Supplementary Material. Further inquiries can be directed to the corresponding author.
Author contributions
BL: Conceptualization, writing—original draft preparation, software; RJ: methodology, investigation validation, formal analysis, resources, YH: data cu-ration, writing—review and editing; JZ: visualization, supervision, project administration, funding acquisition. All authors read and approved the final version of the manuscript.
Funding
This study was supported by Central Public-interest Scientific Institution Basal Re-search Fund, CAFS (2020TD60), earmarked fund for CARS (CARS-45-20), and National Key R&D Program of China (2019YFD0900305).
Conflict of interest
The authors declare that the research was conducted in the absence of any commercial or financial relationships that could be construed as a potential conflict of interest.
Publisher’s note
All claims expressed in this article are solely those of the authors and do not necessarily represent those of their affiliated organizations, or those of the publisher, the editors and the reviewers. Any product that may be evaluated in this article, or claim that may be made by its manufacturer, is not guaranteed or endorsed by the publisher.
Supplementary material
The Supplementary Material for this article can be found online at: https://www.frontiersin.org/articles/10.3389/fmars.2022.1000703/full#supplementary-material
References
Almuktar S. A., Abed S. N., Scholz M. (2018). Wetlands for wastewater treatment and subsequent recycling of treated effluent: A review. Environ. Sci. pollut. Res. 25, 23595–23623. doi: 10.1007/s11356-018-2629-3
Bai X., Zhu X., Jiang H., Wang Z., He C., Sheng L., et al. (2020). Purification effect of sequential constructed wetland for the polluted water in urban river. Water 12, 1054. doi: 10.3390/w12041054
Banerjee S., Maity S., Guchhait R., Chatterjee A., Biswas C., Adhikari M., et al. (2021). Toxic effects of cyanotoxins in teleost fish: A comprehensive review. Aquat. Toxicol. 240, 105971. doi: 10.1016/j.aquatox.2021.105971
Behrends L. L., Houke L., Bailey E., Brown D. (1999). “Reciprocating subsurface flow constructed wetlands for treating high-strength aquaculture wastewater,” in Wetlands and Remediation Conference, Salt Lake City, Utah. 16–17.
Bhateria R., Jain D. (2016). Water quality assessment of lake water: A review. Sustain. Water Resour. Manage. 2, 161–173. doi: 10.1007/s40899-015-0014-7
BU F., Luo G., Xu X., Cao J., Shu W. (2010). Canna indica and acorus calamus ecological floating beds for purification of micro-polluted source water. China Water Wastewater 26, 14–17. doi: 10.19853/j.zgjsps.1000-4602.2010.03.006
Chazarenc F., Brisson J., Comeau Y. (2007). Slag columns for upgrading phosphorus removal from constructed wetland effluents. Water Sci. Technol. 56, 109–115. doi: 10.2166/wst.2007.499
Dai R. H., Wang P. F., Jia P. L., Zhang Y., Chu X. C., Wang Y. F. (2016). A review on factors affecting microcystins production by algae in aquatic environments. World J. Microbiol. Biotechnol. 32, 51. doi: 10.1007/s11274-015-2003-2
FAO. (2020). The State of World Fisheries and Aquaculture 2020. Sustainability in action (Rome). doi: 10.4060/ca9229e
Friedland J. M. (2004). Effectiveness of treatment and diversity of microbial populations within a constructed wetland treating wastewater (Morgantown, West Virginia: West Virginia University).
Hang Pham T. T., Cochevelou V., Khoa Dinh H. D., Breider F., Rossi P. (2021). Implementation of a constructed wetland for the sustainable treatment of inland shrimp farming water. J. Environ. Manage. 279, 111782. doi: 10.1016/j.jenvman.2020.111782
Ip Y. K., Chew S. F. (2010). Ammonia production, excretion, toxicity, and defense in fish: A review. Front. Physiol. 1, 134. doi: 10.3389/fphys.2010.00134
Isaza D. F. G., Cramp R. L., Franklin C. E. (2021). Exposure to nitrate increases susceptibility to hypoxia in fish. Physiol. Biochem. Zool. 94, 124–142. doi: 10.1086/713252
Jesus J. M., Cassoni A. C., Danko A. S., Fiúza A., Borges M.-T. (2017). Role of three different plants on simultaneous salt and nutrient reduction from saline synthetic wastewater in lab-scale constructed wetlands. Sci. Total Environ. 579, 447–455. doi: 10.1016/j.scitotenv.2016.11.074
Jiang J., Song Z., Yang X., Mao Z., Nie X., Guo H., et al. (2017). Microbial community analysis of apple rhizosphere around bohai gulf. Sci. Rep. 7, 1–9. doi: 10.1038/s41598-017-08398-9
Kellock K. A., Moore A. P., Bringolf R. B. (2018). Chronic nitrate exposure alters reproductive physiology in fathead minnows. Environ. pollut. 232, 322–328. doi: 10.1016/j.envpol.2017.08.004
Kroupova H. K., Valentova O., Svobodova Z., Sauer P., Machova J. (2018). Toxic effects of nitrite on freshwater organisms: a review. Rev. Aquac. 10, 525–542. doi: 10.1111/raq.12184
Kumar S., Dutta V. (2019). Constructed wetland microcosms as sustainable technology for domestic wastewater treatment: An overview. Environ. Sci. pollut. Res. 26, 11662–11673. doi: 10.1007/s11356-019-04816-9
Liang W., Wu Z.-b., Cheng S.-p., Zhou Q.-h., Hu H.-y. (2003). Roles of substrate microorganisms and urease activities in wastewater purification in a constructed wetland system. Ecol. Eng. 21, 191–195. doi: 10.1016/j.ecoleng.2003.11.002
Lin Y. F., Jing S. R., Lee D. Y., Chang Y. F., Sui H. Y. (2010). Constructed wetlands for water pollution management of aquaculture farms conducting earthen pond culture. Water Environ. Res. 82, 759–768. doi: 10.2175/106143010X12609736966685
Lin Y.-F., Jing S.-R., Lee D.-Y., Wang T.-W. (2002). Nutrient removal from aquaculture wastewater using a constructed wetlands system. Aquaculture 209, 169–184. doi: 10.1016/S0044-8486(01)00801-8
Liu Y. (2011). The ecological evaluation of to nitrogen and phosphorus removals of domestic sewage for constructed wetland plants (Nanchang China: Nanchang University).
Liu F.-f., Fan J., Du J., Shi X., Zhang J., Shen Y. (2019). Intensified nitrogen transformation in intermittently aerated constructed wetlands: Removal pathways and microbial response mechanism. Sci. Total Environ. 650, 2880–2887. doi: 10.1016/j.scitotenv.2018.10.037
Li G., Wu Z., Cheng S., Liang W., He F., Fu G., et al. (2007). Application of constructed wetlands on wastewater treatment for aquaculture ponds. Wuhan Univ. J. Natural Sci. 12, 1131–1135. doi: 10.1007/s11859-007-0116-7
Li B., Yang Y., Chen J., Wu Z., Liu Y., Xie S. (2018). Nitrifying activity and ammonia-oxidizing microorganisms in a constructed wetland treating polluted surface water. Sci. Total Environ. 628, 310–318. doi: 10.1016/j.scitotenv.2018.02.041
Lu J., Guo Z., Kang Y., Fan J., Zhang J. (2020). Recent advances in the enhanced nitrogen removal by oxygen-increasing technology in constructed wetlands. Ecotoxicol. Environ. Saf. 205, 111330. doi: 10.1016/j.ecoenv.2020.111330
Mahmood T., Zhang J., Zhang G. (2016). Assessment of constructed wetland in nutrient reduction, in the commercial scale experiment ponds of freshwater prawn macrobrachium rosenbergii. Bull. Environ. Contam. Toxicol. 96, 361–368. doi: 10.1007/s00128-015-1713-3
Ministry of Agriculture and Rural Affairs, C. (2007). Requirement for water discharge from freshwater aquaculture pond. (Beijing: Standards Press of China) pp. 1–4.
Molayemraftar T., Peyghan R., Jalali M. R., Shahriari A. (2022). Single and combined effects of ammonia and nitrite on common carp, cyprinus carpio: Toxicity, hematological parameters, antioxidant defenses, acetylcholinesterase, and acid phosphatase activities. Aquaculture 548, 737676. doi: 10.1016/j.aquaculture.2021.737676
Monsees H., Klatt L., Kloas W., Wuertz S. (2017). Chronic exposure to nitrate significantly reduces growth and affects the health status of juvenile Nile tilapia (Oreochromis niloticus l.) in recirculating aquaculture systems. Aquac. Res. 48, 3482–3492. doi: 10.1111/are.13174
Papaevangelou V. A., Gikas G. D., Tsihrintzis V. A., Antonopoulou M., Konstantinou I. K. (2016). Removal of endocrine disrupting chemicals in HSF and VF pilot-scale constructed wetlands. Chem. Eng. J. 294, 146–156. doi: 10.1016/j.cej.2016.02.103
Piedrahita R. H. (2003). Reducing the potential environmental impact of tank aquaculture effluents through intensification and recirculation. Aquaculture 226, 35–44. doi: 10.1016/S0044-8486(03)00465-4
Saeed T., Sun G. (2013). A lab-scale study of constructed wetlands with sugarcane bagasse and sand media for the treatment of textile wastewater. Bioresource Technol. 128, 438–447. doi: 10.1016/j.biortech.2012.10.052
Schulz C., Gelbrecht J., Rennert B. (2003). Treatment of rainbow trout farm effluents in constructed wetland with emergent plants and subsurface horizontal water flow. Aquaculture 217, 207–221. doi: 10.1016/S0044-8486(02)00204-1
Shi Y., Zhang G., Liu J., Zhu Y., Xu J. (2011). Performance of a constructed wetland in treating brackish wastewater from commercial recirculating and super-intensive shrimp growout systems. Bioresource Technol. 102, 9416–9424. doi: 10.1016/j.biortech.2011.07.058
Sindilariu P.-D., Brinker A., Reiter R. (2009). Factors influencing the efficiency of constructed wetlands used for the treatment of intensive trout farm effluent. Ecol. Eng. 35, 711–722. doi: 10.1016/j.ecoleng.2008.11.007
Sindilariu P.-D., Schulz C., Reiter R. (2007). Treatment of flow-through trout aquaculture effluents in a constructed wetland. Aquaculture 270, 92–104. doi: 10.1016/j.aquaculture.2007.03.006
Smith V. H., Tilman G. D., Nekola J. C. (1999). Eutrophication: impacts of excess nutrient inputs on freshwater, marine, and terrestrial ecosystems. Environ. pollut. 100, 179–196. doi: 10.1016/S0269-7491(99)00091-3
Sun L. (2020). Technical points of largemouth bass pond culture. Sci. Fish Farming 10, 39–40. doi: 10.3969/j.issn.1004-843X.2020.10.020
Tepe Y. (2018). “Treatment of effluents from fish and shrimp aquaculture in constructed wetlands,” in Constructed wetlands for industrial wastewater treatment (Chichester, UK: John Wiley & Sons, Ltd), 105–125.
Tilley D. R., Badrinarayanan H., Rosati R., Son J. (2002). Constructed wetlands as recirculation filters in large-scale shrimp aquaculture. Aquac. Eng. 26, 81–109. doi: 10.1016/S0144-8609(02)00010-9
Turcios A., Papenbrock J. (2014). Sustainable treatment of aquaculture effluents–what can we learn from the past for the future? Sustainability 6, 836–856. doi: 10.3390/su6020836
Tuszyńska A., Obarska-Pempkowiak H. (2008). Dependence between quality and removal effectiveness of organic matter in hybrid constructed wetlands. Bioresource Technol. 99, 6010–6016. doi: 10.1016/j.biortech.2007.12.018
Uggetti E., Hughes-Riley T., Morris R. H., Newton M. I., Trabi C. L., Hawes P., et al. (2016). Intermittent aeration to improve wastewater treatment efficiency in pilot-scale constructed wetland. Sci. Total Environ. 559, 212–217. doi: 10.1016/j.scitotenv.2016.03.195
Uusheimo S., Huotari J., Tulonen T., Aalto S., Rissanen A., Arvola L. (2018). High nitrogen removal in a constructed wetland receiving treated wastewater in a cold climate. Environ. Sci. Technol. 52, 13343–13350. doi: 10.1021/acs.est.8b03032
Vohla C., Kõiv M., Bavor H. J., Chazarenc F., Mander Ü. (2011). Filter materials for phosphorus removal from wastewater in treatment wetlands–a review. Ecol. Eng. 37, 70–89. doi: 10.1016/j.ecoleng.2009.08.003
Wang Q., Niu Y., Xie P., Chen J., Ma Z., Tao M., et al. (2010). Factors affecting temporal and spatial variations of microcystins in gonghu bay of lake taihu, with potential risk of microcystin contamination to human health. Sci. World J. 10, 1795–1809. doi: 10.1100/tsw.2010.172
Wang J., Wang W., Xiong J., Li L., Zhao B., Sohail I., et al. (2021). A constructed wetland system with aquatic macrophytes for cleaning contaminated runoff/storm water from urban area in Florida. J. Environ. Manage. 280, 111794. doi: 10.1016/j.jenvman.2020.111794
Wang H. X., Xu J. L., Sheng L. X., Liu X. J. (2018). A review of research on substrate materials for constructed wetlands. Mater. Sci. Forum 913, 917–929. doi: 10.4028/www.scientific.net/MSF.913.917
Wei F. (2002). Monitor and AnalysisMethod of water and wastewater (The fourth edition) (Beijing: Chinese Environmental Science Publication House).
Wu H., Zhang J., Ngo H. H., Guo W., Hu Z., Liang S., et al. (2015). A review on the sustainability of constructed wetlands for wastewater treatment: Design and operation. Bioresource Technol. 175, 594–601. doi: 10.1016/j.biortech.2014.10.068
Xie A., Chen H., You S. (2018). Advance of nitrogen removal in constructed wetland. IOP Conf. Ser.: Mater. Sci. Eng. 301, 012120. doi: 10.1088/1757-899X/301/1/012120
Yang X., Li Y., Wang H., Zhou J., Lv D. (2021). Purification effects of aquatic plants on nitrogen and phosphorus in eutrophic water bodies. J. Dali Univ. 6, 65–67. doi: 10.3969/j.issn.2096-2266.2021.12.013
Zhang S.-y., Zhou Q.-h., Xu D., He F., Cheng S.-p., Liang W., et al. (2010). Vertical-flow constructed wetlands applied in a recirculating aquaculture system for channel catfish culture: effects on water quality and zooplankton. Pol. J. Environ. Stud. 19, 1063–1070.
Keywords: constructed wetland, removal efficiency, total nitrogen, aquaculture effluents, recirculation aquaculture system
Citation: Li B, Jia R, Hou Y and Zhu J (2022) Treating performance of a commercial-scale constructed wetland system for aquaculture effluents from intensive inland Micropterus salmoides farm. Front. Mar. Sci. 9:1000703. doi: 10.3389/fmars.2022.1000703
Received: 22 July 2022; Accepted: 26 August 2022;
Published: 13 September 2022.
Edited by:
Ce Shi, Ningbo University, ChinaReviewed by:
Jiamin Sun, Shanghai Ocean University, ChinaKai Zhang, Pearl River Fisheries Research Institute (CAFS), China
Xiaona Ma, Jiangsu Ocean University, China
Copyright © 2022 Li, Jia, Hou and Zhu. This is an open-access article distributed under the terms of the Creative Commons Attribution License (CC BY). The use, distribution or reproduction in other forums is permitted, provided the original author(s) and the copyright owner(s) are credited and that the original publication in this journal is cited, in accordance with accepted academic practice. No use, distribution or reproduction is permitted which does not comply with these terms.
*Correspondence: Jian Zhu, emh1akBmZnJjLmNu