- 1Dipartimento di Scienze e Ingegneria della Materia, dell’Ambiente ed Urbanistica, Polytechnic University of Marche, Ancona, Italy
- 2Dipartimento di Scienze della Vita e dell’Ambiente, Polytechnic University of Marche, Ancona, Italy
- 3Dipartimento di Biotecnologie Marine, Stazione Zoologica Anton Dohrn, Fano Marine Centre, Fano, Italy
- 4Stazione Zoologica Anton Dohrn, Naples, Italy
- 5Dipartimento di Scienze e Tecnologie Biologiche ed Ambientali, University of Salento, Lecce, Italy
- 6Dipartimento di Biologia, University of Pisa, CoNISMa, Pisa, Italy
Present and past industrial activities in coastal areas have left us a legacy of contamination and habitat degradation with potential implications for human health. Here, we investigated a coastal marine area enclosed in a Site of National Interest (SNI) of the central-western Adriatic (Mediterranean Sea), where priority actions of environmental remediation are required by governmental laws due the high environmental and human risk, and that is off-limits to any human activity since 2002. In particular, our investigation was focused on an area located in front of a chemical industry dismissed more than 3 decades ago. We report that the concentrations of heavy-metal and organic contaminants in the investigated sediments were generally lower than those expected to induce detrimental biological effects. Meiofaunal abundance, biomass and community structure changed among stations, but regardless of the distance from the abandoned industrial plant. Taxa richness within the SNI did not change significantly compared to the controls and the lack of some taxa in the SNI transects was not due to the contamination of the SNI area. The results of this study suggest a natural recovery of the marine area over 2 decades of restrictions on human activities, including fishing and shipping bans. If the hypothesis of the natural recovery of this SNI will be further confirmed by other studies, the plans for the identification and monitoring of the most polluted areas in Italy should necessarily be redefined also in the light of the Water Framework, the Marine Strategy Framework and the Environmental Quality Standard Directives.
Introduction
Coastal zones represent key areas of interaction between ocean and land, and provide a wide range of ecosystem goods and services, such as carbon and nutrient cycling, climate regulation, food provision and recreational activities (Costanza et al., 1997; Barbier, 2012, 2017; Turner and Schaafsma, 2015). However, the rapid urbanization and industrialization of coastal zones and the consequent marine pollution, habitat degradation, alien species invasion and alteration of food webs are determining the loss of these goods and services (Todd et al., 2019).
The Mediterranean coasts host more than 200 petrochemical plants, energy installations and chemical plants (Civili, 2010) and only less than 1% of the total area remains relatively unaffected by human activities (Micheli et al., 2013). In most cases, despite the closure of industrial plants for many years, the ceased release of pollutants into the sea and/or the implementation of environmental regulations, the contamination still persists (Romano et al., 2004; Croudace et al., 2015; Gambi et al., 2020a).
Marine sediments represent the final sink for all contaminants, which can have impacts on marine life at all levels, from prokaryotes to metazoans (Hay Mele et al., 2020; Tangherlini et al., 2020), and especially on those benthic organisms, such as meiofauna, which are directly exposed to contaminants in their habitat (Gambi et al., 2020a). Such effects can be exacerbated by multiple stressors, including climate change (Micheli et al., 2013; Bertocci et al., 2019; Corinaldesi et al., 2022).
Meiofauna are the most abundant metazoans in benthic ecosystems of the world oceans (Fenchel and Finlay, 2004), including coastal environments, and some taxa (e.g., Nematoda) are known to be tolerant to chemical contamination (Snelgrove et al., 1997; Moens et al., 2013; Gambi et al., 2020a). However, investigations on the effects of chemical contamination on meiofaunal assemblages in coastal areas have reported that high levels of mixed contamination (e.g., heavy metals and hydrocarbons) may lead to a decrease in biodiversity (Danovaro et al., 1995; Gyedu-Ababio and Baird, 2006; Gambi et al., 2020a) although without a substantial loss of total abundance and biomass of organisms. Other investigations documented a decrease in abundance and biomass (Danovaro et al., 1995; Kang et al., 2014) and the dramatic deterioration of ecosystem functioning (Hay Mele et al., 2020).
Meiofauna are considered bioindicator organisms, which provide advantages over the use of macrofauna due to their higher abundance and species diversity, small size, rapid turnover, lack of larval dispersal and presence of both tolerant and sensitive species (Danovaro et al., 2009). Some studies have shown faster response of meiofauna compared to macrofauna (Balsamo et al., 2010) and a change in community structure at high concentrations of organic matter and contaminants resulting in a community shift toward more tolerant taxa (Moreno et al., 2008; Kandratavicius et al., 2018).
Coastal areas, especially when subjected to anthropogenic impacts, are often characterized by an altered trophic state, as a result of the organic nutrient enrichment (Dell’Anno et al., 2002). Phytopigment concentrations and the biochemical composition of the organic matter (in terms of proteins, carbohydrates and lipids) in marine sediments can change in response to different anthropogenic sources. For this reason these can be used as a proxy of the benthic trophic state (Dell’Anno et al., 2002). An altered trophic status may have consequences at different levels of ecosystem organization, including changes in the composition of meiofaunal assemblages (Pusceddu et al., 2011), thus providing information on the health of the ecosystem.
In the present study, we conducted the chemical characterization of the sediments and investigated their trophic state and the effects on meiofaunal assemblages in a coastal area of the central-western Adriatic (Mediterranean Sea) located in front of a chemical industry abandoned more than 3 decades ago. This area is currently included among the most heavily polluted sites in Italy (defined as “Sites of National Interest,” SNI), which are off-limits to any human activity (e.g., shipping, fishery and bathing), and where clean-up and remediation actions are required by Italian laws (Ausili et al., 2020). In particular, we compared the health conditions of the benthic ecosystems of the SNI area with those of the northern and southern sediments outside the SNI used as controls. Given the supposed criticality of the SNI in terms of pollution and risk for environmental health, we hypothesized a strong impact on marine benthic communities and trophic state of the investigated area.
Materials and Methods
Study Area
The investigated area, Falconara Marittima on the western coast of the central Adriatic Sea, has an extension of about 1,200 ha (Figure 1), and is included among the most heavily polluted sites in Italy (SNI). There, remediation actions are required by Italian laws and therefore any type of human activity is prohibited, including shipping, fishing and bathing (Ausili et al., 2020). This SNI is an area at high risk of environmental crisis (AERCA; Martuzzi et al., 2002) and also includes a marine area, located to the north, in front of the former Montedison plant, and to the south in front of the mouth of the Esino river and an oil refinery (used since the 1940s as a refining and storage station for petroleum products).
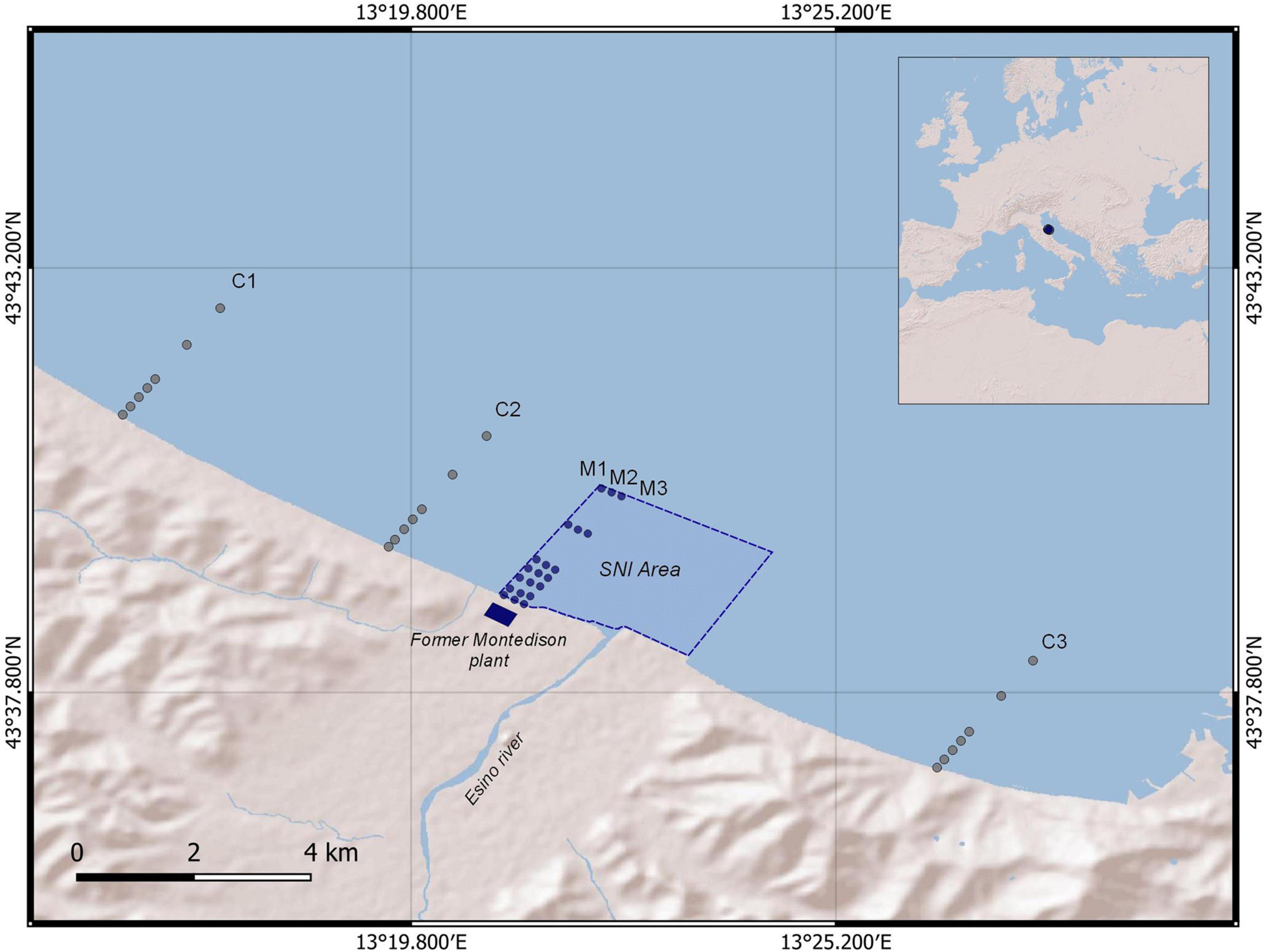
Figure 1. Sampling site and study area. The stations of the control transects (C1, C2, and C3) are represented as gray dots, the SNI transects (M1, M2, and M3) as blue dots and the perimeter of the SNI area as a blue dotted line. The small blue rectangle represents the location of the former Montedison plant.
In the study area the water circulation is cyclonic with permanent or seasonal gyres interconnected with coastal currents such as the Western Adriatic Current that flows southwards (Zavatarelli et al., 2000).
The study area (Figure 1) lies in front of the former Montedison industrial plant, whose activity dates back to 1919 when the production of superphosphate started. In 1944, the plant was acquired by the Montecatini Company, and used as a warehouse by the British Royal Army Service Corps. From 1966 to 1990 (decommissioning year) the chemical pole has produced fertilizers using pyrite and phosphorous, which have been reported to contaminate soil and groundwater of the surrounding area, together with heavy metals, fluorides, hydrocarbons and PAHs (Regione Marche, 2009).
Sampling Strategy
Sediment samples from a total of 42 stations were collected between September and November 2018 along 6 transects from the coast to open water (from 0 to 3 km Supplementary Table 1). Each transect included 7 stations along a bathymetric gradient (from 1 to 12 m depth, thus covering most of the bathymetric range of the circumscribed SNI area, Figure 1) at increasing distance from the potential source of contamination of the former Montedison plant.
In particular, 3 transects were placed in front of the former Montedison plant (defined as M1, M2, and M3) and 3 transects, defined as controls, were placed outside the SNI area: two on the northern side (C1 and C2) upstream of the SIN and the Western Adriatic Current that flows southward, and one on the southern side of the SNI (C3, Figure 1) downstream of the SNI. This sampling strategy was defined to balance the number of transects within the SNI and controls outside the SNI. In addition, we considered 7 sampling points along each transect to analyze the putative effect of contamination on meiofauna assemblages at a small spatial scale (order of magnitude of meters).
Sediment samples were collected at 1-m depth by SCUBA divers and at depths between 3 and 12 m, through a Van Veen grab (sampling surface: 0.08 m2) on board of the R/V Actea.
Surface sediment sub-samples (0–10 cm) for the analysis of inorganic and organic contaminants (see details below in the dedicated paragraph), grain size, quantity and biochemical composition of organic matter (used as a proxy of trophic state), and meiofaunal assemblages were collected from three independent grab deployments by means of Plexiglas manual corers (length: 30 cm, inner diameter: 3.6 cm). Three replicates were collected for each variable. The analyses of inorganic and organic contaminants were conducted in the M1 and M3 transects at all depths (as reported above) and in M2 transect only close to the putative contamination source (at 1-m) and in C1 and C3 transects.
Additional selected sub-superficial sediment layers (10–20 cm) were collected for the analysis of contaminants in the transects M2 and M3 (at 1-m depth, close to the putative contamination source) and C2. Once transported to the laboratory, all the sediment samples were stored at –27°C until the analyses. In addition, a CTD probe (Seabird 911-plus) was used to measure seawater temperature and salinity at all sampling stations.
Sedimentary Grain Size and Concentrations of Contaminants
Sediment grain size was determined by the sieving technique (Danovaro, 2010). Samples were treated with a 10% H2O2 solution to remove organic matter, and the total and non-biogenic grain size distribution determined. Gravel, sand, and silt sizes are reported as % contribution.
Heavy metals and metalloids were extracted from the sediment samples according to the EPA 3051 procedure, then the concentrations of As, Cd, Cr, Hg, Ni, Pb, and Cu were determined by inductively coupled plasma mass spectrometry (ICP-MS) (EPA3051 6020), while the concentrations of Al and Fe were determined by inductively coupled plasma optical emission spectrometry (ICP-OES) (EPA3051 6010). Polycyclic aromatic hydrocarbons and polychlorinated biphenyls (PCB) were extracted from the sediment samples according to the EPA 3545 procedure and analyzed by gas chromatography-mass spectrometry (GC-MS; EPA 8270). The total PAH concentrations were obtained summing those of the 16 congeners quantified (including naphthalene, acenaphthene, fluorene, acenaphthylene, phenanthrene, anthracene, fluoranthene, pyrene, benzo[a]anthracene, chrysene, benzo[b]fluoranthene, benzo[k]fluoranthene, benzo[a]pyr-ene, indeno[1,2,3,-cd]pyrene, dibenzo[a,h]anthracene, benzo[g,h,i] perylene). Moreover, the concentrations of LMW (low molecular weight) and HMW (high molecular weight) PAHs were calculated as the sum of the PAH congeners, distinguished based on their molecular weight. Aliphatic hydrocarbons with C < 12 were analyzed by gas chromatography equipped with a flame ionization detector (GC-FID) (EPA5021 8015), and aliphatic hydrocarbons with C > 12 were determined by GC-FID according to the method proposed by the Italian Institute for Environmental Protection and Research (Balzamo et al., 2012). The total organic carbon (TOC) content was analyzed by catalytic oxidation of C at high temperature (950°C) after hydrochloric acid-based elimination of carbonates (UNI13137, 2002). All analyses of contaminants were entrusted to an independent certified laboratory (Ambiente s.p.a.), accredited by the Italian accreditation body Accredia (accredia.it/en/). For all analytes, standards recovery was 100 ± 20%.
For the analysis of trace elements, ICP Multielement, PLR560 (Exaxol Italia Ltd.) was used as the standard. PAH mix ITPM-023 (Ultra Scientific Ltd.), PCB mix L002M010INEJ (Lab Service Analytica Ltd.), hydrocarbon standard RGO-320-1 (Ultra Scientific Ltd.) and Regular Unleaded Gasoline Solution, RGO-600-1/Unleaded Gasoline Solution, RGO-601-1 (Ultra Scientific Ltd.) were used as standard for PAH, PCB, C > 12 and C < 12 aliphatic hydrocarbon analyses, respectively. For the analysis of TOC the standard used was IQC-106-5, Ultra Scientific (Ltd.).
The mean-effects range medium-quotient (m-ERM-q quotient) was calculated for both heavy metals and PAHs based on the available ERM values for these contaminants (Kowalska et al., 2018). Stations were classified considering the four levels of m-ERM-q: low priority site (<0.1), medium-low priority site (0.1–0.5), high-medium priority site (0.5–1.5), and high priority site (> 1.5) with a 9, 21, 49, and 76% probability of being toxic, respectively (Long et al., 2000).
Biochemical Components of the Sedimentary Organic Matter
Chlorophyll-a, phaeopigment, protein, carbohydrate and lipid concentrations were analyzed according to Danovaro (2010). Briefly, chlorophyll-a and phaeopigments were analyzed fluorometrically (Lorenzen and Jeffrey, 1980; Danovaro, 2010) and total phytopigment concentrations were defined as their sum. Total phytopigments contents were used as a proxy of organic matter of algal origin including the living (chlorophyll-a) and detrital (phaeopigments) fractions (Pusceddu et al., 2009).
Protein, carbohydrate, and lipid concentrations were determined spectrophotometrically (Danovaro, 2010). Concentrations of proteins, carbohydrates, and lipids were converted into carbon equivalents using 0.49, 0.40, and 0.75 mgC mg–1, respectively, as conversion factors and their sum was defined as biopolymeric carbon (BPC, used as an index of trophic state, Pusceddu et al., 2000). The percentage contribution of phytopigment C concentrations to biopolymeric C contents was used to define the trophic state of the investigated sediments (Pusceddu et al., 2009).
Abundance, Biomass and Taxa Richness of Meiofaunal Assemblages
Sediment samples were sieved through 500 and 30-μm mesh to analyze meiofaunal assemblages after ultrasounds treatment. Decantation method was adopted for samples collected at 1 m water depth (due to grain size dominated by gravel and sand; Higgins and Thiel, 1988), whereas density gradient extraction method (using Ludox HS40, diluted to a final density of 1.31 g cm–3) was adopted for all other sediment samples (Danovaro, 2010).
After extraction, all samples were fixed in 4% formalin and stained with Rose Bengal (final concentration 0.5 g L−1, Danovaro, 2010). Meiofaunal samples were sorted, and organisms were counted and identified under a stereomicroscope (Zeiss, × 16–40 magnification) or optical microscope (Zeiss, × 40–100 magnification), when necessary (Heip et al., 1985; Danovaro, 2010).
Meiofaunal biomass was evaluated using bio-volumetric measurements. Nematodes biomass was calculated using the formula V = L × W2 × 0.063 × 10–5 (in which body length, L, and width, W, are expressed in μm; Andrassy, 1956), whereas the biomass of all other taxa was estimated using V = L × W2 × C, where C is a dimensionless factor (specific for each meiofaunal taxon) used to convert L × W2 to body volume, according to models relating body dimensions and volume (Feller and Warwick, 1988). Each body volume was multiplied by an average density of 1.13 g cm–3 to obtain the biomass (μg DW: μg WW = 0.25; DW- dry weight and WW- wet weight, after Wieser, 1960) and the carbon content was 40% of the dry weight (Feller and Warwick, 1988). Meiofaunal abundance was expressed as number of individuals in 10 cm2 and biomass as μgC × 10 cm–2 (Danovaro, 2010).
Meiofaunal taxa richness was determined as number of higher taxa encountered. Nematodes/copepodes (nauplii and adults) ratio was used as biological index (Raffaelli and Mason, 1981) as well as nematodes/kinorhynchs ratio, which was specifically used to assess the potential effect of organic enrichment (when kinorhynchs are absent, the ratio is considered as number of nematodes) (Mirto et al., 2012; Dal Zotto et al., 2016).
Statistical Analyses
To analyze the differences in terms of all variables investigated between SNI and control transects and among water depths, uni- and multivariate distance-based permutational analyses of variance were applied (PERMANOVA; Anderson, 2001; McArdle and Anderson, 2001). All the statistical analyses were carried out using the same experimental design, considering 3 factors as sources of variance: Condition (fixed, 2 levels: SNI and Control); Transect (random and nested in Condition, 3 levels for each Condition: northern control C1, northern control C2, southern control C3, SNI M1, SNI M2, and SNI M3) and Depth (random, 7 levels: 1, 3, 4, 5, 6, 10, 12 m).
Statistical tests to identify differences in organic matter variables (chlorophyll-a, phaeopigment, total phytopigment, protein, carbohydrate, lipid, and BPC concentrations), meiofaunal abundance, biomass and richness of taxa were based on matrixes of Euclidean distance whereas tests for meiofaunal taxonomic composition were based on Bray Curtis similarity matrixes (Anderson, 2001; McArdle and Anderson, 2001). To identify patterns of variability among depths along each transect and among transects at the same depth, pair-wise test were carried out. To visualize differences in the biochemical composition of organic matter and in the trophic state of the investigated stations, bi-plots after Canonical Analysis of Principal Coordinates (CAP) (Anderson and Willis, 2003) were used. Spearman’s correlation vectors were used to identify which variables better explained differences between conditions, transects and depths.
SIMPER analyses were used to determine the percentage dissimilarity in the meiofaunal taxonomic composition among conditions (C1, C2, C3, M1, M2, and M3), transects or depths (cut off value 90%) (Gray, 2000).
Multivariate multiple regression analyses were performed to determine whether meiofaunal taxonomic composition (used as the response variable) was potentially influenced by environmental characteristics (grain size, depth, temperature, and salinity), presence of different contaminants (PAHs, C > 12 and C < 12 hydrocarbons, PCB, heavy metals and metalloids such as As, Cu, Hg, Ni, Cr) and/or trophic resources (phytopigments, BPC). The DistLM was run using the forward model selection procedure and the R2 model selection criteria (Anderson et al., 2008). In the model, only the concentrations of contaminants present in the first 10 cm depth of the sediments were used since it represents the sediment layer where the majority of meiofaunal assemblages were encountered.
Uni- and multivariate PERMANOVA, pair wise tests, CAP, DistLM forward, SIMPER tests were carried out using the routines included in the software PRIMER 6 + (Clarke and Gorley, 2006).
Results
Environmental Variables
Sediment grain size, bottom water temperature and salinity in the 42 stations are reported in Supplementary Table 2. The grain size was quite uniform in all the transects investigated and was generally characterized by a high percentage of sand within 10-m depth (ranging within a relatively wide interval: from 39 to 99%). Between 10 and 12-m depth an increase of the quantitative relevance of the silt-clay fraction was observed (15–45%). The gravel fraction was generally very low (<5%) in all the stations investigated, except for 1 m depth station in the M1 SNI transect, where it accounted for ca. 60%.
Bottom water temperature in the control transects ranged from 19.62 to 21.27°C, whereas in the SNI transects from 19.93 to 20.97°C. Bottom water salinity in the control and SNI transects ranged from 37.59 to 37.92 and from 37.38 to 37.75, respectively.
Contaminants in Sediments
Heavy metal, metalloid, aliphatic hydrocarbon (C < 12 and C > 12), PAH and PCB concentrations are reported in Supplementary Tables 3–6.
Among inorganic contaminants, in the SNI transects, As concentrations ranged from 1.7 to 13 mg kg–1 in the stations located at 1 and 12-m depth in M1 and M3 transects, respectively and, in the control transects from 7.2 to 10 mg kg–1 in the stations located at 1 and 3-m depth in C3 transect, respectively (Figure 2A). A similar pattern was observed for Cr (overall range: 2.3–20.0 mg kg–1, Figure 2D), Cd (overall range: 0.05–0.13 mg kg–1, Figure 2C), Ni (overall range: 2.9–25.0 mg kg–1), Pb (overall: 1.4–7.3 mg kg–1), Va (overall range: 2.5–19.0 mg kg–1) and Zn (overall range: 6.4–34.0 mg kg–1) concentrations. The highest concentrations of Hg (0.03 mg kg–1, Figure 2B) and Cu (7.8 mg kg–1) were also observed in the station at 12 m depth in M3 transect.
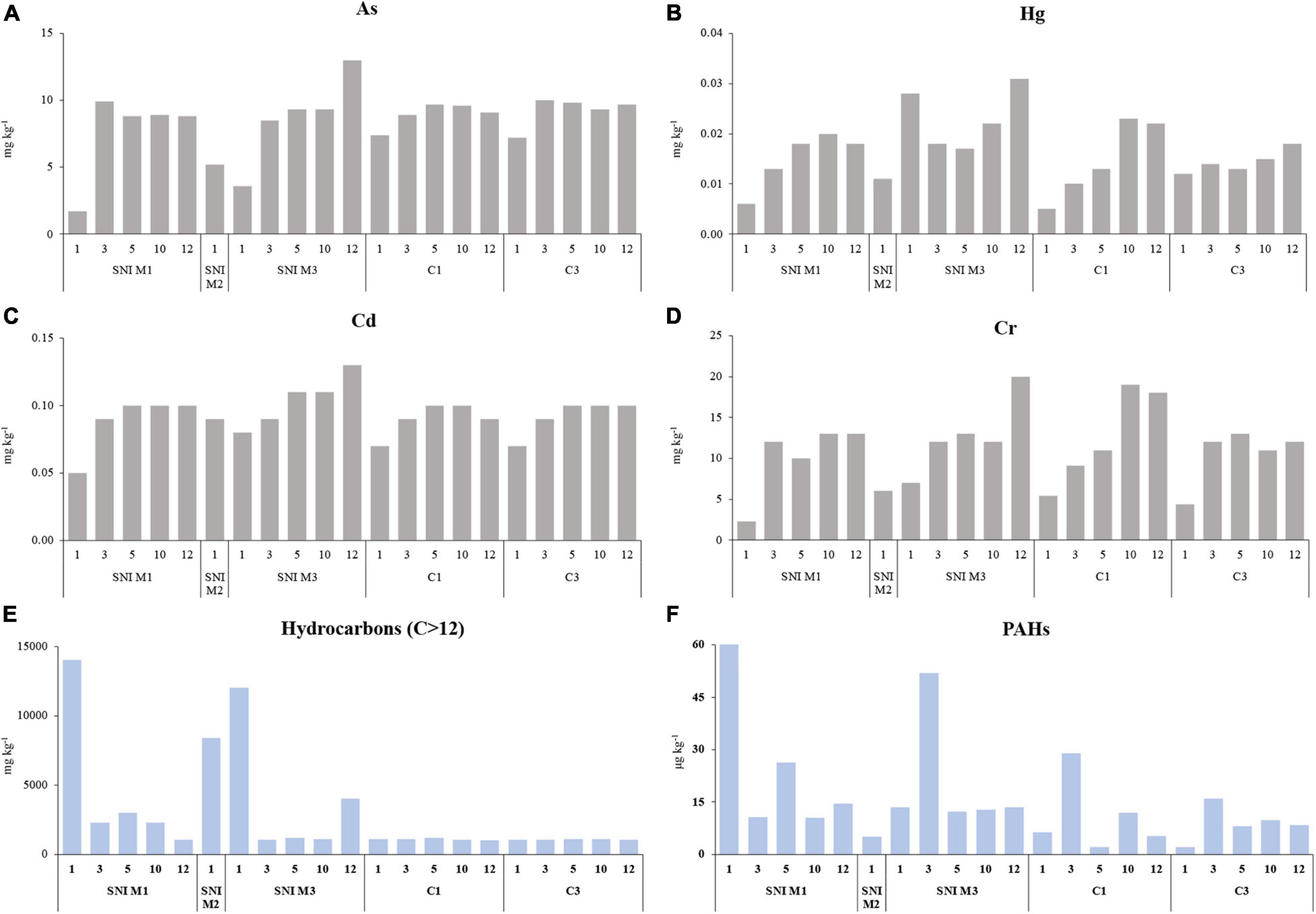
Figure 2. Bathymetric distribution of the contaminant concentrations in the sediments of the SNI (M1, M2, and M3) and control (C1, C2, and C3) transects. (A) Arsenic (As, mg kg–1), (B) Mercury (Hg, mg kg–1), (C) Cadmium (Cd, mg kg–1), (D) Chrome (Cr, mg kg–1), (E) Hydrocarbons C > 12 (mg kg–1), and (F) Polycyclic Aromatic Hydrocarbons (PAHs) concentrations (mg kg–1).
The highest concentrations of aliphatic hydrocarbons C > 12 were observed in some stations of the SNI transects, especially at 1 m depth in M1 transect (14,000 mg kg–1, Figure 2E). In the 10–20 cm sediment layers analyzed, the concentrations of C < 12 and C > 12 hydrocarbons were similar or lower compared to the superficial layers (0–10 cm).
Total PAH concentrations in the sediment ranged from 0.28 to 3757.65 μg kg–1, with the highest value observed at 1 m depth in the M1 transect (Figure 2F). Fluoranthene accounted for 21% of the total PAH concentrations. In all the sediment samples, PAHs concentrations were almost entirely represented by high molecular weight PAHs (PM > 178, representing 40–98%) whereas low molecular weight PAHs (PM < 178) generally contributed to a lower extent (2–60%). In the sub-superficial sediment samples analyzed (10–20 cm), the concentrations of PAHs were 5–9 times higher in M1 and M2 transects compared to the 0–10 cm sediment layer.
PCB congeners concentrations were lower than 0.1 μg kg–1 in both control and SNI transects (Supplementary Table 6), with values much lower than thresholds established by the national (D. M. 152/2006, 173/2016) and international laws (e.g., Canadian Council of Ministers of the Environment 2002).
The m-ERM-q values calculated on the basis of heavy metal and PAH concentrations revealed that all stations of the control and SNI transects were classified as low priority sites, except for the station at 1 m depth of the M1 transect, which was classified as high-priority site, with 76% probability to have toxicological effects.
Concentration of the Biochemical Components and Nutritional Quality of Organic Matter
Chlorophyll-a, phaeopigment, total phytopigment, protein, carbohydrate, lipid, and biopolymeric C (BPC) concentrations, in all sampling stations are reported in Supplementary Table 7.
PERMANOVA analyses showed no significant effect of the factor “Condition,” and a significant effect of the factor “Transect (Condition) × Depth” on Chlorophyll-a, phaeopigment, total phytopigment concentrations (Table 1).
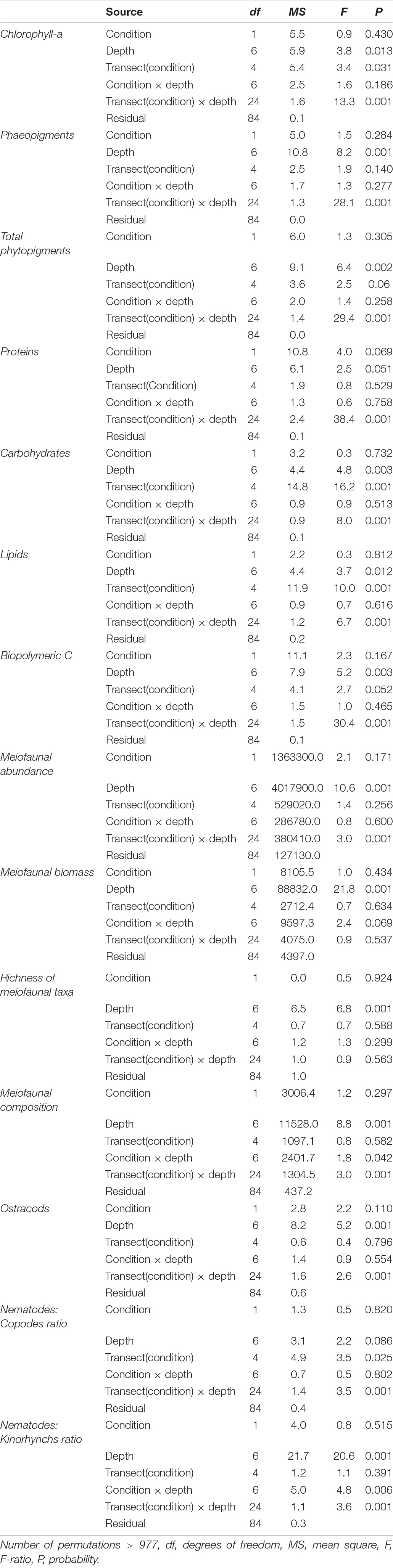
Table 1. Output of PERMANOVA analyses on the concentrations of organic matter biochemical components, meiofaunal abundance, biomass, richness of taxa, and taxonomic composition.
The bathymetric distribution of phytopigment concentrations in all transects is reported in Figure 3A. Overall, average concentrations of phytopigments were higher in the C3 control transect (4.76 ± 0.83μg g–1) than in the other transects where values were very similar (on average 3.31 μg g–1).
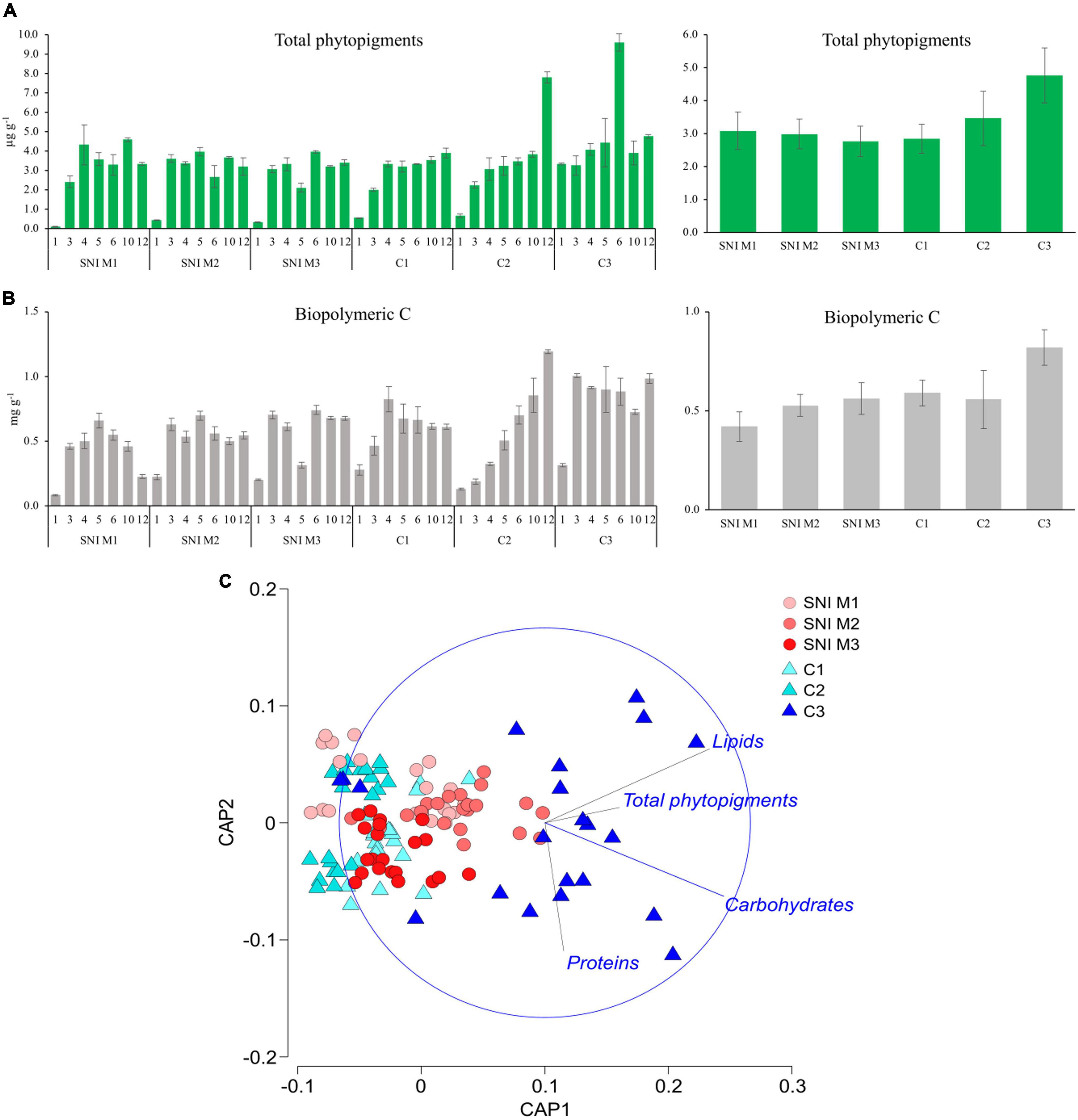
Figure 3. Bathymetric distribution of biochemical components of the sedimentary organic matter in the SNI (M1, M2, and M3) and control (C1, C2, and C3) transects. (A) Phytopigment concentrations in the different stations and transects (on the left) and their average values (on the right), (B) BPC concentrations (on the left) and their average values (on the right), (C) output of canonical analysis of principal coordinates (CAP) based on organic matter biochemical components (protein, carbohydrate, lipid and total phytopigment concentrations).
PERMANOVA analyses showed no significant effect of the factor “Condition,” and a significant effect of the factor “Transect (Condition) × Depth” on concentrations and biochemical composition of sedimentary organic matter (Table 1). Pair-wise tests on concentrations and biochemical composition of organic matter showed significant differences among both control and SNI transects at almost all water depths.
The bathymetric distribution of protein, carbohydrate and lipid concentrations is reported in Supplementary Figure 1. Protein concentrations were on average lower in M1 and M2 transects (0.44 ± 0.09 – 0.52 ± 0.6 mg g–1) than in the other transects (on average 0.80 mg g–1; Supplementary Figure 1A). Carbohydrate and lipid concentrations in the C3 transects were up to > 2 times higher than values found in the other transects (range for carbohydrates: 0.15 ± 0.02–0.40 ± 0.05 mg g–1 in C2 and C3 transects, respectively, range for lipids: 0.13 ± 0.01–0.32 ± 0.05 mg g–1 in M3 and C3 transects, respectively, Supplementary Figures 1B,C).
Along the bathymetric gradient, lower BPC concentrations were observed at shallower stations (1 m depth) compared to the deeper ones (Figure 3B). BPC concentrations ranged from 0.13 ± 0.01 to 1.19 ± 0.02 mgC g–1 in the control stations and from 0.08 ± 0.01 to 0.74 ± 0.03 mgC g−1 in the SNI stations. Overall, the highest average values of BPC were observed in the control transects, especially in C3 (0.82 ± 0.06 mgC g–1) compared to those observed within the SNI area. In both SNI and control transects BPC concentrations were on average 0.6 mgC g−1, while the contribution of autotrophic C to BPC was ca. 23.5%.
CAP analysis on biochemical composition of organic matter along the transects showed that most stations segregated together except for some stations along the C3 transect, driven by increasing concentrations of the different biochemical components of organic matter (Figure 3C).
Abundance, Biomass and Diversity of Meiofauna Assemblages
Overall the PERMANOVA analyses showed no significant effect of the factor “Condition” on meiofaunal abundance, biomass and richness of taxa. A significant effect of the factor “Transect (Condition) × Depth” was observed only on meiofaunal abundance (Table 1). Pair-wise tests showed significant differences among the three control transects and among the three SNI transects at 1 m water depth, and along the bathymetric gradient of each transect.
In particular, average meiofaunal abundances along the SNI transects ranged from 692.7 ± 236.6 to 730.6 ± 161.8 ind. 10 cm–2 (in M1 and M2, respectively) and in the controls from 724.7 ± 135.2 to 1163.7 ± 341.9 ind. 10 cm–2 (in C2 and C1, respectively; Figure 4A). Meiofaunal abundances showed a significant decreasing pattern with increasing water depth from 3 to 12-m depth in all the SNI and control transects, although the lowest meiofauna abundances were observed at 1-m depths (except for the C1 transect; Figure 4A).
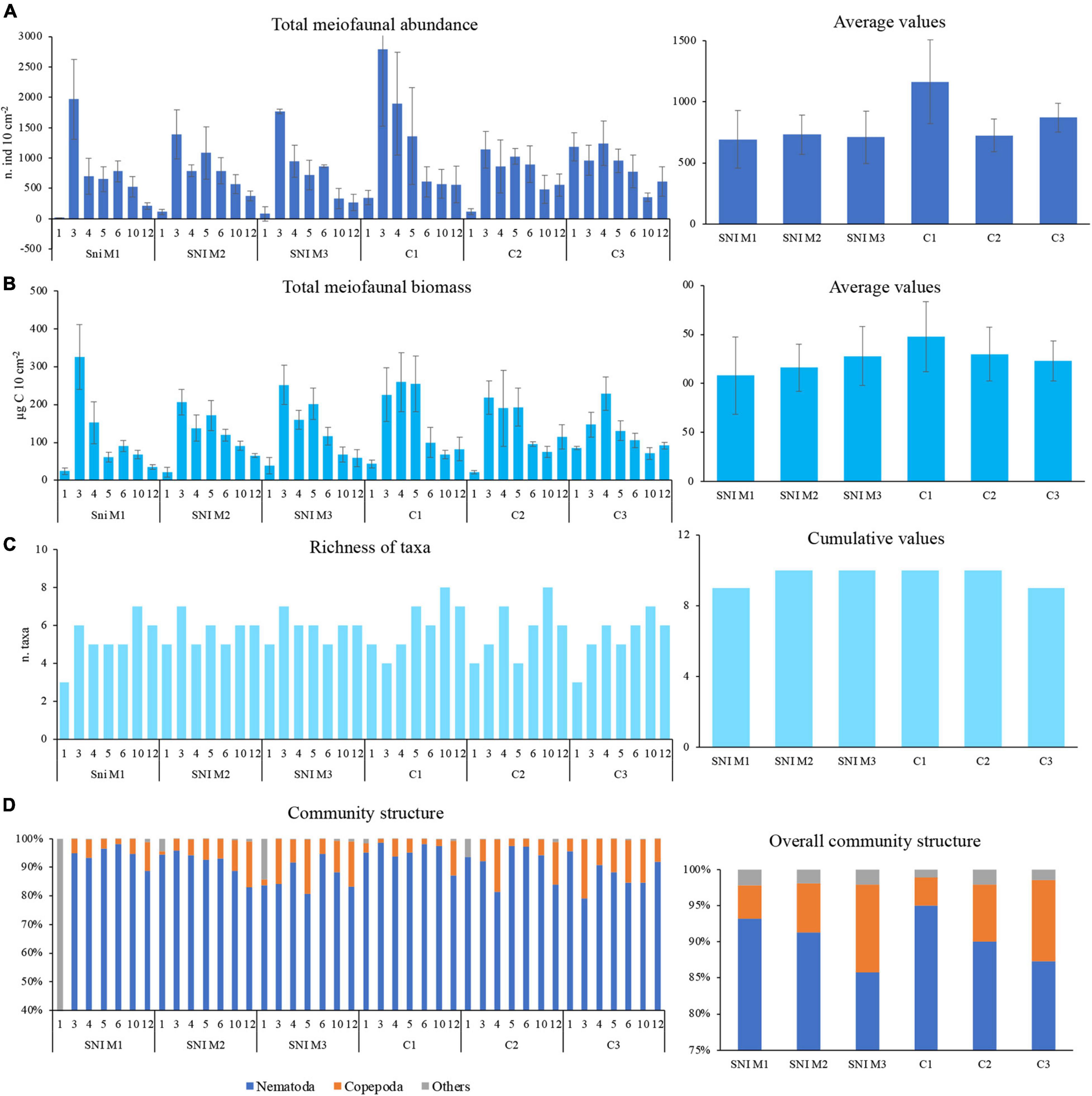
Figure 4. Bathymetric distribution of meiofaunal abundance, biomass and community structure in the sediments of SNI (M1, M2 and M3) and control (C1, C2 and C3) transects. (A) Meiofauna abundances in the different stations (on the left) and transects and their average values (on the right), (B) biomasses in the different stations and transects (on the left) and their average values (on the right); (C) richness of taxa in the different stations and transects (on the left) and their cumulative values (on the right), and (D) community structure in the different stations and transects (on the left) and their overall community structure (on the right). The group “Others” contains Polychaeta, Kinorhyncha, Bivalvia, Acarina, Cumacea, Amphipoda, Gastropoda, Cladocera, Ostracoda, and Tanaidacea.
Significant differences in meiofaunal biomass were found at different depths along each transect, with a general decrease of biomass with increasing water depths (from 3 to 12 m, Figure 4B). The shallowest station (1 m depth) of each transect showed the lowest biomass values. Overall average values of meiofaunal biomasses in the SNI transects were not significantly different from those outside the SNI (ranging from 108.0 ± 39.5 to 147.4 ± 35.7 μgC 10 cm–2 in M1 SNI and C1 control transects, respectively; Figure 4B).
Richness of meiofaunal taxa ranged from 3 to 8 in the control transects and from 3 to 7 in the SNI transects (Figure 4C). The richness of meiofaunal taxa significantly changed along all the transects. More marked differences were observed along the C1 and C2 transects and in the M1 SNI transect (Figure 4C), where stations located at 1-m depth were characterized by the lowest taxa richness (from 3 to 4). The total number of meiofaunal taxa observed both in the SNI and control areas was 9–10 and the presence/absence of meiofaunal taxa in the SNI and control transects did not show a clear pattern.
Meiofaunal assemblages were dominated by nematodes (78–98% of the total abundance, except for SNI M1 at 1 m) at all stations (Figure 4D), followed by harpacticoid copepods (0–20%) and ostracods (0–43%). The contribution of other taxa (mainly polychaetes, kinorhynchs and bivalves) accounted less than 1% each. Temporary meiofaunal taxa (here defined as the juveniles of macrofaunal organisms, sensu Higgins and Thiel, 1988) were represented by polychaetes and bivalves and their average contribution on total meiofauna was < 0.2% in both SNI and control transects. The average abundance of meiofaunal taxa identified in the SNI and control transects is reported in Table 2.
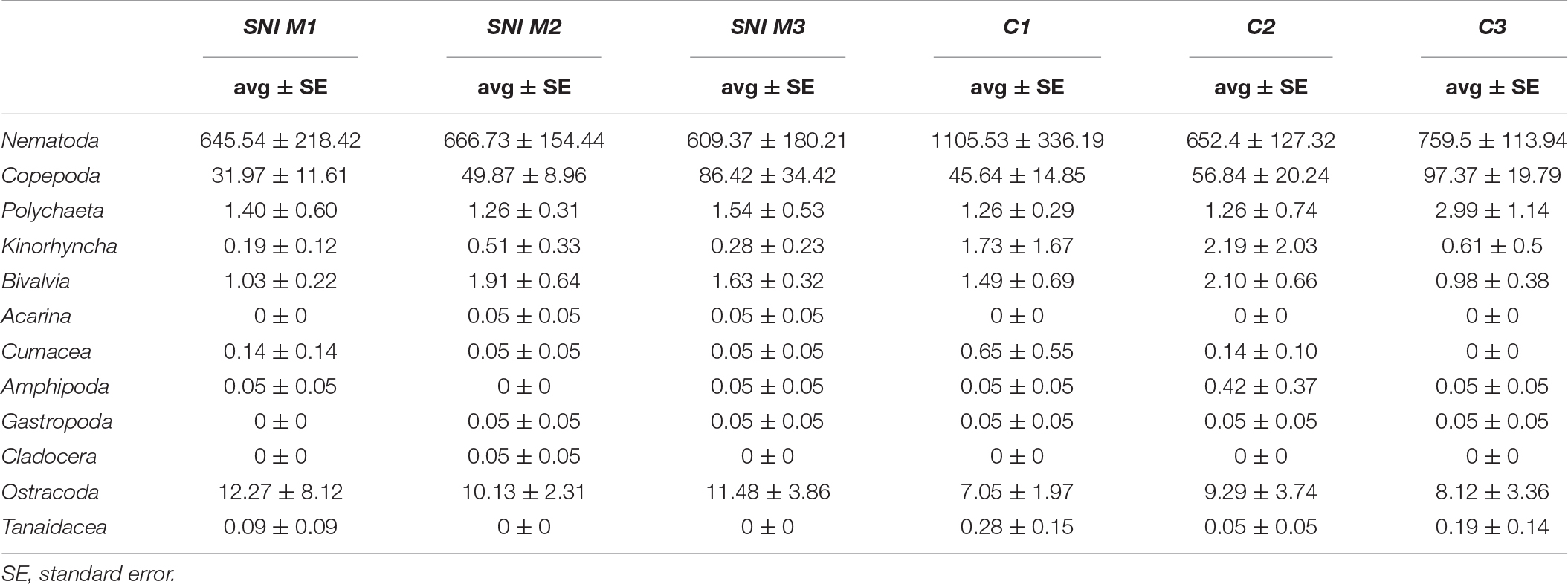
Table 2. List of meiofaunal taxa identified in the SNI and control transects and their average abundance.
PERMANOVA analyses carried out on taxonomic composition of meiofauna revealed a no significant effect of the factor “Condition” and a significant effect of the interactions “Condition × Depth” and “Transect (Condition) × Depth” (Table 1). Significant differences between SNI and Control were found only at 12 m and among transects (at 1 and 3-m depth) of the SNI and control areas (Table 1). A higher contribution of nematodes was found in the control transects compared to the SNI transects (91–95%, and 45–94%, respectively).
Significant differences were also found along the bathymetric gradient in each transect (Table 1). A higher contribution of ostracods was found in the stations located at 1-m depth compared to the deepest stations (Supplementary Figure 3). Although along the bathymetric gradients of the transects significant differences in the number of individuals belonging to ostracods were observed (except for the SNI M2), the differences between the SNI and the control transects were not significant (Table 1).
SIMPER analyses showed an average dissimilarity among the compositions of meiofaunal assemblages in the stations of the control or SNI transects ranging from 10.3 to 44.3% (Supplementary Table 8), with the highest dissimilarity observed at 10-m depth in both cases. Similarly, when we compared the control and SNI transects we found an average dissimilarity among meiofaunal assemblage compositions ranging from 14.6 to 42.4% (at 3 and 10-m depth, respectively).
Overall, nematodes, copepods, ostracods, bivalves, polychaetes and kinorhynchs were the taxa responsible for the observed dissimilarity.
The nematode to copepod abundance ratio ranged from 3.83 to 107.15 in the control transects and from 4.25 to 80.25 in the SNI transects (Supplementary Figure 2). Significant differences were found along the bathymetric gradients, especially in the control transects and among different SNI transects (Table 1). However, differences between SNI and control transects were not observed.
The nematode to kinorhynch abundance ratio ranged from 30.84 to 2745.96 in the control transects (with some significant differences at 1, 3, and 12 m) and from 6.86 to 1806.14 in the SNI transects (Supplementary Figure 2). No significant differences were detected between SNI and control transects (Table 1).
Drivers of the Distribution of Meiofauna Composition
DistLM forward analysis showed that the variance of meiofaunal assemblage composition in the investigated sediments was significantly explained for 75.2% by C > 12 hydrocarbons (49.7%), water depth (12.4%),% of sand (7.1%) and total phytopigments (6.0%) (Supplementary Table 9). When the most contaminated station (SNI M1 transect at 1 m depth) was excluded from the analyses C > 12 aliphatic hydrocarbons remained the variable that mostly influenced meiofaunal assemblage composition (36%) followed by depth (18.3%) and total phytopigment concentrations (9.3%).
Discussion
Industrial activities, even if interrupted for several years, have often left chronic marine pollution as a legacy (Romano et al., 2004; Gambi et al., 2020a; Naidu et al., 2021). This was also the expectation of the marine area facing the chronically contaminated industrial area of Falconara M.ma (central-western Adriatic Sea) enclosed since 2002 among the most polluted Italian sites and among the “problem areas” of the Europe’s seas (Andersen et al., 2019; Ausili et al., 2020). However, the present investigation, revealed that heavy metal concentrations in all the investigated sediments were typically low or at values lower than those expected to cause detrimental biological effects (above Effects-Range-Median; Long et al., 1995). We only found a single exception for the metalloid As, whose higher levels could be due to the release of the former Montedison industry, which produced fertilizers. In terms of organic contaminants, although the concentrations of C > 12 aliphatic hydrocarbons and PAHs were higher in a few stations of the SNI area compared to the control ones, these were orders of magnitudes lower than values found in other chronically contaminated marine areas (Romano et al., 2004, 2008; Morroni et al., 2020; Tangherlini et al., 2020) with values below ERL thresholds of moderately polluted coastal areas of other European basins (Baumard et al., 1998; Bihari et al., 2007; Berto et al., 2009). Both for heavy metals and PAHs, the m-ERM-q values corresponded to low environmental risk levels, with the only exception provided by a station of the SNI area (located very close to a stream outfall potentially releasing contaminants deriving from the abandoned chemical plant), which was classified as at high risk.
To explore the possibility that contaminants have been buried in sub-superficial sediment layers, we also analyzed organic and inorganic contaminants in a subset of samples and observed that only two of them, showed an increase of the concentrations below 10 cm sediment depth. Unfortunately, literature data about the contamination levels in the same area of the SNI are not available or accessible. However, we hypothesize that when the SNI was established, approximately 2 decades ago, the contamination was likely present only in surface sediments, to be then diluted or removed by sediment resuspension or buried over time. The decrease in the contamination levels might be due to the specific environmental characteristics of the area (e.g., hydro-dynamic regime due to the Western Adriatic Current that flows southward; Wang and Pinardi, 2002) or at the high levels of bioturbation (Thibodeaux and Bierman, 2003) and microbial degradation activity (Acosta-González and Marqués, 2016; Dell’Anno et al., 2021) that might have promoted the biological attenuation of the contaminants (Röling and Van Verseveld, 2002).
We also found that the sediments within the SNI were characterized by biopolymeric C concentrations (used as a proxy of trophic state; Pusceddu et al., 2009, 2011) even lower than outside. In particular, the southern control transect was characterized by the highest organic load, and higher carbohydrate and lipid concentrations, which generally indicate a secondary origin of the organic matter (Pusceddu et al., 2009). This can be attributed to the organic enrichment caused by the nearby Esino river outflow (Capotondi et al., 2015; Bianchelli et al., 2018).
Based on the results of the biopolymeric C concentration and its autotrophic contribution, all the investigated sediments, without distinction between SNI and control transects, could be classified as “meso-eutrophic” (Pusceddu et al., 2009), consistently with previous observations in other coastal areas of the Central Adriatic Sea (Bianchelli et al., 2016, 2018).
To better assess the health status of the marine ecosystem in the investigated area, we assessed the responses of meiofauna assemblages. On average, meiofaunal abundance, biomass and richness of taxa in the SNI transects were similar to the controls, despite the high variability among stations at different water depths along each transect. In particular, the stations at 1-m depth, characterized by a coarser sedimentary granulometry, showed different patterns of meiofauna compared to the other stations, but such patterns were independent of the distance from the abandoned industrial plant. The analysis of meiofaunal richness revealed low values (from 3 to 8 taxa per transect), suggesting an overall “poor/moderate environmental quality” according to the environmental status classification based on this descriptor (Danovaro et al., 2004). A similar number or even lower of meiofaunal taxa was reported in extremely impacted sediments from other SNIs (Gambi et al., 2020a) and affected by historical mining (Gambi et al., 2020b). However, since the low richness of higher taxa was here observed also in the control transects and, previously, in other areas of the Adriatic coast (Bianchelli et al., 2016; Semprucci et al., 2016; Semprucci et al., 2017), we argue that should not be directly linked to the chemical impact deriving from the past industrial activity of the Montedison plant or from other nearby sources (i.e., the river and the oil refinery) present within the entire SNI.
Further confirmation of the lack of a negative “SNI effect” on meiofaunal assemblages was obtained by the analysis of the composition of meiofaunal assemblages, which varied among stations and depths, but without significant differences between SNI and control sediments.
These findings were consistent with those obtained by the analysis of the biological indices based on the abundance ratios between nematodes and copepods and between nematodes and kinorhynchs. Copepodes and kinorhynchs have been reported to be affected by organic enrichment/contamination, thus revealing their greater sensitivity compared to other meiofaunal taxa (Mirto et al., 2012; Baguley et al., 2015; Dal Zotto et al., 2016) like nematodes, which seem to be more tolerant to such anthropogenic impacts (Semprucci and Balsamo, 2012).
However, these biological indices did not change between SNI and control transects, and in particular for the nematode to kinorhynch ratio, a decrease was found moving away from the putative source of impact, thus confirming the lack of any negative effects due to the former Montedison plant.
Although no evident criticalities were observed in the SNI transects in terms of contaminations and trophic state when compared to the non-SNI areas, the results of the multivariate analysis indicated that the variance of the meiofaunal assemblage composition was mostly influenced by the concentrations of C > 12 aliphatic hydrocarbons, followed by depth, grain size, and trophic resources. The origin of these low concentrations of aliphatic hydrocarbons remains unclear and could be attributed either to different anthropogenic sources (including activities previously conducted by the former Montedison plant) or to biogenic compounds of terrestrial and marine origin (Bajt, 2012).
Overall, these findings suggest the high sensitivity of meiofaunal assemblages to hydrocarbon contamination, which however, being slight, did not affect the overall health status of these biological components more than outside the SNI.
Conclusion
The results of this study reveal that the levels of organic and inorganic pollutants in the investigated sediments of the Falconara M.ma SNI are generally lower than those expected to be harmful for marine life. The health status of meiofaunal assemblages in terms of abundance, biomass and diversity do not show any sign of biological impact and thus the current classification of the marine area as a chronically/highly polluted site appears unjustified. Due to the high resilience of meiofaunal assemblages, however, we cannot exclude that such a finding is the result of the specific biological component considered, nor that other faunal components (e.g., macrofauna) may be affected by the historical contaminations of the chemical plant. At the same time, the results reported here, allow us to hypothesize possible self-purification and recovery processes also associated with the interdiction to human activities. If the hypothesis of the natural recovery of the investigated area of the SNI of Falconara M.ma were confirmed by other studies, the plans for the identification and monitoring of the most polluted areas in Italy should necessarily be redefined also according to the Water Framework, the Marine Strategy Framework and the Environmental Quality Standard Directives.
Data Availability Statement
The original contributions presented in the study are included in the article/Supplementary Material, further inquiries can be directed to the corresponding author.
Author Contributions
CC conceived the study. AD’A, CC, LM, and IB defined sampling strategy. ML and GL participated in the sampling activity. SB, EF, CG, and ML supervised laboratory activities. CC, SV, SC, ER, AD’A, and SB contributed to data elaboration and interpretation. NS contributed to data visualization. CC, SB, and NS drafted the first version of the manuscript. All authors contributed to manuscript preparation and to the final version.
Funding
This study has been supported by the project BIOBLUTECH “Blue Biotechnologies for restoring marine ecosystems of the contaminated Site of National Interest (SIN) ex Montedison (Falconara M.ma), ID 9204 supported by Cariverona Foundation.
Conflict of Interest
The authors declare that the research was conducted in the absence of any commercial or financial relationships that could be construed as a potential conflict of interest.
Publisher’s Note
All claims expressed in this article are solely those of the authors and do not necessarily represent those of their affiliated organizations, or those of the publisher, the editors and the reviewers. Any product that may be evaluated in this article, or claim that may be made by its manufacturer, is not guaranteed or endorsed by the publisher.
Acknowledgments
We thank Dr. Claudia Ciotti for her support in providing information about the Site of National Interest of Falconara M.ma, and Dr. Martina Meola for her participation in the sampling and laboratory activities.
Supplementary Material
The Supplementary Material for this article can be found online at: https://www.frontiersin.org/articles/10.3389/fmars.2021.813887/full#supplementary-material
References
Acosta-González, A., and Marqués, S. (2016). Bacterial diversity in oil-polluted marine coastal sediments. Curr. Opin. Biotechnol. 38, 24–32. doi: 10.1016/J.COPBIO.2015.12.010
Andersen, J. H., Harvey, T., Murray, C., Green, N., and Reker, J. (2019). Contaminants in Europe’s Seas. Moving Towards a Clean, Non Toxic Marine Environment. Copenhagen: European Environment Agency.
Anderson, M. J. (2001). Permutation tests for univariate or multivariate analysis of variance and regression. Can. J. Fish. Aquat. Sci. 58, 626–639. doi: 10.1139/cjfas-58-3-626
Anderson, M. J., Gorley, R. N., and Clarke, K. R. (2008). PERMANOVA+ for PRIMER: Guide to Software and Statistical Methods. Plymouth: PRIMER-E.
Anderson, M. J., and Willis, T. J. (2003). Canonical analysis of principal coordinates: a useful method of constrained ordination for ecology. Ecology 84, 511–525. doi: 10.1890/0012-9658(2003)084[0511:caopca]2.0.co;2
Andrassy, I. (1956). Die rauminhalts-und gewichtsbestimmung der fadenwürmer (Nematoden). Acta Zool. Hungarica 2, 1–5.
Ausili, A., Bergamin, L., and Romano, E. (2020). Environmental status of Italian coastal marine areas affected by long history of contamination. Front. Environ. Sci. 8:34. doi: 10.3389/FENVS.2020.00034
Baguley, J. G., Montagna, P. A., Cooksey, C., Hyland, J. L., Bang, H. W., Morrison, C., et al. (2015). Community response of deep-sea soft-sediment metazoan meiofauna to the deepwater horizon blowout and oil spill. Mar. Ecol. Progr. Ser. 528, 127–140. doi: 10.3354/meps11290
Bajt, O. (2012). Aliphatic and polycyclic aromatic hydrocarbons in sediments of the Slovenian coastal area (Gulf of Trieste, northern Adriatic). Environ. Monit. Assess. 184, 7439–7452. doi: 10.1007/s10661-011-2511-y
Balsamo, M., Albertelli, G., Ceccherelli, V. U., Coccioni, R., Colangelo, M. A., Curini-Galletti, M., et al. (2010). Meiofauna of the Adriatic Sea: present knowledge and future perspectives. Chem. Ecol. 26, 45–63. doi: 10.1080/02757541003705492
Balzamo, S., Barbizzi, S., Bellaria, V., Belli, M., Calabretta, E., de Zorzi, P., et al. (2012). Validation study of the measurement process of the hydrocarbons C> 12 (with a number of carbon atoms greater than twelve) in contaminated soils. J. Occup. Environ. Hyg. 3, 85–132.
Barbier, E. B. (2012). Progress and challenges in valuing coastal and marine ecosystem services. Rev. Environ. Econ. Policy 6, 1–19. doi: 10.1093/reep/rer017
Barbier, E. B. (2017). Marine ecosystem services. Curr. Biol. 27, R507–R510. doi: 10.1016/j.cub.2017.03.020
Baumard, P., Budzinski, H., and Garrigues, P. (1998). Polycyclic aromatic hydrocarbons in sediments and mussels of the western Mediterranean sea. Environ. Toxicol. Chem. 17, 765–776. doi: 10.1016/j.jhazmat.2009.04.084
Berto, D., Cacciatore, F., Ausili, A., Sunseri, G., Bellucci, L. G., Frignani, M., et al. (2009). Polycyclic aromatic hydrocarbons (PAHs) from diffuse sources in coastal sediments of a not industrialised Mediterranean Island. Water Air Soil Pollut. 200, 199–209. doi: 10.1007/s11270-008-9904-8
Bertocci, I., Dell’Anno, A., Musco, L., Gambi, C., Saggiomo, V., Cannavacciuolo, M., et al. (2019). Multiple human pressures in coastal habitats: variation of meiofaunal assemblages associated with sewage discharge in a post-industrial area. Sci. Total Environ. 655, 1218–1231. doi: 10.1016/j.scitotenv.2018.11.121
Bianchelli, S., Buschi, E., Danovaro, R., and Pusceddu, A. (2018). Nematode biodiversity and benthic trophic state are simple tools for the assessment of the environmental quality in coastal marine ecosystems. Ecol. Indic. 95, 270–287. doi: 10.1016/j.ecolind.2018.07.032
Bianchelli, S., Pusceddu, A., Buschi, E., and Danovaro, R. (2016). Trophic status and meiofauna biodiversity in the Northern Adriatic Sea: insights for the assessment of good environmental status. Mar. Environ. Res. 113, 18–30. doi: 10.1016/j.marenvres.2015.10.010
Bihari, N., Fafandel, M., and Piškur, V. (2007). Polycyclic aromatic hydrocarbons and ecotoxicological characterization of seawater, sediment, and mussel Mytilus galloprovincialis from the Gulf of Rijeka, the Adriatic Sea, Croatia. Arch. Environ. Contam. Toxicol. 52, 379–387. doi: 10.1007/s00244-005-0259-5
Capotondi, L., Bergami, C., Orsini, G., Ravaioli, M., Colantoni, P., and Galeotti, S. (2015). Benthic foraminifera for environmental monitoring: a case study in the central Adriatic continental shelf. Environ. Sci. Pollut. Res. 22, 6034–6049. doi: 10.1007/s11356-014-3778-7
Civili, F. S. (2010). The Land-Based Pollution of the Mediterranean Sea: Present State and Prospects. New Delhi: IEMed.
Corinaldesi, C., Canensi, S., Carugati, L., Martire, M. L., Marcellini, F., Nepote, E., et al. (2022). Organic enrichment can increase the impact of microplastics on meiofaunal assemblages in tropical beach systems. Environ. Pollut. 292(Pt B):118415. doi: 10.1016/j.envpol.2021.118415
Costanza, R., d’Arge, R., de Groot, R., Farber, S., Grasso, M., Hannon, B., et al. (1997). The value of the world’s ecosystem services and natural capital. Nature 387, 253–260.
Croudace, I. W., Romano, E., Ausili, A., Bergamin, L., and Rothwell, R. G. (2015). X-Ray Core Scanners as an Environmental Forensics Tool: A Case Study of Polluted Harbour Sediment (Augusta Bay, Sicily). Dordrecht: Springer, 393–421.
Dal Zotto, M., Santulli, A., Simonini, R., and Todaro, M. A. (2016). Organic enrichment effects on a marine meiofauna community, with focus on Kinorhyncha. Zool. Anzeig. A J. Comp. Zool. 265, 127–140. doi: 10.1016/J.JCZ.2016.03.013
Danovaro, R. (2010). Methods for the Study of Deep-Sea Sediments, Their Functioning and Biodiversity. Boca Raton, FL: CRC Press.
Danovaro, R., Fabiano, M., and Vincx, M. (1995). Meiofauna response to the Agip Abruzzo oil spill in subtidal sediments of the Ligurian Sea. Mar. Pollut. Bull. 30, 133–145. doi: 10.1016/0025-326x(94)00114-o
Danovaro, R., Gambi, C., Höss, S., Mirto, S., Traunspurger, W., and Zullini, A. (2009). “Case studies using nematode assemblage analysis in aquatic habitats,” in Nematodes as Enviornmental Indicators, eds M. J. Wilson and T. Kakouli-Duarte (Wallingford: CABI Publication), 146–171. doi: 10.1079/9781845933852.0146
Danovaro, R., Gambi, C., Mirto, S., Sandulli, R., and Ceccherelli, V. U. (2004). MeiofaunaMediterranean benthos: a manual of methods for its sampling and study. Biol. Mar. Medit 11:55 e97.
Dell’Anno, A., Mei, M. L., Pusceddu, A., and Danovaro, R. (2002). Assessing the trophic state and eutrophication of coastal marine systems: a new approach based on the biochemical composition of sediment organic matter. Mar. Pollut. Bull. 44, 611–622. doi: 10.1016/s0025-326x(01)00302-2
Dell’Anno, F., Rastelli, E., Tangherlini, M., Corinaldesi, C., Sansone, C., Brunet, C., et al. (2021). Highly contaminated marine sediments can host rare bacterial taxa potentially useful for bioremediation. Front. Microbiol. 12:584850. doi: 10.3389/fmicb.2021.584850
Feller, R. J., and Warwick, R. M. (1988). Energetics. Washington, DC: Smithsonian Institution Press.
Fenchel, T. O. M., and Finlay, B. J. (2004). The ubiquity of small species: patterns of local and global diversity. Bioscience 54, 777–784. doi: 10.1073/pnas.1012678108
Gambi, C., Canals, M., Corinaldesi, C., Dell’Anno, A., Manea, E., Pusceddu, A., et al. (2020b). Impact of historical sulfide mine tailings discharge on meiofaunal assemblages (Portmán Bay, Mediterranean Sea). Sci. Total. Environ. 736:139641. doi: 10.1016/j.scitotenv.2020.139641
Gambi, C., Dell’Anno, A., Corinaldesi, C., Lo Martire, M., Musco, L., Da Ros, Z., et al. (2020a). Impact of historical contamination on meiofaunal assemblages: the case study of the Bagnoli-Coroglio Bay (southern Tyrrhenian Sea). Mar. Environ. Res. 156:104907. doi: 10.1016/j.marenvres.2020.104907
Gray, J. S. (2000). The measurement of marine species diversity, with an application to the benthic fauna of the Norwegian continental shelf. J. Exp. Mar. Bio. Ecol. 250, 23–49. doi: 10.1016/s0022-0981(00)00178-7
Gyedu-Ababio, T. K., and Baird, D. (2006). Response of meiofauna and nematode communities to increased levels of contaminants in a laboratory microcosm experiment. Ecotoxicol. Environ. Saf. 63, 443–450. doi: 10.1016/j.ecoenv.2005.01.010
Hay Mele, B., Russo, L., Crocetta, F., Gambi, C., Dell’Anno, A., Danovaro, R., et al. (2020). Ecological assessment of anthropogenic impact in marine ecosystems: the case of Bagnoli Bay. Mar. Environ. Res. 158, 104953. doi: 10.1016/j.marenvres.2020.104953
Heip, C., Vincx, M., and Vranken, G. (1985). The ecology of marine nematodes. Oceanogr. Mar. Biol. 23, 399–489.
Higgins, R. P., and Thiel, H. (1988). Introduction to the Study of Meiofauna. Washington, DC: Smithsonian Institution Press.
Kandratavicius, N., de Ward, C. P., Venturini, N., Giménez, L., Rodriguez, M., and Muniz, P. (2018). Response of estuarine free-living nematode assemblages to organic enrichment: an experimental approach. Mar. Ecol. Progr. Ser. 602, 117–133. doi: 10.3354/meps12699
Kang, T., Min, W. G., Rho, H. S., Park, H. S., and Kim, D. (2014). Differential responses of a benthic meiofaunal community to an artificial oil spill in the intertidal zone. J. Mar. Biol. Ass. U. K. 94, 219–231. doi: 10.1017/s0025315413001501
Kowalska, J. B., Mazurek, R., Gąsiorek, M., and Zaleski, T. (2018). Pollution indices as useful tools for the comprehensive evaluation of the degree of soil contamination–a review. Environ. Geochem. Health 40, 2395–2420. doi: 10.1007/s10653-018-0106-z
Long, E. R., MacDonald, D. D., Severn, C. G., and Hong, C. B. (2000). Classifying probabilities of acute toxicity in marine sediments with empirically derived sediment quality guidelines. Environ. Toxicol. Chem. 19, 2598–2601. doi: 10.1002/etc.5620191028
Long, E. R., Macdonald, D. D., Smith, S. L., and Calder, F. D. (1995). Incidence of adverse biological effects within ranges of chemical concentrations in marine and estuarine sediments. Environ. Manag. 19, 81–97. doi: 10.1007/bf02472006
Lorenzen, C. J., and Jeffrey, S. W. (1980). Determination of chlorophyll in seawater. Unesco Tech. Pap. Mar. Sci 35, 1–20.
Martuzzi, M., Mitis, F., Biggeri, A., Terracini, B., and Bertollini, R. (2002). Environment and health status of the population in areas with high risk of environmental crisis in Italy. Epidemiol. Prev. 26(6 Suppl), sul1–53.
McArdle, B. H., and Anderson, M. J. (2001). Fitting multivariate models to community data: a comment on distance-based redundancy analysis. Ecology 82:290. doi: 10.1890/0012-9658(2001)082[0290:fmmtcd]2.0.co;2
Micheli, F., Halpern, B. S., Walbridge, S., Ciriaco, S., Ferretti, F., Fraschetti, S., et al. (2013). Cumulative human impacts on mediterranean and black sea marine ecosystems: assessing current pressures and opportunities. PLoS One 8:e79889. doi: 10.1371/journal.pone.0079889
Mirto, S., Gristina, M., Sinopoli, M., Maricchiolo, G., Genovese, L., Vizzini, S., et al. (2012). Meiofauna as an indicator for assessing the impact of fish farming at an exposed marine site. Ecol. Ind. 18, 468–476. doi: 10.1016/j.ecolind.2011.12.015
Moens, T., Braeckman, U., Derycke, S., Fonseca, G., Gallucci, F., Gingold, R., et al. (2013). “Ecology of free-living marine nematodes,” in Handbook of Zoology, ed. A. Schmidt-Rhaesa (Berlin: De Gruyter), 109–152. doi: 10.1371/journal.pone.0186140
Moreno, M., Vezzulli, L., Marin, V., Laconi, P., Albertelli, G., and Fabiano, M. (2008). The use of meiofauna diversity as an indicator of pollution in harbours. ICES J. Mar. Sci. 65, 1428–1435. doi: 10.1093/icesjms/fsn116
Morroni, L., d’Errico, G., Sacchi, M., Molisso, F., Armiento, G., Chiavarini, S., et al. (2020). Integrated characterization and risk management of marine sediments: the case study of the industrialized Bagnoli area (Naples, Italy). Mar Environ. Res. 160:104984. doi: 10.1016/j.marenvres.2020.104984
Naidu, R., Biswas, B., Willett, I. R., Cribb, J., Singh, B. K., Nathanail, C. P., et al. (2021). Chemical pollution: a growing peril and potential catastrophic risk to humanity. Environ. Int. 156:106616. doi: 10.1016/j.envint.2021.106616
Pusceddu, A., Bianchelli, S., Gambi, C., and Danovaro, R. (2011). Assessment of benthic trophic status of marine coastal ecosystems: significance of meiofaunal rare taxa. Estuar. Coast. Shelf. Sci. 93, 420–430. doi: 10.1016/j.ecss.2011.05.012
Pusceddu, A., Dell’Anno, A., and Fabiano, M. (2000). Organic matter composition in coastal sediments at Terra Nova Bay (Ross Sea) during summer 1995. Polar Biol. 23, 288–293. doi: 10.1007/s003000050446
Pusceddu, A., Dell’Anno, A., Fabiano, M., and Danovaro, R. (2009). Quantity and bioavailability of sediment organic matter as signatures of benthic trophic status. Mar. Ecol. Prog. Ser. 375, 41–52. doi: 10.3354/meps07735
Raffaelli, D. G., and Mason, C. F. (1981). Pollution monitoring with meiofauna, using the ratio of nematodes to copepods. Mar. Pollut. Bull. 12, 158–163. doi: 10.1016/0025-326x(81)90227-7
Regione Marche (2009). Regione Marche. Available online at: http://www.ambiente.regione.marche.it/Portals/0/Ambiente/Sitiinquinati/2009pianobonifiche_relazionegenerale.pdf (accessed March 29, 2018).
Röling, W. F. M., and Van Verseveld, H. W. (2002). Natural attenuation: what does the subsurface have in store? Biodegradation 13, 53–64. doi: 10.1023/a:1016310519957
Romano, E., Ausili, A., Zharova, N., Celia Magno, M., Pavoni, B., and Gabellini, M. (2004). Marine sediment contamination of an industrial site at Port of Bagnoli, Gulf of Naples, Southern Italy. Mar. Pollut. Bull. 49, 487–495. doi: 10.1016/j.marpolbul.2004.03.014
Romano, E., Bergamin, L., Finoia, M. G., Carboni, M. G., Ausili, A., and Gabellini, M. (2008). Industrial pollution at Bagnoli (Naples, Italy): benthic foraminifera as a tool in integrated programs of environmental characterisation. Mar. Pollut. Bull. 56, 439–457. doi: 10.1016/j.marpolbul.2007.11.003
Semprucci, F., and Balsamo, M. (2012). Free-living marine nematodes as bioindicators: past, present and future perspectives. Environ. Res. J. 6, 17–35.
Semprucci, F., Balsamo, M., and Sandulli, R. (2016). Assessment of the ecological quality (EcoQ) of the Venice lagoon using the structure and biodiversity of the meiofaunal assemblages. Ecol. Ind. 67, 451–457. doi: 10.1016/j.ecolind.2016.03.014
Semprucci, F., Sbrocca, C., Baldelli, G., Tramontana, M., and Balsamo, M. (2017). Is meiofauna a good bioindicator of artificial reef impact? Mar. Biodiv. 47, 511–520. doi: 10.1007/s12526-016-0484-3
Snelgrove, P. V. R., Henry Blackburn, T., Hutchings, P. A., Alongi, D. M., Frederick Grassle, J., Hummel, H., et al. (1997). The importance of marine sediment biodiversity in ecosystem processes. Ambio 26, 578–583.
Tangherlini, M., Corinaldesi, C., Rastelli, E., Musco, L., Armiento, G., Danovaro, R., et al. (2020). Chemical contamination can promote turnover diversity of benthic prokaryotic assemblages: the case study of the Bagnoli-Coroglio bay (southern Tyrrhenian Sea). Mar. Environ. Res. 160:105040. doi: 10.1016/j.marenvres.2020.105040
Thibodeaux, L. J., and Bierman, V. J. (2003). Peer reviewed: the bioturbation-driven chemical release process. Environ. Sci. Technol. 37, 252A–258A. doi: 10.1021/es032518j
Todd, P. A., Heery, E. C., Loke, L. H. L., Thurstan, R. H., Kotze, D. J., and Swan, C. (2019). Towards an urban marine ecology: characterizing the drivers, patterns and processes of marine ecosystems in coastal cities. Oikos 128, 1215–1242. doi: 10.1111/oik.05946
Turner, R. K., and Schaafsma, M. (2015). Coastal zones ecosystem services. Valuat. Ecosyst. Serv. 9, 59–75. doi: 10.1007/978-3-319-17214-9_4
Wang, X. H., and Pinardi, N. (2002). Modeling the dynamics of sediment transport and resuspension in the northern Adriatic Sea. J. Geophys. Res. Ocean 107:3225.
Wieser, W. (1960). Benthic studies in Buzzards Bay: II. The Meiofauna 1. Limnol. Oceanogr. 5, 121–137.
Keywords: marine pollution, trophic state, heavy metals, organic contaminants, meiofauna, highly contaminated marine areas
Citation: Corinaldesi C, Bianchelli S, Rastelli E, Varrella S, Canensi S, Gambi C, Lo Martire M, Musco L, Bertocci I, Fanelli E, Lucia G, Simoncini N and Dell’Anno A (2022) The Paradox of an Unpolluted Coastal Site Facing a Chronically Contaminated Industrial Area. Front. Mar. Sci. 8:813887. doi: 10.3389/fmars.2021.813887
Received: 12 November 2021; Accepted: 28 December 2021;
Published: 24 January 2022.
Edited by:
Paolo Magni, National Research Council (CNR), ItalyReviewed by:
Federica Semprucci, University of Urbino Carlo Bo, ItalyPablo Muniz, Universidad de la República, Uruguay
Maria Flavia Gravina, University of Rome “Tor Vergata”, Italy
Copyright © 2022 Corinaldesi, Bianchelli, Rastelli, Varrella, Canensi, Gambi, Lo Martire, Musco, Bertocci, Fanelli, Lucia, Simoncini and Dell’Anno. This is an open-access article distributed under the terms of the Creative Commons Attribution License (CC BY). The use, distribution or reproduction in other forums is permitted, provided the original author(s) and the copyright owner(s) are credited and that the original publication in this journal is cited, in accordance with accepted academic practice. No use, distribution or reproduction is permitted which does not comply with these terms.
*Correspondence: Cinzia Corinaldesi, Yy5jb3JpbmFsZGVzaUBzdGFmZi51bml2cG0uaXQ=
†These authors have contributed equally to this work