- 1Fisheries and Oceans Canada, Maurice Lamontagne Institute, Mont-Joli, QC, Canada
- 2Fisheries and Oceans Canada, Arctic and Aquatic Research Division, Winnipeg, MB, Canada
- 3Fisheries and Oceans Canada, Northwest Atlantic Fisheries Centre, St. John’s, NL, Canada
Ships and boats may transport whole communities of non-indigenous species (NIS) through hull biofouling, some members of which may become invasive. Several studies have evaluated the diversity of these communities, but very few have analyzed the survival of organisms after their voyages into different and potentially inhospitable conditions. This factor is important to consider because the last port of call approach for risk assessments assumes that if the conditions observed in the last port of call are different from those observed in a receiving port, risks are diminished or null. Using an innovative experimental system, we tested the survival and recovery of the marine blue mussel (Mytilus edulis) and the freshwater zebra mussel (Dreissena polymorpha) by exposing them to adverse salinity conditions at varying temperatures to simulate ships and boats transiting to ports or marinas with contrasting environmental conditions. Both mussel species, which are well-known for their adaptability to new environments as aquatic NIS, survived better at colder temperatures, with blue mussels surviving up to 14 days in freshwater, and zebra mussels up to 8 days in marine water. This highlights the importance of considering the resistance of fouling organisms to adverse conditions in vector and species risk assessments.
Introduction
Aquatic invasive species are non-indigenous species (NIS) that have considerable impacts on ecosystem function, biological community composition, and global economies in marine coastal and freshwater environments (Bax et al., 2003; Molnar et al., 2008; Havel et al., 2015; Gallardo et al., 2016). Important introduction vectors include vessels (e.g., commercial ships and recreational boats) that may transport organisms into novel environments where they can become invasive (Molnar et al., 2008; Seebens et al., 2013). Biofouling and ballast water are the two most important means by which NIS may be transported to different geographic locations by ships and boats (Molnar et al., 2008; Sylvester et al., 2011; Williams et al., 2013; Chan et al., 2016). Regulations have been adopted to prevent the discharge of ballast water (used to maintain ship trim) near coastal habitats since the realization that it can disperse organisms of various life-stages that may establish (IMO [International Maritime Organization], 2004, 2021; Simard and Hardy, 2004; Firestone and Corbett, 2005; Bailey, 2015; Scriven et al., 2015). Although most work on shipping-related introductions of NIS has focused on ballast water (Bailey, 2015), biofouling by a diverse assemblage of fouling organisms (Bailey et al., 2012; Chan et al., 2012; Adams et al., 2014; Linley et al., 2014) on submerged ship surfaces, including hulls, sea chests, etc., may be an equally important vector (Coutts et al., 2010a,b; Sylvester et al., 2011; Chan et al., 2016, 2019). Likewise, biofouling of smaller boats (recreational, fishing, etc.) may be an important source of NIS introductions in many areas (e.g., Davidson et al., 2010; Ashton et al., 2014; Zabin et al., 2014; Pelletier-Rousseau et al., 2019).
Although there are no federal regulations concerning the control of biofouling in Canadian waters, Canada has adopted the voluntary International Guidelines for the Control and Management of Ships’ Biofouling to Minimize the Transfer of Invasive Species proposed by the International Maritime Organization (IMO [International Maritime Organization], 2011) and is a strategic partner to the 2017 GloFouling partnership (Chan et al., 2015; IMO [International Maritime Organization], 2017, 2021). These guidelines focus mostly on the prevention of attachment and removal of organisms from submerged ship surfaces via anti-fouling systems and cleaning programs, respectively. The efficiency of anti-fouling systems varies and cleaning is done on a voluntary basis and thus does not entirely prevent the risk of transferring organisms that accumulate over time and locations (Drake and Lodge, 2007; Sylvester et al., 2011; Chan et al., 2016; Tamburri et al., 2020).
Risk assessments (RAs) are an effective way to identify, evaluate, and estimate the level of threat of a potential NIS or pathway (Bell et al., 2011; Lodge et al., 2016). Ideally, RAs for biofouling include detailed information on all ports of call visited by a vessel along with its travel history to best understand the potential fouling communities associated with a given ship or boat. Normally, information on last port-of-call (LPoC) is used to assess the relative risk of introduction of NIS (Floerl et al., 2005; Bailey et al., 2012; Chan et al., 2012; Adams et al., 2014; Linley et al., 2014; McDonald et al., 2015; Shucksmith and Shelmerdine, 2015), under the assumption that vessels arriving from areas with differing environmental conditions (e.g., from marine to freshwater or temperate to polar waters) will be of low risk as associated organisms are assumed to be killed by inhospitable conditions. However, species within hull fouling communities may be very different from those in the LPoC, given that organisms may have accumulated over time, over voyages to multiple destinations, and subjected to a variety of environmental conditions (Drake and Lodge, 2007; Sylvester et al., 2011). In addition, vessels may only briefly transit to LPoC in zones with contrasting conditions and then quickly return to similar initial conditions (e.g., marine A to freshwater to marine B; risk to marine C would be evaluated as very low since the LPoC was freshwater; Figure 1), although the effect of such brief incursions to inhospitable conditions remains poorly understood (Miller et al., 2018). Taxa such as mollusks (bivalves and gastropods) and barnacles can resist salinity changes by closing their shells, which not only interrupts feeding and ventilation (Foster, 1970; Schoffeniels and Gilles, 1972; Hoyaux et al., 1976; van der Gaag et al., 2016) but also protects them from desiccation and osmotic and temperature stressors (e.g., Borthagaray and Carranza, 2007; Nicastro et al., 2010; McFarland et al., 2015). Many marine invertebrates can also adapt physiologically to a wide range of water temperatures (Harley et al., 2006; Sanford and Kelly, 2011; Sunday et al., 2012). If organisms can endure brief transits into inhospitable conditions, then the risk for certain classes of voyages may be greatly underestimated. Furthermore, there is evidence that certain biofouling species, including bivalves, resist exposure to high temperatures (Rajagopal et al., 2005b; Piola and Hopkins, 2012; Lenz et al., 2018). The extent to which fouling organisms may survive incursions into different inhospitable conditions, after challenges such as a combination of adverse salinity and temperature, remains unknown. This information is crucial as only organisms able to withstand these variations have the potential to invade a new location (Bailey et al., 2012; Bailey, 2015; Schimanski et al., 2016).
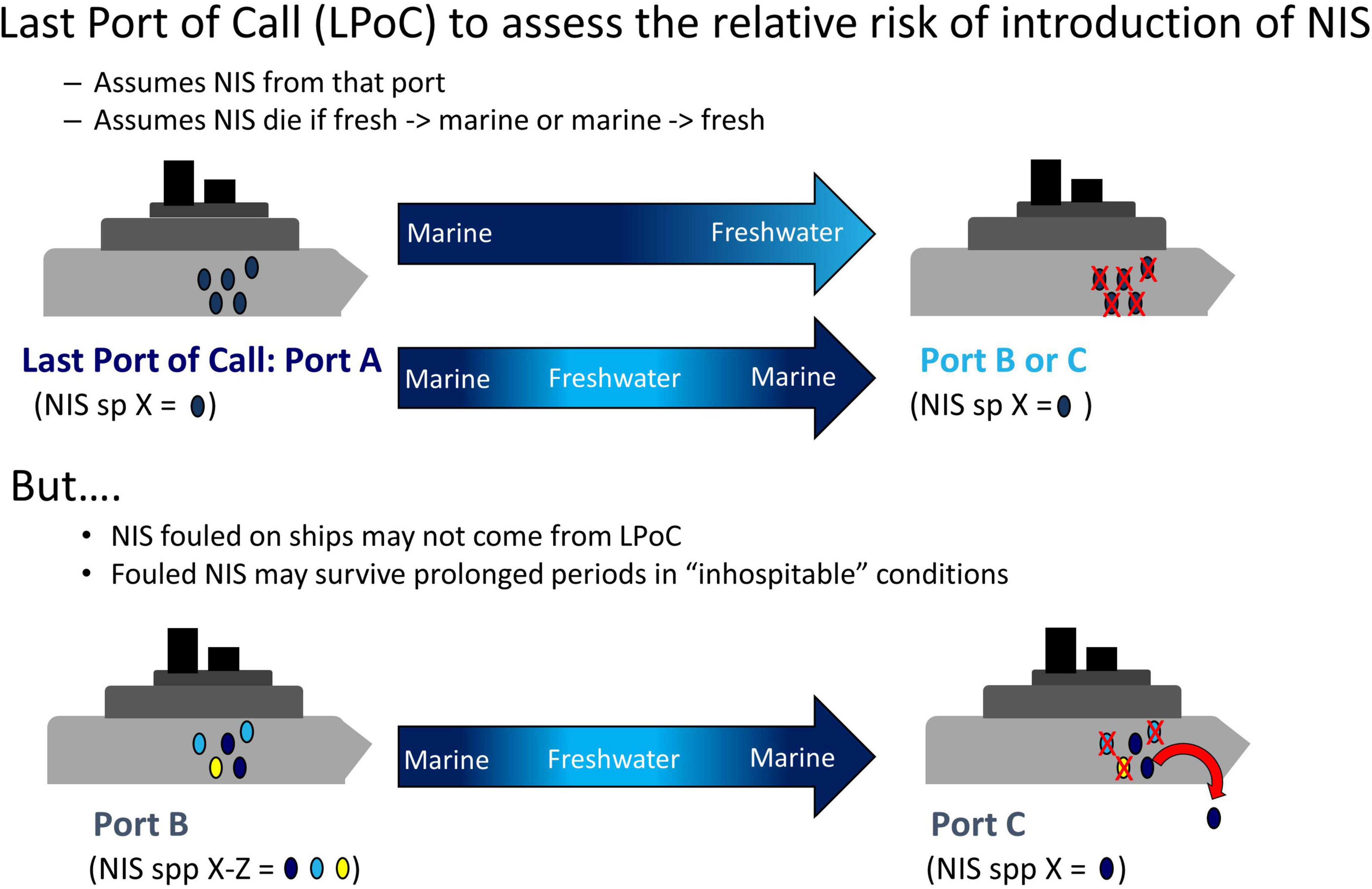
Figure 1. Conceptual diagram of transit between ports. Biofouling risk assessments only consider Last Port of Call (LPoC), but biofouling organisms from other ports with similar conditions can also be found. NIS, non-indigenous species.
Bivalves are aquatic organisms that are likely to be transported via biofouling and can have great impacts on introduced areas (Strayer et al., 1998; Lee and Chown, 2007; Herbert et al., 2016). These bivalves include freshwater mussels, such as the zebra mussel Dreissena polymorpha and other members of the family Dreissenidae, and marine mussels of the family Mytilidae, including Mytilus edulis (e.g., Fofonoff et al., 2021). The objective of this study is thus to investigate the survival of biofouling organisms in environmental conditions simulating those encountered by ships during transits and upon arrival, using these two well-known biofouling bivalves as model species. We used a laboratory system to recreate conditions experienced by hull fouling organisms during transit and measured survival of both mussel species. Blue mussels were (1) acclimatized in saltwater at different temperatures, (2) exposed to freshwater at different temperatures, and (3) returned to initial conditions for recovery; activity and survival were monitored during the different phases. Zebra mussels were exposed to the reverse conditions, i.e., freshwater to marine water and back to freshwater.
Materials and Methods
Study Organisms
Two species of mussels were used for this study, the marine blue mussel (M. edulis) and the freshwater zebra mussel (D. polymorpha), which are well-known for their invasiveness and biofouling capabilities (Berntsson and Jonsson, 2003; Drake and Lodge, 2007; De Ventura et al., 2016). Blue mussels are native to the intertidal shores of the northern Atlantic (Gosling, 2003; Moreau et al., 2005; Gaitán-Espitia et al., 2016) and part of a complex of hybridizing Mytilus species that also includes M. trossulus, M. californicus, and M. galloprovincialis (Koehn, 1991). Mytilus species are known for their plasticity which allows them to adapt to new environments and M. galloprovincialis and M. edulis have been introduced by aquaculture operations on the Pacific coast of North America and many other areas worldwide (Moreau et al., 2005; Gaitán-Espitia et al., 2016; Mathiesen et al., 2017). M. edulis is now established in South America (Hickman, 1992; but see Gaitán-Espitia et al., 2016), Asia (Tang et al., 2002), Africa (Ajani and Oyebola, 2010), and Oceania (Westfall and Gardner, 2010; Colgan and Middelfart, 2011). Blue mussel species are believed to withstand salinities ranging from 4 to 36 PSU (Gosling, 2003; van der Gaag et al., 2016).
Zebra mussels are extremely successful invaders that have spread, in only a few decades, over large regions through ballast water and hull biofouling in fresh- (Karatayev et al., 2015a,b) and brackish water (Mackie and Schloesser, 1996; Strayer et al., 1996; Minchin et al., 2002; Carlton, 2008). Zebra mussels originate from Eurasia and have invaded freshwater bodies in most European countries and in eastern North America, including the freshwater section of the St. Lawrence estuary and the Great Lakes (Johnson and Carlton, 1996; Kwan et al., 2003; Casper et al., 2014). Adult zebra mussels can withstand higher salinities than those usually found in freshwater environments at colder temperatures—up to 16 PSU in natural settings (Mackie and Schloesser, 1996; Hayward and Estevez, 1997).
Sampling Locations
Over 4,000 blue mussels (commercial size, 5–6 cm in length) were purchased from two different mussel farms from the Gaspésie (Quebec, Canada) region: Carleton (June 2018) and Gaspé (October 2018). Both mussel farms are located offshore of estuaries and exposed to seawater conditions [(Carleton (June 2018): 11.5°C, 27.1 PSU and Gaspé (October historical data): 8.0°C, 25.8 PSU)].
Zebra mussels were collected from the Parc Nautique de Lévis (Lévis, QC, Canada) marina on two occasions in June 2018 (20.8°C, 0.1 PSU) and October 2018 (10.9°C; 0.1 PSU). Over 4,000 individuals (1.9–3.0 cm in length) were obtained by SCUBA divers who scraped mussels from the underside of floating wharves.
Both blue and zebra mussels were transported by road (maximum of 4 h) to the laboratory at Maurice-Lamontagne Institute (MLI, Mont-Joli, QC, Canada). They were placed in coolers without water but kept moist with wet towels and cool with ice packs during transport. All organisms were collected and transported with the required permissions and permits. Upon reaching MLI, animals were removed from the coolers, and dead or damaged individuals were discarded. Mussels were considered dead when the shell gape was wide, there was no reaction to direct probing, and body tissues showed signs of decomposition along with a putrid odor (Nichols, 1992). The remaining zebra mussels were placed directly in the freshwater husbandry tanks of the experimental set-up, which was equipped with a high-performance filtration system. Blue mussels were placed in a 1,000 L quarantine tank filled with continuously running freshwater (∼8°C, 0.4 PSU) for 24 h to remove potential associated marine invasive species (Carman et al., 2016). All mussels were subsequently removed from the quarantine tank and any dead individuals were discarded. We recognize that this may constitute a bias (i.e., artificial selection for individuals better adapted to freshwater exposure), however, biosecurity protocols were required by MLI and very little mortality (less than 5%) was observed in blue mussels.
Coolers and all the materials used for both species were soaked for 72 h in a 0.5% chlorine solution for disinfection (Invasive Mussel Collaborative, 2017).
Mussel Husbandry
Mussels were held in meshed rectangular plastic baskets placed in large flow-through tanks (3 × 250 L of freshwater for zebra mussels and 1 × 500 L of filtered saltwater for blue mussels) with discharged water passing through the high-performance filter system, where used water was first filtered by a series of cartridges (100, 50, 5, and 1 micron) to remove particles and gametes, and then circulated through UV-C neon lights (254 nm; 30,000 μWs/cm2). The light regime consisted of 12 h light and 12 h dark (Walz, 1978; Nichols, 1992). Tanks were cleaned twice weekly to remove feces and dead individuals, and mussels were fed [40 mL Reed’s shellfish 1,800 and 40 mL Nanno 3,600 (Nannochloropsis sp.)] three times daily through slow drip-systems which kept the algae in suspension in the water column. Blue mussels were kept at ∼ 4°C (pH 8 and ≥ 27 PSU) in marine water pumped from the St. Lawrence estuary at a depth of ∼15 m, 2 km from shore. After a few weeks, post-catch mortality decreased and stabilized to less than 10 individuals per week. Zebra mussels were kept at 8°C (pH 7, 0.4 PSU), a temperature at which mussels are prevented from releasing gametes, as 12°C is the minimum temperature for spawning (Borcherding, 1991). Temperatures in husbandry tanks were the same as that of the water sources. Calcium levels and pH remained in the range of values that are adequate for survival (calcium levels ≥ 12 mg of Ca2+/L and pH values of 7.4–9.4; McMahon, 1996). Freshwater was supplied from a nearby municipality (Price, Quebec, Canada) and kept 48 h in large reservoirs to allow chlorine to evaporate before being used in the experimental system. All mussels were kept a minimum of 4 weeks in the laboratory prior to their use in trials, as post-catch stress may affect individual mortality up to that period (Kilgour and Baker, 1994). Post-catch mortality of zebra mussels decreased and stabilized to less than 50 individuals per week.
Experimental Set-Up
To recreate conditions experienced during ship transits, we manipulated temperature and salinity using a system of four water circuits: cold freshwater, warm freshwater, cold seawater, and warm seawater (Figure 2). Temperature was manipulated at the inflow of each replicate tank by mixing water from the cold and warm circuits, and salinity was manipulated by a complete change from the freshwater to the marine circuits or vice-versa. Each circuit was composed of a 250 L header tank and a 1.5 horsepower (HP) pump that continuously circulated water through conditioning and distribution loops (Figure 2). In the condition loop, water was first circulated through a sand biofilter and then either chilled with two heat pumps (0.75 and 1.5 HP) mounted in series or warmed using a 24 KW inline heater before returning to the header tank through a degassing column. Another heat pump (5 HP) was occasionally used to supplement system heating or chilling capacity. Distribution loops fed the 40 replicate tanks (15 L plastic buckets) equipped with standpipes; the loop returned excess water to header tanks through the degassing columns. The water used in the experimental tanks was either returned to a header tank through a drain or discarded. Water was renewed in each header tank using float valves to ensure that water was replaced at the same rate as it was disposed of.
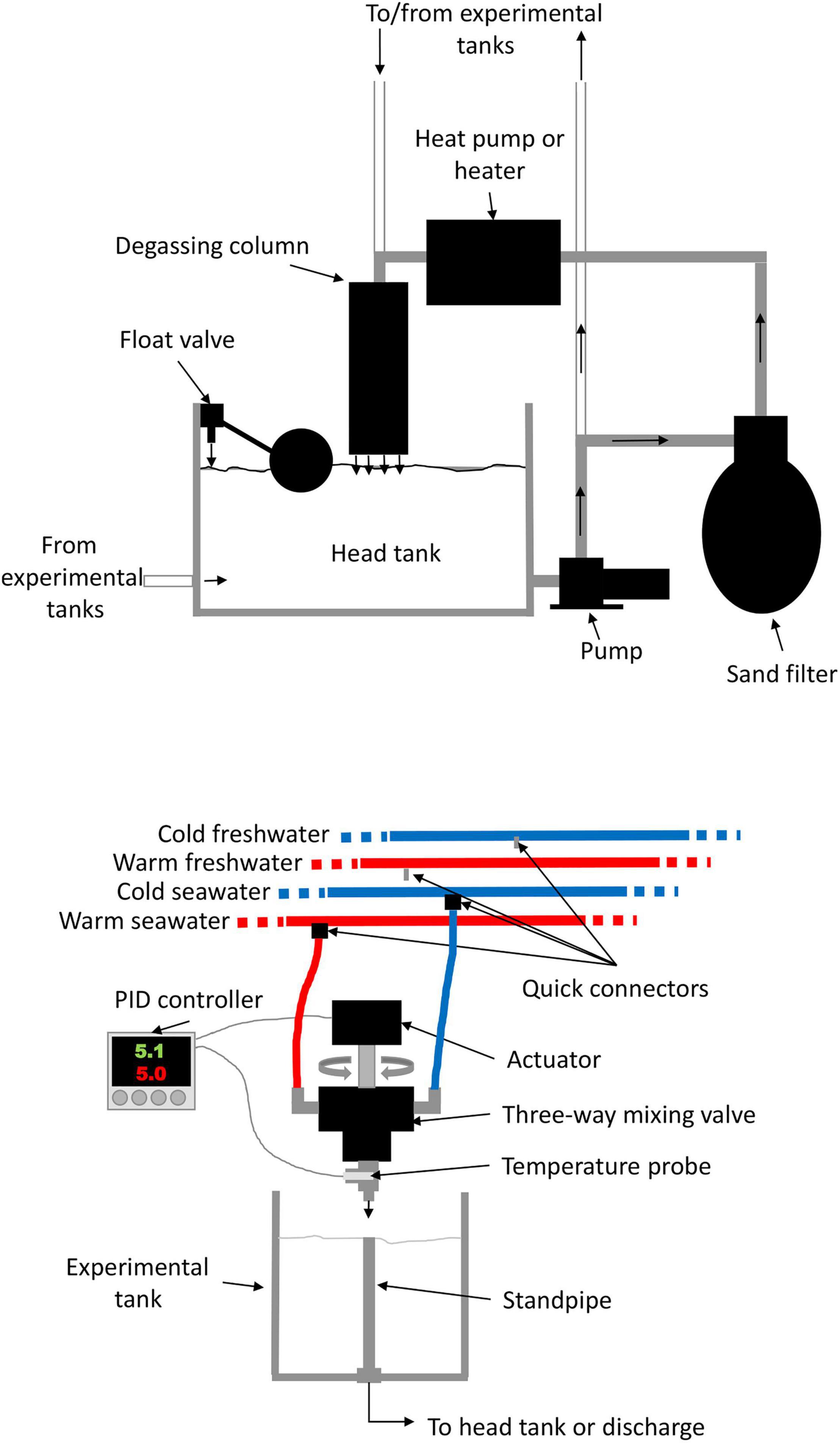
Figure 2. Diagram showing one of the 40 systems used to manipulate temperatures and salinity in the experimental tanks and one of the four systems used to condition the water.
Distribution loops brought water to each experimental tank using an individual automated system (Figure 2). Each system consisted of a three-way ball valve, with one side connected to a cold circuit and the other to a warm circuit, using flexible tubing and quick connectors. The proportional opening of each valve (thus, changing temperature over a continuum of possible temperatures within the range of the difference between the cold and warm circuits) was controlled by an actuator activated by a proportional-integral-derivative temperature controller. Desired temperatures were programmed into the controllers and the system continuously adjusted the valve opening to maintain the target temperature. Water sources were easily changed from saltwater to freshwater (or vice-versa) using quick connectors, allowing mimicking transitions between marine and freshwater environments. Independent data collectors (Onset HOBO, model UA-001-08) were placed in each aquaria and header tanks at the beginning of each experimental run to gather temperature measurements at 15 min intervals.
Treatments and Experimental Runs
We used the experimental laboratory system to mimic various conditions endured by hull fouling organisms during transits between marine and freshwater systems. Mussels were first transferred from the husbandry tank to experimental tanks (20 mussels per tank) filled with water at conditions identical to those in husbandry tanks. Water temperature was then gradually increased or decreased by maximum daily increments of 2°C (Kilgour et al., 1994; Clements et al., 2018), until the desired treatment temperature was reached (“Acclimatization A” in Figure 3). Once reached, it was held constant until all replicates reached their own target (“Acclimatization B” in Figure 3). Twenty-four hours later, water sources were switched and temperature settings adjusted; this resulted in a ∼20-min transition period to the exposure conditions (“Exposure” in Figure 3). These conditions were maintained until > 50% of the mussels (of those alive at the end of the acclimatization period) died for a particular replicate (LC50; Waller et al., 1993). Once this was reached, original conditions were restored for 5 days (“Recovery” in Figure 3) or until all individuals were dead.
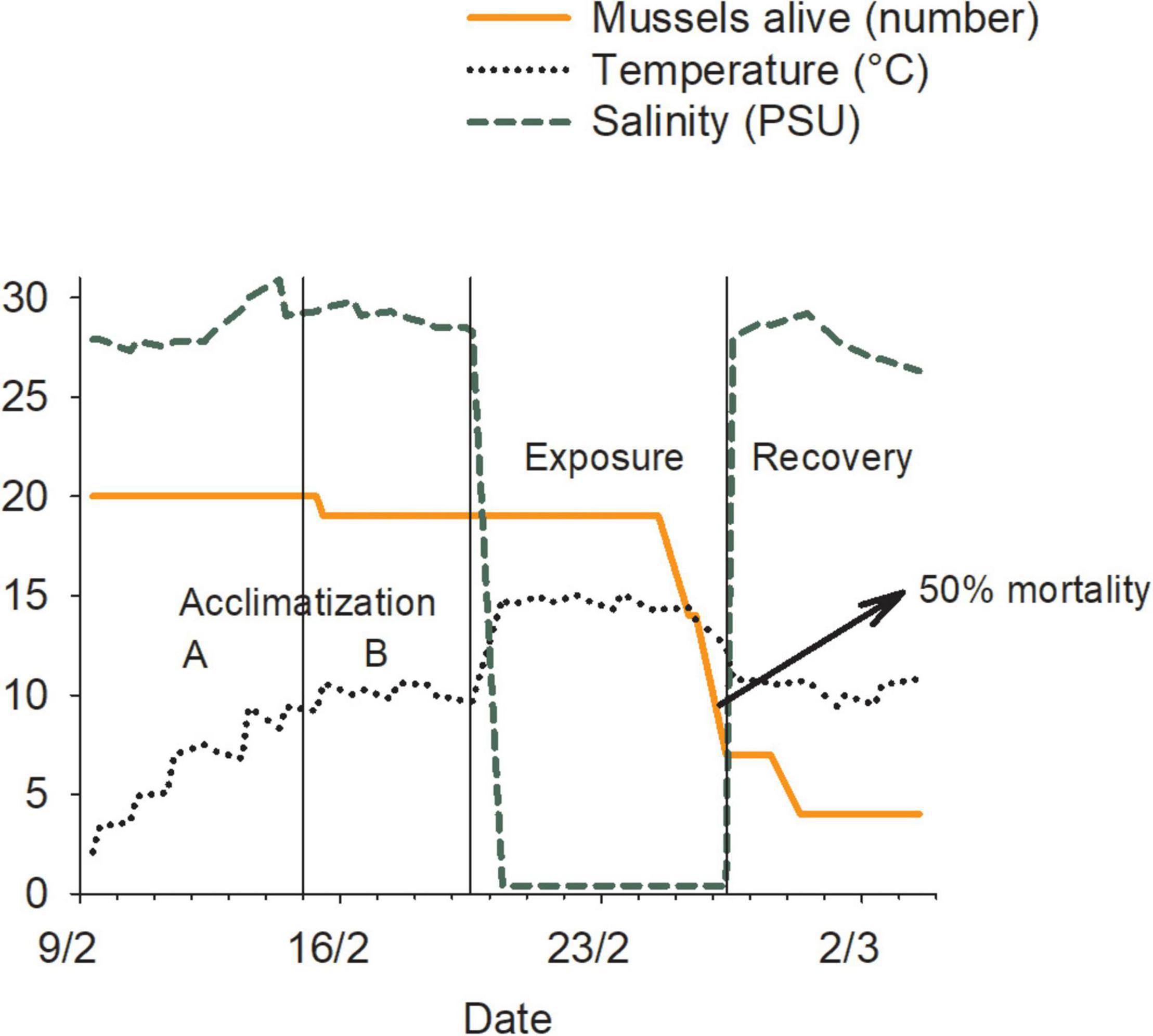
Figure 3. Representation of an experimental trial involving blue mussels (Mytilus edulis) in a 10–15 treatment replicate, showing the variation in water temperature and salinity and the timing of the experimental phases. Units for the different variables are presented in parentheses in the figure legend.
We used a total of 13 transit scenarios with temperatures ranging from 5 to 25°C. For blue mussels, treatments included acclimatization in marine water at 5°C, exposure to freshwater at 5°C, and recovery in marine water at 5 °C; this treatment was termed 5–5 (i.e., Acclimatization-Exposure); using the same terminology, the other treatments were 5–10, 10–5, 10–10, 10–15, 15–10, 15–15, 15–20, 20–15, 20–20, 20–25, 25–20, and 25–25. The treatments were the same for zebra mussels, but the order of exposure to freshwater and marine water was reversed in these scenarios. Recovery conditions were the same as those during acclimatization. In addition, control treatments, where the acclimatization conditions were maintained for the duration of the experiment, were conducted at 5, 10, 15, 20, and 25°C. Because the range of water temperatures (5–25°C) exceeded the capacities of our heating and cooling devices, the treatments were divided into two experimental blocks: the cold blocks with temperatures of 5–10–15°C (conducted in winter 2019) and the warm blocks with temperatures of 15–20–25°C (conducted in fall 2018). Within each temperature block, all treatments and controls were replicated in two separate tanks for both species, and the procedure was repeated over two separate runs, resulting in four replicates of each treatment. Note that the 15–15 treatments and controls at 15 °C were completed for each block. Over the course of the experiment, the salinity of marine water varied between 25 and 30 PSU whereas the salinity of freshwater remained constant at 0.4 PSU. From the transfer of mussels from the husbandry tanks to reaching the initial treatment temperature in experimental tanks, mussel activity was observed to verify that they were able to adapt and function (filter, feed, defecate) for all treatments. The presence of feces or pseudofeces was taken as a sign of normal mussel functioning.
Measurements
Shell lengths of all mussels were measured with digital calipers before being placed into experimental aquaria (mean ± SD = 54.83 ± 2.89 and 24.56 ± 2.23 mm for blue and zebra mussels, respectively, n = 1,600). Once all mussels were in their respective aquaria, temperature and pH were taken twice daily. Ammonia and calcium levels were checked regularly and randomly in aquaria using commercial water testing kits. Mussels were fed once per day with the same mixture of liquid algae as in the husbandry tanks during all phases of the experiment, and feces were removed daily. Observations of mussel filtering activity were recorded following a chart based on stress evaluation (Nichols, 1992), and dead individuals were counted and removed from the experimental tanks. This was performed twice daily over the entire course of the experiment.
Statistical Analyses
Non-negligible mortality was observed in the control treatments for zebra mussels; we therefore used survival analysis to evaluate the effect of temperature on survival, with a separate analysis for each temperature block. We used the proportion of individuals still alive over time (pooled over all replicates from both runs) and ran Kaplan-Meier log-rank tests followed by Holm-Sidak multiple comparisons.
The proportion of individuals filtering (taken as a proxy for mussel activity) for control treatments was analyzed using two-way factorial ANOVAs with the fixed factor Temperature and the random factor Run. Similarly, the proportion of individuals filtering during the acclimatization, exposure, and recovery phases were analyzed separately with two-way factorial ANOVAs with the fixed factor Treatment and the random factor Run. The dependent variable for the analyses of filtering activity was the average number of individuals observed filtering during the twice-daily observations for each replicate. Time to > 50% mortality and proportion of individuals still alive after the recovery period were also analyzed with two-way factorial ANOVAs with the fixed factor Treatment and the random factor Run. All ANOVAs were conducted for each species and block combination separately. Assumptions of homoscedasticity and normality of residuals were evaluated using Bartlett and Kolmogorov-Smirnov tests, respectively, and appropriate transformations were applied when assumptions were not met. Non-significant (p > 0.25) interactions between Treatment and Run were pooled with the error term and calculations of the F-ratio for Treatment were done using this pooled error term. Following detection of a significant Treatment effect, multiple comparisons were done using Tukey post hoc tests.
Results
The experimental system successfully recreated the desired temperatures for the colder treatments (cold block); although some outliers were detected, means and ranges were close to the targets with no overlap among treatments (Figure 4). More deviations from the target temperatures were detected in the warmer treatments (warm block). In particular, variability was high for the 20°C target temperature for both marine and freshwater and the 25°C target could not be reached (mean ± SD; marine 23.58°C ± 1.49; n = 28, and freshwater 23.77°C ± 1.47; n = 28). Still, means among target conditions were largely different from one another and only a few replicates overlapped with other treatments (Figure 4).
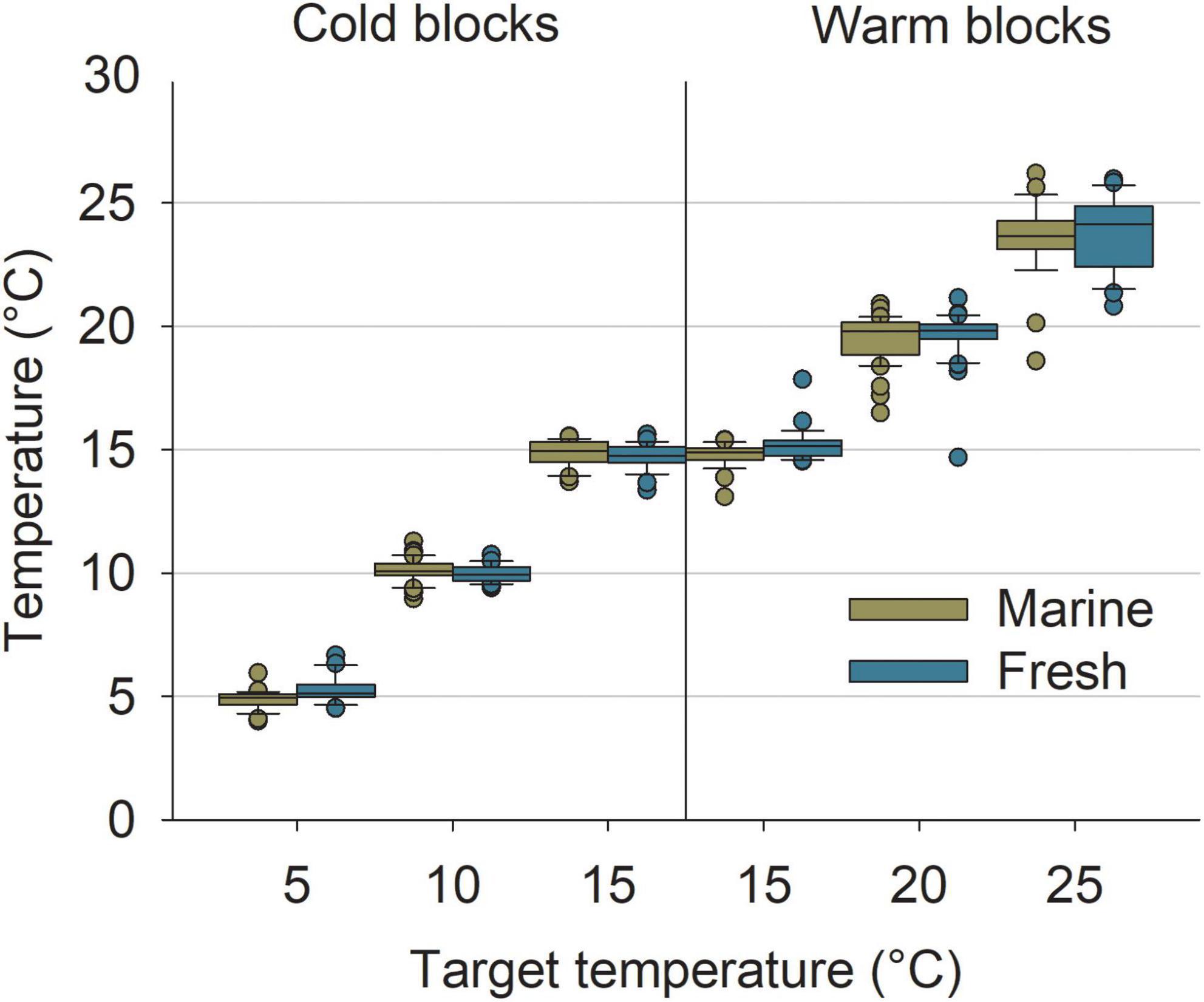
Figure 4. Measured temperatures in the experimental tanks in relation to the different target combinations of temperature and salinity. Replicates represent the mean temperature of a phase (i.e., Acclimatization B, Exposure, or Recovery). Boxes represent 25th and 75th percentiles, lines show median, error bars show 5th and 95th percentile, and dots show outliers.
In general, blue mussel survival was higher than that for zebra mussels in the control and experimental tanks for all temperatures. Blue mussels in the control treatments showed high survival with ∼ 2% mortality (10 out of 480 individuals) during the experimental periods (hence data not analyzed nor presented). In contrast, higher mortality was observed in zebra mussels control tanks with up to 55% dying in the warmer conditions (Figure 5). Survival of zebra mussels differed among temperature treatments for both the cold and warm blocks (Cold: Log-Rank Test = 7.62, df = 2, p = 0.02; Warm: Log-Rank Test = 16.20, df = 2, p < 0.001). In the cold block, multiple comparisons revealed that survival was lower at 5°C compared to 10°C; all other pairs of treatments did not differ significantly. In the warm block, all temperature treatments were significantly different from each other with decreasing survival with increasing temperature (Figure 5).
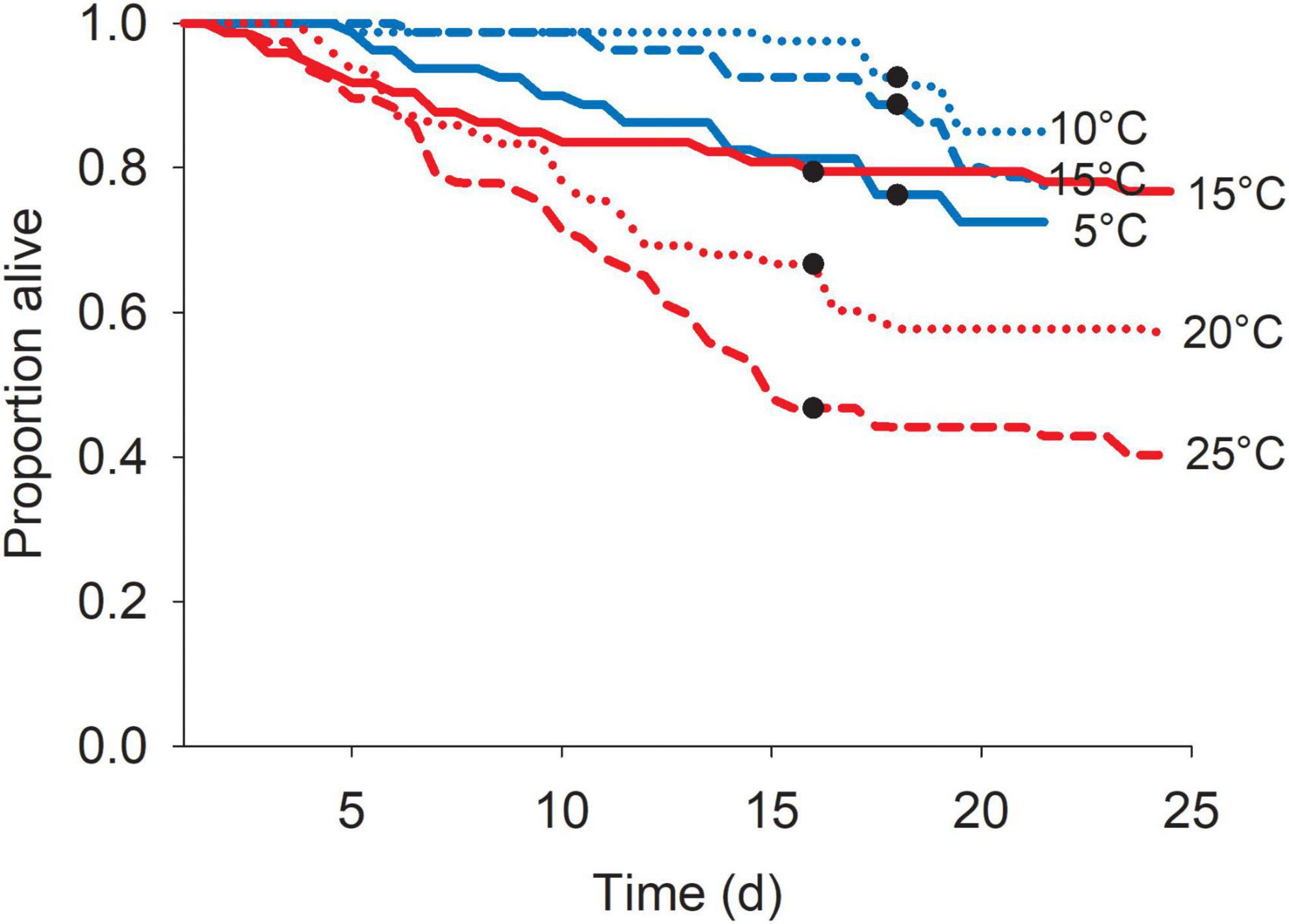
Figure 5. Survival of zebra mussels, Dreissena polymorpha, in control treatments at the various acclimatization temperatures for the cold (blue lines) and warm (red lines) blocks. Values represent the proportion of the total individuals alive over time out of 80 individuals (2 runs × 2 replicates × 20 individuals). Dots show the moment when the shortest of the two runs ended.
Blue mussels showed high filtering activity, regardless of temperature. Blue mussel activity in the control treatments was unrelated to temperature (Table 1) with ∼50 and 40% of individuals filtering in the cold and warm blocks, respectively (Figure 6A). For control zebra mussels in the cold block, between 20 and 30% of individuals were observed filtering with a significant increase in activity with increasing temperatures (Table 1 and Figure 6A). In the warm block, control zebra mussels spent most of their time with their valves closed, with ∼15% of individuals observed filtering, irrespective of temperature (Figure 6A).
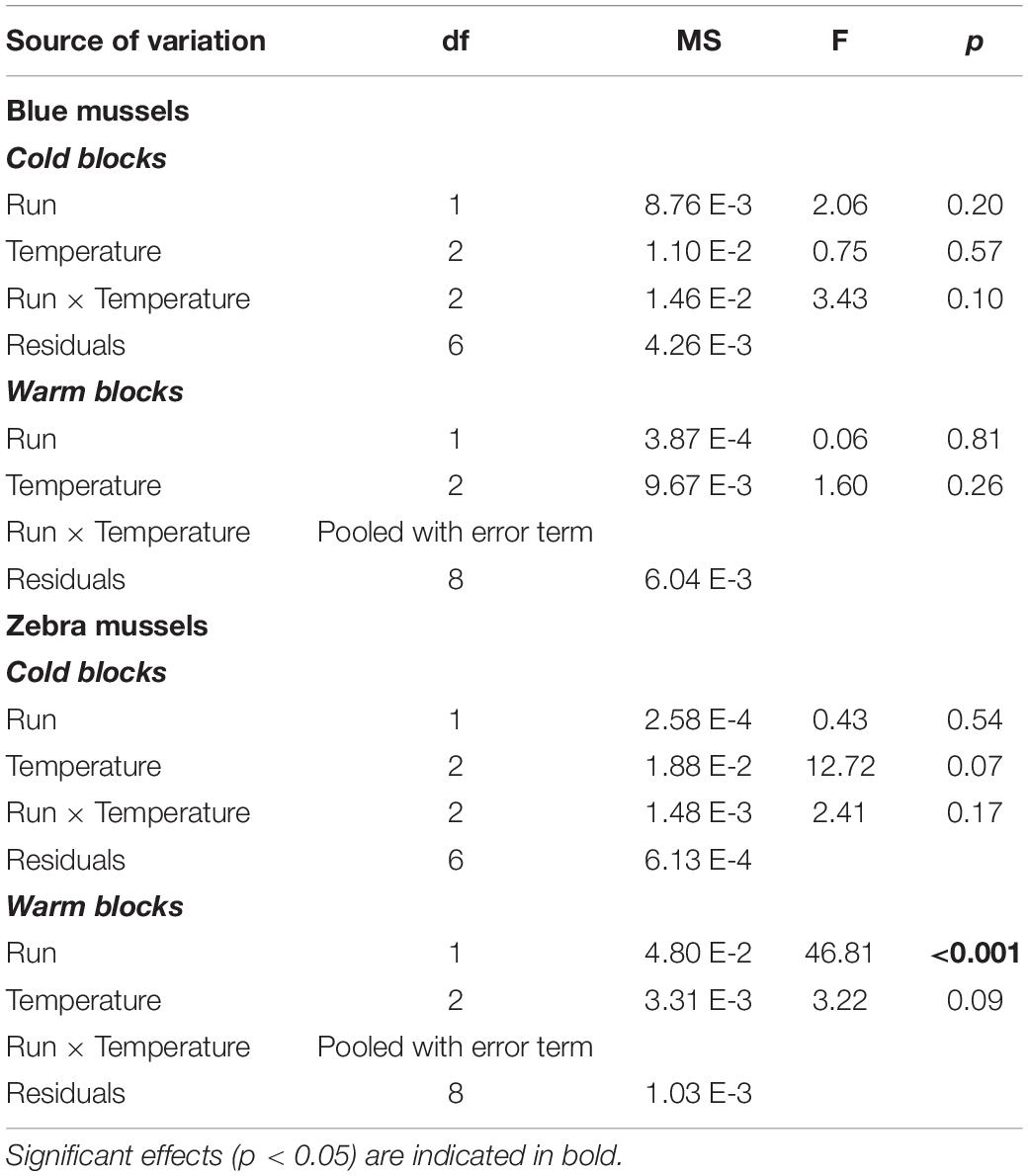
Table 1. Results of two-way factorial ANOVAs evaluating the effect of temperature on the filtration activity of blue mussels (Mytilus edulis) and zebra mussels (Dreissena polymorpha) in the control treatments.
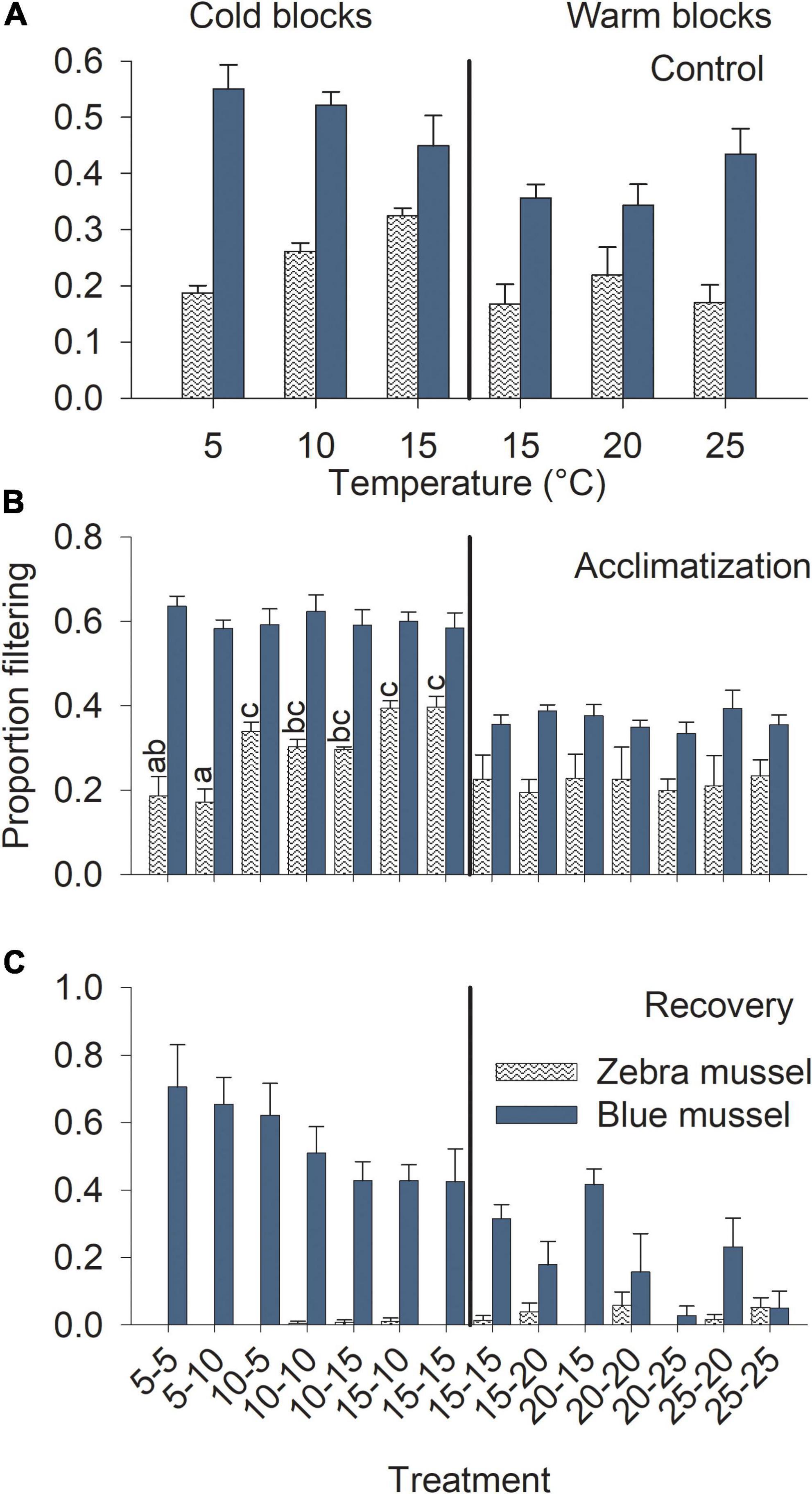
Figure 6. Filtration activity of marine blue mussels (Mytilus edulis) and freshwater zebra mussels (Dreissena polymorpha) in the control treatments at different temperatures (A) and during the acclimatization (B) and recovery (C) phases of the experiment. Bars show mean (+SE, n = 4) proportion of individuals observed filtering and columns not sharing a common letter (within a Block and Species combination) are significantly different (Tukey post hoc tests). Vertical lines show the separation between the cold and warm blocks.
During the acclimatization phase, treatment had no influence on filtering activity of blue mussels in either block (Table 2). Irrespective of temperature, mussels spent about 60 and 40% of the time filtering in the cold and warm blocks, respectively (Figure 6B). Filtering of zebra mussels during the acclimatization phase was significantly influenced by temperature in the cold, but not the warm block (Table 2). In the cold block, there was a gradual increase in filtering activity from ∼20 to 40% with increasing temperature whereas ∼20% of individuals were filtering in all treatments during the warm block (Figure 6B). With the exception of one specimen, all mussels of both species had their valves closed during the exposure phase, thus the data was not analyzed nor presented. Finally, during the recovery phase, treatment had no significant effect on blue mussel activity in either block (Table 2) although there were trends of decreasing activity with increasing temperature in both blocks (Figure 6C). Very few zebra mussels were filtering during the recovery phase and the data was thus not analyzed (Figure 6C).
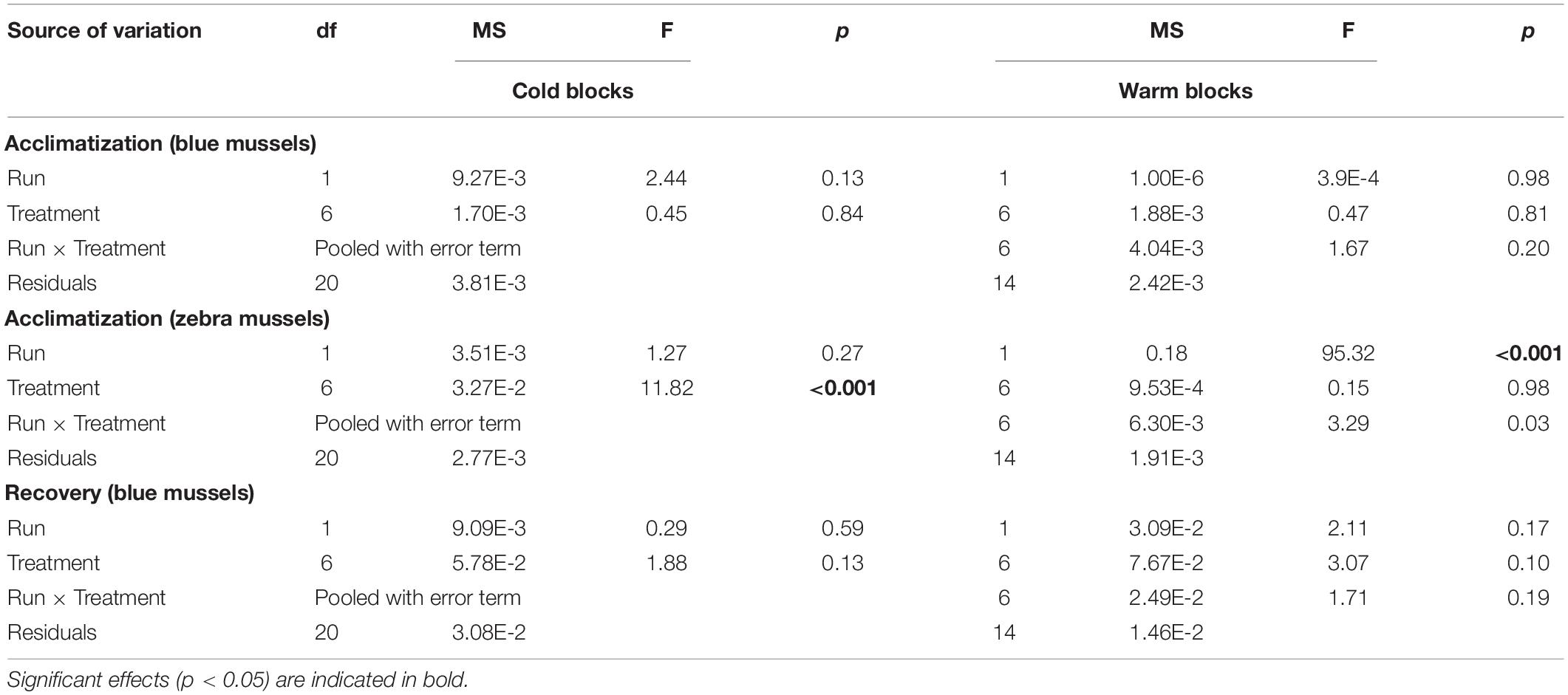
Table 2. Results of two-way factorial ANOVAs evaluating the effect of treatments on the filtration activity of blue mussels (Mytilus edulis) and zebra mussels (Dreissena polymorpha).
Time to reach 50% mortality following exposure to adverse conditions varied significantly among treatments for both species and block combinations (Table 3). Blue mussel LC50 was up to 2 weeks in the coldest treatments, whereas the maximum LC50 observed for zebra mussels was 1 week. The general trend for both species and blocks was a gradual decrease in survival time with increasing temperatures (Figure 7). In particular, exposure temperature, rather than acclimatization temperature, was the main determinant of survival time. Generally, survival times of mussels were more similar among treatments with a common exposure temperature (e.g., 5–5 and 10–5) than among treatments with similar acclimatization temperatures (e.g., 10–10 and 10–15; Figure 7).
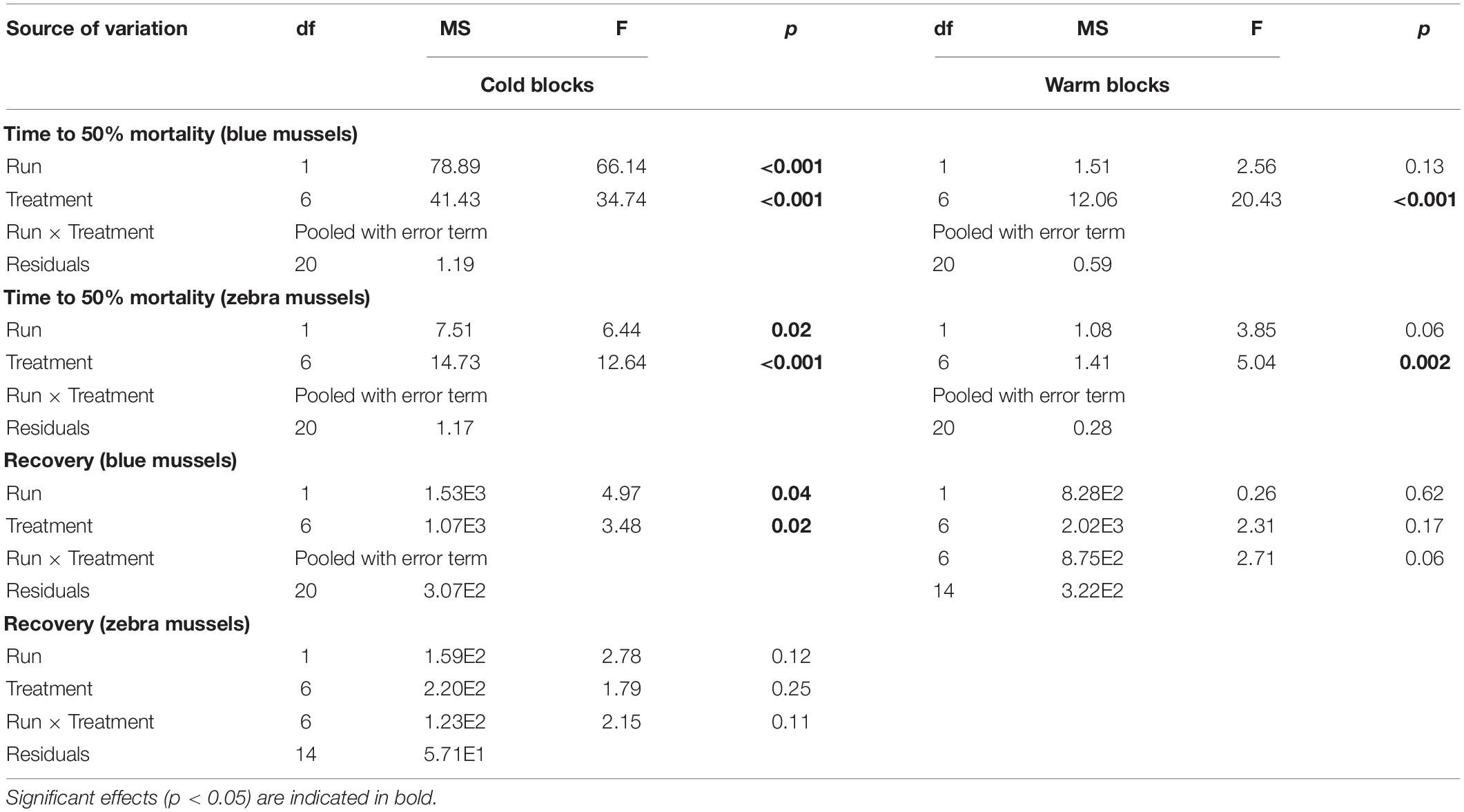
Table 3. Results of two-way factorial ANOVAs evaluating the effect of treatments on the survival of blue mussels (Mytilus edulis) and zebra mussels (Dreissena polymorpha).
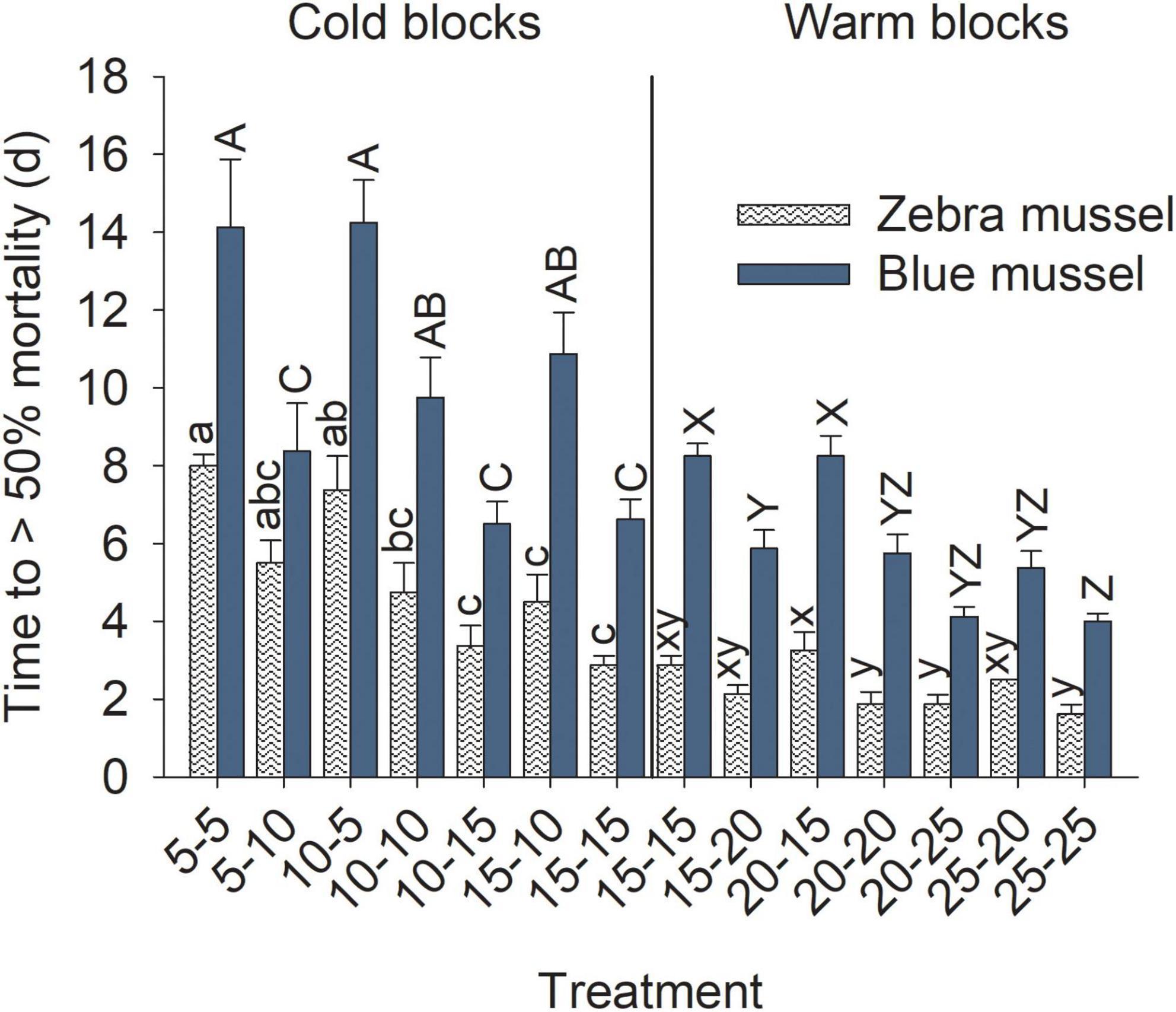
Figure 7. Time to > 50% mortality of marine blue mussels (Mytilus edulis) and freshwater zebra mussels (Dreissena polymorpha) during the exposure phase of the experiment. Bars show mean (+SE, n = 4) and columns not sharing a common letter (within a Block and Species combination) are significantly different (Tukey post hoc tests). Vertical lines show the separation between the cold and warm blocks; capital letters are for blue mussels and lowercase for zebra mussels.
Of those individuals that survived the exposure phase, the proportion that survived through the recovery phase was generally higher for blue mussels in colder conditions than those in warmer ones and it tended to decrease with increasing temperatures (Figure 8); the trend was significant in the cold (data arcsin-square-root transformed), but not the warm block (Table 3). Very few zebra mussels survived to the end of the recovery phase in the cold block and none in the warm block (Figure 8); Treatment had no significant effects for the cold block (Table 3) and data was not analyzed for the warm block.
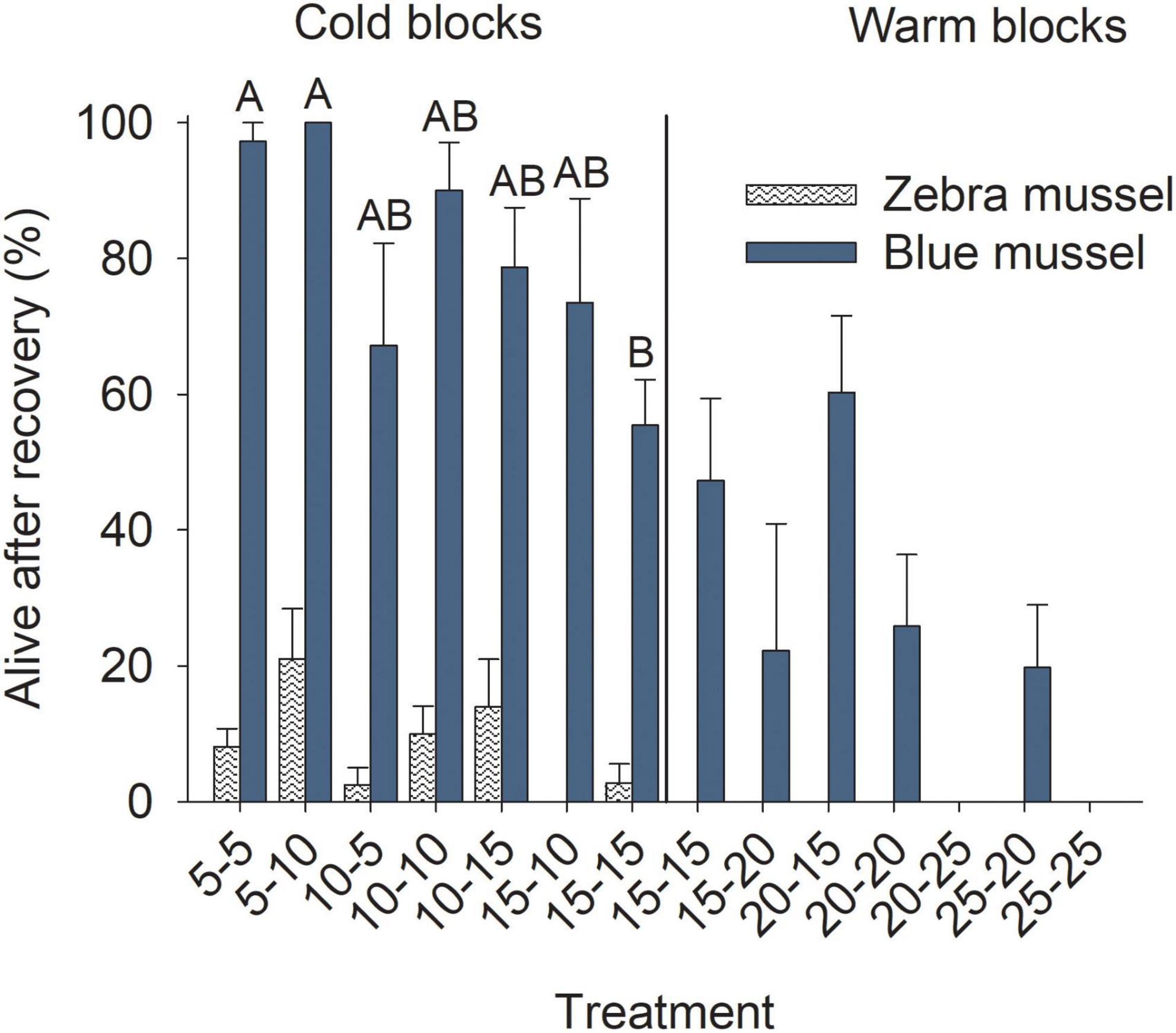
Figure 8. Proportion of marine blue mussels (Mytilus edulis) and freshwater zebra mussels (Dreissena polymorpha) still alive after 5 days of the recovery phase. Bars show mean (+SE, n = 4) and columns not sharing a common letter (within a Block and Species combination) are significantly different (Tukey post hoc tests). Vertical lines show the separation between the cold and warm blocks.
Discussion
Using an innovative experimental system, we demonstrated that well-known biofouling organisms may survive for days to weeks through a combination of inhospitable conditions, particularly at cooler temperatures. This is, to our knowledge, the first time that survival and recovery of biofouling organisms have been tested experimentally in scenarios recreating transits between freshwater and marine environments using two variables (temperature and salinity). These results are important for RA exercises, which have been used as tools to target interventions where the potential to prevent invasions is the greatest (Lodge et al., 2016; Roy et al., 2018). RAs typically base the risk of a pathway on the level of similarity in environmental conditions between the origin and recipient systems (Hayes and Barry, 2008; Floerl et al., 2009; Chan et al., 2012). Emphasis has been put on temperature and salinity as those variables are usually considered key in delimiting geographic ranges of aquatic organisms (Keller et al., 2011; Chan et al., 2013; Havel et al., 2015). Our results suggest that RAs tend to underestimate the risk associated with a transit when it involves a transition between freshwater and marine environments. For example, in previous assessments, a ship with a LPoC in freshwater would be assigned as “low risk” when entering a marine port (Bailey et al., 2012; Chan et al., 2012; Adams et al., 2014; Linley et al., 2014). Survival was affected by temperature during the exposure phase, with both species showing greater survival in colder conditions. This may be due to the bivalves’ defense mechanism, by which they can shut their valves tight under stress and isolate themselves from adverse conditions for an extended time period (Kramer et al., 1989). In such situations, bivalves would be dependent on the oxygen reserves they were able to capture in their valves; given that metabolic activity decreases with decreasing temperatures (Seed and Suchanek, 1992; Braby and Somero, 2006; Anestis et al., 2010), temperature during the exposure phase would determine how long individuals can last on such reserves. While substantial mortality occurred during simulated transits, a significant proportion of individuals survived and recovered in most scenarios tested. These results highlight that exposure to marine or freshwater (for freshwater and marine species, respectively) is no guarantee of a risk-free transit, in particular during short trips in cold waters. We therefore recommend that future RAs take into consideration the travel history of a ship (voyage length and general environmental characteristics of transit route) and the identity of the species being assessed rather than simply the LPoC. Furthermore, RAs are tools used to reduce the risks of NIS being transported to, and established in, new environments. However, in cases where high numbers of NIS are already present (likely established through biofouling), such as in California and New Zealand (Cranfield et al., 1998; Kospartov et al., 2008; Ruiz et al., 2011), RAs have supported the implementation of biofouling regulations (Georgiades et al., 2020; Scianni et al., 2021). However, reactive measures to remove or treat biofouling on ships in an environmentally responsible manner, and testing methods for jurisdictional approvals, are still under development (Scianni and Georgiades, 2019; Tamburri et al., 2020, 2021).
The effects of salinity and temperature variation have been evaluated for many types of organisms (Hoyaux et al., 1976; Berezina, 2003; Braby and Somero, 2006; Calliari et al., 2008; van der Gaag et al., 2016; Bertrand et al., 2017). Such experiments typically evaluate how organisms are affected when one or the other of these variables is increased or decreased, how they react to long exposures to various environmental conditions or a combination of the two. The innovative approach we used allowed changes in salinity and temperature to be performed rapidly to recreate transitions between different environments as experienced by fouling organisms during transits along vessel pathways. That is, to evaluate the capacity of fouling aquatic NIS to survive and acclimate to realistic scenarios of increasing/decreasing salinities and temperature, followed by a return to the initial conditions (e.g., marine A to freshwater to marine B).
This study focused on bivalves, which are known for their tolerance to adverse conditions, including rapid changes in salinity and temperature (Schoffeniels and Gilles, 1972; Hoyaux et al., 1976). Moreover, for mussels (including M. edulis), sequential heat stress events can even enhance their survival and make them more resistant to subsequent exposures (Lenz et al., 2018). Even when heat treatments are used and extreme temperatures are applied as a biofouling treatment technique, mussels have shown to be extremely resistant, with larger mussels generally being more resistant than smaller ones (Rajagopal et al., 2005b; Piola and Hopkins, 2012). Zebra mussels can survive extended periods of high temperatures (around 30°C) but only if their acclimatization temperatures are also elevated (between 15 and 20°C; Spidle et al., 1995). Our results agree with this observed tolerance to higher temperatures in blue mussels, however, zebra mussels did not perform well in the warmer treatments.
Additionally, zebra mussels can adapt to increasing salinities, especially at cooler temperatures (3–12°C) and can gradually acclimate to a combined 20°C/8 PSU, but only after a year of incremental changes in the environment (Mackie and Kilgour, 1992; Kilgour et al., 1994). They have also been shown to live at 8–12 PSU in the Caspian region (Strayer and Smith, 1992), and survived 6 days at salinities of 7.5 PSU and higher (van der Gaag et al., 2016). Our treatments were performed to mimic vessel transit and the acute changes in environmental conditions that a hull fouling community may experience, with changes in salinity occurring at a much faster rate. Any potential tolerance shown by zebra mussels in the Kilgour et al. (1994) study would likely be diminished by the speed of change at which they are exposed while in-transit. Nevertheless, for short transits between colder regions, we show that this species can still tolerate rapid salinity changes. Therefore, zebra mussels accumulated on transiting vessels may be subjected to selection pressure where individuals that have a higher resistance to temperature (Spidle et al., 1995) may actually increase this tolerance through acclimation, similar to what was observed in blue mussels by Lenz et al. (2018); similarly, an increase in tolerance to salinity was observed in the freshwater golden mussel Limnoperna fortunei (Sylvester et al., 2013).
Biofouling communities are diverse and their resistance to inhospitable conditions likely varies widely among species. Organisms that possess ways to physically isolate themselves from harsh environmental conditions, such as bivalves, gastropods, barnacles, and some bryozoans, may weather inhospitable conditions for several days (e.g., up to 18 days for M. galloprovincialis; Atalah et al., 2016 and 34 days for the oyster Crassostrea gigas; Hopkins et al., 2016). By contrast, mortality may occur very rapidly in soft-bodied taxa such as tunicates, sponges, or cnidarians and in taxa that lack a capacity to osmoregulate, such as echinoderms (Coutts et al., 2010a,b; Chan et al., 2016). Survival time under different environmental conditions for most taxa is still unknown and this information would be valuable to include in RAs for biofouling species (Keller et al., 2011; Ibáñez et al., 2014). Once the tolerance of a wide array of species is known, future RAs could include a weighting resistance factor in their calculation according to species type. For instance, a higher risk score to shelled organisms (e.g., bivalves and barnacles), and a lower score to non-shelled/soft tissue organisms (e.g., tunicates and cnidarians). Such adjustments could address assumptions that are commonly used in RA exercises (Bailey et al., 2012; Chan et al., 2012; Adams et al., 2014; Linley et al., 2014) where it is assumed that survival of fouling communities is low when the environmental conditions of the last port of call differ from recipient ports.
The higher survival of mussels under colder conditions may have important consequences from a NIS management point of view. With Arctic ice cover decreasing, the Northwest Passage is expected to see an increase in international shipping traffic (Dawson et al., 2020; Copland et al., 2021). While the Arctic was historically deemed a region with comparatively lower risk to invasion, it may play an increasing role in the spread of NIS. Since water remains cold, even during the summer months when most shipping activity occurs, biofouling organisms may be able to survive for much longer periods of time (when compared to more temperate locations), due to decreased metabolic activity. Many organisms may spawn upon arrival in coastal areas where conditions are somewhat warmer or offer more nutrition than more offshore locations (Minchin and Gollasch, 2003). Combining this with good survival due to cold temperatures in transit could lead to significant spawning events within recipient ports or harbors. Yet, factors other than temperature and salinity may influence the survival of fouling organisms and, as a result, their risk profiles. Factors such as vessel speed, proximity to major vectors or bioinvasion hotspots, or antifouling systems (Coutts et al., 2010a; Sylvester et al., 2011; Ulman et al., 2019) were not considered in this study. Furthermore, it is unknown how changes in water temperature and salinity could affect the assemblage of biofouling organisms, and how this community may in turn affect fouling mussels, considering their tertiary level in the temporal succession of biofouling phases (preceded by biofilm as primary and hard encrusting organisms and some soft algae as secondary fouling phases; Arndt et al., 2021, and the references therein).
Zebra mussel survival was likely underestimated in our study. We encountered difficulties at maintaining captive zebra mussels in good condition, as illustrated by the steady mortality rate in control treatments and husbandry tanks, as well as limited filtering activity. It has been previously shown that tolerance of zebra mussels maintained in experimental setups can be affected by collection season, water quality conditions of locations where mussels are collected, type of food, and length of time in captivity (Kilgour and Baker, 1994, and the references therein). Kilgour and Baker (1994) also observed sub-optimal health in mussels kept under laboratory conditions, possibly due to inadequate temperature and/or feeding. Although temperatures between 14 and 26°C are believed optimal for zebra mussel feeding (Lei et al., 1996), zebra mussels have been maintained under laboratory conditions at temperatures between 4 and 24°C (Nichols, 1992). In our study, we verified, through filtering and production of feces, that the food given was adequate. It was, however, observed that zebra mussels in all experimental treatment and control tanks produced very little byssus, compared to blue mussels which produced byssus in both; thus, zebra mussels may have exposed themselves more often to inhospitable conditions when trying to produce byssus, leading to greater sensitivity (Rajagopal et al., 2002, 2005a). Thus, we hypothesize we may have used individuals that could have been affected by any of these stress sources, making it possible that mussels in better condition would have yielded even longer survival times.
Results of this study clearly show that LPoC protocols for RAs of hull fouling organisms may underestimate the resistance of certain biofouling organisms which transit between freshwater and marine environments. Overly simplistic assumptions may affect overall assessment reliability, possibly resulting in misleading conclusions, depending on the organism being assessed. Hull fouling risk assessment accuracy could be improved by the inclusion of ship travel history (in addition to LPoC) and a resistance factor depending on species type and life history. This study also offers new insight on the role of low temperature during transit, highlighting the future potential role of the Arctic as a route for the spread of NIS. These factors may play an important role in increasing the probability of introduction, and thus calculated risk, since organisms may successfully transition from ports that would previously have been considered to have a low probability for successful transfer due to poor environmental match.
Data Availability Statement
The raw data supporting the conclusions of this article will be made available by the authors, without undue reservation.
Ethics Statement
Ethical review and approval were not required for the animal study because there are no animal ethics requirements for animal studies involving mussels.
Author Contributions
CR, DD, JG, KH, CHM, NS, and CWM contributed to the design and concept of the study. CR was in charge of the experimental setup, with active contributions from DD and M-FL. JMH, CWM, and NS provided scientific advice. DD performed the statistical analyses. CWM and KLH obtained funding. DD, CWM, and KLH provided resources. CR was in charge of writing the original draft of the manuscript, together with DD, JG, and CWM. All authors reviewed and edited the manuscript contributing to the final version.
Funding
Funding for this project was provided by Fisheries and Oceans Canada through a Strategic Program for Ecosystem-based Research and Advice (SPERA) grant.
Conflict of Interest
The authors declare that the research was conducted in the absence of any commercial or financial relationships that could be construed as a potential conflict of interest.
Publisher’s Note
All claims expressed in this article are solely those of the authors and do not necessarily represent those of their affiliated organizations, or those of the publisher, the editors and the reviewers. Any product that may be evaluated in this article, or claim that may be made by its manufacturer, is not guaranteed or endorsed by the publisher.
Acknowledgments
We would like to thank Frédéric Hartog, Anne-Sara Sean, Émilie Simard, and Jean-Daniel Tourangeau-Larivière (MLI divers) for collecting zebra mussels; Rafael Estrada, Olivia Lacasse, Olivier Larouche, Suzie Lévesque, Sonia Robert, François Roy, and Fabrice Simard-Gilbert for technical support; and Yves Gagnon, Jérôme Gagnon, and François Tremblay at the MLI wetlabs for technical and material support. We are grateful to the people from Parc Nautique de Lévis (Lévis, QC, Canada) for their collaboration in giving permission to access the marina facilities to collect zebra mussels.
References
Adams, J. K., Ellis, S. M., Chan, F. T., Bronnenhuber, A. G., Doolittle, J. E., Simard, N., et al. (2014). Relative Risk Assessment For Ship-Mediated Introductions Of Aquatic Nonindigenous Species To The Atlantic Region Of Canada. DFO Canadian Science Advisory Secretariat Research Document 2012/116. 403.
Ajani, E. K., and Oyebola, O. O. (2010). Growth performance of blue mussel (Mytilus edulis) in Ofiki River, Oyo State, Nigeria. Afr. J. Livest Ext. 8, 40–46.
Anestis, A., Pörtner, H., Karagiannis, D., Angelidis, P., Staikou, A., and Michaelidis, B. (2010). Response of Mytilus galloprovincialis (L.) to increasing seawater temperature and to marteliosis: metabolic and physiological parameters. Comp. Biochem. Physiol. A 156, 57–66. doi: 10.1016/j.cbpa.2009.12.018
Arndt, E., Robinson, A., Hester, S., Woodham, B., Wilkinson, P., Gorgula, S., et al. (2021). Factors That Influence Vessel Biofouling And Its Prevention And Management. Final report for CEBRA Project 190803. Melbourne, VIC: Centre of Excellence for Biosecurity Analysis, 98.
Ashton, G., Davidson, I., and Ruiz, G. (2014). Transient small boats as a long-distance coastal vector for dispersal of biofouling organisms. Estuar. Coasts 37, 1572–1581. doi: 10.1007/s12237-014-9782-9
Atalah, J., Brook, R., Cahill, P., Fletcher, L. M., and Hopkins, G. A. (2016). It’s a wrap: encapsulation as a management tool for marine biofouling. Biofouling 32, 277–286. doi: 10.1080/08927014.2015.1137288
Bailey, S. A. (2015). An overview of thirty years of research on ballast water as a vector for aquatic invasive species to freshwater and marine environments. Aquat. Ecosys. Health Manage. 18, 261–268. doi: 10.1080/14634988.2015.1027129
Bailey, S. A., Chan, F., Ellis, S. M., Bronnenhuber, J. E., Bradie, J. N., and Simard, N. (2012). Risk Assessment For Ship-Mediated Introductions Of Aquatic Nonindigenous Species To The Great Lakes And Freshwater St. Lawrence River. DFO Canadian Science Advisory Secretariat Research. 2011/104. Burlington, ON: Fisheries and Oceans Canada, 224.
Bax, N., Williamson, A., Aguero, M., Gonzalez, E., and Geeves, W. (2003). Marine invasive alien species: a threat to global biodiversity. Mar. Policy 27, 313–323. doi: 10.1016/S0308-597X(03)00041-1
Bell, A., Phillips, S., Denny, C., Georgiades, E., and Kluza, D. (2011). Risk Analysis: Vessel Biofouling. Wellington: Ministry of Agriculture and Forestry Biosecurity New Zealand.
Berezina, N. A. (2003). Tolerance of freshwater invertebrates to changes in water salinity. Russ. J. Ecol. 34, 261–266. doi: 10.1023/A:1024597832095
Berntsson, K. M., and Jonsson, P. R. (2003). Temporal and spatial patterns in recruitment and succession of a temperate marine fouling assemblage: a comparison of static panels and boat hulls during the boating season. Biofouling 19, 187–195. doi: 10.1080/08927014.2003.10382981
Bertrand, C., Devin, S., Mouneyrac, C., and Giambérini, L. (2017). Eco-physiological responses to salinity changes across the freshwater-marine continuum on two euryhaline bivalves: Corbicula fluminea and Scrobicularia plana. Ecol. Indic. 74, 334–342. doi: 10.1016/j.ecolind.2016.11.029
Borcherding, J. (1991). The annual reproductive cycle of the freshwater mussel Dreissena polymorpha Pallas in lakes. Oecologia 87, 208–218.
Borthagaray, A. I., and Carranza, A. (2007). Mussels as ecosystem engineers: their contribution to species richness in a rocky littoral community. Acta Oecol. 31, 243–250. doi: 10.1016/j.actao.2006.10.008
Braby, C. E., and Somero, G. N. (2006). Following the heart: temperature and salinity effects on heart rate in native and invasive species of blue mussels (genus Mytilus). J. Exp. Biol. 209, 2554–2566. doi: 10.1242/jeb.02259
Calliari, D., Borg, M. C. A., Thor, P., Gorokhova, E., and Tiselius, P. (2008). Instantaneous salinity reductions affect the survival and feeding rates of the co-occurring copepods Acartia tonsa Dana and A. clausi Giesbrecht differently. J. Exp. Mar. Biol. Ecol. 362, 18–25. doi: 10.1016/j.jembe.2008.05.005
Carlton, J. T. (2008). The zebra mussel Dreissena polymorpha found in North America in 1986 and 1987. J. Great Lakes Res. 34, 770–773. doi: 10.3394/0380-1330-34.4.770
Carman, M., Lindell, S., Green-Beach, E., and Starczak, V. (2016). Treatments to eradicate invasive tunicate fouling from blue mussel seed and aquaculture socks. Manage. Biol. Invasions 7, 101–110. doi: 10.3391/mbi.2016.7.1.12
Casper, A. F., Johnson, L. E., and Glémet, H. (2014). In situ reciprocal transplants reveal species-specific growth pattern and geographic population differentiation among zebra and quagga mussels. J. Great Lakes Res. 40, 705–711. doi: 10.1016/j.jglr.2014.06.005
Chan, F. T., Bailey, S. A., Wiley, C. J., and MacIsaac, H. J. (2013). Relative risk assessment for ballast-mediated invasions at Canadian Arctic ports. Biol. Invasions 15, 295–308. doi: 10.1007/s10530-012-0284-z
Chan, F. T., Bronnenhuber, J. E., Bradie, J. N., Howland, K. L., Simard, N., and Bailey, S. A. (2012). Risk Assessment For Ship-Mediated Introductions Of Aquatic Nonindigenous Species To The Canadian Arctic. DFO Canadian Science Advisory Secretariat Research Document 2011/105. Burlington, ON: Fisheries and Oceans Canada, 93.
Chan, F. T., MacIsaac, H. J., and Bailey, S. A. (2015). Relative importance of vessel hull fouling and ballast water as transport vectors of nonindigenous species to the Canadian Arctic. Can. J. Fish. Aquat. Sci. 72, 1230–1242. doi: 10.1139/cjfas-2014-0473
Chan, F. T., MacIsaac, H. J., and Bailey, S. A. (2016). Survival of ship biofouling assemblages during and after voyages to the Canadian Arctic. Mar. Biol. 163:250. doi: 10.1007/s00227-016-3029-1
Chan, F. T., Stanislawczyk, K., Sneekes, A. C., Dvoretsky, A., Gollasch, S., Minchin, D., et al. (2019). Climate change opens new frontiers for marine species in the Arctic: current trends and future invasion risks. Glob. Change Biol. 25, 25–38. doi: 10.1111/gcb.14469
Clements, J. C., Hicks, C., Tremblay, R., and Comeau, L. A. (2018). Elevated seawater temperature, not pCO2, negatively affects post-spawning adult mussels (Mytilus edulis) under food limitation. Conserv. Physiol. 6:cox078. doi: 10.1093/conphys/cox078
Colgan, D. J., and Middelfart, P. (2011). Mytilus mitochondrial DNA haplotypes in southeastern Australia. Aquat. Biol. 12, 47–53. doi: 10.3354/ab00323
Copland, L., Dawson, J., Tivy, A., Delaney, F., Cook, A., and Creed, I. (2021). Changes in shipping navigability in the Canadian Arctic between 1972 and 2016. Facets 6, 1069–1087. doi: 10.1139/facets-2020-0096
Coutts, A. D. M., Piola, R. F., Hewitt, C. L., Connell, S. D., and Gardner, J. P. A. (2010a). Effect of vessel voyage speed on survival of biofouling organisms: implications for translocation of non-indigenous marine species. Biofouling 26, 1–13. doi: 10.1080/08927010903174599
Coutts, A. D. M., Piola, R., Taylor, M. D., Hewitt, C. L., and Gardner, J. P. (2010b). The effect of vessel speed on the survivorship of biofouling organisms at different hull locations. Biofouling 26, 539–553. doi: 10.1080/08927014.2010.492469
Cranfield, H. J., Gordon, D. P., Willan, R. C., Marshall, B. A., Battershill, C. N., Francis, M. P., et al. (1998). Adventive Marine Species in New Zealand. NIWA Technical Report 34. Wellington: Ministry for Primary Industries.
Davidson, I. C., Zabin, C. J., Chang, A. L., Brown, C. W., Sytsma, M. D., and Ruiz, G. M. (2010). Recreational boats as potential vectors of marine organisms at an invasion hotspot. Aquat. Biol. 11, 179–191. doi: 10.3354/ab00302
Dawson, J., Carter, N., van Luijk, N., Parker, C., Weber, M., Cook, A., et al. (2020). Infusing Inuit and local knowledge into the Low Impact Shipping Corridors: an adaptation to increased shipping activity and climate change in Arctic Canada. Environ. Sci. Policy 105, 19–36. doi: 10.1016/j.envsci.2019.11.013
De Ventura, L., Weissert, N., Tobias, R., Kopp, K., and Jokela, J. (2016). Overland transport of recreational boats as a spreading vector of zebra mussel Dreissena polymorpha. Biol. Invasions 18, 1451–1466. doi: 10.1007/s10530-016-1094-5
Drake, J. M., and Lodge, D. M. (2007). Hull fouling is a risk factor for intercontinental species exchange in aquatic ecosystems. Aquat. Invasions 2, 121–131.
Firestone, J., and Corbett, J. J. (2005). Coastal and port environments: international legal and policy responses to reduce ballast water introductions of potentially invasive species. Ocean Dev. Int. Law. 36, 291–316. doi: 10.1080/00908320591004469
Floerl, O., Inglis, G. J., and Hayden, B. (2005). A Risk-based predictive tool to prevent accidental introductions of nonindigenous marine species. Environ. Manage. 35, 765–778. doi: 10.1007/s00267-004-0193-8
Floerl, O., Inglis, G. J., Dey, K. L., and Smith, A. (2009). The importance of transport hubs in stepping-stone invasions. J. Appl. Ecol. 46, 37–45. doi: 10.1111/j.1365-2664.2008.01540.x
Fofonoff, P. W., Ruiz, G. M., Steves, B., Simkanin, C., and Carlton, J. T. (2021). National Exotic Marine and Estuarine Species Information System. Available online at: http://invasions.si.edu/nemesis [Accessed October 21, 2021].
Foster, B. A. (1970). Responses and acclimation to salinity in the adults of some balanomorph barnacles. Phil. Trans. R. Soc. Ser. B 256, 377–400. doi: 10.1098/rstb.1970.0001
Gaitán-Espitia, J. D., Quintero-Galvis, J. F., Mesas, A., and D’Elía, G. (2016). Mitogenomics of southern hemisphere blue mussels (Bivalvia: Pteriomorphia): insights into the evolutionary characteristics of the Mytilus edulis complex. Sci. Rep. 6:26853. doi: 10.1038/srep26853
Gallardo, B., Claverdo, M., Sanchez, M. I., and Vilà, M. (2016). Global ecological impacts of invasive species in aquatic ecosystems. Glob. Change Biol. 22, 151–163. doi: 10.1111/gcb.13004
Georgiades, E., Kluza, D., Bates, T., Lubarsky, K., Brunton, J., Growcott, A., et al. (2020). Regulating vessel biofouling to support New Zealand’s marine biosecurity system – A blue print for evidence-based decision making. Front. Mar. Sci. 7:390. doi: 10.3389/fmars.2020.00390/full
Gosling, E. M. (2003). “Bivalve culture,” in Bivalve Molluscs, ed. E. M. Gosling (Oxford: Blackwell Publishing Ltd), 284–332. doi: 10.1002/9780470995532
Harley, C. D. G., Hughes, A. R., Hultgren, K. M., Miner, B. G., Sorte, C. J. B., Thornber, C. S., et al. (2006). The impacts of climate change in coastal marine systems. Ecol. Lett. 9, 228–241. doi: 10.1111/j.1461-0248.2005.00871.x
Havel, J. E., Kovalenko, K. E., Thomaz, S. M., Amalfitano, S., and Kats, L. B. (2015). Aquatic invasive species: challenges for the future. Hydrobiologia 750, 147–170. doi: 10.1007/s10750-014-2166-0
Hayes, K. R., and Barry, S. C. (2008). Are there any consistent predictors of invasion success? Biol. Invasions 10, 483–506.
Hayward, D., and Estevez, E. D. (1997). Suitability of Florida Waters To Invasion By The Zebra Mussel, Dreissena Polymorpha. Technical Report no 495. Sarasota, FL: Mote Marine Laboratory Library.
Herbert, R. J. H., Humphreys, J., Davies, C. J., Roberts, C., Fletcher, S., and Crowe, T. P. (2016). Ecological impacts of non-native Pacific oysters (Crassostrea gigas) and management measures for protected areas in Europe. Biodivers. Conserv. 25, 2835–2865. doi: 10.1007/s10531-016-1209-4
Hickman, R. W. (1992). “Mussel cultivation,” in The Mussel Mytilus: Ecology, Physiology, Genetics and Culture, ed. E. M. Gosling (Amsterdam: Elsevier Science Publishers BV), 465–510.
Hopkins, G. A., Prince, M., Cahill, P. L., Fletcher, L. M., and Atalah, J. (2016). Desiccation as a mitigation tool to manage biofouling risks: trials on temperate taxa to elucidate factors influencing mortality rates. Biofouling 32, 1–11. doi: 10.1080/08927014.2015.1115484
Hoyaux, J., Gilles, R., and Jeuniaux, C. (1976). Osmoregulation in molluscs of the intertidal zone. Comp. Biochem. Physiol. A Physiol. 53, 361–365. doi: 10.1016/S0300-9629(76)80157-0
Ibáñez, I., Diez, J., Miller, L. P., Olden, J. D., Sorte, C. J., Blumenthal, D. M., et al. (2014). Integrated assessment of biological invasions. Ecol. Appl. 24, 25–37. doi: 10.1890/13-0776.1
IMO [International Maritime Organization] (2004). International Convention For The Control And Management Of Ships’ Ballast Water And Sediments. London: International Conference on Ballast Water Management for Ships, 36.
IMO [International Maritime Organization] (2011). MEPC.207(62). Guidelines For The Control And Management Of Ships’ Biofouling To Minimize The Transfer Of Invasive Aquatic Species. London: International Maritime Organization, 25.
IMO [International Maritime Organization] (2017). New Global Project To Address Bioinvasion Via Ships’ Hull: Glofouling: IMOUNDP-GEF. Available online at: https://www.imo.org/en/MediaCentre/PressBriefings/Pages/20-biofouling.aspx New global project to address bioinvasions via ships’ hulls (imo.org) [Accessed October 21, 2021].
IMO [International Maritime Organization] (2021). International Convention For The Control And Management Of Ships’ Ballast Water And Sediments, 2004 (BWM 2004): Status of IMO treaties. London: International Maritime Organization, 506–513.
Invasive Mussel Collaborative (2017). Preventing the Spread of Invasive Zebra and Quagga Mussels: A Reference Guide for Methods of Decontaminating Gear and Equipment. Available online at: https://invasivemusselcollaborative.net/resource/gear-and-equipment-decontamination-reference-guide/ [Accessed October 21, 2021].
Johnson, L. E., and Carlton, J. T. (1996). Post-establishment spread in large-scale invasions: dispersal mechanisms of the zebra mussel Dreissena polymorpha. Ecology 77, 1686–1690. doi: 10.2307/2265774
Karatayev, A. Y., Burlakova, L., and Padilla, D. K. (2015b). Zebra versus quagga mussels: a review of their spread, population dynamics, and ecosystem impacts. Hydrobiologia 746, 97–112. doi: 10.1007/s10750-014-1901-x
Karatayev, A. Y., Burlakova, L., Mastitsky, S., and Padilla, D. K. (2015a). Predicting the spread of aquatic invaders: insight from 200 years of invasion by zebra mussels. Ecol. Appl. 25, 430–440. doi: 10.1890/13-1339.1
Keller, R. P., Drake, J. M., Drew, M. B., and Lodge, D. M. (2011). Linking environmental conditions and ship movements to estimate invasive species transport across the global shipping network. Divers. Distrib. 17, 93–102. doi: 10.1111/j.1472-4642.2010.00696.x
Kilgour, B. W., and Baker, M. A. (1994). Effects of season, stock, and laboratory protocols on survival of zebra mussels (Dreissena polymorpha) in bioassays. Arch. Environ. Contam. Toxicol. 27, 29–35. doi: 10.1007/BF002038844
Kilgour, B. W., Mackie, G. L., Baker, M. A., and Keppel, R. (1994). Effects of salinity on the condition and survival of zebra mussels (Dreissena polymorpha). Estuaries 17, 385–393. doi: 10.2307/1352671
Koehn, R. K. (1991). The genetics and taxonomy of species in the genus Mytilus. Aquaculture 94, 125–145. doi: 10.1016/0044-8486(91)90114-M
Kospartov, M., Inglis, G., Seaward, K., van den Brink, A., D’Archino, R., and Ahyong, S. (2008). Non-Indigenous And Cryptogenic Marine Species In New Zealand – Current State Of Knowledge. NIWA Research Report Prepared for MAF-BNZ Project BNZ10740. Wellington: NIWA.
Kramer, K. J., Jenner, H. A., and de Zwart, D. (1989). The valve movement response of mussels: a tool in biological monitoring. Hydrobiologia 188, 433–443. doi: 10.1007/BF00027811
Kwan, K. M., Chan, H. M., and De Lafontaine, Y. (2003). Metal contamination in zebra mussels (Dreissena polymorpha) along the St. Lawrence River. Environ. Monit. Assess. 88, 193–219. doi: 10.1023/A:1025517007605
Lee, J. E., and Chown, S. L. (2007). Mytilus on the move: transport of an invasive bivalve to the Antarctic. Mar. Ecol. Prog. Ser. 339, 307–310.
Lei, J., Payne, B. S., and Wang, S. Y. (1996). Filtration dynamics of the zebra mussel, Dreissena polymorpha. Can. J. Fish. Aquat. Sci. 53, 29–37. doi: 10.1139/cjfas-53-1-29
Lenz, M., Ahmed, Y., Canning-Clode, J., Díaz, E., Eichhorn, S., Fabritzek, A. G., et al. (2018). Heat challenges can enhance population tolerance to thermal stress in mussels: a potential mechanism by which ship transport can increase species invasiveness. Biol. Invasions 20, 3107–3122. doi: 10.1007/s10530-018-1762-8
Linley, R. D., Doolittle, A. G., Chan, F. T., O’Neill, J., Sutherland, T., and Bailey, S. A. (2014). Relative Risk Assessment For Ship-Mediated Introductions Of Aquatic Nonindigenous Species To The Pacific Region of Canada. DFO Canadian Science Advisory Secretariat Research Document 2013/043. Ottawa, ON: Fisheries and Oceans Canada, 208.
Lodge, D. M., Simonin, P. W., Burgiel, S. W., Keller, R. P., Bossenbroek, J. M., Jerde, C. L., et al. (2016). Risk analysis and bioeconomics of invasive species to inform policy and management. Ann. Rev. Environ. Resour. 41, 453–488. doi: 10.1146/annurev-environ-110615-085532
Mackie, G. L., and Kilgour, B. W. (1992). Effects of salinity on growth and survival of zebra mussels (Dreissena polymorpha). J. Shellfish Res. 11:230.
Mackie, G. L., and Schloesser, D. W. (1996). Comparative biology of zebra mussels in Europe and North America: an overview. Amer. Zool. 36, 244–258. doi: 10.1093/icb/36.3.244
Mathiesen, S. S., Thyrring, J., Hemmer-Hansen, J., Berge, J., Sukhotin, A., Leopold, P., et al. (2017). Genetic diversity and connectivity within Mytilus spp. in the subarctic and Arctic. Evol. Appl. 10, 39–55. doi: 10.1111/eva.12415
McDonald, J., Bridgwood, S., and Hourston, M. (2015). Likelihood Of Marine Pest Introduction To The Indian Ocean Territories. Fisheries Research Report No. 264 (1921845856). North Beach, WA: Department of Fisheries, 40.
McFarland, K., Baker, S., Baker, P., Rybovich, M., and Volety, A. K. (2015). Temperature, salinity, and aerial exposure tolerance of the invasive mussel, Perna viridis, in estuarine habitats: Implications for spread and competition with native oysters, Crassostrea virginica. Estuar. Coasts 38, 1619–1628. doi: 10.1007/s12237-014-9903-5
McMahon, R. F. (1996). The physiological ecology of the zebra mussel, Dreissena polymorpha, in North America and Europe. Amer. Zool. 36, 339–363. doi: 10.1093/icb/36.3.339
Miller, A. W., Davidson, I. C., Minton, M. S., Steves, B., Moser, C. S., Drake, L. A., et al. (2018). Evaluation of wetted surface area of commercial ships as biofouling habitat flux to the United States. Biol. Invasions 20, 1977–1990. doi: 10.1007/s10530-018-1672-9
Minchin, D., and Gollasch, S. (2003). Fouling and ships’ hulls: how changing circumstances and spawning events may result in the spread of exotic species. Biofouling 19, 111–122. doi: 10.1080/0892701021000057891
Minchin, D., Lucy, F., and Sullivan, M. (2002). “Zebra mussel: impacts and spread,” in Invasive Aquatic Species of Europe: Distribution, Impacts and Management, eds E. Leppäkoski, S. Gollasch, and S. Olenin (Dordrecht: Springer), 135–146. doi: 10.1007/978-94-015-9956-6_15
Molnar, J. L., Gamboa, R. L., Revenga, C., and Spalding, M. D. (2008). Assessing the global threat of invasive species to marine biodiversity. Front. Ecol. Environ. 6:485–492. doi: 10.1890/070064
Moreau, V., Tremblay, R., and Bourget, E. (2005). Distribution of Mytilus edulis and M. trossulus on the Gaspe coast in relation to spatial scale. J. Shellfish Res. 24, 545–551.
Nicastro, K. R., Zardi, G. I., McQuaid, C. D., Stephens, L., Radloff, S., and Blatch, G. L. (2010). The role of gaping behaviour in habitat partitioning between coexisting intertidal mussels. BMC Ecol. 10:17. doi: 10.1186/1472-6785-10-17
Nichols, S. J. (1992). “Maintenance of the zebra mussel (Dreissena polymorpha) under laboratory conditions,” in Zebra Mussels: Biology, Impacts and Control, eds T. Nalepa and D. Schloesser (Boca Raton, FL: Lewis Publishers), 315–329.
Pelletier-Rousseau, M., Bernier, R., Murray, C. C., Drolet, D., Lacoursière-Roussel, A., Locke, A., et al. (2019). Assessment of recreational boating as a vector for marine non-indigenous species on the Atlantic coast of Canada. Biol. Invasions 21, 2447–2470. doi: 10.1007/s10530-019-01991-1
Piola, R., and Hopkins, G. A. (2012). Thermal treatment as a method to control transfers of invasive biofouling species via vessel sea chests. Mar. Pollut. Bull. 64, 1620–1630. doi: 10.1016/j.marpolbul.2012.05.028
Rajagopal, S., Van Der Velde, G., and Jenner, H. A. (2002). Does status of attachment influence survival time of zebra mussel, Dreissena polymorpha, exposed to chlorination? Environ. Toxicol. Chem. 21, 342–346. doi: 10.1002/etc.5620210216
Rajagopal, S., van der Velde, G., van der Gaag, M., and Jenner, H. A. (2005b). Factors influencing the upper temperature tolerances of three mussel species in a brackish water canal: size, season and laboratory protocols. Biofouling 21, 87–97. doi: 10.1080/08927010500133584
Rajagopal, S., van der Velde, G., Van der Gaag, M., and Jenner, H. A. (2005a). Byssal detachment underestimates tolerance of mussels to toxic compounds. Mar. Pollut. Bull. 50, 20–29. doi: 10.1016/j.marpolbul.2004.08.015
Roy, H. E., Rabitsch, W., Scalera, R., Stewart, A., Gallardo, B., Genovesi, P., et al. (2018). Developing a framework of minimum standards for the risk assessment of alien species. J. Appl. Ecol. 55, 526–538. doi: 10.1111/1365-2664.13025
Ruiz, G. M., Fofonoff, P. W., Steves, B., Foss, S. F., and Shiba, S. N. (2011). Marine invasion history and vector analysis of California: a hotspot for western North America. Divers. Distrib. 17, 362–373. doi: 10.1111/j.1472-4642.2011.00742.x
Sanford, E., and Kelly, M. W. (2011). Local adaptation in marine invertebrates. Ann. Rev. Mar. Sci. 3, 509–535. doi: 10.1146/annurev-marine-120709-142756
Schimanski, K. B., Piola, R. F., Goldstien, S. J., Floerl, O., Grandison, C., Atalah, J., et al. (2016). Factors influencing the en route survivorship and post-voyage growth of a common ship biofouling organism Bugula neritina. Biofouling 32, 969–978. doi: 10.1080/08927014.2016.1217407
Schoffeniels, E., and Gilles, R. (1972). “Ionoregulation and osmoregulation in mollusca,” in Chemical Zoology, Vol. 7, eds M. Florkiss and B. Scheer (New York, NY: Academic Press), 393–420.
Scianni, C., and Georgiades, E. (2019). Vessel in-water cleaning or treatment: Identification of environmental risks and science needs for evidence-based decision making. Front. Mar. Sci. 6:467. doi: 10.3389/fmars.2019.00467
Scianni, C., Lubarsky, K., Ceballos-Osuna, L., and Bates, T. (2021). Yes, we CANZ: initial compliance and lessons learned from regulating vessel biofouling management in California and New Zealand. Manag. Biol. Invasions 12, 727–746. doi: 10.3391/mbi.2021.12.3.14
Scriven, D. R., DiBacco, C., Locke, A., and Therriault, T. W. (2015). Ballast water management in Canada: a historical perspective and implications for the future. Mar. Policy 59, 121–133. doi: 10.1007/s10530-017-1420-6
Seebens, H., Gastner, M. T., and Blasius, B. (2013). The risk of marine bioinvasion caused by global shipping. Ecol. Lett. 16, 782–790. doi: 10.1111/ele.12111
Seed, R., and Suchanek, T. H. (1992). “Population and community ecology of Mytilus,” in The Mussel Mytilus: Ecology, Physiology, Genetics and Culture, ed. E. M. Gosling (Amsterdam: Elsevier Science Publishers BV), 87–157.
Shucksmith, R. J., and Shelmerdine, R. L. (2015). A risk based approach to non-natives species management and biosecurity planning. Mar. Policy 59, 32–43. doi: 10.1016/j.marpol.2015.05.001
Simard, N., and Hardy, M. (2004). The Laurentian Channel as an alternative ballast water exchange zone: risk, analysis and recommendations. DFO Canadian Science Advisory Secretariat Research Document 3848. 40.
Spidle, A. P., May, B., and Mills, E. L. (1995). Limits to tolerance of temperature and salinity in the quagga mussel (Dreissena bugensis) and the zebra mussel (Dreissena polymorpha). Can. J. Fish. Aquat. Sci. 52, 2108–2119. doi: 10.1139/f95-804
Strayer, D. L., Powell, J., Ambrose, P., Smith, L. C., Pace, M. L., and Fischer, D. T. (1996). Arrival, spread, and early dynamics of a zebra mussel (Dreissena polymorpha) population in the Hudson River estuary. Can. J. Fish. Aquat. Sci. 53, 1143–1149. doi: 10.1139/cjfas-53-5-1143
Strayer, D. L., and Smith, L. C. (1992). “Distribution of the zebra mussel (Dreissena polymorpha) in estuaries and brackish water,” in Zebra Mussels: Biology, Impacts and Control, eds T. Nalepa and D. Schloesser (Boca Raton, FL: Lewis Publishers), 715–728.
Strayer, D. L., Smith, L. C., and Hunter, D. C. (1998). Effects of the zebra mussel (Dreissena polymorpha) invasion on the macrobenthos of the freshwater tidal Hudson River. Can. J. Zool. 76, 419–425. doi: 10.1139/z97-212
Sunday, J. M., Bats, A. E., and Dulvy, N. K. (2012). Thermal tolerance and the global redistribution of animals. Nat. Clim. Change 2, 686–690. doi: 10.1038/nclimate1539
Sylvester, F., Cataldo, D., Notaro, C., and Boltovskoy, D. (2013). Fluctuating salinity improves survival of the invasive freshwater golden mussel at high salinity: implications for the introduction of aquatic species through estuarine ports. Biol. Invasions 15, 1355–1366. doi: 10.1007/s10530-012-0373-z
Sylvester, F., Kalaci, O., Leung, B., Lacoursière-Roussel, A., Clarke Murray, C., Choi, F. M., et al. (2011). Hull fouling as an invasion vector: can simple models explain a complex problem? J. Appl. Ecol. 48, 415–423. doi: 10.1111/j.1365-2664.2011.01957.x
Tamburri, M. N., Davidson, I. C., First, M. R., Scianni, C., Newcomer, K. A., Inglis, G. J., et al. (2020). In-water cleaning and capture to remove ship biofouling: an initial evaluation of efficacy and environmental safety. Front. Mar. Sci. 7:437. doi: 10.3389/fmars.2020.00437/full
Tamburri, M. N., Georgiades, E. T., Scianni, C., First, M. R., Ruiz, G. M., and Junemann, C. E. (2021). Technical considerations for development of policy and approvals for in-water cleaning of ship biofouling. Front. Mar. Sci. 8:804766.
Tang, Q.-S., Fang, J.-G., and Liu, H. (2002). Development of mussel culture in China. Bull. Aquacult. Assoc. Canada 102, 66–74.
Ulman, A., Ferrario, J., Forcada, A., Arvanitidis, C., Occhipinti-Ambrogi, A., and Marchini, A. (2019). A Hitchhiker’s guide to Mediterranean marina travel for alien species. J. Environ. Manage. 241, 328–339. doi: 10.1016/j.jenvman.2019.04.011
van der Gaag, M., van der Velde, G., Wijnhoven, S., and Leuven, R. S. E. W. (2016). Salinity as a barrier for ship hull-related dispersal and invasiveness of dreissenid and mytilid bivalves. Mar. Biol. 163:147. doi: 10.1007/s00227-016-2926-7
Waller, D. L., Rach, J. J., Cope, W. G., Marking, L. L., Fisher, S. W., and Dabrowska, H. (1993). Toxicity of candidate molluscicides to zebra mussels (Dreissena polymorpha) and selected nontarget organisms. J. Great Lakes Res. 19, 695–702. doi: 10.1016/S0380-1330(93)71257-5
Walz, N. (1978). The energy balance of the freshwater mussel Dreissena polymorpha Pallas in laboratory experiments and in Lake Constance. I. Pattern of activity, feeding and assimilation efficiency. Arch. Hydrobiol. Suppl. 55, 83–105.
Westfall, K. M., and Gardner, J. P. A. (2010). Genetic diversity of Southern hemisphere blue mussels (Bivalvia: Mytilidae) and the identification of non-indigenous taxa. Biol. J. Linn. Soc. 101, 898–909. doi: 10.1111/j.1095-8312.2010.01549.x
Williams, S. L., Davidson, I. C., Pasari, J. R., Ashton, G. V., Crafton, R. E., Fontana, R. E., et al. (2013). Managing multiple vectors for marine invasions in an increasingly connected world. BioScience 63, 952–966. doi: 10.1525/bio.2013.63.12.8
Keywords: biofouling, environmental tolerance, non-indigenous bivalves, risk assessment, shipping, boating, survival
Citation: Riley C, Drolet D, Goldsmit J, Hill JM, Howland KL, Lavoie M-F, McKenzie CH, Simard N and McKindsey CW (2022) Experimental Analysis of Survival and Recovery of Ship Fouling Mussels During Transit Between Marine and Freshwaters. Front. Mar. Sci. 8:808007. doi: 10.3389/fmars.2021.808007
Received: 02 November 2021; Accepted: 27 December 2021;
Published: 18 January 2022.
Edited by:
Eugene Georgiades, Ministry for Primary Industries, New ZealandReviewed by:
Grant Hopkins, Cawthron Institute, New ZealandCristina Munari, University of Ferrara, Italy
Copyright © 2022 Riley, Drolet, Goldsmit, Hill, Howland, Lavoie, McKenzie, Simard and McKindsey. This is an open-access article distributed under the terms of the Creative Commons Attribution License (CC BY). The use, distribution or reproduction in other forums is permitted, provided the original author(s) and the copyright owner(s) are credited and that the original publication in this journal is cited, in accordance with accepted academic practice. No use, distribution or reproduction is permitted which does not comply with these terms.
*Correspondence: Cyrena Riley, Y3lyZW5hLnJpbGV5QGRmby1tcG8uZ2MuY2E=