- 1Dipartimento di Beni Culturali, University of Bologna, Ravenna, Italy
- 2Centro Interdipartimentale di Ricerca Industriale Fonti Rinnovabili, Ambiente, Mare ed Energia, University of Bologna, Ravenna, Italy
- 3Dipartimento di Scienze Biologiche, Geologiche e Ambientali, University of Bologna, Ravenna, Italy
- 4Consorzio Nazionale Interuniversitario per le Scienze del Mare, Rome, Italy
- 5Istituto di Scienze Marine, Consiglio Nazionale delle Ricerche, Bologna, Italy
- 6Dipartimento di Fisica e Astronomia “Augusto Righi,” University of Bologna, Bologna, Italy
- 7National Institute of Nuclear Physics – Section of Bologna, Bologna, Italy
- 8Dipartimento di Scienze della Vita e dell’Ambiente, Polytechnic University of Marche, Ancona, Italy
- 9Friday Harbor Laboratories, University of Washington, Friday Harbor, WA, United States
The northern Adriatic continental shelf hosts several coralligenous reefs rising from the sedimentary bottom and characterized by three main benthic assemblages, respectively, dominated by algal turfs, encrusting calcareous rhodophyte (ECRs) or erect sponges. Bioconstruction and bioerosion processes have been investigated using recruitment travertine limestone tiles deployed in a random site for each main benthic assemblages off Chioggia, 6.1–14.4 km offshore and 20.2–25.4 m depth. Tiles were retrieved after 3 and 12 years and analyzed by X-ray computed tomography (CT), allowing for non-destructively identifying and quantifying deposited and eroded limestone. The main builders were ECRs, serpulids, bryozoans, barnacles, and the bivalves Anomia ephippium, while the most effective borers were sponges from the genus Cliona and the bivalve Rocellaria dubia. The deposition of limestone after 12 years was greater at the site MR08 dominated by ECRs (12.52 ± 2.22 kg m–2), intermediate at the site P213 dominated by erect sponges (4.20 ± 1.24 kg m–2), and lower in the site P204 dominated by algal turfs (2.20 ± 0.72 kg m–2). At MR08, the deposition rate did not vary much over time (from 1.295 ± 0.270 to 1.080 ± 0.198 kg m–2 a–1), while in the other two sites, it significantly slowed down after the first survey period: from 0.952 ± 0.199 to 0.350 ± 0.103 kg m–2 a–1 at P213, and from 1.470 ± 0.462 to 0.203 ± 0.058 kg m–2 a–1 at P204. The amount of eroded limestone increased with the exposure time, with no significant differences among sites, from 1.13 ± 0.29 to 10.39 ± 1.14 kg m–2 on average at 3 and 12 years, respectively. The bioerosion rate also increased with the exposure time and was slightly higher at MR08 (from 0.682 ± 0.208 to 1.105 ± 0.088 kg m–2 a–1), mostly eroded by Cliona rhodensis in addition to C. viridis, compared to P204 (from 0.267 ± 0.078 to 0.676 ± 0.172 kg m–2 a–1) and P213 (from 0.179 ± 0.065 to 0.816 ± 0.171 kg m–2 a–1). Overall, bioconstruction has overcome the bioerosion processes in 3 years. In 12 years, the estimated net balance was essentially nil at all sites. Combining field experiment and CT analysis, this study provides the first quantification of the bioerosion and bioconstruction processes in the northern Adriatic coralligenous reefs, a fundamental step toward their conservation.
Introduction
The northern Adriatic continental shelf hosts many mesophotic biogenic reefs, arrayed on the sedimentary bottom (see the ‘‘NorthernAdriatic_Reefs’’ compilation at the Marine Geoscience Data System, MGDS1); and characterized by very heterogenous benthic assemblages (Ponti et al., 2011; Falace et al., 2015). Despite the great spatial variability of their benthic communities, these reefs can be considered as peculiar coralligenous “banks” (sensu Ballesteros, 2006) ranging in size from a few to thousands square meters and distributed between 15 and 40 m depth, where twilight conditions occur due to high water turbidity, with a mean light attenuation at the bottom of 1–2% of the surface irradiance (Ponti et al., 2011). The coralligenous reefs are among the richest, most diverse, and threatened habitats in the Mediterranean Sea. They are considered a “hot spot” of species diversity, shaped by bioconstruction and erosion processes, geological events, human impacts, and climate changes (Ballesteros, 2006). Coralligenous assemblages are exposed to anthropogenic disturbances (Claudet and Fraschetti, 2010; Coll et al., 2010, 2012) such as nutrient enrichment (e.g., Gennaro and Piazzi, 2011; Piazzi et al., 2018), increases in water turbidity and sediment deposition rates (e.g., Balata et al., 2005; Mateos-Molina et al., 2015), invasion by non-indigenous species (e.g., Piazzi and Balata, 2008; de Caralt and Cebrian, 2013), mechanical damages by anchoring, fishing nets and divers frequentation (e.g., Bavestrello et al., 1997; Hinz, 2017), and by climate change-related disturbances like exceptional storms (Teixido et al., 2013), acidification and thermal anomalies (Martin and Gattuso, 2009; Turicchia et al., 2018; Garrabou et al., 2019).
Coralligenous reefs dynamic results from the interplay between the building activities, mainly by the multi-stratified accretion of the encrusting calcareous rhodophytes (ECRs), and physical and biological eroding processes (Cerrano et al., 2001; Ingrosso et al., 2018). The biogenic reef accretion and its persistence over time depend on the predominance of the bioconstruction over dissolution and bioerosion processes (Garrabou and Ballesteros, 2000; Cerrano et al., 2001).
ECRs are the major builders of Mediterranean coralligenous reefs, contributing to limestone deposition. However, many sessile invertebrates with calcified skeletons (e.g., scleractinian corals, bryozoans, serpulid polychaetes, and bivalves) also play an important role in the bioconstruction process of the coralligenous reef by increasing and consolidating the limestone structure (Ballesteros, 2006; Ingrosso et al., 2018).
On the other hand, bioerosion is a widespread ecological process that acts at different spatial and temporal scales. It can alter habitat structure and destroy consolidated hard substrate (Davidson et al., 2018). Internal bioerosion (sensu Neumann, 1966) is generally due to a suite of endolithic organisms, including both microborers (<100 μm large) and macroborers (>100 μm; Tribollet and Golubic, 2011). Usually, the bioerosion processes on new substrates show a temporal succession that begins with microborers, settling within 1 year (Tribollet and Golubic, 2005; Färber et al., 2015; Grange et al., 2015), and continue with macroborers, which become dominant after 2–3 years (Bromley et al., 1990; Casoli et al., 2016b,2019). Macroborers comprise various organisms from a wide range of taxonomic groups, including sponges (e.g., Rosell and Uriz, 2002; Calcinai et al., 2011), polychaetes (e.g., Hutchings, 2008; Casoli et al., 2019), sipunculids (e.g., Risk et al., 1995; Gherardi and Bosence, 2001), and bivalves (e.g., Schiaparelli et al., 2005; Casoli et al., 2016b).
Most of the few long-term experiments to simultaneously measure both bioconstruction and bioerosion processes have been conducted in tropical habitats (e.g., Silbiger et al., 2014, 2016). Although some medium- and long-term field experiments on bioerosion processes have been carried out also in the Mediterranean Sea (Bromley et al., 1990; Casoli et al., 2016b,2019; Färber et al., 2016), these processes have never been investigated on coralligenous reefs of the northern Adriatic Sea, whose formation and maintenance of the diversity are poorly known. The purpose of this study is to shed light on the bioconstruction and bioerosion processes taking place in the main coralligenous reef typologies occurring in the northern Adriatic continental shelf.
Various methods allow to compute accretion and bioerosion rates in the field and in the lab, ranging from measuring the change in weight, height, volume, or density of experimental block to impregnating samples with resins to obtain casts or using different X-ray configurations (reviewed in Silbiger et al., 2016). Nowadays, the use of experimental blocks, of the same material or mineralogically similar to the substrate to be studied is the most used approach (Färber et al., 2016; Silbiger et al., 2016). However, separating and measuring the two processes simultaneously is very challenging. For this purpose, imaging methodologies in 3-dimensions (3D) based on computed tomography (CT) are very promising (Rossi et al., 2021). In the present study, the bioconstruction and bioerosion rates and their net balance on the northern Adriatic coralligenous reefs have been investigated in a field experiment using travertine limestone tiles as recruitment panels with two exposure times: 3 and 12 years. Shapes and volumes of the external biological limestone deposits and the internal bioerosion traces were non-destructively identified and measured through high-resolution CT.
Materials and Methods
Study Area and Experimental Sites
In the coralligenous reefs off Chioggia and Venice (northern Adriatic Sea) 3 main typologies of benthic assemblages have been recognized. The reefs differ in the relative abundance of ECRs, algal turf, sponges, and colonial ascidians. As a general pattern, reefs closer to the coast are dominated by algal turfs, while those further away are dominated either by ECRs or by erect sponges (Ponti et al., 2011). Based on these findings, three sites were randomly chosen, each to represent a different assemblages typology: site P204 dominated by algal turfs, P213 dominated by erect sponges, and MR08 dominated by ECRs. In addition to the benthic assemblage typologies, the sites differ in their position, depth and distance from the coast, elevation and extension of the reef, and surrounding sediments’ characteristics (Table 1). P204 was closer to the coast, shallower and much larger and higher than the other two and was surrounded by muddy sediments rich in organic matter. P213 had the deepest and smallest reef. MR08 had slightly less distance from the coast and depth than P213; however, it was surrounded by less muddy sediments but richer in organic matter. Detailed bathymetric and geomorphological maps of this sites are available at the MGDS (Ponti, 2020a,b).

Table 1. Location of the study sites (geographical coordinates, datum WGS84), and their distance from the coast, surrounding seabed depth (referred to the mean lower low water), area and max elevation of the reefs, mud and organic matter (as a percentage of loss on ignition at 450°C in 8 h) in the surrounding sediments.
The chemical-physical and biological characteristics of the waters have been obtained from the daily Mediterranean Sea Physics Reanalysis (Spatial resolution 0.042° × 0.042°, Temporal coverage 1987–2019; Escudier et al., 2020), and the daily Biogeochemical Reanalysis (Spatial resolution 0.042° × 0.042°, Temporal coverage 1999–2019; Teruzzi et al., 2021) models, and from the Mediterranean Sea Reprocessed Remote Sensing Multi Satellite observations (Spatial resolution 1 × 1 km, Temporal coverage 1997–2020; GOS Group, 2021; Supplementary Figures 1–7). According to the climatic analysis, the P204 site, which is shallower and closer to the coast, is usually exposed to temperatures 1–2°C higher than the others in summer, while it is affected by less salty waters in winter. Alkalinity is generally slightly higher at the MR08 site. P204 site experiences a greater reduction of dissolved inorganic carbon in late summer and generally shows a higher concentration of nitrate, ammonium, and phosphate. This leads to higher phytoplankton biomass, especially in spring, and a higher net summer primary production in surface waters. According to the attenuation coefficient of light at 490 nm from satellite observations, higher surface turbidity is usually measured at P204, which is closer to the coast compared to MR08 and P213. However, these satellite measures do not consider the seasonal water column stratification and the bottom nepheloid layers often occurring in the area due to sediment resuspension, especially at P213 which can be also considered very turbid (Ponti M., personal observations).
Experimental Design
In August 2005, forty-eight rough travertine tiles (15.0 × 11.5 × 1.0 cm = 172.5 cm3) were deployed 30–50 cm apart at each site on top of the reef (i.e., P204 at ∼18 m depth, and P213 at ∼24 m, MR08 at ∼21 m; Figures 1A,B). Travertine was chosen because it is a limestone characterized by a high porosity that provides a substrate suitable for many species living in the area, including endobionts, and it is also commercially available in customizable sizes. Each tile was anchored to the bottom using stainless steel washers and nails passing through a central hole (Ø 1.0 cm) and positioned horizontally to avoid any effect due to the substrate orientations (Glasby and Connell, 2001; Virgilio et al., 2006). Four travertine tiles from each site were randomly retrieved in August 2008 and in August 2017, 3 and 12 years after deployment, respectively, to quantify the bioerosion and bioconstruction processes. The other tiles were used in previous studies (Fava et al., 2016) or left in place for further investigations. Altogether 24 tiles were analyzed. Before collection, each tile was photographed underwater using a Canon PowerShot G15 digital camera, equipped with an aluminum underwater case and an INON D2000 S-TTL strobe (Figure 1C). Tiles deployment and retrieving were performed by scuba scientific divers using enriched air nitrox (EAN32).
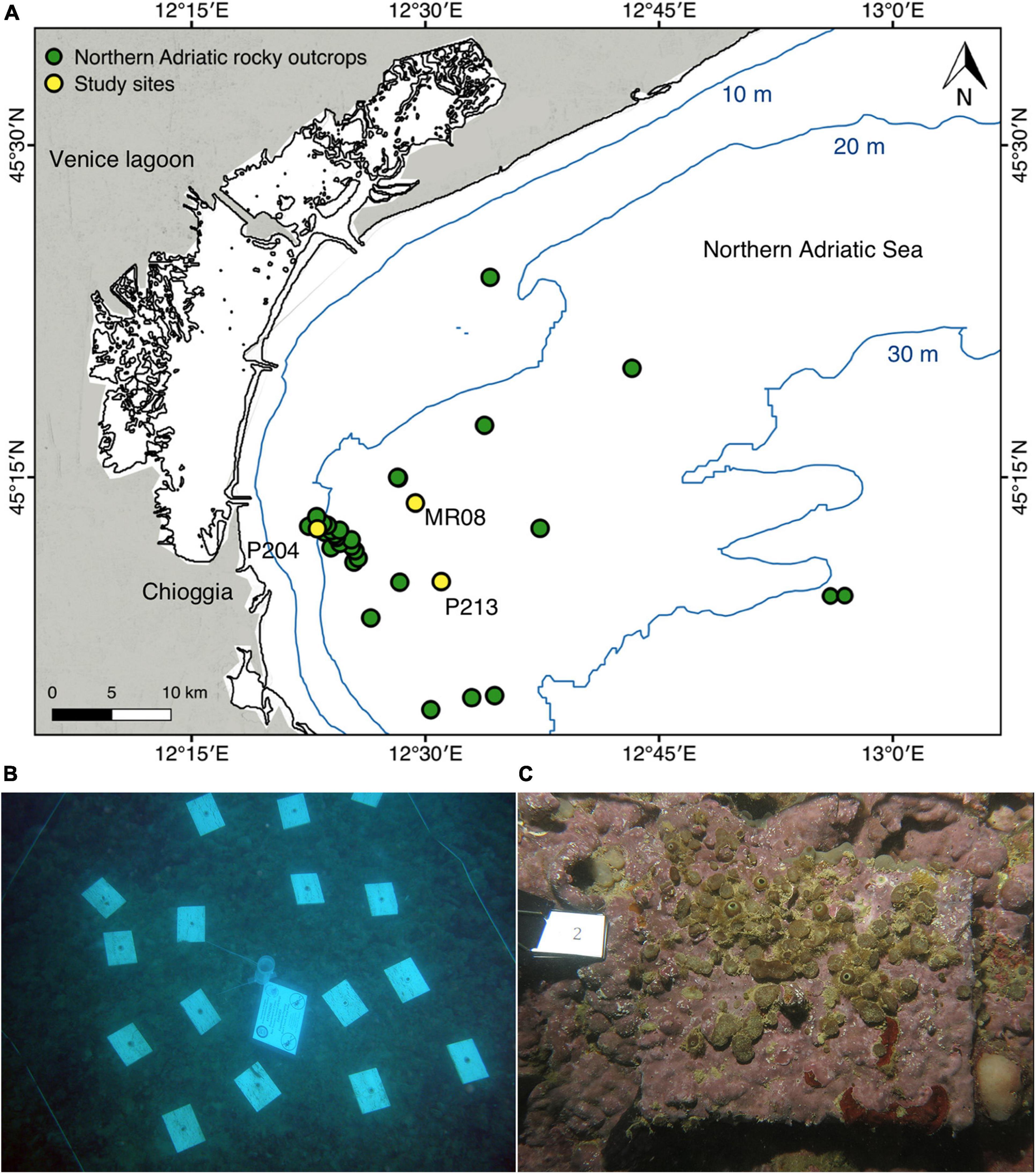
Figure 1. Sites’ location and experimental setup. (A) Map of the study area showing the three experimental sites (P204, P213, MR08), yellow dots, and the main northern Adriatic Sea coralligenous reefs off Chioggia and Venice, green dots (Mercator Projection, Datum WGS84). (B) Example of travertine tiles arrangement at P204 study site. (C) Example of travertine tile exposed for 12 years at MR08 site.
Sample Analysis
The surface of each tile was inspected under a stereomicroscope (Leica Wild M3B and Nikon SMZ1500) to classify to the lowest possible taxonomic level all visible organisms depositing limestone. Traces of all perforating organisms were recorded, and when present and accessible, the organisms occupying holes have been extracted. In particular, the boring sponges’ tissues were taken from the oscula and ostia protruding for identification through analysis of the spicules (Hooper and Van Soest, 2002) under a light microscope (Nikon ECLIPSE 50i).
After drying (80°C for 24 h), each tile was scanned using an experimental CT system composed of a microfocus X-ray source (X-ray tube Kevex PXS10-65W), a flat-panel digital detector (VARIAN PS2520D, CsI conversion screen, 1,536 × 1,920 pixels) with a pixel size of 127 μm, and a precision rotation stage (Physik Instrumente M-038). An X-ray source voltage of 130 kV and a current ranging from 50 to 180 μA were used, and the beam was filtered with a 1.5–3 mm thick aluminum foil. The radiographic projections were acquired over 360° with 0.4° angular increments. Tomographic images were reconstructed using PARREC software developed in-house (Brancaccio et al., 2011). The resulting isotropic voxel size in the adopted configuration was 0.1 mm.
Post-processing of high-resolution CT data (DICOM format files) was conducted using the AMIRA-Avizo software by Thermo Fisher Scientific. No filters were used for volume data processing. The limestone deposits and erosion traces left by different organisms were identified and manually separated in the 3D images (objects segmentation) according to their shape and density, and uniquely labeled. Segmentation was done using the brush tool from the segmentation editor suite interactively every third slice, with subsequent interpolation and check on intervening slices (Ruthensteiner and Heß, 2008). The built and eroded volumes per organism in each tile were quantified with the material statistics module, which allow to calculate the volume in voxel for each separated and labeled object.
Based on their shape and size, the main macro-bioerosion traces left by organisms’ activities were identified in terms of ichnotaxa, i.e., taxa based on the fossilized work of the organisms (descriptions and identification keys from: De Groot, 1977; Bromley and D’Alessandro, 1983, 1984, 1989, 1990; Kelly and Bromley, 1984; Gravina et al., 2019; Wisshak et al., 2019). Whenever possible, ichnotaxa have been attributed to the boring organisms found in the holes and crevices, allowing to associate the bioerosion morphologies to the responsible living species. Ontogenetic phases of boring sponge traces were determined according to Bromley and D’Alessandro (1984) and Bromley and D’Alessandro (1989); while the corresponding ontogenetic phases of living sponges were assessed inspecting tissues, following Hooper and Van Soest (2002).
Data Analysis
The overall percentages of bioconstruction and erosion ascribable to each taxon (or ichnotaxon) were calculated with respect to the initial volume of the tiles, taking into account the preexisting natural fractures of the travertine. Not having high-resolution CT images of the tiles before the deployment, the preexisting fractures inside each tile were assessed at the end of the exposure time within non-eroded portions, assuming a similar amount in the whole tile. However, being the natural fractures a possible preferential corridor for borer organisms, this procedure may lead to an underestimation of the preexisting cavities in the most eroded portions.
Limestone rocks density ranged from 2.3 to 2.7 g cm–3 according to their porosity (Schön, 2011); the initial mean density of employed travertine was 2.5 g cm–3, corresponding to the porosity of 7%, which is in the range of travertine building stones (García-del-Cura et al., 2012; Chentout et al., 2015).
Volumes measured in voxels were converted to cm3. The limestone gain and loss (g) were obtained by multiplying, respectively, the final built and the eroded volumes (cm3) by the compact limestone density (2.7 g cm–3). The results were divided by the tile surface and expressed in limestone mass deposited or eroded per area (kg m–2). Bioconstruction and bioerosion mean annual rates (kg m–2 a–1) were calculated by dividing the limestone gain and loss per square meter by the exposure time (i.e., 3 or 12 years). In the bioconstruction and erosion graphs, the percentage of the experimental tiles initial volume has been reported on the secondary axis.
Differences in built and bioeroded mass per area of each taxon (or ichnotaxon) and in bioconstruction and bioerosion rates among sites (Si: 3 levels, fixed), between exposure time (Et: 2 levels, fixed) and in their interaction (Si × Et) were assessed by two-way crossed analysis of variance (ANOVA, α = 0.05; Underwood, 1997). Cochran’s C-test was used to check the assumption of homogeneity of variances and, when necessary, data transformations were applied. In the ANOVA, when the term Si × Et was significant, the Student–Newman–Keuls (SNK) test was used for post hoc comparisons.
The mean percentage bioconstruction-bioerosion net balance was assessed for each site after the 3- and 12-year exposure times. Differences from zero were tested with a t distribution (α = 0.05).
The statistical analyses were performed in R (R Core Team, 2019) with GAD, a specific package for general ANOVA designs (Sandrini-Neto and Camargo, 2012). Mean values were always reported along with their standard errors (s.e.).
Results
Bioconstruction
The main contributors to limestone deposition were ECRs (Figure 2A), the bivalve Anomia ephippium Linnaeus, 1758 (Figure 2B), serpulid polychaetes (mainly Spirobranchus triqueter (Linnaeus, 1758) and Serpula concharum Langerhans, 1880; Figures 2C,D, respectively), bryozoans [including species belonging to Cyclostomatida and Cheilostomatida, like Figularia sp., Callopora sp., and Celleporina sp. and Schizomavella (Schizomavella) linearis (Hassall, 1841); Figure 2E], and the barnacles Verruca stroemia (O.F. Müller, 1776). When these taxa were over-growing on each other, the single taxon cannot be distinguished on CT images because the limestone they deposit has similar density. For the bioconstruction budget they were collectively categorized as “encrusting organisms.” The mean limestone deposited per area by these encrusting organisms were 3.71 ± 0.77 kg m–2 after 3 years of exposure and 2.20 ± 0.72 kg m–2 after 12 years at the P204 site; 2.86 ± 0.60 and 4.20 ± 1.24 kg m–2 at P213; and 3.88 ± 0.81 and 12.52 ± 2.22 kg m–2 at MR08 (Figure 3A).
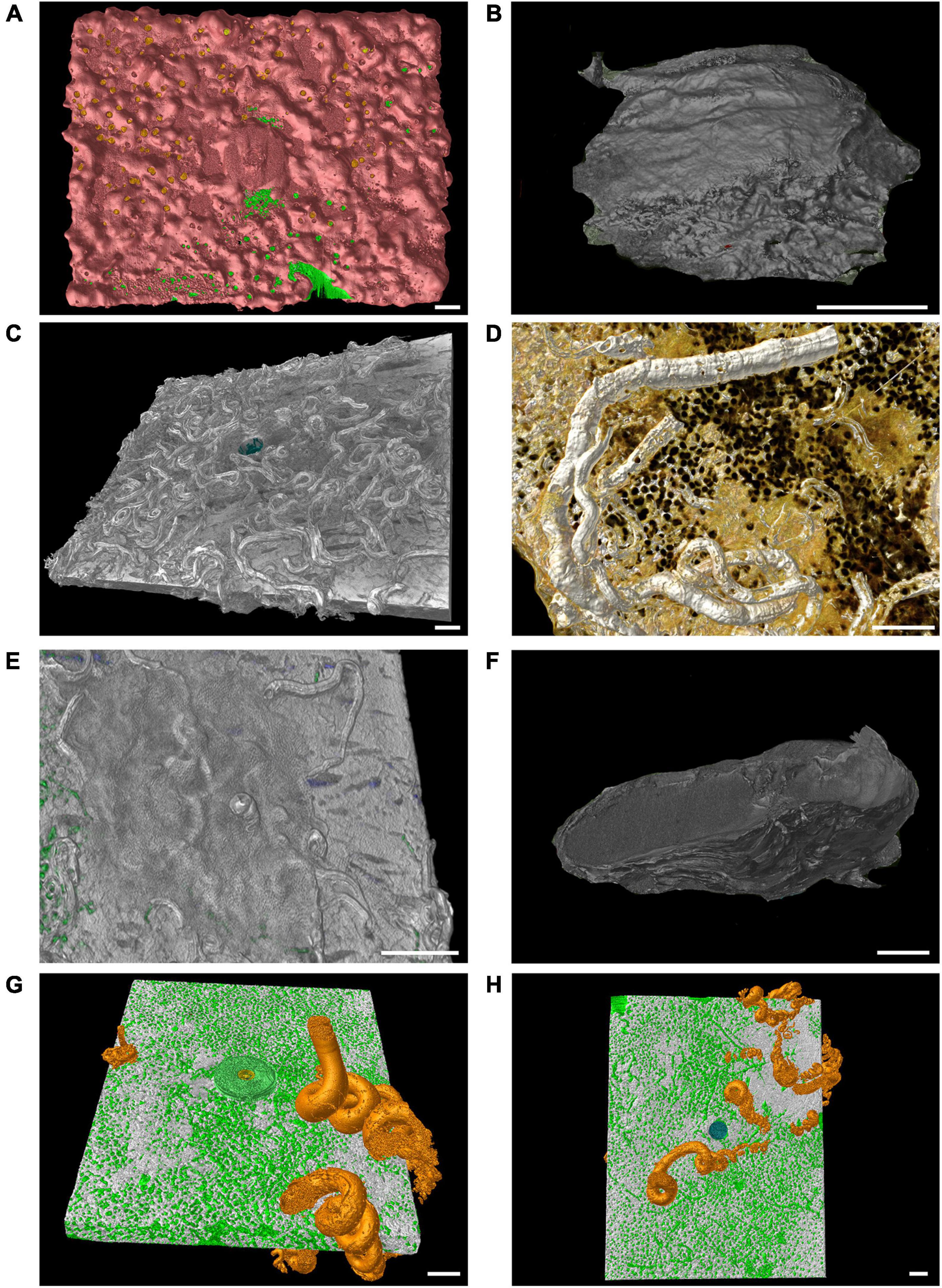
Figure 2. Computed tomography graphic reconstruction of calcareous organisms grown on travertine tiles. (A) Coverage of encrusting calcareous rhodophytes (pink) at MR08 after 12 years. (B) A shell of Anomia ephippium at P204 after 3 years. (C) Serpulid polychaetes tubes (mainly Spirobranchus triqueter) at P204 site after 3 years. (D) Tubes of the serpulid polychaetes Serpula concharum at MR08 after 12 years. (E) The encrusting bryozoan Schizomavella (Schizomavella) linearis which covered the serpulid polychaetes tubes at MR08 after 3 years, in green traces of boring sponges inside the tile. (F) A shell of Magallana gigas found at P204 after 3 years. (G,H) The shell of the vermetid Thylacodes arenarius (bright orange) in the same tile (top and bottom view, respectively) at MR08 after 12 years, in green traces of boring sponges inside the tile. Scale bars are 1 cm long.
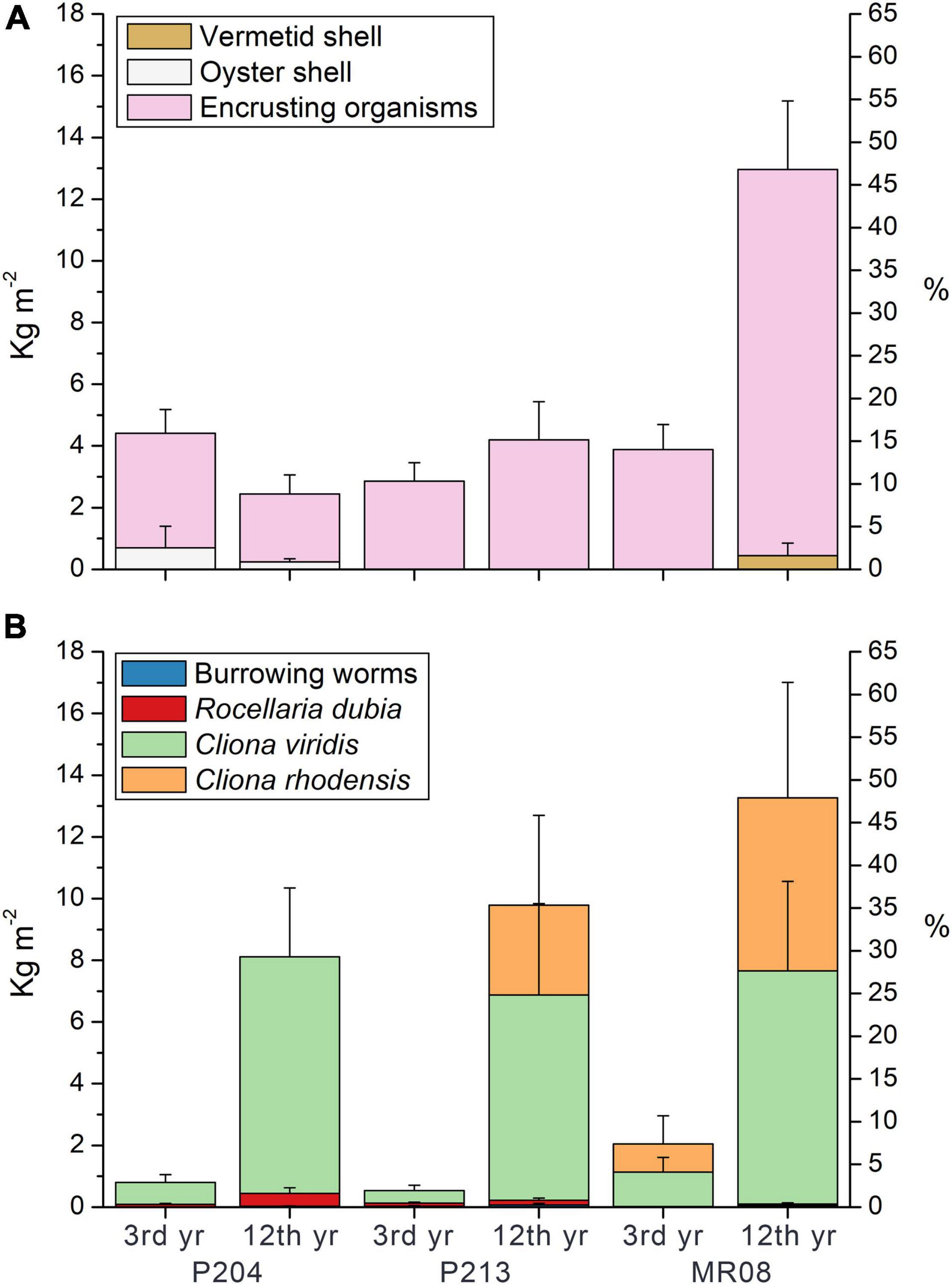
Figure 3. Mean (± s.e.) limestone deposited (A) and eroded (B) per area (kg m−2) by different organisms at each study sites (P204, P213, MR08) and per exposure times (3 and 12 years) measured in the experimental tiles. Percentage axes represent the relative contribution to the bioconstruction and bioerosion, in volume, compared to the initial size of the experimental tiles.
Occasional large contributions to bioconstruction came from the Pacific oyster Magallana gigas (Thunberg, 1793), which shells were found at the P204 site, including a large shell on a tile retrieved after 3 years of exposure (Figure 2F), and some smaller ones on three out of four tiles after 12 years (0.24 ± 0.10 kg m–2 limestone deposited in 12 years). At MR08, after 12 years, three out of four tiles showed shells of vermetid snails Thylacodes arenarius (Linnaeus, 1758), accounting for a mean limestone deposit of 0.45 ± 0.41 kg m–2. Note that vermetid snails can easily develop on both sides of the tiles (Figures 2G,H, top and bottom view, respectively).
The total limestone deposited per area were 4.41 ± 1.39 kg m–2 after 3 years of exposure and 2.44 ± 0.70 kg m–2 after 12 years at the P204 site; 2.86 ± 0.60 and 4.20 ± 1.24 kg m–2 at P213; and 3.88 ± 0.81 and 12.97 ± 2.37 kg m–2 at the MR08 site (Figure 3A). The ANOVA tests on the mean limestone deposited, both as a whole and by encrusting organisms, detected significant differences in the interaction across sites and exposure time (Table 2A). In both analyses, post hoc SNK tests revealed not significant differences in limestone deposition among sites at 3-year exposure time, and between 3 and 12 years at P204 and P213. Conversely, at 12 years, the whole bioconstruction, and that due to encrusting organisms, at MR08 were significantly higher than in the other two sites and compared to the 3-year exposure.
After 3 years of exposure, the mean bioconstruction annual rate ranges from 0.952 ± 0.199 kg m–2 a–1 at P213 to 1.470 ± 0.462 kg m–2 a–1 at P204, while after 12 years, it ranged from 0.203 ± 0.058 kg m–2 a–1 at P204 to 1.080 ± 0.198 kg m–2 a–1 at MR08 (Figure 4A). ANOVA test detected a significant effect of the interaction between the years of exposure and the study sites on the bioconstruction annual rate. According to the SNK tests, the bioconstruction annual rates after 3 years were not significantly different among the three sites. Those estimated at 12 years were significantly lower than after 3 years, both at P204 and P213, while no significant decrease was observed at MR08 (Table 3).
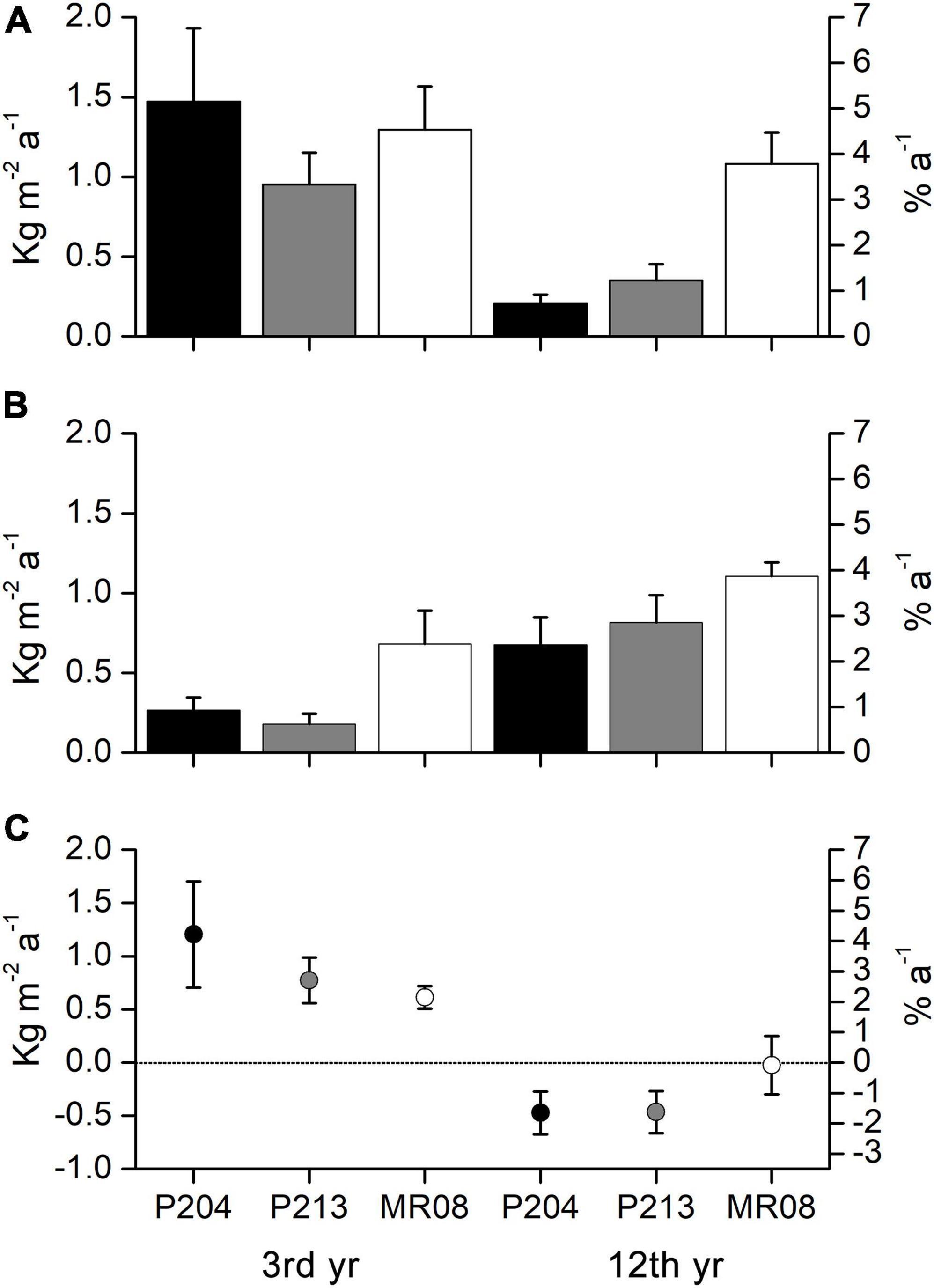
Figure 4. Mean (± s.e.) annual bioconstruction (A) and bioerosion (B) rates (kg m–2 a–1 and % a–1), and annual bioconstruction-bioerosion net balance (C) (kg m–2 a–1 and % a–1) measured on the experimental tiles for each site after 3 and 12 years of exposure (dashed line marks zero net balance). Percentage axes represent the relative contribution to the annual bioconstruction and bioerosion rate and net balance, in volume, compared to the initial size of the experimental tiles.
Bioerosion
Inside the experimental tiles ten different ichnotaxa were identified, five of which were attributed to sponges, four to burrowing worms, and one to endolithic bivalves. Identified ichnotaxa and the related living species, including sponge ontogenetic phases, were reported in Table 4.
Cliona viridis (Schmidt, 1862) was recognized as a trace maker differentiable from the Entobia ichnogenera: Entobia cateniformis Bromley and D’Alessandro, 1984 (Figures 5A,B), Entobia cf ovula Bromley and D’Alessandro, 1984 (Figure 5C) and Entobia cf laquei Bromley and D’Alessandro, 1984 (Figure 5D) both in tiles of 3 and 12 years of exposure at all the study sites.
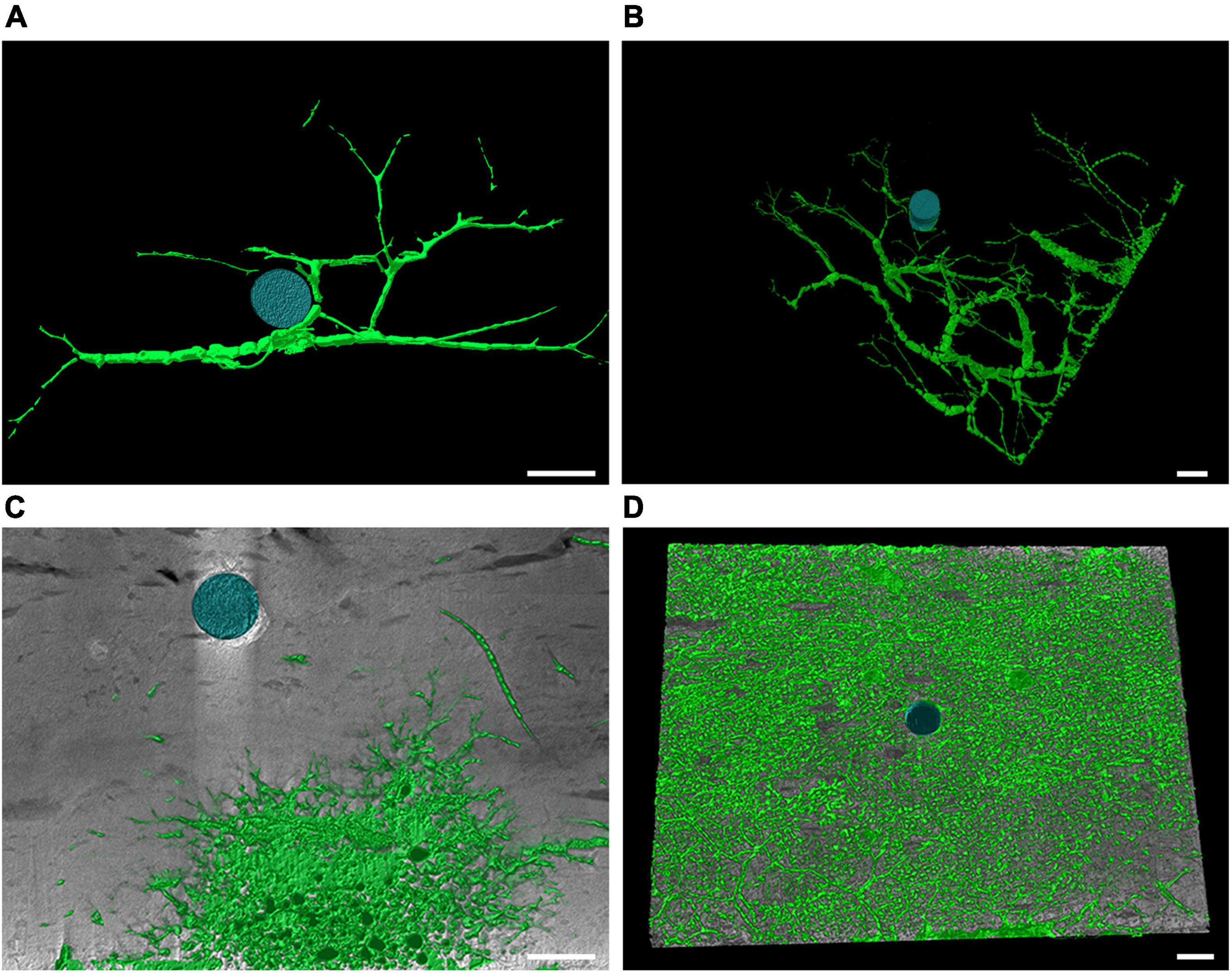
Figure 5. Computed tomography graphic reconstruction of Cliona viridis bioerosion traces in travertine tiles. (A) Entobia cateniformis at P213 after 3 years of exposure. (B) Entobia cateniformis at P204 after 3 years of exposure. (C) Entobia cf ovula at MR08 after 3 years of exposure. (D) Entobia cf laquei at P204 after 12 years of exposure. Scale bars are 1 cm long.
Cliona rhodensis Rützler and Bromley, 1981 was found only in 4 tiles (three at MR08 and one at P213). It was recognized as the trace maker of Entobia cf paradoxa Bromley and D’Alessandro, 1984 in one tile after 3 years at MR08 (Figure 6A) and in two after 12 years at P213 and MR08, and of Entobia cf magna Bromley and D’Alessandro, 1989 only in one 12-year-old tile at MR08 (Figure 6B). C. viridis and C. rhodensis were observed competing for the substrate in the same tile (Figure 6B).
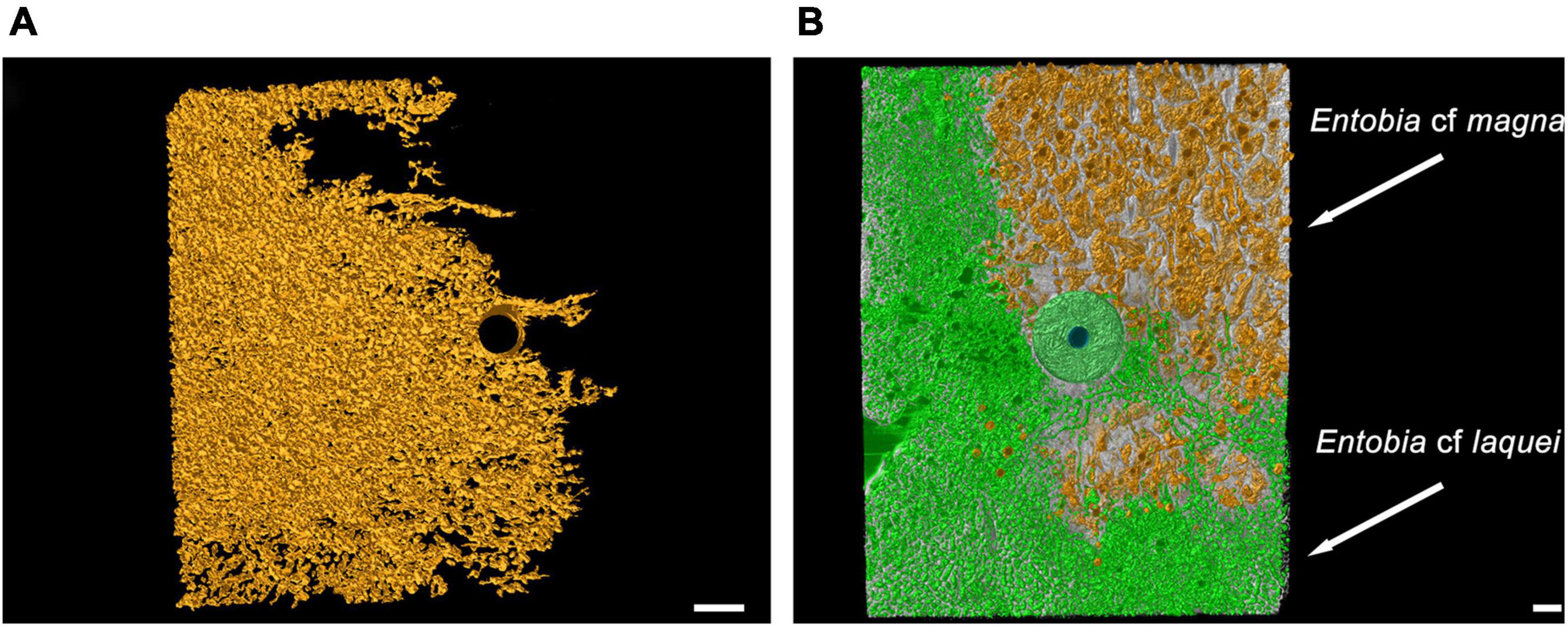
Figure 6. Computed tomography graphic reconstruction of boring sponges’ traces in travertine tiles left by C. rhodensis, in orange, and C. viridis, in green. (A) Entobia cf paradoxa at MR08 after 3 years of exposure. (B) Entobia cf magna and Entobia cf laquei at MR08 after 12 years. The steel washer used to anchor the tile to the seabed, and subsequently incorporated into the bioconstruction, is also visible. Scale bars are 1 cm long.
Traces of burrowing worms belonging to the ichnogenera Caulostrepsis Clarke, 1908 (Figure 7A), Trypanites Mägdefrau, 1932 (Figure 7B), Maeandropolydora Voigt, 1965 (Figure 7C) and Maeandropolydora cf decipiens Voigt, 1965 (Figures 7C,D) have been identified both in tiles exposed for 3 years and those for 12 years at all the study sites. The burrowing worms’ bioerosion traces penetrated from the top or bottom side into the core of the tile (Figures 7A–C). However, it was impossible to identify the worm species because no organisms were retrieved from the holes. The ichnogenera Caulostrepsis, Trypanites, and Maeandropolydora have been collectively categorized as “burrowing worm traces” for bioerosion balance.
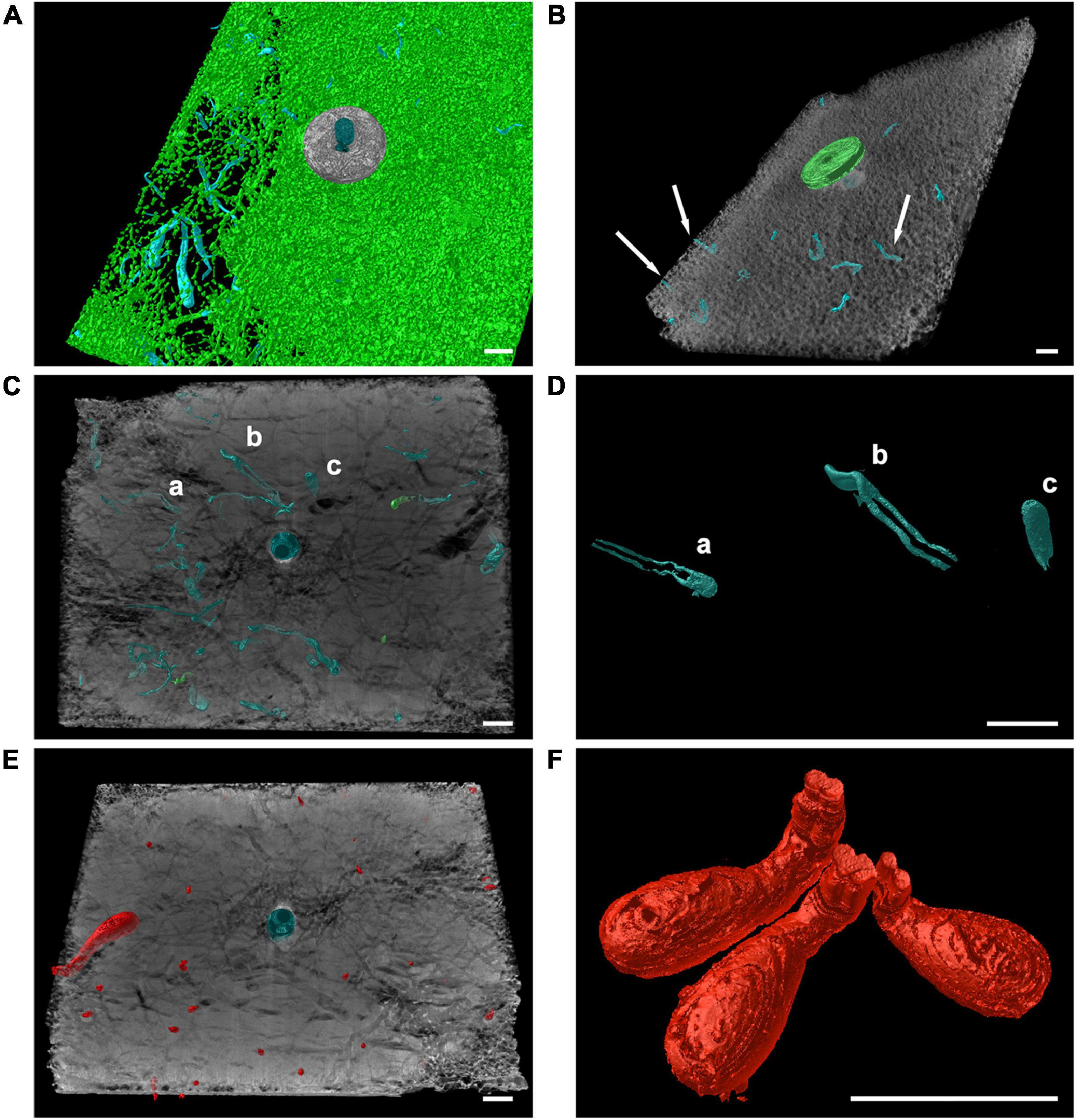
Figure 7. Computed tomography graphic reconstruction of burrowing worms and endolithic bivalves’ traces in travertine tiles. (A) Caulostrepsis traces, in blue, surrounded by Entobia cf laquei traces, in green, at MR08 after 12 years. (B) Burrowing worms traces’ (Trypanites traces indicated by arrows), in blue, at MR08 after 12 years. (C,D) Maeandropolydora traces (Maeandropolydora cf decipiens Voigt, 1965; a, b and c, in blue) at P213 after 12 years. (E) Gastrochaenolites traces at P213 after 12 years, in red. (F) Detail of Gastrochaenolites traces at P204 after 12 years. Scale bars are 1 cm long.
Clavate bivalve boring traces belonging to the ichnogenera Gastrochaenolites Leymerie, 1842 occur in all the tiles at different densities. The organism responsible for these boring was Rocellaria dubia (Pennant, 1777), whose 8-shaped siphonal apertures were observed in the topsides and on the edges of the tiles (Figures 7E,F).
Bioerosion varied among sites, with the exposure period and taxa involved (Figure 3B). Overall, the eroded limestone significantly increased from 3 to 12 exposure years, with no relevant differences among sites (Table 2B). On average, it was 0.80 ± 0.24 and 8.11 ± 2.06 kg m–2 at P204, after 3 and 12 years of exposure, respectively; 0.54 ± 0.19 and 9.79 ± 2.06 kg m–2 at P213; and 2.04 ± 0.63 and 13.26 ± 1.06 kg m–2 at MR08.
The most effective borer was the sponge C. viridis, which was able to dissolve 0.71 ± 0.25 kg m–2 after 3 years of exposure and 7.56 ± 2.23 kg m–2 after 12 years at P204; 0.41 ± 0.17, and 6.65 ± 2.96 kg m–2 at P213, respectively, after 3 and 12 years; and 1.11 ± 0.48 and 7.56 ± 2.90 kg m–2 at MR08. A significant increase was observed over time with no differences among sites (Table 2B). C. rhodensis dissolved 5.60 ± 3.75 kg m–2 after 12 years at MR08.
The limestone removed by burrowing worms significantly increased between years of exposure (Table 2B), and it was 0.004 ± 0.002 kg m–2 after 3 years of exposure and 0.025 ± 0.015 kg m–2 after 12 years at P204; 0.031 ± 0.018 and 0.073 ± 0.045 kg m–2 at P213; and 0.015 ± 0.004 and 0.049 ± 0.028 kg m–2 at MR08.
On average, R. dubia was significantly more effective in burrowing tiles at P204 and P213, with no difference over time (Table 2B). This bivalve was able to remove 0.083 ± 0.032 kg m–2 of limestone in 3 years and 0.416 ± 0.189 kg m–2 in 12 years at P204; 0.100 ± 0.032 kg m–2 and 0.149 ± 0.075 kg m–2 at P213; and 0.002 ± 0.008 kg m–2 and 0.037 ± 0.056 kg m–2 at MR08.
The bioerosion rate significantly increased over time and, in general, was significantly higher at MR08 compared to the other two sites (Table 3). After 3 years, it ranged from 0.179 ± 0.065 kg m–2 a–1 at P213 to 0.682 ± 0.208 kg m–2 a–1 at MR08, while after 12 years, it ranged from 0.676 ± 0.171 kg m–2 a–1 at P204 to 1.105 ± 0.088 kg m–2 a–1 at MR08 (Figure 4B).
Bioconstruction–Bioerosion Net Balance
The mean bioconstruction-bioerosion net balance apparently shows positive values after 3 years of exposure and negative values after 12 years at all study sites (Figure 4C). However, due to a large variability among tiles, according to the t-test (95% confidence interval), the net balance was significantly higher than zero only at P213 (p < 0.05) and MR08 (p < 0.01) after 3 years. All the other estimated mean balances were not significantly different from zero.
Discussion
In the northern Adriatic coralligenous reefs, the main builders are represented by ECRs, the bivalve Anomia ephippium, serpulid polychaetes, bryozoans, and barnacles. The present study shows that in the first 3 years, albeit sustained by different species compositions, total bioconstruction and its annual rate were almost the same at all sites. The differences between bioconstruction at the different sites become evident by analyzing these processes on a longer time scale. In 12 years, limestone deposited is much greater in the reef characterized by a higher abundance of calcareous algae (MR08) than in the other reefs. At MR08, the annual bioconstruction rate estimated in 12 years is equal to that estimated in 3 years, resulting in an almost constant rate over time. On the contrary, in the other two sites, the annual rates estimated in 12 years were significantly lower than those of the first 3 years. These different bioconstruction performances could be due to the differences in species assemblages inhabiting the study sites (Ponti et al., 2011). Indeed, the tiles deployed at MR08 after 12 years on the top side were totally covered by a thick layer of calcareous algae, mainly Lithophyllum incrustans Philippi, 1837, Mesophyllum sp. and Peyssonnelia sp. (Ponti et al., 2014). This result is consistent with the observations on other Mediterranean coralligenous reefs, whose formation and maintenance are mainly attributed to calcareous algae belonging to the genera Lithophyllum, Lithothamnion, Mesophyllum, Neogoniolithon, and Peyssonnelia (Garrabou and Ballesteros, 2000 and references therein). However, the growth of calcareous algae is complex as it can be characterized by periods of growth and shrinkage due to competitive interactions and disturbance events (Steneck, 1986).
The benthic assemblages at P204 and P213 were characterized by higher abundances of fast-growing species, including algal turfs, that may have outcompeted calcareous algae for space, limiting their settlement and growth, as often occurs in rocky coastal bottoms affected by high rates of sediment deposition (Airoldi, 2003). Space competitors include also serpulid polychaetes, which are important players in developing and maintaining the biogenic reefs (Laborel, 1987; Cocito, 2004; Casoli et al., 2016a). They act as secondary builders by shaping the seafloor and as binders by coating and fastening other tubes or partially filling crevices (Sanfilippo et al., 2013; Gravina et al., 2021). Indeed, serpulid polychaetes were abundant both on the top and bottom sides of the tiles; however, at MR08 after 12 years, on the top side, they were completely overgrown by calcareous algae.
Among the other builders, the solitary vermetid gastropods Thylacodes arenarius were only found after 12 years of exposure at MR08 on the top and bottom sides of the tiles. T. arenarius is a subtidal species that lives on hard substrates in dim light conditions and relatively calm waters (Calvo and Templado, 2005). Usually, individuals are covered by calcareous algae, sponges, bryozoans, and other organisms, and in fact here their shells were entirely overgrown by the ECRs.
Finally, at the site near the coast (P204), the proximity to the donor populations could have favored the settlement of fast calcifying organisms like the non-indigenous oyster, Magallana gigas (Bayne, 1999).
In the northern Adriatic coralligenous reefs, boring sponges represent the main cause of bioerosion. They can penetrate and erode limestone materials such as rocks, corals, and mollusk shells (Glynn and Manzello, 2015). They produce a vast system of branched and interconnected galleries in the substrate and at the same time communicate with the surface through numerous openings. The distribution, abundance, and erosion rates of boring sponges can be affected by environmental factors such as nutrients, temperature, turbidity, light, depth, and pH (e.g., Schönberg, 2008; Calcinai et al., 2011; Nava and Carballo, 2013; Silbiger et al., 2014; Marlow et al., 2018). The activities of boring sponges shape the coralligenous habitats by eroding the substrate and creating shelters for organisms (Cerrano et al., 2001; Calcinai et al., 2015), but at the same time they reduce the mechanical stability of the reef (Scott et al., 1988).
Cliona viridis was the dominant agent of erosion into the experimental tiles (up to 28% of the initial volume, on average over 12 years). This species is regarded as one the most abundant and effective borer in the Mediterranean Sea (Rosell et al., 1999; Bertolino et al., 2013), especially in the northern Adriatic mesophotic reefs (Ponti et al., 2011). Species from the Cliona viridis complex commonly displays high bioerosion rates, up to 28 kg m–2 a–1 (Schönberg et al., 2017 and references therein). It should be noted that the erosion produced by this species, although increased over time, was substantially the same at all sites. Although many boring sponges can be negatively affected by high sediment deposition and water turbidity, either by preventing settlement, hampering vital physiological function or controlling survivals (Nava and Carballo, 2013; Bell et al., 2015; Marlow et al., 2018), C. viridis seems to be tolerant to high levels of turbidity (<4 m visibility) and sediment deposition (Carballo et al., 1994, 1996; Carballo, 2006). Conversely, Cliona rhodensis was mainly found at MR08, where the water turbidity is on average lower than the other sites (Ponti et al., 2011). That is in agreement with the higher sensitive against turbidity and sedimentation of C. rhodensis than C. viridis (Carballo et al., 1994, 1996).
Burrowing worms, mainly spionids, sabellids, and cirratulids, are usually important in the early stage of erosion (Hutchings, 2008) and appeared to benefit from the substrate modifications provided by encrusting epibenthos (Casoli et al., 2019; Gravina et al., 2019). Although CT images revealed traces of boring polychaetes into the tiles, it was impossible to attribute the marks left to the responsible species. The mean limestone removed by burrowing worms increased with increasing time exposure and was almost the same at all sites. According to previous study, burrowing worms remove fewer amounts of limestone than sponges; however, by burrowing, they modify and weaken the substrate and, because of their short life span, facilitate the bioerosion of other organisms by leaving their burrows vacant for other borers (Hutchings et al., 1992).
Rocellaria dubia is one of the most common endolithic bivalves in the Mediterranean Sea. It prefers horizontal or slightly inclined surfaces and high sediment deposition (Schiaparelli et al., 2003). It can occur in high density both in the natural and artificial substrate (Schiaparelli et al., 2005; Casoli et al., 2016b). It is easily recognizable through its 8-shaped calcareous holes emerging from the substrate. Despite its wide diffusion and ecological importance, its biology and autecology are poorly known. Its planktonic larvae can settle in crevices or holes in the substrate and then efficiently excavate limestone (Morton et al., 2011; Ponti et al., 2015; Casoli et al., 2016b). R. dubia siphons have been observed both on the top and lateral side of the tiles. The mean limestone removed by R. dubia into the experimental tiles varies among the study sites. It was higher at P204 and P213, characterized by high water turbidity and sedimentation rates. That could be related to the tolerance of this species to high sedimentation rates, thanks to its long siphons protected by aragonitic siphonal tubes (Morton et al., 2011) and the possible competition with boring sponges at MR08. Noteworthy, the mean limestone removed did not increase by increasing the exposure time, confirming that R. dubia is a fast borer (Casoli et al., 2016b) and may have reached its maximum density in the first 3 years.
Considering the overall process, the mean bioerosion rate seems to increase over time, and it was higher at MR08. This may be due to the firm establishment of the sedimentation tolerant Cliona viridis boring sponge at all the sites, while at MR08 it may have been further promoted by less sedimentation. This has provided the boring sponge C. rhodensis with suitable habitat. Moreover, the higher abundance of calcareous algae has in turn provided an additional substrate for long-term bioerosion.
In this field experiment, bioconstruction has overcome the bioerosion processes only in the short term (3 years) and only where the calcareous algae find better conditions for settlement and growth. In the relatively long term (12 years), bioerosion equals the bioconstruction processes at all study sites, with a substantially null net result in terms of limestone budget. Although the external volume of the concretion may increase, its interior gradually empties, and the lower structural resistance could lead to subsequent collapses and compactions.
These observations lead to hypothesize in the investigated northern Adriatic coralligenous reefs a steady state, resulting from the net balance between bioconstruction and bioerosion, regardless by their differences in benthic assemblages. However, this result must be taken with caution due to the high variability observed among the individual experimental units, which are small in volume, and the relative shortness of the study period, which, although long for a field experiment, is not comparable with life span of these reefs, estimated in 5–7,000 years (Gordini et al., 2012; Tosi et al., 2017). Furthermore, due to the spatial and temporal scale adopted in the experiment, the results achieved cannot consider the effect of disturbances such as the worsening of environmental conditions or mechanical damage to the reefs caused by trawling that, with some gear, can displace and crumble square meters of reef in seconds.
The benthic assemblages of the northern Adriatic coralligenous reefs are characterized by high spatial heterogeneity and relatively low temporal variability (Ponti et al., 2011). Multiple interacting abiotic and biotic factors are affecting the structure of these communities over space and time. The spatial distribution of the three main types of benthic assemblages found has been related to geographic and environmental variables, like distance from the coast, depth, water turbidity, and sediment load (Ponti et al., 2011; Curiel et al., 2012; Falace et al., 2015). However, settlement and recruitment processes, related to the reproduction strategies, dispersive capacity, and distance of the donor populations, play a fundamental role in structuring these communities (Fava et al., 2016). Moreover, these reefs are impacted by various anthropogenic disturbances like dystrophic crises mostly related to eutrophication (Kollmann and Stachowitsch, 2001; Zuschin and Stachowitsch, 2009; Tomasovych et al., 2017), and intense trawling activities and littering (Pranovi et al., 2000; Melli et al., 2017). Ultimately, the long-time persistence and development of these biogenic reefs rely on the dynamics between bioconstruction and bioerosion processes. When the activities of boring organisms exceed the actions of builders, these reefs risk a shift from builder-dominant to a boring-dominant, leading to an unbalance of net accretion, as already occurred in many tropical coral reefs (e.g., Rose and Risk, 1985; Ward-Paige et al., 2005; Marulanda-Gómez et al., 2017).
The present study provides the first baseline knowledge on the bioerosion and bioconstruction dynamics over space and time in the northern Adriatic Sea coralligenous reefs. This was achieved by coupling a field experiment with high-resolution computed tomography, which allows to investigate the interior of the substrates in a non-destructive way offering new opportunities and novel perspectives on ecological researches (Gutierrez et al., 2018). The method applied is very promising, however there is room for improvement. Manual segmentation of CT 3D models can be time-consuming and challenging, and semi/automated methods, like those realized by Titschack et al. (2015) and Färber et al. (2016), should be further implemented to separate and distinguish interconnected empty spaces putatively due to different species based on the corresponding traces. The procedure could be achieved using supervised machine learning algorithms and methods for shape recognition and segmentation (Rossi et al., 2021).
The experimental material adopted (i.e., travertine) can influence the bioconstruction and bioerosion rates (Calcinai et al., 2008). Travertine is a porous limestone characterized by natural fractures, which made the experiment more realistic. However, as suggested by Silbiger et al. (2016), an initial scan of the experimental tiles would have been preferable to quantify the volume of the natural fractures and their role as a possible preferential corridor for borer organisms.
The ichnotaxonomy approach, originally developed as a hierarchical classification system for fossil traces, poses some limits when applied to investigate current bioerosion processes. Ichnogenera and ichnospecies identification are often tricky because the pattern of erosion morphology may have been affected by the microtexture of the calcareous substrates (e.g., Calcinai, 2004; Calcinai et al., 2008), and the traces made by different organisms were often merged into a network of connected cavities, a likely consequence of several generations of traces of endoliths (Färber et al., 2016).
However, combining CT and morphological taxonomy on sampled organisms made it possible to attribute different bioerosion traces (ichnotaxa) to the boring species. Six out of the nine identified ichnotaxa were attributed to 3 living species. It was acknowledged that Cliona viridis and C. rhodensis could produce different traces, depending on their ontogenetic phase.
Conclusion
Manipulative field experiments using artificial substrates (e.g., travertine) that resemble natural ones, combined with CT technology, allow exploring the mechanisms underlying the formation and maintenance of biogenic reefs. However, much of these processes remain to be investigated, especially in coralligenous and other temperate reefs typologies. With an increasing number of coralligenous reefs being subjected to anthropogenic disturbances in the Mediterranean Sea (Micheli et al., 2013; Bevilacqua et al., 2018), the balance between bioconstruction and bioerosion may be changed in favor of erosion with severe consequences for their conservation.
The implementation of experimental and innovative approaches could help the design of appropriate conservation strategies of coralligenous habitats in the Mediterranean Sea that are characterized by long-lived species, high spatial variability, and limited temporal changes (Teixidó et al., 2011) and are among the most vulnerable habitats (Ponti et al., 2021) to the current increases of anthropogenic disturbances and climate change.
Data Availability Statement
The raw data supporting the conclusions of this article will be made available by the authors, without undue reservation.
Author Contributions
ET and MP: conceptualization, formal analysis, and writing original draft. ET, MP, MB, and BC: investigation. MA, MM, and AS: resources. ET: visualization. MA, MP, and ET: funding acquisition. All authors: writing—review and editing.
Funding
This work has been supported by Research Projects of National Interest (PRIN), funded by the Italian Ministry of University and Research: “Reef ReseArcH—Resistance and Resilience of Adriatic Mesophotic Biogenic Habitats to Human and Climate Change Threats” (Call 2015; Prot. 2015J922E4; 2017–2020) and it was finalized during the participation of MP to the EU funded project “CoAStal and marine waters integrated monitoring systems for ecosystems proteCtion AnD managemEnt” (CASCADE; Interreg V-A Italy-Croatia CBC Programme 2014–2020, ID Number 10255941). This work was part of ET’s Ph.D. thesis at the University of Bologna. ET benefited from a Marco Polo fellowship granted by the University of Bologna.
Conflict of Interest
The authors declare that the research was conducted in the absence of any commercial or financial relationships that could be construed as a potential conflict of interest.
Publisher’s Note
All claims expressed in this article are solely those of the authors and do not necessarily represent those of their affiliated organizations, or those of the publisher, the editors and the reviewers. Any product that may be evaluated in this article, or claim that may be made by its manufacturer, is not guaranteed or endorsed by the publisher.
Acknowledgments
We would like to thank Francesco Colosio for helping in the setup of the experiment in 2005, Azzurra Bastari and Daniela Pica for their help in spicules and bryozoan identification, respectively. We kindly acknowledge the two reviewers and the editor for the constructive comments that improved the quality of the manuscript.
Supplementary Material
The Supplementary Material for this article can be found online at: https://www.frontiersin.org/articles/10.3389/fmars.2021.790869/full#supplementary-material
Footnotes
References
Airoldi, L. (2003). The effects of sedimentation on rocky coast assemblages. Oceanogr. Mar. Biol. Annu. Rev. 41, 161–236.
Balata, D., Piazzi, L., Cecchi, E., and Cinelli, F. (2005). Variability of Mediterranean coralligenous assemblages subject to local variation in sediment deposition. Mar. Environ. Res. 60, 403–421. doi: 10.1016/j.marenvres.2004.12.005
Ballesteros, E. (2006). Mediterranean coralligenous assemblages: a synthesis of present knowledge. Oceanogr. Mar. Biol. Annu. Rev. 44, 123–195. doi: 10.1201/9781420006391
Bavestrello, G., Cerrano, C., Zanzi, D., and CattaneoVietti, R. (1997). Damage by fishing activities in the gorgonian coral Paramuricea clavata in the Ligurian Sea. Aquat. Conserv. 7, 253–262. doi: 10.1002/(sici)1099-0755(199709)7:3<253::aid-aqc243<3.0.co;2-1
Bayne, B. L. (1999). Physiological components of growth differences between individual oysters (Crassostrea gigas) and a comparison with Saccostrea commercialis. Physiol. Biochem. Zool. 72, 705–713. doi: 10.1086/316714
Bell, J. J., McGrath, E., Biggerstaff, A., Bates, T., Cardenas, C. A., and Bennett, H. (2015). Global conservation status of sponges. Conserv. Biol. 29, 42–53. doi: 10.1111/cobi.12447
Bertolino, M., Cerrano, C., Bavestrello, G., Carella, M., Pansini, M., and Calcinai, B. (2013). Diversity of Porifera in the Mediterranean coralligenous accretions, with description of a new species. Zookeys 336, 1–37. doi: 10.3897/zookeys.336.5139
Bevilacqua, S., Guarnieri, G., Farella, G., Terlizzi, A., and Fraschetti, S. (2018). A regional assessment of cumulative impact mapping on Mediterranean coralligenous outcrops. Sci. Rep. 8:1757. doi: 10.1038/s41598-018-20297-1
Brancaccio, R., Bettuzzi, M., Casali, F., Morigi, M. P., Levi, G., Gallo, A., et al. (2011). Real-time reconstruction for 3-D CT applied to large objects of cultural heritage. IEEE Trans. Nucl. Sci. 58, 1864–1871. doi: 10.1109/tns.2011.2158850
Bromley, R. G., and D’Alessandro, A. (1983). Bioerosion in the Pleistocene of southern Italy: Ichnogenera Caulostrepsis and Maeandropolydora. Riv. Ital. Paleontol. Stratigr. 89, 283–309.
Bromley, R. G., and D’Alessandro, A. (1984). The ichnogenus Entobia from the Miocene, Pliocene and Pleistocene of southern Italy. Riv. Ital. Paleontol. Stratigr. 90, 227–296.
Bromley, R. G., and D’Alessandro, A. (1989). Ichnological study of shallow marine endolithic sponges from the Italian coast. Riv. Ital. Paleontol. Stratigr. 95, 279–314.
Bromley, R. G., and D’Alessandro, A. (1990). Comparative analysis of bioerosion in deep and shallow water, Pliocene to recent, Mediterranean Sea. Ichnos 1, 43–49. doi: 10.1080/10420949009386330
Bromley, R. G., Hanken, N. M., and Asgaard, U. (1990). Shallow marine bioerosion: preliminary results of an experimental study. Bull. Geol. Soc. Den. 38, 85–99. doi: 10.37570/bgsd-1990-38-09
Calcinai, B. (2004). Bioerosion micro-patterns as diagnostic characteristics in boring sponges. Boll. Mus. Ist. Biol. Univ. Genova 68, 229–238.
Calcinai, B., Bavestrello, G., Cerrano, C., and Gaggero, L. (2008). “Substratum microtexture affects the boring pattern of Cliona albimarginata (Clionaidae, Demospongiae),” in Current Developments in Bioerosion, eds M. Wisshak and L. Tapanila (Berlin: Springer-Verlag), 203–211.
Calcinai, B., Bavestrello, G., Cuttone, G., and Cerrano, C. (2011). Excavating sponges from the Adriatic Sea: description of Cliona adriatica sp. nov. (Demospongiae: Clionaidae) and estimation of its boring activity. J. Mar. Biol. Assoc. U. K. 91, 339–346. doi: 10.1017/s0025315410001050
Calcinai, B., Bertolino, M., Bavestrello, G., Montori, S., Mori, M., Pica, D., et al. (2015). Comparison between the sponge fauna living outside and inside the coralligenous bioconstruction: a quantitative approach. Mediterr. Mar. Sci. 16, 413–418. doi: 10.12681/mms.900
Calvo, M., and Templado, J. (2005). Reproduction and sex reversal of the solitary vermetid gastropod Serpulorbis arenarius. Mar. Biol. 146, 963–973. doi: 10.1007/s00227-004-1490-8
Carballo, J. L. (2006). Effect of natural sedimentation on the structure of tropical rocky sponge assemblages. Ecoscience 13, 119–130.
Carballo, J. L., Naranjo, S. A., and Garcia-Gomez, J. C. (1996). Use of marine sponges as stress indicators in marine ecosystems at Algeciras Bay (southern Iberian Peninsula). Mar. Ecol. Prog. Ser. 135, 109–122. doi: 10.3354/meps135109
Carballo, J. L., Sanchez-Moyano, J. E., and Garcia-Gomez, J. C. (1994). Taxonomic and ecological remarks on boring sponges (Clionidae) from the Straits of Gibraltar (southern Spain): tentative bioindicators? Zool. J. Linn. Soc. 112, 407–424. doi: 10.1111/j.1096-3642.1994.tb00329.x
Casoli, E., Bonifazi, A., Ardizzone, G., and Gravina, M. F. (2016a). How algae influence sessile marine organisms: the tube worms case of study. Estuar. Coast. Shelf Sci. 178, 12–20. doi: 10.1016/j.ecss.2016.05.017
Casoli, E., Ricci, S., Antonelli, F., Sacco Perasso, C., Belluscio, A., and Ardizzone, G. (2016b). Impact and colonization dynamics of the bivalve Rocellaria dubia on limestone experimental panels in the submerged Roman city of Baiae (Naples, Italy). Int. Biodeterior. Biodegrad. 108, 9–15. doi: 10.1016/j.ibiod.2015.11.026
Casoli, E., Ricci, S., Antonelli, F., Perasso, C. S., Ardizzone, G., and Gravina, M. F. (2019). Colonization dynamic on experimental limestone substrata: the role of encrusting epilithics favouring boring polychaetes. Hydrobiologia 842, 101–112. doi: 10.1007/s10750-019-04028-9
Cerrano, C., Bavestrello, G., Bianchi, C. N., Calcinai, B., Cattaneo-Vietti, R., Morri, C., et al. (2001). “The role of sponge bioerosion in Mediterranean coralligenous accretion,” in Mediterranean Ecosystems, eds F. Faranda, L. Guglielmo, and G. Spezie (Milan: Springer), 235–240. doi: 10.1007/978-88-470-2105-1_30
Chentout, M., Alloul, B., Rezouk, A., and Belhai, D. (2015). Experimental study to evaluate the effect of travertine structure on the physical and mechanical properties of the material. Arab. J. Geosci. 8, 8975–8985. doi: 10.1007/s12517-015-1910-8
Claudet, J., and Fraschetti, S. (2010). Human-driven impacts on marine habitats: a regional meta-analysis in the Mediterranean Sea. Biol. Conserv. 143, 2195–2206. doi: 10.1016/j.biocon.2010.06.004
Cocito, S. (2004). Bioconstruction and biodiversity: their mutual influence. Sci. Mar. 68, 137–144. doi: 10.3989/SCIMAR.2004.68S1137
Coll, M., Piroddi, C., Albouy, C., Lasram, F. B., Cheung, W. W. L., Christensen, V., et al. (2012). The Mediterranean Sea under siege: spatial overlap between marine biodiversity, cumulative threats and marine reserves. Glob. Ecol. Biogeogr. 21, 465–480. doi: 10.1111/j.1466-8238.2011.00697.x
Coll, M., Piroddi, C., Steenbeek, J., Kaschner, K., Lasram, F. B., Aguzzi, J., et al. (2010). The biodiversity of the Mediterranean Sea: estimates, patterns, and threats. PLoS One 5:8. doi: 10.1371/journal.pone.0011842
Curiel, D., Falace, A., Bandelj, V., Kaleb, S., Solidoro, C., and Ballesteros, E. (2012). Species composition and spatial variability of macroalgal assemblages on biogenic reefs in the northern Adriatic Sea. Bot. Mar. 55, 625–638. doi: 10.1515/bot-2012-0166
Davidson, T. M., Altieri, A. H., Ruiz, G. M., and Torchin, M. E. (2018). Bioerosion in a changing world: a conceptual framework. Ecol. Lett. 21, 422–438. doi: 10.1111/ele.12899
de Caralt, S., and Cebrian, E. (2013). Impact of an invasive alga (Womersleyella setacea) on sponge assemblages: compromising the viability of future populations. Biol. Invasions 15, 1591–1600. doi: 10.1007/s10530-012-0394-7
De Groot, R. A. (1977). Boring sponges (Clionidae) and their trace fossils from the coast near Rovinj (Yugoslavia). Geol. Mijnb. 56, 168–181.
Escudier, R., Clementi, E., Omar, M., Cipollone, A., Pistoia, J., Aydogdu, A., et al. (2020). Data From: Mediterranean Sea Physical Reanalysis (CMEMS MED-Currents) (Version 1) Set. Copernicus Monitoring Environment Marine Service (CMEMS). doi: 10.25423/CMCC/MEDSEA_MULTIYEAR_PHY_006_004_E3R1
Falace, A., Kaleb, S., Curiel, D., Miotti, C., Galli, G., Querin, S., et al. (2015). Calcareous bio-concretions in the northern Adriatic Sea: habitat types, environmental factors that influence habitat distributions, and predictive modeling. PLoS One 10:e0140931. doi: 10.1371/journal.pone.0140931
Färber, C., Titschack, J., Schonberg, C. H. L., Ehrig, K., Boos, K., Baum, D., et al. (2016). Long-term macrobioerosion in the Mediterranean Sea assessed by micro-computed tomography. Biogeosciences 13, 3461–3474. doi: 10.5194/bg-13-3461-2016
Färber, C., Wisshak, M., Pyko, I., Bellou, N., and Freiwald, A. (2015). Effects of water depth, seasonal exposure, and substrate orientation on microbial bioerosion in the Ionian Sea (Eastern Mediterranean). PLoS One 10:e0126495. doi: 10.1371/journal.pone.0126495
Fava, F., Ponti, M., and Abbiati, M. (2016). Role of recruitment processes in structuring coralligenous benthic assemblages in the Northern Adriatic continental shelf. PLoS One 11:e0163494. doi: 10.1371/journal.pone.0163494
García-del-Cura, M. Á, Benavente, D., Martínez-Martínez, J., and Cueto, N. (2012). Sedimentary structures and physical properties of travertine and carbonate tufa building stone. Constr. Build. Mater. 28, 456–467. doi: 10.1016/j.conbuildmat.2011.08.042
Garrabou, J., and Ballesteros, E. (2000). Growth of Mesophyllum alternans and Lithophyllum frondosum (Corallinales, Rhodophyta) in the northwestern Mediterranean. Eur. J. Phycol. 35, 1–10. doi: 10.1080/09670260010001735571
Garrabou, J., Gómez-Gras, D., Ledoux, J.-B., Linares, C., Bensoussan, N., López-Sendino, P., et al. (2019). Collaborative database to track mass mortality events in the Mediterranean Sea. Front. Mar. Sci. 6:707. doi: 10.3389/fmars.2019.00707
Gennaro, P., and Piazzi, L. (2011). Synergism between two anthropic impacts: Caulerpa racemosa var. cylindracea invasion and seawater nutrient enrichment. Mar. Ecol. Prog. Ser. 427, 59–70. doi: 10.3354/meps09053
Gherardi, D. F. M., and Bosence, D. W. J. (2001). Composition and community structure of the coralline algal reefs from Atol das Rocas, South Atlantic, Brazil. Coral Reefs 19, 205–219. doi: 10.1007/s003380000100
Glasby, T. M., and Connell, S. D. (2001). Orientation and position of substrata have large effects on epibiotic assemblages. Mar. Ecol. Prog. Ser. 214, 127–135. doi: 10.3354/meps214127
Glynn, P. W., and Manzello, D. P. (2015). “Bioerosion and coral reef growth: a dynamic balance,” in Coral Reefs in the Anthropocene, ed. C. Birkeland (Dordrecht: Springer), 67–97. doi: 10.1007/978-94-017-7249-5_4
Gordini, E., Falace, A., Kaleb, S., Donda, F., Marocco, R., and Tunis, G. (2012). “Methane-related carbonate cementation of marine sediments and related macroalgal coralligenous assemblages in the northern Adriatic Sea,” in Seafloor Geomorphology as Benthic Habitat, eds P. T. Harris and E. K. Baker (Amsterdam: Elsevier Inc), 185–200. doi: 10.1016/b978-0-12-385140-6.00009-8
GOS Group (2021). Data From: Mediterranean Sea Reprocessed Remote Sensing Reflectances and Attenuation Coefficient at 490nm from Multi Satellite Observations. Global Ocean Satellite Monitoring and Marine Ecosystem Study Group (GOS) of the Italian National Research Council (CNR, Rome). E.U. Copernicus Marine Service Information. Available online at: https://resources.marine.copernicus.eu/product-detail/OCEANCOLOUR_MED_OPTICS_L4_NRT_OBSERVATIONS_009_039/INFORMATION
Grange, J. S., Rybarczyk, H., and Tribollet, A. (2015). The three steps of the carbonate biogenic dissolution process by microborers in coral reefs (New Caledonia). Environ. Sci. Pollut. R. 22, 13625–13637. doi: 10.1007/s11356-014-4069-z
Gravina, M. F., Antonelli, F., Sacco Perasso, C., Cesaretti, A., Casoli, E., and Ricci, S. (2019). The role of polychaetes in bioerosion ofsubmerged mosaic floors in the Underwater Archaeological Park of Baiae (Naples,Italy). Facies 65:19. doi: 10.1007/s10347-019-0563-6
Gravina, M. F., Pierri, C., Mercurio, M., Nonnis Marzano, C., and Giangrande, A. (2021). Polychaete diversity related to different mesophotic bioconstructions along the southeastern Italian coast. Diversity 13:239. doi: 10.3390/d13060239
Gutierrez, Y., Ott, D., Topperwien, M., Salditt, T., and Scherber, C. (2018). X-ray computed tomography and its potential in ecological research: a review of studies and optimization of specimen preparation. Ecol. Evol. 8, 7717–7732. doi: 10.1002/ece3.4149
Hinz, H. (2017). “Impact of bottom fishing on animal forests: science, conservation, and fisheries management,” in Marine Animal Forests: the Ecology of Benthic Biodiversity Hotspots, eds S. Rossi, L. Bramanti, A. Gori, and C. Orejas (Cham: Springer International Publishing), 1041–1059. doi: 10.1016/j.jenvman.2019.02.105
Hooper, J. N. A., and Van Soest, R. W. M. (eds) (2002). Systema Porifera. A guide to the Classification of Sponges. New York, NY: Kluwer Academic.
Hutchings, P. A. (2008). “Role of polychaetes in bioerosion of coral substrates,” in Current Developments in Bioerosion, eds M. Wisshak and L. Tapanila (Heidelberg: Springer), 249–264. doi: 10.1007/978-3-540-77598-0_13
Hutchings, P. A., Kiene, W. E., Cunningham, R. B., and Donnelly, C. (1992). Spatial and temporal patterns of non-colonial boring organisms (polychaetes, sipunculans and bivalve mollusks) in Porites at Lizard Island, Great Barrier Reef. Coral Reefs 11, 23–31. doi: 10.1007/bf00291931
Ingrosso, G., Abbiati, M., Badalamenti, F., Bavestrello, G., Belmonte, G., Cannas, R., et al. (2018). Mediterranean bioconstructions along the Italian coast. Adv. Mar. Biol. 79, 61–136. doi: 10.1016/bs.amb.2018.05.001
Kelly, S. R. A., and Bromley, R. G. (1984). Ichnological nomenclature of clavate borings. Palaentology 27, 793–807.
Kollmann, H., and Stachowitsch, M. (2001). Long-term changes in the benthos of the northern Adriatic Sea: a phototransect approach. Mar. Ecol. 22, 135–154. doi: 10.1046/j.1439-0485.2001.01761.x
Marlow, J., Schönberg, C. H. L., Davy, S. K., Haris, A., Jompa, J., and Bell, J. J. (2018). Bioeroding sponge assemblages: the importance of substrate availability and sediment. J. Mar. Biol. Assoc. U. K. 99, 343–358. doi: 10.1017/s0025315418000164
Martin, S., and Gattuso, J. P. (2009). Response of Mediterranean coralline algae to ocean acidification and elevated temperature. Glob. Change Biol. 15, 2089–2100. doi: 10.1111/j.1365-2486.2009.01874.x
Marulanda-Gómez, Á, López-Victoria, M., and Zea, S. (2017). Current status of coral takeover by an encrusting excavating sponge in a Caribbean reef. Mar. Ecol. 38:e12379. doi: 10.1111/maec.12379
Mateos-Molina, D., Palma, M., Ruiz-Valentin, I., Panagos, P., Garcia-Charton, J. A., and Ponti, M. (2015). Assessing consequences of land cover changes on sediment deliveries to coastal waters at regional level over the last two decades in the northwestern Mediterranean Sea. Ocean Coast. Manage. 116, 435–442. doi: 10.1016/j.ocecoaman.2015.09.003
Melli, V., Angiolillo, M., Ronchi, F., Canese, S., Giovanardi, O., Querin, S., et al. (2017). The first assessment of marine debris in a site of community importance in the north-western Adriatic Sea (Mediterranean Sea). Mar. Pollut. Bull. 114, 821–830. doi: 10.1016/j.marpolbul.2016.11.012
Micheli, F., Halpern, B. S., Walbridge, S., Ciriaco, S., Ferretti, F., Fraschetti, S., et al. (2013). Cumulative human impacts on Mediterranean and Black Sea marine ecosystems: assessing current pressures and opportunities. PLoS One 8:e79889. doi: 10.1371/journal.pone.0079889
Morton, B., Peharda, M., and Petric, M. (2011). Functional morphology of Rocellaria dubia (Bivalvia: Gastrochaenidae) with new interpretations of crypt formation and adventitious tube construction, and a discussion of evolution within the family. Biol. J. Linn. Soc. 104, 786–804. doi: 10.1111/j.1095-8312.2011.01763.x
Nava, H., and Carballo, J. L. (2013). Environmental factors shaping boring sponge assemblages at Mexican Pacific coral reefs. Mar. Ecol. Evol. Persp. 34, 269–279. doi: 10.1111/maec.12012
Neumann, A. C. (1966). Observations on coastal erosion in Bermuda and measurements of boring rate of sponge Cliona lampa. Limnol. Oceanogr. 11, 92–108. doi: 10.4319/lo.1966.11.1.0092
Piazzi, L., Atzori, F., Cadoni, N., Cinti, M. F., Frau, F., and Ceccherelli, G. (2018). Benthic mucilage blooms threaten coralligenous reefs. Mar. Environ. Res. 140, 145–151. doi: 10.1016/j.marenvres.2018.06.011
Piazzi, L., and Balata, D. (2008). The spread of Caulerpa racemosa var. cylindracea in the Mediterranean Sea: an example of how biological invasions can influence beta diversity. Mar. Environ. Res. 65, 50–61. doi: 10.1016/j.marenvres.2007.07.002
Ponti, M. (2020a). Data From: Single-Beam Bathymetry Data (ESRI ASCII Grid Format) of Selected Mesophotic Biogenic Reefs from the Northern Adriatic Sea. Integrated Earth Data Applications (IEDA). doi: 10.26022/IEDA/329803
Ponti, M. (2020b). Data From: Single-Beam Bathymetry Data (GeoTIFF Image Format) of Selected Mesophotic Biogenic Reefs from the Northern Adriatic Sea. Integrated Earth Data Applications (IEDA). doi: 10.26022/IEDA/329814
Ponti, M., Falace, A., Rindi, F., Fava, F., Kaleb, S., and Abbiati, M. (2014). “Beta diversity patterns in northern Adriatic coralligenous outcrops,” in Proceedings of the 2nd Mediterranean Symposium on the Conservation of the Coralligenous and Other Calcareous Bio-Concretions, eds C. Bouafif, H. Langar, and A. Ouerghi (Portorož: UNEP/MAP-RAC/SPA).
Ponti, M., Fava, F., and Abbiati, M. (2011). Spatial-temporal variability of epibenthic assemblages on subtidal biogenic reefs in the northern Adriatic Sea. Mar. Biol. 158, 1447–1459. doi: 10.1007/s00227-011-1661-3
Ponti, M., Fava, F., Perlini, R. A., Giovanardi, O., and Abbiati, M. (2015). Benthic assemblages on artificial reefs in the northwestern Adriatic Sea: does structure type and age matter? Mar. Environ. Res. 104, 10–19. doi: 10.1016/j.marenvres.2014.12.004
Ponti, M., Linares, C., Cerrano, C., Rodolfo-Metalpa, R., and Hoeksema, B. W. (2021). Editorial: biogenic reefs at risk: facing globally widespread local threats and their interaction with climate change. Front. Mar. Sci. 8:793038. doi: 10.3389/fmars.2021.793038
Pranovi, F., Raicevich, S., Franceschini, G., Farrace, M. G., and Giovanardi, O. (2000). Rapido trawling in the northern Adriatic Sea: effects on benthic communities in an experimental area. ICES J. Mar. Sci. 57, 517–524. doi: 10.1006/jmsc.2000.0708
R Core Team (2019). R: A Language and Environment for Statistical Computing Version 3.6.1 [Online]. Available: https://www.r-project.org/ (accessed September 5, 2019).
Risk, M. J., Sammarco, P. W., and Edinger, E. N. (1995). Bioerosion in Acropora across the continental-shelf of the Great Barrier reef. Coral Reefs 14, 79–86. doi: 10.1007/bf00303427
Rose, C. S., and Risk, M. J. (1985). Increase in Cliona delitrix infestation of Montastrea cavernosa heads on an organically polluted portion of the Grand Cayman fringing reef. Mar. Ecol. 6, 345–363. doi: 10.1111/j.1439-0485.1985.tb00142.x
Rosell, D., and Uriz, M. J. (2002). Excavating and endolithic sponge species (Porifera) from the Mediterranean: species descriptions and identification key. Org. Divers. Evol. 2, 55–86. doi: 10.1078/1439-6092-00033
Rosell, D., Uriz, M. J., and Martin, D. (1999). Infestation by excavating sponges on the oyster (Ostrea edulis) populations of the Blanes littoral zone (north-western Mediterranean Sea). J. Mar. Biol. Assoc. U. K. 79, 409–413. doi: 10.1017/s0025315498000526
Rossi, P., Ponti, M., Righi, S., Castagnetti, C., Simonini, R., Mancini, F., et al. (2021). Needs and gaps in optical underwater technologies and methods for the investigation of marine animal forest 3D-structural complexity. Front. Mar. Sci. 8:591292. doi: 10.3389/fmars.2021.591292
Ruthensteiner, B., and Heß, M. (2008). Embedding 3D models of biological specimens in PDF publications. Microsc. Res. Tech. 71, 778–786. doi: 10.1002/jemt.20618
Sandrini-Neto, L., and Camargo, M. G. (2012). GAD: An R Package for ANOVA Designs From General Principles [Online]. Available: https://CRAN.R-project.org/package=GAD (accessed September 5, 2019).
Sanfilippo, R., Vertino, A., Rosso, A., Beuck, L., Freiwald, A., and Taviani, M. (2013). Serpula aggregates and their role in deep-sea coral communities in the southern Adriatic Sea. Facies 59, 663–677. doi: 10.1007/s10347-012-0356-7
Schiaparelli, S., Franci, G., Albertelli, G., and Cattaneo-Vietti, R. (2003). Autoecologia del bivalve perforatore Gastrochaena dubia lungo la falesia del promontorio di Portofino. Atti Associazione Italiana Oceanologia e Limnologia 16, 29–36.
Schiaparelli, S., Franci, G., Albertelli, G., and Cattaneo-Vietti, R. (2005). A nondestructive method to evaluate population structure and bioerosion activity of the boring bivalve Gastrochaena dubia. J. Coast. Res. 21, 383–386. doi: 10.2112/03-0054.1
Schönberg, C. H. L., Fang, J. K.-H., and Carballo, J. L. (2017). “Bioeroding sponges and the future of coral reefs,” in Climate Change, Ocean Acidification and Sponges: Impacts Across Multiple Levels of Organization, eds J. L. Carballo and J. J. Bell (Berlin: Springer International Publishing), 179–372. doi: 10.1007/978-3-319-59008-0_7
Schönberg, C. H. L. (2008). “A history of sponge erosion: from past myths and hypotheses to recent approaches,” in Current Developments in Bioerosion, eds M. Wisshak and L. Tapanila (Berlin: Springer-Verlag), 165–202. doi: 10.1007/978-3-540-77598-0_9
Scott, P. J. B., Moser, K. A., and Risk, M. J. (1988). Bioerosion of concrete and limestone by marine organisms: a 13 year experiment from Jamaica. Mar. Pollut. Bull. 19, 219–222. doi: 10.1016/0025-326x(88)90234-2
Silbiger, N. J., Guadayol, O., Thomas, F. I. M., and Donahue, M. J. (2014). Reefs shift from net accretion to net erosion along a natural environmental gradient. Mar. Ecol. Prog. Ser. 515, 33–44. doi: 10.3354/meps10999
Silbiger, N. J., Guadayol, Ò, Thomas, F. I. M., and Donahue, M. J. (2016). A novel μCT analysis reveals different responses of bioerosion and secondary accretion to environmental variability. PLoS One 11:e0153058. doi: 10.1371/journal.pone.0153058
Steneck, R. S. (1986). The ecology of coralline algal crusts: convergent patterns and adaptative strategies. Annu. Rev. Ecol. Syst. 17, 273–303. doi: 10.1146/annurev.es.17.110186.001421
Teixido, N., Casas, E., Cebrian, E., Linares, C., and Garrabou, J. (2013). Impacts on coralligenous outcrop biodiversity of a dramatic coastal storm. PLoS One 8:e53742. doi: 10.1371/journal.pone.0053742
Teixidó, N., Garrabou, J., and Harmelin, J.-G. (2011). Low dynamics, high longevity and persistence of sessile structural species dwelling on Mediterranean coralligenous outcrops. PLoS One 6:e23744. doi: 10.1371/journal.pone.0023744
Teruzzi, A. Di Biagio, V., Feudale, L., Bolzon, G., Lazzari, P., Salon, S., et al. (2021). Data from: Mediterranean Sea Biogeochemical Reanalysis (CMEMSMED-Biogeochemistry, MedBFM3 System) (Version 1) Set. Copernicus Monitoring Environment Marine Service (CMEMS). doi: 10.25423/CMCC/MEDSEA_MULTIYEAR_BGC_006_008_MEDBFM3
Titschack, J., Baum, D., De Pol-Holz, R., López Correa, M., Forster, N., Flögel, S., et al. (2015). Aggradation and carbonate accumulation of Holocene Norwegian cold-water coral reefs. Sedimentology 62, 1873–1898. doi: 10.1111/sed.12206
Tomasovych, A., Gallmetzer, I., Haselmair, A., Kaufman, D. S., Vidovic, J., and Zuschin, M. (2017). Stratigraphic unmixing reveals repeated hypoxia events over the past 500 yr in the northern Adriatic Sea. Geology 45, 363–366. doi: 10.1130/g38676.1
Tosi, L., Zecchin, M., Franchi, F., Bergamasco, A., Da Lio, C., Baradello, L., et al. (2017). Paleochannel and beach-bar palimpsest topography as initial substrate for coralligenous buildups offshore Venice, Italy. Sci. Rep. 7:1321. doi: 10.1038/s41598-017-01483-z
Tribollet, A., and Golubic, S. (2005). Cross-shelf differences in the pattern and pace of bioerosion of experimental carbonate substrates exposed for 3 years on the northern Great Barrier Reef, Australia. Coral Reefs 24, 422–434. doi: 10.1007/s00338-005-0003-7
Tribollet, A., and Golubic, S. (2011). “Reef bioerosion: agents and processes,” in Coral Reefs: An Ecosystem in Transition, eds Z. Dubinsky and N. Stambler (Dordrecht: Springer), 435–449. doi: 10.1007/978-94-007-0114-4_25
Turicchia, E., Abbiati, M., Sweet, M., and Ponti, M. (2018). Mass mortality hits gorgonian forests at Montecristo Island. Dis. Aquat. Org. 131, 79–85. doi: 10.3354/dao03284
Virgilio, M., Airoldi, L., and Abbiati, M. (2006). Spatial and temporal variations of assemblages in a Mediterranean coralligenous reef and relationships with surface orientation. Coral Reefs 25, 265–272. doi: 10.1007/s00338-006-0100-2
Ward-Paige, C. A., Risk, M. J., Sherwood, O. A., and Jaap, W. C. (2005). Clionid sponge surveys on the Florida Reef Tract suggest land-based nutrient inputs. Mar. Pollut. Bull. 51, 570–579. doi: 10.1016/j.marpolbul.2005.04.006
Wisshak, M., Knaust, D., and Bertling, M. (2019). Bioerosion ichnotaxa: review and annotated list. Facies 65:39. doi: 10.1007/s10347-019-0561-8
Keywords: Mediterranean Sea, ecological processes, accretion, biogenic structures, recruitment panels, 3D visualization, climate change, mesophotic
Citation: Turicchia E, Abbiati M, Bettuzzi M, Calcinai B, Morigi MP, Summers AP and Ponti M (2022) Bioconstruction and Bioerosion in the Northern Adriatic Coralligenous Reefs Quantified by X-Ray Computed Tomography. Front. Mar. Sci. 8:790869. doi: 10.3389/fmars.2021.790869
Received: 07 October 2021; Accepted: 20 December 2021;
Published: 28 January 2022.
Edited by:
Cataldo Pierri, University of Bari Aldo Moro, ItalyReviewed by:
Maria Flavia Gravina, University of Rome “Tor Vergata”, ItalyChiara Lombardi, Italian National Agency for New Technologies, Energy and Sustainable Economic Development (ENEA), Italy
Copyright © 2022 Turicchia, Abbiati, Bettuzzi, Calcinai, Morigi, Summers and Ponti. This is an open-access article distributed under the terms of the Creative Commons Attribution License (CC BY). The use, distribution or reproduction in other forums is permitted, provided the original author(s) and the copyright owner(s) are credited and that the original publication in this journal is cited, in accordance with accepted academic practice. No use, distribution or reproduction is permitted which does not comply with these terms.
*Correspondence: Eva Turicchia, ZXZhLnR1cmljY2hpYTJAdW5pYm8uaXQ=
†ORCID: Eva Turicchia, orcid.org/0000-0002-8952-9028; Marco Abbiati, orcid.org/0000-0002-2426-4524; Matteo Bettuzzi, orcid.org/0000-0003-3464-6574; Barbara Calcinai, orcid.org/0000-0002-9004-0051; Maria Pia Morigi, orcid.org/0000-0001-5697-2325; Adam P. Summers, orcid.org/0000-0003-1930-9748; Massimo Ponti, orcid.org/0000-0002-6521-1330