- 1Sorbonne Université, CNRS, Laboratoire d’Océanographie de Villefranche (LOV), Villefranche-sur-Mer, France
- 2Institute for Marine and Antarctic Studies, University of Tasmania, Hobart, TAS, Australia
- 3Sorbonne Université, LOCEAN-IPSL, CNRS/IRD/MNHN, Paris, France
A recent paradigm explains that the downward pumping of biogenic carbon in the ocean is performed by the combined action of six different biological carbon pumps (BCPs): the biological gravitational pump, the physically driven pumps (Mixed Layer Pump, Eddy Subduction Pump and Large-scale Subduction Pump), and the animal-driven pumps (diurnal and seasonal vertical migrations of zooplankton and larger animals). Here, we propose a research community approach to implement the new paradigm through the integrated study of these BCPs in the World Ocean. The framework to investigate the BCPs combines measurements from different observational platforms, i.e., oceanographic ships, satellites, moorings, and robots (gliders, floats, and robotic surface vehicles such as wavegliders and saildrones). We describe the following aspects of the proposed research framework: variables and processes to be measured in both the euphotic and twilight zones for the different BCPs; spatial and temporal scales of occurrence of the various BCPs; selection of key regions for integrated studies of the BCPs; multi-platform observational strategies; and upscaling of results from regional observations to the global ocean using deterministic models combined with data assimilation and machine learning to make the most of the wealth of unique measurements. The proposed approach has the potential not only to bring together a large multidisciplinary community of researchers, but also to usher the community toward a new era of discoveries in ocean sciences.
Introduction: The Ocean Biological Carbon Pump, a Paradigm Shift
Until recently, the efforts of the research community studying the downward transport of biogenic carbon in the ocean were focused on the gravitational sinking of biogenic particles to depth. This mechanism was the central paradigm driving studies on the biological carbon pump (BCP), the concept of which was created by Volk and Hoffert (1985). However, a growing number of papers alluded to other pathways of biogenic carbon transfer to depth, including physical mechanisms of downward carbon injection and vertical migrations of organisms.
According to the recently formulated paradigm, the downward pumping of biogenic carbon in the ocean is performed by the combined action of six biological carbon pumps (BCPs). The word “biological” in BCP refers to the transfer of biogenic material to depth, mediated by either biological or physical processes. The six BCPs are known as the Biological Gravitational Pump, three physically mediated pumps (the Mixed Layer Pump, the Eddy Subduction Pump, the Large-scale Subduction Pump; the latter includes Ekman pumping), and two animal-mediated pumps (vertical migrations of zooplankton both diel and ontogenic) (Boyd et al., 2019). Fish, jellyfish and other animals larger than zooplankton also perform diel vertical migrations, and may thus contribute to the animal-mediated pumps (Pinti et al., 2021).
The mechanisms by which biogenic carbon is transferred to depth differ among the six BCPs. The gravitational BCP transfers carbon downwards by sinking biogenic particles. The physically driven BCPs transport particulate and dissolved organic carbon (POC and DOC, respectively) and particulate inorganic carbon (PIC; mostly calcareous plankton tests) downwards by physical injection and mixing of surface waters into the lower water column. This transport occurs over a large range of spatial and temporal scales, and is due to several physical mechanisms. In Boyd et al. (2019), the physically driven BCPs include the mixed-layer, large-scale subduction and eddy subduction pumps. The mixed-layer BCP refers to the seasonal detrainment of biogenic material following dynamic shifts in the depth of the mixed-layer (Dall’Olmo et al., 2016). The eddy subduction BCP refers to the downward advective transport by intense, small-scale vertical velocities (Omand et al., 2015). The large-scale subduction BCP primarily refers to the downward advective transport by weak, large-scale downward velocities associated with Ekman pumping (Levy et al., 2013). It should also be noted that the eddy and large-scale subduction pumps transport POC and DOC not only vertically but also laterally. The two vertical migration BCPs refer to the downward transport of carbon by migrating zooplankton, which egest (sinking fecal pellets), excrete (DOC) and respire (CO2) in the water column some of the carbon they had ingested in surface waters, but release most of it at depth where some of them also contribute carbon from their carcasses. Ultimately, most of the organic carbon transferred to depth is respired to CO2 in deep waters, and a small fraction is incorporated into the sediments. Some of the biogenic CO2 in the water column and the biogenic carbon in sediments are sequestered, i.e., stored out of contact with the atmosphere on climatically significant timescales (decades to centuries) (i.e., “Sequestration” in IPCC, 2019).
This new paradigm stresses two important aspects related to ocean carbon sequestration in the context of the progressive accumulation of anthropogenic carbon in the Earth System. First, it is considered that the vertical gradient of dissolved inorganic carbon (DIC) in the ocean (on average ∼200 μmol higher at 2000 m than at surface Keppler et al., 2020) is mainly due to the combined action of solubility pump (physical transfer of DIC from surface to depth, essentially at high latitudes in winter) and the BCPs (Levy et al., 2013), which both act against mixing processes that tend to homogenize DIC on the vertical (Volk and Hoffert, 1985; Gruber and Sarmiento, 2002). The recently recognized multiplicity of the BCPs and their combined contribution to carbon export – significantly higher than previously thought (Boyd et al., 2019) – stress the need to re-examine the contributions of the different mechanisms of downward transfer of organic carbon to the vertical DIC gradient. Second, while the BCPs do not specifically contribute to mitigate the ongoing increase in anthropogenic CO2, the biological and physical drivers of BCPs could be impacted by climate change (Bopp et al., 2013), which could result in positive feedbacks enhancing CO2 accumulation in the atmosphere (Kwon et al., 2009) and subsequently global warming. Hence, it is timely to obtain baseline knowledge on the drivers and magnitude of the different BCPs, against which to assess ongoing and future changes, and also to improve climate change models.
Here we propose a framework for an integrated observational approach of the six BCPs in the World Ocean based on the new paradigm of Boyd et al. (2019). Until recently, studies on each of the different BCPs have mainly been conducted separately. It was not recognized that these different BCPs were distinct components of a global mechanism of biogenic carbon pumping and sequestration in the ocean. Indeed, each BCP was generally investigated by specialists of a given discipline (e.g., biological, chemical or physical oceanographers, or marine biogeochemists or sedimentologists), using specific tools (i.e., observations at sea, satellite remote sensing, and/or modeling), based on observations made using one or a few of the existing observation platforms (i.e., satellites, research vessels, profiling Argo floats, gliders, and/or moorings). There was no conceptual framework to bring together the different approaches, observations and underlying processes.
The situation has changed with the recognition that the different mechanisms of biogenic matter export that had been investigated in isolation until then were complementary BCPs, each contributing in a distinctive way to downward carbon export and sequestration, often in different oceanic conditions (Boyd et al., 2019; Resplandy et al., 2019). At the same time, new methods were developed to investigate some of the BCPs, often using a time-series approach. One example is the application of measurements made with profiling BGC-Argo floats (called “floats” in the remainder of this paper, for simplicity) to quantify processes attributed to the biological gravitational pump (Estapa et al., 2017, 2019; Briggs et al., 2020; Moreau et al., 2020), the mixed layer pump (Dall’Olmo and Mork, 2014; Lacour et al., 2019), the eddy subduction pump (Llort et al., 2018), and diel vertical migrations of zooplankton (Haentjens et al., 2020). Another example is the use of gliders to address biological gravitational pump processes (Briggs et al., 2011) and investigate and quantify the eddy subduction pump (Omand et al., 2015; Stukel et al., 2017) as part of process-oriented studies. Most recently, multi-platform and multidisciplinary approaches (i.e., satellite and robotic observations associated with process studies) are being implemented in a few ocean regions. The goal is to develop in-depth comprehensive and mechanistic understanding of the link between upper layer phytoplankton production and export processes into the mesopelagic zone (e.g., Siegel et al., 2016; JETZON group Martin et al., 2020).
The above description of concurrent developments in concepts, measurements and observational platforms stresses the need to develop integrated observational approaches to quantify and better characterize the BCPs across the World Ocean, and also indicates that sensors and platforms needed to do so already exist (Chai et al., 2020) and complement the established and emerging applications based on satellite remote sensing (Brewin et al., 2021). The developing framework to investigate the BCPs should naturally combine measurements from multiple observational platforms, which presently include research vessels, satellites, instrumented moorings, and robots. The word “robots” used here and below refers to gliders and floats, and other “emerging” autonomous platforms such as unmanned surface vehicles (USVs: wavegliders and saildrones). The framework should involve biological, chemical and physical oceanographers, and marine biogeochemists and sedimentologists, combining field observations at sea, satellite remote sensing, and modeling.
Using combinations of platforms, researchers can now deploy sensors to make the observations required to simultaneously estimate key variables for the different BCPs, over relevant spatio-temporal scales. This paper proposes an integrated community observational framework to investigate the BCPs in the World Ocean to better quantify global estimates of carbon storage in the oceans and the underlying drivers of each BCP.
Biological Carbon Pump Variables and Processes to Be Measured
Carbon Flux Metrics, and Biogeochemically Relevant Variables, Stocks, Processes and Fluxes
The various BCPs have different mechanisms, and each operates over specific spatial and temporal domains. To intercompare these BCPs and estimate their potential contributions to the total carbon fluxes at regional and global scale, specific metrics have to be defined for characterizing the magnitude and fate of carbon fluxes in the water column. The most studied BCP to date has been the biological gravitational pump, for which metrics were defined and progressively improved (Buesseler and Boyd, 2009; Buesseler et al., 2020). Fluxes are expressed in units of mass per unit area and per unit time, and three key metrics are regularly used: BCP strength, i.e., magnitude of the POC flux at a reference export depth, which is often set at the fixed value of 100 m; export ratio, i.e., downward POC flux at the reference export depth expressed as a fraction of Net Primary Production (NPP); and transfer efficiency, i.e., ratio of the POC flux at a given depth below the reference export depth expressed as a fraction of the POC flux at the export depth. Changing the reference export depth can drastically change the meaning of metrics and, for example, it was proposed that carbon flux-related studies should use as reference, instead of fixed depth (100 m), the geographically variable lower boundary of the euphotic zone (EZ, where irradiance is 1% or 0.1% of the surface value) (Buesseler and Boyd, 2009; Buesseler et al., 2020). The EZ is the zone of de novo particle production, and its base marks the beginning of the twilight zone (TZ, where there is not enough light for photosynthesis, but enough for vision).
The metrics developed for the biological gravitational BCP cannot be used directly for the five other BCPs, which either inject water with its POC and DOC content to various depths in the water column or actively transport organic carbon in the bodies of vertically migrating organisms to intermediate or deeper waters. Below the depths reached by water injection or vertical migrations, the POC transferred by the five other BCPs becomes part of the biological gravitational BCP for the remainder of its downward journey. Hence, transfer efficiency measured below the injection or vertical migration depths include the effects of the five other pumps on the POC flux.
Beside addressing the flux of biogenic carbon exported downwards from surface waters and its attenuation during its transit through the water column, an ultimate goal of carbon cycle studies is the estimation of the amount of carbon that is sequestered, i.e., stored out of contact with the atmosphere on climatically significant timescales (decades to centuries). It is often considered that the carbon stored below the bottom of the TZ (which marks the beginning of the aphotic zone, where there is not enough light for vision) is sequestered. The reference depth for sequestration is often taken as 1000 m (e.g., Legendre and Rivkin, 2005). Other authors have recently argued that the maximum annual mixed layer depth is a more appropriate reference depth horizon to estimate the flux of POC that will be ultimately sequestered (Palevsky and Doney, 2021).
However, Siegel et al. (2021) showed that the time during which organic carbon remineralized to CO2 remains in the ocean progressively increases with depth, i.e., at any depth some of the CO2 leaks rapidly to the atmosphere and a fraction is stored for a long time in the ocean. In this study, the authors showed that a larger fraction of CO2 is stored in the ocean for a longer time as depth increases, and this varies according to location in the World Ocean. The study addressed direct (industrial) injection of CO2 at different depths, but the conclusions would be the same for organic carbon transferred by BCPs and remineralized to CO2 at these depths. Implementing this approach requires the estimation of the amount of organic carbon remineralized to CO2 as it moves downwards in the water column, which can be done using the values of attenuation of the downward flux of organic carbon described in section “The Euphotic Zone Variables and Processes, and Their Measurements.”
In the present study, we consider the stocks and fluxes of biogenic carbon within and through the EZ and the TZ. Most studies of the biological gravitational pump consider particles and aggregates ≤ 10 mm, but sinking organic carbon also includes larger materials such as carcasses of dead fish and marine mammals (blue whales can reach almost 30 m). It was proposed that whaling and fisheries have reduced the potential export of sinking carbon from the EZ (Pershing et al., 2010; Mariani et al., 2020), but we do not include carcasses in the stocks and fluxes of biogenic carbon within and through the EZ and the TZ considered in the present study. Table 1 summarizes the main variables and processes that should ideally be measured in the two zones of the water column.
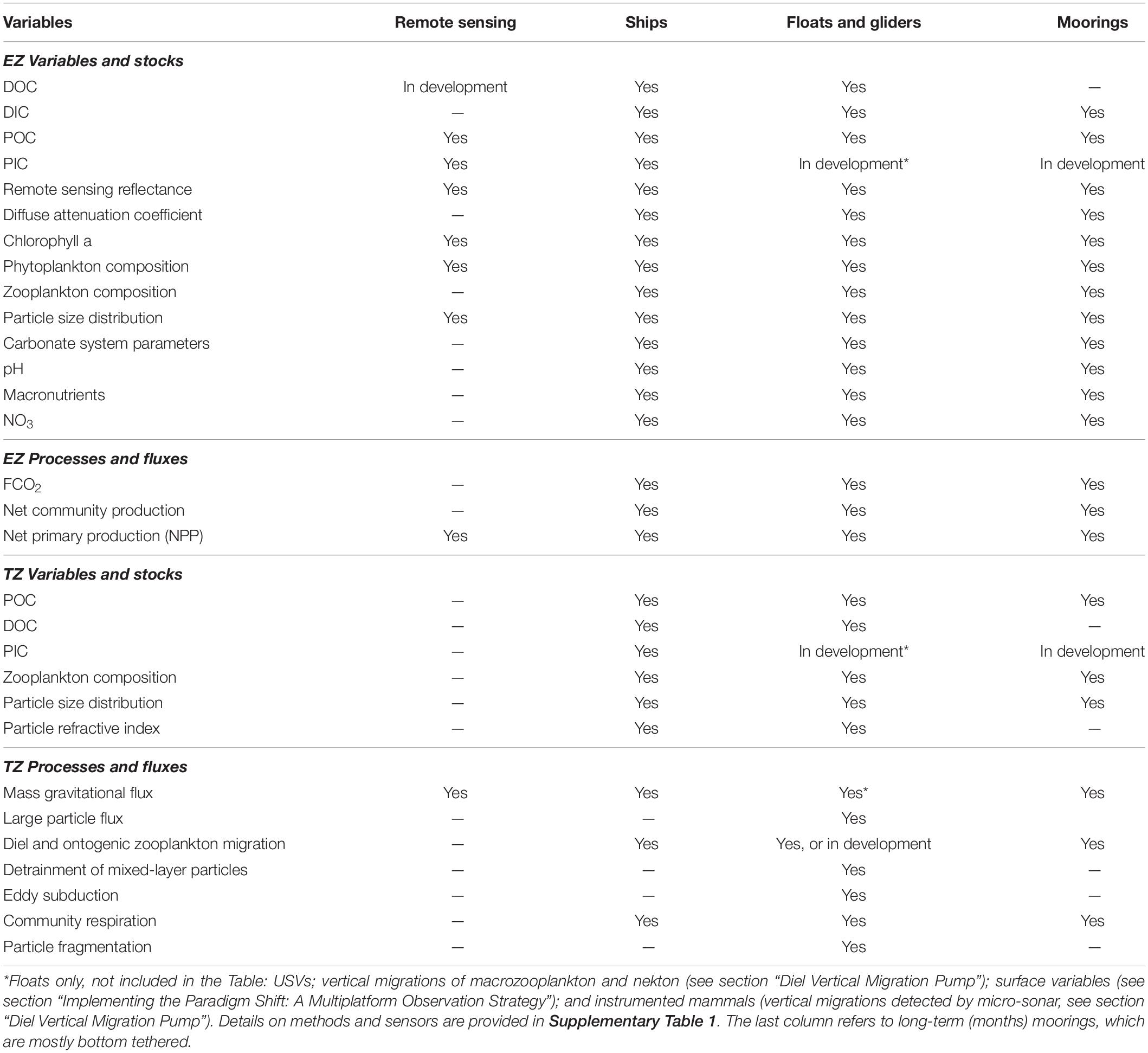
Table 1. Availability of methods and sensors for measuring or estimating biogeochemically relevant variables, stocks, processes and fluxes in the EZ and TZ potentially involved in the BCPs using different sampling platforms.
Supplementary Table 1 details the methods and sensors to measure or estimate the variables and processes described in this section. Most of the methods and sensors in the Table presently exist, and a few other are in development. In addition, all the variables in the Table have already been measured or estimated from at least one of the following observation platforms: satellites, ships, robots, moorings.
The Euphotic Zone Variables and Processes, and Their Measurements
The Euphotic Zone Variables and Processes
The key stocks to be systematically measured in the EZ primarily include POC, DOC and PIC. These stocks are the sources of the biogenic carbon exported by gravitational sinking and/or the physically or animal-meditated BCPs. The passive optics-based measurements important for BCP studies are the reflectance, which is a measurement of ocean color from the ratio of spectral upwelling radiance to spectral downwelling irradiance, and the diffuse attenuation coefficient, which quantifies the exponential decrease of light with depth as influenced by optically significant substances (phytoplankton, colored dissolved organic matter). These measurements are essential to retrieve key EZ variables both from space and in situ [e.g., Chlorophyll a (Chla), phytoplankton communities, see below], and will likely be improved in the near feature using hyperspectral radiometry on both satellites and in situ platforms (Uitz et al., 2015).
Other key variables are phytoplankton biomass, through its conventional proxy Chla, community composition, and particle size distribution. In carbon biogeochemical studies, phytoplankton community composition refers to broad groups whose abundances can be quantified based on optical properties or photosynthetic pigment composition, e.g., diatoms, coccolithophores, and other large and small phytoplankton (Uitz et al., 2006; Cetinic et al., 2015; Chase et al., 2020). Phytoplankton biomass, community composition and particle size distribution influence food web pathways, largely determine the paths of algal carbon through the food webs (Michaels and Silver, 1988; Legendre and Lefevre, 1995; Boyd and Newton, 1999; Leung et al., 2021), and are thus key drivers of the export ratio and the transfer efficiency of carbon from the EZ to the TZ. The broad phytoplankton groups can also be estimated from space (Alvain et al., 2005; Uitz et al., 2006; IOCCG, 2014; Bracher et al., 2017; El Hourany et al., 2019), and are among the key variables of ocean biogeochemical models, where such groups and broad zooplankton assemblages are called plankton functional types (Le Quéré et al., 2005; Aumont et al., 2015; Dutkiewicz et al., 2015). Particle size distribution can also be estimated from space (Kostadinov et al., 2009, 2010).
The concentrations of dissolved nitrate, oxygen and inorganic carbon (NO3, O2 and DIC, respectively) are essential complementary measurements for at least two reasons. First, NO3 is generally the main limiting nutrient, and consequently a key variable in biogeochemical models where N is often the “currency” used for variables and fluxes. Second, temporal variations in NO3, O2 and DIC at appropriate timescales can be used to estimate the carbon flux called Net Community Production (NCP, i.e., phytoplankton production minus pelagic community respiration).
The NCP is an essential quantity to be measured as it provides an estimate of how much organic carbon can potentially be exported from the EZ to the TZ. This estimate is required to assess whether the downward flux of organic carbon transferred from the EZ to the TZ by the combined six BCPs during one year or longer is in balance with the remineralization of organic carbon to CO2 within the TZ plus its export below the TZ into the aphotic zone. However, to potentially assess such a balance (or unbalance), the choice of the temporal scale over which NCP is integrated is critical. Given that the various BCPs have distinctive periods or seasons of occurrence (Boyd et al., 2019; Resplandy et al., 2019, see section “Spatial and Temporal Scales of Occurrence of the Various Pumps”), it is imperative to assess the NCP over at least one full year (annual NCP). This ensures that the variations linked to all BCPs are captured, and is the best way for closing the carbon budget.
Additionally, consecutive vertical Chla profiles can be combined in bio-optical models to estimate NPP (defined in section “Carbon Flux Metrics, and Biogeochemically Relevant Variables, Stocks, Processes and Fluxes”), which provides a broad framework for the analysis of BCPs and is sometimes essential for the estimation of key metrics (e.g., export ratio). Similarly, the CO2 fluxes derived from pCO2 measurements at the ocean-atmosphere interface, although not strictly required for the purpose of quantifying the BCPs, can potentially provide a complementary measurement to refine the BCPs’ roles in given ocean regions by amplifying or mitigating their impact on carbon sequestration according to the sign of the CO2 fluxes (i.e., sink vs source).
In addition, information on zooplankton abundance in the EZ at different times of the day (diel migrations) and across different seasons (ontogenic migrations) is essential to estimate the roles of the diel and ontogenic vertical migration pumps. The minimum information required to place bounds on the magnitudes of these BCPs are measurements of zooplankton biomass and/or abundance, if possible for different functional types such as gelatinous and crustacean zooplankton (e.g., Le Quéré et al., 2005).
The Euphotic Zone Measurements – From Satellites to Ships to Miniature Sensors
For more than a century, measurements of the above EZ variables and processes have been conducted on samples collected from oceanographic ships, and there is no need to review the methods of these measurements here as they are well known. In the last few decades, specialists of ocean remote sensing have also devised algorithms to estimate most of the carbon EZ variables from satellite information, except those pertaining to zooplankton although some recent studies have proposed approaches to derive zooplankton or animal biomass from satellite data. The latter approaches include direct estimation of the biomass of a highly concentrated swarm of colored zooplankton (Basedow et al., 2019), indirect assessment of the distribution of zooplankton biomass in the North Atlantic from satellite derived-indicators (Druon et al., 2019), and daily vertical migrations of ocean animals at the global scale using LIDAR (Behrenfeld et al., 2019).
Overall, satellite-based approaches are essential for upscaling observations of local processes associated with any of the BCPs to regional and even global scales. Furthermore, satellite observations are expected to be increasingly used in the future with the ongoing and planned diversification of satellite sensors, e.g., the Surface Water and Ocean Topography (SWOT) altimeter, which will allow to increase the resolution of sub-mesoscale processes associated with the eddy subduction BCP (Morrow et al., 2019); the Plankton, Aerosol, Cloud ocean Ecosystem (PACE) hyperspectral ocean color instrument, which may provide detailed phytoplankton functional types in ocean surface waters (Groom et al., 2019); and new sensors to be developed using LIDAR and polarimetry, which are expected to provide depth-resolved surface properties as well as information on particle morphology and chemical composition (Jamet et al., 2019).
In the last decades, electronic, optical and other sensors have progressively allowed the in situ estimation of most of the above EZ variables, including some pertaining to zooplankton, and these sensors have been deployed from oceanographic ships or on instrumented moorings. In the last two decades, miniature versions of several of these sensors were deployed on such autonomous platforms as gliders and floats. Autonomous platforms with miniature sensors provide the operational tools required for understanding and quantifying the different BCPs. Indeed, the deployment of multi-instrumented autonomous platforms can fill observational gaps over ranges of spatial and temporal scales that were out of reach until recently. They can in particular operate in areas (e.g., high latitudes) and at times of the year (e.g., winter) critical for the assessment of some BCPs (e.g., mixed layer and eddy subduction pumps) where and when other observation platforms can hardly be operated or used efficiently, such as ships (harsh sea conditions) or satellites (thick cloud cover). Additionally, because autonomous platforms are able to operate over long time periods (seasons to years), they could potentially assess the temporal succession in dominance of different BCPs and their possible interactions. Finally, long-term operation of autonomous platforms also ensures they will observe and record key events such as successive phases of vertical mixing or those of the spring phytoplankton bloom and their effects on BCPs, which events could be missed or be only partly observed by other platforms.
Given that the miniature sensors mounted on autonomous platforms are relatively recent and the uses of all of them are not necessarily known by the whole community, we briefly describe these sensors and their applications in BCP studies here (extensive reviews can be found in Roemmich et al., 2019; Chai et al., 2020; Claustre et al., 2020). The use of autonomous platforms equipped with miniature sensors to investigate the different BCPs is detailed in section “Implementing the Paradigm Shift: A Multiplatform Observation Strategy.”
Miniature active optical sensors with low electrical consumption measuring Chla fluorescence (FChla, fluorometer), the particle backscattering coefficient (bbp, backscattering meter) and the particle light attenuation coefficient (cp, transmissometer) have been deployed on floats, gliders and moorings, where they have provided comprehensive (long-term) time series of phytoplankton and POC stocks (Boss and Behrenfeld, 2010; Mignot et al., 2018). These remotely acquired measurements were not only complementary of those derived from satellite data, but also provided better understanding of the processes at play. For example, short-term phytoplankton blooms in the North Atlantic during winter (i.e., well before the “spring” bloom) were discovered by examining float time-series of Chla and bbp (Lacour et al., 2017) (see section “Toward a New Era of Discoveries”). Also, explicit links between bloom dynamics in the EZ and export processes to the TZ were evidenced using information on phytoplankton particles in the water column collected by gliders (Briggs et al., 2011) and subsequently floats (Briggs et al., 2020).
Furthermore, the combination of different active optical measurements, sometimes using complementary ship-acquired data, led to improved description of community composition (Cetinic et al., 2015), including the succession of blooms of various phytoplankton groups over several seasonal cycles (Rembauville et al., 2017) and the declining phases of coccolithophorid blooms (Xing et al., 2014; Terrats et al., 2020). When bbp or cp are measured with high vertical resolution, their variance in the EZ can be inverted into average particle size (Briggs et al., 2013), which provides a complementary and independent assessment of phytoplankton community structure as well as a surface indication of potential export to the TZ (Rembauville et al., 2017). Finally and taking advantage of birefringent properties of calcite (Guay and Bishop, 2002), on-going development of transmissometer with polarization detection capabilities should provide direct in situ quantification of PIC (Neukermanns, pers. comm.).
In recent years, several approaches have been developed to estimate the annual NCP using time series of chemical measurements related to phytoplankton dynamics (O2, pH, or NO3), which are generally acquired by floats. The principle of these methods is as follows: first, a mixed-layer physical model is developed in which O2, DIC or NO3 are considered as passive tracers (Plant et al., 2016; Briggs et al., 2018); and second, the model values are subtracted from those measured by the float, which encompass both physical and biological signals, thus providing the active biological signal. The estimation of NCP based on different variables produces different estimates, which can be used to constrain the NCP value (Briggs et al., 2018).
Based on empirical relationships between NCP and DOC determined from ship-based measurement at station PAPA in the North Pacific, (Bif and Hansell, 2019) were able to infer DOC time series from corresponding NO3 observations acquired by floats. Such DOC-inferred (virtual) measurements are of potential interest for better constraining total carbon export (i.e., POC + DOC) by physically mediated pumps (i.e., mixed layer or eddy subduction pumps; see section “The Twilight Zone Variables and Processes, and Their Measurement” below). In addition, the concentration of DOC in the EZ and its subsequent physical transport through to the TZ (by the mixed layer and eddy subduction pumps) can be estimated with approaches similar to those used for particles (Llort et al., 2018; Lacour et al., 2019), but it is not possible to estimate DOC in the TZ at present.
Finally, in the same way as used to infer DOC (Bif and Hansell, 2019), artificial intelligence methods combine P, T, S, and O2 measured by floats (or any other platform) to accurately predict vertical profiles of seven “virtual” variables. These are three macronutrients (NO3, PO4, and Si(OH)4) and the four parameters of the carbonate system (pCO2, pH, alkalinity, and DIC) (Sauzède et al., 2017; Bittig et al., 2018). Temporal variations in virtual variables could be used to estimate processes in both the EZ and the TZ.
The Twilight Zone Variables and Processes, and Their Measurement
In the TZ, two main types of measurements are needed, the first is required to improve the understanding of mechanisms, and the second to help constrain the vertical budgets of chemical elements, primarily carbon. Variables and processes of the first type are used to characterize the various pumps that transfer POC, PIC and DOC from the EZ down to different depth horizons in the TZ and below (see sections “Gravitational Pump” to “Seasonal Migration Pump”), and those of the second type, essentially related to the biological gravitational pump, are used to characterize the mesopelagic particles and to constrain the processes that determine the vertical attenuation of the particle flux within the EZ (see section “Characterization of Mesopelagic Particles”). Vertical attenuation generally refers to the decrease in the total amount of sinking POC with increasing depth.
Table 2 summarizes the range of depths potentially reached by the POC and DOC transferred from the EZ to depth by each of the six BCPs. The Table shows that the six pumps contribute to transfer organic particles to depth, but only the physical injection pumps also transfer dissolved organic compounds from EZ waters, where they are produced by phytoplankton and the food web. It is assumed in Table 2 that each of the three physically driven pumps will inject both POC and DOC from the EZ to the TZ, whereas only POC will be carried downwards by the biological gravitational and zooplankton migration pumps. It is also known that the remineralization of POC and DOC at greater depths releases CO2 in the water column that will remain in the ocean for longer average times before escaping to the atmosphere (Siegel et al., 2021). As explained in Section 1, the long-term storage of CO2 on climatically significant timescales (decadal to century) is called carbon sequestration, and Siegel et al. (2021) show the fraction of sequestered CO2 at different depths depends on the conditions of vertical mixing and circulation.
Table 2 shows that the biological gravitational pump transfers POC to great depths, although the food web respires to CO2 most of the POC sinking from the EZ during its downward transit. The three physical injection pumps and the two vertical migration pumps transfer POC to different depths in the TZ, below which this POC continues its downward journey as part of the biological gravitational pump. Because the transfer of POC by the five non-gravitational pumps is rapid, it bypasses remineralization in the upper water column above the delivery depth of each pump. This depth may vary considerably among regions and seasonally, and it is thus important to characterize it for the five pumps in order to obtain realistic carbon budgets in the TZ. This also stresses the importance of characterizing mesopelagic particles in the TZ. It is generally assumed that most of the DOC carried into the TZ by the three physical particle injection pumps and the DOC released there by the food web is respired to CO2 by bacteria in the TZ. Some of this DOC may be transformed into refractory DOC (mean radiocarbon age of thousands of years; Hansell, 2013), and thus become part of the microbial carbon pump (Jiao et al., 2011; Legendre et al., 2015).
Measurements of TZ variables and processes have been conducted from oceanographic ships for more than a century, and using instrumented moorings since several decades. These measurements cannot be made from satellite remote sensing, although some TZ variables, such as POC (or its proxy backscattering, bbp), can be estimated from remotely sensed information combined with parameters derived from in situ observations over the water column (EZ + TZ) (Sauzède et al., 2016; Sauzède et al., 2020). These approaches are reviewed in CEOS (2014) and Siegel et al. (2016), and one is mentioned in the next section in relation with the biological gravitational pump. We briefly explain below the approaches used to estimate TZ variables and processes for each of the six BCPs, with some emphasis on those made from autonomous platforms for the same reason as in section “The Euphotic Zone Measurements – From Satellites to Ships to Miniature Sensors.”
Gravitational Pump
Historically, the downward particle flux of the biological gravitational pump has been estimated in situ from either moored sediment traps (Martin et al., 1987; Honjo et al., 2008), ship-based measurement of thorium-234 disequilibrium in particles (Buesseler et al., 2001), or vertical profiles of particle size spectra measured with the Underwater Video Profiler (Guidi et al., 2009). In parallel, global methods have been proposed based on satellite remote sensing combined with empirical models of POC export and attenuation (e.g., Henson et al., 2012; Guidi et al., 2015) or food-web models (Siegel et al., 2014). Given the large uncertainties on the global and regional estimates from these methods (e.g., Boyd et al., 2019), new observational approaches are needed to better assess the spatio-temporal variability of the biological gravitational pump, and identify and quantify its main drivers.
Additionally, the biological gravitational flux estimated from POC collected in moored sediment traps or using models based on trap data encompass the contribution of POC by the physically and biologically mediated pumps in the TZ. This could lead to double accounting (Boyd et al., 2019) if the deep POC flux was entirely ascribed to the sole biological gravitational pump without taking into account the specific contributions of the other BCPs to the POC flux in the TZ. The implementation of new observational methods will provide the information required to assess the contribution of each BCP to the biogenic carbon flux at different depths in the TZ, and thus prevent double accounting.
As explained above for the EZ (see section “The Euphotic Zone Measurements – From Satellites to Ships to Miniature Sensors”), information on phytoplankton and POC can be derived from measurements of miniature sensors based on optics (FChla, bbp and cp) or imagery (Underwater Vision Profiler, UVP), which have been or are being implemented on floats, gliders and moorings. The UVP provides in situ measurements of particles > 100 μm and zooplankton (Picheral et al., 2010), and a miniaturized version of the sensor is being deployed on robots (Claustre et al., 2020). Vertical profiles of UVP particles were also used to estimate particle flux attenuation and its spatio-temporal variability (Guidi et al., 2009, 2015). In addition, the Optical Sediment Trap (OST), introduced by Bishop et al. (2004), Bishop (2009) and improved by Estapa et al. (2013, 2017, 2019), can be used to quantify the downward flux of particles at any depth, including just below the EZ, over long periods of time (years). The principle is the collection of sinking particles on the upward-facing window of a vertically oriented transmissometer on a float, which can be flushed clean through a pumping system at regular intervals (Bishop and Wood, 2009). The recorded signal can be split into two components, i.e., a relatively steady flux of small particles, and a mainly stochastic flux of large particles. The depth at which a float drifts determines which component of the biological gravitational pump is quantified, e.g., export from the EZ, particle attenuation at various depths in the TZ, or remaining flux below the TZ. Robotic approaches can thus complement sediment traps and thorium-234-based methods for estimating the particle flux and its vertical attenuation within the TZ.
Optical sediment traps on floats drifting at “parking depth” (e.g., 1000 m) intercept sinking particles of the biological gravitational pump, and also particles carried downwards by other pumps depending on their activities. The same is true of conventional sediment traps. Deconvoluting the trap signal into its pump components is possible using additional independent and concurrent measurements, such as vertical particle size distributions and zooplankton abundances on the vertical (UVP). Trap measurements at given depths provide maximum estimates in the magnitude of the biological gravitational pump at this depth, i.e., assuming no contributions from other BCPs.
Miniaturized sensors are now being deployed on robots, where they enable the characterization of sinking particles within the TZ and the processes that control their fate. For example, bbp can be divided into three types of particles, i.e., small refractory (background), small labile, and large fast-sinking (Briggs et al., 2020), provided that the profiles are acquired with high vertical resolution (∼1 m). These components can be used to quantify the fragmentation rate of large particles into suspended ones (Briggs et al., 2020), or changes in the sinking rate of large particles (Briggs, pers. comm.) In addition, the deployment of miniaturized UVP on robots will provide detailed quantitative information on large-particle stocks in terms of sizes and types, i.e., fecal pellets, phyto-aggregates, marine snow, and zooplankton taxa. Furthermore, combining backscattering and transmissometer measurements enabled the derivation of the backscattering ratio of the particle assemblage, which was inverted into the refractive index (Twardowski et al., 2001), whose low values were related to labile organic material and high values were typical of refractory organic or mineral material. The refractive index is a dimensionless quantity characterizing the behavior of light within a particle with respect to water. Its values are used to infer the presence of calcite-ballasted particles in the TZ after coccolithophorid blooms in the EZ (Neukermanns et al., pers. comm.).
The above techniques, methods and sensors for characterizing the types of particles or quantifying vertical POC flux attenuation were developed and generally implemented independently from each other in the past. From now on and for the first time, the ongoing progress in robot technology (e.g., new and more flexible electronics, additional batteries for extended missions, and more sensors) will allow the simultaneous implementation of several of these methods (or all of them) on the same platforms, which is expected to become a usual community approach in the near future. This will create unique opportunities to develop new synergistic methods to characterize the particles involved in vertical fluxes, and quantify these fluxes for a wide range of spatial and temporal domains (Nayak and Twardowski, 2020).
Mixed Layer Pump
The mixed layer pump is a 2-D mechanism (spatio-temporal dimensions: vertical and time) that can occur over different time scales, and results from stratification-mixing cycles causing an entrainment-detrainment cycle of particles between surface and deeper waters. The first occurrence of the mixed layer pump was actually identified at the seasonal scale for DOC before it was recognized for POC (Copin-Montegut and Avril, 1993) and modeled (Levy et al., 1998). At the seasonal time scale, shoaling of the mixed layer in spring followed by isolation of a particle-laden stratum was evidenced through time-series of vertical profiles of density and backscattering acquired by floats (Dall’Olmo and Mork, 2014; Kheireddine et al., 2020). Global estimation of the mixed layer pump associated with late winter-spring transitioning was proposed and implemented by Dall’Olmo et al. (2016), using annual time-series of satellite- derived surface POC within the mixed layer estimated from temperature and salinity data from Argo floats collocated with satellite data. At the intra-seasonal time scale, model results (Resplandy et al., 2019) suggest that small-scale heterogeneity of the mixed layer can drive intermittent export events. The strong horizontal variability of satellite surface chlorophyll observations in late winter and early spring supports the view of intermittent dilution events (also observed with autonomous profiling floats) at intra-seasonal time scale (Lacour et al., 2017; Keerthi et al., 2021).
Alternative methods have been proposed to estimate POC fluxes associated with events belonging to the mixed layer pump using simultaneous vertical profiles of FChla to estimate mixing layer depth, i.e., the effective layer where mixing does occur), density (to estimate mixed layer depth, i.e., resulting from long-term mixing) and bbp (to estimate POC) (Lacour et al., 2019). Glider measurements of bbp (to estimate POC) in the northeast Atlantic during one year provided high-frequency observations of export from the EZ showing that the mixed layer pump contributed between 5% and 25% of the total sinking and injected export flux (Bol et al., 2018).
Eddy Subduction Pump
The eddy subduction pump is a complex 3-D process (spatio-temporal dimensions: vertical, horizontal and time). Comprehensive understanding and quantification of this pump require high-resolution transects of POC with towed instruments (Stukel et al., 2017) or gliders (Omand et al., 2015) to evidence anomalies along vertical profiles (e.g., increases in POC and/or O2 content at a given depth horizon that are not due to a deep chlorophyll maximum). Converting the POC anomalies in the water column into POC fluxes from such observations requires additional constraints on vertical velocities that are difficult to estimate from observations (Pietri et al., 2021) and are generally derived from models (Omand et al., 2015; Stukel et al., 2017).
Floats are not a priori the best platforms for characterizing the full 3-D structure of a eddy subduction event, but anomalies in vertical profiles of biogeochemical properties recorded by floats can be used to quantify the eddy subduction pump using constraints on vertical velocities (Llort et al., 2018). Given the widespread and expected future global distribution of floats, mapping such anomalies could provide the best way to quantify the global contribution of eddy subduction to the pumping of biogenic carbon, and characterize its spatial and temporal variability. The float approach would provide complements to process studies conducted with towed instrument and/or gliders. A remaining uncertainty concerns the role of small-scale upward vertical velocities, as model results suggest that the downward flux of the eddy subduction pump might be largely compensated by an upward eddy flux (Resplandy et al., 2019). Methods based on anomalies in vertical profiles may provide an upper bound for the eddy subduction pump, and models could be used to constrain the net eddy subduction flux derived from in situ measurements, e.g., from floats.
Large-Scale Subduction Pump
In the case of purely vertical transport across a fixed export depth, the large-scale subduction pump reduces to the Ekman pump, i.e., the transport of POC and DOC from the seasonal mixed layer into the ocean interior by large-scale wind-driven Ekman pumping. However, some authors suggested the use of a varying export depth (e.g., Buesseler et al., 2020), in which case the large-scale subduction pump includes, in addition to Ekman pumping, an horizontal transport component across the horizontal gradient of the varying export depth.
Estimating the magnitude of the large-scale subduction pump requires the integration of large numbers of measurements of physical and geochemical properties made at the scale of ocean basins and over annual cycles, which raises issues of matching and merging measurements made at different space and time scales. It may also be that velocities derived from circulation models would be enough for the physical component. Assessing future changes in this pump will involve the integration of data in different ocean basins, including measurements from gliders, floats, moorings, ships, USVs and remote sensing, and estimating these changes will likely be based on modeling (e.g., Resplandy et al., 2019), in particular involving data assimilation (DeVries and Weber, 2017; Verdy and Mazloff, 2017).
Diel Vertical Migration Pump
Historically, the way to address the effect of diel vertical migrations of zooplankton on carbon export has been indirect. Methods have generally relied on estimating the biomass of migrant organisms combined with relationships linking their specific metabolic rates (e.g., respiration, excretion, egestion) to their biomasses or size using allometric relationships. The estimation of migrants was derived from plankton net hauls (Giering et al., 2014; Kiko and Hauss, 2019) or high-frequency sonar (Giorli et al., 2018) or UVP (Kiko et al., 2020) measurements, while metabolic rates were generally estimated on live plankton on board ships or in the laboratory (e.g., Ikeda, 2014).
In addition, fish, jellyfish and other animals larger than zooplankton perform diel vertical migrations, but had not generally been considered as part of the diel vertical migration pump. However, these organisms may actively transport significant amounts of carbon from the EZ deep into the TZ, and thus contribute to the diel vertical migration pump (Pinti et al., 2021). Studies based on acoustic sensors carried by gliders (Reiss et al., 2021) or USV (saildrones) (De Robertis et al., 2021) have showcased the potential of this approach to monitor macrozooplankton or fish. Such technological advances may become tools of choice for characterizing diel vertical migrations of large organisms, but their role is not explicitly considered in the remainder of this paper or included in Figure 1 because the study advocating it (Pinti et al., 2021) has not been published in peer-reviewed literature as yet.
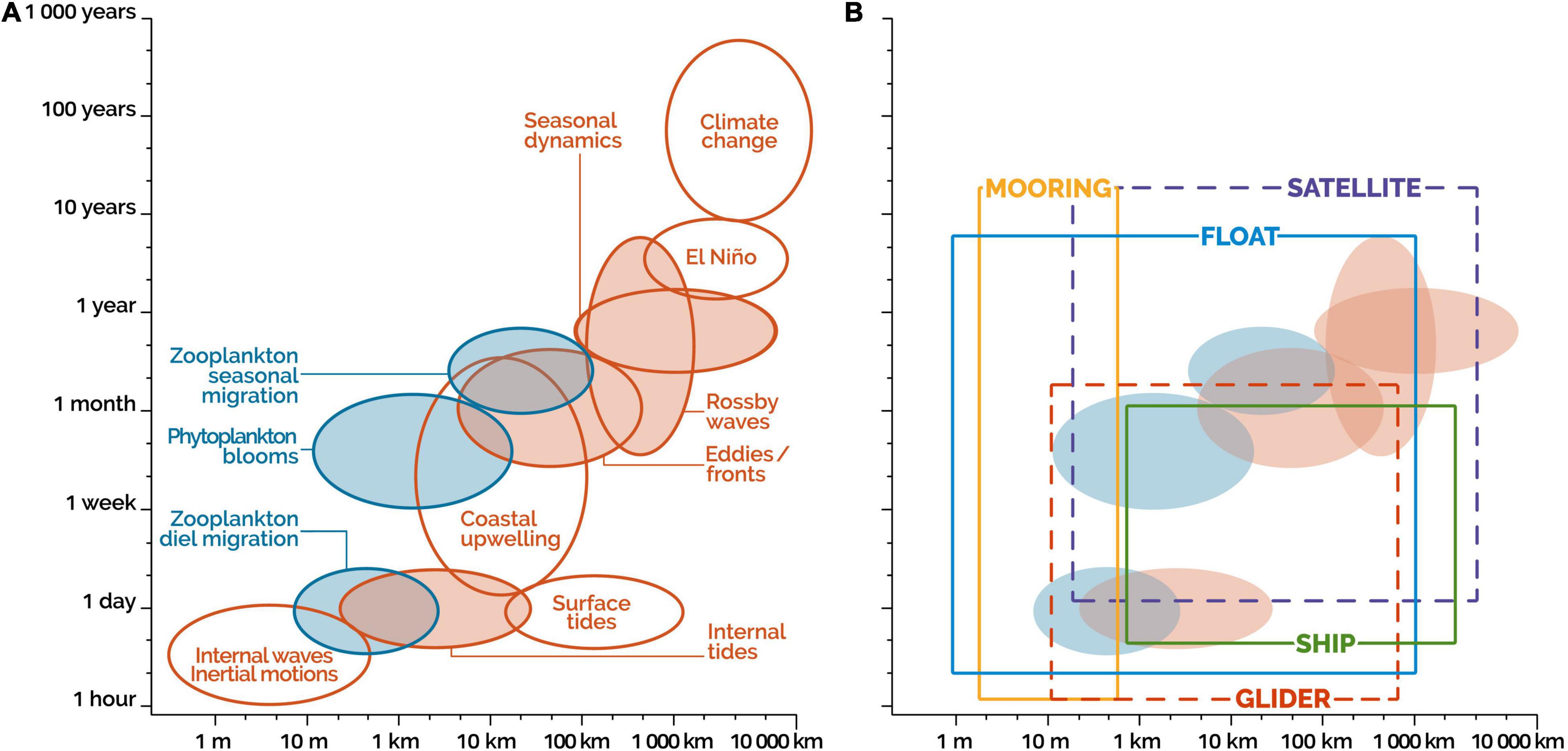
Figure 1. Semiquantitative representation of spatial (geographical, horizontal) and temporal scales of important marine physical dynamic (red ellipses) and ecosystem (blue ellipses) processes (modified from Chai et al., 2020, Figure 2). (A) Filled ellipses: processes of significance to BCPs. (B) BCP-relevant processes from panel (A) (ellipses) and spatio-temporal ranges of five observation platforms (rectangles): satellites, ships, floats, gliders, and moorings. The geographical ranges of fleets of mobile platforms and mooring networks will be wider than those of individual mobile platforms and moorings illustrated in panel (B).
Expected improvements in understanding and quantifying the diel vertical migration pump will rely on the possibility of estimating the biomasses of migrant organisms from robotic measurements at high temporal resolution (e.g., twice a day) and over the full annual cycle. Potential for this has been showcased using anomalies in optical measurements (FChla, bbp, CDOM) from floats (Boyd et al., 2019). Such anomalies were later analyzed in a more systematic way to characterize the regional and seasonal variability of diel vertical migrations from a BGC-Argo database (Haentjens et al., 2020). Micro-sonar sensors mounted on elephant seals (Goulet et al., 2019) have shown clear signatures of diel vertical migration (Guinet, pers. comm.), and these low-electrical consumption sensors have a strong potential to be implemented on floats and gliders. Finally, miniature UVPs deployed on floats, gliders and moorings could be used to quantify (using on-board Artificial Intelligence) the characteristics of particles and the main groups of mesozooplankton, including those performing diel vertical migrations.
As in the case of the biological gravitational pump (see section “Gravitational Pump”), it is expected that additional, possibly yet unanticipated, understanding of the diel migration pump will result from combining sensors that had been used independently until now. This refers to the simultaneous deployment of acoustic and imaging sensors (e.g., Goulet et al., 2019; Picheral et al., 2021) on the same platforms, especially that these methods are non-intrusive and should thus not suffer (which remains to be confirmed) from the same sampling bias as plankton net hauls (e.g., Catul et al., 2011). Imaging and acoustics measurements made by the same robots would directly link the acoustic signals to organisms on the images, and the resulting relationships would be useful for estimating the biomasses of migrating animals detected by acoustics at large spatio-temporal scales.
Seasonal Migration Pump
The ontogenic migration pump, also called seasonal lipid pump (Jonasdottir et al., 2015), is a regional high-latitude process that can be estimated by the same approaches as those used to quantify the diel vertical migration pump, i.e., combining biomass estimates with specific metabolic rates. The biomass of overwintering copepods was sometimes estimated by ship-based winter surveys, but the required extensive cruises are very difficult to conduct in the harsh high-latitude seas during these periods.
As was the case for diel vertical migrations, sampling with robots equipped with optical, imaging and acoustic sensors could provide the spatio-temporal information required to properly quantify this presently highly unconstrained pump. However, copepods Calanus hyperboreus were observed to overwinter in layers a few tens of meters thick just above the seabed in waters > 2000 m in the northern North Atlantic (Visser et al., 2017, and refs. therein), and detecting such layers of organisms near the seabed will be a technical challenge. Addressing the ontogenic migration pump would thus use these same tools as for the diel vertical migration pump, but would be more specific in term of the locations targeted, i.e., high-latitude areas.
Characterization of Mesopelagic Particles
The nature of particles in the TZ (i.e., large vs. small, labile organic vs. refractory organic or mineral) is of paramount importance to assess the fate of POC transferred to depth by the six BCPs, as explained above (third paragraph of section “The Twilight Zone Variables and Processes, and Their Measurement”). In addition, this information is required for estimating the efficiency of the six BCPs, defined as the time during which the exported biogenic carbon is sequestered from the atmosphere in the ocean (Boyd et al., 2019). Existing optical and imagery sensors can now be used to characterize particle assemblages as could never be done in the past (Nayak and Twardowski, 2020). Indeed, it was explained in section “Gravitational Pump” how these sensors deployed on robots provide estimates of the following categories of sinking particles that impact BCP efficiency: small refractory, small labile, and fast-sinking large particles; for the large particles, the sizes of fecal pellets, phyto-aggregates and marine snow; the refractive index, whose low values are related to labile organic material and high values are typical of refractory organic or mineral material; and the presence of calcite-ballasted particles in the TZ after coccolithophorid blooms in the EZ, inferred from the refractive index.
Following the decline of any type of phytoplankton bloom, the fragmentation of large aggregates into suspended particles may be of key importance for balancing the TZ carbon budget (Giering et al., 2014). In high-latitude areas, it was shown that on average 50% of the attenuation of the downward biomass flux of post-bloom aggregates is due to their fragmentation into smaller-sized suspended particles (Briggs et al., 2020). The method is based on the analysis of spikes in vertical profiles of optical proxies, which reflect the alteration of particle characteristics (Chla, bbp) along the profiles (Briggs et al., 2011). It could be complemented by concurrent UVP measurements, for better defining the types of particles involved in the fragmentation. Finally, the downward flux of particles quantified by the OST technique (Estapa et al., 2017) could also be characterized using the types of particles (e.g., large vs. small) in the sinking assemblages recognized in simultaneous UVP records.
Spatial and Temporal Scales of Occurrence of the Various Pumps
Until recently, it was thought that the biological pumping of carbon to depth in the ocean was mostly linked to the spring phytoplankton bloom, via gravitational sinking of organic particles. As a consequence of this paradigm, many ship-based programs on the biological pump were conducted at temperate or sub-polar latitudes in late winter or early spring, as these regions and periods offered the highest probability of encountering large phytoplankton blooms. The corollary is that several other regions and/or periods were poorly sampled or neglected, and the other BCPs were largely ignored. The emergence of a broader vision of the various export mechanisms of biogenic carbon from the EZ to the TZ and carbon sequestration at depth (Boyd et al., 2019) led to the recognition that the pumping of biogenic carbon is more complex than previously thought. The new paradigm not only involves multiple export mechanisms, but also considers that each of them occurs at distinctive spatial and temporal scales (Boyd et al., 2019).
The modeling study of Resplandy et al. (2019), which considers the combined effects of four BCPs –gravitational, mixed layer, eddy subduction, and Ekman (i.e., the two zooplankton migration pumps are not taken into account) – and considers a large region of the North Atlantic Ocean (between the sub-tropical and sub-polar gyres), showcases the critical spatial and temporal scales of occurrence of each pump. The magnitude of the gravitational and physical-injection BCPs is much higher in the sub-polar areas than in the sub-tropical gyres, indicating that the various export processes are region- and even latitude-dependent. In addition, the physically mediated BCPs are dominant in winter-early spring, and the biological gravitational pump is mainly active in spring. We already know from other studies that ontogenic migrations essentially occur in the summer and early autumn at high latitudes (Kobari et al., 2010; Jonasdottir et al., 2015; Boyd et al., 2019). Similarly, diurnal migrations are both region-dependent (Bianchi et al., 2013) and season-dependent as light is their main trigger (Cottier et al., 2006). Hence, the temporal succession of the various transfer mechanisms appears highly constrained by seasonality, which is generally stronger at higher than lower latitudes.
The present field estimates of the respective contributions of the various mechanisms to the biological pumping of carbon are still preliminary, and are presently quite disconnected as they rely on independent studies that each focused on one or a few BCPs (Boyd et al., 2019). The challenge from now on is to develop approaches that investigate the various BCPs in integrated ways, and address not only several pumps but also possible biogeochemical links and interactions among them.
Figure 1A illustrates the spatial (geographic) and temporal scales of important marine physical dynamic (red ellipses) and ecosystem (blue ellipses) processes, and highlights the processes of significance to BCPs (filled ellipses). The spatio-temporal range of any ellipse is debatable, and only provides an indication of the spatial and temporal extent of the process. Overall, Figure 1A generally shows that the combined spatial scales of the BCP-relevant processes range between about 10 m and 1000 km, and the combined temporal scales range between about one day and 10 years. It follows that sampling strategies for investigating the BCPs should be designed to capture variability over this spatio-temporal range. These strategies are examined in the next sections (Figure 1B is discussed in section “Implementing the paradigm Shift: A Multiplatform Observation Strategy”).
In order to capture the variability of the various BCPs and the sequence of their occurrences and magnitudes according to regions and seasons, their characteristics should be measured at a variety of locations over several years. At each location, many different variables and fluxes should be measured in the EZ and the TZ (see section “Biological Carbon Pump Variables and Processes to be Measured”) over appropriate temporal scales. As the resources for making the observations will always be limited, cost-effective observational strategies should be developed, and each of these strategies should maximize the scientific return with respect to the observational effort. In this context, a first important prerequisite will be the careful selection of sampling regions (see section “Selecting Representative Regions and Sampling Depths for Integrated Studies of the Pumps”). A second prerequisite for cost effectiveness will be the definition of appropriate observational strategies to get the required information in each region (see section “Implementing the Paradigm Shift: A Multiplatform Observation Strategy”). Finally, the upscaling of regional information to the global scale, in particular through models assimilating biogeochemical data (Verdy and Mazloff, 2017), will require the implementation of similar observation strategies in several key regions or even at the global scale (see section “From Regional Knowledge to Global Scale”).
Selecting Representative Regions and Sampling Depths for Integrated Studies of the Pumps
The selection of representative regions should be primarily based on existing knowledge concerning the global distributions of the BCPs. For example, the magnitude of the gravitational and the three physically driven pumps varied among the subpolar, jet and subtropical regions in a modeling study, and the contributions of the four pumps to total export from the EZ varied during the course of the year (Resplandy et al., 2019). Similarly, field-based studies have shown enhanced activity of some of the BCPs at high latitudes at different times of the year (Boyd et al., 2019), i.e., biological gravitational pump in spring and early summer (e.g., Briggs et al., 2011, 2020), mixed layer and eddy subduction pumps during the winter-spring transition (e.g., Omand et al., 2015; Dall’Olmo et al., 2016), and ontogenic migration pump during the summer-autumn transition (Jonasdottir et al., 2015). In contrast, the large-scale subduction pump is active the whole year in subtropical gyres.
Beside this general framework, a pragmatic and cost-effective approach for selection of regions could be guided by the following two simple principles. First, representative regions should together cover the wide range of export regimes already identified by studies on the biological gravitational pump. This means covering as comprehensively as possible the space domain corresponding to the wide range of observed values of transfer efficiency vs. export ratio (defined in section “The Euphotic Zone Variables and Processes”) (Buesseler and Boyd, 2009; Buesseler et al., 2020). It would also anchor future studies to the existing information concerning the different export regimes, while providing additional resolution and improved (mechanistic) understanding. Second, at least some of these regions should be located in waters where different biologically and/or physically mediated BCPs present clear signals, including situations when they co-occur or occur sequentially (Stukel et al., 2017). The latter information could be derived from models (Resplandy et al., 2019) and/or in situ observations (Jonasdottir et al., 2015).
Another way of selecting representative regions could be to focus on the range of spatio-temporal variability of two main determinants of the amount of biogenic carbon transferred to depth by the BCPs, i.e. (1) the drivers of phytoplankton growth, because they set the magnitude of potential export, and (2) the key driving characteristics of each BCP, which determine the main routes of organic carbon transport from surface to depth.
First, the primary drivers of phytoplankton growth are euphotic-zone irradiance and the supply of nutrients. At the large scale, the dominant variability in euphotic-zone irradiance and nutrient supply is modulated by the regimes of mixing and stratification, which determine how phytoplankton use the two primary resources. The distributions of euphotic-zone irradiance, nutrient supply and mixing-stratification generally show latitudinal gradients. Hence, selecting regions along a gradient from poles to equator would encompass the dominant drivers of phytoplankton growth. At a given latitude, the nutrient status of water masses could be partitioned into iron-replete or iron-deficient, the latter determining High-Nutrient Low-Chlorophyll (HNLC) regions. At high latitudes, the Southern Ocean and North Pacific HNLCs contrast with the iron-replete North Atlantic Ocean. At low latitudes, the Equatorial Pacific HNLC contrasts with the iron-replete Equatorial Atlantic and Indian Oceans.
Second, the drivers of the different BCPs are quite diverse. The mixed layer pump is primarily driven by the magnitude of mixing and the intermittency in conditions of mixing-restratification at high- and mid-latitudes during the winter-spring transition, which depend on the heat flux and wind stress. The eddy subduction pump is related to the intensity of eddy activity, which is variable regionally and seasonally, and is strongest close to western boundary currents (Ajayi et al., 2020). The large-scale subduction pump is strongest in the center of oligotrophic subtropical gyres, and is permanent. Diurnal vertical migrations are essentially driven by light (e.g., Cohen and Forward, 2009), of which the distribution depends on latitude, season, and cloud coverage combined with water transparency (which essentially reflect Chla concentration in the open ocean). Finally, seasonal vertical migrations, which are restricted to subpolar and polar regions, occur over amplitudes that are mostly determined by the (deepest) depth of the winter mixed layer, below which diapausing zooplankton are sheltered from ocean turbulence.
Regions delineated on the basis of seasonal cycles of phytoplankton dynamics and production (e.g., Longhurst regions, Longhurst, 2010) are also of special relevance to the BCPs because phytoplankton dynamics and production in the EZ largely determine the amount of organic carbon transferred to the TZ (e.g., Siegel et al., 2016), not only through the biological gravitational pump but also through physically mediated pumps (Omand et al., 2015; Dall’Olmo et al., 2016) and animal-mediated vertical transport (e.g., Jonasdottir et al., 2015). Such an approach has also the advantage of being easy to derive from ocean color remote sensing. This has been showcased in the Mediterranean Sea by the implementation of float-based observational strategies (D’Ortenzio et al., 2020; Le Traon et al., 2020) designed to understand the biological-physical processes driving phytoplankton dynamics in ocean color-derived bioregions (D’Ortenzio and d’Alcala, 2009) (D’Ortenzio et al., 2012). Additionally, selecting regions on the basis of their surface satellite ocean-color characteristics would facilitate the upscaling of regional information, including flux estimates, to the global scale. Finally, satellite altimetry could also be used to select regions and period, identified by the highest kinetic energy fields and where the eddy subduction pump might expectedly be the strongest.
A bioregionalization of the global ocean was recently proposed based on annual time series of vertical profiles of Chla and bbp acquired by BGC-floats (Bock et al., 2021). This approach not only evidences surface patterns already identified in satellite-based regionalization (e.g., Hardman-Mountford et al., 2008; D’Ortenzio and d’Alcala, 2009), but also uniquely characterizes seasonal patterns of particle dynamics at various depth horizons within TZ. This new way of using large, global BGC-Argo data sets has great potential to improve region selection.
Additional characteristics of the EZ or the TZ could also contribute to the selection of representative regions. Such characteristics include: the presence of doming isopycnals in tropical waters, where the upward flux of nutrients in the EZ cause hotspots of NPP (Voituriez and Herbland, 1981; Duteil et al., 2009); oxygen minimum zones in the TZ, which modify the downward flux of organic carbon (Cavan et al., 2017; Weber and Bianchi, 2020); and areas of large recurring blooms of phytoplankton with special flux characteristics associated with bio-mineral ballasting, e.g., diatoms (fast sinking) or coccolithophorids (production of carbonate) (Le Quéré et al., 2005).
Finally and complementary to these, new methods to analyze climate change projections from Earth System models (Boyd et al., 2015) have identified regional differences in biological and biogeochemical variables and processes (e.g., phytoplankton biomass and physiological rates) associated with environmental forcing that include climate change stressors. This new type of information could be used to identify sentinel regions where targeted process studies could be implemented to monitor long-term anthropogenically driven changes in the different BCPs, and also regions where some BCPs might be strongly impacted by these changes (e.g., physically mediated pump impacted by progressive increase in stratification).
In addition, (Reygondeau et al., 2018) proposed a biogeographic approach for the delineation of biogeochemical regions in the mesopelagic layer, based on the same concept as used by Longhurst (2010) for the EZ. Consideration of these mesopelagic regions could help to determine the choice of regions and the relevant vertical extent of the TZ in each region. This delineation relies in particular on oxygen concentration, the POC flux and the vertical distribution of temperature, which are fundamental variables for BCP studies. The locations of the resulting TZ regions mirror to some extent those of the EZ regions, with an obvious meridional/latitudinal component. However, the regional boundaries of the two sets of regions may differ, as a result of biogeochemical characteristics or processes that occur only in TZ and thus do not affect the boundaries of surface regions (e.g., oxygen deficient zones). Additionally, (Reygondeau et al., 2018) showed that the depth of the interface between the mesopelagic and the bathypelagic layers increases from the pole (< 600 m) to the equator (> 1500 m and even 2500 m in the Pacific Ocean). Consideration of a regionally varying lower limit of the TZ combined with a varying depth of the EZ based on vertical light attenuation (Buesseler et al., 2020) could potentially lead to dynamic adjustments of the depths sampled in the EZ and the TZ.
Implementing the Paradigm Shift: A Multiplatform Observation Strategy
The implementation of an observational strategy for the integrated study of the new multiple BCP paradigm (see section “Introduction: The Ocean Biological Carbon Pump, a Paradigm Shift”) will require the acquisition of quantitative information on the variables and processes needed to assess the contributions of the different BCPs to the pumping of biogenic carbon in oceans. It would also need to concurrently document the environmental drivers of each of these pumps. To do this, comprehensive and integrated time series of multidisciplinary variables and processes (see section “Spatial and Temporal Scales of Occurrence of the Various Pumps”) should be constructed for the different sampling regions (see section “Selecting Representative Regions and Sampling Depths for Integrated Studies of the Pumps”). Note that the development of such integrated observations dedicated to the BCP would be fully in line with ongoing plans of implementing sustained monitoring the ocean in various regions (e.g., deYoung et al., 2019; Hermes et al., 2019) to progressively develop a Global Ocean Observing System (GOOS).
We examined above the spatial and temporal ranges of marine physical dynamics and ecosystem processes of significance to BCPs (Figure 1A). In Figure 1B, we superimpose the spatio-temporal ranges of five observation platforms (rectangles: satellites, ships, floats, gliders, and moorings) on those of the BCP-relevant processes (ellipses), to characterize the potential role of each platform in BCP studies. While Figure 1B illustrates the spatio-temporal ranges of individual platforms and moorings, the geographical ranges of fleets of mobile platforms and networks of moorings will be wider than those of individual platforms. Given that these extended ranges would depend on the configurations of the fleets of mobile platforms and networks of moorings, these ranges are not illustrated in Figure 1B. At first glance, the Figure shows that no platform covers the combined spatio-temporal ranges of all BCP-relevant processes, which points out that the BCP observation strategy should be multiplatform. The potential contributions of the different observation platforms to the generation of long-term time series to study the air-sea CO2 flux and the six BCPs described in the following paragraphs are summarized in Table 3.
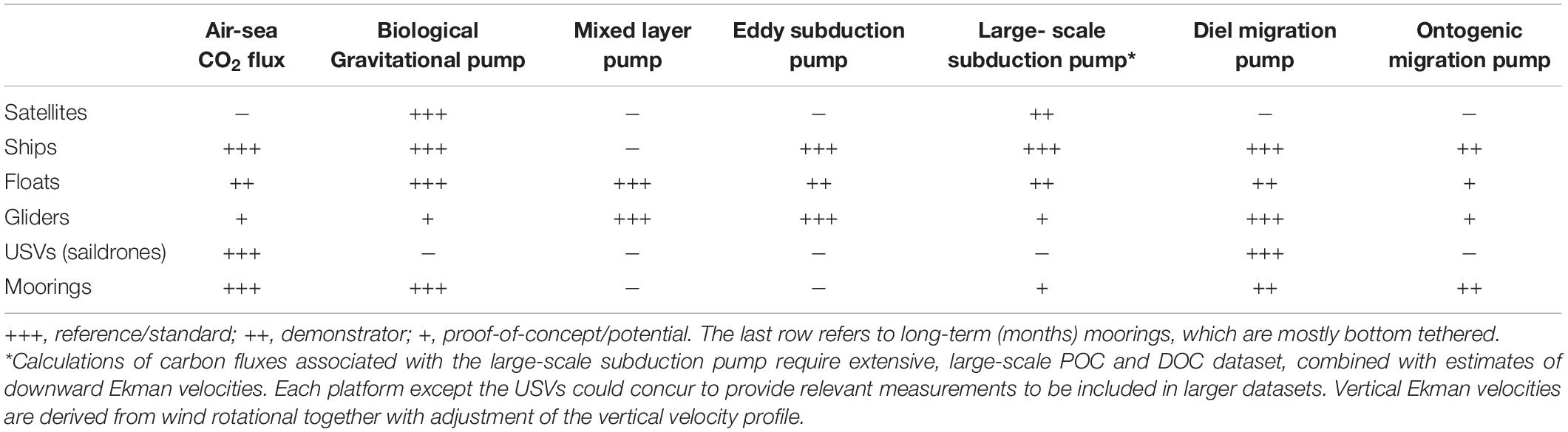
Table 3. Potential contributions of the different observation platforms to the creation of a multiplatform strategy to investigate the air-sea CO2 flux and the six BCPs.
The six pumps together have aspects that belong to different oceanographic disciplines, i.e., biological, chemical and physical oceanography, and marine biogeochemistry and sedimentology. The conventional approach to investigate marine phenomena that involve several disciplines is to organize multidisciplinary programs, which often involve multidisciplinary cruises with sometimes multiple ships (e.g., the JGOFS North Atlantic Bloom Experiment Ducklow and Harris, 1993, or the mesoscale in situ iron fertilization experiments Boyd et al., 2007). Multidisciplinary voyages will continue to be essential over the next decades to investigate detailed variables and processes of the six BCPs, especially processes not currently amenable to automatic measurements. Measurement of biogeochemical variables on discrete samples will also be used to validate the calibration of sensors mounted on autonomous platforms at the time of their deployment or after, and also to validate new approaches as was done, for example, for the OST methods (Estapa et al., 2019). However, assessing the variability of BCPs among environments and over seasons and years will require continuous measurements of some of their characteristics in several ocean regions over long periods of at least one year, which could be implemented through multiplatform observation strategies.
As explained in section “The Euphotic Zone Measurements – From Satellites to Ships to Miniature Sensors,” satellite remote sensing already provides long-term synoptic horizontal information on some of the key variables that drive the BCPs in the EZ, and upcoming new sensors with extended capabilities have the potential to improve the study of some BCPs (Brewin et al., 2021). Examples given in that previous section are: LIDAR, to be used for retrieval of bio-optical properties deeper than one attenuation length in the water column (roughly one fifth of the EZ), to which passive radiometers are limited (Jamet et al., 2019), and quantification of zooplankton diurnal migrations (Behrenfeld et al., 2019); increased spectral resolution of hyperspectral sensors (e.g., PACE satellite mission), to better resolve phytoplankton communities (IOCCG, 2014); and improved altimetry measurements (SWOT mission), for better resolution of sub-mesoscale processes in the eddy subduction BCP (Morrow et al., 2019). Remote sensing could also be used to derive estimates of some relevant processes in the TZ (see section “Gravitational Pump”). This approach thus covers the horizontal (X-Y) and time (T) axes for some key variables, and can potentially provide information on the depth (Z) distributions of a limited number of variables.
In situations where deep-water instrumented moorings are operated in the long term, these moorings combined with monthly or more frequent cruises already provide uniquely detailed information that could be used to investigate temporal changes in the magnitude of some of the BCPs. The latter include the biological gravitational and diel vertical migration pumps, and possibly some of the physically mediated pumps. Measurements on such moorings are generally made at discrete depths, but the recent deployment of vertical profiling systems like wire walkers (Pinkel et al., 2011) and the SeaCycler (Send et al., 2013) on some moorings provides vertically resolved data at various time intervals. In addition, sediment traps deployed at different depths record the sinking particles over time intervals of pre-set duration. Examples of such deep-water moorings are BATS (oligotrophic subtropical North Atlantic), DYFAMED (oligotrophic Mediterranean), ESTOC (oligotrophic subtropical North Atlantic), HOT (oligotrophic subtropical North Pacific), PAP (eutrophic temperate North Atlantic) and SOTS (subAntarctic zone southwest of Australia). The data from such moorings could be used to document variations in some of the local BCPs over a wide range of time scales. Hence the records from these moorings provide information that is very detailed on the T axis and is more or less resolved for the Z axis depending on the configuration of the mooring. Information from satellites and deep-water moorings could be complemented by models to derive preliminary estimates of the variability of the BCPs on the four X, Y, Z and T axes.
Some of the key BCP variables cannot be estimated from satellite remote sensing, especially at depth in the TZ, and many of the physical, biological and biogeochemical conditions encountered in offshore waters are not covered by the existing instrumented moorings in deep waters. To move forward with the investigation of spatio-temporal variability of the BCPs in the global ocean, the key BCP variables should be observed along the X-Y-Z-T axes directly. Using present and developing technology, this could be done by deploying fleets of instrumented robots (especially floats and gliders) for long periods (i.e., several years), combined with complementary ship cruises and satellite remote sensing. Robots would be deployed during the first voyage of each study, and measurements would be made during cruises to “sea-truth” some of the automatically sampled as well as the remotely sensed variables and processes (e.g., Cetinic et al., 2012; Haentjens et al., 2017). Additional measurements would be made to estimate important BCP variables and processes that could not be directly or indirectly estimated from robot data. Also, datasets (called “machine learning datasets”) could be acquired by ships to train machine learning algorithms used to derive virtual variables (see section “The Euphotic Zone Measurements – From Satellites to Ships to Miniature Sensors”) from robot observations (e.g., Rembauville et al., 2017).
Marine robots also include sea-surface saildrones and wave gliders, which are USVs powered by wind and solar energy (saildrones) or by wave motion and solar energy (wave gliders) and carry navigational instruments and sensors, which can be scientific. These sea-surface robots can collect data on variables that include air-sea exchanges of heat and gas (CO2) and ocean-surface acidification. They can also record water-column acoustic signals using ADCP or echosounder, as a way to cost-effectively monitor animal biomass (Mordy et al., 2017; Chu et al., 2019) and thus potentially contribute to quantify the diel vertical migration pump.
The following bullets provide examples of measurements using the platforms cited in Table 3 concerning the air-sea CO2 flux and the six BCPs.
• Satellites. Biological gravitational pump: carbon flux out of EZ; ocean color. Large-scale subduction pump: POC; ocean color.
• Oceanographic ships. Air-sea CO2 flux: FCO2; pCO2 sensor in pumped surface water. Biological gravitational pump: particle sinking; thorium-234 disequilibrium. Mixed layer pump: T, S, Chla, cp, bbp; CTD + bio-optical package. Eddy subduction pump: T, S, Chla, cp, bbp, O2; towed CTD + bio-optical package + O2 sensor. Large-scale subduction pump: POC and DOC; chemical analysis of discrete samples. Diel migration pump: zooplankton; plankton nets, underwater vision profiler, high-frequency sonar. Ontogenic migration pump: zooplankton; plankton nets.
• Floats. Air-sea CO2 flux (FCO2): T, S, O2; CTD + O2 sensor + neural network. Biological gravitational pump: particle sinking; optical sediment trap, underwater vision profiler. Mixed layer pump: T, S, Chla, cp, bbp; CTD + bio-optical package. Eddy subduction pump: T, S, Chla, cp, bbp, O2; CTD + bio-optical package + O2 sensor. Large-scale subduction pump: bbp (proxy for POC); bio-optical package. Diel migration pump: zooplankton; underwater vision profiler, high-frequency sonar. Ontogenic migration pump: zooplankton; underwater vision profiler, high-frequency sonar. Examples are described in Boyd et al. (2019).
• Gliders. Air-sea CO2 flux (FCO2): T, S, O2; CTD + O2 sensor + neural network. Biological gravitational pump: particle sinking; underwater vision profiler. Mixed layer pump: T, S, Chla, cp, bbp; CTD + bio-optical package. Eddy subduction pump: T, S, Chla, cp, bbp, O2; CTD + bio-optical package + O2 sensor. Large-scale subduction pump: bbp (proxy for POC); bio-optical package. Diel migration pump: zooplankton; underwater vision profiler; high-frequency sonar. Ontogenic migration pump: zooplankton; underwater vision profiler; high-frequency sonar.
• Saildrones and wave gliders (USVs). Air-sea CO2 flux: FCO2; pCO2 sensor. Diel migration pump: zooplankton; high-frequency sonar.
• Moorings. Air-sea CO2 flux (FCO2): pCO2 sensor. Biological gravitational pump: particle sinking; sediment traps, underwater vision profiler. Large-scale subduction pump: bbp (proxy for POC); bio-optical package. Diel migration pump: zooplankton; underwater vision profiler, acoustic backscattering. Ontogenic migration pump: zooplankton; underwater vision profiler.
A practical aspect of implementation is the development of community synergies for the study of BCPs. One approach to do this could be to investigate BCPs in regions or at locations where long-term observing programs are conducted by the international oceanographic community, e.g., the OceanSITES long-term observing stations1, and the GO-SHIP sections (Sloyan et al., 2019). This could be mutually beneficial for sharing ship time, calibration measurements and data. Areas regularly visited by research vessels could thus be considered when selecting the sampling regions discussed in section “Selecting Representative Regions and Sampling Depths for Integrated Studies of the Pumps.”
From Regional Knowledge to Global Scale
One long-term objective of the proposed research community effort should be the assessment of the spatio-temporal variability of BCPs at the scale of the global ocean. This would be a key step in developing baseline information and knowledge on the magnitudes of the different BCPs, an important component in the assessment of the ocean’s responses to climate change. Developing such a global understanding would require upscaling the results of regional observations to the global ocean, which could be achieved through the following four steps.
The first step would be to acquire long-term time series in selected regions, which would capture the temporal variability and magnitude of key EZ and TZ variables and processes involved in biologically and physically mediated export of biogenic carbon in the TZ by the different BCPs. A condition for successful upscaling would be that the selected sampling regions represent the diversity of the different export modes (Jonasdottir et al., 2015; Boyd et al., 2019; Resplandy et al., 2019) and their associated spatio-temporal scales (see section “Selecting Representative Regions and Sampling Depths for Integrated Studies of the Pumps”).
The second step would be to select EZ variables and/or processes whose time series could be obtained at the global scale, most likely by remote sensing or reanalysis, and could be used as predictors of export. For example, it could be possible to derive from remote sensing and reanalyses both export-relevant EZ metrics (e.g., NPP, community composition, phytoplankton phenology) and physical-chemical drivers (e.g., heat fluxes, temperature, mixed layer depth, nutrients).
The third step would be to relate export and TZ variables and also export processes to the EZ variables and/or processes (predictors) selected in the second step. The wealth of simultaneous TZ and EZ measurements acquired as part of a global coordinated sampling effort could be used to develop models linking TZ processes with those occurring in the EZ. Over the last years, empirical models based on artificial intelligence (i.e., neural networks) have been developed to estimate various biogeochemical variables from physical-chemical water-column drivers (e.g., Bittig et al., 2018) or from surface satellite-based properties (Sauzède et al., 2020). Such artificial intelligence-based approaches could be used to estimate, from globally measurable EZ predictors, metrics of the EZ-TZ carbon flux that are difficult to acquire at the global scale.
In the fourth and final step, the empirical relationships (with their uncertainties) between the selected EZ predictors and TZ variables and export would be used for upscaling time series of regional export processes by the different BCPs to full annual cycles at the global scale. The regional values for the different BCPs would be combined to estimate global annual export. Such upscaling approaches would be implemented in parallel to global biogeochemical modeling focused on the BCPs, assimilating satellite and in situ data (e.g., DeVries and Weber, 2017; Verdy and Mazloff, 2017). Ideally the two approaches should converge toward similar global estimates.
Neural networks are potentially highly appropriate for the above global upscaling given that they can be considered as advanced global interpolation methods (Landschutzer et al., 2016; Roshan and DeVries, 2017). Using artificial-intelligence-based relationships applied to large datasets resulting from a variety of platforms (floats, gliders, satellites, ships, USVs) would provide quantitative information on carbon fluxes in the global ocean.
Toward a New Era of Discoveries
The deployment of modern sensors in different areas of the global ocean in recent years has resulted in several exciting, unexpected discoveries, and it is expected that the deployments proposed above would lead to similar discoveries. Here are five examples of recent discoveries.
Examination of plankton images recorded with the Underwater Vision Profiler (UVP5) during the Tara Oceans expedition of 2009–2012 led (Biard et al., 2016) to discover that giant predatory protists (> 600 μm) belonging to the Rhizaria supergroup were extremely abundant in the vast oligotrophic intertropical open ocean. These were so abundant that their carbon biomass was equivalent to that of all other mesozooplankton (size range 0.2–20 mm). This came as a surprise given that rhizarians are generally absent from plankton data, not because they are not present in the water column but instead because they are damaged beyond recognition by plankton nets. In addition, (Guidi et al., 2016) found from Tara Oceans data that rhizarians were strongly associated with carbon export at 150 m in the oligotrophic ocean. Hence, organisms that had seldom been seen by specialists in plankton samples were found to make a large part of the carbon biomass of total mesozooplankton, and to be involved in the deep carbon flux in the oligotrophic ocean.
Similarly, the deployment of a new pelagic in situ video recording system (PELAGIOS, Hoving et al., 2019) led (Christiansen et al., 2018) to discover a new species belonging to the fragile polychete genus Poeobius, which feeds on organic particles by deploying a free-floating mucous net or by catching particles with its tentacles. After discovering the new species, the authors identified it on UVP5 images collected down to 600 m in the North Atlantic from 2012 to 2015, and their analysis of the resulting data showed that high abundances of Poeobius sp. were associated with strongly reduced particle concentrations and fluxes in the layers directly beneath these polychetes. Hence, the animal was not only new to science, but it may have affected the biological gravitational pump in a way that was previously unknown.
The North Atlantic Ocean subpolar gyre is seldom sampled in winter because of its harsh weather conditions. The deployment of 21 floats in 2013–2014 created a unique time series of observations which showed that, in January-March when deep convection is the rule in this region, there were periods of low winds and reduced heat loss from the ocean leading to significant reduction in the depth of the mixed layer (30% of the vertical profiles had mixed layer depths < 100 m). These unexpected hydrodynamic conditions favored the development of “ephemeral” winter phytoplankton blooms, likely due to diatoms, each being wiped out by the next storm (Lacour et al., 2017). It was hypothesized that these previously unknown transient blooms potentially fueled the mesopelagic ecosystem and contributed to the downward carbon flux, at a period when biological fluxes are expected to be very low or nil. Hence, the unexpected periods of shallower mixed layer during the harsh North Atlantic winter were accompanied by yet unknown ephemeral phytoplankton blooms, which could be a new BCP mechanism.
Because of iron limitation, the Southern Ocean is considered to be essentially a High Nutrient Low Chlorophyl (HNLC) system, except in some specific areas where there is local iron delivery (e.g., downstream of shallow areas, and near ice melting areas Ardyna et al., 2017). It was therefore not anticipated to register any significant phytoplankton blooms in typically HLNC open ocean waters. Hence, the float-based observations of two massive blooms in low iron “oligotrophic” waters of the Southern Ocean came as a surprise (Ardyna et al., 2019). These blooms occurred downstream of active hydrothermal vents along the Southwest Indian Ridge more than 3000 m below the ocean surface, and were triggered by the mixing of these waters into the upper layer caused by flow-topography interactions. Hence, the unexpected observation of hotspots of high phytoplankton production in the oligotrophic Southern Ocean, fueled by the release of iron thousands of meters below the ocean surface, led to the discovery of a new mechanism of biological production in oligotrophic areas, with potential contribution to the BCP.
Glider transects crossing mesoscale eddies in the North Atlantic during the spring bloom showed anomalous biogeochemical features in the upper twilight zone (Omand et al., 2015). These occurred in a layer comprised between 100 and 350 m where elevated POC, Chla and oxygen concentrations were recorded. High resolution modeling confirmed that these anomalies were the signature of subduction processes at the sub-mesoscale, corresponding to active transport of surface waters and their biogenic content through 1–10 km-long filaments generated at eddy periphery. The authors developed parametrization of the eddy-driven POC flux to upscale such eddy-driven subduction flux events to the global ocean, and showed that these events could be of similar magnitude as the carbon flux associated with the biological gravitational pump during the spring bloom period in the subpolar ocean. Later on and using the same principle of detecting anomalies in multidisciplinary BGC-Argo profiles in the Southern Ocean, (Llort et al., 2019) concluded to a lower average contribution of eddy subduction to the export flux, although some extremely strong events could be of singular importance at the local scale. Hence, the observation of anomalous biogeochemical features in the upper TZ led to the discovery of a yet unknown physically driven BCP mechanism, now called eddy subduction pump (see sections “Introduction: The Ocean Biological Carbon Pump, a Paradigm Shift” and “Eddy Subduction Pump”).
The above five examples show how the large-scale and long-term deployment of modern sensors in different areas of the global ocean as well as the new interdisciplinary approach to the resulting data has led to exciting discoveries in recent years. It is thus expected that the research community approach described in this paper will not only bring together a large community of biological, chemical and physical oceanographers and marine biogeochemists and sedimentologists, but also usher the community in a new era of discoveries in ocean sciences.
Take-Home Points
Key points concerning the implementation of the research observational community approach proposed in this paper are as follow:
• The downward pumping of biogenic carbon in the ocean is performed by the combined action of six biological carbon pumps (BCPs) (see section “Introduction: The Ocean Biological Carbon Pump, a Paradigm Shift”).
• Here we propose a framework for an integrated community observational framework to investigate the BCPs in the World Ocean, in order to better quantify global estimates of carbon storage in the oceans and the underlying the drivers of each BCP (see section “Introduction: The Ocean Biological Carbon Pump, a Paradigm Shift”).
• The framework would involve biological, chemical and physical oceanographers and marine biogeochemists and sedimentologists, using field observations at sea, satellite remote sensing, and modeling (see section “Introduction: The Ocean Biological Carbon Pump, a Paradigm Shift”).
• The framework would combine measurements from several observational platforms, which presently include research vessels, satellites, instrumented moorings, and robots (i.e., gliders, floats, and USVs) (see section “Introduction: The Ocean Biological Carbon Pump, a Paradigm Shift”).
• Methods and sensors already exist to measure the key variables and processes in the euphotic and twilight zones, and have already been implemented on at least one of the observation platforms (see section “Biological Carbon Pump Variables and Processes to be Measured”).
• The characteristics of the various BCPs would be measured at a variety of locations over several years in order to capture their variability and the sequence of their occurrences and magnitudes according to regions and seasons (see section “Spatial and Temporal Scales of Occurrence of the Various Pumps”).
• Sampling would be conducted in representative regions, which could be selected based on a number of alternative or complementary approaches (see section “Selecting Representative Regions and Sampling Depths for Integrated Studies of the Pumps”).
• Implementing the observational strategy would require the acquisition of comprehensive and integrated time series of multidisciplinary variables and processes in the different sampling regions, the duration of the time series being at least one year and ideally several years (see section “Implementing the Paradigm Shift: A Multiplatform Observation Strategy”).
• Community synergies would be developed for investigating the BCPs, such as implementing the proposed studies in regions or at locations where long-term observing programs are conducted by the international oceanographic community (see section “Implementing the Paradigm Shift: A Multiplatform Observation Strategy”).
• Assessing the spatio-temporal variability of BCPs at the scale of the global ocean would require upscaling the results of regional observations to the global ocean, which could be achieved through mechanistic modeling and artificial-intelligence-based relationships applied to large datasets (see section “From Regional Knowledge to Global Scale”).
• The proposed research community approach would not only bring together a large community of biological, chemical and physical oceanographers and marine biogeochemists and sedimentologists, but also usher the community in a new era of discoveries in ocean sciences (see section “Toward a New Era of Discoveries”).
Author Contributions
The manuscript was drafted by HC and LL, and all authors contributed to its improvement and final form.
Funding
This study was funded by a European Research Council Advanced Grant to HC for the REFINE project (Grant Agreement 834177), a Laureate Fellowship of the Australian Research Council to PB (FL160100131). ML acknowledges support from CNES and Agence Nationale de Recherche (ANR-16-CE01-0014).
Conflict of Interest
The authors declare that the research was conducted in the absence of any commercial or financial relationships that could be construed as a potential conflict of interest.
Publisher’s Note
All claims expressed in this article are solely those of the authors and do not necessarily represent those of their affiliated organizations, or those of the publisher, the editors and the reviewers. Any product that may be evaluated in this article, or claim that may be made by its manufacturer, is not guaranteed or endorsed by the publisher.
Acknowledgments
We thank Thomas Boniface for drafting Figure 1.
Supplementary Material
The Supplementary Material for this article can be found online at: https://www.frontiersin.org/articles/10.3389/fmars.2021.780052/full#supplementary-material
Footnotes
References
Ajayi, A., Le Sommer, J., Chassignet, E., Molines, J.-M., Xu, X., Albert, A., et al. (2020). Spatial and temporal variability of the North Atlantic eddy field from two kilometric-resolution ocean models. J. Geophys. Res. Oceans 125:e2019JC015827. doi: 10.1029/2019JC015827
Alvain, S., Moulin, C., Dandonneau, Y., and Breon, F. M. (2005). Remote sensing of phytoplankton groups in case 1 waters from global SeaWiFS imagery. Deep Sea Res. I Oceanogr. Res. Pap. 52, 1989–2004. doi: 10.1016/J.Dsr.2005.06.015
Ardyna, M., Claustre, H., Sallee, J. B., D’Ovidio, F., Gentili, B., van Dijken, G., et al. (2017). Delineating environmental control of phytoplankton biomass and phenology in the Southern Ocean. Geophys. Res. Lett. 44, 5016–5024. doi: 10.1002/2016gl072428
Ardyna, M., Lacour, L., Sergi, S., d’Ovidio, F., Sallée, J. B., Rembauville, M., et al. (2019). Hydrothermal vents trigger massive phytoplankton blooms in the Southern Ocean. Nat. Commun. 10:2451. doi: 10.1038/s41467-019-09973-6
Aumont, O., Ethe, C., Tagliabue, A., Bopp, L., and Gehlen, M. (2015). PISCES-v2: an ocean biogeochemical model for carbon and ecosystem studies. Geosci. Model Dev. 8, 2465–2513. doi: 10.5194/gmd-8-2465-2015
Basedow, S. L., McKee, D., Lefering, I., Gislason, A., Daase, M., Trudnowska, E., et al. (2019). Remote sensing of zooplankton swarms. Sci. Rep. 9:686. doi: 10.1038/s41598-018-37129-x
Behrenfeld, M. J., Gaube, P., Della Penna, A., O’Malley, R. T., Burt, W. J., Hu, Y. X., et al. (2019). Global satellite-observed daily vertical migrations of ocean animals. Nature 576, 257–261. doi: 10.1038/s41586-019-1796-9
Bianchi, D., Galbraith, E. D., Carozza, D. A., Mislan, K. A. S., and Stock, C. A. (2013). Intensification of open-ocean oxygen depletion by vertically migrating animals. Nat. Geosci. 6, 545–548. doi: 10.1038/ngeo1837
Biard, T., Stemmann, L., Picheral, M., Mayot, N., Vandromme, P., Hauss, H., et al. (2016). In situ imaging reveals the biomass of giant protists in the global ocean. Nature 532, 504–507. doi: 10.1038/nature17652
Bif, M. B., and Hansell, D. A. (2019). Seasonality of dissolved organic carbon in the upper Northeast Pacific Ocean. Glob. Biogeochem. Cycles 33, 526–539. doi: 10.1029/2018GB006152
Bishop, J. K. B. (2009). Autonomous observations of the ocean biological carbon pump. Oceanography 22, 182–193.
Bishop, J. K. B., and Wood, T. J. (2009). Year-round observations of carbon biomass and flux variability in the Southern Ocean. Glob. Biogeochem. Cycles 23:GB2019. doi: 10.1029/2008gb003206
Bishop, J. K. B., Wood, T. J., Davis, R. E., and Sherman, J. T. (2004). Robotic observations of enhanced carbon biomass and export at 55 degrees S during SOFeX. Science 304, 417–420. doi: 10.1126/science.1087717
Bittig, H. C., Steinhoff, T., Claustre, H., Fiedler, B., Williams, N. L., Sauzède, R., et al. (2018). An alternative to static climatologies: robust estimation of open Ocean CO2 Variables and nutrient concentrations from T, S, and O2 data using bayesian neural networks. Front. Mar. Sci. 5:328. doi: 10.3389/fmars.2018.00328
Bock, N., Cornec, M., Claustre, H., and Duhamel, S. (2021). Biogeographical classification of the global ocean from BGC-Argo floats. Glob. Biogeochem. Cycles [Epub ahead of print].
Bol, R., Henson, S. A., Rumyantseva, A., and Briggs, N. (2018). High-frequency variability of small-particle carbon export flux in the Northeast Atlantic. Glob. Biogeochem. Cycles 32, 1803–1814. doi: 10.1029/2018GB005963
Bopp, L., Resplandy, L., Orr, J. C., Doney, S. C., Dunne, J. P., Gehlen, M., et al. (2013). Multiple stressors of ocean ecosystems in the 21st century: projections with CMIP5 models. Biogeosciences 10, 6225–6245. doi: 10.5194/bg-10-6225-2013
Boss, E., and Behrenfeld, M. (2010). In situ evaluation of the initiation of the North Atlantic phytoplankton bloom. Geophys. Res. Lett. 37:e044174. doi: 10.1029/2010gl044174
Boyd, P. W., Claustre, H., Levy, M., Siegel, D. A., and Weber, T. (2019). Multi-faceted particle pumps drive carbon sequestration in the ocean. Nature 568, 327–335. doi: 10.1038/s41586-019-1098-2
Boyd, P. W., Jickells, T., Law, C. S., Blain, S., Boyle, E. A., Buesseler, K. O., et al. (2007). Mesoscale iron enrichment experiments 1993-2005: synthesis and future directions. Science 315:612. doi: 10.1126/science.1131669
Boyd, P. W., Lennartz, S. T., Glover, D. M., and Doney, S. C. (2015). Biological ramifications of climate-change-mediated oceanic multi-stressors. Nat. Clim. Change 5, 71–79. doi: 10.1038/nclimate2441
Boyd, P. W., and Newton, P. P. (1999). Does planktonic community structure determine downward particulate organic carbon flux in different oceanic provinces? Deep Sea Res. I Oceanogr. Res. Pap. 46, 63–91. doi: 10.1016/S0967-0637(98)00066-1
Bracher, A., Bouman, H. A., Brewin, R. J. W., Bricaud, A., Brotas, V., Ciotti, A. M., et al. (2017). Obtaining phytoplankton diversity from ocean color: a scientific roadmap for future development. Front. Mar. Sci. 4:55. doi: 10.3389/fmars.2017.00055
Brewin, R. J. W., Sathyendranath, S., Platt, T., Bouman, H., Ciavatta, S., Dall’Olmo, G., et al. (2021). Sensing the ocean biological carbon pump from space: a review of capabilities, concepts, research gaps and future developments. Earth Sci. Rev. 217:103604. doi: 10.1016/j.earscirev.2021.103604
Briggs, E. M., Martz, T. R., Talley, L. D., Mazloff, M. R., and Johnson, K. S. (2018). Physical and biological drivers of biogeochemical tracers within the seasonal Sea Ice Zone of the Southern ocean from profiling floats. J. Geophys. Res. Oceans 123, 746–758. doi: 10.1002/2017JC012846
Briggs, N., Dall’Olmo, G., and Claustre, H. (2020). Major role of particle fragmentation in regulating biological sequestration of CO2 by the oceans. Science 367:791. doi: 10.1126/science.aay1790
Briggs, N., Perry, M. J., Cetinic, I., Lee, C., D’Asaro, E., Gray, A. M., et al. (2011). High-resolution observations of aggregate flux during a sub-polar North Atlantic spring bloom. Deep Sea Res. I Oceanogr. Res. Pap. 58, 1031–1039. doi: 10.1016/j.dsr.2011.07.007
Briggs, N., Slade, W. H., Boss, E., and Perry, M. J. (2013). Method for estimating mean particle size from high-frequency fluctuations in beam attenuation or scattering measurements. Appl. Optics 52, 6710–6725. doi: 10.1364/ao.52.006710
Buesseler, K. O., Benitez-Nelson, C., van der loeff, M. R., Andrews, J., Ball, L., Crossin, G., et al. (2001). An intercomparison of small- and large-volume techniques for thorium-234 in seawater. Mar. Chem. 74, 15–28.
Buesseler, K. O., and Boyd, P. W. (2009). Shedding light on processes that control particle export and flux attenuation in the twilight zone of the open ocean. Limnol. Oceanogr. 54, 1210–1232. doi: 10.4319/lo.2009.54.4.1210
Buesseler, K. O., Boyd, P. W., Black, E. E., and Siegel, D. A. (2020). Metrics that matter for assessing the ocean biological carbon pump. Proc. Natl. Acad. Sci. U.S.A. 117, 9679–9687. doi: 10.1073/pnas.1918114117
Catul, V., Gauns, M., and Karuppasamy, P. K. (2011). A review on mesopelagic fishes belonging to family Myctophidae. Rev. Fish Biol. Fish. 21, 339–354. doi: 10.1007/s11160-010-9176-4
Cavan, E. L., Trimmer, M., Shelley, F., and Sanders, R. (2017). Remineralization of particulate organic carbon in an ocean oxygen minimum zone. Nat. Commun. 8:14847. doi: 10.1038/ncomms14847
CEOS (2014). Strategy for Carbon Observations from Space. The Committee on Earth Observation Satellites (CEOS). Response to the Group on Earth Observations (GEO) Carbon Strategy. Chōfu: JAXA and I&A Corporation.
Cetinic, I., Perry, M. J., Briggs, N. T., Kallin, E., D’Asaro, E. A., and Lee, C. M. (2012). Particulate organic carbon and inherent optical properties during 2008 North Atlantic bloom experiment. J. Geophys. Res. Oceans 117:C06028. doi: 10.1029/2011jc007771
Cetinic, I., Perry, M. J., D’Asaro, E., Briggs, N., Poulton, N., Sieracki, M. E., et al. (2015). A simple optical index shows spatial and temporal heterogeneity in phytoplankton community composition during the 2008 North Atlantic Bloom Experiment. Biogeosciences 12, 2179–2194. doi: 10.5194/bg-12-2179-2015
Chai, F., Johnson, K. S., Claustre, H., Xing, X., Wang, Y., Boss, E., et al. (2020). Monitoring ocean biogeochemistry with autonomous platforms. Nat. Rev. Earth Environ. 1, 315–326. doi: 10.1038/s43017-020-0053-y
Chase, A. P., Kramer, S. J., Haëntjens, N., Boss, E. S., Karp-Boss, L., Edmondson, M., et al. (2020). Evaluation of diagnostic pigments to estimate phytoplankton size classes. Limnol. Oceanogr. Methods [Epub ahead of print]. doi: 10.1002/lom3.10385
Christiansen, S., Hoving, H. J., Schutte, F., Hauss, H., Karstensen, J., Kortzinger, A., et al. (2018). Particulate matter flux interception in oceanic mesoscale eddies by the polychaete Poeobius sp. Limnol. Oceanogr. 63, 2093–2109. doi: 10.1002/lno.10926
Chu, D., Parker-Stetter, S., Hufnagle, L. C., Thomas, R., Getsiv-Clemons, J., Gauthier, S., et al. (2019). 2018 Unmanned Surface Vehicle (Saildrone) acoustic survey off the west coasts of the United States and Canada. Paper presented at OCEANS 2019 MTS/IEEE SEATTLE, 27-31 Oct. 2019, (Piscataway, NJ: IEEE).
Claustre, H., Johnson, K. S., and Takeshita, Y. (2020). Observing the Global Ocean with biogeochemical-argo. Annu. Rev. Mar. Sci. 12, 23–48. doi: 10.1146/annurev-marine-010419-010956
Cohen, J. H., and Forward, R. B. (2009). “Zooplankton diel vertical migration - a review of proximate control,” in Oceanography and Marine Biology: An Annual Review, ed. S. J. Hawkins (Boca Raton, FL: Taylor & Francis), 77–109. doi: 10.1201/9781420094220.ch2
Copin-Montegut, G., and Avril, B. (1993). Vertical distribution and temporal variation of dissolved organic carbon in the North-Wtesren Mediterranean Sea. Deep Sea Res. I 40, 1963–1972. doi: 10.1016/0967-0637(93)90041-Z
Cottier, F. R., Tarling, G. A., Wold, A., and Falk-Petersen, S. (2006). Unsynchronised and synchronised vertical migration of zooplankton in a high Arctic fjord. Limnol. Oceanogr. 51, 2586–2599. doi: 10.4319/lo.2006.51.6.2586
Dall’Olmo, G., Dingle, J., Polimene, L., Brewin, R. J. W., and Claustre, H. (2016). Substantial energy input to the mesopelagic ecosystem from the seasonal mixed-layer pump. Nat. Geosci. 9, 820–823. doi: 10.1038/ngeo2818
Dall’Olmo, G., and Mork, K. A. (2014). Carbon export by small particles in the Norwegian Sea. Geophys. Res. Lett. 41, 2921–2927. doi: 10.1002/2014gl059244
De Robertis, A., Levine, M., Lauffenburger, N., Honkalehto, T., Ianelli, J., Monnahan, C. C., et al. (2021). Uncrewed surface vehicle (USV) survey of walleye pollock, Gadus chalcogrammus, in response to the cancellation of ship-based surveys. ICES J. Mar. Sci. 78, 2797–2808. doi: 10.1093/icesjms/fsab155
DeVries, T., and Weber, T. (2017). The export and fate of organicmatter in the ocean: new constraints from combining satellite and oceanographic tracer observations. Glob. Biogeochem. Cycles 31, 535–555. doi: 10.1002/2016GB005551
deYoung, B., Visbeck, M., de Araujo Filho, M. C., Baringer, M. O., Black, C. A., Buch, E., et al. (2019). An integrated all-atlantic ocean observing system in 2030. Front. Mar. Sci. 6:428. doi: 10.3389/fmars.2019.00428
D’Ortenzio, F., Antoine, D., Martinez, E., and d’Alcala, M. R. (2012). Phenological changes of oceanic phytoplankton in the 1980s and 2000s as revealed by remotely sensed ocean-color observations. Glob. Biogeochem. Cycles 26:GB4003. doi: 10.1029/2011gb004269
D’Ortenzio, F., and d’Alcala, M. R. (2009). On the trophic regimes of the Mediterranean Sea: a satellite analysis. Biogeosciences 6, 139–148.
D’Ortenzio, F., Taillandier, V., Claustre, H., Prieur, L., Leymarie, E., Mignot, A., et al. (2020). Biogeochemical argo: the test case of the NAOS mediterranean array. Front. Mar. Sci. 7:120. doi: 10.3389/fmars.2020.00120
Druon, J.-N., Hélaouët, P., Beaugrand, G., Fromentin, J.-M., Palialexis, A., and Hoepffner, N. (2019). Satellite-based indicator of zooplankton distribution for global monitoring. Sci. Rep. 9:4732. doi: 10.1038/s41598-019-41212-2
Ducklow, H. W., and Harris, R. P. (1993). Introduction to the JGOFS North Atlantic bloom experiment. Deep Sea Res. II Top. Stud. Oceanogr. 40, 1–8. doi: 10.1016/0967-0645(93)90003-6
Duteil, O., Lazar, A., Dandonneau, Y., Wainer, I., and Menkes, C. (2009). Deep chlorophyll maximum and upper ocean structure interactions: case of the Guinea Thermal Dome. J. Mar. Res. 67, 239–271. doi: 10.1357/002224009789051191
Dutkiewicz, S., Hickman, A. E., Jahn, O., Gregg, W. W., Mouw, C. B., and Follows, M. J. (2015). Capturing optically important constituents and properties in a marine biogeochemical and ecosystem model. Biogeosciences 12, 4447–4481. doi: 10.5194/bg-12-4447-2015
El Hourany, R., Saab, M. A. A., Faour, G., Aumont, O., Crepon, M., and Thiria, S. (2019). Estimation of secondary phytoplankton pigments from satellite observations using self-organizing maps (SOMs). J. Geophys. Res.-Oceans 124, 1357–1378. doi: 10.1029/2018JC014450
Estapa, M. L., Buesseler, K., Boss, E., and Gerbi, G. (2013). Autonomous, high-resolution observations of particle flux in the oligotrophic ocean. Biogeosciences 10, 5517–5531. doi: 10.5194/bg-10-5517-2013
Estapa, M. L., Durkin, C., Buesseler, K., Johnson, R., and Feen, M. (2017). Carbon flux from bio-optical profiling floats: calibrating transmissometers for use as optical sediment traps. Deep Sea Res. I 120, 100–111. doi: 10.1016/j.dsr.2016.12.003
Estapa, M. L., Feen, M. L., and Breves, E. (2019). Direct observations of biological carbon export from profiling floats in the subtropical North Atlantic. Glob. Biogeochem. Cycles 23, 282–300. doi: 10.1029/2018GB006098
Giering, S. L. C., Sanders, R., Lampitt, R. S., Anderson, T. R., Tamburini, C., Boutrif, M., et al. (2014). Reconciliation of the carbon budget in the ocean’s twilight zone. Nature 507, 480–483. doi: 10.1038/nature13123
Giorli, G., Drazen, J. C., Neuheimer, A. B., Copeland, A., and Au, W. W. L. (2018). Deep sea animal density and size estimated using a Dual-frequency IDentification SONar (DIDSON) offshore the island of Hawaii. Prog. Oceanogr. 160, 155–166. doi: 10.1016/j.pocean.2018.01.002
Goulet, P., Guinet, C., Swift, R. N., Madsen, P. T., and Johnson, M. W. (2019). A miniature biomimetic sonar and movement tag to study the biotic environment and predator-prey interactions in aquatic animals. Deep Sea Res. I Oceanogr. Res. Pap. 148, 1–11. doi: 10.1016/j.dsr.2019.04.007
Groom, S., Sathyendranath, S., Ban, Y., Bernard, S., Brewin, R., Brotas, V., et al. (2019). Satellite Ocean colour: current status and future perspective. Front. Mar. Sci. 6:485.
Gruber, N., and Sarmiento, J. (2002). Large-scale biogeochemical-physical interactions in elemental cycles. Sea 12, 337–399.
Guay, C. K. H., and Bishop, J. K. B. (2002). A rapid birefringence method for measuring suspended CaCO3 concentrations in seawater. Deep Sea Res. I Oceanogr. Res. Pap. 49, 197–210. doi: 10.1016/s0967-0637(01)00049-8
Guidi, L., Chaffron, S., Bittner, L., Eveillard, D., Larhlimi, A., Roux, S., et al. (2016). Plankton networks driving carbon export in the oligotrophic ocean. Nature 532, 465–469. doi: 10.1038/nature16942
Guidi, L., Legendre, L., Reygondeau, G., Uitz, J., Stemmann, L., and Henson, S. A. (2015). A new look at ocean carbon remineralization for estimating deepwater sequestration. Glob. Biogeochem. Cycles 29, 1044–1059. doi: 10.1002/2014gb005063
Guidi, L., Stemmann, L., Jackson, G. A., Ibanez, F., Claustre, H., Legendre, L., et al. (2009). Effects of phytoplankton community on production, size and export of large aggregates: a world-ocean analysis. Limnol. Oceanogr. 54, 1951–1963. doi: 10.4319/lo.2009.54.6.1951
Haentjens, N., Boss, E., and Talley, L. D. (2017). Revisiting Ocean Color algorithms for chlorophyll a and particulate organic carbon in the Southern Ocean using biogeochemical floats. J. Geophys. Res. Oceans 122, 6583–6593. doi: 10.1002/2017jc012844
Haentjens, N., Della Penna, A., Briggs, N., Karp-Boss, L., Gaube, P., Claustre, H., et al. (2020). Detecting mesopelagic organisms using biogeochemical-Argo floats. Geophys. Res. Lett. 47:e2019GL086088. doi: 10.1029/2019GL086088
Hansell, D. A. (2013). Recalcitrant dissolved organic carbon fractions. Annu. Rev. Mar. Sci. 5, 421–445. doi: 10.1146/annurev-marine-120710-100757
Hardman-Mountford, N. J., Hirata, T., Richardson, K. A., and Aiken, J. (2008). An objective methodology for the classification of ecological pattern into biomes and provinces for the pelagic ocean. Remote Sens. Environ. 112, 3341–3352. doi: 10.1016/j.rse.2008.02.016
Henson, S. A., Sanders, R., and Madsen, E. (2012). Global patterns in efficiency of particulate organic carbon export and transfer to the deep ocean. Glob. Biogeochem. Cycles 26:1028. doi: 10.1029/2011gb004099
Hermes, J. C., Masumoto, Y., Beal, L. M., Roxy, M. K., Vialard, J., Andres, M., et al. (2019). A sustained ocean observing system in the Indian Ocean for climate related scientific knowledge and societal needs. Front. Mar. Sci. 6:355. doi: 10.3389/fmars.2019.00355
Honjo, S., Manganini, S. J., Krishfield, R. A., and Francois, R. (2008). Particulate organic carbon fluxes to the ocean interior and factors controlling the biological pump: a synthesis of global sediment trap programs since 1983. Prog. Oceanogr. 76, 217–285. doi: 10.1016/j.pocean.2007.11.003
Hoving, H. J., Christiansen, S., Fabrizius, E., Hauss, H., Kiko, R., Linke, P., et al. (2019). The Pelagic In situ Observation System (PELAGIOS) to reveal biodiversity, behavior, and ecology of elusive oceanic fauna. Ocean Sci. 15, 1327–1340. doi: 10.5194/os-15-1327-2019
Ikeda, T. (2014). Respiration and ammonia excretion by marine metazooplankton taxa: synthesis toward a global-bathymetric model. Mar. Biol. 161, 2753–2766. doi: 10.1007/s00227-014-2540-5
IOCCG (2014). “Phytoplankton functional types from space,” in Reports of the International Ocean-Colour Coordinating Group, No. 15, ed. Sathyendranath, S. (Dartmouth: IOCCG).
IPCC (2019). “Annex I: glossary, van Diemen, R. (Coordinating Editor),” in Climate Change and Land: An IPCC Special Report on Climate Change, Desertification, Land Degradation, Sustainable Land Management, Food Security, and Greenhouse Gas Fluxes in Terrestrial Ecosystems, eds J. S. P. R. Shukla, E. Calvo Buendia, V. Masson-Delmotte, H.-O. Pörtner, D. C. Roberts, P. Zhai, et al. (Geneva: IPCC).
Jamet, C., Ibrahim, A., Ahmad, Z., Angelini, F., Babin, M., Behrenfeld, M. J., et al. (2019). Going beyond standard ocean color observations: lidar and polarimetry. Front. Mar. Sci. 6:251. doi: 10.3389/fmars.2019.00251
Jiao, N. Z., Herndl, G. J., Hansell, D. A., Benner, R., Kattner, G., Wilhelm, S. W., et al. (2011). The microbial carbon pump and the oceanic recalcitrant dissolved organic matter pool. Nat. Rev. Microbiol. 9:555. doi: 10.1038/nrmicro2386-c5
Jonasdottir, S. H., Visser, A. W., Richardson, K., and Heath, M. R. (2015). Seasonal copepod lipid pump promotes carbon sequestration in the deep North Atlantic. Proc. Natl. Acad. Sci. U.S.A. 112, 12122–12126. doi: 10.1073/pnas.1512110112
Keerthi, M. G., Lévy, M., and Aumont, O. (2021). Intermittency in phytoplankton bloom triggered by modulations in vertical stability. Sci. Rep. 11:1285. doi: 10.1038/s41598-020-80331-z
Keppler, L., Landschützer, P., Gruber, N., Lauvset, S. K., and Stemmler, I. (2020). Seasonal carbon dynamics in the Near-Global Ocean. Glob. Biogeochem. Cycles 34:e2020GB006571. doi: 10.1029/2020GB006571
Kheireddine, M., Dall’Olmo, G., Ouhssain, M., Krokos, G., Claustre, H., Schmechtig, C., et al. (2020). Organic carbon export and loss rates in the Red Sea. Glob. Biogeochem. Cycles 34:e2020GB006650.
Kiko, R., Brandt, P., Christiansen, S., Faustmann, J., Kriest, I., Rodrigues, E., et al. (2020). Zooplankton-mediated fluxes in the eastern tropical North Atlantic. Front. Mar. Sci. 7:358. doi: 10.3389/fmars.2020.00358
Kiko, R., and Hauss, H. (2019). On the estimation of zooplankton-mediated active fluxes in oxygen minimum zone regions. Front. Mar. Sci. 6:741. doi: 10.3389/fmars.2019.00741
Kobari, T., Inoue, Y., Nakamura, Y., Okamura, H., Ota, T., Nishibe, Y., et al. (2010). Feeding impacts of ontogenetically migrating copepods on the spring phytoplankton bloom in the Oyashio region. Deep Sea Res. II Top. Stud. Oceanogr. 57, 1703–1714. doi: 10.1016/j.dsr2.2010.03.014
Kostadinov, T. S., Siegel, D. A., and Maritorena, S. (2009). Retrieval of the particle size distribution from satellite ocean color observations. J. Geophys. Res. Oceans 114:C09015. doi: 10.1029/2009jc005303
Kostadinov, T. S., Siegel, D. A., and Maritorena, S. (2010). Global variability of phytoplankton functional types from space: assessment via the particle size distribution. Biogeosciences 7, 3239–3257. doi: 10.5194/bg-7-3239-2010
Kwon, E. Y., Primeau, F., and Sarmiento, J. L. (2009). The impact of remineralization depth on the air-sea carbon balance. Nat. Geosci. 2, 630–635. doi: 10.1038/ngeo612
Lacour, L., Ardyna, M., Stec, K. F., Claustre, H., Prieur, L., Poteau, A., et al. (2017). Unexpected winter phytoplankton blooms in the North Atlantic subpolar gyre. Nat. Geosci. 10, 836–839. doi: 10.1038/ngeo3035
Lacour, L., Briggs, N., Claustre, H., Ardyna, M., and Dall’Olmo, G. (2019). The intra-seasonal dynamics of the mixed layer pump in the subpolar North Atlantic Ocean: a BGC-Argo float approach. Glob. Biogeochem. Cycles 33, 266–281. doi: 10.1029/2018GB005997
Landschutzer, P., Gruber, N., and Bakker, D. C. E. (2016). Decadal variations and trends of the global ocean carbon sink. Glob. Biogeochem. Cycles 30, 1396–1417. doi: 10.1002/2015gb005359
Le Quéré, C., Harrison, S. P., Prentice, I. C., Buitenhuis, E. T., Aumont, O., Bopp, L., et al. (2005). Ecosystem dynamics based on plankton functional types for global ocean biogeochemistry models. Glob. Change Biol. 11, 2016–2040. doi: 10.1111/j.1365-2468.2005.01004.x
Le Traon, P. Y., D’Ortenzio, F., Babin, M., Leymarie, E., Marec, C., Pouliquen, S., et al. (2020). Preparing the new phase of argo: scientific achievements of the NAOS project. Front. Mar. Sci. 7:838. doi: 10.3389/fmars.2020.577408
Legendre, L., and Lefevre, J. (1995). Microbial food webs and the export of biogenic carbon in Oceans. Aquat. Microb. Ecol. 9, 69–77. doi: 10.3354/ame009069
Legendre, L., and Rivkin, R. B. (2005). Integrating functional diversity, food web processes, and biogeochemical carbon fluxes into a conceptual approach for modeling the upper ocean in a high-CO2 world. J. Geophys. Res. 110:C09S17. doi: 10.1029/2004JC002530
Legendre, L., Rivkin, R. B., Weinbauer, M. G., Guidi, L., and Uitz, J. (2015). The microbial carbon pump concept: potential biogeochemical significance in the globally changing ocean. Prog. Oceanogr. 134, 432–450. doi: 10.1016/j.pocean.2015.01.008
Leung, S. W., Weber, T., Cram, J. A., and Deutsch, C. (2021). Variable particle size distributions reduce the sensitivity of global export flux to climate change. Biogeosciences 18, 229–250. doi: 10.5194/bg-18-229-2021
Levy, M., Bopp, L., Karleskind, P., Resplandy, L., Ethe, C., and Pinsard, F. (2013). Physical pathways for carbon transfers between the surface mixed layer and the ocean interior. Glob. Biogeochem. Cycles 27, 1001–1012. doi: 10.1002/gbc.20092
Levy, M., Memery, L., and Andre, J. M. (1998). Simulation of primary production and export fluxes in the Northwestern Mediterranean Sea. J. Mar. Res. 56, 197–238. doi: 10.1357/002224098321836163
Llort, J., Langlais, C., Matear, R., Moreau, S., Lenton, A., and Strutton, P. G. (2018). Evaluating southern ocean carbon eddy-pump from biogeochemical argo floats. J. Geophys. Res. Oceans 123, 971–984. doi: 10.1002/2017JC012861
Llort, J., Lévy, M., Sallée, J. B., and Tagliabue, A. (2019). Nonmonotonic response of primary production and export to changes in mixed-layer depth in the Southern Ocean. Geophys. Res. Lett. 46, 3368–3377. doi: 10.1029/2018GL081788
Mariani, G., Cheung William, W. L., Lyet, A., Sala, E., Mayorga, J., Velez, L., et al. (2020). Let more big fish sink: fisheries prevent blue carbon sequestration—half in unprofitable areas. Sci. Adv. 6:eabb4848. doi: 10.1126/sciadv.abb4848
Martin, A., Boyd, P., Buesseler, K., Cetinic, I., Claustre, H., Giering, S., et al. (2020). The oceans’ twilight zone must be studied now, before it is too late. Nature 580, 26–28. doi: 10.1038/d41586-020-00915-7
Martin, J. H., Knauer, G. A., Karl, D. M., and Broenkow, W. W. (1987). VERTEX - Carbon cycling in the Northeast Pacific. Deep Sea Res. A Oceanogr. Res. Pap. 34, 267–285. doi: 10.1016/0198-0149(87)90086-0
Michaels, A. F., and Silver, M. W. (1988). Primary production, sinking fluxes and the microbial food web. Deep Sea Res. A Oceanogr. Res. Pap. 35, 473–490. doi: 10.1016/0198-0149(88)90126-4
Mignot, A., Ferrari, R., and Claustre, H. (2018). Floats with bio-optical sensors reveal what processes trigger the North Atlantic bloom. Nat. Commun. 9:190. doi: 10.1038/s41467-017-02143-6
Mordy, C. W., Cokelet, E. D., De Robertis, A., Jenkins, R., Kuhn, C. E., Lawrence-Slavas, N., et al. (2017). Advances in ecosystem research: saildrone surveys of oceanography, fish, and marine mammals in the Bering Sea. Oceanography 30, 113–115. doi: 10.5670/oceanog.2017.230
Moreau, S., Boyd, P. W., and Strutton, P. G. (2020). Remote assessment of the fate of phytoplankton in the Southern Ocean sea-ice zone. Nat. Commun. 11:3108. doi: 10.1038/s41467-020-16931-0
Morrow, R., Fu, L.-L., Ardhuin, F., Benkiran, M., Chapron, B., Cosme, E., et al. (2019). Global observations of fine-scale ocean surface topography with the Surface Water And Ocean Topography (SWOT) mission. Front. Mar. Sci. 6:232. doi: 10.3389/fmars.2019.00232
Nayak, A. R., and Twardowski, M. (2020). ‘Breaking’ news : particle fragmentation is a missing piece of the ocean carbon budget. Science 367, 738–739. doi: 10.1126/science.aba7109
Omand, M. M., D’Asaro, E. A., Lee, C. M., Perry, M. J., Briggs, N., Cetinic, I., et al. (2015). Eddy-driven subduction exports particulate organic carbon from the spring bloom. Science 348, 222–225. doi: 10.1126/science.1260062
Palevsky, H. I., and Doney, S. C. (2021). Sensitivity of 21st century ocean carbon export flux projections to the choice of export depth horizon. Glob. Biogeochem. Cycles 35:e2020GB006790. doi: 10.1029/2020GB006790
Pershing, A. J., Christensen, L. B., Record, N. R., Sherwood, G. D., and Stetson, P. B. (2010). The impact of whaling on the ocean carbon cycle: why bigger was better. PLoS One 5:e12444. doi: 10.1371/journal.pone.0012444
Picheral, M., Berry, F., Brousseau, D., Fevre, S., Abidi, E., Coindat, J., et al. (2021). UVP6: underwater imaging sensor of particle size spectra and plankton, for autonomous and remote platforms. Limnol. Oceanogr. Methods. [Epub ahead of print].
Picheral, M., Guidi, L., Stemmann, L., Karl, D. M., Iddaoud, G., and Gorsky, G. (2010). The underwater vision profiler 5: an advanced instrument for high spatial resolution studies of particle size spectra and zooplankton. Limnol. Oceanogr. Methods 8, 462–473. doi: 10.4319/lom.2010.8.462
Pietri, A., Capet, X., D’Ovidio, F., Levy, M., Le Sommer, J., Molines, J. M., et al. (2021). Skills and limitations of the adiabatic omega equation: how effective is it to retrieve oceanic vertical circulation at mesoscale and submesoscale? J. Phys. Oceanogr. 51, 931–954. doi: 10.1175/JPO-D-20-0052.1
Pinkel, R., Goldin, M. A., Smith, J. A., Sun, O. M., Aja, A. A., Bui, M. N., et al. (2011). The wirewalker: a vertically profiling instrument carrier powered by ocean waves. J. Atmos. Ocean. Technol. 28, 426–435. doi: 10.1175/2010JTECHO805.1
Pinti, J., DeVries, T., Norin, T., Serra-Pompei, C., Proud, R., Siegel, D. A., et al. (2021). Metazoans, migrations, and the ocean’s biological carbon pump. bioRxiv [Preprint]. doi: 10.1101/2021.03.22.436489
Plant, J. N., Johnson, K. S., Sakamoto, C. M., Jannasch, H. W., Coletti, L. J., Riser, S. C., et al. (2016). Net community production at Ocean Station Papa observed with nitrate and oxygen sensors on profiling floats. Glob. Biogeochem. Cycles 30, 859–879. doi: 10.1002/2015gb005349
Reiss, C. S., Cossio, A. M., Walsh, J. E., Cutter, G. R., and Watters, G. M. (2021). Glider-based estimates of meso-zooplankton biomass density: a fisheries case study on antarctic krill (Euphausia superba) around the Northern Antarctic Peninsula. Front. Mar. Sci. 8:256. doi: 10.3389/fmars.2021.604043
Rembauville, M., Briggs, N., Ardyna, M., Uitz, J., Catala, P., Penkerc’h, C., et al. (2017). Plankton assemblage estimated with BGC-argo floats in the Southern Ocean: implications for seasonal successions and particle export. J. Geophys. Res. Oceans 122, 8278–8292. doi: 10.1002/2017jc013067
Resplandy, L., Levy, M., and McGillicuddy, D. J. (2019). Effects of eddy-driven subduction on ocean biological carbon pump. Glob. Biogeochem. Cycles 33, 1071–1084. doi: 10.1029/2018GB006125
Reygondeau, G., Guidi, L., Beaugrand, G., Henson, S. A., Koubbi, P., MacKenzie, B. R., et al. (2018). Global biogeochemical provinces of the mesopelagic zone. J. Biogeogr. 45, 500–514. doi: 10.1111/jbi.13149
Roemmich, D., Alford, M. H., Claustre, H., Johnson, K., King, B., Moum, J., et al. (2019). On the future of argo: a global, full-depth, multi-disciplinary array. Front. Mar. Sci. 6:439. doi: 10.3389/fmars.2019.00439
Roshan, S., and DeVries, T. (2017). Efficient dissolved organic carbon production and export in the oligotrophic ocean. Nat. Commun. 8:2036. doi: 10.1038/s41467-017-02227-3
Sauzède, R., Bittig, H. C., Claustre, H., Pasqueron de Fommervault, O., Gattuso, J. P., Legendre, L., et al. (2017). Estimates of water-column nutrient concentrations and carbonate system parameters in the global ocean: a novel approach based on neural networks. Front. Mar. Sci. 4:128. doi: 10.3389/fmars.2017.00128
Sauzède, R., Claustre, H., Uitz, J., Jamet, C., Dall’Olmo, G., D’Ortenzio, F., et al. (2016). A neural network-based method for merging ocean color and Argo data to extend surface bio-optical properties to depth: retrieval of the particulate backscattering coefficient. J. Geophys. Res.-Oceans 121, 2552–2571. doi: 10.1002/2015JC011408
Sauzède, R., Johnson, J. E., Claustre, H., Camps-Valls, G., and Ruescas, A. B. (2020). Estimation of oceanic particulate organic carbon with machine learning ISPRS Annals of the Photogrammetry. Remote Sens. Spatial Inform. Sci. 2, 949–956. doi: 10.5194/isprs-annals-V-2-2020-949-2020
Send, U., Fowler, G., Siddall, G., Beanlands, B., Pittman, M., Waldmann, C., et al. (2013). SeaCycler: a moored open-ocean profiling system for the upper ocean in extended self-contained deployments. J. Atmos. Ocean. Technol. 30, 1555–1565. doi: 10.1175/JTECH-D-11-00168.1
Siegel, D. A., Buesseler, K. O., Behrenfeld, M. J., Benitez-Nelson, C. R., Boss, E., Brzezinski, M. A., et al. (2016). Prediction of the export and fate of global ocean net primary production: the EXPORTS science plan. Front. Mar. Sci. 3:22. doi: 10.3389/fmars.2016.00022
Siegel, D. A., Buesseler, K. O., Doney, S. C., Sailley, S. F., Behrenfeld, M. J., and Boyd, P. W. (2014). Global assessment of ocean carbon export by combining satellite observations and food-web models. Glob. Biogeochem. Cycles 28, 181–196. doi: 10.1002/2013gb004743
Siegel, D. A., DeVries, T., Doney, S. C., and Bell T. (2021). Assessing the sequestration time scales of some ocean-based carbon dioxide reduction strategies. Environ. Res. Lett. 16:104003. doi: 10.1088/1748-9326/ac0be0
Sloyan, B. M., Wanninkhof, R., Kramp, M., Johnson, G. C., Talley, L. D., Tanhua, T., et al. (2019). The global ocean ship-based hydrographic investigations program (GO-SHIP): a platform for integrated multidisciplinary ocean science. Front. Mar. Sci. 6:445. doi: 10.3389/fmars.2019.00445
Stukel, M. R., Aluwihare, L. I., Barbeau, K. A., Chekalyuk, A. M., Goericke, R., Miller, A. J., et al. (2017). Mesoscale ocean fronts enhance carbon export due to gravitational sinking and subduction. Proc. Natl. Acad. Sci. U.S.A. 114, 1252–1257. doi: 10.1073/pnas.1609435114
Terrats, L., Claustre, H., Cornec, M., Mangin, A., and Neukermans, G. (2020). Detection of coccolithophore blooms with BioGeoChemical-Argo floats. Geophys. Res. Lett. 47:e2020GL090559. doi: 10.1029/2020GL090559
Twardowski, M. S., Boss, E., Macdonald, J. B., Pegau, S., Barnard, A. H., and Zaneveld, J. R. (2001). A model for estimating bulk refractive index from the optical backscattering ratio and the implication for understanding particle composition in case I and case II waters. J. Geophys. Res. 106, 129–214.
Uitz, J., Claustre, H., Morel, A., and Hooker, S. B. (2006). Vertical distribution of phytoplankton communities in open ocean: an assessment based on surface chlorophyll. J. Geophys. Res. Oceans 111:C08005. doi: 10.1029/2005jc003207
Uitz, J., Stramski, D., Reynolds, R. A., and Dubranna, J. (2015). Assessing phytoplankton community composition from hyperspectral measurements of phytoplankton absorption coefficient and remote-sensing reflectance in open-ocean environments. Remote Sens. Environ. 171, 58–74. doi: 10.1016/j.rse.2015.09.027
Verdy, A., and Mazloff, M. R. (2017). A data assimilating model for estimating Southern Ocean biogeochemistry. J. Geophys. Res.-Oceans 122, 6968–6988. doi: 10.1002/2016jc012650
Visser, A. W., Grønning, J., and Jónasdóttir, S. H. (2017). Calanus hyperboreus and the lipid pump. Limnol. Oceanogr. 62, 1155–1165. doi: 10.1002/lno.10492
Voituriez, B., and Herbland, A. (1981). Primary production in the tropical atlantic ocean mapped from oxygen values of equalant 1 and 2. Bull. Mar. Sci. 31, 853–863.
Volk, T., and Hoffert, M. (1985). Ocean carbon pumps: analysis of relative strengths and efficiencies in ocean-driven atmospheric CO2 changes. Geophys. Monogr. Ser. 32, 99–110. doi: 10.1029/GM032p0099
Weber, T., and Bianchi, D. (2020). Efficient particle transfer to depth in oxygen minimum zones of the Pacific and Indian Oceans. Front. Earth Sci. 8:376. doi: 10.3389/feart.2020.00376
Keywords: biological carbon pumps, robotic observations, integrated observations, ships, satellites, floats, gliders, moorings
Citation: Claustre H, Legendre L, Boyd PW and Levy M (2021) The Oceans’ Biological Carbon Pumps: Framework for a Research Observational Community Approach. Front. Mar. Sci. 8:780052. doi: 10.3389/fmars.2021.780052
Received: 20 September 2021; Accepted: 28 October 2021;
Published: 29 November 2021.
Edited by:
Fabien Roquet, University of Gothenburg, SwedenReviewed by:
Dennis Arthur Hansell, University of Miami, United StatesHelena Hauss, GEOMAR Helmholtz Center for Ocean Research Kiel, Germany
Copyright © 2021 Claustre, Legendre, Boyd and Levy. This is an open-access article distributed under the terms of the Creative Commons Attribution License (CC BY). The use, distribution or reproduction in other forums is permitted, provided the original author(s) and the copyright owner(s) are credited and that the original publication in this journal is cited, in accordance with accepted academic practice. No use, distribution or reproduction is permitted which does not comply with these terms.
*Correspondence: Hervé Claustre, aGVydmUuY2xhdXN0cmVAaW1ldi1tZXIuZnI=