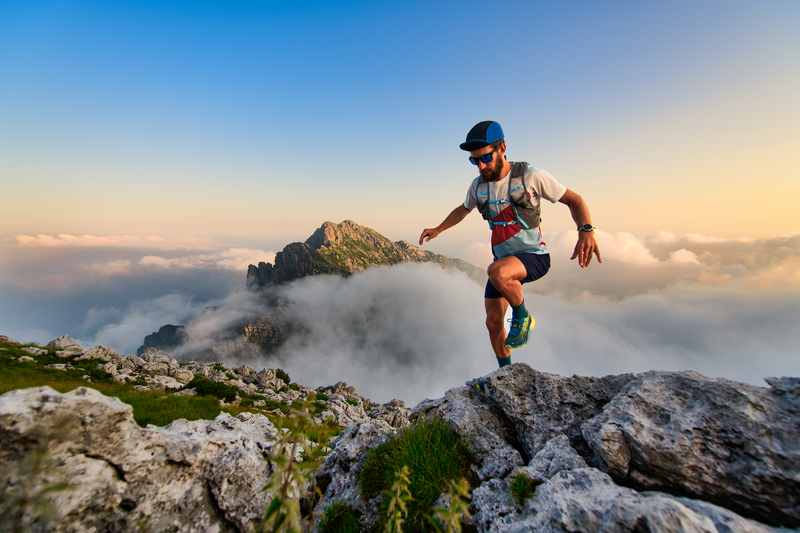
95% of researchers rate our articles as excellent or good
Learn more about the work of our research integrity team to safeguard the quality of each article we publish.
Find out more
ORIGINAL RESEARCH article
Front. Mar. Sci. , 10 November 2021
Sec. Marine Biotechnology and Bioproducts
Volume 8 - 2021 | https://doi.org/10.3389/fmars.2021.766282
This article is part of the Research Topic Cyanobacterial and Microalgal Compounds: Chemical Ecology and Biotechnological Potentials. View all 6 articles
A multidisciplinary approach was used to assess chemical ecological dietary interactions between marine organisms as a tool to isolate novel ecologically relevant compounds with biotechnological potential. First, laboratory-based feeding preference assays of the sea hare Dolabrifera nicaraguana (previously known as D. dolabrifera), an anaspidean mollusc, were conducted by simultaneously offering six food options collected from nearby tidal pools in the Coiba National Park in the Tropical Eastern Pacific of Panama. An evaluation of preferred dietary repertoire revealed D. nicaraguana significantly preferred cf. Lyngbya sp. over the cyanobacterium Symploca sp., green alga Chaetomorpha sp., and red alga Spyridia sp. A no-choice feeding assay using cf. Lyngbya sp. or green alga Cladophora sp. supported this finding. Secondly, we conducted bioactivity-guided fractionation using the preferred food source of D. nicaraguana, the ‘hair-like” cf. Lyngbya sp. from which we also isolated and elucidated two new depsipeptide compounds, veraguamide M (1) and veraguamide N (2). Veraguamides M (1) and N (2) showed in vitro activity toward the malaria-causing parasite Plasmodium falciparum with GI50 values of 4.2 and 4.3 μM, respectively, and therapeutic windows of 7.0–8.0 (based on moderate cytotoxicities to mammalian Vero cells with GI50 values of 29.3 and 34.1 μM, respectively). Veraguamide N (2) was also active against Leishmania donovani, the causative agent of visceral leishmaniasis, with a GI50 value of 6.9 μM. We then evaluated sequestration of these new compounds by D. nicaraguana used in the feeding assays and found trace amounts of the dietary sequestered compounds. Finally, we evaluated sequestration of these new compounds by the sea hare Stylocheilus rickettsi (previously known as S. striatus) that were grazing on the cf. Lyngbya sp. used in the feeding assays and found both to be sequestered. This study is the first example whereby compounds with significant activity against tropical parasites have been found in both the sea hare S. rickettsi and its cyanobacterial food source. These results suggest that chemical ecological studies involving sea hares and cyanobacteria continue to provide a diverse source of bioactive compounds with biotechnological potential.
Marine cyanobacteria have significant biotechnological potential including as food, fuel, fertilizers, and in mariculture (Thajuddin and Subramanian, 2005; Nunnery et al., 2010). Cyanobacteria are renowned for the wealth of secondary metabolites they produce (Burja et al., 2001; Blunt et al., 2012), many of which have been studied for their potential as pharmaceutical leads, cosmetics, vitamins, enzymes, or for pollution abatement (Thajuddin and Subramanian, 2005; Rotter et al., 2021). In nature, cyanobacteria produce a diverse combination of metabolites, some highly toxic, to maximize survival in competitive habitats where there are a range of grazers (Nagle and Paul, 1999). Many of these cyanobacterial secondary metabolites act as feeding deterrents to generalist grazers, such as crabs, fish, and sea urchins (Pennings et al., 1997; Nagle and Paul, 1998; Capper et al., 2006b), which allows cyanobacteria to avoid predation (Nagle and Paul, 1999; Capper et al., 2016). Fish and other potential grazers often have individualized responses to cyanobacterial secondary metabolites, wherein a compound that deters one herbivore may not deter another (Pennings et al., 1997).
The polyphyletic genus formally known as Lyngbya is renowned for its large number of secondary metabolites with biotechnological potential (Thajuddin and Subramanian, 2005; Rotter et al., 2021). Lyngbya majuscula, in particular has been shown to produce a wide array of bioactive secondary metabolites (Liu and Rein, 2010). However, the generic determination in many of these studies was largely based on morphology with the cyanobacterial consortium formally classified as Lyngbya majuscula forming filamentous mats or tangled masses up to 50 cm long (Littler and Littler, 2000). Although Lyngbya is known for the array of secondary metabolites it produces, this is likely due to an underestimation of cyanobacterial consortium biodiversity (Engene et al., 2011). With advances in cyanobacterial phylogenetics using the 16S rRNA gene, this morphologically similar cyanobacterial consortium was revealed to contain multiple evolutionarily distinct groups (Engene et al., 2013a). Phylogenetic analysis has revealed the cryptic diversity of Lyngbya, resulting in several new genera, including Moorena (formerly Moorea), Okeania, Dapis, and Neolyngbya, among others (Engene et al., 2012, 2013a,b, 2018; Caires et al., 2018; Tronholm and Engene, 2019). Because of the complexity in identification for this benthic cyanobacterial consortium, in this manuscript we will refer to Lyngbya majuscula as cf. Lyngbya sp.
Cyanobacterial secondary metabolites comprise a highly diverse range of compounds (Engene et al., 2013a). The bulk of these marine natural products have been isolated from Okeania spp. and Moorena producens (Engene et al., 2013a,b; Tronholm and Engene, 2019). In addition, several Panamanian compounds from Okeania, Moorena, and Dapis genera have shown activity against the tropical parasites Plasmodium falciparum and Leishmania donovani, the causative agents of malaria and leishmaniasis, respectively (Supplementary Tables 1, 2; Linington et al., 2007; McPhail et al., 2007; Gutierrez et al., 2010; Sanchez et al., 2010; Balunas et al., 2012).
These diverse cyanobacterial secondary metabolites can stimulate feeding by specialized herbivores, such as sea hares (Anaspidea: Opisthobranchia) (Pennings et al., 1993; Nagle et al., 1998; Arthur et al., 2009), while providing a safe haven by lowering encounter rates with reef predators who are deterred by the metabolites (Cruz-Rivera and Paul, 2006). Many sea hare species have the capacity to sequester these dietary metabolites and store them in their digestive glands, rather than in external organs, ink or eggs (Pennings and Paul, 1993; Capper et al., 2005). As the predator has to consume the sea hare before being exposed to the toxins (de Nys et al., 1996), it has been suggested that concentrating sequestered compounds in the digestive gland might: (1) aid in detoxifying a diet rich in secondary metabolites (Pennings et al., 1999); (2) aid in the storage of toxic compounds too metabolically expensive to break down (Pennings and Paul, 1993; Capper and Paul, 2008); or (3) act as a site where chemical modification occurs to the molecule before sending through the blood to the ink gland for further modification and use in chemical defense (Kamio et al., 2010). Originally it was thought that these secondary metabolites were produced de novo by sea hares (Kato and Scheuer, 1974; Faulkner, 1984; Pettit et al., 1987), although it is now widely accepted that these compounds are of dietary origin (Stallard and Faulkner, 1974; Paul and Pennings, 1991; Rogers et al., 1995; Capper et al., 2005).
Sea hares from the Dolabrifera genus (Cuvier, 1817) are common opisthobranch molluscs in pantropical waters, especially in the Americas (Rudman, 2003; Valdés et al., 2018). Molecular and morphological research has revealed five taxa within the Dolabrifera genus, of which D. nicaraguana is endemic to the eastern Pacific (Valdés et al., 2018). Dolabrifera spp. defense mechanisms are limited as they do not produce ink (Prince and Johnson, 2006), but do produce a white milky secretion from their mantles when threatened (Ghazali, 2006). They often live under intertidal boulders (Kay, 1979) and avoid predation by emerging to feed during daytime ebbing tide once the tide falls below their tidal pools (Himstead and Wright, 2018). Field observations suggested that Dolabrifera spp. feed on diatoms, microalgae, and algal mats (Miller, 1969; Marshall and Willan, 1999; Cimino and Ghiselin, 2009; Nimbs et al., 2017), whereas Dolabrifera sp. have been reported to feed on cf. Lyngbya sp. and Enteromorpha clathrata when kept in an aquarium with no other food (Prince and Johnson, 2006). However, the preferred diet of Dolabrifera spp. remains unknown.
Metabolites extracted from Dolabrifera spp. have exhibited variable palatability and toxicity. For example, whilst the skin and body walls of Dolabrifera sp. were palatable to the common intertidal hermit crab Pagurus samuelis (Takagi et al., 2010), extracts from its mid-gut gland were lethal when injected into mice at a high dose (200 mg/kg) (Waston, 1973). In other studies, egg masses of Dolabrifera sp. have shown antibacterial properties (Benkendorff et al., 2001). A sterol compound (5α,8α-epidioxycholest-6-en-3β-ol) isolated from D. nicaraguana (formerly D. dolabrifera) digestive gland previously demonstrated activity against L. donovani (Clark et al., 2013). A polypropionate metabolite, dolabriferol, isolated from Dolabrifera sp. skin (Ciavatta et al., 1996) and similar compounds, dolabriferol B and C, exhibited inhibitory effects against Mycobacterium tuberculosis (Jiménez-Romero et al., 2012). Given that little is known about Dolabrifera spp. feeding preferences or their ability to sequester dietary metabolites, further study presents an opportunity for marine chemical ecological research and bioactive metabolite investigations.
Sea hares from the Stylocheilus genus (Quoy and Gaimard, 1832) have a circumtropical distribution (Camacho-García et al., 2005). There are ongoing changes to the taxonomy of S. striatus, where phylogenetic testing revealed three allopatric species—S. striatus (Indo-Pacific), S. rickettsi (Eastern Pacific) and S. polyomma (Western Atlantic) (Bazzicalupo et al., 2020). Thus, in this manuscript to refer to a few or all the species we will use Stylocheilus spp. A species designation will be included when it is known based on geographical location of the sea hares. Stylocheilus spp. are specialist grazers of cyanobacteria, preferring cf. Lyngbya sp. over several other cyanobacteria and algae choices (Paul and Pennings, 1991; Capper et al., 2006a; Cruz-Rivera and Paul, 2006). Some cyanobacterial secondary metabolites, such as malyngamide A and B, are preferred by S. striatus while acting as deterrents to other grazers (Pennings et al., 1996; Nagle et al., 1998). S. striatus sequesters dietary compounds from cf. Lyngbya sp., storing them in their digestive glands (Kato and Scheuer, 1974; Rose et al., 1978; Gallimore and Scheuer, 2000).
Often these sequestered secondary metabolites show a variety of bioactive properties, such as tumor promotion and anti-proliferation (Kikumori et al., 2012; Youssef et al., 2015; Supplementary Table 3). In some cases, S. striatus transforms these potent compounds in its digestive gland to less toxic forms through acetylation, for example transformation of lyngbyatoxin A to lyngbyatoxin A acetate (Gallimore et al., 2000) and malyngamide B to malyngamide B acetate (Paul and Pennings, 1991; Supplementary Table 3). The ecological connection of Stylocheilus spp. with cf. Lyngbya sp. extends beyond their diet, as chemical cues encourage settlement and development of larvae (Switzer-Dunlap and Hadfield, 1977). Additionally, Stylocheilus spp. have shown feeding attraction to artificial food containing extracts rich in secondary metabolites (Capper et al., 2016). While these cyanobacteria were identified as Lyngbya at the time, S. striatus graze on several of the newly classified cyanobacteria genera, Okeania sp., M. producens, Lyngbya sp., and Dapis sp., altering their feeding preferences based on secondary metabolite type and concentration in their food (Capper et al., 2016). The ecological role, however, of sequestered dietary compounds in Stylocheilus spp. has not yet been fully determined (Bornancin et al., 2017).
In this study, we sought to evaluate the marine chemical ecological interactions of sea hares with their food sources by characterizing dietary metabolites that are sequestered by the sea hares. We also tested these metabolites for anti-parasitic bioactivity, thus linking the ecological interactions with biotechnological applications. Our objectives were to: (1) establish laboratory-based feeding preference of D. nicaraguana; (2) isolate and identify bioactive compounds from the preferred food source cf. Lyngbya sp.; (3) determine sequestration of the compounds from cf. Lyngbya sp. in the sea hares D. nicaraguana and S. rickettsi; and (4) test these sequestered compounds against tropical parasitic diseases in bioassays of relevance to the host country of Panama.
Collections were made in May 2006 during low tide in the littoral zone along an extensive rocky shoreline peninsula between Playa Blanca and Boca Grande, Coiba Island, within Coiba National Park, Veraguas, Panama (07°23′50″ N, 81°39′00″ W) (Figure 1A). The collection site was situated on a large expanse of sedimentary rock weathered to sea-level, extending approximately 0.1 km2 from the tip of the peninsula (Figure 1B). At low tide, many tidal pools and exposed boulders provided ample habitat for a variety of organisms on the underside of boulders and in tidal pools.
Figure 1. (A) The Coiba National Park, Panama, and the location of the Boca Grande field site, with (B) the field site at low tide [image from 12/Mar/2012 Worldview (WV02) within the world imagery basemap in ArcMap 10.8.1 (ESRI et al., 2021)].
All cyanobacterial and algal samples were found growing in tidal pools located nearby the boulders where sea hares were collected. Three cyanobacterial samples, fixed to the sandy bottom, rocks or bedrock, were collected including a cf. Lyngbya sp. cyanobacterium in a mat assemblage, a cf. Lyngbya sp. cyanobacterium with a hair-like morphology, and a Symploca sp. (Figures 2A–C, respectively). Three algal species were collected including a red alga, Spyridia sp. and two green algae, Chaetomorpha sp. and Cladophora sp. (Figures 2D–F, respectively). A 4.5 L bag of each species was collected and then frozen at −20°C for chemical analyses. Voucher specimens were preserved in EtOH:seawater (70:30) and maintained at −20°C for identification. Additional samples of cyanobacteria and algae were maintained until required for feeding assays in aerated aquaria in fresh seawater with 12:12 h light:dark at ambient temperature at the Liquid Jungle Lab, Canales de Tierra, Pacific coast of Veraguas or at Naos Marine and Molecular Laboratories at the Smithsonian Tropical Research Institute, Panama City.
Figure 2. Multiple choice assay food options: cyanobacteria (A) “mat” assemblage cf. Lyngbya sp., (B) “hair-like” cf. Lyngbya sp., (C) Symploca sp.; and algae (D) Spyridia sp. and (E) Chaetomorpha sp. No-choice assay food options: (B) “hair-like” cf. Lyngbya sp. and (F) alga Cladophora sp. Scale bars indicate 1 cm of length. Photos taken by K. Clark.
Nine S. rickettsi (Figure 3A) were collected in the field grazing on cf. Lyngbya sp. with the hair-like morphology (Figure 2B) and were allowed to continue to graze in the laboratory in aquaria (ambient temperature, 12:12 h light:dark) for 5 days, fasted for 24 h (to allow gut evacuation), euthanized in freezing seawater and stored at −20°C until tissue analysis was performed. Feeding studies were not conducted on these collections because of the extensive literature on the feeding preferences and metabolite sequestration of S. rickettsi (formerly S. striatus) (Kato and Scheuer, 1974; Rose et al., 1978; Paul and Pennings, 1991; Gallimore and Scheuer, 2000; Capper et al., 2006a; Cruz-Rivera and Paul, 2006).
Figure 3. Sea hares (A) Stylocheilus rickettsi and (B) Dolabrifera nicaraguana in the field, and (C) D. nicaraguana dissection. Field photo taken by A. Ibañez, laboratory photo taken by K. Clark, and hand-drawn dissection by K. Clark.
Eighteen D. nicaraguana (Figure 3B) were collected in the same area as the food choices from the underside of uneven boulders in the intertidal zone. Three of these D. nicaraguana were used without laboratory feeding to assess their exposure to the food choices in the wild. They were fasted, euthanized, and stored as described above. Two additional animals died in the laboratory and were kept as vouchers. The remaining 13 animals were maintained in aquaria as above and were used in both multiple choice and no-choice feeding assays.
Dolabrifera nicaraguana (n = 12, average initial weight 2.6 ± 0.4 g) were placed individually in separate 2 L aquaria in fresh seawater. Each sea hare was provided a choice between five food types offered simultaneously: “mat-like” and “hair-like” morphologies of cf. Lyngbya sp. (average initial wet weight 809 ± 174 mg and 506 ± 117 mg, respectively); Symploca sp. (average initial wet weight 407 ± 109 mg); the red alga, Spyridia sp. (average initial wet weight 151 ± 32 mg); and the green alga, Chaetomorpha sp. (average initial wet weight 448 ± 121 mg). Food items were blotted, weighed, and placed at the bottom of 2 L aquaria with fresh seawater. There were twelve treatment (herbivore) aquaria and twelve control (no-herbivore) aquaria. The no-herbivore control aquaria were used to assess changes in algal mass throughout the experiment in the absence of herbivores (Cronin and Hay, 1996). Food items were removed after 60 h, blotted and reweighed. The amount of food consumed for each of the five food types was calculated for each replicate using the equation:
where Ti and Tf are the initial and final weights of the treatments, and Ci and Cf are the initial and final weights of the controls (Cronin and Hay, 1996). The Friedman test was used to detect significant differences in consumption of the different food types by D. nicaraguana (Erickson et al., 2006; Capper and Paul, 2008). A Nemenyi post hoc test was used to examine all possible pair-wise combinations for significant differences between groups (P < 0.05) (Conover, 1998).
To confirm food preferences, a no choice assay, using the same D. nicaraguana from the multiple-choice feeding assays above, was initiated after animals were fasted for 36 h. The no choice assays consisted of two D. nicaraguana feeding groups, including the cyanobacterium cf. Lyngbya sp. with “hair-like” morphology (n = 6, average initial wet weight 70 ± 22 mg) or the green alga Cladophora sp. (n = 6, average initial wet weight 41 ± 8 mg). The green alga Cladophora sp. was used instead of the green alga Chaetomorpha sp. because we had already established in the multiple-choice assay that the D. nicaraguana did not preferentially feed on Chaetomorpha sp. Food items were blotted dry, weighed, and divided into treatment and no-herbivore control group aquaria, as described above. The cf. Lyngbya sp. fed D. nicaraguana had an average initial animal weight of 2.1 ± 0.4 g while the Cladophora sp. fed D. nicaraguana had an average initial animal weight of 2.3 ± 0.3 g. A control group was included to assess changes in cyanobacterial (n = 6, average initial wet weight 65 ± 34 mg) and algal mass (n = 6, average initial wet weight 40 ± 12 mg) throughout the experiment in the absence of herbivores (Peterson and Renaud, 1989). After 60 h, food items were removed, blotted and weights taken as described above. Post-assay, sea hares were fasted for 24 h to allow gut evacuation, euthanized in freezing seawater, and stored at −20°C. The proportion of food consumed was calculated using equation 1. A Mann–Whitney U test was used to determine whether there were significant differences between algal and cyanobacterial fed groups.
Low-resolution mass spectra (MS) were obtained in MeOH and analyzed by direct injection on a JEOL LCmate mass spectrometer (Tokyo, Japan). Nuclear magnetic resonance (NMR) spectra were collected using a JEOL Eclipse 400 MHz spectrometer (United Kingdom). MS/MS analyses were conducted using a Finnigan LTQ MS (Thermo-Electron Corporation). High performance liquid chromatography (HPLC) was carried out in reverse phase using a Prontosil-120 C18 analytical column (4.6 mm × 250 mm, Bischoff Chromatography, Leonberg, Germany) and a Merck Hitachi HPLC (Tokyo, Japan) containing dual pumps (L-7100) and a diode array detector (L-7455) monitoring at 210 nm.
Sea hare D. nicaraguana (n = 3) not used in the feeding assays, D. nicaraguana (n = 6) used in the multiple choice assay and in the cf. Lyngbya sp. no-choice feeding assay, and D. nicaraguana (n = 6) used in the multiple choice assay and in the green alga Cladophora sp. no-choice feeding assay were weighed and dissected, separating digestive gland, skin (including parapodia, foot, and head), mucus gland (also referred to as albumen gland), and grouping the remaining internal organs (Figure 3C and Supplementary Table 4). S. rickettsi (n = 9) were weighed and dissected, separating digestive gland, skin, and grouping the remaining internal organs (Supplementary Figure 1 and Supplementary Table 4). To obtain sufficient material for chemical analysis, tissue samples were grouped, with one or two individuals per replicate, as shown in Supplementary Table 4. Tissue samples were then lyophilized, weighed, and pulverized prior to dissolving in 1:1 EtOAc:MeOH, sonicating for 20 min, and extracting twice over 48 h. Samples were rinsed in 1:1 EtOAc:MeOH, filtered under vacuum, and dried via rotary evaporation. The excrement collected from D. nicaraguana and S. striatus during their 24 h fast, an egg mass from S. striatus, and mucus from D. nicaraguana were also dried and extracted as described above.
The cf. Lyngbya sp. cyanobacterium with “hair-like” morphology was lyophilized, ground, and 35 g of powdered sample was extracted by soaking in 1:1 EtOAc:MeOH and decanting three times over 72 h. The EtOAc:MeOH extract (given the code 9401) was collected and dried under rotary evaporation, producing 1.68 g of extract. The cf. Lyngbya sp. residue was then extracted by soaking in 1:1 EtOH:H2O and decanting twice over 48 h. The EtOH:H2O extract (given the code 9402) was collected, dried under rotary evaporation and then lyophilized to remove any remaining water, producing 0.4 g of extract. Both extracts were analyzed by MS, with the EtOAc:MeOH extract (9401) exhibiting mass peaks of m/z 887.1, 717.5, 703.5, 537.4, 313.3, 279.2, 245.2, 227.2 and 213.2. The EtOH:H2O extract (9402) had two non-solvent peaks m/z 717.5 and 285.2.
The EtOAc:MeOH extract was fractionated using a Supelco Discovery DSC-18 reverse phase (RP) solid phase extraction (SPE) cartridge, with a bed weight of 10 g and 60 mL tube volume. The C18 RP-SPE cartridge was first equilibrated in 1:1 MeOH:H2O. After, loading 500 mg of the EtOAc:MeOH (9401) extract onto the cartridge, the sample was sequentially eluted under manually applied pressure with 150 ml each of 1:1, 3:2, 7:3, 4:1, and 5:1 MeOH:H2O followed by 150 ml each of 100% MeOH, 100% EtOAc, and 100% acetone. The resulting eight fractions (coded 9401A through 9401H) were dried and assessed for bioactivity.
To rapidly assess a range of biological activity, in vitro bioassays already available through the Panama International Cooperative Biodiversity Group (ICBG) were employed as previously described (Moreno et al., 2011; Pavlik et al., 2013) to detect biological activity using P. falciparum (malaria), L. donovani (leishmaniasis), Trypanosoma cruzi (Chagas’ disease), MCF-7 breast cancer cells, and Vero mammalian cells (used to estimate overall cytotoxicity). Samples were initially screened at 10 μg/mL, with active samples then being further tested to determine the concentration to inhibit 50% growth (GI50). Selectivity indices were calculated by dividing the GI50 value for Vero cell cytotoxicity by the GI50 value of the same sample toward the disease (e.g., P. falciparum, L. donavani, T. cruzi, MCF-7 cells).
Fraction 9401D, eluted with 4:1 MeOH:H2O, was found to exhibit activity against P. falciparum in the malaria assay (GI50 of 1.0 μg/mL) and was thus used for compound isolation via RP-HPLC. After dissolving in MeOH and filtering at 0.45 μm (Altech 17 mm PTFE syringe filters), compound isolation was accomplished using an isocratic system of 55% acetonitrile (CH3CN) and 45% H2O, with a flow rate of 1 ml/min to yield veraguamides M (1) and N (2), eluting at 23 and 31 min, respectively.
MS/MS was carried out on compounds 1 and 2 using a Finnigan LTQ MS (Thermo-Electron Corporation) using Tune Plus software version 1.0, as previously described (Mevers et al., 2011). Spectral files were converted to mzXML files (publicly accessible at http://gnps.ucsd.edu under MassIVE accession no. MSV000080055) and analyzed using previously described algorithms (Liu et al., 2009; Ng et al., 2009; Mohimani et al., 2011). Compounds 1 and 2 were analyzed by 1H and 13C NMR, recorded in methylene chloride-d2 (CD2Cl2, Cambridge Isotope Laboratories, Inc., Andover, MA, United States) (Supplementary Figures 2–5).
Veraguamide M (1): amorphous solid; [α]23D –49.5 (c 0.5, CHCl3); 1H NMR (400 MHz, CD2Cl2) δ 6.25 (d, J = 8.6 Hz, 1H), 4.88 (d, J = 7.7 Hz, 1H), 4.77 (p, J = 4.8 Hz, 2H), 4.69 (dd, J = 8.5, 5.3 Hz, 1H), 4.05 (d, J = 10.2 Hz, 1H), 3.97 (d, J = 10.4 Hz, 1H), 3.76 (dt, J = 9.4, 6.7 Hz, 1H), 3.55 (dt, J = 9.4, 6.9 Hz, 1H), 3.40 (s, 2H), 2.93 (d, J = 11.2 Hz, 6H), 2.35–2.15 (m, 3H), 2.15–1.88 (m, 3H), 1.81–1.62 (m, 1H), 1.67–1.35 (m, 4H), 1.32–1.08 (m, 4H), 1.07 (d, J = 6.5 Hz, 3H), 1.07–0.79 (m, 20H); 13C NMR (100 MHz, CD2Cl2) δ 173.3, 172.2, 171.0, 169.8, 165.9, 83.8, 76.9, 76.4, 68.6, 66.3, 64.0, 57.3, 52.6, 50.5, 47.3, 42.1, 38.8, 35.7, 35.2, 29.6, 29.4, 28.8, 28.3, 28.1, 26.0, 25.5, 25.1, 24.6, 23.7, 19.9, 19.7, 18.1, 16.4, 15.7, 14.1, 13.9, 11.4, 11.3, 10.7; ESIMS/MS m/z 689.44, 590.36, 524.35, 490.36, 470.31, 452.28, 411.33, 339.23, 297.23, 228.19. HRESIMS [M + H]+ m/z 717.4797 (calcd for C39H65N4O8, 717.4802).
Veraguamide N (2): amorphous solid; 1H NMR (400 MHz, CD2Cl2) δ 6.22 (t, J = 10.5 Hz, 1H), 4.86 (dd, J = 13.9, 7.2 Hz, 1H), 4.80–4.73 (m, 2H), 4.71–4.64 (m, 2H), 4.07–3.85 (m, 2H), 3.80–3.67 (m, 1H), 3.59–3.48 (m, 1H), 3.41 (s, 2H), 2.98–2.87 (m, 7H), 2.29–2.10 (m, 2H), 2.04–1.89 (m, 2H), 1.66–1.59 (m, 2H), 1.44 (s, 2H), 1.22 (dd, J = 14.0, 8.4 Hz, 4H), 1.12–0.77 (m, 26H); 13C NMR (100 MHz, CD2Cl2) δ 173.3, 171.0, 170.8, 169.9, 165.7, 83.9, 77.3, 77.0, 76.5, 68.6, 66.3, 64.0, 57.2, 52.6, 47.2, 42.0, 38.9, 35.7, 35.2, 29.6, 29.4, 28.7, 28.2, 28.0, 25.9, 25.61, 25.57, 25.2, 23.7, 19.92, 19.87, 19.7, 18.2, 18.1, 17.8, 16.4, 15.7, 14.1, 11.4, 11.3, 10.8; HRESIMS [M + H]+ m/z 703.4641 (calcd for C38H63N4O8, 703.4646).
Extracts of sea hare tissues, egg mass, and excrement were analyzed via MS to determine the presence of compounds 1 and 2. In addition, 1H-NMR of extracts of the sea hare digestive gland and skin tissues were obtained in CDCl3 to compare with those of the pure compounds 1 and 2. HPLC was used to evaluate compound sequestration using an isocratic system of 68% CH3CN and 32% H2O at 1 mL/min. Compounds 1 and 2 eluted at 13 and 15 min, respectively, and were compared with chromatograms of the digestive glands and skin extracts from the feeding assay D. nicaraguana, control D. nicaraguana, and S. rickettsi.
In the multiple choice feeding assay, D. nicaraguana individuals were found to exhibit significant differences in preferences for the cyanobacterial and algal food types (Friedman test, P < 0.001). The Nemenyi post hoc test revealed no significant difference in preference between the two cf. Lyngbya species but found significant differences in consumption of the “hair-like” assemblage cf. Lyngbya sp. and the other food choices, including red alga Spyridia sp., cyanobacterium Symploca sp., and green alga Chaetomorpha sp. (P < 0.05, unadjusted; Figure 4A). Following the multiple choice assay, a no choice assay was performed using the “hair-like” cyanobacterium cf. Lyngbya sp. and the green algae Cladophora sp. In isolation, D. nicaraguana consumed more “hair-like” cf. Lyngbya sp. than it did the green alga Cladophora sp. (Figure 4B, Mann–Whitney U test, P = 0.008).
Figure 4. Control adjusted mean (±SE) food consumption (mg, blotted wet weight) of algal or cyanobacterial food options by D. nicaraguana: (A) Multiple choice feeding assay (n = 12; Friedman Test, P < 0.001). Means with different letters are significantly different (P < 0.05) as per an unadjusted Nemenyi post hoc test. cf. Lyngbya sp. (1) refers to “hair-like” morphology and cf. Lyngbya sp. (2) refers to “mat-like” morphology; (B) No choice feeding assay (Mann–Whitney U test, P = 0.008) with either Cladophora sp. (n = 6) or cf. Lyngbya sp. (n = 6).
Given that there were significant preferences for the “hair-like” cf. Lyngbya sp. by D. nicaraguana, and that S. rickettsi, known to sequester cf. Lyngbya sp. secondary metabolites, was found grazing on the “hair-like” cf. Lyngbya sp., the “hair-like” collection of cf. Lyngbya sp. was used for further activity-guided isolation. Two extracts were obtained (EtOAc:MeOH and EtOH:H2O) and analyzed by MS with the EtOAc:MeOH extract prioritized for further compound isolation. Fractions were tested for biological activity, with fraction D (eluted with 4:1 MeOH:H2O) exhibiting activity against the malaria parasite P. falciparum (73.5% inhibition of parasite growth at 10 μg/mL) and MCF-7 cancer cells (70% cell death indicated by negative growth), and with little to no activity in the leishmaniasis or Chagas’ disease assays (18.3 and 9.7% inhibition, respectively), and with low cytotoxicity (GI50 = 27 μg/mL). Compound isolation continued with RP HPLC, yielding two compounds (1 and 2) with molecular weights of 717 and 703 (Supplementary Figure 6).
Using HRESIMS, [M + H]+ peaks consistent with molecular formulas of C39H64N4O8 and C38H62N4O8, were obtained for compounds 1 and 2, respectively. Literature comparisons revealed several possible compounds with these molecular formula from cyanobacteria (Nakao et al., 1998; Mevers et al., 2011; Salvador et al., 2011) and 1H and 13C NMR revealed substantially overlapping signals (Supplementary Figures 2–5). Therefore, MS/MS data was obtained for compound 1 and analyzed using software designed for sequencing cyclic peptides (Liu et al., 2009; Ng et al., 2009; Mohimani et al., 2011) and previously used with compounds from the same family [e.g., veraguamide E (3) (Mevers et al., 2011; Salvador et al., 2011)]. Using both manual and computational comparisons of the MS/MS fragmentation patterns, the locations of structural modifications were determined, as compared with veraguamide A (Supplementary Figures 7, 8). Compound 1 was found to have two rearrangements as compared with veraguamide E (3) including substitution of an N-Me-Ile in place of an N-Me-Val in the first residue clockwise from the HMoya, as well as an N-Me-Val in place of the N-Me-Ile for the fourth residue clockwise from the HMoya, thus resulting in designation as a new compound given the trivial name veraguamide M. Stereochemical assignment was not possible with the limited quantities of 1, although the sign and magnitude of the optical rotation is consistent with that reported for veraguamide E (3) (Salvador et al., 2011), making it likely that the absolute configuration of veraguamide M (1) is identical to that reported for compound 3. Similar analyses were performed for compound 2, resulting in the identification of another new compound named veraguamide N. Veraguamide N (2) was found to have one less methyl group, resulting in a 2-hydroxyisovaleric acid (Hiva) group in place of the 2-hydroxy-2-methylpentanoic acid (Hmpa) group found in the third residue clockwise from the HMoya group of compound 1 (Figure 5).
Figure 5. Planar structure of the new compounds veraguamide M (1) and veraguamide N (2) with comparison to four related metabolites, veraguamides E (3) and D (4) and kulomo‘opunalide-1 (5) and kulomo‘opunalide-2 (6).
The animal tissues were analyzed under the same conditions as the compounds, veraguamides M (1) and N (2), and demonstrated that there was strong evidence of dietary compound sequestration by S. rickettsi, and possible evidence of compound sequestration by D. nicaraguana (Table 1 and Supplementary Figure 9). Sequestration of veraguamide M (1) and veraguamide N (2) was confirmed through MS, 1H-NMR, and HPLC analysis in all three digestive gland replicates of S. rickettsi (Table 1 and Supplementary Figure 9). Additionally, veraguamide M (1) and veraguamide N (2) were evident in the MS and 1H-NMR spectral data of the digestive gland of S. rickettsi (Table 1 and Supplementary Figure 9). These compounds were also evident in the MS of the other internal organs of S. rickettsi (Table 1). Excrement from the 24-h fasting period was evaluated using MS and showed evidence of veraguamide M (1), although an egg mass collected during the study did not show evidence of either compounds using MS (Table 1).
Dolabrifera nicaraguana, previously not known to sequester compounds from its diet, showed possible evidence of compound sequestration in our study (Table 1). Separate HPLC and MS analyses of the digestive gland of the D. nicaraguana fed the “hair-like” cf. Lyngbya sp. and of the tissues of D. nicaraguana not used in feeding assays exhibited low abundance signals indicative of possible evidence of the presence of veraguamide M (1) (Table 1). Veraguamide N (2) was not detected in D. nicaraguana tissues (Table 1). Other D. nicaraguana tissue samples, including skin, other internal organs, mucus gland, and mucus did not show evidence of compound sequestration, as assessed by MS and HPLC.
Veraguamide M (1) showed moderate activity against P. falciparum with a GI50 value of 4.2 μM and moderate cytotoxicity to mammalian Vero cells with a GI50 value of 29.3 μM (Table 2), resulting in a selectivity index of 7.0. Veraguamide N (2) showed moderate activity against P. falciparum with a GI50 value of 4.3 μM and L. donovani with a GI50 value of 6.9 μM, moderate cytotoxicity to mammalian Vero cells with a GI50 value of 34.1 μM (Table 2), resulting in selectivity indices of 7.9 and 5.0 for antimalarial and anti-leishmanial activity, respectively.
Table 2. Biological activity (GI50 values) for veraguamide M (1), veraguamide N (2), and the digestive gland extract of Stylocheilus rickettsi fed exclusively cf. Lyngbya sp.
Since both sequestered metabolites showed activity toward P. falciparum, sea hare tissues were also screened for bioactivity. D. nicaraguana tissue samples, including the digestive gland, skin and other internal organs, from animals used in the feeding assays and control animals were shown to be inactive (Table 1). In contrast, an extract from the S. rickettsi digestive gland showed similar antimalarial activity as the cyanobacterial compounds, veraguamides M (1) and N (2), with a GI50 value of 3.0 μg/mL (Table 2). The S. rickettsi digestive gland extract was also cytotoxic to mammalian Vero cells with a GI50 of 10 μg/mL, consistent with the detection of the two veraguamides by MS and NMR (Table 1) in the digestive gland extract. Extracts from the S. rickettsi skin, other internal organs, and excrement were shown to be inactive (Table 1).
Multiple choice feeding assay results revealed D. nicaraguana significantly preferred both cf. Lyngbya sp. morphologies “hair-like” and “mat” assemblage more than the cyanobacterium Symploca sp., the green alga Chaetomorpha sp., or the red alga Spyridia sp. In no-choice assays, the “hair-like” cf. Lyngbya sp. cyanobacterium was also significantly preferred to the green alga Cladophora sp. Whilst Prince and Johnson (2006) observed D. nicaraguana consuming cf. Lyngbya sp. in a no-choice feeding experiment, they also observed it consuming the green alga Enteromorpha clathrata. As no collections were made of E. clathrata, this alga could not be used in feeding preference assays reported herein. As a generalist grazer, it is likely that D. nicaraguana may capitalize on variety of food types, including diatoms, algal mats, and bacterial biofilms (Miller, 1969; Marshall and Willan, 1999; Rudman, 2003; Cimino and Ghiselin, 2009; Nimbs et al., 2017), as part of wider dietary repertoire. These grazing habits could be beneficial to D. nicaraguana, allowing it to survive varying environmental conditions and food availability. In a previous study in Panama City, at Punta Culebra on Naos island, scores of D. nicaraguana were reported to emerge to forage during the daytime once the tide fell below their tidal pools (Himstead and Wright, 2018). In our study, D. nicaraguana were also collected during this tidal period, although we did not find any individuals on the food treatment types used in our feeding assays, but rather nearby on the undersides of boulders. While D. nicaraguana preferred cf. Lyngbya sp. in our laboratory-based feeding assays, further research is required to assess the diet of D. nicaraguana in the wild.
Although there was possible evidence for sequestration of veraguamide M (1) in the digestive gland of D. nicaraguana, the low abundance signals found in the HPLC and MS analyses were not definitive. Additionally, D. nicaraguana tissue samples were biologically inactive, further suggesting minimal sequestration of veraguamides M (1) or N (2). It is possible that if the D. nicaraguana feeding assays ran for longer periods of time [e.g., 10 to 20 days (Pennings and Paul, 1993; Capper et al., 2005)] that these compounds may have bioaccumulated and demonstrated stronger evidence for sequestration. Additionally, detection of sequestered secondary metabolites may also depend on the ability of the organism to store or detoxify these compounds into less toxic metabolites (Capper et al., 2005). While some sea hares can sequester secondary metabolites with no apparent harm (Paul and Pennings, 1991), D. nicaraguana may not be able to tolerate these secondary metabolites and may, therefore, metabolize them into less harmful compounds.
In contrast, it is well known that Stylocheilus spp. sequester dietary-derived compounds from Lyngbya spp. and store them in their digestive glands (Pennings and Paul, 1993). Herein, S. rickettsi was found to sequester and store the cf. Lyngbya sp. compounds veraguamide M (1) and N (2), mainly in its digestive gland, but also in skin, other internal organs, and excrement. However, only the digestive gland of S. rickettsi exhibited biological activity, demonstrating the accumulation of sequestered compounds in the digestive gland. Moreover, the digestive gland extract showed similar antimalarial activity as the cyanobacterial compounds, while it was more cytotoxic toward mammalian Vero cells than were either of the sequestered compounds, suggesting there may be additional cytotoxic compounds stored in the S. rickettsi digestive gland. This is plausible given that Stylocheilus species process diverse secondary metabolites from their food (Paul and Pennings, 1991; Pennings and Paul, 1993; de Nys et al., 1996; Pennings et al., 1996; Capper et al., 2005) and concentrate the bulk of sequestered compounds in their digestive gland (Pennings and Paul, 1993). S. rickettsi provided an excellent opportunity to compare sequestration to an animal whose capability of sequestering was uncertain, in this case D. nicaraguana. In addition, this is the first study to demonstrate these phenomena in S. rickettsi, a species located in the Eastern Pacific. Additional studies incorporating the isolated compounds into artificial diets for sea hare feeding experiments are needed to fully assess consumption and subsequent compound sequestration by the sea hares.
Ongoing phylogenetic assessments of marine benthic cyanobacteria have revealed that these cyanobacteria are much more diverse than previously thought (Engene et al., 2011). Although cf. Lyngbya sp. and related species may be morphologically similar, their genetic diversity has allowed them to produce effective and biodiverse chemical defenses (Engene et al., 2013a). Stylocheilus are known to graze on several of these new genera (Capper et al., 2016), including cyanobacteria recently reclassified using molecular sequencing as Okeania sp., Moorena producens, Lyngbya sp., and Dapis sp. (Engene et al., 2013a,b, 2018; Tronholm and Engene, 2019). Stylocheilus are also known to exhibit an altered feeding preference based on secondary metabolite type (Capper and Paul, 2008; Capper et al., 2016). Thus, it may be that Stylocheilus species select their food based on morphology of cyanobacteria and the secondary metabolites they produce.
Stylocheilus species are located in tropical oceans and have been geographically separated for at least the last three million years, with the closure of the Isthmus of Panama (Bacon et al., 2015; O’Dea et al., 2016), diverging into three allopatric species found in the Indo-Pacific, Western Atlantic, and Eastern Pacific (Bazzicalupo et al., 2020). Although these geographical barriers likely resulted in Stylocheilus speciation, multiple species from this genus are known to evade predation by preferring metabolite rich Lyngbya spp. cyanobacterial assemblages, thus benefiting from the structural diversity of the defensive compounds found in these diverse cyanobacterial species. Results of this study are consistent with previous findings and confirm the food preference of S. rickettsi for these filamentous cyanobacteria, consistent with other species of Stylocheilus (Pennings and Paul, 1993; Pennings et al., 1996; Capper and Paul, 2008).
Veraguamides are encompassed within the kulolide superfamily of related cyclodepsipeptides produced by marine benthic cyanobacteria (Boudreau et al., 2012). In 2011, veraguamides A to L were published in parallel efforts (Mevers et al., 2011; Salvador et al., 2011) (two representative structures shown in Figure 5). Veraguamides A-G were isolated from Symploca cf. hydnoides from reef habitats in Guam (Salvador et al., 2011) and veraguamides A-C and H-L were isolated from Okeania sp. PAC-17-FEB-10-2 from an intertidal area offshore of a small island within Coiba National Park, Panama (Mevers et al., 2011). Interestingly, veraguamides A to C were isolated from two different cyanobacterial genera, as well as from geographically isolated locations in the Indo-Pacific and Eastern Pacific. It is possible that the cyanobacterial source of veraguamides M (1) and N (2), which we refer to as cf. Lyngbya sp., may be Okeania sp., since the morphologically similar cyanobacteria were both collected in Coiba National Park in Panama and contain similar compounds. However, it was not possible to carry out phylogenetic testing on the voucher specimens collected for the present study.
In 1998, two related depsipeptides within the kulolide superfamily, kulomo‘opunalide-1 (5) and -2 (6), were isolated from the marine gastropod Philinopsis speciosa, collected in the intertidal area offshore of O‘ahu, Hawai‘i (Nakao et al., 1998). P. speciosa is a generalist carnivore, predating on opisthobranch molluscs, including S. striatus (Nakao et al., 1998; Zamora-Silva and Malaquias, 2016). In feeding experiments, Nakao et al. (1998) demonstrated that P. speciosa fed on S. striatus, and they were able to isolate kulolide-1, a related depsipeptide, from both the predator and its prey. In addition, they noted that S. striatus feeds on cf. Lyngbya sp., although isolation and/or identification was not performed from the cyanobacterial food source (Nakao et al., 1998). However, inclusive of this current study, there are now three distinct reports of isolation of veraguamides from at least two genera of marine cyanobacteria (Mevers et al., 2011; Salvador et al., 2011). Thus, the dietary source of the veraguamide-type compounds, kulomo‘opunalide-1 (5) and -2 (6) and kulolide-1, were likely from marine cyanobacteria, sequestered by S. striatus and then accumulated by its predator P. speciosa. Additionally, P. speciosa had higher concentrations than S. striatus, suggesting that P. speciosa bioaccumulated this dietary-derived compound (Nakao et al., 1998).
Numerous compounds isolated from cyanobacteria have been shown to exhibit strong antimalarial properties (Supplementary Table 1; Fennell et al., 2003; Linington et al., 2007; McPhail et al., 2007; Barbaras et al., 2008; Gutierrez et al., 2010; Shao et al., 2015). Several cyanobacterial compounds, such as nostocarboline (Barbaras et al., 2008), dolastatin-10 (Fennell et al., 2003), and dragomabin (McPhail et al., 2007) have selectivity indexes two to three orders of magnitude greater than veraguamides M (1) and N (2). There are only a few known cyanobacterial compounds, however, with anti-leishmanial properties against L. donovani (Barbaras et al., 2008; Balunas et al., 2010, 2012; Sanchez et al., 2010). Herein, veraguamide N (2) was shown to exhibit modest anti-leishmanial properties (Supplementary Table 2). In addition, some of the previously isolated veraguamides showed moderate to weak cytotoxicity against cancer cell lines, although none of the previous veraguamide isolates were assessed for anti-leishmanial or antimalarial properties (Mevers et al., 2011).
Marine chemical ecology studies have important applications in the field of natural products based drug discovery (Simmons et al., 2005; Matthew et al., 2007; Wan et al., 2021). There are several examples of potential anticancer agents, sourced from marine cyanobacteria, which have been shown to be sequestered by the sea hare S. striatus (Luesch et al., 2002) including malyngamide A (Paul and Pennings, 1991), lyngbyatoxin A (Cardellina et al., 1979; Capper et al., 2005) and aplysiatoxin (Kato and Scheuer, 1974; Supplementary Table 3). The findings presented in this study provide further examples of dietary-derived cf. Lyngbya sp. compounds, veraguamide M (1) and N (2), sequestered by S. rickettsi and possibly by D. nicaraguana, which are also active against the clinically important diseases malaria and leishmaniasis.
Both sequestered compounds are active toward protozoan parasites, with this bioactivity evident in the digestive gland of the S. rickettsi. However, it remains untested whether dietary-acquired secondary metabolites provide defense against protozoan parasites in molluscs. Marine parasites, however, affect all trophic levels from individual to ecosystems, regulating host abundance, modifying traits, and can indirectly affect species interactions and community structure (Coen and Bishop, 2015). Molluscs have a complex response to parasites, including anatomic barriers, immune cell response, and physiological elements such as the complement system including proteins mainly synthesized in the liver (Al-Khalaifah and Al-Nasser, 2019).
In laboratory-based feeding assays and using food sources collected from nearby tidal pools, D. nicaraguana was offered a variety of cyanobacteria and algae food options. Out of all the food options offered, D. nicaraguana significantly preferred cf. Lyngbya sp. Trace amounts of bioactive compounds isolated from cf. Lyngbya sp., were found in the Dolabrifera tissues. This study suggests that Dolabrifera, like fellow sea hare Stylocheilus, may have the capacity to sequester and store dietary-derived compounds with biotechnological potential, albeit in much lower abundance. Further feeding studies with the isolated compounds incorporated into artificial diets are needed to fully assess sequestration by D. nicaraguana.
Moreover, this study is the first example whereby compounds with significant activity against parasites responsible for tropical diseases have been found in both sea hares and their cyanobacterial food source. This study provides additional evidence that chemical ecological studies of sea hares and their cyanobacterial food sources not only provide insight into trophic relationships in marine invertebrates and their food sources but may also facilitate the search for compounds with important biological activities and biotechnological potential.
The LC-MS/MS datasets presented in this study can be found at http://gnps.ucsd.edu in the MassIVE repository (accession: MSV000080055).
KEC collected the field samples, ran the feeding assays and carried out statistical analysis under the advice of AC and VJP. KEC processed samples under the advice of AC, VJP, and MJB. KEC, W-TL, AMF, PCD, and MJB ran analytical analyses on the cyanobacterial samples. AA, GDT, and LH carried out the bioassays. W-TL and MJB elucidated the cyanobacterial compounds. KEC was supervised by TJ, TLC, and MJB. KEC and MJB wrote the manuscript. All authors assisted in editing the manuscript.
Financial support was received through the Natural Sciences and Engineering Research Council of Canada – Canada Graduate Scholarships - Master’s (NSERC CGS M), Levinson and STRI/McGill NEO fellowships (to KEC). Funding for this research was also provided by the Fogarty International Center International Cooperative Biodiversity Group (ICBG) program based in Panama (U01 TW006634, PI William H. Gerwick).
PCD has stock in Sirenas and Galileo and is a scientific advisor to Cybele and scientific advisor and co-founder of Ometa and Enveda.
The remaining authors declare that the research was conducted in the absence of any commercial or financial relationships that could be construed as a potential conflict of interest.
All claims expressed in this article are solely those of the authors and do not necessarily represent those of their affiliated organizations, or those of the publisher, the editors and the reviewers. Any product that may be evaluated in this article, or claim that may be made by its manufacturer, is not guaranteed or endorsed by the publisher.
We would like to thank the Ministerio de Ambiente de Panamá (MiAmbiente) formerly known as the Autoridad Nacional del Ambiente (ANAM) for providing access to the collection sites and the biological samples. We would also like to thank F. Vargas and B. Teke for carrying out ESIMS/MS acquisition (University of California, San Diego), A. Hermosillo (Universidad de Guadalajara) for participating in the initial exploratory scuba trip to Coiba to locate sea hare habitats, A. Ibáñez (STRI) for field assistance, R. Noble for advice on statistical analyses, Jean Pigozzi of the Liquid Jungle Lab located on Isla de Canales de la Tierra, and Naos Marine and Molecular Laboratories at STRI for laboratory and aquarium space, C. F. Gurgel (Smithsonian Marine Station, Fort Pierce) for identifying the algae samples, R. Pike (CREO) for performing sea hare MS analysis, and the Panama ICBG technicians M. Ng, L. Pineda, R. Contreras and L. D. Ureña for carrying out bioassays.
The Supplementary Material for this article can be found online at: https://www.frontiersin.org/articles/10.3389/fmars.2021.766282/full#supplementary-material
Al-Khalaifah, H., and Al-Nasser, A. (2019). “Immune response of molluscs,” in Molluscs, eds G. Diarte-Plata and R. Escamilla-Montes (IntechOpen).
Arthur, K. E., Paul, V. J., Paerl, H. W., O’Neil, J. M., Joyner, J., and Meickle, T. (2009). Effects of nutrient enrichment of the cyanobacterium Lyngbya sp. on growth, secondary metabolite concentration and feeding by the specialist grazer Stylocheilus striatus. Mar. Ecol. Prog. Ser. 394, 101–110. doi: 10.3354/meps08311
Bacon, C. D., Silvestro, D., Jaramillo, C., Smith, B. T., Chakrabarty, P., and Antonelli, A. (2015). Biological evidence supports an early and complex emergence of the Isthmus of Panama. Proc. Natl. Acad. Sci. U.S.A. 112, 6110–6115. doi: 10.1073/pnas.1423853112
Balunas, M. J., Grosso, M. F., Villa, F. A., Engene, N., McPhail, K. L., Tidgewell, K., et al. (2012). Coibacins A-D, antileishmanial marine cyanobacterial polyketides with intriguing biosynthetic origins. Org. Lett. 14, 3878–3881. doi: 10.1021/ol301607q
Balunas, M. J., Linington, R. G., Tidgewell, K., Fenner, A. M., Urena, L., Togna, G. D., et al. (2010). Dragonamide E, a modified linear lipopeptide from Lyngbya majuscula with antileishmanial activity. J. Nat. Prod. 73, 60–66. doi: 10.1021/np900622m
Barbaras, D., Kaiser, M., Brun, R., and Gademann, K. (2008). Potent and selective antiplasmodial activity of the cyanobacterial alkaloid nostocarboline and its dimers. Bio. Med. Chem. Lett. 18, 4413–4415. doi: 10.1016/j.bmcl.2008.06.049
Bazzicalupo, E., Crocetta, F., Gosliner, T. M., Berteaux-Lecellier, V., Camacho-García, Y. E., Chandran, B. K. S., et al. (2020). Molecular and morphological systematics of Bursatella leachii de Blainville, 1817 and Stylocheilus striatus Quoy & Gaimard, 1832 reveal cryptic diversity in pantropically distributed taxa (Mollusca:Gastropoda:Heterobranchia). Invertebr Syst. 34, 535–568. doi: 10.1071/IS19056
Benkendorff, K., Davis, A., and Bremner, J. (2001). Chemical defense in the egg masses of benthic invertebrates: an assessment of antibacterial activity in 39 mollusks and 4 polychaetes. J. Invert. Pathol. 78, 109–118. doi: 10.1006/jipa.2001.5047
Blunt, J. W., Copp, B. R., Keyzers, R. A., Munro, M. H. G., and Prinsep, M. R. (2012). Marine natural products. Nat. Prod. Rep. 29, 144–222. doi: 10.1039/C2NP00090C
Bornancin, L., Bonnard, I., Mills, S. C., and Banaigs, B. (2017). Chemical mediation as a structuring element in marine gastropod predator-prey interactions. Nat. Prod. Rep. 34, 644–676. doi: 10.1039/C6NP00097E
Boudreau, P. D., Byrum, T., Liu, W.-T., Dorrestein, P. C., and Gerwick, W. H. (2012). Viequeamide A, a cytotoxic member of the kulolide superfamily of cyclic depsipeptides from a marine button cyanobacterium. J. Nat. Prod. 75, 1560–1570. doi: 10.1021/np300321b
Burja, A. M., Banaigs, B., Abou-Mansour, E., Burgess, G. J., and Wright, P. C. (2001). Marine cyanobacteria - a prolific source of natural products. Tetrahedron 57, 9347–9377. doi: 10.1016/S0040-4020(01)00931-0
Caires, T. A., de Mattos Lyra, G., Hentschke, G. S., de Gusmão Pedrini, A., Sant’Anna, C. L., and de Castro Nunes, J. M. (2018). Neolyngbya gen. nov. (Cyanobacteria, Oscillatoriaceae): A new filamentous benthic marine taxon widely distributed along the Brazilian coast. Mol Phylogenet Evol 120, 196–211. doi: 10.1016/j.ympev.2017.12.009
Camacho-García, Y., Gosliner, T. M., and Valdés, Á (2005). Field Guide to the Sea Slugs of the Tropical Eastern Pacific. San Francisco, CA: California Academy of Sciences.
Capper, A. I, Tibbetts, R., O’Neil, J. M., and Shaw, G. R. (2006b). Feeding preference and deterrence in rabbitfish Siganus fuscescens for the cyanobacterium Lyngbya majuscula in Moreton Bay, South-East Queensland, Australia. J. Fish. Biol. 68, 1589–1609. doi: 10.1111/j.1095-8649.2006.01048.x
Capper, A. I., Tibbetts, R., O’Neil, J. M., and Shaw, G. R. (2006a). Dietary selectivity for the toxic cyanobacterium Lyngbya majuscula and resultant growth rates in two species of opisthobranch mollusc. J. Exp. Mar. Biol. Ecol. 331, 133–144. doi: 10.1016/j.jembe.2005.10.009
Capper, A., and Paul, V. J. (2008). Grazer interactions with four species of Lyngbya in southeast Florida. Harmful Algae 7, 717–728. doi: 10.1016/j.hal.2008.02.004
Capper, A., Erickson, A. A., Ritson-Williams, R., Becerro, M. A., Arthur, K. A., and Paul, V. J. (2016). Palatability and chemical defences of benthic cyanobacteria to a suite of herbivores. J. Exp. Mar. Biol. Ecol. 474, 100–108. doi: 10.1016/j.jembe.2015.09.008
Capper, A., Tibbetts, I., O’Neil, J., and Shaw, G. (2005). The fate of Lyngbya majuscula toxins in three potential consumers. J. Chem. Ecol. 31, 1594–1606. doi: 10.1007/s10886-005-5800-5
Cardellina, J. H. II, Marner, F. J., and Moore, R. E. (1979). Seaweed dermatitis: structure of lyngbyatoxin A. Science 204, 193–195. doi: 10.1126/science.107586
Ciavatta, M. L., Gavagnin, M., Puliti, R., and Cimino, G. (1996). Dolabriferol: a new polypropionate from the skin of the anaspidean mollusc Dolabrifera dolabrifera. Tetrahedron 52, 121831–121838. doi: 10.1016/0040-4020(96)00764-8
Cimino, G., and Ghiselin, M. T. (2009). Chemical defense and the evolution of opisthobranch gastropods. Proc. Calif. Acad. Sci. 60:175.
Clark, K. E., Capper, A., Della Togna, G., Paul, V. J., Romero, L. I., Johns, T., et al. (2013). Ecology and bioassay-guided drug discovery for treatments of tropical parasitic disease: 5α,8α-Epidioxycholest-6-en-3β-ol isolated from the mollusk Dolabrifera dolabrifera shows significant activity against Leishmania donovani. Nat. Prod. Commun. 8, 1537–1540. doi: 10.1177/1934578X1300801109
Coen, L. D., and Bishop, M. J. (2015). The ecology, evolution, impacts and management of host–parasite interactions of marine molluscs. J. Invertebr. Pathol. 131, 177–211. doi: 10.1016/j.jip.2015.08.005
Cronin, G., and Hay, M. E. (1996). Susceptibility to herbivores depends on recent history of both the plant and animal. Ecology 77, 1531–1543. doi: 10.2307/2265549
Cruz-Rivera, E., and Paul, V. J. (2006). Feeding by coral reef mesograzers: algae or cyanobacteria? Coral. Reefs 25, 617–627. doi: 10.1007/s00338-006-0134-5
Cuvier, G. L. (1817). Le règne Animal Distribué d’après son Organisation: Pour Servir de Base a l’histoire Naturelle des Animaux et d’introduction a l’anatomie Comparée, Les Reptiles, Les Poissons, Les Mollusques et Les Annélides, Vol. 2, (Paris: Chez Déterville), 532. doi: 10.5962/bhl.title.41460
de Nys, R., Steinberg, P. D., Rogers, C. N., Charlton, T. S., and Duncan, M. W. (1996). Quantitative variation of secondary metabolites in the sea hare Aplysia parvula and its host plant Delisea pulchra. Mar. Ecol. Prog. Ser. 130, 135–146.
Engene, N., Choi, H., Esquenazi, E., Rottacker, E. C., Ellisman, M. H., Dorrestein, P. C., et al. (2011). Underestimated biodiversity as a major explanation for the perceived rich secondary metabolite capacity of the cyanobacterial genus Lyngbya. Environ. Microbiol. 13, 1601–1610. doi: 10.1111/j.1462-2920.2011.02472.x
Engene, N., Gunasekera, S. P., Gerwick, W. H., and Paul, V. J. (2013a). Phylogenetic inferences reveal a large extent of novel biodiversity in chemically rich tropical marine cyanobacteria. Appl. Environ. Microbiol. 79, 1882–1888. doi: 10.1128/aem.03793-12
Engene, N., Paul, V. J., Byrum, T., Gerwick, W. H., Thor, A., and Ellisman, M. H. (2013b). Five chemically rich species of tropical marine cyanobacteria of the genus Okeania gen. nov. (Oscillatoriales, Cyanoprokaryota). J. Phycol. 49, 1095–1106. doi: 10.1111/jpy.12115
Engene, N., Rottacker, E. C., Kaštovský, J., Byrum, T., Choi, H., Ellisman, M. H., et al. (2012). Moorea producens gen. nov., sp. nov. and Moorea bouillonii comb. nov., tropical marine cyanobacteria rich in bioactive secondary metabolites. Int. J. Syst. Evol. Microbiol. 62, 1171–1178. doi: 10.1099/ijs.0.033761-0
Engene, N., Tronholm, A., and Paul, V. J. (2018). Uncovering cryptic diversity of Lyngbya: the new tropical marine cyanobacterial genus Dapis (Oscillatoriales). J. Phycol. 54, 435–446. doi: 10.1111/jpy.12752
Erickson, A. A., Paul, V. J., Van Alstyne, K. L., and Kwiatkowski, L. M. (2006). Palatability of macroalgae that use different types of chemical defenses. J. Chem. Ecol. 32, 1883–1895. doi: 10.1007/s10886-006-9116-x
ESRI, Maxar, GeoEye, Earthstar Geographics, Cnes/Airbus Ds, Usda, Usgs, et al. (2021). Basemap: World Imagery. ArcGIS Desktop 10.8.1.
Faulkner, D. J. (1984). Marine natural products: metabolites of marine algae and herbivorous marine molluscs. Nat. Prod. Rep. 1, 251–280.
Fennell, B. J., Carolan, S., Pettit, G. R., and Bell, A. (2003). Effects of the antimitotic natural product dolastatin 10, and related peptides, on the human malarial parasite Plasmodium falciparum. J. Antimicrob. Chemother. 51, 833–841. doi: 10.1093/jac/dkg151
Gallimore, W. A., and Scheuer, P. J. (2000). Malyngamides O and P from the sea hare Stylocheilus longicauda. J. Nat. Prod. 63, 1422–1424. doi: 10.1021/np0000365
Gallimore, W. A., Galario, D. L., Lacy, C., Zhu, Y., and Scheuer, P. J. (2000). Two complex proline esters from the sea hare Stylocheilus longicauda. J. Nat. Prod. 63, 1022–1026. doi: 10.1021/np990640j
Ghazali, S. R. (2006). Displays of Defense: Behavioral Differences in Antagonist Avoidance in Four Opisthobranch Mollusks. Berkeley, California: University of California in Berkeley.
Gutierrez, M., Tidgewell, K., Capson, T. L., Engene, N., Almanza, A., Schemies, J., et al. (2010). Malyngolide dimer, a bioactive symmetric cyclodepside from the Panamanian marine cyanobacterium Lyngbya majuscula. J. Nat. Prod. 73, 709–711. doi: 10.1021/np9005184
Himstead, A., and Wright, W. G. (2018). Precise foraging schedule in an intertidal euopisthobranch mollusk. Mar. Freshw. Behav. Phy. 51, 131–141. doi: 10.1080/10236244.2018.1505430
Jiménez-Romero, C., González, K., and Rodríguez, A. D. (2012). Dolabriferols B and C, non-contiguous polypropionate esters from the tropical sea hare Dolabrifera dolabrifera. Tetrahedron. Lett. 53, 6641–6645. doi: 10.1016/j.tetlet.2012.09.068
Kamio, M., Nguyen, L., Yaldiz, S., and Derby, C. (2010). How to produce a chemical defense: Structural elucidation and anatomical distribution of Aplysioviolin and Phycoerythrobilin in the sea hare Aplysia californica. Chem. Biodivers 7, 1183–1197. doi: 10.1002/cbdv.201000006
Kato, Y., and Scheuer, P. J. (1974). Aplysiatoxin and debromoaplysiatoxin, constituents of the marine mollusk Stylocheilus longicauda (Quoy and Gaimard, 1824). J. Am. Chem. Soc. 96, 2245–2246. doi: 10.1021/ja00814a041
Kikumori, M., Yanagita, R. C., Tokuda, H., Suzuki, N., Nagai, H., Suenaga, K., et al. (2012). Structure–activity studies on the spiroketal moiety of a simplified analogue of debromoaplysiatoxin with antiproliferative activity. J. Med. Chem. 55, 5614–5626. doi: 10.1021/jm300566h
Linington, R. G., Gonzalez, J., Urena, L. D., Romero, L. I., Ortega-Barria, E., and Gerwick, W. H. (2007). Venturamides A and B: antimalarial constituents of the panamanian marine Cyanobacterium Oscillatoria sp. J. Nat. Prod. 70, 397–401. doi: 10.1021/np0605790
Littler, D. S., and Littler, M. M. (2000). Caribbean Reef Plants. Washington, DC: Offshore Graphics, Inc.
Liu, L., and Rein, K. S. (2010). New peptides isolated from Lyngbya species: a review. Mar. Drugs 8, 1817–1837. doi: 10.3390/md8061817
Liu, W.-T., Ng, J., Meluzzi, D., Bandeira, N., Gutierrez, M., Simmons, T. L., et al. (2009). Interpretation of tandem mass spectra obtained from cyclic nonribosomal peptides. Anal. Chem. 81, 4200–4209. doi: 10.1021/ac900114t
Luesch, H., Harrigan, G. G., Goetz, G., and Horgen, F. D. (2002). The cyanobacterial origin of potent anticancer agents originally isolated from sea hares. Curr. Med. Chem. 9, 1791–1806. doi: 10.2174/0929867023369051
Marshall, J. G., and Willan, R. C. (1999). Nudibranchs of Heron Island, Great Barrier Reef: a Survey of the Opisthobranchia (sea slugs) of Heron and Wistari reefs., Leiden: Backhuys.
Matthew, S., Ross, C., Rocca, J. R., Paul, V. J., and Luesch, H. (2007). Lyngbyastatin 4, a dolastatin 13 analogue with elastase and chymotrypsin inhibitory activity from the marine cyanobacterium Lyngbya confervoides. J. Nat. Prod. 70, 124–127. doi: 10.1021/np060471k
McPhail, K. L., Correa, J., Linington, R. G., Gonzalez, J., Ortega-Barria, E., Capson, T. L., et al. (2007). Antimalarial linear lipopeptides from a Panamanian strain of the marine cyanobacterium Lyngbya majuscula. J. Nat. Prod. 70, 984–988. doi: 10.1021/np0700772
Mevers, E., Liu, W.-T., Engene, N., Mohimani, H., Byrum, T., Pevzner, P. A., et al. (2011). Cytotoxic veraguamides, alkynyl bromide-containing cyclic depsipeptides from the marine cyanobacterium cf. Oscillatoria margaritifera. J. Nat. Prod. 74, 928–936. doi: 10.1021/np200077f
Miller, M. C. (1969). The habits and habitats of the opisthobranch molluscs of the British Solomon Islands. Phil. Trans. R. Soc. Lond. B 255, 541–548. doi: 10.1098/rstb.1969.0024
Mohimani, H., Yang, Y.-L., Liu, W.-T., Hsieh, P.-W., Dorrestein, P. C., and Pevzner, P. A. (2011). Sequencing cyclic peptides by multistage mass spectrometry. Proteomics 11, 3642–3650. doi: 10.1002/pmic.201000697
Moreno, E., Varughese, T., Spadafora, C., Arnold, A. E., Coley, P. D., Kursar, T. A., et al. (2011). Chemical constituents of the new endophytic fungus Mycosphaerella sp. nov. and their anti-parasitic activity. Nat. Prod. Commun. 6:1934578X1100600620. doi: 10.1177/1934578x1100600620
Nagle, D. G., and Paul, V. J. (1998). Chemical defense of a marine cyanobacterial bloom. J. Exp. Mar. Biol. Ecol. 225, 29–38. doi: 10.1016/S0022-0981(97)00205-0
Nagle, D. G., and Paul, V. J. (1999). Production of secondary metabolites by filamentous tropical marine cyanobacteria: ecological functions of the compounds. J. Phycol. 35, 1412–1421. doi: 10.1046/j.1529-8817.1999.3561412.x
Nagle, D. G., Camacho, F. T., and Paul, V. J. (1998). Dietary preferences of the opisthobranch mollusc Stylocheilus longicauda for secondary metabolites produced by the tropical cyanobacterium Lyngbya majuscula. Mar. Biol. 132, 267–273. doi: 10.1007/s002270050392
Nakao, Y., Yoshida, W. Y., Szabo, C. M., Baker, B. J., and Scheuer, P. J. (1998). More peptides and other diverse constituents of the marine mollusk Philinopsis speciosa. J. Org. Chem. 63, 3272–3280. doi: 10.1021/jo9719867
Ng, J., Bandeira, N., Liu, W.-T., Ghassemian, M., Simmons, T. L., Gerwick, W., et al. (2009). Dereplication and de novo sequencing of nonribosomal peptides. Nat. Methods 6, 596–599. doi: 10.1038/nmeth.1350
Nimbs, M. J., Willan, R. C., and Smith, S. D. A. (2017). A Historical summary of the distribution and diet of Australian sea hares (Gastropoda: Heterobranchia: Aplysiidae). Zool Stud. 56:e35. doi: 10.6620/ZS.2017.56-35
Nunnery, J. K., Mevers, E., and Gerwick, W. H. (2010). Biologically active secondary metabolites from marine cyanobacteria. Curr. Opin. Biotechnol. 21, 787–793. doi: 10.1016/j.copbio.2010.09.019
O’Dea, A., Lessios, H. A., Coates, A. G., Eytan, R. I., Restrepo-Moreno, S. A., Cione, A. L., et al. (2016). Formation of the Isthmus of Panama. Sci. Adv. 2:e1600883. doi: 10.1126/sciadv.1600883
Paul, V. J., and Pennings, S. C. (1991). Diet-derived chemical defenses in the sea hare Stylocheilus longicauda (Quoy et Gaimard 1824). J. Exp. Mar. Biol. Ecol. 151, 227–243. doi: 10.1016/0022-0981(91)90126-H
Pavlik, C. M., Wong, C. Y. B., Ononye, S., Lopez, D. D., Engene, N., McPhail, K. L., et al. (2013). Santacruzamate A, a potent and selective histone deacetylase inhibitor from the Panamanian marine cyanobacterium cf. Symploca sp. J. Nat. Prod. 76, 2026–2033. doi: 10.1021/np400198r
Pennings, S. C., and Paul, V. J. (1993). Sequestration of dietary secondary metabolites by three species of sea hares: location, specificity and dynamics. Mar. Biol. 117, 535–546. doi: 10.1007/BF00349763
Pennings, S. C., Nadeau, M. T., and Paul, V. J. (1993). Selectivity and growth of the generalist herbivore Dolabella auricularia feeding upon complementary resources. Ecology 74, 879–890. doi: 10.2307/1940813
Pennings, S. C., Pablo, S. R., and Paul, V. J. (1997). Chemical defenses of the tropical, benthic marine cyanobacterium Hormothamnion enteromorphoides: diverse consumers and synergisms. Limnol. Oceanogr. 42, 911–917. doi: 10.4319/lo.1997.42.5.0911
Pennings, S. C., Paul, V. J., Dunbar, D. C., Hamann, M. T., Lumbang, W. A., and Novack, B. (1999). Unpalatable compounds in the marine gastropod Dolabella auricularia: distribution and effect of diet. J. Chem. Ecol. 25, 735–755. doi: 10.1023/A:1020832414766
Pennings, S. C., Weiss, A. M., and Paul, V. J. (1996). Secondary metabolites of the cyanobacterium Microcoleus lyngbyaceus and the sea hare Stylocheilus longicauda: Palatability and toxicity. Mar. Biol. 126, 735–743. doi: 10.1007/BF00351340
Peterson, C. H., and Renaud, P. E. (1989). Analysis of feeding preference experiments. Oecologia 80:82. doi: 10.1007/BF00789935
Pettit, G. R., Kamano, Y., Herald, C. L., Tuinman, A. A., Boettner, F. E., Kiza, H., et al. (1987). The isolation and structure of a remarkable marine animal antineoplastic constituent: dolastatin 10. J. Am. Chem. Soc. 109, 6883–6995. doi: 10.1021/ja00256a070
Prince, J. S., and Johnson, P. M. (2006). Ultrastructural comparison of Aplysia and Dolabrifera ink glands suggests cellular sites of anti-predator protein production and algal pigment processing. J Mollus Stud 72, 349–357. doi: 10.1093/mollus/eyl017
Quoy, J. R., and Gaimard, J. P. (1832). Voyage de decouvertes de L’Astrolabe pendant les annees 1826-1827-1828-1829, sous le commandement de M.J. Dumont D’Urville, Zoologie. 2, 1–686.
Rogers, C. N., Steinberg, P. D., and de Nys, R. (1995). Factors associated with oligophagy in two species of sea hares (Mollusca: Anaspidea). J. Exp. Mar. Biol. Ecol. 192, 47–73. doi: 10.1016/0022-0981(95)00057-X
Rose, A. F., Scheuer, P. J., Springer, J. P., and Clardy, J. (1978). Stylocheilamide, an unusual constituent of the sea hare Stylocheilus longicauda. J. Am. Chem. Soc. 100, 7665–7670. doi: 10.1021/ja00492a039
Rotter, A., Barbier, M., Bertoni, F., Bones, A. M., Cancela, M. L., Carlsson, J., et al. (2021). The essentials of marine biotechnology. Front. Mar. Sci. 8:629. doi: 10.3389/fmars.2021.629629
Rudman, W. B. (2003). Comment on My First Sea Slug by Stanisław Sitek. Sea Slug Forum. Available online at: http://www.seaslugforum.net/showall/doladola (accessed March 3, 2006).
Salvador, L. A., Biggs, J. S., Paul, V. J., and Luesch, H. (2011). Veraguamides A-G, cyclic hexadepsipeptides from a dolastatin 16-producing cyanobacterium Symploca cf. hydnoides from Guam. J. Nat. Prod. 74, 917–927. doi: 10.1021/np200076t
Sanchez, L. M., Lopez, D., Vesely, B. A., Della Togna, G., Gerwick, W. H., Kyle, D. E., et al. (2010). Almiramides A-C: discovery and development of a new class of leishmaniasis lead compounds. J. Med. Chem. 53, 4187–4197. doi: 10.1021/jm100265s
Shao, C.-L., Linington, R. G., Balunas, M. J., Centeno, A., Boudreau, P., Zhang, C., et al. (2015). Bastimolide A, a potent antimalarial polyhydroxy macrolide from the marine cyanobacterium Okeania hirsuta. J. Org. Chem. 80, 7849–7855. doi: 10.1021/acs.joc.5b01264
Simmons, T. L., Andrianasolo, E., McPhail, K., Flatt, P., and Gerwick, W. H. (2005). Marine natural products as anticancer drugs. Mol. Cancer Ther. 4, 333–342.
Stallard, M. O., and Faulkner, D. J. (1974). Chemical constituents of the digestive gland of the sea hare Aplysia californica—I. importance of diet. Comput. Biochem. Physiol. B 49, 25–35. doi: 10.1016/0305-0491(74)90218-1
Switzer-Dunlap, M., and Hadfield, M. G. (1977). Observations on development, larval growth and metamorphosis of four species of aplysiidae (Gastropoda: Opisthobranchia) in laboratory culture. J. Exp. Mar. Biol. Ecol. 29, 245–261. doi: 10.1016/0022-0981(77)90069-7
Takagi, K. K., Ono, N. N., and Wright, W. G. (2010). Interspecific variation in palatability suggests cospecialization of antipredator defenses in sea hares. Mar. Ecol. Prog. Ser. 416, 137–144. doi: 10.3354/meps08738
Thajuddin, N., and Subramanian, G. (2005). Cyanobacterial biodiversity and potential applications in biotechnology. Curr. Sci. 89, 47–57.
Tronholm, A., and Engene, N. (2019). Moorena gen. nov., a valid name for “Moorea Engene & al.” nom. inval. (Oscillatoriaceae, Cyanobacteria). Notulae Algarum 122, 1–2.
Valdés, Á, Breslau, E., Padula, V., Schrödl, M., Camacho, Y., Malaquias, M. A. E., et al. (2018). Molecular and morphological systematics of Dolabrifera Gray, 1847 (Mollusca: Gastropoda: Heterobranchia: Aplysiomorpha). Zool. J. Linn. Soc. 184, 31–65. doi: 10.1093/zoolinnean/zlx099
Wan, M.-C., Qin, W., Lei, C., Li, Q.-H., Meng, M., Fang, M., et al. (2021). Biomaterials from the sea: future building blocks for biomedical applications. Bioact Mater 6, 4255–4285. doi: 10.1016/j.bioactmat.2021.04.02
Waston, M. (1973). Midgut gland toxins of Hawaiian sea hares-1.isolation and preliminary toxicological observations. Toxicon 2, 259–267. doi: 10.1016/0041-0101(73)90053-6
Youssef, D. T., Shaala, L. A., Mohamed, G. A., Ibrahim, S. R., Banjar, Z. M., Badr, J. M., et al. (2015). 2,3-seco-2,3-dioxo-lyngbyatoxin a from a red sea strain of the marine cyanobacterium Moorea producens. Nat. Prod. Res. 29, 703–709. doi: 10.1080/14786419.2014.982647
Keywords: marine chemical ecology, sea hares and cyanobacteria, cf. Lyngbya sp. (formerly Lyngbya majuscula), Dolabrifera nicaraguana (formerly D. dolabrifera), Stylocheilus rickettsi (formerly S. striatus), bioactive secondary metabolites, tropical parasitic diseases, veraguamides
Citation: Clark KE, Capper A, Liu W-T, Fenner AM, Almanza A, Della Togna G, Herrera L, Johns T, Paul VJ, Dorrestein PC, Capson TL and Balunas MJ (2021) Sequestration and Cyanobacterial Diet Preferences in the Opisthobranch Molluscs Dolabrifera nicaraguana and Stylocheilus rickettsi. Front. Mar. Sci. 8:766282. doi: 10.3389/fmars.2021.766282
Received: 28 August 2021; Accepted: 27 September 2021;
Published: 10 November 2021.
Edited by:
Mirko Mutalipassi, Anton Dohrn Zoological Station, ItalyReviewed by:
Raju Mohanraju, Pondicherry University, IndiaCopyright © 2021 Clark, Capper, Liu, Fenner, Almanza, Della Togna, Herrera, Johns, Paul, Dorrestein, Capson and Balunas. This is an open-access article distributed under the terms of the Creative Commons Attribution License (CC BY). The use, distribution or reproduction in other forums is permitted, provided the original author(s) and the copyright owner(s) are credited and that the original publication in this journal is cited, in accordance with accepted academic practice. No use, distribution or reproduction is permitted which does not comply with these terms.
*Correspondence: Marcy J. Balunas, bWFyY3kuYmFsdW5hc0B1Y29ubi5lZHU=
†Present address: Angela Capper, Coastal Marine Ecosystems Research Centre (CMERC), School of Health Medical and Applied Sciences, CQUniversity, Gladstone, QLD, Australia; Gina Della Togna, Universidad Interamericana de Panamá, Dirección de Investigación, Panama City, Panama; Todd L. Capson, Institut de Physique du Globe de Paris, Université de Paris, Paris, France
Disclaimer: All claims expressed in this article are solely those of the authors and do not necessarily represent those of their affiliated organizations, or those of the publisher, the editors and the reviewers. Any product that may be evaluated in this article or claim that may be made by its manufacturer is not guaranteed or endorsed by the publisher.
Research integrity at Frontiers
Learn more about the work of our research integrity team to safeguard the quality of each article we publish.