- 1CCMAR, CIMAR, Universidade do Algarve, Faro, Portugal
- 2Centre for Blue Governance, University of Portsmouth, Portsmouth, United Kingdom
- 3IBAP - Instituto da Biodiversidade e Áreas Protegidas, Bissau, Guinea-Bissau
- 4PNBA - National Park of the Banc d’Arguin, Nouadhibou, Mauritania
- 5Associação Projecto Vitó, Sao Filipe, Cabo Verde
- 6CIBIO-InBIO, Centro de Investigação em Biodiversidade e Recursos Geneticos, Porto, Portugal
Marine Protected Areas (MPAs) must function as networks with sufficient stepping-stone continuity between suitable habitats to ensure the conservation of naturally connected regional pools of biodiversity in the long-term. For most marine biodiversity, population connectivity is mediated by passively dispersed planktonic stages with contrasting dispersal periods, ranging from a few hours to hundreds of days. These processes exert a major influence on whether threatened populations should be conserved as either isolated units or linked metapopulations. However, the distance scales at which individual MPAs are connected are insufficiently understood. Here, we use a biophysical model integrating high-resolution ocean currents and contrasting dispersal periods to predict connectivity across the Network of MPAs in Western Africa. Our results revealed that connectivity differs sharply among distinct ecological groups, from highly connected (e.g., fish and crustacea) to predominantly isolated ecosystem structuring species (e.g., corals, macroalgae and seagrass) that might potentially undermine conservation efforts because they are the feeding or nursery habitats required by many other species. Regardless of their dispersal duration, all ecological groups showed a common connectivity gap in the Bijagós region of Guinea-Bissau, highlighting the important role of MPAs there and the need to further support and increase MPA coverage to ensure connectivity along the whole network. Our findings provide key insights for the future management of the Network of MPAs in Western Africa, highlighting the need to protect and ensure continuity of isolated ecosystem structuring species and identifying key regions that function as stepping-stone connectivity corridors.
Introduction
Conservation theory has long highlighted the need for ensuring connectivity between protected areas, but the focus has been mainly on highly mobile taxa, particularly among vertebrates (Magris et al., 2014; Balbar and Metaxas, 2019). Despite their potential keystone role in ecosystems, such large mobile vertebrates are a small fraction of all biodiversity. Most populations along species ranges are passively connected, often by abiotic factors, with distance scales that are insufficiently understood. This is particularly striking for marine species, the majority of which are dispersed by planktonic stages that differ greatly in longevity, ranging from a few hours to hundreds of days (Shanks et al., 2003; Treml et al., 2012). The duration of planktonic dispersal can exert a major influence on connectivity and consequently, on whether populations function and should be managed as either, isolated units or a metapopulation with sources and stepping stone regions allowing a regionally larger biodiversity pool to persist (D’Aloia et al., 2017; Assis et al., 2021). To ensure conservation of regional pools of biodiversity, marine protected areas (MPAs) must function as networks with sufficient continuity of suitable habitats, allowing passive connectivity by ensuring stepping stone migration nodes for all species.
Maintaining effective connectivity is particularly relevant in the scope of offsetting impacts of climate change and other localized disturbances, such as overexploitation or pollution. Well-connected networks of MPAs can reduce and reverse disturbances through the replenishment of the impacted populations from external connected sources, thus, enhancing resilience and decreasing the risk of local extinctions (Magris et al., 2014; Fung et al., 2017). For instance, climate-induced mass mortality events (e.g., extreme conditions during heatwaves; Garrabou et al., 2009) can be reversed by replenishing impacted populations from well-connected sources (Bonin et al., 2016). Even extremely low connectivity achieved only through sporadic or rare events, can be sufficient to bridge distinct oceanographic regions shaped by strong oceanographic barriers and displaying distinct communities across taxa (Spalding et al., 2007). Evidence of sporadic gene flow events across such major barriers have been reported for different taxa (Lessios et al., 1998; Waters and Roy, 2004; Baus et al., 2005; Assis et al., 2018).
The importance of maximizing connectivity in marine management and conservation has been stressed in the Convention on Biological Diversity target 11 (also known as the Aichi Target 11), mandating the establishment of effectively managed and well-connected MPAs (or other spatial conservation measures). The post-2020 Global Biodiversity Framework (CBD; Convention on Biological Diversity (2021), currently being discussed, which aims to expand protection cover to at least 30% of sea areas, also places emphasis on the implementation of well-connected systems of protected areas. However, in practice, connectivity has rarely been considered in management planning (Balbar and Metaxas, 2019), often leaving connectivity gaps that generate isolated subnetworks, far from optimized for effective conservation. For instance, the European network of no-take MPAs has numerous isolated areas, which can compromise effective connectivity of biodiversity, despite being in a global “hotspot” of MPAs (Assis et al., 2021). In general, the extent to which MPA networks are able to provide the ecological benefits of connected metapopulations is unknown. Evaluating their potential connectivity status and identifying potential gaps is thus important for better planning and application of resources in management and conservation (Andrello et al., 2017; Lindegren et al., 2018). This is even more urgent for regions still harboring high but threatened biodiversity, currently undergoing great pressures (e.g., climate change or overexploitation).
The MPAs of the Western coast of Africa between Mauritania and Sierra Leone represent a case of important commercial (e.g., fisheries resources) and ecosystem-supporting species (e.g., seagrasses, macroalgae, corals and other invertebrates) under pressure (Gascuel et al., 2007; Durand et al., 2013). These MPAs were initially delineated to protect large and threatened vertebrates but have progressively been considering also the protection of fisheries resources and traditional means of human subsistence. However, most of the marine biodiversity of these coastlines has not been considered in this planning, including ecological groups that form structural ecosystems upon which other species depend for feeding and nursery habitats. The number of MPAs in the region has expanded greatly with the creation of the Regional Marine and Coastal Program (PRCM), from 35 in 2003 to 88 in 2018, with coverage augmenting from 17,000 to 27,000 km2 (Failler et al., 2020c). The creation of the Network for West African MPAs (Réseau Régional d’Aires Marine Protegées en Afrique de l’Ouest; RAMPAO) in 2007, aimed to enhance the coherence of MPAs and ensure that they were fully represented at the political and public policy levels, but cross-border connectivity was neglected then, with MPAs being implemented at regional or national levels. Soon after the RAMPAO implementation, connectivity between MPAs was recognized as decisive to ensure overall coherence of the MPAs network (Weigel et al., 2007). However, the RAMPAO was mainly a demand drive process initiated by both the countries and the communities and thus not used to ensure suitable migratory corridors between MPAs. An overall assessment of biodiversity connectivity across all countries in the RAMPAO is thus important to integrate management and conservation actions of the network as a whole.
This study aims to address this knowledge gap by inferring marine connectivity between MPAs across borders along the West African network of MPAs (from Mauritania to Sierra-Leone, inc., Cape Verde archipelago) for major ecological groups of marine biodiversity with distinct planktonic dispersal traits mediated by oceanographic currents. These are inferred using a bio-physical modeling framework and network analyses, to estimate and illustrate the different connectivity levels across passively dispersed marine taxa as a function of their propagule duration (PD) and to identify key areas acting as connectivity corridors essential for preventing network fragmentation. Compared to other approaches aimed to estimate connectivity (e.g., genetic and chemical element analyses; Hellberg et al., 2002; Gillanders, 2005), which generally address single species or ecological groups (Pujolar et al., 2013; Johansson et al., 2015; Assis et al., 2018), and despite some potential limitations linked to unknown biological parameters (e.g., behavior and competence of larvae), bio-physical modeling allows covering multiple taxa through PD parametrization, as well as broad spatial and temporal scales. When incorporating high-resolution oceanographic and geophysical data, this line of modeling increases the realism of connectivity processes, and recurrently produces estimates matching those from empirical data (Pujolar et al., 2013; Assis et al., 2015, 2018; Buonomo et al., 2016; Cunha et al., 2017). This study provides the first connectivity assessment of West African MPAs, filling an important gap of scientific information needed for the planning of the overall coherence of the regional MPA network.
Materials and Methods
Study Region and Marine Protected Area Network
The present study focuses on the officially registered MPAs in a region of Western Africa comprising the coastline between Mauritania and Sierra-Leone, including the Cape-Verde archipelago, coinciding with the distribution of the Network for West African MPAs (RAMPAO). The database produced by Failler et al. (2020c) was used as a starting point for assembling a spatial database of MPA polygons matching MPA limits. This database was pruned to exclusively consider the polygon boundaries that were strictly marine, outside closed or inland embayments. To this end, a high-resolution polygon defining global land masses (Haklay and Weber, 2008) was used as an erasing clipping mask over the MPA polygons. The resulting group of MPAs is hereafter designated in this study the west African MPA network.
Connectivity Model
Connectivity estimates of the West African MPA network were inferred using a bio-physical model (Assis et al., 2015, 2018; Ntuli et al., 2020) feeding on daily data from the Hybrid Coordinate Ocean Model (HYCOM; version 2.2), a high-resolution hindcast of three-dimensional ocean velocity fields available for the global ocean at a spatial resolution of 0.08°, with 40 standard depth levels, and covering the period from 1992, onward. The HYCOM model is forced by wind stress, wind speed, precipitation and heat flux, assimilating data from satellites and in situ measurements from an array of bathythermographs, moored buoys and Argo floats across the globe (for detailed information on HYCOM please refer to Chassignet et al., 2007). This allows estimating connectivity transport and barriers, while mimicking, within the limits of the spatial and temporal resolution of HYCOM, key oceanographic processes such as oceanic eddies, fronts, filaments and meandering currents (Lett et al., 2008). While no comprehensive validation of HYCOM is available for the study area, model performance assessed for broadscale oceanographic patterns (e.g., in South Pacific, North Atlantic Ocean) found accurate estimates of the main features of current flow (i.e., direction and intensity; Fossette et al., 2012; Kendall et al., 2013). Additionally, connectivity estimates based on HYCOM data recurrently explained genetic and demographic traits of numerous marine taxa across the global ocean (e.g., Assis et al., 2015, 2018; Buonomo et al., 2016; Klein et al., 2016; Cunha et al., 2017; Ntuli et al., 2020). These considered the basic dispersal ecology of species, with no integration of specific parameters like buoyancy capacity or behavior (e.g., vertical migration in the water column), which could influence dispersal estimates (Romero et al., 2012). However, at large spatial scales, as in the present study, the approach is suitable to estimate connectivity (Shanks et al., 2003; Cowen et al., 2006; Assis et al., 2015, 2018; Ntuli et al., 2020).
The bio-physical model estimated connectivity through individual particles representing propagules (i.e., any type of planktonic dispersal stage such as gametes, spores, seeds, larvae, drifting fragments) that were advected passively by ocean currents. The particles were released from sites located 1 km apart (here defined as source sites) along the limits of each MPA polygon, on a daily basis, throughout a complete year. Land mass was defined by a high-resolution polygon (Haklay and Weber, 2008). The geographical position of each particle was determined hourly, using bilinear interpolation of the northward and eastward components of the ocean velocity fields over each individual trajectory (e.g., Assis et al., 2015, 2018; Buonomo et al., 2016; Cunha et al., 2017). Particles were left to drift passively for up to 140 days (before restricting this by PD traits per group, see below) until they eventually ended up on land, a MPA, or got lost in the open ocean. Their individual trajectories were aggregated to develop pairwise probability matrices of connectivity between MPAs. Each pairwise probability of connectivity between MPAs was calculated by dividing the number of particles released from MPA i that reached MPA j, by the total number of particles released from MPA i. Individual yearly simulations were run for a 10-year period (2008–2017) to account for interannual variability, using non-aggregated data from HYCOM at the maximum temporal/spatial resolution available to preserve oceanographic processes occurring at the scales of days/tens of kilometers to realistically capture the movement of marine organisms (Putman and He, 2013).
Network Analyses
A graph-theoretical approach, accounting for stepping-stone processes, was used to infer and visualize connectivity patterns between MPAs (Buonomo et al., 2016). This is based on two elements, the nodes and the edges connecting the nodes (Rozenfeld et al., 2008). In the present study, MPAs defined the nodes and the pairwise connectivity matrix resulting from the 10-year simulation defined the strength of the edges between the nodes. The degree of network connectivity was inferred by determining the number of isolated MPAs (i.e., no edge connections), the number of distinct aggregations formed by closed edge connections (i.e., clusters), as well as the mean number of connections per MPA (i.e., degree centrality). Betweenness centrality was determined to infer the influence of each node on network connectivity. Nodes bridging clusters of MPAs have high betweenness centrality (Ospina-Alvarez et al., 2020), thus MPAs with higher betweenness values might function as stepping-stones between clusters and prevent fragmentation of the overall network. To facilitate interpretation, betweenness measures were normalized between 0 and 1, with 1 representing the highest betweenness value, proportionally to the relative importance as stepping-stones to maintain connectivity (Ospina-Alvarez et al., 2020). This normalization was performed by considering the maximum and minimum values of betweenness retrieved per cluster, in order to capture the less/more central MPAs, at the scales of each network aggregation.
Graph results were produced individually per PD period (i.e., from 1 to 140 days), and applied to ecological groups sharing similar dispersal ecology. To this end, a database of PD estimates available for marine species at global scales (Assis et al., 2021) was filtered to include species that occur in the study area (Supplementary Information 1) by consulting the online databases Algaebase (Guiry and Guiry, 2021), Aquamaps (Kaschner et al., 2019) and SeaLifeBase (Palomares and Pauly, 2021), which incorporate literature information and expert knowledge, as well as species range maps from the Ocean Biodiversity Information System (Ocean Biodiversity Information System, 2021) and the Global Biodiversity Information Facility (Global Biodiversity Information Facility, 2021). These groupings based on similar PD were (G1) Cnidaria, Tunicata and Porifera with a PD of 1.72 days (range: 0–8; n = 8), (G2) Macroalgae and Seagrass with a PD of 5.75 days (range: 1–15; n = 4), (G3) Bryozoa, Mollusca and Polychaeta with a PD of 17.00 days (range: 0–52; n = 9) and (G4) Pisces, Crustacea and Echinodermata with a PD of 35.79 days (range: 0–140; n = 79; Figure 1).
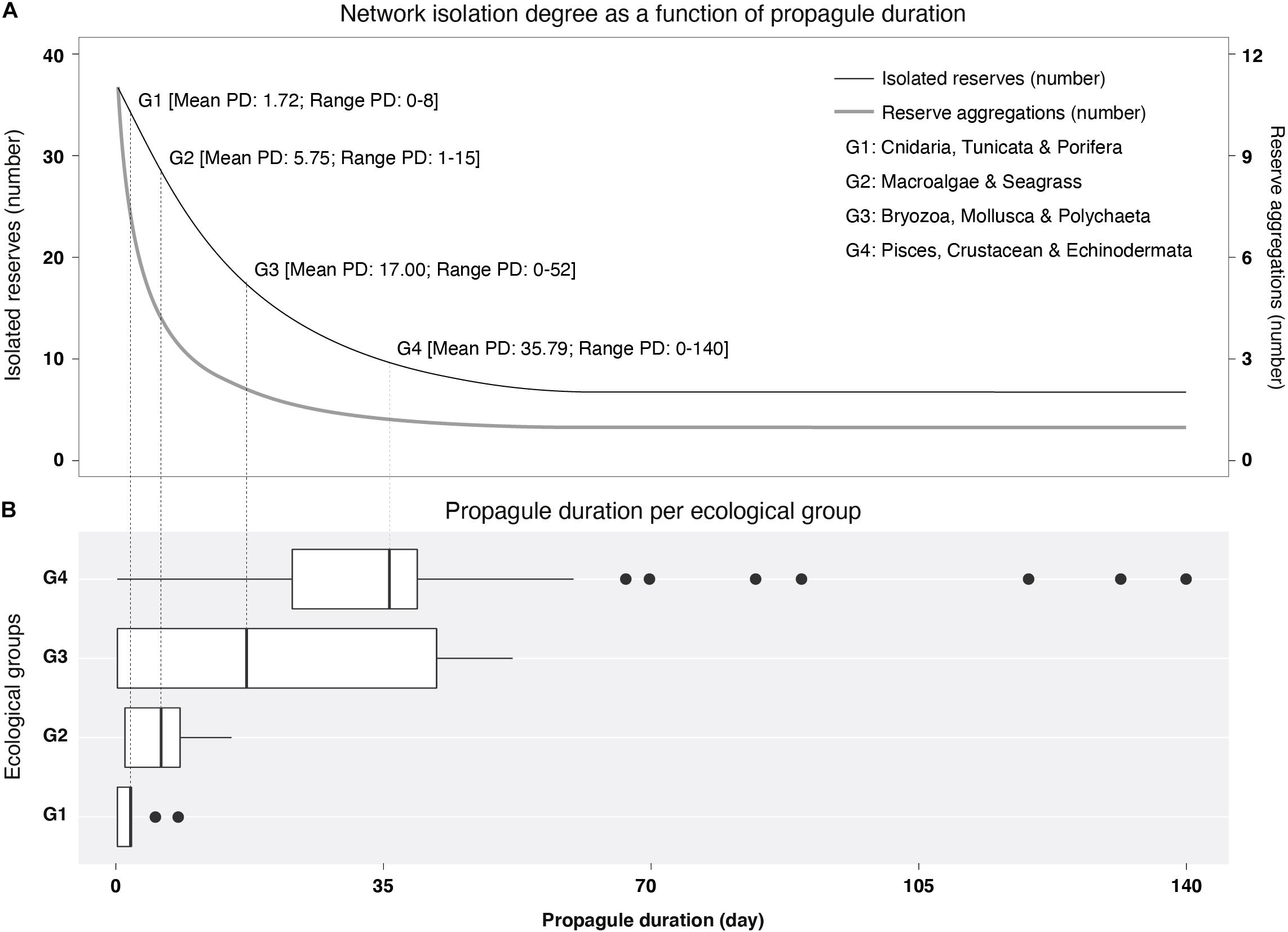
Figure 1. (A) Number of isolated Marine Protected Areas (MPAs) (thin line) and MPA aggregations (i.e., number of clusters; thick line) as a function of propagule duration (PD). (B) Distribution of PD per ecological group. Vertical lines in boxplots depict the mean PD for each ecological group.
Biophysical modeling was developed in R (R Development Core Team, 2021) using the packages: bigmemory, data.table, dismo, doparallel, geosphere, gstat, raster, and vegan (please refer to Supplementary Information 2 for the source code). Network analyses were performed in R using the package igraph. In particular, degree centrality was determined with the function degree, betweenness centrality was determined with the function betweenness and the clusters with the function components using the mode “weak”.
Results
Western African Reserve Network
Of the 88 MPAs included in the West African reserve network database produced by Failler et al. (2020c), 73 outside closed or inland embayments were included in the biophysical model (Supplementary Informations 3, 4). Senegal and Cape Verde had the highest number of MPAs (27 and 17, respectively; Table 1), Mauritania had the highest proportion of its Economic Exclusive Zone (EEZ) protected (3.67%; Table 1). Gambia and Western Sahara had the lower number of MPAs and proportion of EEZ protected (Table 1). In addition, MPAs in the study area are generally small: 66 (out of 73) are less than 400 km2, and 71 are less than 1,000 km2 (Supplementary Informations 3, 4).
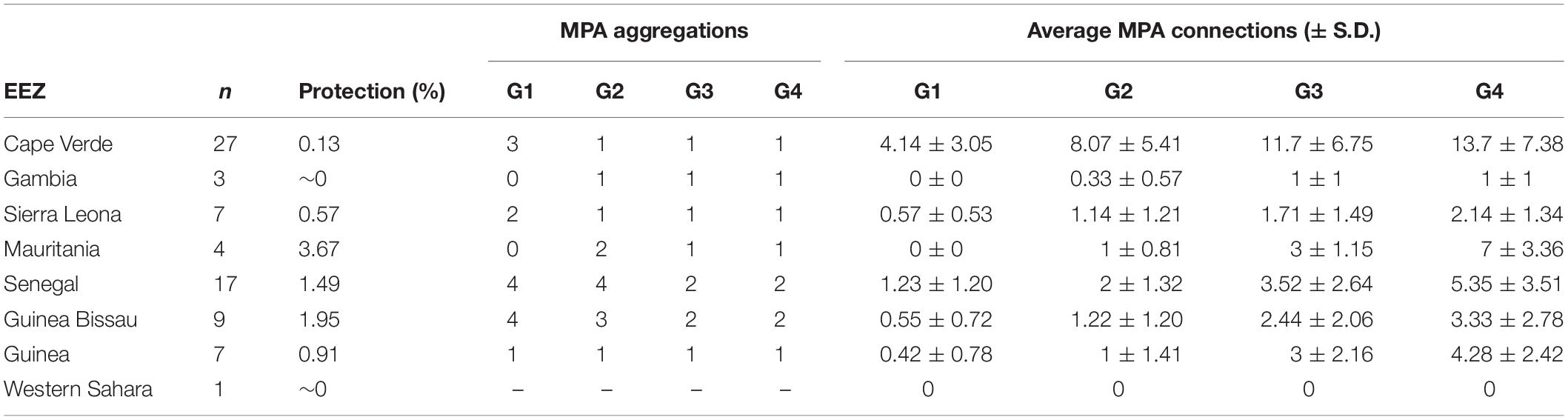
Table 1. Protection degree and potential connectivity, as the number of Marine Protected Area (MPA) aggregations (i.e., clusters) and the average number of MPA connections (i.e., degree centrality), determined per Exclusive Economic Zone (EEZ) and ecological groups (considering the mean propagule duration (PD) of species within each group; G1: Cnidaria, Tunicata & Porifera; G2: Macroalgae & Seagrass; G3: Bryozoa, Mollusca & Polychaeta; G4: Pisces, Crustacean & Echinodermata).
Network Connectivity
The biophysical modeling simulating dispersal events over the 10-year period released a total of 9,169,030 particles from 2,510 source sites, which resulted in 2,381,058 connectivity events (25.97% of all possible events). As anticipated, analyses showed a positive correlation between increasing PD and connectivity. Increasing PD from 1 to 140 days decreased the number of isolated MPAs (no edge connections from 37 to 8; Figure 1) and their distinct aggregations (clusters from 9 to 2; Figure 1), while increasing the mean number of connections per MPA (mean degree centrality from 1.14 to 9.51; Supplementary Information 5).
The contrasting dispersal potential (PD periods) of each ecological group was well linked to the potential connectivity of the West African MPA network. Ecological groups with shorter PD, namely G1 (Cnidaria, Tunicata, and Porifera) and G2 (Macroalgae and Seagrass), represent a more fragmented protection network (clusters: 9–10), with higher MPA isolation (no edge connections: 18–31) and less connections per MPA, on average (degree centrality: 1.53–2.82; Figure 2). The lower potential connectivity of such groups fails to link MPAs as a coherent network even within national borders. On the contrary, the longer mean PD of G3 (Bryozoa, Mollusca and Polychaeta) and G4 (Pisces, Crustacea and Echinodermata) allows population admixture between MPAs across international borders (clusters: 3–5; no edge connections: 10–11; degree centrality: 3.01– 5.41; Figure 2). In particular, the G3 taxa are linked across MPAs from Mauritania to northern Guinea Bissau, while G4 further links these with the Cape Verde MPAs, forming a broad, distinct aggregation. Also, G4 enabled the aggregation of the two southern clusters formed by the MPAs of southern Guinea-Bissau, Guinea, and Sierra Leone into one (Figure 2). Yet, even this group with higher dispersal potential retains a major gap in connectivity in central Guinea Bissau (Figure 2 and Table 1).
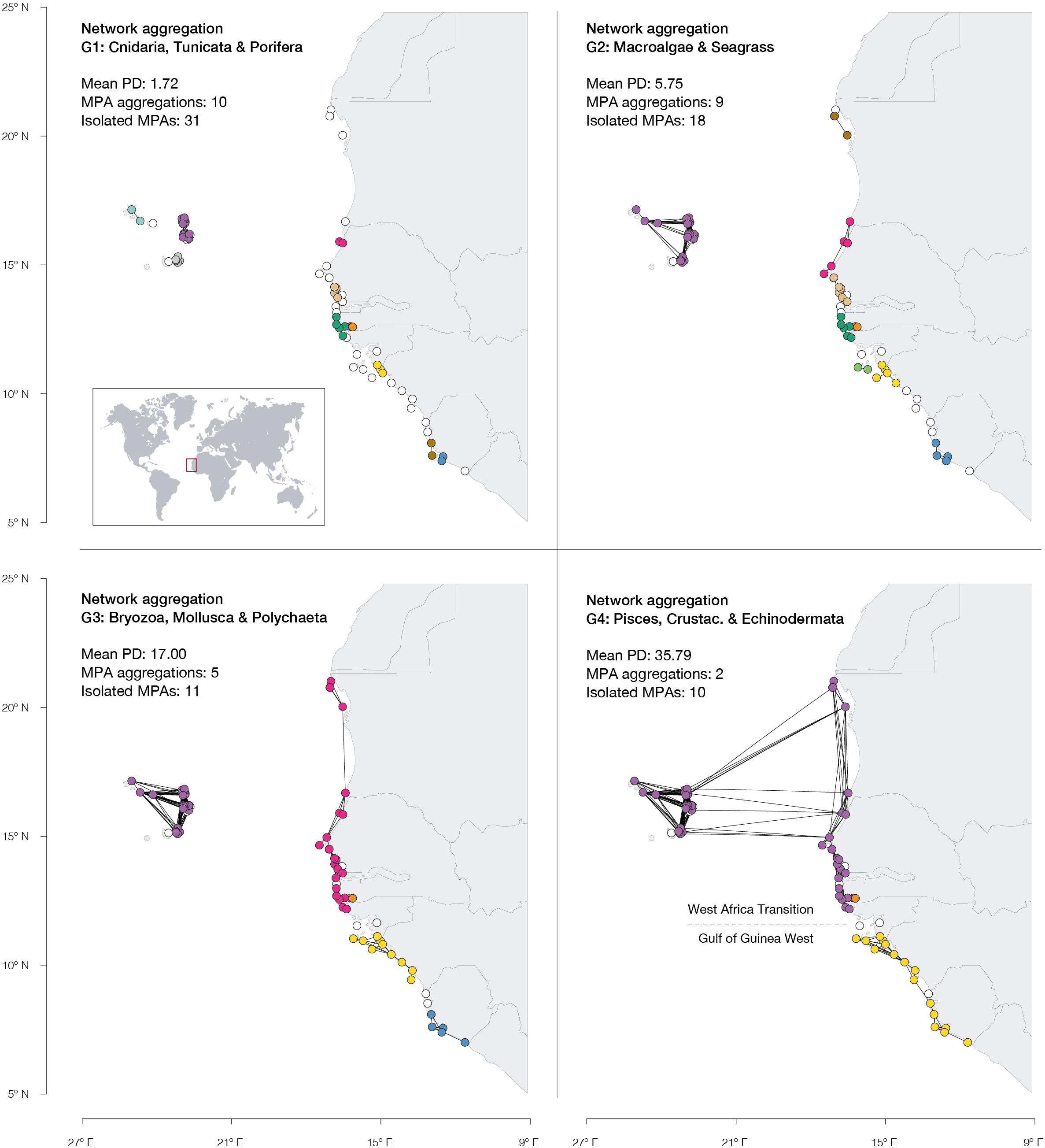
Figure 2. Potential connectivity of the West African MPA network for distinct ecological groups (defined based on similar mean PD). MPAs are depicted as circles, colored according to their cluster. Isolated MPAs are white and lines between reserves represent potential connectivity links. The number of isolated MPAs and MPA aggregations are shown per ecological group. Dashed line shows the biogeographic transition zone between the ecoregions “West Africa Transition” and “Gulf of Guinea West” (Spalding et al., 2007; Costello et al., 2017).
Marine Protected Area isolation was observed in some cases to occur in all marine groups independently of their distinct PD periods. Within ecological groups, PD periods are not always homogeneous, showing large dispersion of values, with some species with much longer PD than the mean of each ecological group (Figure 1). However, even in a best-case connectivity scenario of 140 days of PD, in which MPAs aggregated into one single coherent network, there were still 8 MPAs remaining isolated (Supplementary Information 5). This isolation could be explained by MPA size; our assessment of the relation between MPA size with their degree of isolation, revealed that the isolated MPAs were significantly smaller than connected ones (extent of connected MPAs was, on average, 210.62 ± 795.45 km2 and the extent of isolated MPAs was 3.96 ± 10.54 km2; Supplementary Information 5).
The MPAs with the highest betweenness centrality, thus contributing for migratory flow between more MPAs, were identified along the shorelines of Cape Verde archipelago, Senegal, Guinea and Sierra Leone for G1, G2, and G3. For G4, higher centrality was identified in Senegal, Guinea and Sierra Leone.
Discussion
The bio-physical modeling approach based on high-resolution data provided key insights about the potential connectivity in the west African MPA network for species dispersed by ocean currents, which comprise most marine biodiversity. The results revealed that connectivity differs sharply among distinct ecological groups, from highly connected to predominantly isolated. Remarkably, regardless of their dispersal duration, all ecological groups have in common a major connectivity gap in the Bijagós region of Guinea-Bissau, which coincided with a well-known biogeographic transition zone. This novel information has strong implications for biodiversity conservation and management (Magris et al., 2014; Assis et al., 2021), revealing the extreme importance of conserving the important and rare connectivity nodes persisting in the Bijagós islands, upon which most north-south links depend along this global region.
Using the range of planktonic dispersal durations (PD period) for all types of macroscopic marine species, provided a global overview of connectivity across major ecological groups. This approach revealed how ecological groups with distinct dispersal potential were affected by the MPA network spatial distribution and aggregation, as previously shown for the European context (Assis et al., 2021) and for particular ecological groups (e.g., Selkoe and Toonen, 2011). As observed elsewhere, discrete connections between adjacent MPAs, for taxa with poor dispersal capacity, can increase in number and expand in distance (from tens to hundreds of km) for increasing PD periods (Shanks et al., 2003; Krueck et al., 2017; Assis et al., 2021). The size of MPA can further play an important role in network connectivity (Assis et al., 2021).
The reduced connectivity inferred for ecosystem structuring species (i.e., corals, tunicates, sponges, macroalgae, and seagrasses) with short PD, resulted in multiple distinct MPA aggregations (i.e., clusters), higher probability of MPA isolation and reduced number of stepping-stone corridors. Conversely, species with longer PD (i.e., fish and crustaceans), potentially connect more MPAs across greater distances. Such a relationship is in line with previous studies (e.g., Treml et al., 2012) and corroborates the expectations of genetic diversity across ecological groups. Corals (Pilczynska et al., 2019), sponges (Duran et al., 2004), and marine forests of macroalgae (Johansson et al., 2015; Assis et al., 2018) and seagrasses (Alberto et al., 2008) recurrently exhibit well-defined genetic structure at short spatial scales, while in contrast, fish (Klein et al., 2016), crustaceans (Heras et al., 2019) and echinoderms (Maltagliati et al., 2010) tend to display more homogeneous genetic patterns, although not completely panmictic across all distributional ranges (Schunter et al., 2011). Taxa with planktonic dispersed stages considered in our study represent the large majority of macroscopic marine species. Even for mobile species, able to swim actively while performing migrations or ontogenetic shifts in habitat use (Abecasis et al., 2009; Almpanidou et al., 2019), PD alone can explain more than 50% of the variation in genetic dispersal estimates (Kinlan and Gaines, 2003). Taxa without planktonic dispersal stages might also benefit from some aid in transportation by oceanographic currents, as suggested for some taxa like green turtles (e.g., Jensen et al., 2020), but not others (e.g., Briscoe et al., 2016).
The low network connectivity predicted for ecosystem structuring species may undermine conservation efforts if retention is not sufficient to maintain population sizes. This information was unknown until now and may provide useful guidelines for future management of the West Africa network of MPA (Balbar and Metaxas, 2019). In particular, stepping-stone reserves for ecosystem structuring species are particularly relevant to ensure biodiversity persistence under future environmental changes and overexploitation, and increase the network resilience (Fung et al., 2017). For instance, massive mortality events (e.g., Garrabou et al., 2009) can be reversed through the replenishment of impacted populations from well-connected neighboring locations (Bonin et al., 2016). Particular MPAs of Cape Verde, Guinea, Senegal, and Sierra Leone with higher betweenness values (Figure 3) may play a key role toward connectivity in the face of future environmental changes, by acting as stepping-stones for the dispersal of propagules between different islands or regions/countries. Importantly, the few data describing population structure along the West African coastal waters and its network of MPAs already reports losses at the ecosystem level (seagrass, rocky bottom communities and coral reefs; Failler et al., 2020a; Chefaoui et al., 2021). However, betweenness centrality is strongly dependent on network configuration, and one MPA can be particularly important in promoting stepping-stone connectivity for a given ecological group, but not for others. For instance, the aforementioned region of Cape Verde contains a set of MPAs which are central for ecosystem structuring species (G1 and G2), but of lower importance for species with increased dispersal potential (G3 and G4). As connections increase, so does the size of clusters, and consequently the MPAs with higher betweenness centrality change to those strictly ensuring within-cluster connectivity between large water masses (e.g., Dakar in Senegal linking continual coastlines with Cape Verde archipelago).
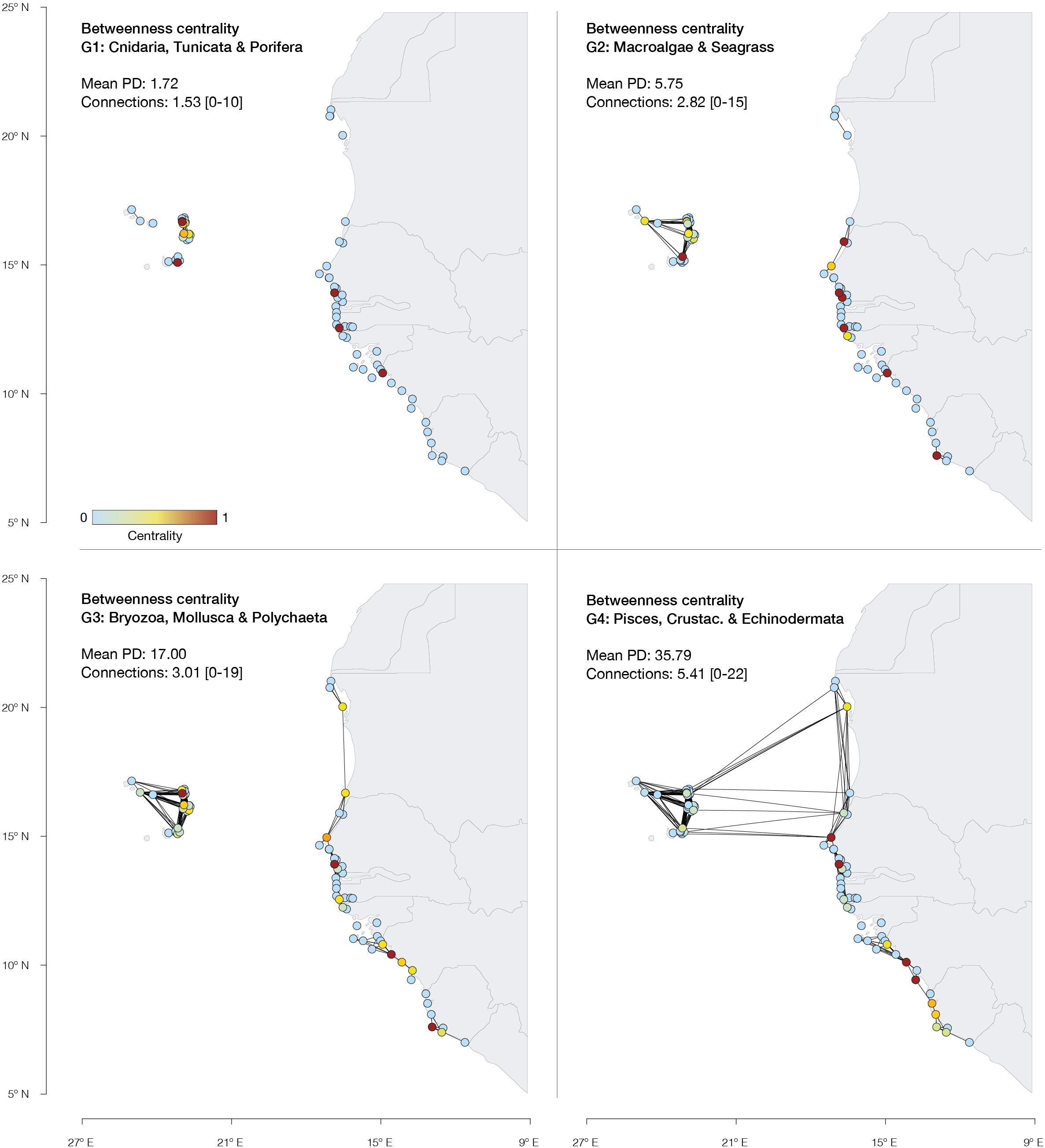
Figure 3. Betweenness centrality (the influence of each node over connectivity of every pair of nodes) of the West African MPA network for distinct ecological groups (defined based on similar mean PD). MPAs are depicted as circles, colored according to their betweenness centrality. Red circles depict central MPAs (above the 95th percentile of the distribution of betweenness centrality). Lines between reserves represent potential connectivity links. The mean connections per MPA are shown per ecological group.
The higher network connectivity predicted for the groups with higher dispersal potential (Bryozoa, Mollusca, Polychaeta, Pisces, Crustacea, and Echinodermata) may be encouraging for resource management, particularly for commercially targeted species (e.g., fish). However, the persistence of better connected populations relies on habitat traits, for feeding and reproduction, that are often dependent on ecosystem structuring species (Vassallo et al., 2013; Lefcheck et al., 2019) with poor network connectivity. Thus, habitat structuring species should be prioritized as management targets for marine connectivity both inside and outside the MPAs of the West African network, given their ecological role for other groups that despite being more coherently protected could still become limited in terms of suitable habitats. Not ensuring the future protection of essential habitats for commercial species might result in a stressor adding on to the already observed pattern of overexploitation, as recently reported for West African fish species (Gascuel et al., 2007; Atkinson et al., 2011; Durand et al., 2013). It must be highlighted that more MPAs do not resolve all main threats to connectivity of such essential structural taxa. Worldwide, ecosystems of sessile invertebrates and sea vegetation are frequently threatened by terrestrial disturbances caused by land management, particularly the consequences of removal of natural terrestrial vegetation cover that cause massive erosion, turbidity and sedimentation, destroying marine nursery and feeding habitats for fisheries, that cannot be prevented solely by MPAs.
A major connectivity gap was identified for all biodiversity in the central Guinea-Bissau area. This region lacks stepping-stone MPAs, and coincides with the transition zone (Supplementary Information 6) between the biogeographical eco-regions West Africa Transition and Gulf of Guinea West (Spalding et al., 2007; Costello et al., 2017), an oceanographic boundary zone. Transition zones have naturally low connectivity and increased genetic differentiation (Gourene et al., 1993; Pouyaud and Agnèse, 1995; Chikhi et al., 1998; Durand et al., 2005, 2013). In this scenario, the few connectivity events taking place across this region of Guinea-Bissau, which includes the very rich an unique Bijagós islands, can have strong implications on the effectiveness of the entire network of West Africa MPAs. This can be particularly important in mediating climate-induced range shifts, as conserving the natural (low) connectivity across transition zones provides protection of natural corridors for species to disperse tracking suitable habitat within scales of climate velocity. Thus, the implementation of more stepping-stone MPAs in the Bijagós islands is expected to enhance population resilience to climate change and to increase the overall effectiveness of the entire West African MPA network.
In contrast with Guinea-Bissau, the large connectivity gap identified between the Cape Verde Islands and continental Africa for taxa with limited dispersal capacities, is caused by the large distances lacking coastline habitat, and therefore additional stepping stone MPA planning is not possible. This natural isolation is responsible for the rich, high endemicity of Cape Verde biodiversity, but leaves the archipelago and its MPAs more vulnerable due to total dependence on local retention. Large-scale raft events that could replenish potentially affected populations in Cape Verde islands are unlikely, and thus a higher protection regime, to increase the islands’ MPA efficiency (Zupan et al., 2018), would be particularly important to minimize their vulnerability. Notably, isolation was still found in regions with high MPA coverage (e.g., Cape Verde and Senegal), possibly linked to the small size of MPAs, since MPA size is a major determinant of connectivity (Supplementary Information 5; Assis et al., 2021). Therefore, increasing the size of existing MPAs is an alternative and/or complementary measure to the implementation of new MPAs, which could help conservation of connectivity and restore ecological processes and population sizes (Claudet et al., 2008; McLeod et al., 2009; Cheng et al., 2019). So far, the implementation of MPAs has been done at a national level with the aim to preserve and manage biodiversity. A novel approach at a multinational scale is now decisive to achieve an effective network of MPAs following the goals of RAMPAO. Setting-up a transformative agenda is thus required to ensure the development of a network that is both based on national inspiration and regional connectivity. As RAMPAO aims to federate MPAs in the region through a common vision oriented toward maintenance of biodiversity and ecosystem services, RAMPAO can play a leading role ensuring that connectivity information is used to enhance the consistency of its network.
The bio-physical modeling approach considered a key ecological trait (i.e., duration of propagules; Assis et al., 2021) and oceanographic processes (e.g., fronts and eddies; Chassignet et al., 2007), as well as the actual shape, size and spatial structure of the MPAs to realistically estimate biodiversity connectivity (Assis et al., 2021). Additional drivers like the behavior (e.g., diel migration; Romero et al., 2012), competency and settlement success of larvae, as well as marine debris and translocations, could also play an important role in connectivity (Anadón et al., 2013; Faillettaz et al., 2018; Manel et al., 2019). However, their incorporation into modeling is still challenging, particularly because some of these drivers may vary between species, between individuals of the same species or between development stages (e.g., competency; Endo et al., 2019). In addition, connectivity of coastal areas at short distances might be strongly determined by regional patterns of tidal flows and near-shore circulation (Findlay and Allen, 2002; Cowen and Sponaugle, 2009) that might not be completely represented in HYCOM, nor in additional circulation models (Chassignet et al., 2006). Thus, only a comprehensive assessment of population genetic differentiation across distinct biodiversity groups or a validation of the HYCOM model for the study area (e.g., by passive drifters) could bring to light such limitations. Nevertheless, previous studies across the globe, comparing independent genetic and demographic data with our bio-physical model, systematically showed the central role of PD alone and oceanographic transport and barriers structuring population connectivity, and maintaining or diluting the genetic signatures of past changes (Buonomo et al., 2016; Lourenço et al., 2017; Pascual et al., 2017; Assis et al., 2018; Nicastro et al., 2019; Ntuli et al., 2020).
Our results show that connectivity in the West Africa network of MPAs differs sharply between major ecological groups, and provide unique insights for conservation and management actions, highlighting regions requiring additional protection (e.g., Bijagós region of Guinea-Bissau) to ensure functional connectivity corridors between major oceanographic regions (in line with terrestrial migration corridors; Ward et al., 2020). Initially dedicated to the protection of key threatened species such as birds in Mauritania and Senegal, manatees in Senegalese and Gambian estuaries, seals in the north of Mauritania, turtles in Cape Verde and Bijagos archipelagos, the MPAs of West Africa have progressively been implemented for the protection of other valuable biodiversity (Leeney and Poncelet, 2015; Laudisoit et al., 2017) and are continuing to work toward global improvement. As observed globally, the performance of the West African countries regarding the Aichi target 11 is overall poor (Failler et al., 2020a) yet, the new Global Biodiversity Framework pursues positive trends in biodiversity, while structured to focus on ecosystems and processes (Phang et al., 2020) and promoting involvement of stakeholders, at various levels. Management of the West African MPA network does not yet fully consider the potential biodiversity impacts coming from human activities and climate change (Failler et al., 2020b), and measures are predominantly aimed at preventing overfishing and terrestrial overexploitation (Failler et al., 2020a). However, our results show that MPA management in the West Africa should strongly focus on ecosystem structuring species (e.g., corals, sponges, macroalgae, and seagrasses), which are the essential nursery and feeding habitats of numerous commercial and essential subsistence species, besides threatened species, and might suffer the impacts of global climate change (Assis et al., 2017), as well as additional local stressors like marine invasions (Giakoumi and Pey, 2017), bottom trawling (Fragkopoulou et al., 2021) and water pollution (Abessa et al., 2018). These results provide a solid basis that will help coordinate efforts between the different countries/stakeholders toward the implementation of an efficient network of MPAs in West Africa.
Data Availability Statement
The original contributions presented in the study are included in the article/Supplementary Material, further inquiries can be directed to the corresponding author.
Author Contributions
JA, PF, EF, DA, GT-G, AR, ES, HD, and EAS conceived the ideas. JA, EF, and DA analyzed the data. JA, EF, DA, and EAS led the writing. All authors reviewed the manuscript.
Funding
This study was supported by the Foundation for Science and Technology (FCT) of Portugal through projects UID/Multi/04326/2020, PTDC/BIA-CBI/6515/2020, transitional norm - DL57/2016/CP1361/CT0035 and DL57/2016/CP1361/CT0036, fellowship SFRH/BD/144878/2019 and by a Pew Marine Fellowship. Additional support came from MAVA and PRCM/STM POOOA4/OA9 and EU H2020 grant agreement No 854248 (TROPIBIO).
Conflict of Interest
The authors declare that the research was conducted in the absence of any commercial or financial relationships that could be construed as a potential conflict of interest.
Publisher’s Note
All claims expressed in this article are solely those of the authors and do not necessarily represent those of their affiliated organizations, or those of the publisher, the editors and the reviewers. Any product that may be evaluated in this article, or claim that may be made by its manufacturer, is not guaranteed or endorsed by the publisher.
Supplementary Material
The Supplementary Material for this article can be found online at: https://www.frontiersin.org/articles/10.3389/fmars.2021.765053/full#supplementary-material
Supplementary Information 1 | Comprehensive list of species’ propagule duration (PD).
Supplementary Information 2 | Source code for the biophysical modeling available at: http://github.com/jorgeassis/biophysicalModelling.
Supplementary Information 3 | Comprehensive list of components of the West African Marine Protected Area (MPA) Network.
Supplementary Information 4 | Vector shapefile for geographic information systems with digitized polygons of the West African MPA Network available in Figshare: doi: 10.6084/m9.figshare.17059919.
Supplementary Information 5 | Additional results (figures and tables).
Supplementary Information 6 | Animation showing a simulation of potential dispersal running for the year 2010. Random release cells (in different colors) were chosen to better illustrate the path of individual particles.
References
Abecasis, D., Bentes, L., and Erzini, K. (2009). Home range, residency and movements of Diplodus sargus and Diplodus vulgaris in a coastal lagoon: connectivity between nursery and adult habitats. Estuar. Coast. Shelf Sci. 85, 525–529. doi: 10.1016/j.ecss.2009.09.001
Abessa, D. M. S., Albuquerque, H. C., Morais, L. G., Araújo, G. S., Fonseca, T. G., Cruz, A. C. F., et al. (2018). Pollution status of marine protected areas worldwide and the consequent toxic effects are unknown. Environ. Pollut. 243(Pt B), 1450–1459. doi: 10.1016/j.envpol.2018.09.129
Alberto, F., Massa, S., Manent, P., Diaz-Almela, E., Arnaud-Haond, S., Duarte, C. M., et al. (2008). Genetic differentiation and secondary contact zone in the seagrass Cymodocea nodosa across the Mediterranean-Atlantic transition region. J. Biogeogr. 35, 1279–1294. doi: 10.1111/j.1365-2699.2007.01876.x
Almpanidou, V., Markantonatou, V., and Mazaris, A. D. (2019). Thermal heterogeneity along the migration corridors of sea turtles: implications for climate change ecology. J. Exp. Mar. Bio. Ecol. 520:151223. doi: 10.1016/j.jembe.2019.151223
Anadón, J. D., Mancha-Cisneros, M. D. M., Best, B. D., and Gerber, L. R. (2013). Habitat-specific larval dispersal and marine connectivity: implications for spatial conservation planning. Ecosphere 4:82. doi: 10.1890/ES13-00119.1
Andrello, M., Guilhaumon, F., Albouy, C., Parravicini, V., Scholtens, J., Verley, P., et al. (2017). Global mismatch between fishing dependency and larval supply from marine reserves. Nat. Commun. 8:16039. doi: 10.1038/ncomms16039
Assis, J., Araújo, M. B., and Serrão, E. A. (2017). Projected climate changes threaten ancient refugia of kelp forests in the North Atlantic. Glob. Chang. Biol. 24, 1365–2486. doi: 10.1111/gcb.13818
Assis, J., Fragkopoulou, E., Serrão, E. A., Costa, B. H., Gandra, M., and Abecasis, D. (2021). Weak biodiversity connectivity in the European network of no-take marine protected areas. Sci. Total Environ. 773, 1–24. doi: 10.1016/j.scitotenv.2021.145664
Assis, J., Serrão, E. A., Coelho, N. C., Tempera, F., Valero, M., and Alberto, F. (2018). Past climate changes and strong oceanographic barriers structured low - latitude genetic relics for the golden kelp Laminaria ochroleuca. J. Biogeogr. 45, 2326–2336. doi: 10.1111/jbi.13425
Assis, J., Zupan, M., Nicastro, K. R., Zardi, G. I., McQuaid, C. D., and Serrão, E. A. (2015). Oceanographic conditions limit the spread of a marine invader along Southern African Shores. PLoS One 10:e0128124. doi: 10.1371/journal.pone.0128124
Atkinson, L. J., Leslie, R. W., Field, J. G., and Jarre, A. (2011). Changes in demersal fish assemblages on the west coast of South Africa, 1986–2009. Afr. J. Mar. Sci. 33, 157–170. doi: 10.2989/1814232X.2011.572378
Balbar, A. C., and Metaxas, A. (2019). The current application of ecological connectivity in the design of marine protected areas. Glob. Ecol. Conserv. 17:e00569. doi: 10.1016/j.gecco.2019.e00569
Baus, E., Darrock, D. J., and Bruford, M. W. (2005). Gene-flow patterns in Atlantic and Mediterranean populations of the Lusitanian sea star Asterina gibbosa. Mol. Ecol. 14, 3373–3382. doi: 10.1111/j.1365-294X.2005.02681.x
Bonin, M. C., Harrison, H. B., Williamson, D. H., Frisch, A. J., Saenz-Agudelo, P., Berumen, M. L., et al. (2016). The role of marine reserves in the replenishment of a locally impacted population of anemonefish on the Great Barrier Reef. Mol. Ecol. 25, 487–499. doi: 10.1111/mec.13484
Briscoe, D. K., Parker, D. M., Balazs, G. H., Kurita, M., Saito, T., Okamoto, H., et al. (2016). Active dispersal in loggerhead sea turtles (Caretta caretta) during the ‘lost years’. Proc. R. Soc. B Biol. Sci. 283:20160690. doi: 10.1098/rspb.2016.0690
Buonomo, R., Assis, J., Fernandes, F., Engelen, A. H., Airoldi, L., and Serrão, E. (2016). Habitat continuity and stepping-stone oceanografic explain population genetic connectivity of the brown alga Cystoseira amentacea. Mol. Ecol. 26, 766–780. doi: 10.5061/dryad.dk563
Chassignet, E. P., Hurlburt, H. E., Smedstad, O. M., Halliwell, G. R., Hogan, P. J., Wallcraft, A. J., et al. (2006). “Ocean prediction with the Hybrid Coordinate Ocean Model (HYCOM),” in Ocean Weather Forecasting: An Integrated View of Oceanography, eds E. P. Chassignet and J. Verron (Dordrecht: Springer), 413–426. doi: 10.1007/1-4020-4028-8_16
Chassignet, E. P., Hurlburt, H. E., Smedstad, O. M., Halliwell, G. R., Hogan, P. J., Wallcraft, A. J., et al. (2007). The HYCOM (HYbrid Coordinate Ocean Model) data assimilative system. J. Mar. Syst. 65, 60–83. doi: 10.1016/j.jmarsys.2005.09.016
Chefaoui, R. M., Duarte, C. M., Tavares, A. I., Frade, D. G., Cheikh, M. A. S., Ba, M. A., et al. (2021). Predicted regime shift in the seagrass ecosystem of the Gulf of Arguin driven by climate change. Glob. Ecol. Conserv. 31:e01850. doi: 10.1016/j.gecco.2021.e01890
Cheng, B. S., Altieri, A. H., Torchin, M. E., and Ruiz, G. M. (2019). Can marine reserves restore lost ecosystem functioning? A global synthesis. Ecology 100:e02617. doi: 10.1002/ecy.2617
Chikhi, L., Bonhomme, F., and Agnèse, J. F. (1998). Low genetic variability in a widely distributed and abundant clupeid species, Sardinella aurita. New empirical results and interpretations. J. Fish Biol. 52, 861–878. doi: 10.1111/j.1095-8649.1998.tb00588.x
Claudet, J., Osenberg, C. W., Benedetti-Cecchi, L., Domenici, P., García-Charton, J. A., Pérez-Ruzafa, Á., et al. (2008). Marine reserves: size and age do matter. Ecol. Lett. 11, 481–489. doi: 10.1111/j.1461-0248.2008.01166.x
Convention on Biological Diversity (2021). First Draft of the Post-2020 Global Biodiversity Framework. Nairobi: United Nations Environmental Programme.
Costello, M. J., Tsai, P., Wong, P. S., Cheung, A. K. L., Basher, Z., and Chaudhary, C. (2017). Marine biogeographic realms and species endemicity. Nat. Commun. 8:1057. doi: 10.1038/s41467-017-01121-2
Cowen, R. K., Paris, C. B., and Srinivasan, A. (2006). Scaling of Connectivity in Marine Populations. Science 311, 522–527. doi: 10.1126/science.1122039
Cowen, R. K., and Sponaugle, S. (2009). Larval dispersal and marine population connectivity. Annu. Rev. Mar. Sci. 1, 443–466. doi: 10.1146/annurev.marine.010908.163757
Cunha, R. L., Assis, J., Madeira, C., Seabra, R., Lima, F. P., Lopes, E. P., et al. (2017). Drivers of Cape Verde archipelagic endemism in keyhole limpets. Sci. Rep. 7:41817. doi: 10.1038/srep41817
D’Aloia, C. C., Daigle, R. M., Côté, I. M., Curtis, J. M. R., Guichard, F., and Fortin, M. J. (2017). A multiple-species framework for integrating movement processes across life stages into the design of marine protected areas. Biol. Conserv. 216, 93–100. doi: 10.1016/j.biocon.2017.10.012
Duran, S., Pascual, M., Estoup, A., and Turon, X. (2004). Strong population structure in the marine sponge Crambe crambe (Poecilosclerida) as revealed by microsatellite markers. Mol. Ecol. 13, 511–522. doi: 10.1046/j.1365-294X.2004.02080.x
Durand, J. D., Guinand, B., Dodson, J. J., and Lecomte, F. (2013). Pelagic Life and Depth: coastal Physical Features in West Africa Shape the Genetic Structure of the Bonga Shad, Ethmalosa fimbriata. PLoS One 8:e77483. doi: 10.1371/journal.pone.0077483
Durand, J. D., Tine, M., Panfili, J., Thiaw, O. T., and Laë, R. (2005). Impact of glaciations and geographic distance on the genetic structure of a tropical estuarine fish, Ethmalosa fimbriata (Clupeidae, S. Bowdich, 1825). Mol. Phylogenet. Evol. 36, 277–287. doi: 10.1016/j.ympev.2005.01.019
Endo, C. A. K., Gherardi, D. F. M., Pezzi, L. P., and Lima, L. N. (2019). Low connectivity compromises the conservation of reef fishes by marine protected areas in the tropical South Atlantic. Sci. Rep. 9:8634. doi: 10.1038/s41598-019-45042-0
Failler, P., Touron-Gardic, G., Traoré, M. S., and Phang, S. C. (2020c). Evaluating the official achievement of Aichi Target 11 for West African countries: a twofold challenge of accuracy and catching-up. Sci. Total Environ. 698:134284. doi: 10.1016/j.scitotenv.2019.134284
Failler, P., Touron-Gardic, G., Drakeford, B., Sadio, O., and Traoré, M.-S. (2020a). Perception of threats and related management measures: the case of 32 marine protected areas in West Africa. Mar. Policy 117:103936. doi: 10.1016/j.marpol.2020.103936
Failler, P., Touron-Gardic, G., Sadio, O., and Traoré, M.-S. (2020b). Perception of natural habitat changes of West African marine protected areas. Ocean Coast. Manag. 187:105120. doi: 10.1016/j.ocecoaman.2020.105120
Faillettaz, R., Paris, C. B., and Irisson, J. O. (2018). Larval fish swimming behavior alters dispersal patterns from marine protected areas in the North-Western Mediterranean Sea. Front. Mar. Sci. 5:97. doi: 10.3389/fmars.2018.00097
Findlay, A. M., and Allen, L. G. (2002). Temporal patterns of settlement in the temperate reef fish Paralabrax clathratus. Mar. Ecol. Prog. Ser. 238, 237–248.
Fossette, S., Putman, N. F., Lohmann, K. J., Marsh, R., and Hays, G. C. (2012). A biologist’s guide to assessing ocean currents: a review. Mar. Ecol. Prog. Ser. 457, 285–301. doi: 10.3354/meps09581
Fragkopoulou, E., Serrão, E. A., Horta, P. A., Koerich, G., and Assis, J. (2021). Bottom Trawling Threatens Future Climate Refugia of Rhodoliths Globally. Front. Mar. Sci. 7:594537. doi: 10.3389/fmars.2020.594537
Fung, E., Imbach, P., Corrales, L., Vilchez, S., Zamora, N., Argotty, F., et al. (2017). Mapping conservation priorities and connectivity pathways under climate change for tropical ecosystems. Clim. Change 141, 77–92. doi: 10.1007/s10584-016-1789-8
Garrabou, J., Coma, R., Bensoussan, N., Bally, M., Chevaldonné, P., Cigliano, M., et al. (2009). Mass mortality in Northwestern Mediterranean rocky benthic communities: effects of the 2003 heat wave. Glob. Chang. Biol. 15, 1090–1103. doi: 10.1111/j.1365-2486.2008.01823.x
Gascuel, D., Labrosse, P., Meissa, B., Taleb Sidi, M. O., and Guénette, S. (2007). Decline of demersal resources in North-West Africa: an analysis of Mauritanian trawl-survey data over the past 25 years. African J. Mar. Sci. 29, 331–345. doi: 10.2989/AJMS.2007.29.3.3.333
Giakoumi, S., and Pey, A. (2017). Assessing the effects of marine protected areas on biological invasions: a global review. Front. Mar. Sci. 4:49. doi: 10.3389/FMARS.2017.00049
Gillanders, B. M. (2005). Using elemental chemistry of fish otoliths to determine connectiv-ity between estuarine and coastal habitats. Estuar. Coast. Shelf Sci. 64, 47–57. doi: 10.1016/j.ecss.2005.02.005
Global Biodiversity Information Facility (2021). Available online at: https://www.gbif.org (accessed October, 2021).
Gourene, A., Pouyaud, L., and Agnese, J. F. (1993). Importance de certaines caractéristiques biologiques dans la structuration génétique des espèces de poissons: le cas de Ethmalosa fimbriata et Sarotherodon melanotheron. J. Ivoir. D’Océanol. Limnol. 2, 55–69.
Guiry, M. D., and Guiry, G. M. (2021). AlgaeBase. Available online at: https://www.algaebase.org (accessed October, 2021).
Haklay, M., and Weber, P. (2008). OpenStreet map: user-generated street maps. IEEE Pervasive Comput. 1, 12–18. doi: 10.1109/MPRV.2008.80
Hellberg, M. E., Burton, R. S., Neigel, J. E., and Palumbi, S. R. (2002). Genetic assessment of connectivity among marine populations. Bull. Mar. Sci. 70, 273–290.
Heras, S., Planella, L., García-Marín, J. L., Vera, M., and Roldán, M. I. (2019). Genetic structure and population connectivity of the blue and red shrimp Aristeus antennatus. Sci. Rep. 9:13531. doi: 10.1038/s41598-019-49958-5
Jensen, M. P., Dalleau, M., Gaspar, P., Lalire, M., Jean, C., Ciccione, S., et al. (2020). Seascape Genetics and the Spatial Ecology of Juvenile Green Turtles. Genes 11:278. doi: 10.3390/genes11030278
Johansson, M. L., Alberto, F., Reed, D. C., Raimondi, P. T., Coelho, N. C., Young, M. A., et al. (2015). Seascape drivers of Macrocystis pyrifera population genetic structure in the northeast Pacific. Mol. Ecol. 24, 4866–4885. doi: 10.1111/mec.13371
Kaschner, K., Kesner-Reyes, K., Garilao, C., Segschneider, J., Rius-Barile, J., Rees, T., et al. (2019). AquaMaps: Predicted Range Maps for Aquatic Species. Available online at: https://www.aquamaps.org. (accessed October, 2021).
Kendall, M. S., Poti, M., Wynne, T. T., Kinlan, B. P., and Bauer, L. B. (2013). Consequences of the life history traits of pelagic larvae on interisland connectivity during a changing climate. Mar. Ecol. Prog. Ser. 489, 43–59. doi: 10.3354/meps10432
Kinlan, B. P., and Gaines, S. D. (2003). Propagule dispersal in marine and terrestrial environments: a community perspective. Ecology 84, 2007–2020. doi: 10.1890/01-0622
Klein, M., Teixeira, S., Assis, J., Serrão, E. A., Gonçalves, E. J., and Borges, R. (2016). High interannual variability in connectivity and genetic pool of a temperate clingfish matches oceanographic transport predictions. PLoS One 11:e0165881. doi: 10.1371/journal.pone.0165881
Krueck, N. C., Ahmadia, G. N., Green, A., Jones, G. P., Possingham, H. P., Riginos, C., et al. (2017). Incorporating larval dispersal into MPA design for both conservation and fisheries. Ecol. Appl. 27, 925–941. doi: 10.1002/eap.1495
Laudisoit, A., Collet, M., Muyaya, B., Vanhoutte, N., and Verheyen, E. (2017). West African Manatee Trichechus senegalensis (LINK, 1795) in the Estuary of the Congo River (Democratic Republic of the Congo): review and Update. J. Biodivers. Endanger. Species 05, 1–11. doi: 10.4172/2332-2543.1000181
Leeney, R. H., and Poncelet, P. (2015). Using fishers’ ecological knowledge to assess the status and cultural importance of sawfish in Guinea-Bissau. Aquat. Conserv. Mar. Freshw. Ecosyst. 25, 411–430. doi: 10.1002/aqc.2419
Lefcheck, J. S., Hughes, B. B., Johnson, A. J., Pfirrmann, B. W., Rasher, D. B., Smyth, A. R., et al. (2019). Are coastal habitats important nurseries? A meta-analysis. Conserv. Lett. 12, 1–12. doi: 10.1111/conl.12645
Lessios, H. A., Kessing, B. D., and Robertson, D. R. (1998). Massive gene flow across the world’s most potent marine biogeographic barrier. Proc. R. Soc. London. Ser. B Biol. Sci. 265, 583–588. doi: 10.1098/rspb.1998.0334
Lett, C., Verley, P., Mullon, C., Parada, C., Brochier, T., Penven, P., et al. (2008). A Lagrangian tool for modelling ichthyoplankton dynamics. Environ. Model. Softw. 23, 1210–1214. doi: 10.1016/j.envsoft.2008.02.005
Lindegren, M., Holt, B. G., MacKenzie, B. R., and Rahbek, C. (2018). A global mismatch in the protection of multiple marine biodiversity components and ecosystem services. Sci. Rep. 8:4099. doi: 10.1038/s41598-018-22419-1
Lourenço, C. R., Nicastro, K. R., McQuaid, C. D., Chefaoui, R. M., Assis, J., Taleb, M. Z., et al. (2017). Evidence for rangewide panmixia despite multiple barriers to dispersal in a marine mussel. Sci. Rep. 7:10279. doi: 10.1038/s41598-017-10753-9
Magris, R. A., Pressey, R. L., Weeks, R., and Ban, N. C. (2014). Integrating connectivity and climate change into marine conservation planning. Biol. Conserv. 170, 207–221. doi: 10.1016/j.biocon.2013.12.032
Maltagliati, F., Di Giuseppe, G., Barbieri, M., Castelli, A., and Dini, F. (2010). Phylogeography and genetic structure of the edible sea urchin Paracentrotus lividus (Echinodermata: Echinoidea) inferred from the mitochondrial cytochrome b gene. Biol. J. Linn. Soc. 100, 910–923. doi: 10.1111/j.1095-8312.2010.01482.x
Manel, S., Loiseau, N., Andrello, M., Fietz, K., Goñi, R., Forcada, A., et al. (2019). Long-distance benefits of marine reserves: Myth or Reality? Trends Ecol. Evol. 34, 342–354. doi: 10.1016/j.tree.2019.01.002
McLeod, E., Salm, R., Green, A., and Almany, J. (2009). Designing marine protected area networks to address the impacts of climate change. Front. Ecol. Environ. 7, 362–370. doi: 10.1890/070211
Nicastro, K. R., Assis, J., Serr, E. A., Pearson, G. A., Valero, M., Jacinto, R., et al. (2019). Congruence between fine-scale genetic breaks and dispersal potential in an estuarine seaweed across multiple transition zones. ICES J. Mar. Sci. 77, 371–378. doi: 10.1093/icesjms/fsz179
Ntuli, N. N., Nicastro, K. R., Zardi, G. I., Assis, J., McQuaid, C. D., and Teske, P. R. (2020). Rejection of the genetic implications of the “Abundant Centre Hypothesis” in marine mussels. Sci. Rep. 10:604. doi: 10.1038/s41598-020-57474-0
Ocean Biodiversity Information System (2021). Available online at: https://obis.org (accessed October, 2021).
Ospina-Alvarez, A., de Juan, S., Alós, J., Basterretxea, G., Alonso-Fernández, A., Follana-Berná, G., et al. (2020). MPA network design based on graph theory and emergent properties of larval dispersal. Mar. Ecol. Prog. Ser. 650, 309–326. doi: 10.3354/meps13399
Palomares, M. L. D., and Pauly, D. (2021). SeaLifeBase. Available online at: https://www.sealifebase.ca (accessed October, 2021).
Pascual, M., Rives, B., Schunter, C., and MaCpherson, E. (2017). Impact of life history traits on gene flow: a multispecies systematic review across oceanographic barriers in the Mediterranean Sea. PLoS One 12:e0176419. doi: 10.1371/journal.pone.0176419
Phang, S. C., Failler, P., and Bridgewater, P. (2020). Addressing the implementation challenge of the global biodiversity framework. Biodivers. Conserv. 29, 3061–3066. doi: 10.1007/s10531-020-02009-2
Pilczynska, J., Cocito, S., Boavida, J., Serrão, E. A., Assis, J., Fragkopoulou, E., et al. (2019). Genetic diversity increases with depth in red gorgonian populations of the Mediterranean Sea and the Atlantic Ocean. PeerJ 7:e6794. doi: 10.7717/peerj.6794
Pouyaud, L., and Agnèse, J. F. (1995). Différenciation Génétique des Populations de Sarotherodon Melanotheron, Rüppell, 1853. Available online at: http://www.documentation.ird.fr/hor/fdi:010005197 (accessed August 2021).
Pujolar, J. M., Schiavina, M., Di Franco, A., Melià, P., Guidetti, P., Gatto, M., et al. (2013). Understanding the effectiveness of marine protected areas using genetic connectivity patterns and Lagrangian simulations. Divers. Distrib. 19, 1531–1542. doi: 10.1111/ddi.12114
Putman, N. F., and He, R. (2013). Tracking the long-distance dispersal of marine organisms: sensitivity to ocean model resolution. J. R. Soc. Interface. 10. doi: 10.1098/rsif.2012.0979
R Development Core Team (2021). R: A Language and Environment for Statistical Computing. Vienna: R Foundation for Statistical Computing. Available online at: https://www.R-project.org
Romero, M. R., Walker, K. M., Cortez, C. J., Sanchez, Y., Nelson, K. J., Ortega, D. C., et al. (2012). Larval Diel Vertical Migration of the Marine Gastropod Kelletia kelletii (Forbes, 1850). J. Mar. Sci. 2012:386575. doi: 10.1155/2012/386575
Rozenfeld, A. F., Arnaud-Haond, S., Hernández-García, E., Eguíluz, V. M., Serrão, E. A., and Duarte, C. M. (2008). Network analysis identifies weak and strong links in a metapopulation system. Proc. Natl. Acad. Sci. U.S.A. 105, 18824–18829. doi: 10.1073/pnas.0805571105
Schunter, C., Carreras-Carbonell, J., MacPherson, E., Tintor, É. J., Vidal-Vijande, E., Pascual, A., et al. (2011). Matching genetics with oceanography: directional gene flow in a Mediterranean fish species. Mol. Ecol. 20, 5167–5181. doi: 10.1111/j.1365-294X.2011.05355.x
Selkoe, K. A., and Toonen, R. J. (2011). Marine connectivity: a new look at pelagic larval duration and genetic metrics of dispersal. Mar. Ecol. Prog. Ser. 436, 291–305. doi: 10.3354/meps09238
Shanks, A. L., Grantham, B. A., and Carr, M. H. (2003). Propagule dispersal distance and the size and spacing of marine reserves. Ecol. Appl. 13, 159–169.
Spalding, M. D., Fox, H. E., Allen, G. R., Davidson, N., Finlayson, M. A. X., Halpern, B. S., et al. (2007). Marine ecoregions of the world: a bioregionalization of coastal and shelf areas. Bioscience 57, 573–583. doi: 10.1641/B570707
Treml, E. A., Roberts, J. J., Chao, Y., Halpin, P. N., Possingham, H. P., and Riginos, C. (2012). Reproductive output and duration of the pelagic larval stage determine seascape-wide connectivity of marine populations. Integr. Comp. Biol. 52, 525–537. doi: 10.1093/icb/ics101
Vassallo, P., Paoli, C., Rovere, A., Montefalcone, M., Morri, C., and Bianchi, C. N. (2013). The value of the seagrass Posidonia oceanica: a natural capital assessment. Mar. Pollut. Bull. 75, 157–167. doi: 10.1016/j.marpolbul.2013.07.044
Ward, M., Saura, S., Williams, B., Ramírez-Delgado, J. P., Arafeh-Dalmau, N., Allan, J. R., et al. (2020). Just ten percent of the global terrestrial protected area network is structurally connected via intact land. Nat. Commun. 11:4563. doi: 10.1038/s41467-020-18457-x
Waters, J. M., and Roy, M. S. (2004). Out of Africa: the slow train to Australasia. Syst. Biol. 53, 18–24. doi: 10.1080/10635150490264671
Weigel, J., Féral, F., and Cazalet, B. (2007). Les Aires Marines Protégées d’Afrique de l’Ouest: Gouvernance et Politiques Publiques. Available online at: https://core.ac.uk/download/pdf/39839902.pdf (accessed August 2021).
Keywords: MPA networks, stepping-stone connectivity, biophysical modeling, biodiversity conservation, resource management
Citation: Assis J, Failler P, Fragkopoulou E, Abecasis D, Touron-Gardic G, Regalla A, Sidina E, Dinis H and Serrao EA (2021) Potential Biodiversity Connectivity in the Network of Marine Protected Areas in Western Africa. Front. Mar. Sci. 8:765053. doi: 10.3389/fmars.2021.765053
Received: 26 August 2021; Accepted: 01 November 2021;
Published: 30 November 2021.
Edited by:
Rowan Trebilco, Centre for Marine Socioecology, AustraliaReviewed by:
Scott Condie, Oceans and Atmosphere (CSIRO), AustraliaMarco Andrello, Istituto per lo Studio degli Impatti Antropici e Sostenibilità in Ambiente Marino (IAS), Consiglio Nazionale delle Ricerche (CNR), Italy
Copyright © 2021 Assis, Failler, Fragkopoulou, Abecasis, Touron-Gardic, Regalla, Sidina, Dinis and Serrao. This is an open-access article distributed under the terms of the Creative Commons Attribution License (CC BY). The use, distribution or reproduction in other forums is permitted, provided the original author(s) and the copyright owner(s) are credited and that the original publication in this journal is cited, in accordance with accepted academic practice. No use, distribution or reproduction is permitted which does not comply with these terms.
*Correspondence: Jorge Assis, am9yZ2VtZmFAZ21haWwuY29t; Ester A. Serrao, ZXNlcnJhb0B1YWxnLnB0