- 1 School of Marine Science, School of Environmental Science and Engineering, Southern Marine Science and Engineering Guangdong Laboratory (Zhuhai), Guangdong Provincial Key Laboratory of Marine Resources and Coastal Engineering, Sun Yat-sen University, Guangzhou, China
- 2Pearl River Estuary Marine Ecosystem Research Station, Ministry of Education, Zhuhai, China
- 3Guangdong Provincial Key Laboratory of Environmental Pollution Control and Remediation Technology, Sun Yat-sen University, Guangzhou, China
- 4Guangdong Marine Development Planning Research Center, Guangzhou, China
- 5Simon F.S. Li Marine Science Laboratory, School of Life Sciences, and Earth System Science Programme, The Chinese University of Hong Kong, Shatin, Hong Kong SAR, China
Fine root dynamics have the potential to contribute to ecosystem biogeochemical cycling, especially for carbon. This is particularly true in mangroves which are the most productive and carbon-rich ecosystems of the world. However, few studies comprehensively evaluated the contribution of mangrove fine root dynamics to soil organic carbon accumulation. In southern China, while the introduced fast-growing Sonneratia apetala and native shrubby Kandelia obovata have been widely used in mangrove reforestation/afforestation programs since the mid-1980s, their implications and ecosystem services are still unclear. Here we show distinct differences in fine root dynamic among 12-year-old S. apetala, K. obovata monocultures, and their mixed stand using root coring, ingrowth core, and intact-core methods. Soil organic carbon storage was examined by soil coring method. One-year observation showed significant differences among the three mangrove plantations in fine root biomass, necromass, turnover rate, and decomposition decay rate constant. Soil organic carbon stock was 15.8 ± 0.8, 7.8 ± 0.5, and 11.9 ± 1.6 Mg C ha–1 for K. obovata, S. apetala monocultures and their mixed stand, respectively. Live fine root biomass, fine root necromass, annual fine root production and fine root mass decay rate constant are significantly correlated to soil organic carbon content across plantations. We suggest that mangrove fine root dynamics were mainly affected by soil nutrient conditions and species composition. Mixed stands may not have higher soil organic carbon storage than monocultures. The functional trait of different mangrove species is responsible to determine the carbon storage function of mixed stands. Fine roots play an important role in carbon storage, and fine root dynamics have a significant effect on carbon sequestration in mangrove ecosystems. The shrubby native K. obovata had a higher potential for belowground carbon sequestration and storage than the tall introduced S. apetala.
Introduction
Fine roots (<2 mm in diameter) are the most physiologically active component of the belowground plant biomass involved in resource acquisition, nutrient exchange and organic matter decomposition (Iversen et al., 2017). Fine roots account for 10–30% of total forest tree biomass and 30–50% of total net primary production in global terrestrial ecosystem (Castañeda-Moya et al., 2011; McCormack et al., 2015b). Consequently, fine root turnover represents a major pathway for carbon and nutrient fluxes from plants to soil (Dornbush et al., 2002). While inhabiting a relatively steady environment compared to their aboveground counterparts, fine roots dynamics are still influenced by internal and external moderators, e.g., the intensity of intraspecific competition (Wang D. et al., 2019), seasonal variation of temperature and precipitation (Ibrahim et al., 2020; Sun et al., 2020), nutrient availability (Wang W. J. et al., 2019), or species composition in the community (Caplan et al., 2019). These complex feedbacks pose significant challenges to understanding fine root dynamics and their implications for key ecosystem processes.
Mangrove ecosystems are among the most productive and ecologically important ecosystems of the world (Li et al., 2019). Mangroves are vital blue carbon resource, with their soil carbon comprising more than 50% of the total C stock within the system (Adame et al., 2017). Mangrove plants are capable of allocating higher proportions of their total biomass to belowground roots in response to nutrient limitation and anoxic condition (Castañeda-Moya et al., 2011). Recent studies suggested that mangrove fine roots have a higher contribution to soil C than that of litterfall, and the positive effect on soil C accretion is attributable mainly to fine root production (Poungparn et al., 2016; Liu et al., 2017; Xiong et al., 2017). Yet, few studies comprehensively evaluated the contribution of mangrove fine root dynamics to soil organic carbon accumulation.
In southern China, the introduced Sonneratia apetala Buch.-Ham. and native Kandelia obovata Sheue, H.Y. Liu & J. Yong have been widely used in many mangrove afforestation programs for almost three decades. S. apetala is a tall and fast-growing species, whereas K. obovata is a shrubby species that commonly forms forests with higher tree densities (He et al., 2018). More attention on the ecosystem services derivable from such afforestation programs, e.g., carbon sequestration, has been supposed for an improved approach to global mangrove restoration (Lee et al., 2019). S. apetala forests have been reported to demonstrate much higher soil carbon accumulation rates as well as higher belowground root biomass than those of the native species (Ren et al., 2009, 2010). However, studies of biomass and carbon allocation in tropical and subtropical mangrove ecosystems suggest that shrubby forests allocate relatively more biomass or carbon to roots than do tall mangroves under unfavorable environmental conditions (Lovelock, 2008; Castañeda-Moya et al., 2011, 2013). In addition, Chen et al. (2012) reported that mixed mangrove plantations had higher soil carbon accumulation than the monocultures. Therefore, how soil carbon storage may differ based on contrasting morphological and functional traits of these two species, as well as the community setting, e.g., monocultures versus mixed plantation, would have significant implications for species selection and habitat management in carbon-based mangrove afforestation programs. In this study, we investigated the fine root dynamics of S. apetala and K. obovata monocultures as well as mixed stands of both species. The linkage between their fine root dynamics and soil organic carbon sequestration was also explored to provide insights into the mechanism of fine root contribution to soil organic carbon accumulation. We hypothesized that fine root dynamics is dependent on plant trait and therefore affects soil C accumulation. To test these hypotheses, the present study examined (1) live fine root biomass and fine root necromass distribution, fine root productivity, turnover rate, and decomposition in S. apetala and K. obovata monocultures, and their mixed stands; (2) soil organic carbon stock profiles among these three mangrove plantations and unvegetated tidal flats; and (3) the effects of fine root dynamics on soil organic carbon accumulation.
Materials and Methods
Study Sites
The study site was located in Hanjiang River Estuary of Chenghai District, Shantou City, Guangdong Province of China (23.45° N, 116.88° E) (Figure 1). The area is characterized by a subtropical monsoon climate, with 1,672 mm of annual precipitation, mostly between April and September. The seasonal mean air temperatures are: 21.0°C in spring (March–May), 28.3°C in summer (June–August), 23.7°C in autumn (September-November) and 15.2°C in winter (December–February), respectively. Tides are irregularly semi-diurnal with an range from 0.37 to 2.40 m. In 2005, two monospecific stands respectively dominated by K. obovata and S. apetala were established on the muddy tidal flats (4.7% sand, 88.2% silt, and 7.1% clay) at similar tidal elevation of 1.45–1.55 m. Every seedling was planted about 3 m apart. After 12 years, the of K. obovata (KO) and S. apetala (SA) monocultures experienced natural self-thinning process with different stem density, tree height and basal area per tree (He et al., 2020). Seedlings of K. obovata had also naturally colonized into stands of S. apetala and developed an understory shrub layer along the border of monospecific plantations, and finally became a mixed stand with both S. apetala and K. obovata (SK), with a density of 2,600 stems ha–1. The average daily flooding period of these plantations is 10.3 h, which is suitable for the growth of both species (Ye et al., 2003; Chen et al., 2004; Cheng et al., 2015).
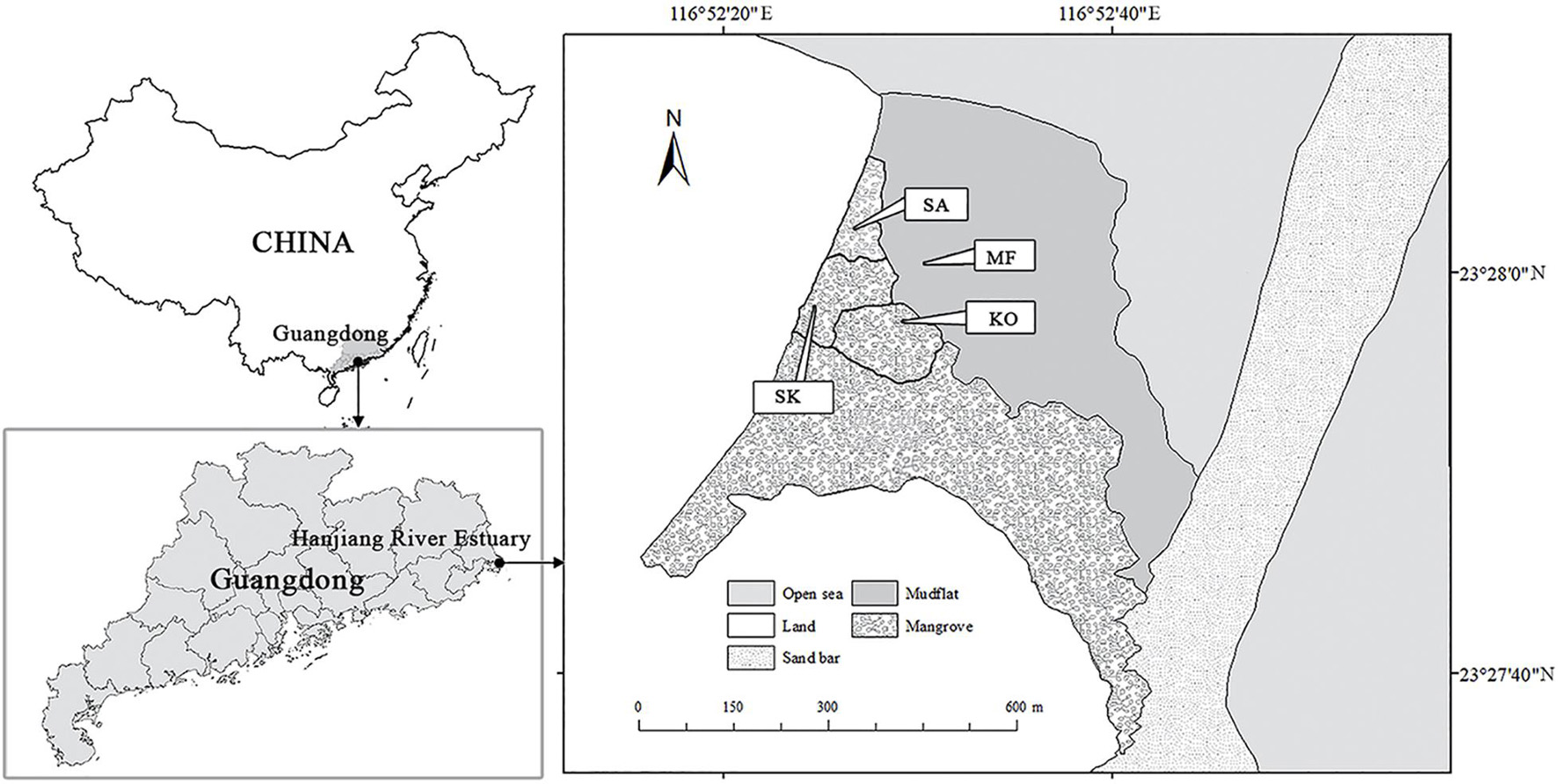
Figure 1. Locality of the study mangrove forests at Hanjiang River Estuary, Guangdong Province, southern China.
Measurement on Fine Root Biomass and Necromass
Fine roots biomass and necromass were determined by the root coring method (He et al., 2018, 2020). Four plots of 10 m × 10 m (>10 m apart from each other) were randomly established at each plantation in each season over a year: October (autumn) of 2016, January (winter), April (spring) and July (summer) of 2017. The same sampling protocols were applied to all plots. At each sampling time, three standard trees were randomly selected in each plot. One soil core (11 cm diameter × 1 m depth) was taken from the central position under the canopy of each standard tree selected. Then, each soil core was sectioned into five vertical segments (0–20, 20–40, 40–60, 60–80 and 80–100 cm depth). Three core segments of the same soil depth from each plot were pooled into a composite sample for subsequent root separation. In total, 45 samples were collected at each sampling time.
In the laboratory, the cores were washed over a 0.25 mm mesh sieve with tap water and roots over 2 mm in diameter were discarded. The remaining fine roots were then separated into live and dead fractions with 11 and 6% colloidal silica (Ludox TM, Sigma-Aldrich Inc., United States), following Robertson and Dixon (1993). Live fine roots would float on the top and dead fine roots sink to the bottom of the colloidal silica, as live fine roots have lower specific gravity than dead fine roots. The separated fine roots were then oven-dried at 65°C to a constant weight.
Measurement on Fine Root Production and Turnover Rate
Fine root production was estimated using the ingrowth core method (Poungparn et al., 2016). In early December 2016, a total of 36 ingrowths soil cores were vertically inserted into the forest substrate (3 replicates × 4 collection times × 4 plots per plantation) to 1 m depth until the top end of the core was level with the substrate surface. Each ingrowth cores composed of five sub-cores (11 cm diameter × 20 cm length) constructed by nylon mesh bags (1 cm pore size), and were completely filled with root-free soil collected from the adjacent unvegetated mudflat after sieving through a nylon mesh to ensure it was entirely root-free. Then every five cores were strung together onto a nylon rope to form a 1-m long ingrowth core. The ingrowth cores were retrieved after 90 (March 2017), 180 (June 2017), 270 (September 2017), and 360 (December 2017) days, respectively, and three cores were collected from each plot at each harvest time. The roots from the ingrowth cores were washed over a 0.25 mm sieve with tap water and were sorted into live and dead roots and oven-dried according to the methods mentioned previously.
Measurement on Fine Root Decomposition
Fine root decomposition was measured using an intact-core method that closely mimics in situ fine roots decay conditions (Dornbush et al., 2002). Since cores were sampled from field soil and maintained as intact units, the initial mass of fine roots within the cores was unknown. Hence, the mass loss estimated from the intact cores were estimated based on change in population means through time, but not change in individual samples. This approach required sufficient replicate cores to accurately quantify the mean fine roots mass at each sampling time. The number of cores required was determined prior to the experiment. For this purpose, initial sampling was conducted in the mangrove forest in early December 2016, with the coefficient of variation demonstrating no apparent decline after 11 samples. Therefore, this sample size was applied to the plantations in fine root decomposition experiment.
Based on the result of the pilot sample size experiment, eleven standard trees were randomly selected for root coring within each plantation in early March 2017. Four root cores (11 cm diameter × 1 m depth) were taken from the middle position under the canopy of each tree. Cores were vertically divided into five 20-cm segments: 0–20, 20–40, 40–60, 60–80, and 80–100 cm. For each core, segments were put in 0.1 mm pore size nylon mesh bag (11 cm diameter × 20 cm length), respectively. Then the five mesh bags were strung to form a 1-m intact soil core and placed into the ground holes, which had been excavated earlier from root core sampling to the corresponding depth interval. A total of 132 intact cores (11 replicates × 4 collection times × 3 plantations) were processed in all plantations. The intact cores were collected after 90 (June 2017), 180 (September 2017), and 270 (December 2017), and 360 (March 2018) days, respectively. Eleven intact cores were removed from each plantation at each collection time. Upon collection, fine roots (<2 mm) were washed over a 0.25 mm mesh sieve with tap water, then sorted and dried at 65°C until constant weight.
Soil Sampling
Soil cores were collected with PVC tubes (1 m depth × 11 cm diameter) in March 2017. Five soil cores were randomly collected from three mangrove plantations and the nearby mudflat (MF). The soil cores were sectioned into five layers (0–20, 20–40, 40–60, 60–80 and 80–100 cm). Soil samples were then air-dried for the following chemical analyses.
Fine Root and Soil Chemical Analyses
Organic carbon (OC), total nitrogen (TN) and total phosphorous (TP) content (g⋅kg–1) of fine roots from the decomposition experiment and the soil samples were measured in the laboratory. Fine roots from biomass determination in March 2017 were also measured, which provided the initial OC, TN, and TP contents of fine roots tissue. All mass values reported in this paper refer to ash-free dry mass. Dry fine roots and soil samples were ground to a fine powder. Soil samples were determined following the modified Walkley-Black method for measuring OC content in soil (Schumacher, 2002; Ha et al., 2017). The organic content of the fine roots samples were analyzed using loss-on-ignition method (Heiri et al., 2001; Ha et al., 2017). The TN concentration of fine roots and soil were measured with an elemental analyzer (Thermo-Finnigan EA1112, Milan, Italy). The TP content was determined using a K2S2O8 digestion at high pressure followed by the ammonium molybdate spectrophotometry method (Xuluc-Tolosa et al., 2003; Song et al., 2015).
The soil bulk density was obtained by the ratio of the oven-dried mass of soil and the original wet sample taken by the volumetrically fixed PVC cores (He et al., 2018). The air-dried fine power of soil samples was also used for soil pH and salinity determination. Soil pH and salinity were measured with 1:2.5 (w/v) and 1:5 (w/v) ratio of soil to deionized water by pH meter and YSI-ProPlis multiprobe sensor (Incorporated, OH, United States), respectively (He et al., 2018).
Data Analysis
The mean value of live and dead fine roots obtained in all four sampling times were used to analyze the distribution of fine root biomass and necromass. The dried fine root biomass and fine root necromass was used for calculating fine root production during each period of study using the Decision-Matrix method (Fairley and Alexander, 1985; Ostonen et al., 2005; Brunner et al., 2013; Xiong et al., 2017). Annual fine root production was calculated by summing up all production values between successive pairs of data throughout a full year (Brunner et al., 2013; Poungparn et al., 2016). The turnover rate of fine roots was calculated by dividing annual fine root production by mean biomass (Brunner et al., 2013). For calculating the fine root decay rate constant (k), data of decomposition experiment were fitted to the negative exponential decay model (Dornbush et al., 2002):
where y (%) is the percentage of initial mass remaining at time t (month), and k is the decay rate constant (month–1).
The soil organic carbon stock was calculated by multiplying the soil bulk density and the soil organic carbon content. The organic carbon stock of the five soil layers was summed to estimate the soil organic carbon stock for each sampling site (He et al., 2020).
All statistical analysis were performed with R 3.5.0 (R Foundation for Statistical Computing, Vienna, Austria). Log -root transformations were applied to meet requirements for data normality and homogeneity of variances, if necessity. One-way ANOVA analyses were conducted to determine the differences of soil parameters among the three mangrove plantations and the adjacent mudflat at different depths, with plantation as a fixed factor. Live fine root biomass and fine root necromass within three plantations at different depths were also compared by one-way ANOVA. The difference in soil parameters, fine root mass (live and necromass), annual production, turnover and decomposition rate among sites and soil depths or seasons were compared by two-way ANOVAs, with soil depth or season, and species/sites as fixed factors. Linear regression was used to explore trends between soil nutrient content and fine root production. Relationships between fine root mass (live and dead) production and decay rate constants with organic carbon in soil were also determined by linear regression.
Results
Spatial Variation of Soil Properties
The mean values of soil pH were highest in mudflat, and pH in the three mangroves soil tended to increase by soil depth (Figure 2A). Conversely, soil salinity was highest in KO, followed by SA and SK (there was no statistic variation between SA and SK), and lowest in mudflat soil (Figure 2B). Soil bulk density (SBD) in the four study sites increased with depth, with significant differences across soil depths and habitats (p < 0.05; Figure 2C and Table 1). The mean soil organic carbon (SOC) content (0–100 cm) was highest in KO (33.0 g⋅kg–1), nearly 1.6, 3.5, and 6.0 times higher than those of SK (21.0 g⋅kg–1), SA (9.6 g⋅kg–1), and MF (5.5 g⋅kg–1), respectively. The SOC content significantly decreased with soil depth in the two monocultures but no significant trend was found in SK and MF (Figure 2D). A significant difference was detected across habitats, with a significant difference also among soil depths (p < 0.01; Table 1). There were significant differences in soil total phosphorus content (STP) among soil depths and habitats (p < 0.01; Figure 2E and Table 1). Soil total nitrogen content (STN) showed a pattern similar to SOC content, that the STN content decreased significantly with depth in the monocultures (p < 0.001; Figure 2F). Significant difference among habitats was also detected (p < 0.001; Table 1). The mean soil organic carbon stock of KO was 15.8 ± 0.8 Mg OC ha–1, almost 1.3, 2.0, and 3.4 times higher than those of SK, SA and MF, respectively.
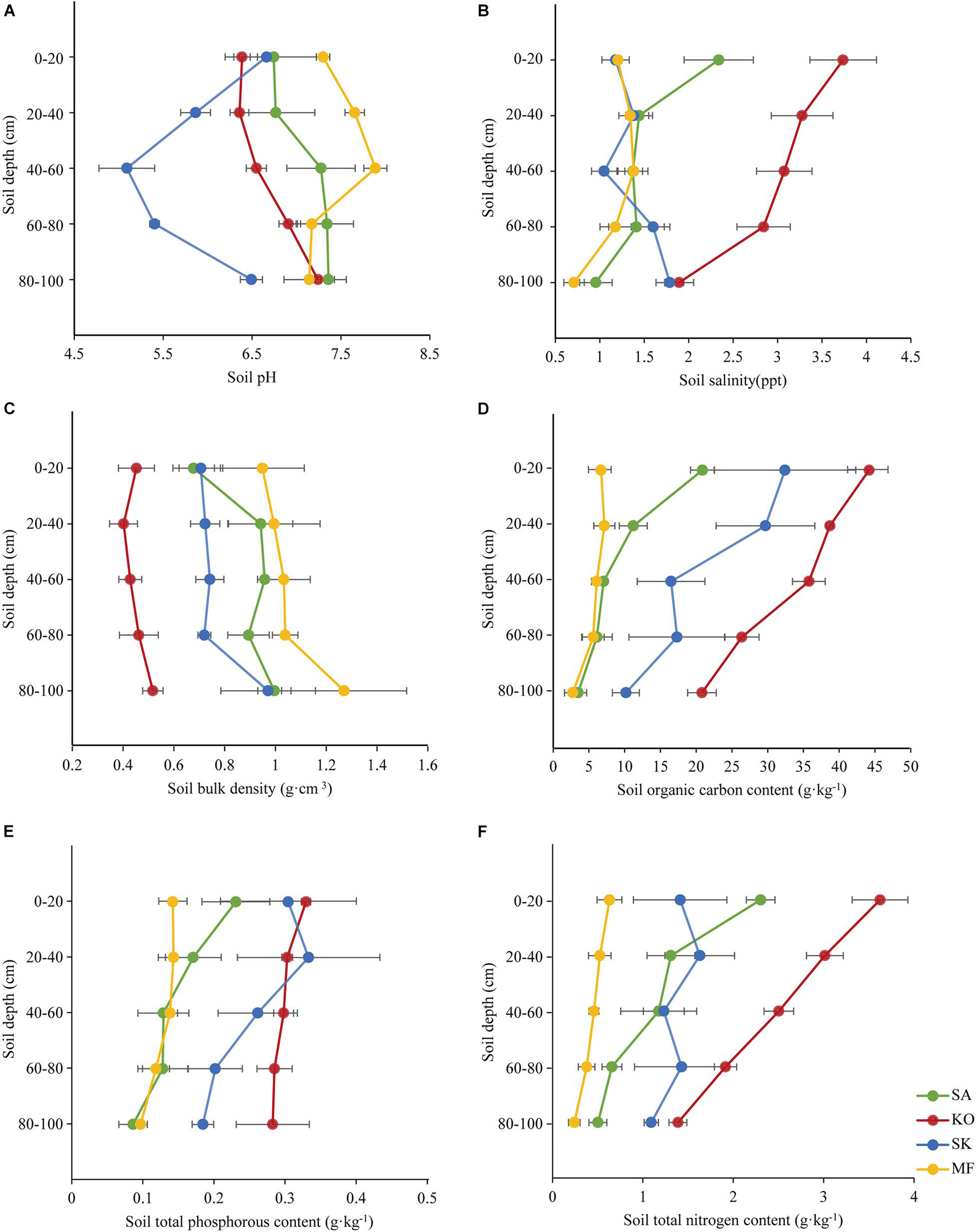
Figure 2. Soil properties and their vertical distribution in K. obovata (KO), S. apetala (SA) monocultures, mixed plantation (SK), and mudflat (MF) at Hanjiang River Estuary, south China. (A) Soil pH. (B) Soil salinity. (C) Soil bulk density. (D) Soil organic carbon content. (E) Soil total phosphorous content. (F) Soil total nitrogen content. Error bars represent 1SE, and SE represents standard error (n = 5). Data of soil pH, salinity, bulk density, and soil organic carbon content in K. obovata and S. apetala monocultures and the mudflat are from He et al. (2018).
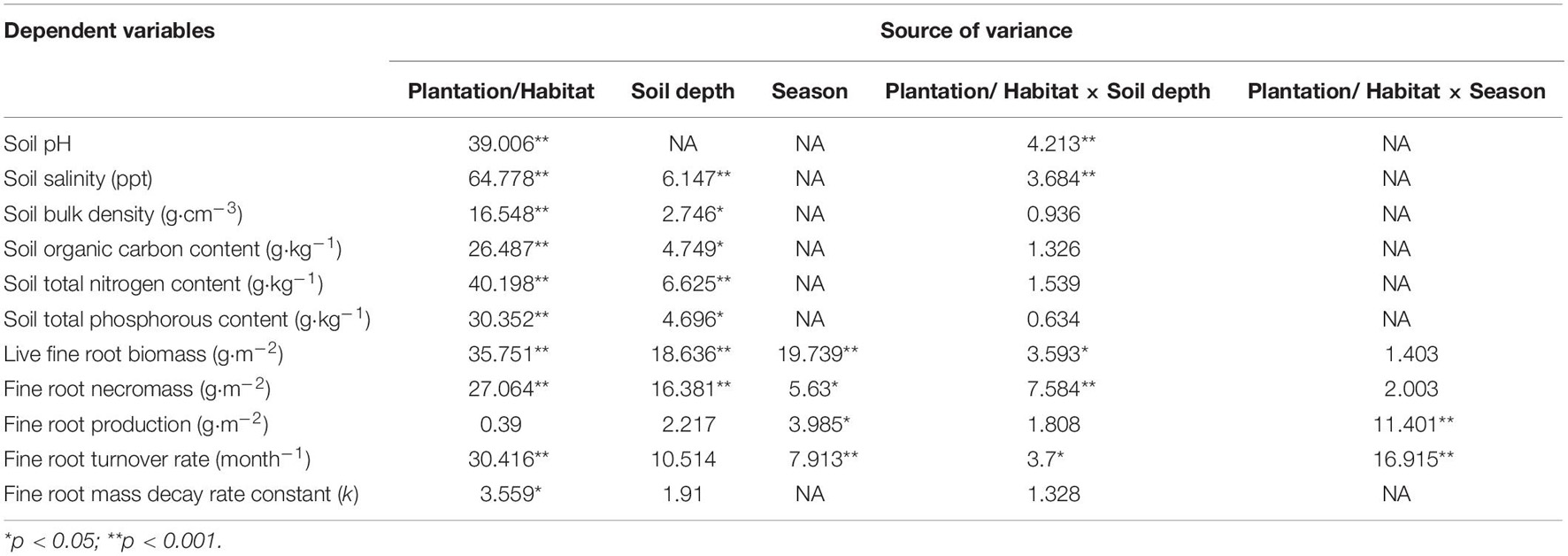
Table 1. F values of two-way ANOVA testing the differences in fine root dynamics and soil variables among different mangrove species/habitats and soil depth in Hanjiang River Estuary, south China.
Vertical Profile and Seasonal Variation of Live Fine Root Biomass and Necromass
In three mangrove plantation types, live fine root biomass was significantly decreasing with soil depth (p < 0.01; Figure 3A). Similarly, fine root necromass in SA and KO was significantly negatively correlated with soil depth, while such pattern was reversed in SK (p < 0.001; Figure 3B).
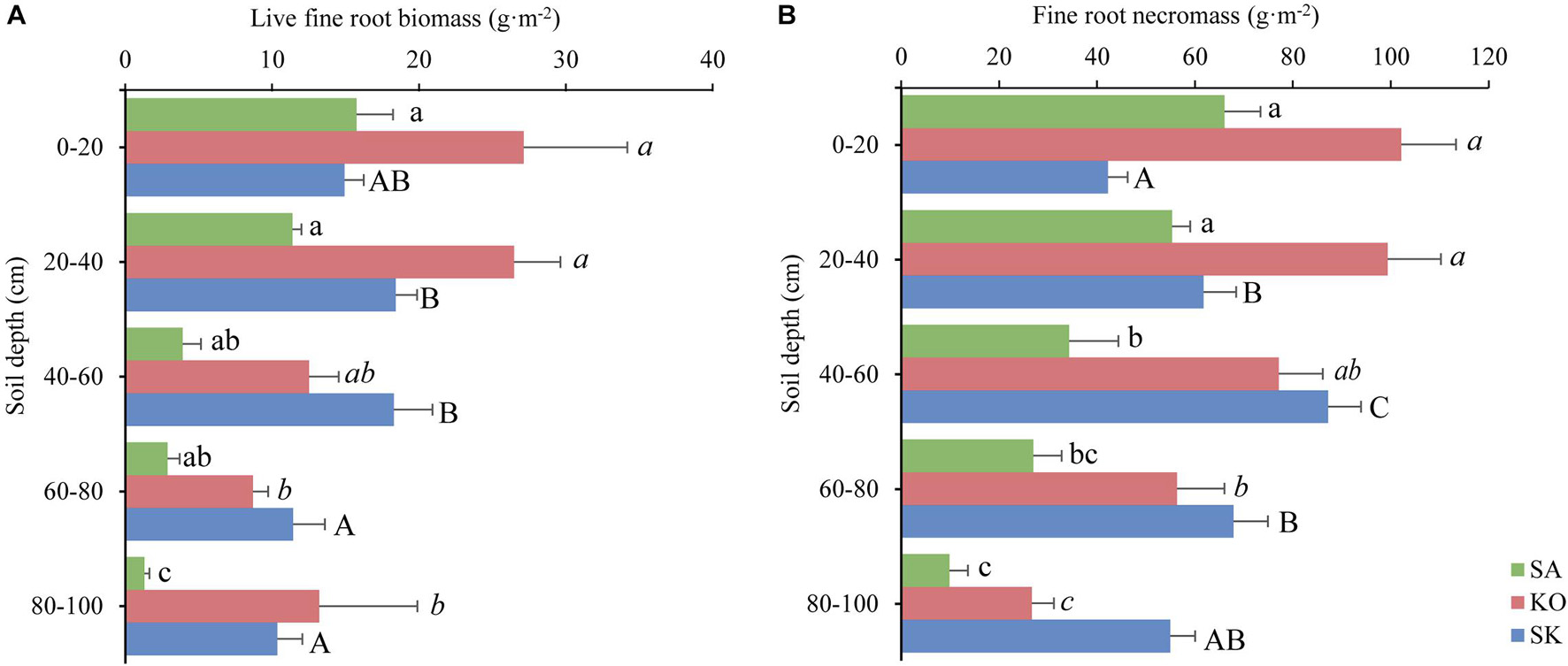
Figure 3. Spatial distribution of live fine root biomass (A) and necromass (B) (mean ± 1SE) in K. obovata (KO), S. apetala (SA) monocultures and mixed plantation (KS) at Hanjiang River Estuary, south China. Different letters indicate significant differences among different soil depths within the same plantation type (p < 0.05).
The annual average live fine root biomass of the plantations was 35.2 ± 2.5 g⋅m–2 for SA, 88.0 ± 4.3 g⋅m–2 for KO, and 73.4 ± 6.7 g⋅m–2 for SK. Significant differences existed among plantation types and soil depths (p < 0.001; Figure 3A and Table 1). There was a significant interaction between plantation types and soil depth on live fine root biomass (p < 0.05; Table 1). In the two monocultures, KO exhibited higher live fine root biomass than SA in the upper soil layer (0–40 cm), where 72 and 66% of K. obovata and S. apetala’s live fine root biomass occurred, respectively. The overall fine root necromass peaked in KO, followed by SK and SA. Significant differences in fine root necromass existed among plantation types and soil depths (p < 0.001; Figure 3B and Table 1).
In terms of seasonal patterns, there were significant interactions between season and plantation on both live fine root biomass and fine root necromass (p < 0.05; Figure 4 and Table 1). Among four seasons, the minimum live fine root biomass occurred in spring (Figure 4). However, the maximum live fine root biomass of SA and SK were detected in autumn, while that of KO was found in summer (Figure 4). Fine root necromass in all plantations were significantly higher than live fine root biomass, with significant differences among seasons and plantation types (p < 0.05; Figure 4 and Table 1).
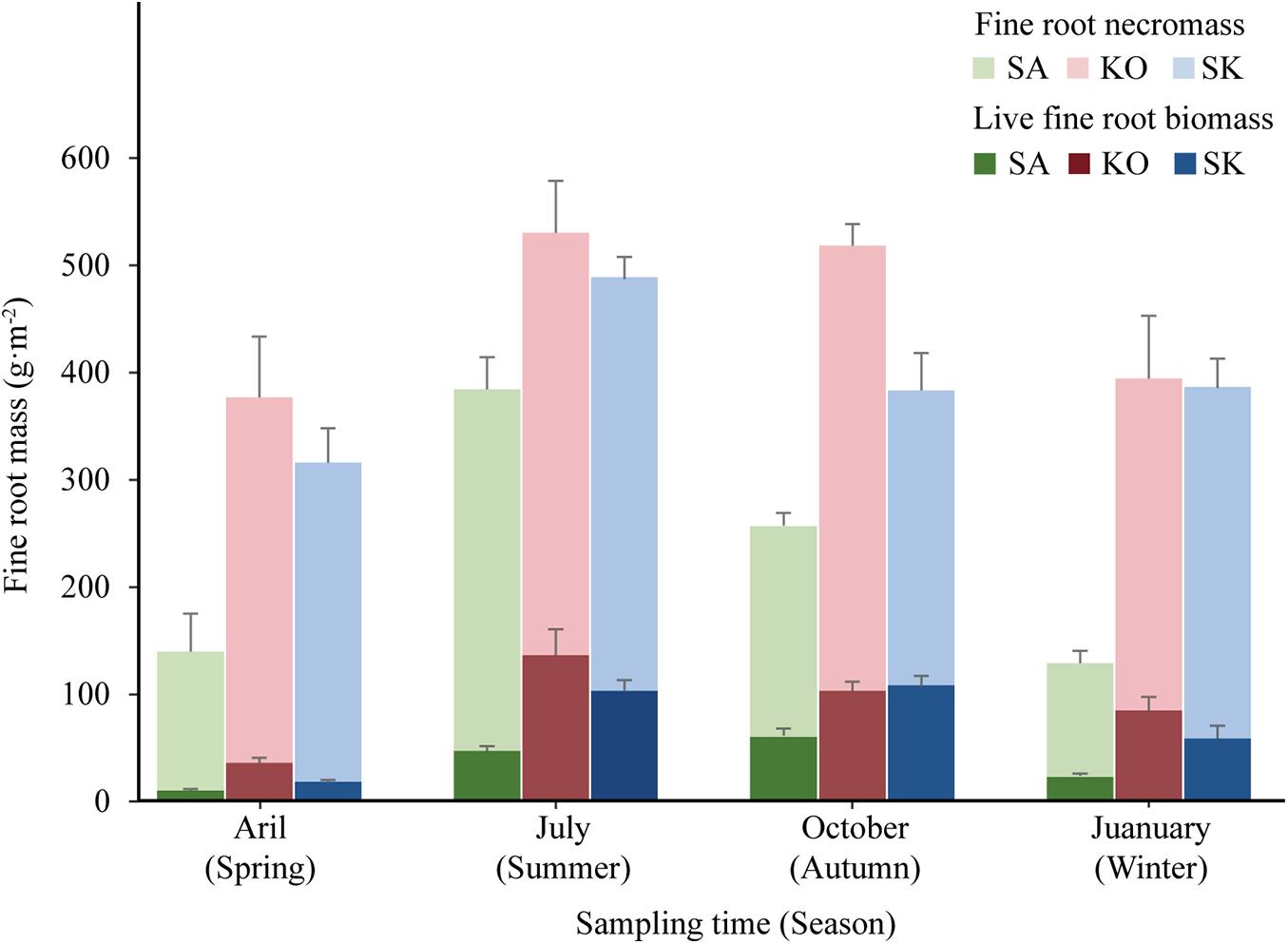
Figure 4. Seasonal variation of live fine root biomass and fine root necromass (mean ± 1SE) in SA, KO, and SK plantation at Hanjiang River Estuary, south China.
Fine Root Production and Turnover Rate
Annual fine root production of mangroves in the three plantations ranged between 143.4 and 166.6 g⋅m–2 yr–1. The annual fine root production of KO and SK were 1.2 and 1.1 times higher than that of SA, respectively. Fine root production differed significantly among seasons, which had a significant interaction with plantation types (p < 0.05; Tables 1, 2). Annual fine root production was positively correlated to soil nutrient (p < 0.001; Figure 5).
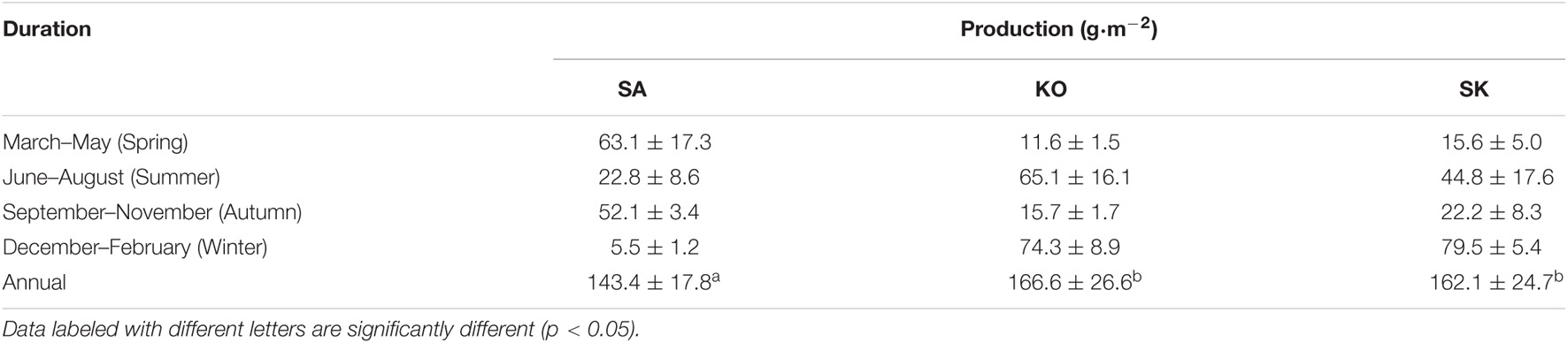
Table 2. Fine root production (mean ± SE, n = 4 plots) in the three plantations in Hanjiang River Estuary, south China.
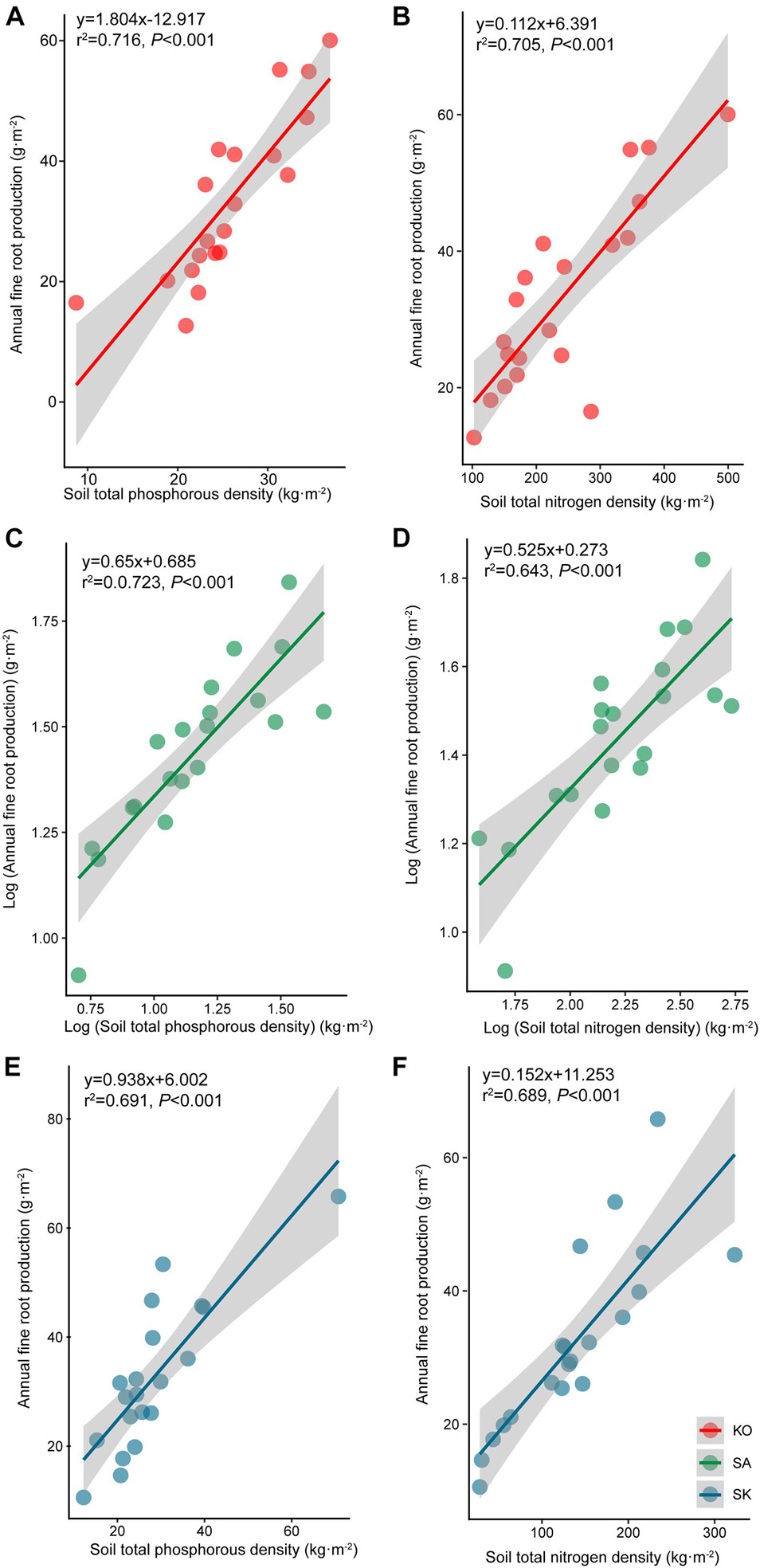
Figure 5. Regression analysis between soil total phosphorous (A–C) and nitrogen content (D–F) and annual fine root production for S. apetala (SA), K. obovata (KO) monocultures and the mixed (SK) plantations at Hanjiang River Estuary, south China.
Fine root turnover rate was fastest in SA (4.1 ± 0.5 yr–1), followed by SK (2.2 ± 0.3 yr–1), and slowest in KO (1.9 ± 0.3 yr–1). KO and SK displayed similar fine root turnover patterns in the year, with maximum and minimum rates in spring and summer, respectively, while SA demonstrated an opposite seasonal pattern (Figure 6). A significant interaction effect between season and plantation was also detected (p < 0.001; Table 1).
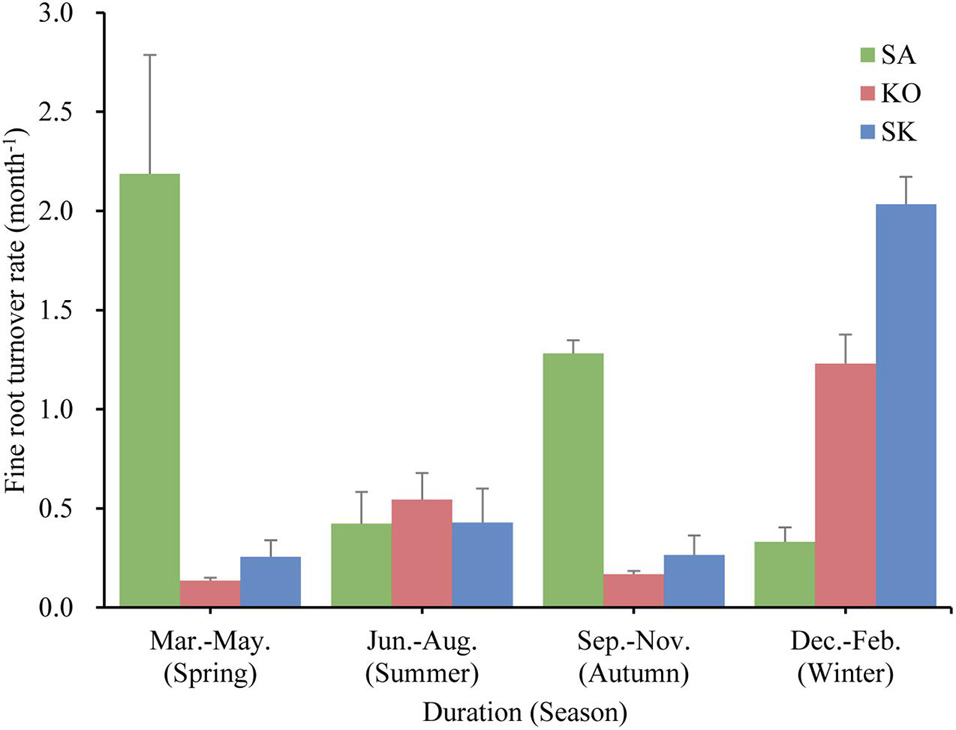
Figure 6. Fine root turnover rate (month– 1) for S. apetala (SA), K. obovata (KO) monocultures and the mixed (SK) plantations in different seasons over a year at Hanjiang River Estuary, south China.
Fine Root Decomposition
The fine root mass in the intact cores lost 85.5, 26.6, and 38.9% for SA, KO and SK, respectively, over a 12-month decomposition period. The average decomposition rate constant (k) of SA fine root was significantly higher than those for KO and SK (p < 0.001; Figure 7A). This suggests that SA fine roots were not as recalcitrant as those of KO and SK, with faster decomposition dynamics.
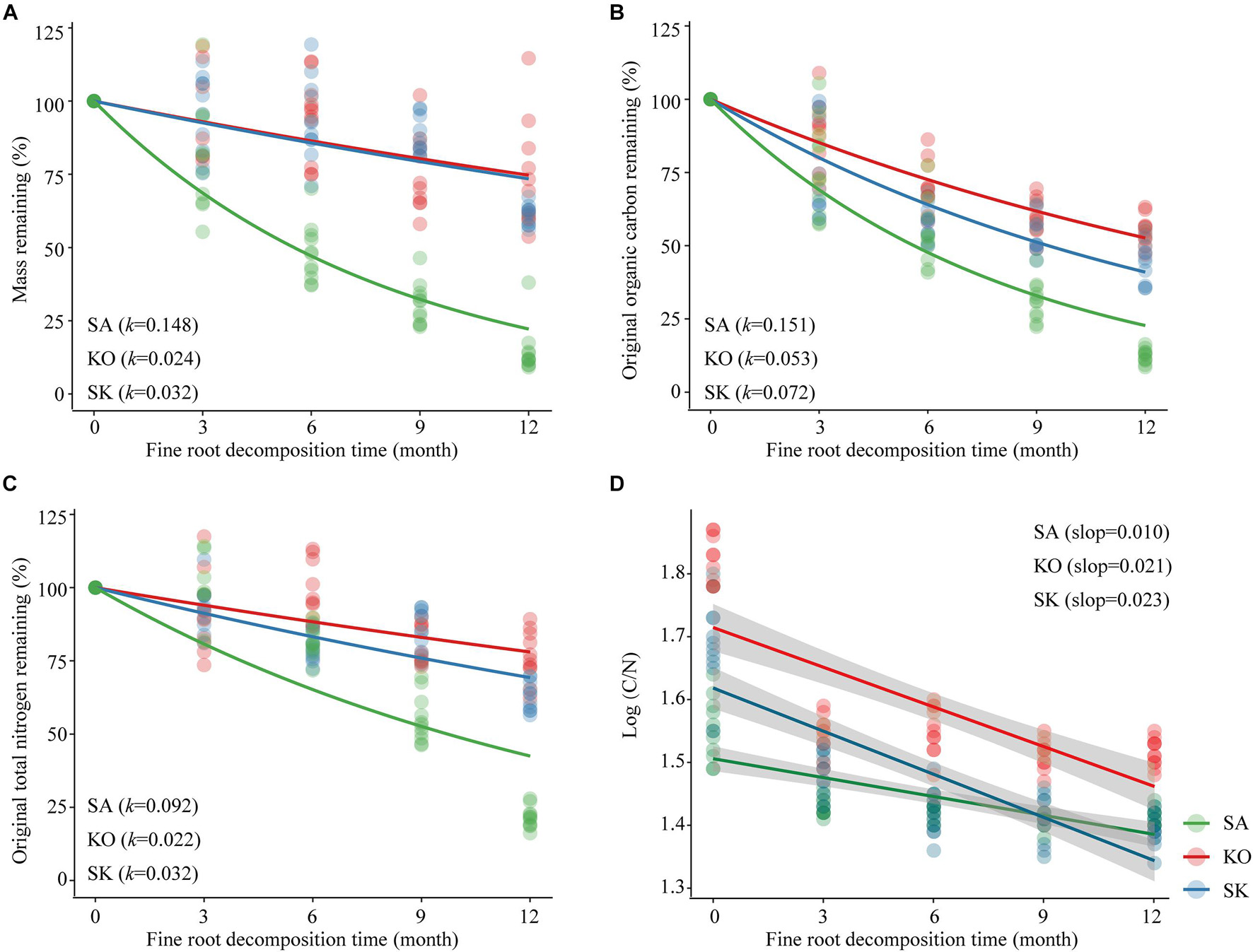
Figure 7. Loss of mass (A), organic carbon (B), total nitrogen (C) and changes in C/N (D) for fine root of S. apetala (SA), K. obovata (KO) monocultures and the mixed (SK) plantations upon decomposition at Hanjiang River Estuary, south China. k values were estimated based on the negative exponential decay model. Data were normalized such that 100% equals the t = 0 intercept of the exponential decay regression. Linear best fits for (d): SA (y = –0.01x + 1.506, r2 = 0.513); KO (y = –0.021x + 1.714, r2 = 0.562); SK (y = –0.023x + 1.619, r2 = 0.668). All regressions are significant (p < 0.001).
The initial chemical composition of fine root tissue differed significantly among plantation types (p < 0.001). SK fine roots were characterized by the highest organic carbon (OC) (549.8 ± 40.4 g kg–1) and total nitrogen (TN) (11.0 ± 0.6 g⋅kg–1) contents, while the lowest fine root OC content was found in SA (328.2 ± 13.0 g⋅kg–1), and the lowest fine root TN content occurred in KO (7.1 ± 0.4 g⋅kg–1). Higher initial C/N ratio was associated with KO (65.3 ± 2.1) than in SA (35.5 ± 1.5) and SK (51.1 ± 2.9). After 12-month decomposition, the proportions of original fine root OC remaining in the intact cores were 12.3% for SA, 44.8% for SK, and 55.0% for KO. The proportion of original TN remaining had a similar pattern as that of OC, with SA, SK, KO at 21.6, 63.0, 76.4%, respectively. As with mass loss, there were significant differences in OC and TN loss among different plantation types (p < 0.001). The decomposition rate constant (k) indicated that fine root OC and TN loss rates were highest in SA, followed by SK and KO (Figures 7B,C). Fine root C/N ratio declined significantly through time within intact cores (p < 0.001; Figure 7D). The slopes indicate that the monthly decreases of fine root C/N ratio in KO and SK were significantly higher than that in SA (p < 0.001; Figure 7D).
Effect of Fine Root Dynamic on Soil Organic Carbon Accumulation
Soil organic carbon density in the three mangrove plantations was positively correlated to live fine root biomass and necromass (p < 0.05; Figures 8A,B). Similarly, significant positive correlation was detected between soil organic carbon density and annual fine root production (p < 0.05; Figures 8C,D). Soil organic carbon stock was highest in KO, followed by SK and SA, same as the profile exhibited by live fine root biomass, fine root necromass, and annual fine root production. The high fine root mass and productivity likely contributed to soil organic carbon storage in KO. Soil organic carbon was negatively correlated with the decay rate constant, indicating that slower fine root decomposition rate may be responsible for higher soil organic carbon stock. Overall, fine root dynamics seems to have a significant effect on mangrove soil organic carbon stock, and KO had higher potential for organic carbon accumulation than SA and SK through fine root production and turnover.
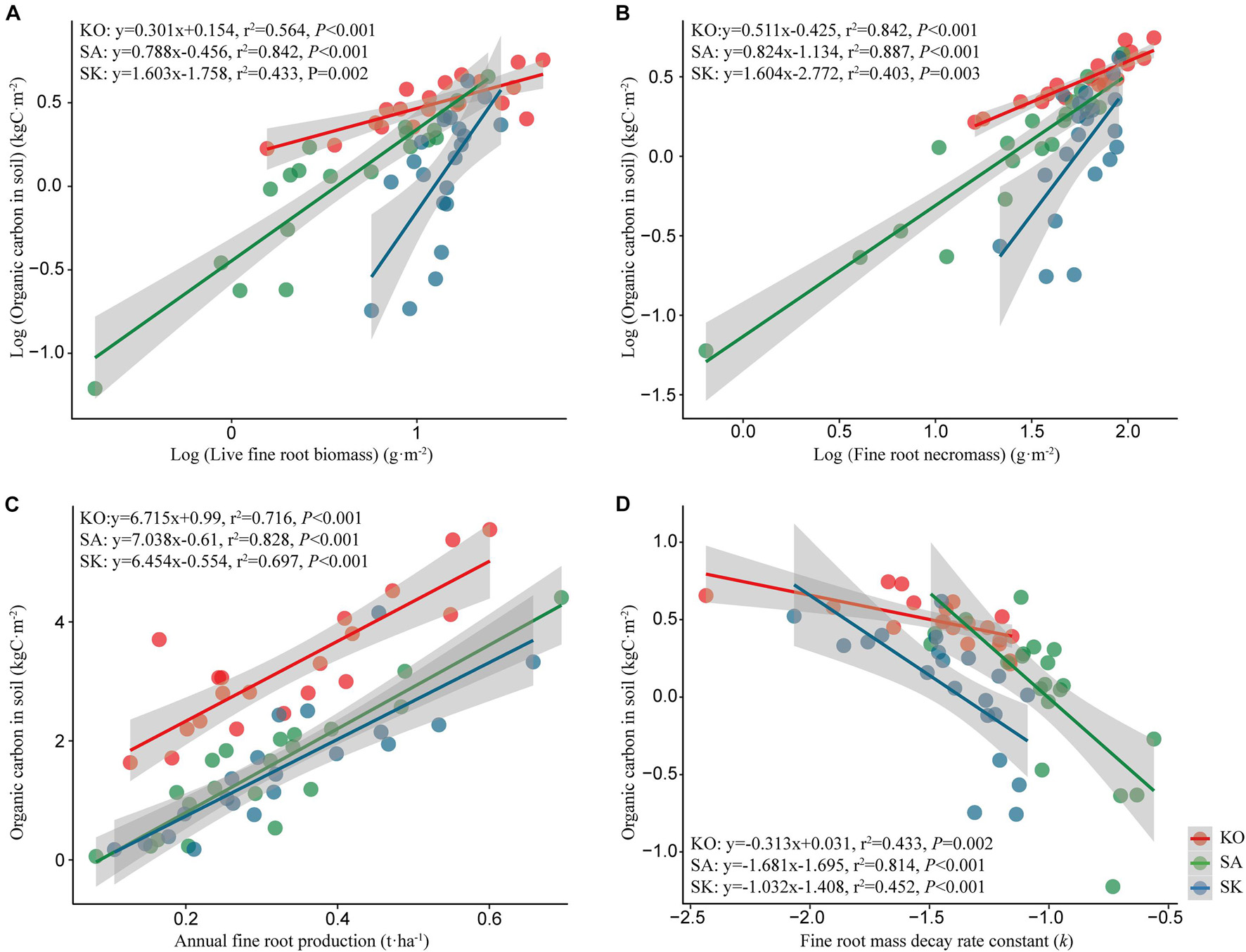
Figure 8. Regression analysis between organic carbon in soil and live fine root biomass (A), fine root necromass (B), annual fine root production (C), and fine root mass decay rate constant (D) for S. apetala (SA), K. obovata (KO) monocultures and the mixed (SK) plantations at Hanjiang River Estuary, south China.
Discussion
Key Drivers on Modifying the Fine Root Dynamics
Live Fine Root Biomass and Fine Root Necromass
The spatial pattern of live fine root biomass is in broad accordance with previous studies in mangrove forests, that live fine root biomass not only varied with mangrove species but also with soil depth (Figure 3; Ha et al., 2017; He et al., 2018, 2020). Usually, live fine roots mainly occurred in shallow soil layers (Adame et al., 2017; Ha et al., 2017; Xiong et al., 2017). The observed high amounts of fine roots in surface soil layers were attributed to the availability of nutrients and less anoxic conditions (Yuan and Chen, 2010; Srikanth et al., 2016; He et al., 2018). In the current study, the profile of live fine root biomass differed between the two monospecific plantations (Table 1). Live fine root biomass was concentrated at different soil layers between monocultures of K. obovata (0–40 cm) and S. apetala (0–60 cm) (Figure 3), which is closely related to their morphological traits. More superficial fine roots were required in K. obovata to ensure gas exchange and nutrient uptake due to the absence of typical pneumatophores (Okello et al., 2019; Al-Khayat and Alatalo, 2021). In contrast, pneumatophores in S. apetala helps reduce stress from anoxic conditions, allowing supportive and absorptive roots to penetrate into deeper soil layers (He et al., 2018, 2020).
Usually, large amounts of dead fine roots accumulate in mangrove substrate (Chalermchatwilai et al., 2011). Consistent with studies on Rhizophora and Avicennia species, dead fine roots accounted for >80% of the total fine root mass in both KO and SA plantations (Figure 3) (Alongi and Dixon, 2000; Alongi et al., 2000, 2003). Other studies support that this fine root necromass component is refractory and may be able to provide long-term carbon storage (Tamooh et al., 2008). Fine root necromass in KO significantly higher than that of SA may reflect K. obovata has higher capacity in long-term carbon storage than S. apetala. Besides, SK had significantly higher fine root mass than SA. This profile may be attributed to the complementary vertical niches in both above and belowground space utilization in this mixed-species zone. The seasonal pattern of live fine root biomass and necromass may be species specific (Figure 4), dependent on fine root growth and mortality in accordance with the soil environment, e.g., temperature, moisture content, redox condition, and nutrient availability (Xiong et al., 2017).
Fine Root Production and Turnover
Characterized by the intolerance to canopy shade and fast growth rate of S. apetala, SA was featured with significantly lower stem density, resulting in its lower fine root production than KO. In SK, the colonizing K. obovata increased the overall tree density in the original S. apetala plantation. Complementary root traits in the same assemblage drove the two mangrove species to make more sufficient utilization of belowground space (Brassard et al., 2011; Rolo and Moreno, 2012; Lai et al., 2017). Root systems can continuously adjust to changes in soil conditions and floral composition to maximize plant return (Ward et al., 2013). These responses resulted in significantly higher fine root production of SK than SA (Table 2). However, the growth of the colonizing K. obovata was hampered due to shading from S. apetala’s canopy (Peng et al., 2016; Jiang et al., 2019). In general, significantly lower overall stem density and fine root production was evident in the mixed SK than KO monoculture (Table 2). Therefore, the presence of complementary rooting traits was a key determinant on fine root productivity in the mixed stand, also affecting the density of trees (Brassard et al., 2011).
Poungparn et al. (2016) suggested that the variability of fine root production across seasons was underpinned by soil temperature. As mangroves are commonly occur in oligotrophic environments (Xiong et al., 2017), most mangrove species are highly responsive to variations in nutrient availability (Adame et al., 2017). The positive correlation between annual fine root production and soil nutrients suggests that mangrove fine root production may be nutrient dependent at our study sites (Figure 5).
The turnover rates calculated in the present study were within the range of 0.15–9.60 yr–1, used in 17 ecosystem models parameterized for terrestrial forests (McCormack et al., 2015a). Our results support that slower fine root turnover rates resulted in greater standing fine root biomass (McCormack et al., 2015b). Lower fine root turnover rate coupled with higher nutrient content suggested that fine roots may be maintained as long as the nutrients obtained outweigh the cost of keeping them alive. This agrees with the results of root lifespan analysis by Eissenstat and Yanai (1997) that roots in nutrient-rich environments should live longer than in nutrient-limited conditions. Previous studies have demonstrated that soil temperature at least partly control the timing and duration of fine root growth (Yuan and Chen, 2010; Xiong et al., 2017; Muhammad-Nor et al., 2019; Poungparn et al., 2020). Since coarse roots generally do not show seasonal dynamics (Xiong et al., 2017), the pronounced seasonal variation of fine root turnover rate suggests that fine roots represent the most dynamic component in mangrove root systems and are the major contributor to root turnover (Figure 6).
Fine Root Decomposition
Our results in fine root decomposition were consistent with previous studies in showing generally lower decomposition rates in mangrove fine roots compared with those of terrestrial ecosystems (Silver and Miya, 2001; Huxham et al., 2010). Species identity is the main driver of fine root decomposition which may be attributed to the difference in physiology and biochemistry (Ouyang et al., 2017). In the current study, highest soil pH in SA may attribute to the faster organic matter and fine root debris decomposition rate of S. apetala than that of K. obovate (He et al., 2018). S. apetala aerates the sediment through their pneumatophores (Figure 7A), increases oxygenation of the sediment and general permeability of their root system, resulting in faster fine root decay, compared with fine root of K. obovata that limited oxygen transport from above-ground parts (Ouyang et al., 2017). Apart from oxygen transport, different relative nutrient concentrations in fine roots represented as initial C/N ratio between these species may be responsible for such difference (Solly et al., 2014; Sariyildiz, 2015; Ouyang et al., 2017). S. apetala had higher initial TN and lower OC contents compared with K. obovata, resulting in a lower initial C/N ratio in its fine roots. In SK, the higher fine roots initial TN and OC contents may be attributed to the colonization of K. obovata at the understory, contributing to their slower decomposition than in SA (Huxham et al., 2010). The composition of plant community may also affect fine root decomposition through species specific rhizospheric microbial activities (Prieto et al., 2017).
The quality of fine root litter carbon can also affect the carbon-use efficiency of decomposers, leading to differences in the fine root decomposition process (Manzoni et al., 2010). The investment in enzyme production increases with the complexity and content of substrates such as cellulose, tannins, and lignin (Prieto et al., 2017; Song et al., 2017; Pradisty et al., 2021). The organic carbon loss during fine roots decomposition is mainly attributed to the degradation of these components (Melillo et al., 1989). Fine root decay is an important source of minerals in mangrove soil (Silver and Miya, 2001). A meta-analysis of decomposition studies in mangrove also identified a strong relationship between soil nitrogen content and fine root decomposition rate (Poret et al., 2007). The accumulation or release of nutrients derived from decomposing fine root primarily depends on the quality of fine root substrate and the stoichiometric requirements of the microbial decomposers (Song et al., 2017). Net N loss occurs generally when the initial N content is between 6 and 28 g⋅kg–1 or the C/N ratio is lower than the critical threshold of 5–15. In the present study, the initial fine root N content (7.2–11.0 g⋅kg–1) was in the lower range of the threshold, resulting in N loss in all three mangrove plantations. Consistent with studies on mangrove leaves and branch, the C/N ratio decreased during fine root decomposition as N immobilization occurred via microbial activities. Accumulation of refractory fine root material with enhanced nitrogen levels represents a nutrient storage mechanism within mangrove ecosystems (Cebrián and Williams, 1998). However, in our study, the fine root C/N ratios were > 15 over the decomposition period, but net N loss still took place (Figures 7C,D). This unexpected trend may be attributed to the adaptation of microbial decomposer communities to nutrient-limited substrates in mangroves, shifting their stoichiometric requirements. Additionally, decomposers may decrease their C use efficiency to adapt to the low N concentration substrates, thus increasing the critical C/N ratios of fine root litter for net N mineralization in mangrove ecosystems.
Effect of Fine Root Dynamics on Soil Organic Carbon Accumulation
Mangrove plants strongly influence C sequestration in soil through detritus input (He et al., 2020). In this study, soil C increased over time after mangrove plantations had been established on mudflats, as has been reported before (Liu et al., 2014; Kelleway et al., 2016). A high proportion of mangrove soil C is derived from fine roots (Strand et al., 2008; He et al., 2018). This underpins the significantly positive correlation between the spatial distributional patterns of live fine root biomass, necromass, and soil organic carbon across different soil layers, reiterating the contribution of fine roots dynamics to soil vertical accretion and formation (Castañeda-Moya et al., 2011). Simulation models of organic matter content and bulk density also predict that variations in fine root turnover (continuous production, mortality and decomposition) have a strong effect on soil organic carbon accumulation, as has been confirmed by the current study and other empirical studies (Castañeda-Moya et al., 2011; Liu et al., 2017). Therefore, the increased fine root productivity, coupled with reduced fine root decomposition in the anoxic soil environment enhance carbon storage by mangroves. The higher fine root productivity may represent higher belowground carbon allocation and leads to more C inputs to soil, contributing to the high proportion of ecosystem carbon stored underground in mangroves (Donato et al., 2011). For belowground carbon accumulation, root production rate must exceed carbon loss rate from soil (Middleton and McKee, 2001). This may be supported by a large amount of undecomposed fine root necromass may contribute to the high organic matter accumulation. Therefore, a significant correlation between organic carbon in soil and fine root metrics (biomass, necromass, production and decomposition rates) reflects the importance of fine root contribution to soil organic carbon, and the significant effect of fine root dynamics have on soil organic carbon accumulation (Figure 8). The much greater contribution of K. obovata fine roots to soil organic carbon accumulation than S. apetala was due to the significantly slower turnover rates of fine roots of K. obovata relative to those of S. apetala.
Implications of Species Traits for Carbon-Based Mangrove Afforestation
Our study revealed significant differences associated with species traits with respect to the fine root dynamics (live fine root biomass, fine root necromass, productivity, turnover rate and decomposition rate) as well as soil organic carbon content. In this study, SA had lower fine root mass and productivity due to the intensive demand for light and fast-growing characteristics of S. apetala (Chen et al., 2012; Zhu et al., 2020), with significantly stronger self-thinning effect compared with the K. obovata plantation. Combining with its higher fine root turnover and decomposition rates, fine roots of S. apetala may have a lower contribution to soil organic carbon accumulation than those of K. obovata, as the balance between productivity and decomposition of fine roots in the anoxic environment crucially determines the belowground carbon storage in mangrove soils.
Competition for light under a dense canopy in an osmotically-stressed environment is the major hurdle for the establishment of an understory shrub layer in mangrove vegetation (Janzen, 1985; Hogarth, 2015). Chen et al. (2012) suggested that mixed species stands had higher soil carbon storage than monocultures in mangrove plantations. Peng et al. (2016) also reported that mixed mangrove communities might play a more important role in carbon accumulation than monocultures. In the current study, the mixed SK had higher fine root mass and productivity, and lower fine root turnover rate and decomposition rate than SA, attributable to the positive effect of colonization by K. obovata. However, our data also indicated that KO had higher potential than SK for belowground carbon storage. The growth and development of K. obovata in the mixed stand were limited by light availability under the dense canopy of S. apetala, resulting in its lower stem density than in KO. Impacted by the reduced stem density, the mixed SK may not have higher fine root mass and productivity than KO. Despite the presence of K. obovata fine roots has changed the overall fine root litter substrate quality in SK, the mixed fine root litter may still have faster decomposition rates than their counterparts in KO. Combining with our previous study which indicating the sediment carbon pool of S. apetala plantation reached a stable state after 12 years of growth, while that of K. obovata plantation gradually stabilized upon long-term growth (Wu et al., 2020). Therefore, the native K. obovata is preferred to the fast-growing S. apetala for mangrove afforestation for its fine root dynamics and contribution to soil carbon storage at the forest scale, especially for long-term carbon-based mangrove restoration programs. Where the tall S. apetala has been introduced, it can coexist with some shrubby native species (e.g., Kandelia obovata, Aegiceras corniculatum) by partitioning vertical spatial niches both above- and belowground. In locations where S. apetala has already established (e.g., Southern China), eradication is not an effective option but native species can still be introduced to the S. apetala plantations to establish mixed stands to improve the capacity of mangrove plantations for carbon sequestration. This approach of conducting mangrove restoration or reforestation using multiple species with complementary functional traits and niches would prove to bring significantly more ecosystem benefits than the monospecific approach (Lee et al., 2019).
Conclusion
Our analyses show that mangrove fine root dynamics (fine root mass, production, turnover rate, and decomposition rate) were mainly affected by soil nutrient condition and species composition. In addition, season (temperature) also contributed to the dynamics of fine root mass, production, and turnover. The fine root dynamics and soil organic carbon stock of 12-year mangrove plantations respectively dominated by the introduced S. apetala and native K. obovata suggested the latter species had a higher potential for belowground carbon sequestration and storage than S. apetala. Apart from the previous studies, the differences in fine root dynamics and soil organic carbon storage between the colonized S. apetala plantation and the K. obovata monoculture indicated that mixed communities may not have higher soil organic carbon storage than monocultures. Functional trait of different mangrove species is a key factor to determine the carbon storage function of mixed stands. A significant linear correlation exists between soil organic carbon and live, dead fine root biomass, productivity as well as decomposition rate, suggesting that fine roots play an important role in carbon storage, and fine root dynamics have a significant effect on carbon sequestration in mangrove ecosystems. The overall soil organic carbon stock in the K. obovata monoculture was significantly higher than that of S. apetala. Therefore, K. obovata is recommended in soil carbon sequestration and storage oriented mangrove restoration programs rather than S. apetala. These two mangrove species have contrasting but complementary functional traits. For enhanced carbon accumulation, the native K. obovata can be introduced to existing S. apetala plantations to establish mixed communities.
Data Availability Statement
The original contributions presented in the study are included in the article/Supplementary Material, further inquiries can be directed to the corresponding author/s.
Author Contributions
ZYH, YP, and ZH originally planned and designed the research. ZYH, YP, HS, and ZY performed the field work. ZYH, HS, ZY, LZ, and MW contributed to the laboratory analysis. XY produced all the figures. ZYH wrote the first draft of the manuscript. YP, ZH, and SL further improved the manuscript. All the authors commented and approved the manuscript.
Funding
This study was supported by a China Postdoctoral Science Foundation funded project (2020M672939), Guangdong Basic and Applied Basic Research Foundation (2020A1515110657), National Natural Science Foundation Grant of China (41771095, 42106159, 51761135022, and 91951207), and Project supported by Innovation Group Project of Southern Marine Science and Engineering Guangdong Laboratory (Zhuhai) (SML2020SP004, Nos. 311021003 and 311021004), ANCODE (Applying nature-based coastal defense to the world’s largest urban area-from science to practice) project, a three-way international funding through the Chinese National Natural Science Foundation (NSFC, Grant 51761135022), the Netherlands Organization for Scientific Research (NWO, lead funder, Grant ALWSD.2016.026), the U.K. Research Councils (UKRI Grant EP/R024537/1), Fundamental Research Funds for the Central Universities of China (20lgzd16), and Guangdong Provincial Department of Science and Technology (2019ZT08G090).
Conflict of Interest
The authors declare that the research was conducted in the absence of any commercial or financial relationships that could be construed as a potential conflict of interest.
Publisher’s Note
All claims expressed in this article are solely those of the authors and do not necessarily represent those of their affiliated organizations, or those of the publisher, the editors and the reviewers. Any product that may be evaluated in this article, or claim that may be made by its manufacturer, is not guaranteed or endorsed by the publisher.
Acknowledgments
The authors sincerely thank Siluo Chen and Yanping Guo for their help with fieldwork; Zhixiong Chen, Liqing Guo, and Qingjuan Fan for their help with laboratory instruments.
Supplementary Material
The Supplementary Material for this article can be found online at: https://www.frontiersin.org/articles/10.3389/fmars.2021.763922/full#supplementary-material
References
Adame, M. F., Cherian, S., Reef, R., and Stewart-Koster, B. (2017). Mangrove root biomass and the uncertainty of belowground carbon estimations. For. Ecol. Manag. 403, 52–60. doi: 10.1016/j.foreco.2017.08.016
Al-Khayat, J. A., and Alatalo, J. M. (2021). Relationship between tree size, sediment mud content, oxygen levels, and pneumatophore abundance in the mangrove tree species Avicennia marina (Forssk.). Vierh. J. Mar. Sci. Eng. 9:100. doi: 10.3390/jmse9010100
Alongi, D., Tirendi, F., and Clough, B. (2000). Below-ground decomposition of organic matter in forests of the mangroves Rhizophora stylosa and Avicennia marina along the arid coast of Western Australia. Aquat. Bot. 68, 97–122. doi: 10.1016/S0304-3770(00)00110-8
Alongi, D. D., and Dixon, P. P. (2000). Mangrove primary production and above-and below-ground biomass in Sawi Bay, Southern Thailand. Phuket Mar. Biol. Center Spec. Publication 22, 31–38.
Alongi, D. M., Clough, B. F., Dixon, P., and Tirendi, F. (2003). Nutrient partitioning and storage in arid-zone forests of the mangroves Rhizophora stylosa and Avicennia marina. Trees 17, 51–60. doi: 10.1007/s00468-002-0206-2
Brassard, B. W., Chen, H. Y., Bergeron, Y., and Paré, D. (2011). Differences in fine root productivity between mixed−and single−species stands. Funct. Ecol. 25, 238–246. doi: 10.1111/j.1365-2435.2010.01769.x
Brunner, I., Bakker, M. R., Björk, R. G., Hirano, Y., Lukac, M., Aranda, X., et al. (2013). Fine-root turnover rates of european forests revisited: an analysis of data from sequential coring and ingrowth cores. Plant Soil 362, 357–372. doi: 10.1007/s11104-012-1313-5
Caplan, J. S., Meiners, S. J., Flores-Moreno, H., and McCormack, M. L. (2019). Fine-root traits are linked to species dynamics in a successional plant community. Ecology 100:e02588. doi: 10.1002/ecy.2588
Castañeda-Moya, E., Twilley, R. R., and Rivera-Monroy, V. H. (2013). Allocation of biomass and net primary productivity of mangrove forests along environmental gradients in the Florida Coastal Everglades, USA. For. Ecol. Manag. 307, 226–241. doi: 10.1016/j.foreco.2013.07.011
Castañeda-Moya, E., Twilley, R. R., Rivera-Monroy, V. H., Marx, B. D., Coronado-Molina, C., and Ewe, S. M. (2011). Patterns of root dynamics in mangrove forests along environmental gradients in the Florida Coastal Everglades, USA. Ecosystems 14, 1178–1195. doi: 10.2307/41505942
Cebrián, J., and Williams, M. (1998). Accumulation on autotrophic nutrient content in ecosystems. Ecol. Lett. 1, 165–170. doi: 10.1046/j.1461-0248.1998.00040.x
Chalermchatwilai, B., Poungparn, S., and Patanaponpaiboon, P. (2011). Distribution of fine-root necromass in a secondary mangrove forest in Trat Province. Eastern Thailand. Scienceasia 37, 1–5. doi: 10.2306/scienceasia1513-1874.2011.37.001
Chen, L., Wang, W., and Lin, P. (2004). Influence of water logging time on the growth of kandelia candel seedlings. Acta Oceanol. Sin. 1, 149–157. doi: 10.1029/2003JC001866
Chen, L., Zeng, X., Tam, N. F., Lu, W., Luo, Z., Du, X., et al. (2012). Comparing carbon sequestration and stand structure of monoculture and mixed mangrove plantations of Sonneratia caseolaris and S. apetala in Southern China. For. Ecol. Manag. 284, 222–229. doi: 10.1016/j.foreco.2012.06.058
Cheng, H., Wang, Y. S., Fei, J., Jiang, Z. Y., and Ye, Z. H. (2015). Differences in root aeration, iron plaque formation and waterlogging tolerance in six mangroves along a continues tidal gradient. Ecotoxicology 24, 1659–1667. doi: 10.1007/s10646-015-1474-0
Donato, D. C., Kauffman, J. B., Murdiyarso, D., Kumianto, S., Stidham, M., and Kanninen, M. (2011). Mangroves among the most carbon-rich forests in the tropics. Nat. Geosci. 4, 293–297. doi: 10.1038/ngeo1123
Dornbush, M. E., Isenhart, T. M., and Raich, J. W. (2002). Quantifying fine-root decomposition: an alternative to buried litterbags. Ecology 83, 2985–2990. doi: 10.2307/3071834
Eissenstat, D., and Yanai, R. (1997). The ecology of root lifespan. Adv. Ecol Res. 27, 1–60. doi: 10.1016/S0065-2504(08)60005-7
Fairley, R., and Alexander, I. J. (1985). “Methods of calculating fine root production in forests,” in Special Publication of The British Ecological Society, eds A. H. Fitter et al. (Oxford: Blackwell), 37–42.
Ha, T. H., Marchand, C., Aimé, J., Dang, H. N., Phan, N. H., Nguyen, X. T., et al. (2017). Belowground carbon sequestration in a mature planted mangroves (Northern Viet Nam). For. Ecol. Manag. 407, 191–199. doi: 10.1016/j.foreco.2017.06.057
He, Z., Peng, Y., Guan, D., Hu, Z., Chen, Y., and Lee, S. Y. (2018). Appearance can be deceptive: shrubby native mangrove species contributes more to soil carbon sequestration than fast-growing exotic species. Plant Soil 432, 425–436. doi: 10.1007/s11104-018-3821-4
He, Z., Sun, H., Peng, Y., Hu, Z., Cao, Y., and Lee, S. Y. (2020). Colonization by native species enhances the carbon storage capacity of exotic mangrove monocultures. Carbon Balance Manage. 15:28. doi: 10.1186/s13021-020-00165-0
Heiri, O., Lotter, A. F., and Lemcke, G. (2001). Loss on ignition as a method for estimating organic and carbonate content in sediments: reproducibility and comparability of results. J. Paleolimnol. 25, 101–110. doi: 10.1023/A:1008119611481
Hogarth, P. J. (2015). The Biology of Mangroves and Seagrasses, 3rd Edn. Oxford: Oxford University Press.
Huxham, M., Langat, J., Tamooh, F., Kennedy, H., Mencuccini, M., Skov, M. W., et al. (2010). Decomposition of mangrove roots: effects of location, nutrients, species identity and mix in a Kenyan Forest. Estuar. Coast Shelf Sci. 88, 135–142. doi: 10.1016/j.ecss.2010.03.021
Ibrahim, F., Adu-Bredu, S., Addo-Danso, S. D., Duah-Gyamfi, A., Manu, E. A., and Mahli, Y. (2020). Patterns and controls on fne-root dynamics along a rainfall gradient in Ghana. Trees 2, 1–13. doi: 10.1007/s00468-020-01970-3
Iversen, C. M., McCormack, M. L., Powell, A. S., Blackwood, C. B., Freschet, G. T., Kattge, J., et al. (2017). A global fine-root ecology database to address below-ground challenges in plant ecology. New Phytol. 215, 15–26. doi: 10.1111/nph.14486
Janzen, D. H. (1985). Mangroves: where’s the understory? J. Trop. Ecol. 1, 89–92. doi: 10.1017/S0266467400000122
Jiang, Z. M., Guan, W., Xiong, Y. M., Li, M., Chen, Y. J., and Liao, B. W. (2019). Interactive effects of intertidal elevation and light level on early growth of five mangrove species under Sonneratia apetala Buch. Ham. plantation canopy: turning monocultures to mixed forests. Forests 10, 83. doi: 10.3390/f10020083
Kelleway, J. J., Saintilan, N., Macreadie, P. I., Skilbeck, C. G., Zawadzki, A., and Ralph, P. J. (2016). Seventy years of continuous encroachment substantially increases ‘Blue Carbon’capacity as mangroves replace intertidal salt marshes. Glob. Chang. Biol. 22, 1097–1109. doi: 10.1111/gcb.13158
Lai, Z., Liu, J., Zhang, Y., Wu, B., Qin, S., Sun, Y., et al. (2017). Introducing a shrub species in a degraded steppe shifts fine root dynamics and soil organic carbon accumulations, in Northwest China. Ecol. Eng. 100, 277–285. doi: 10.1016/j.ecoleng.2017.01.001
Lee, S. Y., Hamilton, S., Barbier, E. B., Primavera, J., and Lewis, R. R. (2019). Better restoration policies are needed to conserve mangrove ecosystems. Nat. Ecol. Evol. 3, 870–872. doi: 10.1038/s41559-019-0861-y
Li, H., Hu, Y., Sun, Y., Silva, A. O. D., Muir, D. G. G., Wang, W., et al. (2019). Bioaccumulation and translocation of tetrabromobisphenol A and hexabromocyclododecanes in mangrove plants from a national nature reserve of Shenzhen City, South China. Environ. Int. 129, 239–246. doi: 10.1016/j.envint.2019.05.034
Liu, H. X., Ren, H., Hui, D. F., Wang, W. Q., Liao, B. W., and Cao, Q. X. (2014). Carbon stocks and potential carbon storage in the mangrove forests of China. J. Environ. Manage. 133, 86–93. doi: 10.1016/j.jenvman.2013.11.037
Liu, X., Xiong, Y., and Liao, B. (2017). Relative contributions of leaf litter and fine roots to soil organic matter accumulation in mangrove forests. Plant Soil 421, 493–503. doi: 10.1007/s11104-017-3477-5
Lovelock, C. E. (2008). Soil respiration and belowground carbon allocation in mangrove forests. Ecosystems 11, 342–354. doi: 10.2307/40296288
Manzoni, S., Trofymow, J. A., Jackson, R. B., and Porporato, A. (2010). Stoichiometric controls on carbon, nitrogen, and phosphorus dynamics in decomposing litter. Ecol. Monogr. 80, 89–106. doi: 10.1890/09-0179.1
McCormack, M. L., Dickie, I. A., Eissenstat, D. M., Fahey, T. J., Fernandez, C. W., Guo, D., et al. (2015b). Redefining fine roots improves understanding of below-ground contributions to terrestrial biosphere processes. New Phytol. 207, 505–518. doi: 10.1111/nph.13363
McCormack, M. L., Crisfield, E., Raczka, B., Schnekenburger, F., Eissenstat, D. M., and Smithwick, E. A. (2015a). Sensitivity of four ecological models to adjustments in fine root turnover rate. Ecol. Model. 297, 107–117. doi: 10.1016/j.ecolmodel.2014.11.013
Melillo, J. M., Aber, J. D., Linkins, A. E., Ricca, A., Fry, B., and Nadelhoffer, K. J. (1989). Carbon and nitrogen dynamics along the decay continuum: plant litter to soil organic matter. Plant Soil 115, 189–198. doi: 10.1007/BF02202587
Middleton, B., and McKee, K. (2001). Degradation of mangrove tissues and implications for peat formation in belizean island forests. J. Ecol. 89, 818–828. doi: 10.2307/3072155
Muhammad-Nor, S. M., Huxham, M., Salmon, Y., Duddy, S. J., Mazars-Simon, A., Mencuccini, M., et al. (2019). Exceptionally high mangrove root production rates in the Kelantan Delta, Malaysia; an experimental and comparative study. For. Ecol. Manag. 444, 214–224. doi: 10.1016/j.foreco.2019.04.026
Okello, J. A., Kairo, J. G., Dahdouh-Guebas, F., Beeckman, H., and Koedam, N. (2019). Mangrove trees survive partial sediment burial by developing new roots and adapting their root, branch and stem anatomy. Trees 34, 37–49. doi: 10.1007/s00468-019-01895-6
Ostonen, I., Lõhmus, K., and Pajuste, K. (2005). Fine root biomass, production and its proportion of NPP in a fertile middle-aged norway spruce forest: comparison of soil core and ingrowth core methods. For. Ecol. Manag. 212, 264–277. doi: 10.1016/j.foreco.2005.03.064
Ouyang, X., Lee, S. Y., and Connolly, R. M. (2017). Global patterns of root decomposition rates and the fate of root carbon production in mangroves and saltmarsh. Earth Sci. Rev. 166, 53–63. doi: 10.1016/j.earscirev.2017.01.004
Peng, Y., Diao, J., Zheng, M., Guan, D., Zhang, R., Chen, G., et al. (2016). Early growth adaptability of four mangrove species under the canopy of an introduced mangrove plantation: implications for restoration. For. Ecol. Manag. 373, 179–188. doi: 10.1016/j.foreco.2016.04.044
Poret, N., Twilley, R. R., Rivera-Monroy, V. H., and Coronado-Molina, C. (2007). Belowground decomposition of mangrove roots in Florida coastal Everglades. Estuaries Coast. 30, 491–496. doi: 10.1007/BF02819395
Poungparn, S., Charoenphonphakdi, T., Sangtiean, T., and Patanaponpaiboon, P. (2016). Fine root production in three zones of secondary mangrove forest in Eastern Thailand. Trees 30, 467–474. doi: 10.1007/s00468-015-1220-5
Poungparn, S., Komiyama, A., Umnouysin, S., Rodtassana, C., Sangtiean, T., Maknual, C., et al. (2020). Ten-year estimation of net primary productivity in mangrove forest under a tropical monsoon climate in esstern Thailand: significance of the temperature environment in the dry season. Forests 11:987. doi: 10.3390/f11090987
Pradisty, N. A., Amir, A. A., and Zimmer, M. (2021). Plant species- and stage-specific differences in microbial decay of mangrove leaf litter: the older the better? Oecologia 195, 843–858. doi: 10.1007/s00442-021-04865-3
Prieto, I., Birouste, M., Zamora-Ledezma, E., Gentit, A., Goldin, J., Volaire, F., et al. (2017). Decomposition rates of fine roots from three herbaceous perennial species: combined effect of root mixture composition and living plant community. Plant Soil 415, 359–372. doi: 10.1007/s11104-016-3163-z
Ren, H., Chen, H., Li, Z., and Han, W. (2010). Biomass accumulation and carbon storage of four different aged Sonneratia apetala plantations in Southern China. Plant Soil 327, 279–291. doi: 10.1007/s11104-009-0053-7
Ren, H., Lu, H., Shen, W., Huang, C., Guo, Q., Li, Z., et al. (2009). Sonneratia apetala Buch. Ham in the mangrove ecosystems of China: an invasive species or restoration species? Ecol. Eng. 35, 1243–1248. doi: 10.1016/j.ecoleng.2009.05.008
Robertson, A., and Dixon, P. (1993). Separating live and dead fine roots using colloidal silica: an example from mangrove forests. Plant Soil 157, 151–154. doi: 10.1007/BF00038759
Rolo, V., and Moreno, G. (2012). Interspecific competition induces asymmetrical rooting profile adjustments in shrub-encroached open oak woodlands. Trees 26, 997–1006. doi: 10.1007/s00468-012-0677-8
Sariyildiz, T. (2015). Effects of tree species and topography on fine and small root decomposition rates of three common tree species (Alnus glutinosa, Picea orientalis and Pinus sylvestris) in Turkey. For. Ecol. Manag. 335, 71–86. doi: 10.1016/j.foreco.2014.09.030
Schumacher, B. A. (2002). Methods for the Determination of Total Organic Carbon (toc) in Soils and Sediments. Las Vegas, NV: Ecological Risk Assessment Support Center, United States Environmental Protection Agency.
Silver, W. L., and Miya, R. K. (2001). Global patterns in root decomposition: comparisons of climate and litter quality effects. Oecologia 129, 407–419. doi: 10.1007/s004420100740
Solly, E. F., Schöning, I., Boch, S., Kandeler, E., Marhan, S., Michalzik, B., et al. (2014). Factors controlling decomposition rates of fine root litter in temperate forests and grasslands. Plant Soil 382, 203–218. doi: 10.1007/s11104-014-2151-4
Song, X., Li, Q., and Gu, H. (2017). Effect of nitrogen deposition and management practices on fine root decomposition in Moso Bamboo Plantations. Plant Soil 410, 207–215. doi: 10.1007/s11104-016-2997-8
Song, X., Zhou, G., Gu, H., and Qi, L. (2015). Management practices amplify the effects of n deposition on leaf litter decomposition of the Moso Bamboo Forest. Plant Soil 395, 391–400. doi: 10.1007/s11104-015-2578-2
Srikanth, S., Lum, S. K. Y., and Chen, Z. (2016). Mangrove root: adaptions and ecological importance. Trees 30, 451–465. doi: 10.1007/s00468-015-1233-0
Strand, A. E., Pritchard, S. G., McCormack, M. L., Davis, M. A., and Oren, R. (2008). Irreconcilable differences: fine-root life spans and soil carbon persistence. Science 319, 456–458. doi: 10.1126/science.1151382
Sun, L. F., Hirano, T., Yazaki, T., Teramoto, M., and Liang, N. S. (2020). Fine root dynamics and partitioning of root respiration into growth and maintenance components in cool temperate deciduous and evergreen forests. Plant Soil 446, 471–486. doi: 10.1007/s11104-019-04343-z
Tamooh, F., Huxham, M., Karachi, M., Mencuccini, M., Kairo, J., and Kirui, B. (2008). Below-Ground root yield and distribution in natural and replanted mangrove forests at Gazi Bay, Kenya. For. Ecol. Manag. 256, 1290–1297. doi: 10.1016/j.foreco.2008.06.026
Wang, D., Olatunji, O. A., and Xiao, J. L. (2019). Thinning increased fine root production, biomass, turnover rate and understory vegetation yield in a Chinese fir plantation. For. Ecol. Manag. 440, 92–100. doi: 10.1016/j.foreco.2019.03.012
Wang, W. J., Mo, Q. F., Han, X. G., Hui, D. F., and Shen, W. J. (2019). Fine root dynamics responses to nitrogen addition depend on root order, soil layer, and experimental duration in a subtropical forest. Biol. Fertil. Soils 55, 723–736. doi: 10.1007/s00374-019-01386-3
Ward, D., Wiegand, K., and Getzin, S. (2013). Walter’s two-layer hypothesis revisited: back to the roots! Oecologia 172, 617–630. doi: 10.1007/s00442-012-2538-y
Wu, M., He, Z., Fung, S., Cao, Y., Guan, D., Peng, Y., et al. (2020). Species choice in mangrove reforestation may influence the quantity and quality of long-term carbon sequestration and storage. Sci. Total Environ. 14:136742. doi: 10.1016/j.scitotenv.2020.136742
Xiong, Y., Liu, X., Guan, W., Liao, B., Chen, Y., Li, M., et al. (2017). Fine root functional group based estimates of fine root production and turnover rate in natural mangrove forests. Plant Soil 413, 83–95. doi: 10.1007/s11104-016-3082-z
Xuluc-Tolosa, F., Vester, H., Ramırez-Marcial, N., Castellanos-Albores, J., and Lawrence, D. (2003). Leaf litter decomposition of tree species in three successional phases of tropical dry secondary forest in Campeche, Mexico. For. Ecol. Manag. 174, 401–412. doi: 10.1016/S0378-1127(02)00059-2
Ye, Y., Tam, N. F., Wong, Y., and Lu, C. (2003). Growth and physiological responses of two mangrove species (Bruguiera gymnorrhiza and Kandelia candel) to waterlogging. Environ. Exp. Bot. 49, 209–221. doi: 10.1016/S0098-8472(02)00071-0
Yuan, Z., and Chen, H. Y. (2010). Fine root biomass, production, turnover rates, and nutrient contents in boreal forest ecosystems in relation to species, climate, fertility, and stand age: literature review and meta-analyses. Crit. Rev. Plant Sci. 29, 204–221. doi: 10.1080/07352689.2010.483579
Keywords: fine root dynamics, mangrove, monoculture, mixed plantation, organic carbon storage
Citation: He Z, Sun H, Yu X, Yin Z, Wu M, Zhao L, Hu Z, Peng Y and Lee SY (2021) Monoculture or Mixed Culture? Relevance of Fine Root Dynamics to Carbon Sequestration Oriented Mangrove Afforestation and Restoration. Front. Mar. Sci. 8:763922. doi: 10.3389/fmars.2021.763922
Received: 24 August 2021; Accepted: 27 September 2021;
Published: 20 October 2021.
Edited by:
Zhi-jun Dai, East China Normal University, ChinaReviewed by:
Jong Seong Khim, Seoul National University, South KoreaLuzhen Chen, Xiamen University, China
Copyright © 2021 He, Sun, Yu, Yin, Wu, Zhao, Hu, Peng and Lee. This is an open-access article distributed under the terms of the Creative Commons Attribution License (CC BY). The use, distribution or reproduction in other forums is permitted, provided the original author(s) and the copyright owner(s) are credited and that the original publication in this journal is cited, in accordance with accepted academic practice. No use, distribution or reproduction is permitted which does not comply with these terms.
*Correspondence: Zhan Hu, aHV6aDlAbWFpbC5zeXN1LmVkdS5jbg==; Yisheng Peng, cHlpc2hAbWFpbC5zeXN1LmVkdS5jbg==