- 1Marine Ecology, GEOMAR Helmholtz Centre for Ocean Research Kiel, Kiel, Germany
- 2Department of Ecology, Leibniz Centre for Tropical Marine Research, Bremen, Germany
- 3Institute for Chemistry and Biology of the Marine Environment, University of Oldenburg, Oldenburg, Germany
- 4Institute of Clinical Molecular Biology, Christian-Albrechts University Kiel, Kiel, Germany
- 5AUT Lab for Cephalopod Ecology & Systematics, School of Science, Auckland University of Technology, Auckland, New Zealand
- 6Institute for Marine Ecosystem and Fishery Science, University Hamburg, Hamburg, Germany
The deep sea is among the largest, most biologically diverse, yet least-explored ecosystems on Earth. Baseline information on deep-sea biodiversity is crucial for understanding ecosystem functioning and for detecting community changes. Here, we established a baseline of cephalopod community composition and distribution off Cabo Verde, an archipelago in the eastern tropical Atlantic. This baseline served to test the hypothesis that Cabo Verde is biogeographically separated from other Macaronesian archipelagos and allowed the identification of cephalopod species which may play a role in the Macaronesian carbon cycle and oceanic food web. To investigate cephalopod community composition, this study used 746 individual cephalopods obtained by nets (0–1000 m) and 52 cephalopod encounters during video surveys with either towed camera (0–2500 m) or manned submersible (0–375 m). Additionally, environmental DNA (eDNA) metabarcoding on 105 seawater samples (50–2500 m), using an 18S rRNA universal cephalopod primer pair, and a species-specific primer pair for Taningia danae resulted in the detection of 32 cephalopod taxa. When combined, the three methods detected a total of 87 taxa, including 47 distinct species. Each method contributed between 7 and 54% of taxa that were not detected by the other methods, indicating that multiple methodological approaches are needed for optimal deep-sea cephalopod biodiversity assessments. This study documents the occurrences of six species and three genera for the first time in waters surrounding Cabo Verde. Video surveys and eDNA analysis detected Taningia danae recurrently (100–2500 m). eDNA metabarcoding proved to be a powerful tool for cephalopod biodiversity monitoring and complementary to traditional sampling methods. When also including literature records, Cabo Verde hosts at least 102 cephalopod taxa including 30 families and 64 benthic and pelagic species. The total number and species composition of Cabo Verde cephalopods is similar to the Canary Islands and Azores, two known cephalopod biodiversity hotspots, but the Cabo Verde octopus fauna seems to differ. Due to a range of life history characteristics, we hypothesize that the squids Taningia danae (Octopoteuthidae) and Sthenoteuthis pteropus (Ommastrephidae) are important in the carbon cycle of Macaronesia. As a cephalopod biodiversity hotspot Cabo Verde could function as a model region to investigate cephalopod biology and ecology in a rapidly changing Atlantic Ocean.
Introduction
The deep sea (ocean depths below 200 m) is home to one of the most diverse communities on earth with the highest faunal biomass and greatest number of individual organisms (Robison, 2004; Ramirez-Llodra et al., 2010). The pelagic zone of the deep sea comprises 73% of the deep-sea water volume, yet despite this vast size, remains the least explored habitat with less than 0.0001% of its volume having been sampled (Herring, 2002; Ramirez-Llodra et al., 2010). Climate change and anthropogenic impacts are influencing the deep sea on a global scale (Keeling et al., 2010; Levin and Le Bris, 2015; Schmidtko et al., 2017; Breitburg et al., 2018) and altered environmental gradients in temperature and oxygen may cause changes in deep-sea species diversity and distribution (Robison, 2009). To preserve essential deep-sea ecosystem services both within and beyond exclusive economic zones, and to accurately evaluate the impacts of ocean changes, baseline information on deep-sea ecosystem functioning and biodiversity is needed (Robison, 2009; Thurber et al., 2014). This is particularly true for deep-sea cephalopods, abundant marine invertebrates that are known to respond rapidly to environmental change and play a pivotal role in the food web.
Cephalopods are marine mollusks that connect lower and higher trophic levels as both predators and prey (Clarke et al., 1996; Piatkowski et al., 2001). They occur from coastal areas to the deep sea and are typically predators, feeding on fish, crustaceans, other cephalopods and some species, also on gelatinous zooplankton (Hoving et al., 2014; Hoving and Haddock, 2017; Merten et al., 2017; Villanueva et al., 2017). A range of predators, e.g., cetaceans, tuna, swordfish and sharks hunt for cephalopods as their primary prey (Clarke, 1996a,b; Smale and Clarke, 1996). Most cephalopod species are semelparous, that is, after a single reproductive event, the individuals die and, depending on the species, either sink to the seafloor or float to the surface (Nesis et al., 1998; Boyle and Rodhouse, 2005; Hoving et al., 2015). Hence, post-spawning mortality events may result in localized pulses of carcasses, creating organically enriched patches contributing to regional carbon fluxes and providing scavengers with food (Stockton and DeLaca, 1982; Martin and Christiansen, 1997; Hoving et al., 2017). The biology, ecology and physiology of only 8% (∼60 species) of the 800 extant cephalopod species known today have been investigated in detail (Jereb and Roper, 2010), with these studies being biased towards commercially important or coastal species. Much less is known about the 45% of species comprising non-commercially exploited, open-ocean or deep-sea squids and octopuses (Sweeney and Roper, 1998).
In the Atlantic Ocean, 43 cephalopod families are known with 34 of these occurring exclusively in the open ocean (Rosa et al., 2008). The highest cephalopod species diversity in the northern hemisphere is found in the western North Atlantic (74 species). Lowest cephalopod diversities are found in polar regions (14 species in the Arctic Ocean, 22 species in the Antarctic Ocean) (Rosa et al., 2008). Known Atlantic hotspots near oceanic islands are the Canary Islands (83 cephalopod species, Escánez et al., 2020) and the Azores (83 cephalopod species, Pereira et al., 2016; Visser et al., 2021). Another potential hotspot of cephalopod biodiversity is the Cabo Verde archipelago in the eastern tropical Atlantic, but a comprehensive overview of cephalopod diversity is lacking for this region. The topography of Cabo Verde induces local upwellings and high biological productivity (Doty and Oguri, 1956; Gove et al., 2016). The islands accommodate a unusually high number of endemic species and an eclectic mix of temperate, subtropical and tropical taxa (Duda and Rolán, 2005; Sampaio et al., 2018; Freitas et al., 2019). Due to the islands’ steep slopes, deep-sea ecosystems occur within the exclusive economic zone of the archipelago of Cabo Verde. Historically, the archipelago has been included with the Azores, Madeira, Selvagens and the Canary Islands in the Macaronesian biogeographic region (Ávila, 2000, 2005; Borges et al., 2010; Wirtz et al., 2013; Cordeiro and Ávila, 2015; Freitas et al., 2019). However, the coastal and benthic composition of marine biota of Cabo Verde differ significantly from other Macaronesian archipelagos (Freitas et al., 2019), with the Cabo Verde front potentially functioning as a biogeographical barrier preventing the dispersal of marine organisms from and to other island groups in the North (Freitas et al., 2019). The North-West African Upwelling may form another biogeographical barrier between Cabo Verde and the African mainland limiting marine species dispersal by upwelling cold water to the surface (Türkay, 1982; Terashima et al., 2007; Wirtz, 2012; Freitas et al., 2019). To test the hypothesis that Cabo Verdes’ cephalopod fauna is distinct from other Macaronesian archipelagos, this study provides an overview of Cabo Verde cephalopod community composition combining novel data from seven expeditions, complemented by literature records.
The paucity of knowledge about deep-sea cephalopod biodiversity and distribution is in part due to the difficulties associated with sampling these organisms. Net sampling surveys are biased by gear characteristics such as mesh size or net mouth opening as well as deployment parameters (e.g., towing speed and depth) (Heino et al., 2011). Video surveys and in situ observations with remotely operated vehicles (ROVs) and towed camera systems have successfully documented certain cephalopod species throughout the water column and can also document a range of behaviors and ecological interactions (Bush et al., 2009; Hoving et al., 2017, 2019a; Robison et al., 2017; Vecchione, 2019). However, the light associated with underwater video surveys and the noise of hydraulic systems on ROVs may result in selective and species-specific documentation. As a general rule, net sampling and ROV surveys are expected to result in avoidance behavior and sampling bias in favor of species with reduced swimming capacity and less sensitive sensory capabilities (Clarke, 1977; Wormuth and Roper, 1983).
A cost-effective, non-invasive tool to study community compositions is the metabarcoding of environmental DNA (eDNA), i.e., the genetic material that organisms leave in the water. eDNA analysis is a rapidly advancing field and becoming widely used in: biomonitoring and conservation, establishing local biodiversity patterns, temporal dynamics in migration and detection of range expansions, as well as documenting rare and invasive species occurrences (Taberlet et al., 2012; Sigsgaard et al., 2017; Yamamoto et al., 2017; Cowart et al., 2018; Everett and Park, 2018; Jeunen et al., 2019; Laroche et al., 2020). For cephalopods, eDNA analysis has thus far been used in two field studies. The first study designed a species-specific eDNA primer and was able to detect the giant squid Architeuthis dux in Japanese waters (Wada et al., 2020). The second study used universal cephalopod primers to reconstruct cephalopod community composition in the foraging zones of deep hunting predators off the Azores, detecting 39 cephalopod taxa from 17 families from depths between 50 and 1600 m (Visser et al., 2021). The latter study showed that not all taxa can be detected with the general 18S primer being used. Therefore, a species-specific primer for specific elusive squid should additionally be developed. Here, we developed such a primer for Taningia danae (Octopoteuthidae), a large species that was encountered frequently during video surveys around Cabo Verde, but which was not detected with nets or the same universal 18S cephalopod primer used here.
Using a combination of optical, physical and genetic sampling as well as data from the literature, the aim of this study was to set a baseline for cephalopod community composition and vertical distribution off Cabo Verde. The community baseline could then be used to compare cephalopod community compositions from Cabo Verde with those of the Canary Islands and Azores to test the hypothesis that Cabo Verde’s cephalopod communities are distinct from Macaronesia. Additionally, such a diversity assessment will allow more accurate predictions to be made as to which cephalopod species may contribute substantially to the regional carbon cycle.
Materials and Methods
Cephalopod Surveys
Sampling
Net catches of cephalopods were obtained during the cruises POS320/2 (March 2005), MSM49 (November/December 2015) and WH383 (March/April 2015) off Cabo Verde at a total of 18 stations at depths between 0 and 1000 m (Figure 1). Cephalopods were caught during POS320/2 with either an Isaacs-Kidd midwater trawl (IKMT) with a 6 m2 net opening, 4 mm mesh size equipped with a flowmeter, a Hydro-Bios Multinet Maxi with a 0.5 m2 net opening and 500 μm mesh size between the surface and 250 m water depth, or an 80 feet bottom trawl (Kraus, 2005). Net sampling during MSM49 was conducted with two types of multiple opening/closing nets (MOCNESS) and an IKMT. The smaller MOCNESS had a net opening of 1 m2 opening (three nets with a mesh size of 2 mm and six nets with a mesh size of 335 μm) and the larger MOCNESS a net opening of 10 m2 (five nets, mesh size: 1.5 mm) and were deployed between the surface to 1000 m. The IKMT had a net opening of 7 m2 and ended in a cod end of 500 μm mesh size. It was deployed to a maximum depth of 500 m (Christiansen et al., 2016). During WH383 a pelagic trawl (‘Aalnetz’, Engel Netze, Bremerhaven, Germany) with a mouth opening of 16 × 30 m, length of 150 m including a multiple net opening-closing device, 260 meshes by 180 cm stretched mesh size at the front, a cod end 20 mm stretched mesh-opening and a 1.8 mm inlet sewn into last 1 m of cod end (Harrison, 1967) was used with a multisampler (Construction Services AS, Bergen, Norway; Engås et al., 1997) allowing depth-stratified sampling (Fock, 2015; Fock and Czudaj, 2019). During WH383 three strata (mean vertical extension of ca. 40 m) were trawled mostly during night, though once during daytime, at depths between 30 and 700 m in horizontal tows for 30 min per stratum with a mean speed of three knots (2.8–3.3 kn). During this cruise, night trawls took place at 10:00 pm local time, and the day-time trawl at 12:00 pm local time. Onboard, cephalopods were identified morphologically to the lowest taxonomic level possible (species, genus, or family), with whole specimens preserved in formalin as voucher. In addition, tissue samples from some specimens were collected and preserved in ethanol for barcoding and the genetic reference database used for eDNA metabarcoding.
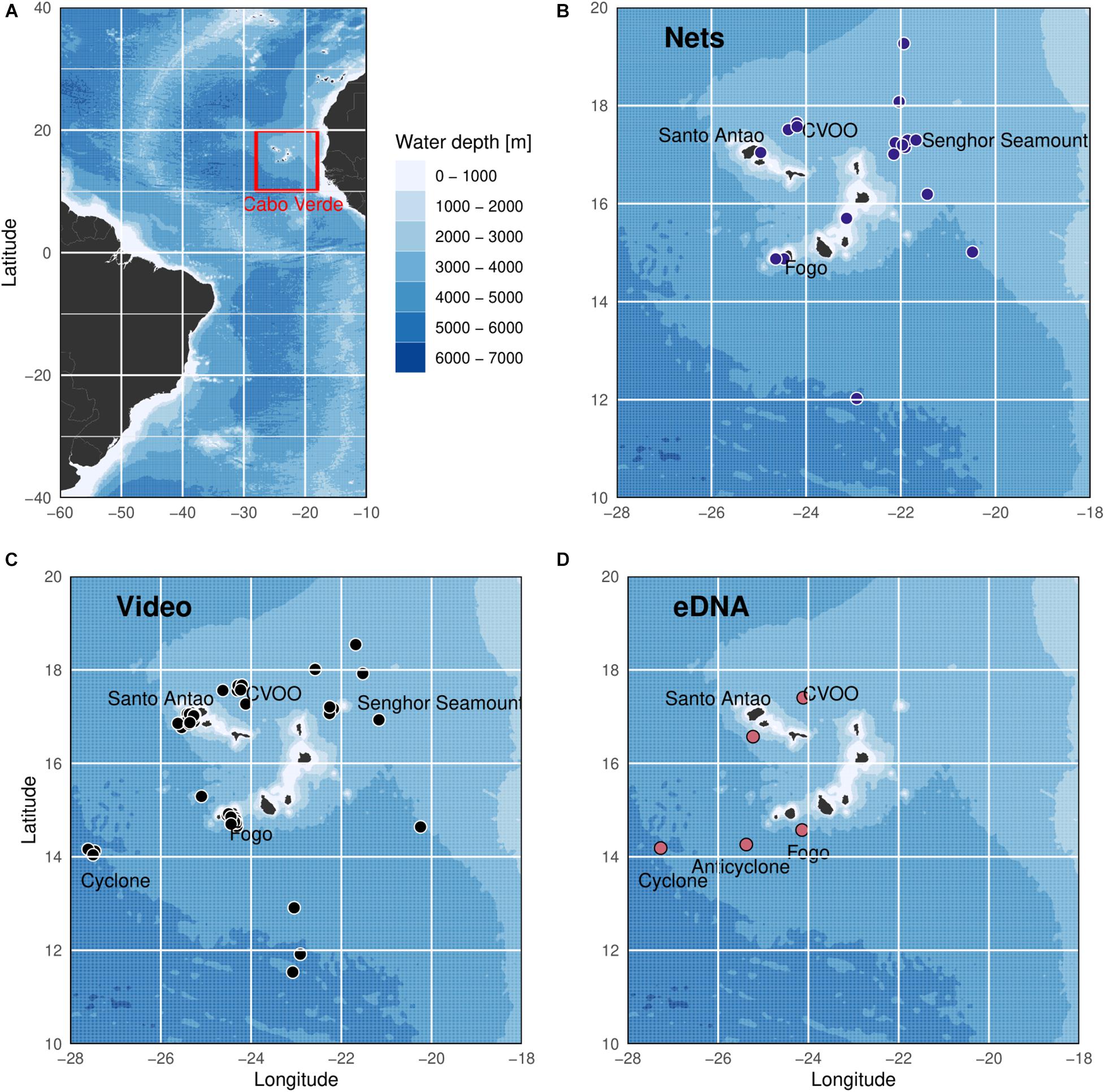
Figure 1. Sampling sites in the exclusive economic zone of Cabo Verde in the eastern tropical Atlantic. (A) Cabo Verde in the eastern tropical Atlantic. (B) Blue circles show the 18 net stations collecting cephalopods during the cruises POS320/2, MSM49 and WH383 between 2015 and 2018. (C) Black circles visualize the 40 video deployments conducted during the cruises MSM49, MSM61, POS520, POS532, and M119 between 2015 and 2019. (D) Red circles depict the five eDNA sampling stations collected during POS532 in 2019.
In situ Observations
Pelagic video transects with the Pelagic In-Situ Observation System (PELAGIOS, Hoving et al., 2019a) were conducted during the cruises MSM49 (Christiansen et al., 2016) (transects between 30 and 1000 m, total hours of observations > 80 h), MSM61 (Fiedler et al., 2020) (transects between 80 and 1200 m, total hours of observations > 32 h), POS520 (Hoving et al., 2018, p. 520) (transects between 30 and 2500 m, total hours of observations 27 h), POS532 (Hoving et al., 2019b) (transects between 30 and 990 m, total hours of observations 19 h) and M119 (Brandt, 2016) (transects between 50 and 700 m, total hours of observations > 20 h) between 2015 and 2019 (Figure 1). PELAGIOS is a battery powered, high-definition camera system that is towed horizontally via a single-wired conductive sea-cable at 0.5 m s –1. Approximately 0.45 m3 of the water column in front of the camera is illuminated with an LED array. The attached depth sensor and/or a sensor for conductivity, temperature and depth (CTD) with oxygen sensor allows for hydrographic measurements and depth monitoring during transects. Pelagic video transects lasted between 11 and 33 min per depth, towing the camera horizontally at specified depths. A deep-sea telemetry system allows for transmission of a low-resolution preview of the recorded video. Part of the data of the PELAGIOS transects collected during MSM49 are presented in Hoving et al. (2019a) with a focus on the methodology and using video from one station (Senghor NW) as an example for data analysis. The PELAGIOS cephalopod data from MSM49 was also analyzed with focus on gelatinous fauna in Hoving et al. (2020), but with no detailed taxonomic information on cephalopods presented, which is provided here. During the cruises POS520 and POS532 the manned submersible JAGO (GEOMAR, Helmholtz Centre for Ocean Research, Kiel, Hissmann and Schauer, 2017) was used for 30 deployments, each of approximately 4 h duration, between the surface and 375 m water depth. During the dives, video was recorded by a high-resolution camera (see details in Stenvers et al., 2021). The videos taken during the PELAGIOS and JAGO dives were annotated manually using the Video Annotation and Reference System (VARS) developed at the Monterey Bay Aquarium Research Institute (Schlining and Jacobsen, 2006), which allows annotation with the congruent collection of video frames.
eDNA Collection
A total of 105 eDNA samples were taken during POS532 in February 2019 from five stations (Hoving et al., 2019b; Figure 1). The stations off the islands Santo Antão and Fogo were close to the coast (maximum sampled depth 2500 m), CVOO (Cabo Verde Ocean Observatory) was an open ocean station (maximum sampled depth 2200 m) and the stations Cyclone and Anticyclone were located within eddies that had formed in the wake of Fogo and had propagated southwards (maximum sampled depths 2200 and 600 m, respectively) (Supplementary Figure 1). All stations described in this study were located inside the exclusive economic zone of Cabo Verde. Per sampled depth, three biological replicates, each of 2 l of seawater were collected from three different 10 l Niskin bottles mounted on a CTD rosette. For filtration, 0.22 μm pore size Sterivex-GP filter (Merck Millipore) were directly connected to the Niskin bottle with sterile tubing and two liter of seawater filtered per filter. Each of the replicates was filtered separately and included individually in the following analysis. The filters were closed with sterile plastic caps and stored at –80°C until further processing in the laboratory.
DNA Extraction
The DNA extraction protocol followed Visser et al. (2021). Briefly, DNA was extracted from the filters using the DNeasy Blood and Tissue Kit (Qiagen) with modified volumes. The DNA extractions were processed in sterile conditions under a clean bench to minimize contamination. After cleaning the outside of every filter with bleach, 720 μl of ATL-buffer and 80 μl of Proteinase K were added directly into the filter and incubated at 56°C for 2 h with agitation. After incubation, 600 μl of the buffer mix from the filter was transferred to a 2.0 ml Eppendorf tube and mixed with 600 μl AL-buffer and 600 μl 99% high grade ethanol. After this step, the normal DNeasy Blood and Tissue protocol was followed. DNA was eluted in 2 × 30 μl AE-buffer from the kit.
Rigorous laboratory measures were followed to reduce contamination. Only single-use consumables and single-capped PCR-tubes were used. DNA extractions, pre- and post-PCR were physically separated and sampling devices as well as laboratory equipment and surfaces cleaned with 50% bleach or RNAse AWAY (Carl Roth) containing bleach. To control for possible contaminations, negative controls were included at the filtration (MilliQ instead of seawater), DNA extraction (a blank filter was extracted and treated the same way as eDNA filter samples) and PCR (PCR-grade water instead of DNA sample) stages. As indicated below, positive controls were also included at the PCR stage.
Amplification and Sequencing of Cephalopod eDNA
A universal cephalopod primer targeting the nuclear 18S rRNA gene (Ceph18S_forward: CGCGGCGCTACATATTAGAC, Ceph18S_reverse: GCACTTAACCGACCGTCGAC, amplicon length = 140 – 190 bp, de Jonge et al., 2021) was used. The mitochondrial 16S rRNA locus (Jarman et al., 2006) was amplified as well following Visser et al. (2021). However, the positive controls on this sequencing run failed, so this data was excluded from further analysis.
For PCR amplification of the 18S rRNA gene, a 1-Step PCR protocol was used with the Illumina linker, Illumina index, Illumina adapter and a spacer for greater variability directly added to the primer sequence resulting in primer lengths of between 86 and 97 bp. All samples were amplified in duplicate resulting in six PCR products per sampling depth and site (three biological replicates × two PCR replicates) with individual Illumina tags for every replicate. Three positive controls (tissue derived DNA from Filippovia knipovitchi and Rossia palpebrosa, two cephalopod species that do not occur in the eastern Atlantic and one mock community with ∼10 ng/μl of each positive control species) and one negative control (PCR-grade water instead of DNA extract in the PCR reaction) were run in duplicate with individual taqs, and included on every PCR run.
The PCR reaction had a total volume of 25 μl and included 10 μl TaqMan Environmental Master Mix 2.0 (Applied Biosystems), 9 μl PCR-grade H2O, 0.5 μl forward primer (10 μM), 0.5 μl reverse primer (10 μM) and 5 μl DNA extract. A touchdown PCR program was applied starting with an initial denaturation step at 95°C for 5 min, 8 cycles of 94°C for 30 s, 70°C for 30 s (decreasing this temperature by 1°C after every cycle) and 72°C for 1 min. Subsequently, 32 additional cycles were run with 94°C for 30 s, 62°C for 30 s, and 72°C for 1 min. The program was terminated by a final extension step of 72°C for 5 min.
DNA fragment sizes were verified on a 1.5% agarose gel stained with GelRed (Biotum). The PCR products were measured using a Qubit fluorometer and then pooled in equimolar concentrations, resulting in one library. The fragment size of the library was validated on a 2% agarose gel stained with GelRed (Biotum). Subsequently, the correct band was cut out (band size between 300 and 380 bp) and purified using the Zymoclean Gel DNA Recovery Kit (Zymo Research) following the manufacturer’s protocol. The library pool was quantified with the Qubit dsDNA HS Assay Kit (Molecular Probes Life Technologies). The insert size distribution was determined with the Tapestation4200: D5000 ScreenTape (Agilent). The working solution was diluted to 2 nM and the loading solution was prepared according to the Agilent protocol. The library pools were loaded with 8 pM. To increase diversity, 20% PhiX was spiked in the library. Sequencing was done on an Illumina MiSeq with the MiSeq Reagent Kit v3, 600 cycles (PE), 2x 300 bp (Illumina) at the Institute of Clinical Molecular Biology (IKMB), Kiel, Germany.
Reference Database
As a reference for the obtained eDNA data, the 18S database described in Visser et al. (2021) was used. Briefly, cephalopod sequences from the SILVA 18S database were used to recursively search the NCBI Genbank database (accessed in June 2020) using a BLAST-based script from the EukRef project1 until no further cephalopod sequences were found. This resulted in 169 sequences from 119 species. These cephalopod sequences were combined with all other eukaryotic 18S sequences from the SILVA database to prevent spurious assignments of non-cephalopod amplicons. Additionally, 18S rRNA sequences from 16 tissue extracts of voucher specimens collected during two research cruises (MSM49, WH383) in the eastern tropical Atlantic and North Atlantic were sequenced and added to this database (GenBank accession numbers OK663489: OK663503). DNA was extracted from tissue samples using the DNeasy Blood and Tissue kit (Qiagen) following the manufacturers protocol. DNA concentrations were measured with the NanoDrop Spectrophotometer (Thermo Fisher) and amplified with the same primer used for eDNA metabarcoding, Ceph18S. For PCR amplification, we used the same parameters as for the touchdown PCR program outlined above. All PCR products were Sanger sequenced on a 3730xl DNA analyzer (Applied Biosystems) at the Institute of Clinical Molecular Biology (IKMB) in Kiel, Germany. Forward and reverse strand sequencing of the PCR products was performed using the Sanger Sequencing Kit (Applied Biosystems). Primers and low-quality ends were trimmed from the sequences, checked manually, edited, and assembled using CodonCode Aligner (version 3.7.1). Information on the construction of the reference database can be found in Visser et al. (2021) and resulted in a total of 34,811 sequences of which 169 originate from cephalopods representing 107 species for the 18S rRNA gene.
Bioinformatic Analysis
The raw sequences from the eDNA metabarcoding were demultiplexed by the sequencing center (IKMB) and forward and reverse PCR primer sequences were removed with cutadapt version 1.18 (Martin, 2011). The subsequent bioinformatic analysis was conducted with the R package DADA2 version 1.15.0 (Callahan et al., 2016, p. 2) in RStudio version 1.1.463 (R Core Team, 2018; RStudio Team., 2020). Paired reads were merged and trimmed at a quality score ≤ 2. The maximum expected error rate that was accepted was set to three for forward reads and five for reverse reads. Expected errors (EE) are calculated from EE = sum(10^(-Q/10)) with Q being the quality score. Potential chimeras were removed and an amplicon sequence variant table created. Taxonomic assignment of unique reads was performed using IDTAXA with the R package DECIPHER version 2.6.0 (Murali et al., 2018) using the databases described above as training set. Taxonomic assignments occurring in the negative controls and blanks were removed from the corresponding plates or batch of samples, respectively. After taxonomic assignments by IDTAXA, sequences that were assigned to only genus or family level were rechecked with applying the naïve Bayesian classifier method used by BLASTn in GenBank (Altschul et al., 1990). A hit by BLASTn was accepted to species level with a percent identity of at least 99% and no closer hit than 90% to another species.
Species-Specific Taningia danae Primer Design
The universal Ceph18S primer targeting the 18S rRNA gene used in this study could not discriminate reliably to species level within the family Octopoteuthidae (de Jonge et al., 2021). To obtain this information, a novel species-specific primer targeting the cytochrome oxidase I (COI) region of the mitochondrial gene of Taningia danae and amplifying a 250 bp segment was designed using Primer3 (Untergasser et al., 2012) (Taningia_COI_forward: TCATGCAGGTCCCTCTGTTG, Taningia_COI_reverse: AGGTGTTGGTATAAGATGGGGT, amplicon length = ∼250 bp). Sequences for designing the primer were obtained from the GenBank database (NCBI, Accession: AY393902.1, Accession: MG591434.1, Accession: EU735402.1). The specificity of the primer was checked by carrying out an in-silico PCR with ecoPCR by OBITools (Ficetola et al., 2010). The in silico results showed amplification of T. danae, but also 15 other species, mostly belonging to Gonatidae, that do not occur in Cabo Verdean waters. Subsequently, the specificity of the primer was tested on DNA samples derived from tissue from seven cephalopod species (Bathypolypus sp., Megaleledone sp., Gonatus sp., Rossia sp., Brachioteuthis sp., Pareledone felix) and T. danae and sequenced using the Sanger Sequencing Kit (Applied Biosystems). The DNA was extracted with the DNeasy Blood and Tissue Kit (Qiagen) according to manufacturer’s protocol and diluted in 100 μl TE-Buffer.
Analysis of eDNA Samples With Taningia danae Primer
A subset of eDNA seawater samples from four stations (Santo Antão, Fogo, CVOO, Cyclone) were re-analyzed with the species-specific T. danae primer developed for this study, along with the above-mentioned universal cephalopod primer. All biological replicates were pooled and analyzed together with a positive (DNA extract of T. danae as a template) and a negative (PCR-grade water instead of DNA template) control, amplified via PCR in triplicate to increase the detection probability, which resulted in a total of 16 samples. Each PCR mixture contained 10 μl TaqMan Environmental Master Mix 2.0 (Applied Biosystems), 7 μl PCR-grade water, 0.5 μl dsDNase (ArcticZymes Technologies), 0.5 μl DTT Inactivation Buffer (ArcticZymes Technologies), 1 μl forward primer (10 μM), and 1 μl reverse primer (10 μM). The PCR-Mix was incubated for 20 min at 37°C to reduce contaminating DNA, followed by 20 min at 60°C for DNAse deactivation. After incubation, 5 μl DNA template was added to the PCR-mix and run with the following program: Initial denaturation step for 10 min at 95°C, 30 cycles with 94°C for 30 s, an annealing temperature of 60°C for 30 s and 72°C for 1 min, followed by a final elongation step of 72°C for 5 min.
All PCR products were examined on a 1.5% Agarose gel stained with GelRed (Biotum). Only samples showing a band on the gel were sequenced using the Sanger Sequencing Kit (Applied Biosystems) at IKMT. Sequences were processed with CodonCode Aligner v. 3.7.1.2. The forward and reverse primer sequences were cut off and forward and reverse reads assembled. All sequences were run against the NCBI database (22/01/2021) using BLASTn (Altschul et al., 1990). Only hits with a percent identity > 96% and e-value below 1e-80 were accepted as positive detections for Taningia danae (Supplementary Table 1).
Statistical Analysis
A species accumulation curve (SAC) for the number of sampled sites was calculated only for eDNA using specaccum from the R package vegan (Oksanen et al., 2019). The method random was used calculating the mean SAC and its standard deviation from 100 random permutations of the data (Gotelli and Colwell, 2001). The Jaccard index, a measure of community dissimilarity, was calculated with the function vegdist (Oksanen et al., 2019) to measure similarity between the Canary Islands, Cabo Verde and Azores for all cephalopod taxa and for taxa belonging to the order Octopoda based on presence/absence data.
Biodiversity Analysis
To compare cephalopod taxa occurring in the waters around Cabo Verde, the Canary Islands and the Azores, an extensive literature research was conducted. For the Cabo Verde islands, the species list generated in this study incorporated observations from three studies describing cephalopods in Cabo Verdean waters (Voss et al., 1998; Collins et al., 2001; Clarke, 2006) and our data. For the Azores, the species list from Visser et al. (2021) was used as it included all currently available data from both the literature and eDNA. For the Canary Islands, a recent review on cephalopod diversity off the Canary Islands was used, which included species from 48 published documents (Escánez et al., 2020) following the authors’ exclusion of species that were only hypothesized to occur off the Canary Islands due to their wide geographical distribution, but have not as yet been positively detected there.
Results
Community Composition and Distribution of Cephalopods Off Cabo Verde
Net Catches
Trawling in the Cabo Verde exclusive economic zone (EEZ) during three research cruises in 2005 (POS320) and 2015 (MSM49 and WH383) resulted in the collection of 746 specimens belonging to 69 taxa (Supplementary Table 1, observations that could only be identified to the level Cephalopoda (n = 1) or Teuthida (n = 9) were excluded from further analysis due to low taxonomic resolution, all included taxa were identified to either species, genus or family level). Of the 69 taxa, 41 could be identified to species level, with these belonging to 20 families. The most frequently captured taxon (in terms of number of individuals collected) was Liocranchia reinhardtii (Cranchiidae, 25%, n = 184, Supplementary Table 2), which occurred over the whole sampled depth range from the surface to 1000 m (Figure 2). The next most frequently captured species’ were Pterygioteuthis gemmata (Pyroteuthidae, 12%, n = 93) occurring between 0 and 1000 m and Abraliopsis atlantica (Enoploteuthidae, 9%, n = 65), which was captured from 0 to 1000 m (Figure 2 and Supplementary Table 2). All other taxa represented less than 6% (n < 41) in terms of frequency. Twenty-two taxa were only observed once, e.g., Ornithoteuthis antillarum, Ommastrephes bartramii, and Taonius pavo. The most frequently encountered family in the catches was the Cranchiidae with 43% (n = 318), to which the most abundant species collected, Liocranchia reinhardtii belonged. Pyroteuthidae made up 17.2% (n = 128) of the total captured cephalopods followed by Enoploteuthidae with 16.8% (n = 125). All other families made up less than 4% (n < 30). The Cranchiidae was also the family with the highest species diversity collected (16 taxa, 11 could be identified to species level) followed by Enoploteuthidae (eight taxa, five could be identified to species level). All other families were represented by fewer than seven taxa. Families represented by only one taxon were Argonautidae, Bathyteuthidae, Lepidoteuthidae, Lycoteuthidae, Pholidoteuthidae, Sepiidae, Sepiolidae, and Vampyroteuthidae (Table 1). Taningia danae was not captured.
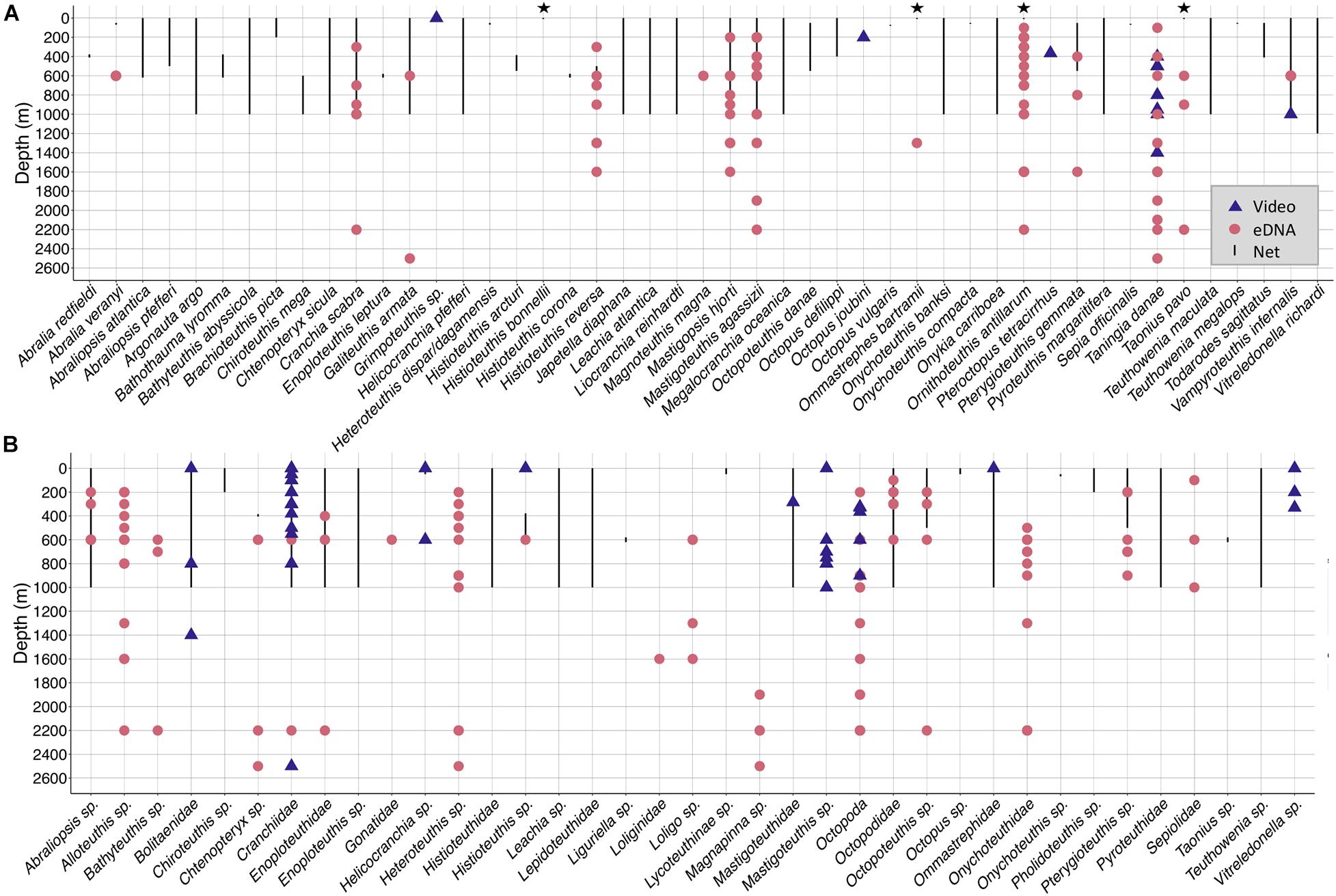
Figure 2. Depth distribution of taxa detected with eDNA, net catches and video surveys conducted off Cabo Verde in the eastern tropical Atlantic. Red dots depict eDNA detections, blue triangles video observations and black bars the depth distribution found in net catches with opening/closing nets. Black stars are cephalopods that fell out of the nets, so that the corresponding depth can not be assigned. Some taxa were observed in videos during down- or upcasts, in this case, the maximum depth of the down- or upcast was used. The taxa Cephalopoda and Teuthida were excluded from this graph. Many net tows were conducted with open nets from 1000 m to the surface, making it impossible to define at which specific depth the cephalopod individual was captured. Those tows are defined by the solid bars spanning from 0 to 1000 m. (A) Depth distribution of all species caught by nets, observed by video surveys and detected with eDNA metabarcoding off Cabo Verde. (B) Depth distribution of all genera, families and one order detected with nets, video surveys and eDNA metabarcoding off Cabo Verde.
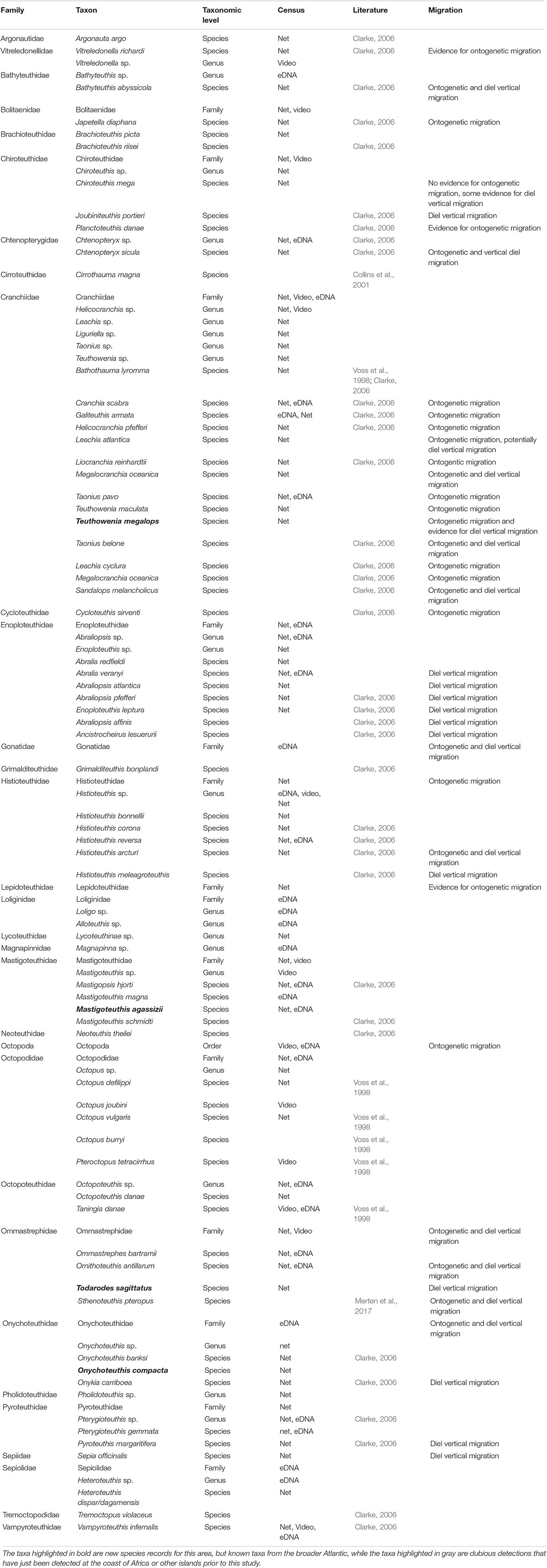
Table 1. Species list of detected cephalopod species off Cabo Verde in the eastern tropical Atlantic compiled by the eDNA approach, net trawls, and video surveys from this study combined with literature data.
Pelagic Video Surveys
During five research cruises between 2015 and 2019, 52 cephalopod individuals were observed (excluding observations that could only be identified to Teuthida, n = 162 or Cephalopoda, n = 2, all taxa included were identified to species, genus or family level) with either PELAGIOS or JAGO (Supplementary Table 3). Ninety percent (n = 47) of individuals were observed with PELAGIOS and 10% with JAGO (n = 5). Most individuals could not be identified to species or genus level, because the video footage did not reveal sufficient taxonomic characteristics. In total, 14 taxa were identified as belonging to one order (Octopoda), four families (Cranchiidae, Mastigoteuthidae, Ommastrephidae, Bolitaenidae), five genera (Mastigoteuthis, Helicocranchia, Histioteuthis, Grimpoteuthis, and Vitreledonella) and four species (Taningia danae, Octopus joubini, Pteroctopus tetracirrhus, and Vampyroteuthis infernalis). Most cephalopods observed in situ belonged to the family Cranchiidae with 32% of individuals (n = 17) and occurred between 50 and 2500 m (Figure 2). Bolitaenidae was the second most observed family, with 6% of individuals (n = 3), occurring below 800 m to depths of up to 1400 m. The most frequently observed cephalopod which could be identified to species level was T. danae with seven observations (12%) at depths of between 400 and 1400 m (Figure 2). The observations of T. danae differed from the other cephalopod observations since T. danae seemed to be attracted to the lights of the towed camera system (Figure 3). Individuals came very close to the camera and distinct taxonomic features were visible including the large photophores on the arms (II), the purple body color and hooks on the arms. Individuals approached the camera system, some even charged it and then swam away. We observed some individuals “flashing” their arm photophores by opening and closing the covering tissue over the photophores. Octopoda (n = 5) were detected between 323 and 900 m, including the benthic Atlantic pygmy octopus Octopus joubini at 200 m and Pteroctopus tetracirrhus at 365 m (Figure 2). Both these species were detected with JAGO.
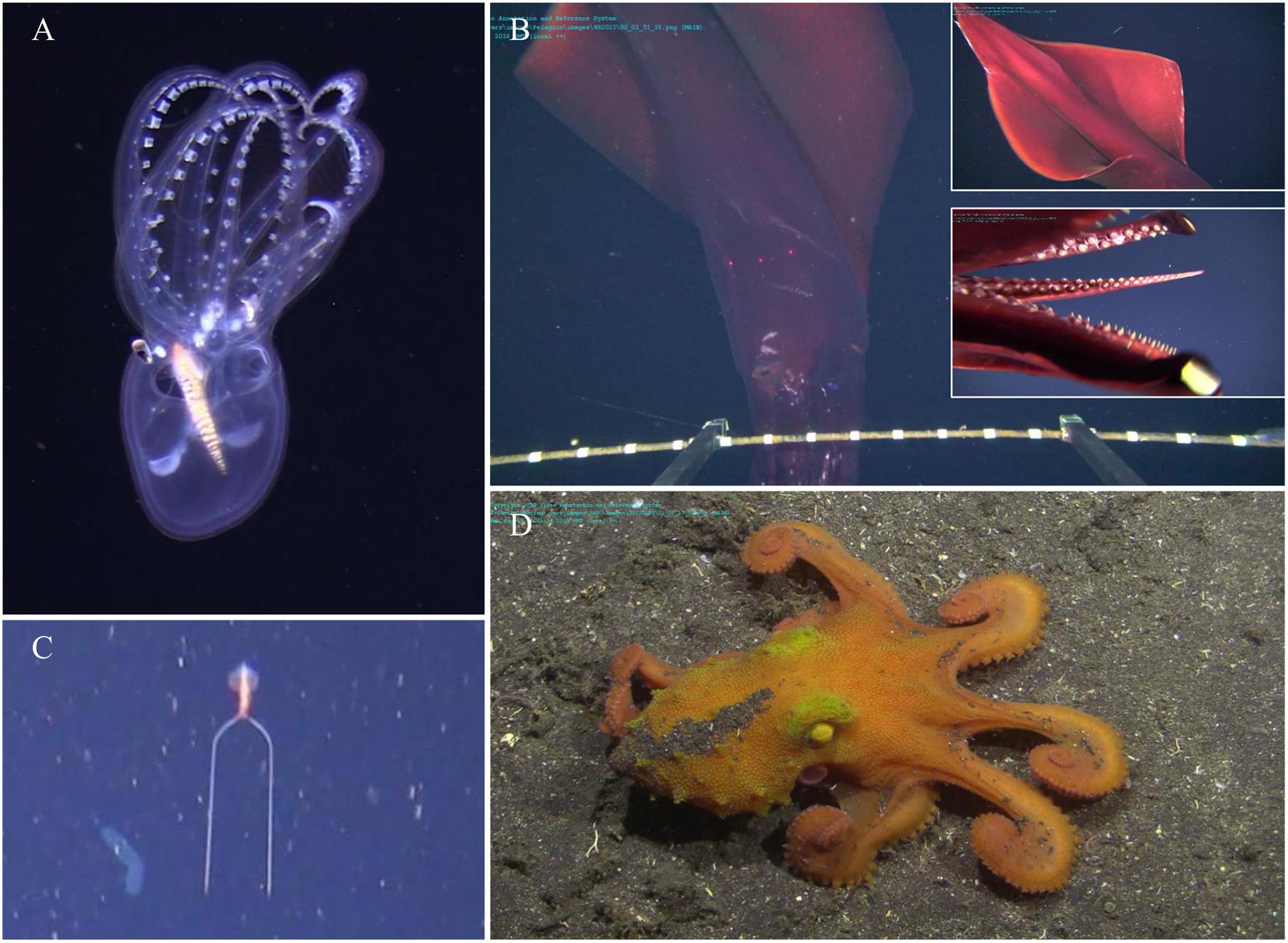
Figure 3. Pictures of cephalopods detected with JAGO and PELAGIOS off Cabo Verde in the eastern tropical Atlantic. (A) The glass octopus Vitreledonella sp. between 300 and 330 m photographed with the manned submersible JAGO during the cruise POS532. (B) The octopoteuthid Taningia danae approaching the towed underwater camera-system PELAGIOS at 600 m during the cruise MSM49. The small pictures are also T. danae during the cruise M119 (lower right) and POS520 (upper right) with unknown depths. (C) Mastigoteuthis sp. detected with PELAGIOS during the cruise POS520 at 285 m. (D) The Atlantic fourhorn octopus Pteroctopus tetracirrhus at 365 m detected with JAGO during POS532.
Environmental DNA Metabarcoding
A total of 14,130,249 sequences were obtained after sequencing with a mean (±standard deviation [SD]) of 53,523 (± 48,799) reads per sample. The number of reads in the negative controls ranged between 11 and 3,693 excluding the contamination of the positive controls, which amounted to 37% of the contamination in the negative controls. After the DADA2 analysis, 2,897,366 sequences remained that were classified into 908 unique amplicon sequence variants (ASVs) with a mean (±SD) of 196 (±2233) reads per ASV (range 1 – 96,089 reads). A conservative approach was applied for data cleaning and all taxa present in the negative controls deleted from the corresponding samples. In detail, all taxa present in a negative control (filtration negative control, DNA extraction negative control and/or PCR negative control) were removed from the samples that were either processed in the same filtration and/or DNA extraction step or run on the same PCR plate as the negative control. After deleting rare ASVs with a frequency below 10 reads per sample and excluding contaminants from the negative controls as well as removing of the positive controls, 78 ASVs remained (Table 2). The remaining ASVs ranged between 11 and 4,035 reads with a mean (±SD) of 102 (±294) reads. The number of reads per sample ranged between 132 and 59,494 with a mean (±SD) of 15,069 (±11,337).
Environmental DNA analysis of seawater samples from POS532 in 2019 resulted in the detection of 32 taxa (Figure 2). Thirty-eight percent of the detections could be identified to species level (n = 12), 31% to genus (n = 10), 22% to family (n = 7), 6% to order (n = 2), and 3% to class (n = 1). ASVs that were only assigned to the taxa Cephalopoda or Teuthida and not further were excluded from the following analyses due to their low taxonomic resolution, which resulted in the detection of 30 taxa in total with eDNA. All included taxa were assigned to species, genus or family level. Species or genera that were found with eDNA, but are not known to occur in the eastern tropical Atlantic Ocean were reduced to the next lower taxonomic level known from the Atlantic. This approach was applied to Doryteuthis pealeii (western Atlantic species), which was assigned to Loliginidae, Heteroteuthis hawaiiensis (Pacific species) which was assigned to Heteroteuthis sp. and Pterygioteuthis microlampas (Pacific species) which was assigned to Pterygioteuthis sp. Pterygioteuthis microlampas and H. hawaiiensis are morphologically very similar to their sister species P. gemmata and H. dispar, respectively, both of which occur in the Atlantic (Lindgren, 2010; Judkins et al., 2016). All four species were underrepresented in the database here used (just one reference sequence included) and were therefore potentially difficult to differentiate by the used marker gene.
Ornithoteuthis antillarum (Ommastrephidae) was the most frequently detected taxon (47% detections) with eDNA analysis, followed by Octopoda (34%, not including octopus taxa identified to genus or species level), Alloteuthis sp. (34%, Loliginidae), Mastigoteuthis agassizii (31%, Mastigoteuthidae) and Heteroteuthis sp. (31%, Heteroteuthidae) (Supplementary Figure 2). All other taxa were found in less than 30% of the samples. Considering the frequency of detections (detections/total samples analyzed ∗ 100%) the most commonly detected families with eDNA were Mastigoteuthidae (14%), Ommastrephidae (11%) and Loliginidae (10%).
The depth distribution of the eDNA data of all taxa detected was consistent with the known depth distribution patterns of the cephalopods that were detected (Figure 2). Exceptions were the occurrence of shallow and midwater species (Chtenopteryx sp., Loliginidae, Loligo sp., Alloteuthis sp. and O. antillarum) in deep water (below 1000 m). Most of the taxa (69%, n = 22) were detected throughout the water column from shallow to deep depths, whereas 25% of the detected taxa only occurred in the upper 1000 m, including Abralia veranyi, Abraliopsis sp., Histioteuthis sp., Magnoteuthis magna, Octopodidae, Pterygioteuthis sp., Sepiolidae and Vampyroteuthis infernalis. Six percent of the taxa were only detected below 1000 m (Ommastrephes bartramii at 1300 m and Magnapinna sp. between 1900 and 2500 m).
The number of taxa per surveyed depth ranged between one and 26. The highest number of taxa was detected at depths of 600 (26 taxa) and 2200 m (15 taxa, Supplementary Figure 3). Twenty-nine taxa were detected in the upper 1000 m and 23 taxa below 1000 m. When considering species only, the highest number of species (n = 10 species) was found at 600 m, followed by five species at 900, 1000, 1300, 1600, and 2200 m (Supplementary Figure 3). However, these results should be interpreted with caution since the sampling effort at different depths was imbalanced, ranging from one to four sampled stations at each depth. On the other hand, the distribution of cephalopod eDNA off Santo Antão and Fogo, two of the stations with the most sampled depths, and with sampled depths between the two stations being identical, showed a similar trend in the upper 1000 m with the highest number of species at 600 m (17 taxa). Below 1000 m, highest numbers of species were detected at 1600 m with nine species (Supplementary Figure 4).
The Taningia danae primer successfully amplified T. danae tissue samples to species level (>98% sequence match in Genbank). All matches ranged between 96 and 99% identity and the next species hit was below 90% identity (Supplementary Table 4). The six test cephalopod species (Bathypolypus sp., Megaleledone sp., Gonatus sp., Rossia sp., Brachioteuthis sp., Pareledone felix) were not amplified by the species-specific T. danae primer. Taningia danae eDNA was detected off Fogo, Santo Antão and the open ocean station CVOO between 100 and 2500 m depth (Supplementary Table 4). Only the negative control and two eDNA samples (Fogo at 400 m and Santo Antão at 200 m) yielded no amplification.
Cephalopod Diversity Off Cabo Verde: eDNA, Nets, Video, and Literature Combined
Nets, video surveys and eDNA were able to detect 69, 14, and 30 cephalopod taxa off Cabo Verde, respectively. Three taxa (Cranchiidae, Histioteuthis sp. and Vampyroteuthis infernalis) were identified by all three methods, 15 taxa by eDNA and nets only, four taxa by nets and video only and one taxon by eDNA and video only (Figure 4A, excluding Cephalopoda and Teuthida). However, it is important to point out that the different methods were used in different areas, seasons and years, with the exception of data collected during specific cruises (e.g., PELAGIOS observations and MOCNESS net catches during MSM49; eDNA, PELAGIOS, and JAGO during POS532). Therefore, a direct comparison between the three methods across the full data set described here is not possible. The video surveys were able to detect six taxa that were not detected by any other census used in this study, whereas eDNA was able to detect 11 additional taxa and nets 47 additional taxa. The taxonomic resolution was highest in net catches with 61% of detected taxa being identified to species level, 21% to genus and 15% to family. In comparison, eDNA was able to detect 38% to species, 31% to genus and 22% to family level (Figures 4B,C). The SAC for the eDNA approach showed a flattening of the curve and a decrease in standard deviation after sampling five stations, however, the curve did not reach the asymptote (Figure 5). Video surveys identified 27% to species, 40% to genus and 27% to family level, but from the video data fewer taxa were determined than the other censuses (Figures 4B,C). Net catches seemed to be the most efficient sampling technique with 69 identified cephalopod taxa compared to 30 and 14 taxa for eDNA and video, respectively. However, the net data included three cruises, while the eDNA data is only originating from one cruise. When comparing the eDNA data to the three net sampling cruises individually (Figure 6), the predominance of nets over eDNA is no longer so obvious. The overlap in detected species between eDNA and nets for all cruises ranged between 9 and 20%, while eDNA contributed 20 – 43% of additional species knowledge to species detected with nets. However, the eDNA data was obtained during a different cruise than the net data, therefore, a direct comparison can be misleading.
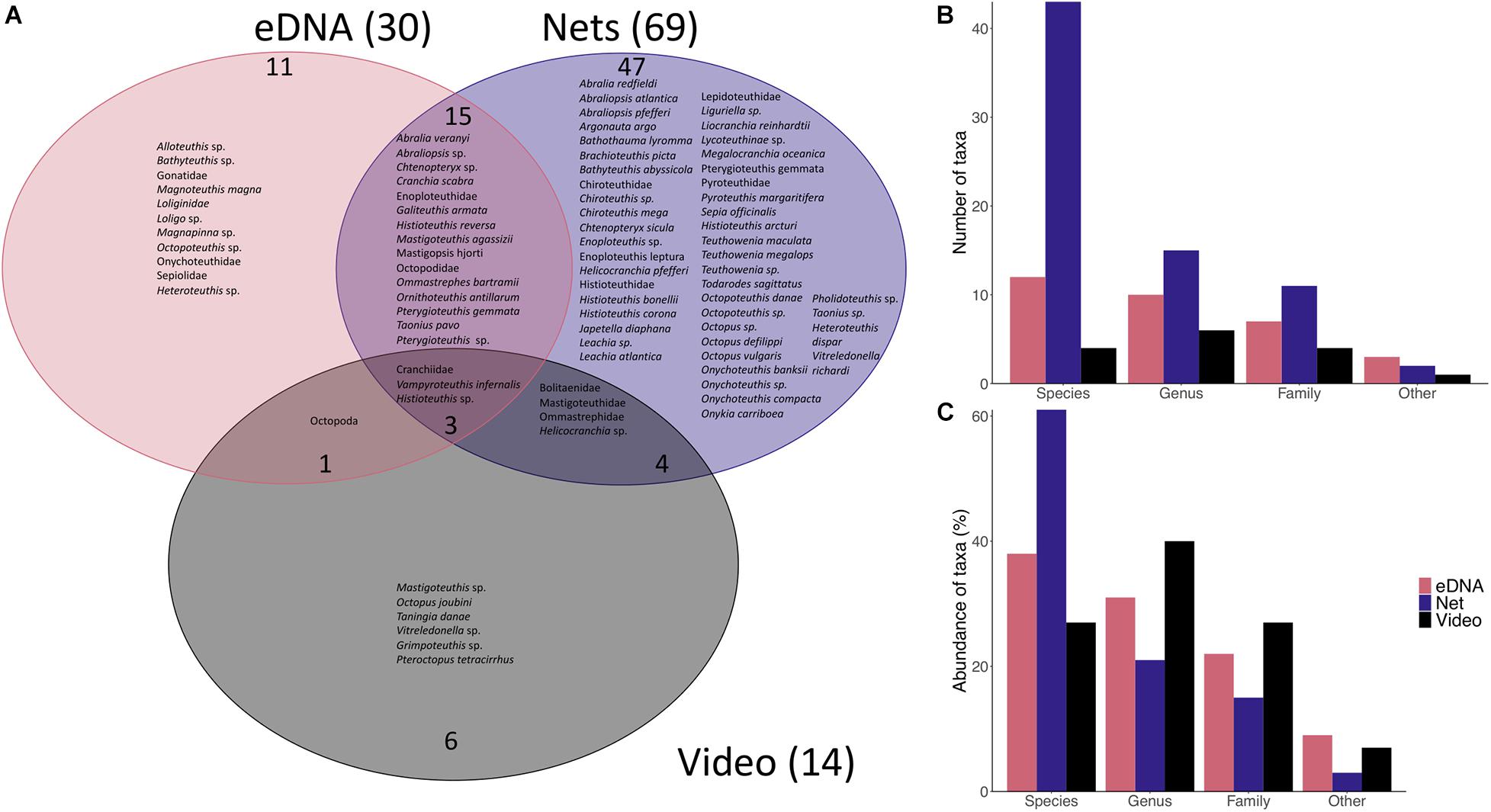
Figure 4. Comparison between taxa found with eDNA, net catches and video surveys off Cabo Verde. (A) Venn diagram showing the taxa detected with eDNA (30 in total), net catches (69 in total) and video surveys (14 in total) and their overlap (excluding Cephalopda and Teuthida). (B) Number of taxa that could be identified to species, genus, family or any other taxonomic level of the eDNA approach, net catches and video surveys. (C) Percentage of taxa that could be identified to species, genus, family or any other taxonomic level with the eDNA approach, net catches and video surveys.
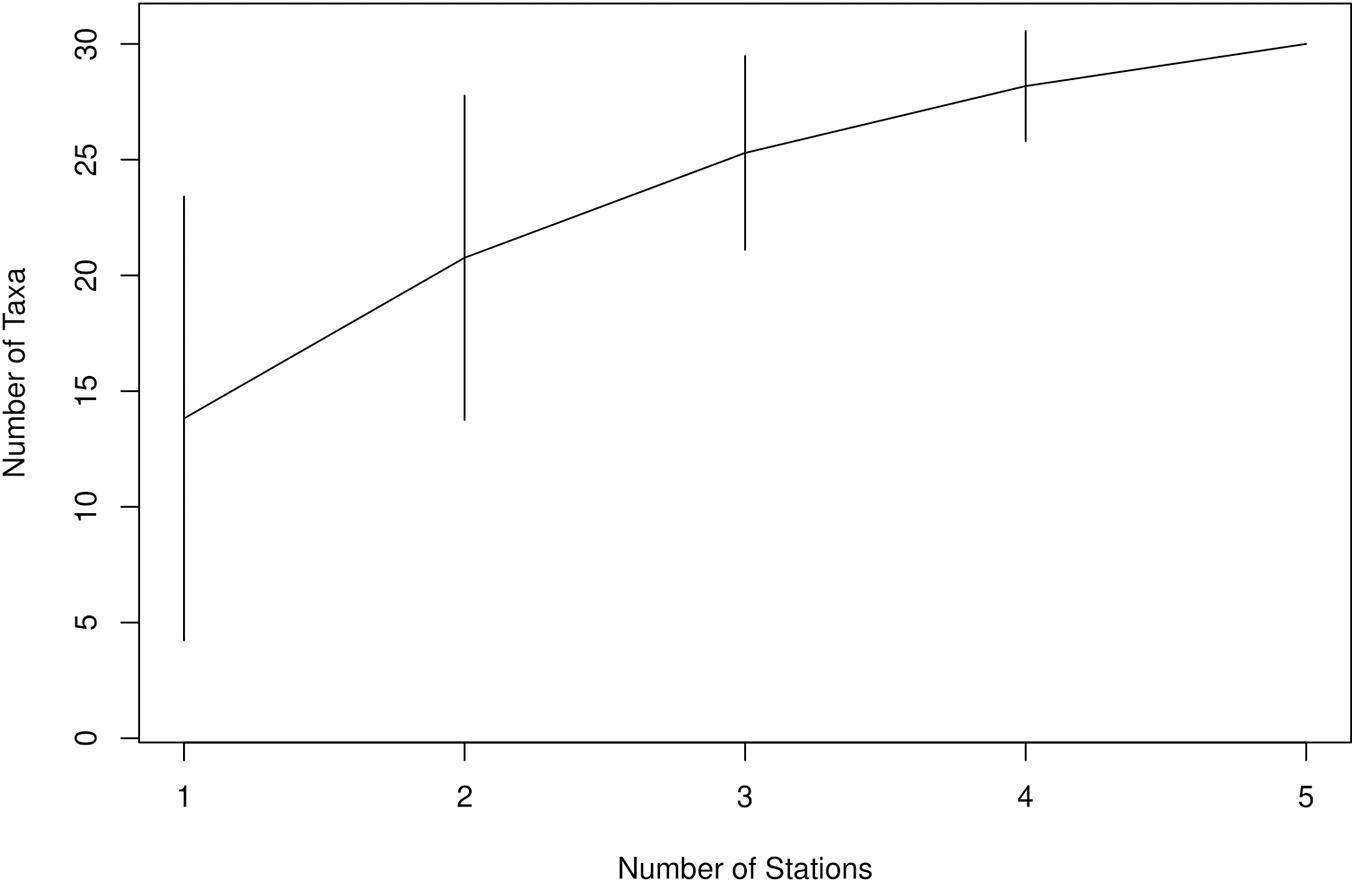
Figure 5. Species accumulation curve derived from the numbers of taxa for a certain number of sampled sites for stations sampled for eDNA metabarcoding. Error bars represent the standard deviation.
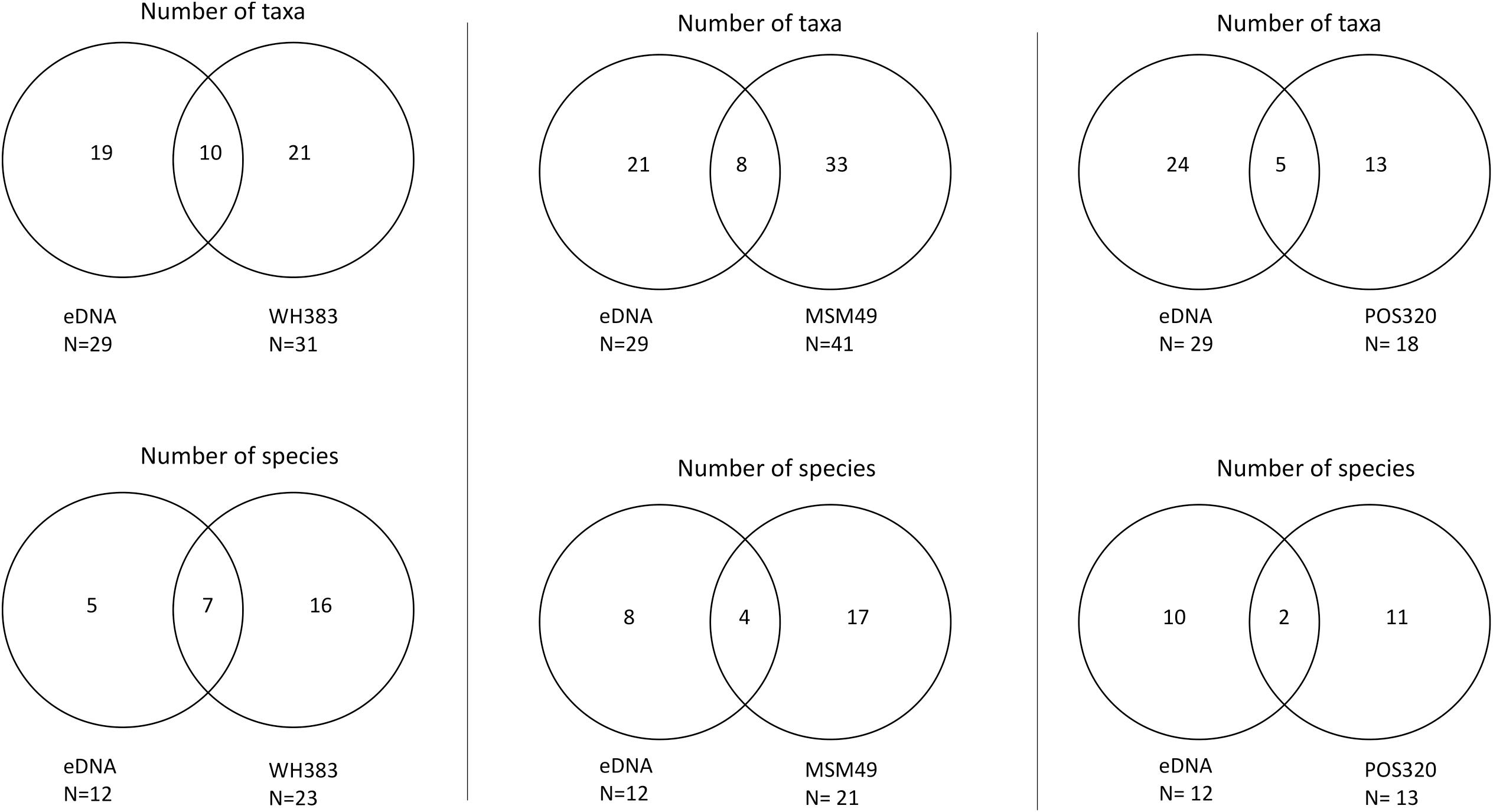
Figure 6. Comparison of eDNA data with each of the three cruises (WH383, MSM49, POS320) collecting cephalopods with net trawls. The upper Venn diagrams show the number of taxa per method and cruise. The lower Venn diagrams show the number of species per method and cruise.
The three censuses combined detected three new genera and six new species records for Cabo Verde waters including Teuthowenia megalops, Todarodes sagittatus, Onychoteuthis compacta, Mastigopsis hjorti, Magnoteuthis magna, Mastigoteuthis agassizii, Loligo sp., Alloteuthis sp., and Magnapinna sp. These taxa are known from the Atlantic Ocean, but had not been recorded around Cabo Verde prior to the current study (Jereb and Roper, 2010). Combining the results of all censuses applied here and the literature review (Voss et al., 1998; Collins et al., 2001; Clarke, 2006) resulted in the detection of 102 taxa of which 64 were identified to species level (Table 1).
Cephalopod Composition Off Cabo Verde in Comparison With the Azores and Canary Islands
For the Canary Islands, Cabo Verde and Azores 83, 64, and 83 cephalopod species have been reported to date, belonging to 31, 30, and 36 families, respectively (Figure 7). For all three archipelagos, the Cranchiidae was the family with the highest number of species (21 for Cabo Verde and eleven for the Canary Islands and Azores). The second most speciose family for Cabo Verde and the Azores was the Enoploteuthidae (ten and eight species, respectively). For the Canary Islands, Octopodidae, Histioteuthidae, and Enoploteuthidae were the second most diverse families with six species each. Thirty-eight species were found to occur in the EEZ of all three archipelagos. The Canary Islands overlapped with five and 17 species with Cabo Verde and the Azores, respectively, whereas Cabo Verde and the Azores shared seven cephalopod species (Figure 7). Cephalopods that only occurred off one island group included 23 species off the Canary Islands, 21 species off the Azores and 14 species off Cabo Verde. The overlapping octopus community composition between Canary Islands, Azores and Cabo Verde consisted of four pelagic species, in addition to Octopus vulgaris (Figure 8) which is benthic. Four octopus species only occurred off Cabo Verde, whereas the Azores harbored six and the Canary Islands five species that do not occur at the other archipelagos.
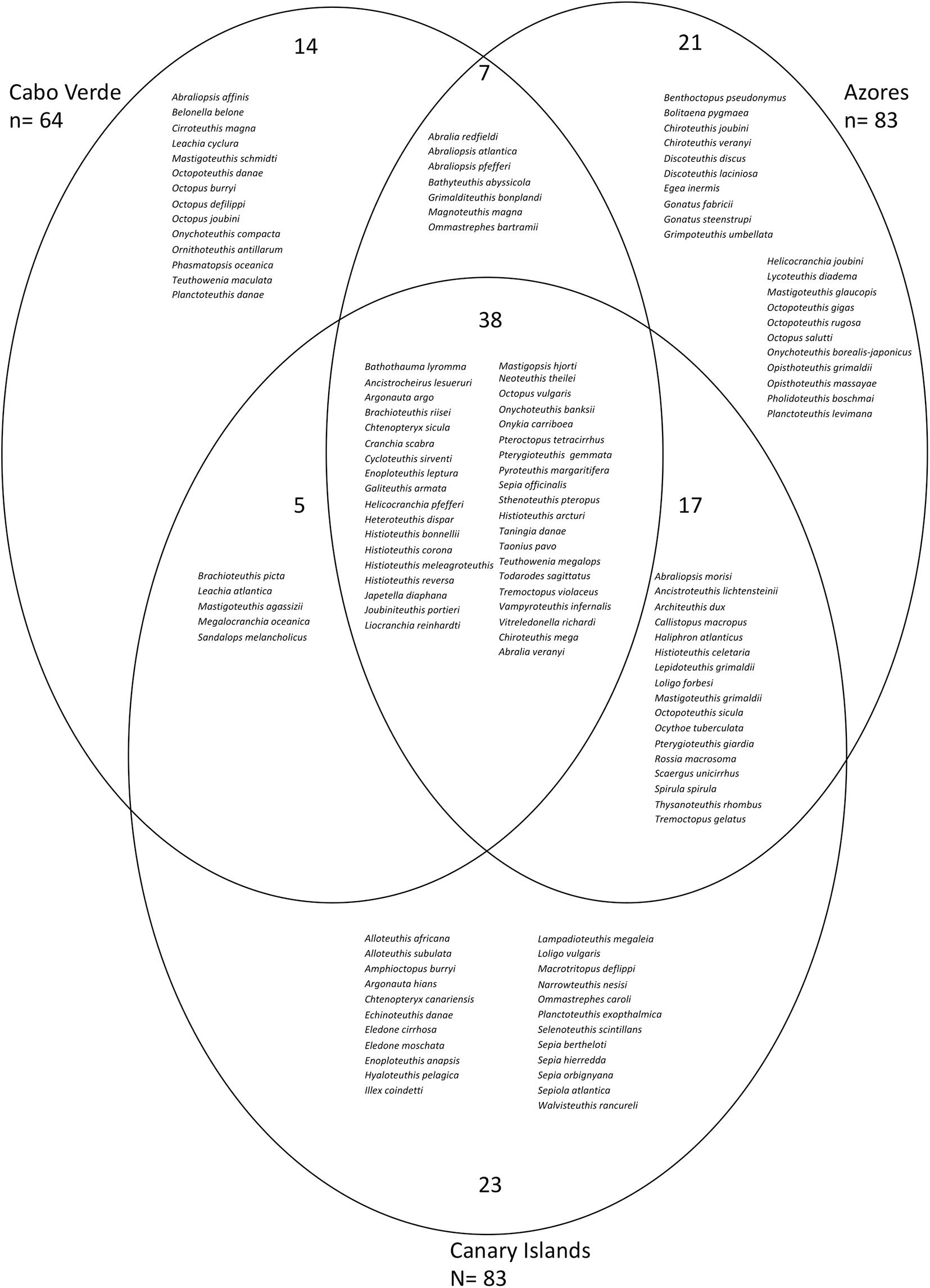
Figure 7. Biodiversity of cephalopod species off Cabo Verde, Canary Islands, and the Azores in the eastern Atlantic Ocean from the literature (Visser et al., 2021 for Azores; Escánez et al., 2020 for the Canary Islands) and this study. Overlapping spheres show overlapping species for the corresponding geographical regions. The number of species shared between different geographical regions or being unique are shown in black inside the circles. Only taxa that were identified to species level are included.
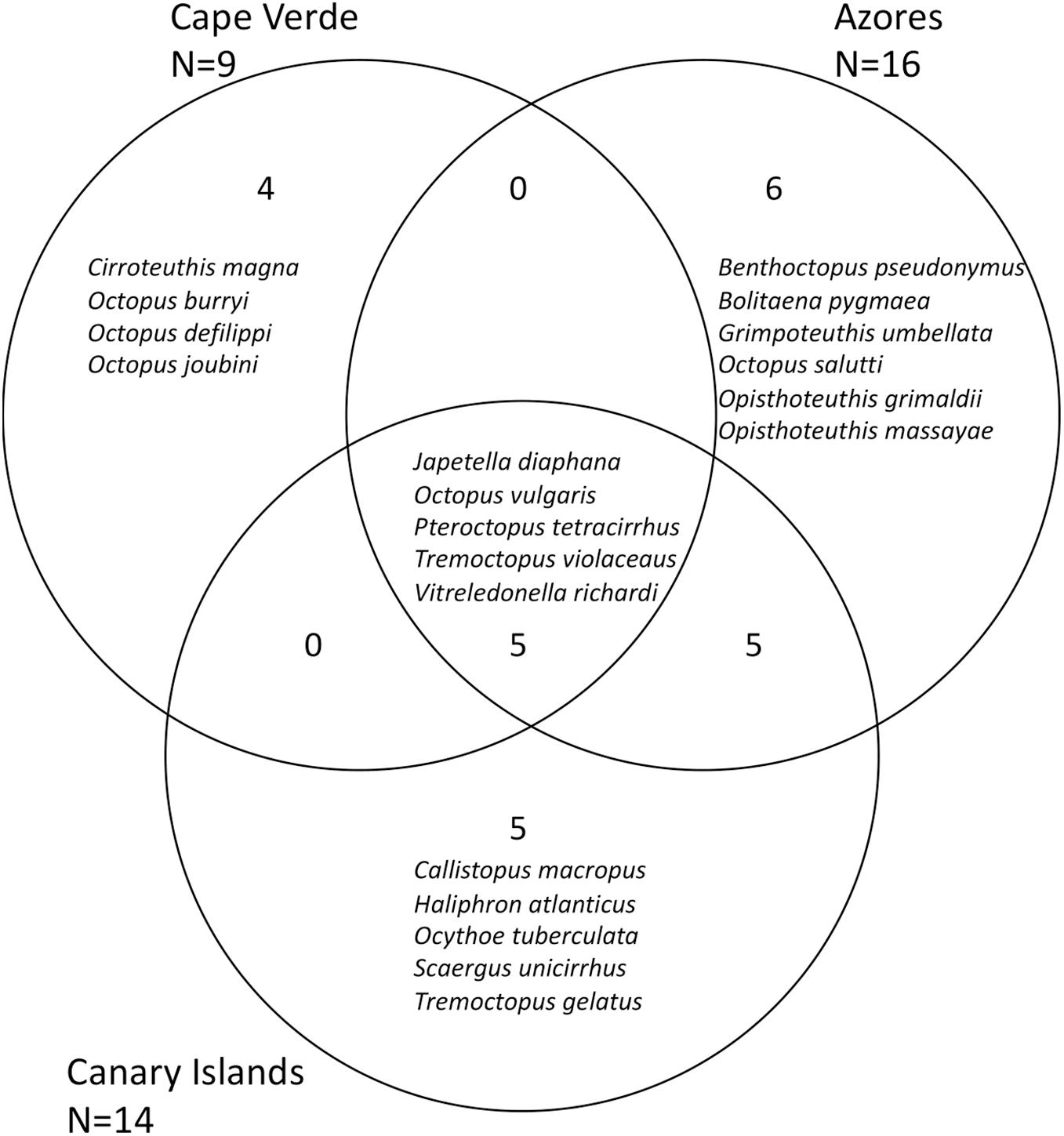
Figure 8. Biodiversity of octopus species off Cabo Verde, Canary Islands and the Azores in the eastern Atlantic Ocean. The number of taxa shared between different geographical regions or only occurring in one geographical region are shown in black inside the circles.
The Jaccard index measures dissimilarity in species composition between the three archipelagos and in this study this index ranged between 0.50 and 0.60, indicating that all islands shared between 40 and 50% of cephalopod species (Supplementary Table 5). When reducing the analysis to the Order Octopoda, the Jaccard index ranged between 0.5 and 0.75, with Azores and Canary Islands sharing 50% of octopus species, in contrast to Cabo Verde which only shared 25–27% of octopus species between the other two archipelagos (Supplementary Table 6).
Discussion
Cabo Verde as a Biodiversity Hotspot for Cephalopods
Combining the data from this study with records from the literature indicates that Cabo Verde is home to at least 102 cephalopod taxa of which 64 were identified to species level from 30 families. Nine of these taxa are rare and were detected in this region for the first time by this study. This includes three genera and six species detected with eDNA are known from the Atlantic Ocean, but had not been recorded around Cabo Verde before (Jereb and Roper, 2010). With 64 confirmed species, Cabo Verde can be considered a biodiversity hotspot for cephalopods comparable to the Canary Islands (Escánez et al., 2020) and Azores (see literature in Visser et al., 2021). This species richness is also comparable to those reported from other northern hemisphere open ocean systems identified as areas with high cephalopod species diversities such as the western North Atlantic (74 species), the North Sargasso Sea (68 species), the North African Subtropical Sea (72 species), and the Mediterranean outflow (70 species) (Rosa et al., 2008). The high species richness observed off Cabo Verde may be explained by the sampling sites being in vicinity of several seamounts (e.g., Senghor Seamount) and islands (Cabo Verde), topographical features that are known to enhance species diversity, creating various ecological niches for different species to thrive and evolve (Worm et al., 2003). Additionally, Cabo Verde is located at intermediate latitudes where temperate, subtropical and tropical species ranges overlap, leading to high diversities across trophic levels for predator and prey species (Worm et al., 2003). Many of the here detected cephalopod species belong to deep-sea families (Hoving et al., 2014) such as Cranchiidae, Histioteuthidae, Octopoteuthidae, Mastigoteuthida, and Chiroteuthida. This indicates that the identification of Cabo Verde as being a cephalopod diversity hotspot may be driven particularly by diversity in the deep sea. However, cephalopod diversity in shallow and coastal waters needs to be explored in more detail for a complete understanding of what is driving overall regional cephalopod community composition.
Two unexpected genera were detected with eDNA: Loligo and Alloteuthis. These genera are coastal and not known from Cabo Verde. They have not been detected by the demersal fisheries active around the islands prior to this study. The detections are unlikely to be false positives since they were absent from the corresponding negative controls. Another possibility may be the transport of eDNA by predators. Apex predators such as sperm whales, blue sharks and swordfish are known to feed on Loligo off the Azores and migrate to Cabo Verde (Clarke, 1956, Clarke et al., 1995, 1996), potentially releasing feces, and therefore eDNA, in Cabo Verdean waters. However, it can also not be ruled out that Loligo and Alloteuthis are undetected species in Cabo Verdean waters. Loligo and Alloteuthis are both distributed from tropical to temperate and subpolar coastal waters and occur along the northern and central west coast of Africa (Jereb and Roper, 2010). This indicates that species’ distributions may be broader than anticipated and eDNA detections of unknown and unexpected species must be considered carefully. In this context it is worth noting that in the South Atlantic Ocean the bathypelagic and benthopelagic squid Gonatopsis octopedatus has been dispersed more than 15,000 km from its region of original species distribution in the North Pacific, potentially by deep-sea currents (Arkhipkin et al., 2010).
This study was able to detect rare and elusive species with eDNA. One example is the bigfin squid Magnapinna sp. This squid is a bathypelagic species that is primarily known from underwater observations > 1800 m in the Pacific (Osterhage et al., 2020), Indian and Atlantic Ocean (Vecchione et al., 2001). Only a handful of damaged or juvenile specimens have been captured in the Pacific Ocean (Vecchione and Young, 1998). No observations or records have been made to date from the Cabo Verde region, although individuals have been captured 500 miles south of Cabo Verde from depths of between 998 and 1962 m in 2007 (Piatkowski, personal communication). This squid species has distinctive and relatively long, slim arms reaching lengths of over 1.5 m, compared to a mantle length of ∼15 cm (Osterhage et al., 2020). Magnapinna sp. is suggested to be globally distributed in bathyal and abyssal depths (Vecchione et al., 2001). This genus was solely detected with eDNA in this study and repeatedly in deep waters (1900 – 2500 m) off Cabo Verde, which is consistent with its known depth distribution in the Atlantic (Vecchione et al., 2001).
Vertical Distribution of Cephalopod Species Off Cabo Verde
The depth ranges stated in this study are a combination of general presence/absence of taxa. Since the cephalopod data presented here from the net catches is imbalanced with regard to day/night sampling, we do not differentiate between day and night distributions. In the Atlantic open ocean, cephalopod species diversity is known to be highest in the upper 200–1000 m and decreases with depth (Rosa et al., 2008) resulting in a bell-shaped diversity pattern with respect to depth. The same trend has been observed in pelagic fishes and invertebrates (Haedrich et al., 1980; Moranta et al., 1998; Smith and Brown, 2002; D’Onghia et al., 2004; Cartes et al., 2011; Papiol et al., 2012; Gaertner et al., 2013; Farré et al., 2016). High mesopelagic diversities coincide with enhanced primary productivity and optimal oxygen concentrations in overlying water layers (Levin et al., 2001) resulting in sinking of organic matter and high food supply at intermediate depths. Additionally, in this depth zone, predation pressure from top predators is intermediate and balanced by high or moderate production by low trophic levels providing prey for cephalopods and therefore facilitating their occurrence and coexistence (Gage and Tyler, 1991; Roxburgh et al., 2004). Cephalopod taxa community composition around the Cabo Verde islands of Santo Antão and Fogo, the two stations for which eDNA sampling was balanced with respect to depth, followed the same above-mentioned bell-shaped diversity pattern, with the highest number of species detected in the upper 1000 m, peaking at 600 m. This peak in cephalopod community composition correlates with species and biomass aggregations of myctophid fishes at 400–700 m in the central equatorial Atlantic (Kinzer and Schulz, 1985), which are, together with other fish species, primary prey of several cephalopods (Hoving et al., 2014; Merten et al., 2017; Villanueva et al., 2017). Overall cephalopod community composition decreased below 1000 m in comparison to the upper 1000 m, however, two peaks in cephalopod community composition were present at 1600 and 2200 m. The distribution of cephalopod species occurring in bathyal and abyssal depths is less well known than the distribution of epi- and mesopelagic species, partially due to difficulties in sampling the deep sea. Therefore, the species community composition is likely underestimated in these depths. The water layer immediately above the seafloor, the benthic boundary layer (BBL), is expected to support increased biomasses and species diversity (Brandt et al., 2007), which may explain the here observed peak in cephalopod species community composition at 2200 m depths. A previous study on eDNA of cephalopods in the hunting zones of Cuvier’s beaked whales (Ziphius cavirostris) showed that some predators specifically hunt in the BBL (Visser et al., 2021). Additionally, the high abundances of e.g., fish and crustaceans reported in the BBL (Dauvin and Vallet, 2006) suggests that BBL assemblages are of great importance for deep-sea food webs and nutrient cycling.
Due to the repeated observations of Taningia danae during video transects, but their absence in nets, we were particularly interested in the vertical distribution of this large squid, and hence developed and applied a species-specific primer on the eDNA samples. This species is recognized as one of the largest mesopelagic squid in tropical and subtropical oceans worldwide (Clarke, 1966) growing up to 150 kg and 1.7 m mantle length. Knowledge of this species is based on small juvenile individuals caught with mid-water nets (Roper and Vecchione, 1993), records generated from sperm whale stomach content analysis (Clarke, 1967; Okutani and Satake, 1978), specimens from fishing nets (González et al., 2003; Quetglas et al., 2006) and in situ observations with midwater camera systems (Kubodera et al., 2007; Gomes-Pereira and Tojeira, 2014; Hoving et al., 2019a). The presence of hundreds of beaks of T. danae in sperm whale stomachs suggests that this species is a very abundant deep-sea squid (Clarke, 1996b). However, adults are rarely caught with nets, potentially due to their occurrence at considerable depths and their strong swimming capabilities. The relatively frequent detections of T. danae during the video transects may be the result of T. danae being attracted to the light of the camera system (Hoving et al., 2019a) as seen in other studies applying lured camera systems in midwater (Kubodera et al., 2007; Gomes-Pereira and Tojeira, 2014). Juvenile and young T. danae undergo ontogenetic migration from the surface to 200 – 300 m water depth (Roper and Vecchione, 1993) and larger specimens perform short distance diel vertical migration from depths of 600 – 900 m during the day to shallower depths of 240 – 500 m during the night (Kubodera et al., 2007). Considering the hunting grounds of sperm whales and deep-sea sharks, adult T. danae were thought to occur below 1000 m (Clarke and Merrett, 1972). This deep occurrence is supported by the eDNA and video data of this study. The video surveys observed T. danae between 400 and 1400 m, whereas eDNA detected T. danae between 100 and 2500 m, with the majority of detections at 1600 m. The fact that T. danae was detected frequently in the eDNA samples highlights the potential importance and abundance of this elusive top-predator in deep-sea ecosystems, and shows the ability of eDNA to detect species that are rarely captured in nets.
Comparing Cabo Verde Cephalopod Biodiversity With Other Macaronesian Islands
The three archipelagos Cabo Verde, the Canary Islands and Azores investigated in this study share overall cephalopod community composition. However, the data indicates a difference in octopus community composition between Cabo Verde and the Azores as well as Canary Islands, suggesting a separation of Cabo Verde when focusing specifically on benthic octopuses. These findings are in line with studies proposing a biogeographical separation of Cabo Verde from Macaronesia based on the dispersal of benthic and coastal metazoan species (Freitas et al., 2019). Biogeographical separation and endemism are tightly linked to the larval dispersal ability of organisms as dispersal directly influences gene flow within and among populations. Populations may become either genetically more similar when subject to frequent mixing or they may become more isolated when mixing and dispersal is reduced or absent. There are strong inter-specific differences in cephalopod dispersal abilities. Cephalopod species that hatch planktonic paralarvae tend to be smaller and reach broader distributional ranges than species with larger, benthic hatchlings (Villanueva et al., 2016). Additionally, species with offshore larval distribution have been shown to have larger mean geographic ranges than species with inshore larval distribution (Macpherson and Raventos, 2006), a trend also observed in fish (Macpherson and Raventos, 2006), highlighting the importance of oceanographic currents as powerful dispersal mechanisms for pelagic species (Rowell et al., 1985; Saito and Kubodera, 1993; Bower et al., 1999; Roberts and van den Berg, 2005; Martins et al., 2014; Downey-Breedt et al., 2016).
Due to their muscular anatomy, strong swimming capabilities and highly mobile life style, some squid species are able to actively migrate hundreds of kilometers. For instance, the ommastrephid squid Sthenoteuthis pteropus spawns in the eastern equatorial Atlantic (Zuyev et al., 2002) and its paralarvae are quickly dispersed in the equatorial zone. Adult females are known to migrate 2500 km from Cabo Verde to Madeira and back (Zuev and Nikolsky, 1993; Zuyev et al., 2002). Other oceanic squids occurring off the Cabo Verde islands also occur off the Azores and Canary Islands, such as Taningia danae and Todarodes sagittatus. Due to their mobility and ability to cope with different oceanographic conditions, many squid species are able to sustain broad horizontal and vertical distribution patterns (Childress and Seibel, 1998; Zuyev et al., 2002; Rosa and Seibel, 2010; Hoving and Robison, 2012; Rodhouse et al., 2014; Doubleday et al., 2016). For species with limited swimming capacities, pelagic egg masses may facilitate dispersal (Young and Harman, 1985; Roberts et al., 2011).
The majority of octopus species have a short planktonic larval stage followed by a benthic adult lifestyle, and as such have a limited dispersal potential due to e.g., larger hatchling sizes, a characteristic linked with limited dispersal (Villanueva et al., 2016) and association with certain substrates. For example, the benthic octopus Pareledone turqueti is unable to disperse between sites that are separated by ocean depths > 1000 m, which presents a major physical barrier for the species (Allcock et al., 1997). This limited dispersal ability and therefore reduced gene flow in octopuses is also applicable to the common octopus Octopus vulgaris (Sampaio et al., 2018). The common octopus is the only benthic octopus occurring at all three archipelagos, the Canary Islands, Azores and Cabo Verde. However, O. vulgaris populations off Cabo Verde potentially show a developing endemism of this species (Sampaio et al., 2018). The other octopus species from the current study to occur across the three archipelagos were all pelagic octopuses that brood their eggs in midwater or have pelagic paralarvae (Japetella diaphana, Pteroctopus tetracirrhus, Vitreledonella richardi, and Tremoctopus violaceus). Therefore with increased dispersal abilities potentially comparable with those of oceanic squids. The oceanographic features of Cabo Verde form natural barriers to the north of the islands and toward the west coast of Africa, whereas the Canary Islands and Azores are connected by the Azores Current and the Madeira current, forming a seaway, with several shallow seamounts functioning as stepping stones for marine organisms (Freitas et al., 2019). As a result, benthic or benthopelagic organisms with reduced mobility, in contrast with the high mobility of squids, may become separated from species of the other archipelagos, leading to a separation of Cabo Verde from Macaronesia. This is indicated in octopus species composition in this study. To fully understand the biogeography of Macaronesia, not only benthic and coastal organisms should be taken into account, but also the pelagic, open ocean fauna should be considered.
Potential Role of Cephalopods in the Carbon Cycle of the Deep Sea of Cabo Verde and Macaronesia
An active pathway of vertical carbon distribution by cephalopods is achieved by vertical migration. Many cephalopods spend the paralarval and juvenile phase of their lifecycle in upper surface waters, benefiting from primary production (Hoving et al., 2014). As they grow and mature, they descend to deeper layers to reproduce, transporting carbon from shallow to deep waters via ontogenetic migration. The second active pathway of carbon distribution is diel vertical migration, a common trait of many deep-sea cephalopods (Jereb and Roper, 2010; Hoving et al., 2014; Judkins and Vecchione, 2020). These cephalopods migrate close to the surface at night for feeding and descend to deeper layers during the day, to potentially avoid visual predators or to maintain their energy reserves in the colder, deeper water (Sutton, 2013). Off Cabo Verde, 31% of the confirmed cephalopod taxa are known to undergo ontogenetic migration such as Sthenoteuthis pteropus, Taningia danae and most of the cranchiids (Jereb and Roper, 2010; Judkins and Vecchione, 2020). Thirty-five are also known to perform diel vertical migration (e.g., Sthenoteuthis pteropus, Taningia danae as well as many enoploteuthids) (Jereb and Roper, 2010).
Carbon can also be distributed passively when cephalopods die after reproduction, either sinking to the seafloor or floating to the surface, depending on the species (Fields, 1965; Stockton and DeLaca, 1982; Roper and Vecchione, 1996; Martin and Christiansen, 1997; Nesis et al., 1998; Boyle and Rodhouse, 2005; Hoving et al., 2017). To date, there are no records of cephalopod carcasses on the seafloor in the Cabo Verde region. However, stomach content analysis of deep-sea scavengers off the Cabo Verde islands have found ommastrephid squid in the stomach contents of the cusk eel (Neobythites sp.) and the velvet belly lanternshark (Etmopterus spinax) (Clarke and Merrett, 1972). Both scavengers are benthic and likely fed on carcasses rather than actively hunted epi- and mesopelagic squid.
Species in the Cabo Verde area which may form substantial foodfalls when they die (settling to the seafloor) are T. danae and S. pteropus. Taningia danae is suggested to be associated with bottom waters when spawning (Nesis, 1987), indicating that this species may be consumed by benthic organisms after spawning and death. Given the estimated high abundance and large size (∼1.70 m mantle length), biomass from this species may contribute significantly to the regional carbon cycle of the deep sea. The orangeback flying squid S. pteropus is another abundant and dominant squid species, in terms of numbers of individuals and biomass (instantaneous biomass: 4–6.5 million tons, annual total biomass production = 34–52 million tons) in the eastern tropical Atlantic (Zuyev et al., 2002; Jereb and Roper, 2010). Sthenoteuthis pteropus can reach maximum mantle lengths of 65 cm and has a broad distribution from Madeira (36°N) in the North to South Africa (32°S) in the South. This species’ distribution spans the whole of Macaronesia and individuals are known to migrate thousands of kilometers from Cabo Verde to Madeira and back. Spawning of S. pteropus is not linked to bottom waters, but occurs in the epipelagic zone. This species is not neutrally buoyant and individuals sink to the seafloor when they stop swimming. Tissues from this species have high protein concentrations (17%) (Zuyev et al., 2002), rendering them as a valuable food source. Sthenoteuthis pteropus undergoes ontogenetic and diel vertical migration down to 1200 m water depth (Moiseev, 1991), resulting in active transport of carbon from the surface to the mesopelagic ocean. Due to its high abundance, wide distribution and migration ability, good nutritional value, large size, spawning style and negative buoyancy, S. pteropus may transport carbon from epi- and mesopelagic zones to the deep-sea benthos.
The Use of Physical, Optical and Genetic Censuses for Biodiversity Assessment
The results from the three methods employed in this study each indicated differing cephalopod families to be regionally dominant. Mastigoteuthidae followed by Ommastrephidae and Loliginidae were the most commonly detected families with eDNA, whereas with nets, the most commonly detected families were Cranchiidae followed by Pyroteuthidae and Enoploteuthidae. This is in line with net data from the literature from the eastern North Atlantic, with Cranchiidae (Liocranchia reinhardtii) being the most commonly detected family in rectangular midwater trawls caught in 1968 and 1972, followed by Onychoteuthidae (Onychotetuhis banksii) (Clarke, 2006). However, Pyroteuthidae and Enoploteuthidae only represented 5 and 2%, respectively, of the samples collected by the trawls described in Clarke (2006). This highlights the potential collection bias toward certain species, as a function of the trawling gear characteristics being used, and therefore that differences in counts can occur within methods (i.e., between different trawls) as well as between methods (i.e., between eDNA and trawl catch analysis). For example, in Clarke (2006), three different types of nets were used with a net opening of between 0.5 m2 (Multinet) and 480 m2 (pelagic trawl) and mesh sizes of between 335 μm and 4 mm. In the current study, smaller net openings and mesh sizes, such as those of the IKMT and Multinet, mainly caught paralarvae and small individuals between 4 and 190 mm mantle length, whereas the pelagic trawl captured larger individuals of between 14 and 240 mm mantle length. However, even the pelagic trawl used here was not able to catch very large squids. Video surveys conducted by different gears also resulted in differing sampling bias. The manned submersible JAGO used in this study is loud under water, potentially scaring organisms away. PELAGIOS on the other hand is attached to a research vessel via cable, causing vibrations in the water column along the cable length, which could also influence observations. Both gears emit light that may attract some species and repel others. The majority of observed cephalopods that could be identified to at least family level was within video data collected with PELAGIOS, whereas within JAGO video data, Cranchiids, which are often less mobile, were more often observed. Within JAGO data cephalopods were often observed in mid escape, moving swiftly and therefore could not be identified to high taxonomic resolution.
The video observation results were in general accordance with the observations from the nets, both detecting the Cranchiidae as the most abundant family. The second most commonly detected family were the Mastigoteuthidae which aligned with the eDNA results. This discrepancy in assessment of the most frequently observed families reported by these three methods may partially be the result of differing characteristics of the sampling methods. Cranchiids are abundant and when small often less mobile, therefore they are more easily caught with nets than some other families. Ommastrephids on the other hand are highly mobile, muscular and often larger squids that may be able to easily escape approaching nets. As a defense strategy, ommastrephids and mastigoteuthids also release large amounts of ink when being threatened. This ink, which contains DNA, may result in a higher abundance of eDNA in their environment than that associated with some of the other families, e.g., cranchiids. Cranchiids have been observed to eject ink inside their mantle cavity for camouflage, therefore not releasing it externally (Hunt, 1996). This may reduce the amount of eDNA released by that cephalopod group. Information on eDNA shedding rates of cephalopods is needed, as the size of an individual does not necessarily reflect the amount of e.g., tissue being shed. Further, differing oceanographic conditions might influence the detectability of eDNA (Pinfield et al., 2019).
The three sampling approaches applied in this study complemented each other in genera and species detections. It seems on first examination of the data that net tows were the most effective sampling method for assessing cephalopod deep-sea diversity, since specimens for reference databases were also collected. However, when comparing the eDNA data with trawl data from all cruises individually, net tows were either more effective, comparable or less effective in finding taxa when compared to the eDNA approach. Between 9 and 20% of species were detected with both the eDNA and net approach, indicating a considerable contribution of 20 – 43% in species detections when combining eDNA with net trawls. However, direct and quantitative comparisons between and within the three sampling methods applied here was not possible and was not the priority of the current study, as the sampled sites, seasons, years, targeted depths and gear used within and between methods differed substantially, as well as the sample sizes. The volume of water that was sampled with CTD niskin bottles for the eDNA approach was several magnitudes less than that which was physically and optically sampled by nets and video surveys, respectively. As the rarefaction curve for eDNA did not reach the asymptote from the data collected, sampling more stations and depths would likely have resulted in additional detected taxa. Similarly, the addition of more targeted marker genes may have increased the number of species and taxa detected by the eDNA methodological approach. By increasing the sampling size for eDNA samples we can expect eDNA to become progressively more equivalent, or even superior, to the net trawl methodological approach in detecting taxa, as has been the case in other studies focusing on other organisms, such as fish (Thomsen et al., 2012; Boussarie et al., 2018; Govindarajan et al., 2021). However, reference specimens are required from a region to compare with obtained eDNA sequences.
This study highlights that the complementary use of different methods yields the most holistic view of deep-sea cephalopod communities. Nets remain indispensable since reference specimens are needed to detect, compare and validate sequences obtained with eDNA. However, both the eDNA approach and video surveys are non-invasive and appropriate for integration into the surveillance and management plans of marine ecosystems, but efforts should be increased to incorporate more primers into the analysis procedure and also the seafloor should be sampled for eDNA analysis.
Data Availability Statement
The datasets presented in this study can be found in online repositories. The names of the repository/repositories and accession number(s) can be found below: NCBI (Accession: AY393902.1, MG591434.1, EU735402.1). PANGAEA repository: https://doi.pangaea.de/10.1594/PANGAEA.937568.
Ethics Statement
Ethical review and approval was not required for the animal study because the cephalopods that were captured with nets were dead when they came on board. No experiments were conducted. Cabo Verde has not ratified the Nagoya protocol. To fulfill the national access and benefit sharing (ABS) regulations of Cabo Verde, we obtained the required permits for sample collection and publication of results based on samples collected in Cabo Verde waters from the Direcao Nacional do Ambiente (National Directorate for the Environment of CabonVerde).
Author Contributions
H-JH conceived the study. VM, H-JH, TB, TR, and OP planned the study. VM, JS, and JF conducted the lab work. AL, UP, SC, HH, BC, AD, and H-JH contributed the data. VM and H-JH analyzed the data and wrote the manuscript. TB, TR, OP, JF, JS, AL, HH, UP, SC, BC, and AD critically reviewed the manuscript. All authors contributed to the article and approved the submitted version.
Funding
Shiptime on RVs Maria S. Merian, Meteor and supporting funds and shiptime on Poseidon were provided by the German Research Foundation (Deutsche Forschungsgemeinschaft, DFG) (grant MSM49 to BC; MSM61 to H-JH; POS520 to H-JH; POS532 to H-JH and HH). H-JH was funded by grant CP1218 of the Cluster of Excellence 80 “The Future Ocean.” “The Future Ocean” was funded within the framework of the Excellence Initiative by the DFG on behalf of the German federal and state governments. H-JH is currently funded by the DFG grant the German Research Foundation (DFG) Emmy Noether Research Junior Group grant of H-JH (HO 5569/2-1) and GEOMAR’s POF III OCEANS program and POF IV. JF was funded by the DFG-project 4074495230 “High-Throughput Sequencing.”
Conflict of Interest
The authors declare that the research was conducted in the absence of any commercial or financial relationships that could be construed as a potential conflict of interest.
Publisher’s Note
All claims expressed in this article are solely those of the authors and do not necessarily represent those of their affiliated organizations, or those of the publisher, the editors and the reviewers. Any product that may be evaluated in this article, or claim that may be made by its manufacturer, is not guaranteed or endorsed by the publisher.
Acknowledgments
We thank the crew and participants of the cruises POS320/2, M119, MSM49, WH383, MSM61, POS520, and POS532 by helping collecting samples and special thanks goes to Heino Fock for the possibility to sample on WH383. Thanks goes to Hendrik Hampe and Stella Luna Scheer for collecting eDNA samples during POS532. We thank the institute of Clinical Molecular Biology in Kiel for providing Sanger sequencing as supported in part by the DFG Cluster of Excellence “Inflammation at Interfaces” and “Future Ocean.” We thank the technicians T. Naujoks and C. Noack for technical support. We thank Autun Purser for his revisions. We also thank the editor and reviewers for their constructive comments. We also thank Peter Brandt, Björn Fiedler, and Johannes Karstensen for the opportunity to collect samples during their research cruises.
Supplementary Material
The Supplementary Material for this article can be found online at: https://www.frontiersin.org/articles/10.3389/fmars.2021.760108/full#supplementary-material
Footnotes
References
Allcock, A. L., Brierley, A. S., Thorpe, J. P., and Rodhouse, P. G. (1997). Restricted gene flow and evolutionary divergence between geographically separated populations of the Antarctic octopus Pareledone turqueti. Mar. Biol. 129, 97–102. doi: 10.1007/s002270050150
Altschul, S. F., Gish, W., Miller, W., Myers, E. W., and Lipman, D. J. (1990). Basic local alignment search tool. J. Mol. Biol. 215, 403–410. doi: 10.1016/S0022-2836(05)80360-2
Arkhipkin, A. I., Laptikhovsky, V. V., and Brickle, P. (2010). An antipodal link between the north pacific and South Atlantic Ocean? Deep Sea Res. 57, 1009–1011. doi: 10.1016/j.dsr.2010.05.004
Ávila, S. P. (2000). Shallow-water marine molluscs of the azores: biogeographical relationships. Arquipelago Life Mar. Sci. 2, 99–131.
Ávila, S. P. (2005). Processos e Padrões de Dispersão e Colonização nos Rissoidae (Mollusca: Gastropoda) dos Açores (Biology/Palaeontology). Ponta Delgada: Universidade dos Açores.
Borges, P. A. V., Costa, A. C., Cunha, R., Gabriel, R., Gonçalves, V., Martins, A., et al. (2010). A List of the Terrestrial and Marine Biota From the Azores. Cascais: Principia.
Boussarie, G., Bakker, J., Wangensteen, O. S., Mariani, S., Bonnin, L., Juhel, J.-B., et al. (2018). Environmental DNA illuminates the dark diversity of sharks. Sci. Adv. 4:eaa9661. doi: 10.1126/sciadv.aap9661
Bower, J. R., Seki, M. P., Young, R. E., Bigelow, K. A., Hirota, J., and Flament, P. (1999). Cephalopod paralarvae assemblages in Hawaiian Islands waters. Mar. Ecol. Prog. Ser. 185, 203–212.
Boyle, P., and Rodhouse, P. (eds) (2005). “Life cycle,” in Cephalopods: Ecology and Fisheries, (Oxford: Blackwell Science Ltd), 80–100.
Brandt, A., De Broyer, C., De Mesel, I., Ellingsen, K. E., Gooday, A. J., Hilbig, B., et al. (2007). The biodiversity of the deep Southern Ocean benthos. Philos. Trans. R. Soc. B Biol. Sci. 362, 39–66. doi: 10.1098/rstb.2006.1952
Brandt, P. (2016). Oxygen Variability and Tropical Atlantic Circulation. Germany: DFG-Senatskommission für Ozeanographie.
Breitburg, D., Levin, L. A., Oschlies, A., Grégoire, M., Chavez, F. P., Conley, D. J., et al. (2018). Declining oxygen in the global ocean and coastal waters. Science 359:aam7240. doi: 10.1126/science.aam7240
Bush, S. L., Robison, B. H., and Caldwell, R. L. (2009). Behaving in the dark: locomotor, chromatic, postural, and bioluminescent behaviour of the deep-sea squid octopoteuthis deletron young 1972. Biol. Bull. 216, 7–22. doi: 10.1086/BBLv216n1p7
Callahan, B. J., McMurdie, P. J., Rosen, M. J., Han, A. W., Johnson, A. J. A., and Holmes, S. P. (2016). DADA2: high-resolution sample inference from Illumina amplicon data. Nat. Methods 13, 581–583. doi: 10.1038/nmeth.3869
Cartes, J. E., Mamouridis, V., and Fanelli, E. (2011). Deep-sea suprabenthos assemblages (crustacea) off the Balearic Islands (western mediterranean): mesoscale variability in diversity and production. J. Sea Res. 65, 340–354. doi: 10.1016/j.seares.2011.02.002
Childress, J. J., and Seibel, B. A. (1998). Life at stable low oxygen levels: adaptations of animals to oceanic oxygen minimum layers. J. Exp. Biol. 201:1223.
Christiansen, B., Buchholz, C., Buchholz, F., Xupeng, C., Christiansen, S., Denda, A., et al. (2016). SEAMOX: the influence of seamounts and oxygen minimum zones on pelagic fauna in the eastern tropical atlantic cruise no. MSM49 november 28 - december 21, 2015 las palmas de gran canaria (spain) - mindelo (republic of cape verde). Maria Merian Berichte MSM 49:42.
Clarke, M. R. (1966). A review of the systematics and ecology of oceanic squids. Adv. Mari. Biol. 4, 91–300. doi: 10.1016/S0065-2881(08)60314-4
Clarke, M. R. (1967). A deep-sea squid, taningia danae, joubin 1931. Symp. Zool. Soc. Lond. 19, 127–143.
Clarke, M. R. (1996a). Cephalopods as prey. III. cetaceans. Philos. Trans. R. Soc. B Biol. Sci. 351, 1053–1065. doi: 10.1098/rstb.1996.0093
Clarke, M. R. (1996b). The role of cephalopods in the world’s oceans: an introduction. Philos. Trans. R. Soc. B Biol. Sci. 351, 979–983.
Clarke, M. R. (2006). Oceanic cephalopod distribution and species diversity in the eastern north Atlantic. Arquipél. Life Mar. Sci. 23, 27–46.
Clarke, M. R., Clarke, D. C., Martins, H. R., and Da Silva, H. M. (1996). The diet of the blue shark (Prionace glauca L.) in Azorean waters. Arquipél. Ciênc. Biológicas E Mar. 14A, 41–56.
Clarke, M. R., Clarke, D. C., and Silva, H. M. (1995). The diet of swordfish (Xiphias gladius) in Azorean waters. Arquipelago Life Mar. Sci. 13A, 53–69.
Clarke, M. R., and Merrett, N. (1972). The significance of squid, whale and other remains from the stomachs of bottom-living deep-sea fish. J. Mar. Biol. Assoc. U.K. 52, 599–603. doi: 10.1017/S0025315400021603
Collins, M. A., O’Dea, M., and Henriques, C. (2001). A large Cirroteuthis magna (cephalopoda: cirroctopoda) caught on the cape verde terrace (North Atlantic). J. Mar. Biol. Assoc. U.K. 81, 357–358. doi: 10.1017/S0025315401003915
Cordeiro, R., and Ávila, S. P. (2015). New species of Rissoidae (mollusca, gastropoda) from the archipelago of the Azores (northeast Atlantic) with an updated regional checklist for the family. ZooKeys 480, 1–19. doi: 10.3897/zookeys.480.8599
Cowart, D. A., Murphy, K. R., and Cheng, C.-H. C. (2018). Metagenomic sequencing of environmental DNA reveals marine faunal assemblages from the West Antarctic peninsula. Mar. Genomics 37, 148–160. doi: 10.1016/j.margen.2017.11.003
Dauvin, J.-C., and Vallet, C. (2006). The near-bottom layer as an ecological boundary in marine ecosystems: diversity, taxonomic composition and community definitions. Hydrobiologia 555, 49–58. doi: 10.1007/s10750-005-1105-5
de Jonge, D., Merten, V., Bayer, T., Puebla, O., Reusch, T. B. H., and Hoving, H.-J. T. (2021). A novel metabarcoding primer pair for environmental DNA analysis of cephalopoda (mollusca) targeting the nuclear 18S rRNA region. R. Soc. Open Sci. 8:201388. doi: 10.1098/rsos.201388
D’Onghia, G., Politou, C.-Y., Bozzano, A., Lloris, D., Rotllant, G., Sion, L., et al. (2004). Deep-water fish assemblages in the mediterranean sea. Sci. Mar. Mediterr. Deep Sea Biol. 68, 87–99. doi: 10.3989/scimar.2004.68s387
Doty, M. S., and Oguri, M. (1956). The Island Mass Effect. ICES J. Mar. Sci. 22, 33–37. doi: 10.1093/icesjms/22.1.33
Doubleday, Z. A., Prowse, T. A. A., Arkhipkin, A., Pierce, G. J., Semmens, J., Steer, M., et al. (2016). Global proliferation of cephalopods. Curr. Biol. 26, R406–R407. doi: 10.1016/j.cub.2016.04.002
Downey-Breedt, N. J., Roberts, M. J., Sauer, W. H. H., and Chang, N. (2016). Modelling transport of inshore and deep-spawned chokka squid (Loligo reynaudi) paralarvae off South Africa: the potential contribution of deep spawning to recruitment. Fish. Oceanogr. 25, 28–43. doi: 10.1111/fog.12132
Duda, T. F., and Rolán, E. (2005). Explosive radiation of cape verde conus, a marine species flock. Mol. Ecol. 14, 267–272. doi: 10.1111/j.1365-294X.2004.02397.x
Engås, A., Skeide, R., and West, C. W. (1997). The ‘multisampler’: a system for remotely opening and closing multiple codends on a sampling trawl. Fish. Res. 29, 295–298. doi: 10.1016/S0165-7836(96)00545-0
Escánez, A., Guerra, Á, Riera, R., and Rocha, F. J. (2020). Revised species records reveal the canary islands as a cephalopod biodiversity hotspot. Reg. Stud. Mar. Sci. 41:101541. doi: 10.1016/j.rsma.2020.101541
Everett, M. V., and Park, L. K. (2018). Exploring deep-water coral communities using environmental DNA. results telepresence-enabled oceanogr. Explor 150, 229–241. doi: 10.1016/j.dsr2.2017.09.008
Farré, M., Tuset, V. M., Cartes, J. E., Massutí, E., and Lombarte, A. (2016). Depth-related trends in morphological and functional diversity of demersal fish assemblages in the western mediterranean Sea. Prog. Oceanogr. 147, 22–37. doi: 10.1016/j.pocean.2016.07.006
Ficetola, G. F., Coissac, E., Zundel, S., Riaz, T., Shehzad, W., Bessiere, J., et al. (2010). An in silico approach for the evaluation of DNA barcodes. BMC Genom. 11:434. doi: 10.1186/1471-2164-11-434
Fiedler, B., Hoving, H. J. T., Schütte, F., Czudaj, S., Genio, L., Cunha, M., et al. (2020). Intra-Annual Variability of Biological, Chemical and Physical Parameters at the Senghor SeamountCruise No. MSM61. Germany: Maria Merian Berichte Gutachterpanel Foschungsschiffe, 30.
Fields, W. G. (1965). The structure, development, food relations, reproduction and life history of the squid loligo opalescens berry. UC San Diego LibrScripps Digit. Collect. 131, 1–108.
Fock, H. O. (2015). Report of the cruise 383 of the FRV walther herwig III. Fed. Res. Inst. Rural Areas For. Fish. 11, 1–11.
Fock, H. O., and Czudaj, S. (2019). Size structure changes of mesopelagic fishes and community biomass size spectra along a transect from the equator to the bay of biscay collected in 1966–1979 and 2014–2015. ICES J. Mar. Sci. 76, 755–770. doi: 10.1093/icesjms/fsy068
Freitas, R., Romeiras, M., Silva, L., Cordeiro, R., Madeira, P., González, J. A., et al. (2019). Restructuring of the ‘macaronesia’ biogeographic unit: a marine multi-taxon biogeographical approach. Sci. Rep. 9:15792. doi: 10.1038/s41598-019-51786-6
Gaertner, J.-C., Maiorano, P., Mérigot, B., Colloca, F., Politou, C.-Y., Gil De Sola, L., et al. (2013). Large-scale diversity of slope fishes: pattern inconsistency between multiple diversity indices. PLoS One 8:e66753. doi: 10.1371/journal.pone.0066753
Gage, J. D., and Tyler, P. A. (1991). Deep-Sea Biology: A Natural history of Organisms at the Deep-sea Floor. Cambridge: Cambridge University Press.
Gomes-Pereira, J. N., and Tojeira, I. (2014). The cephalopod taningia danae joubin, 1931 observed near bottom at over 2,000 m depth on seine seamount. Mar. Biodivers. 44, 151–155. doi: 10.1007/s12526-013-0197-9
González, Á, Guerra, A., and Rocha, F. (2003). New data on the life history and ecology of the deep-sea hooked squid taningia danae. Sarsia N. Atl. Mar. Sci. 88, 1–6. doi: 10.1080/00364820310002524
Gotelli, N. J., and Colwell, R. K. (2001). Quantifying biodiversity: procedures and pitfalls in the measurement and comparison of species richness. Ecol. Lett. 4, 379–391. doi: 10.1046/j.1461-0248.2001.00230.x
Gove, J. M., McManus, M. A., Neuheimer, A. B., Polovina, J. J., Drazen, J. C., Smith, C. R., et al. (2016). Near-island biological hotspots in barren ocean basins. Nat. Commun. 7:10581. doi: 10.1038/ncomms10581
Govindarajan, A. F., Francolini, R. D., Jech, J. M., Lavery, A. C., Llopiz, J. K., Wiebe, P. H., et al. (2021). Exploring the use of environmental DNA (eDNA) to detect animal taxa in the mesopelagic zone. Front. Ecol. Evol. 9:146. doi: 10.3389/fevo.2021.574877
Haedrich, R. L., Rowe, G. T., and Polloni, P. T. (1980). The megabenthic fauna in the deep sea south of New England, USA. Mar. Biol. 57, 165–179. doi: 10.1007/BF00390735
Harrison, C. M. H. (1967). “On methods for sampling mesopelagic fishes,” in Aspects of Marine Zoology, ed. N. B. Marshall 71–119.
Heino, M., Porteiro, F. M., Sutton, T. T., Falkenhaug, T., Godø, O. R., and Piatkowski, U. (2011). Catchability of pelagic trawls for sampling deep-living nekton in the mid-North Atlantic. ICES J. Mar. Sci. 68, 377–389. doi: 10.1093/icesjms/fsq089
Hissmann, K., and Schauer, J. (2017). Manned submersible “JAGO.”. J. Large Scale Res. Facil. 3, 1–12.
Hoving, H. J. T., Bush, S. L., Haddock, S. H. D., and Robison, B. H. (2017). Bathyal feasting: post-spawning squid as a source of carbon for deep-sea benthic communities. Proc. R. Soc. B Biol. Sci. 284:20172096. doi: 10.1098/rspb.2017.2096
Hoving, H. J. T., Christiansen, S., Fabrizius, E., Hauss, H., Kiko, R., Linke, P., et al. (2019a). The pelagic in situ observation system (PELAGIOS) to reveal biodiversity, behavior, and ecology of elusive oceanic fauna. Ocean Sci. 15, 1327–1340.
Hoving, H. J. T., Hauss, H., Freitas, R., Hissmann, K., Osborn, K., Scheer, S., et al. (2019b). The Role of Gelatinous Macrozooplankton in the Deep-Sea Carbon Transport in Cape Verde, POSEIDON-Berichte (Kiel, Germany: GEOMAR), 38.
Hoving, H. J. T., and Haddock, S. H. D. (2017). The giant deep-sea octopus Haliphron atlanticus forages on gelatinous fauna. Sci. Rep. 7:44952. doi: 10.1038/srep44952
Hoving, H. J. T., Hauss, H., Schütte, F., Merten, V., Fabrizius, E., Hissmann, K., et al. (2018). Biological baseline studies in the pelagic deep seas of cape verde. Cruise Rep. 29, 1–29.
Hoving, H.-J. T., Laptikhovsky, V. V., and Robison, B. H. (2015). Vampire squid reproductive strategy is unique among coleoid cephalopods. Curr. Biol. 25, R322–R323. doi: 10.1016/j.cub.2015.02.018
Hoving, H. J. T., Neitzel, P., Hauss, H., Christiansen, S., Kiko, R., Robison, B. H., et al. (2020). In situ observations show vertical community structure of pelagic fauna in the eastern tropical North Atlantic off cape verde. Sci. Rep. 10:21798. doi: 10.1038/s41598-020-78255-9
Hoving, H. J. T., Perez, J. A. A., Bolstad, K. S. R., Braid, H. E., Evans, A. B., Fuchs, D., et al. (2014). The study of deep-sea cephalopods. Adv. Mari. Biol. 67, 235–359. doi: 10.1016/B978-0-12-800287-2.00003-2
Hoving, H. J. T., and Robison, B. H. (2012). Vampire squid: detritivores in the oxygen minimum zone. Proc. R. Soc. B Biol. Sci. 279, 4559–4567. doi: 10.1098/rspb.2012.1357
Hunt, J. C. (1996). The Behaviour and Ecology of Midwater Cephalopods From Monterey Bay: Submersible and Labaratory Observations Ph. D, Thesis. Los Angeles: University of California.
Jarman, S. N., Redd, K. S., and Gales, N. J. (2006). Group-specific primers for amplifying DNA sequences that identify amphipoda, cephalopoda, echinodermata, gastropoda, isopoda, ostracoda and thoracica. Mol. Ecol. 6, 268–271. doi: 10.1111/j.1471-8286.2005.01172.x
Jereb, P., and Roper, C. F. E. (2010). Cephalopods of the world. an annotated and illustrated catalogue of cephalopod species known to date. FAO Species Cat. Fish. Purp. 2:60.
Jeunen, G., Knapp, M., Spencer, H. G., Lamare, M. D., Taylor, H. R., Stat, M., et al. (2019). Environmental DNA (eDNA) metabarcoding reveals strong discrimination among diverse marine habitats connected by water movement. Mol. Ecol. Resour. 19, 426–438. doi: 10.1111/1755-0998.12982
Judkins, H., and Vecchione, M. (2020). Vertical distribution patterns of cephalopods in the northern gulf of mexico. Front. Mar. Sci. 7:47. doi: 10.3389/fmars.2020.00047
Judkins, H. L., Vecchione, M., and Rosario, K. (2016). Morphological and molecular evidence of heteroteuthis dagamensis in the Gulf of Mexico. Bull. Mar. Sci. 91, 51–57. doi: 10.5343/bms.2015.1061
Keeling, R. F., Arne, K., and Gruber, N. (2010). Ocean deoxygenation in a warming world. Annu. Rev. Mar. Sci. 2, 199–229. doi: 10.1146/annurev.marine.010908.163855
Kinzer, J., and Schulz, K. (1985). Vertical distribution and feeding patterns of midwater fish in the central equatorial Atlantic. Mar. Biol. 85, 313–322. doi: 10.1007/BF00393252
Kraus, G. (2005). Biosphere –Hydrosphere – Geosphere Interactions at Seamounts, Cruise No. POS320/2. Bremen, Germany, 57.
Kubodera, T., Koyama, Y., and Mori, K. (2007). Observations of wild hunting behaviour and bioluminescence of a large deep-sea, eight-armed squid, taningia danae. Proc. Biol. Sci. 274, 1029–1034. doi: 10.1098/rspb.2006.0236
Laroche, O., Kersten, O., Smith, C. R., and Goetze, E. (2020). Environmental DNA surveys detect distinct metazoan communities across abyssal plains and seamounts in the western clarion clipperton zone. Mol. Ecol. 29, 4588–4604. doi: 10.1111/mec.15484
Levin, L. A., Etter, R. J., Rex, M. A., Gooday, A. J., Smith, C. R., Pineda, J., et al. (2001). Environmental influences on regional deep-sea species diversity. Annu. Rev. Ecol. Syst. 32, 51–93. doi: 10.1146/annurev.ecolsys.32.081501.114002
Levin, L. A., and Le Bris, N. (2015). The deep ocean under climate change. Science 350, 766–768. doi: 10.1126/science.aad0126
Lindgren, A. R. (2010). Systematics and distribution of the squid genus Pterygioteuthis (cephalopoda: oegopsida) in the eastern tropical pacific ocean. J. Molluscan Stud. 76, 389–398. doi: 10.1093/mollus/eyq028
Macpherson, E., and Raventos, N. (2006). Relationship between pelagic larval duration and geographic distribution of mediterranean littoral fishes. Mar. Ecol. Prog. Ser. 327, 257–265.
Martin, B., and Christiansen, B. (1997). Diets and standing stocks of benthopelagic fishes at two bathymetrically different midoceanic localities in the northeast Atlantic. Deep Sea Res. Part Oceanogr. Res. Pap. 44, 541–558. doi: 10.1016/S0967-0637(97)00008-3
Martin, M. (2011). Cutadapt removes adapter sequences from high-throughput sequencing reads. EMBnetjournal 17:200. doi: 10.14806/ej.17.1.200
Martins, R. S., Roberts, M. J., Lett, C., Chang, N., Moloney, C. L., Camargo, M. G., et al. (2014). Modelling transport of chokka squid (Loligo reynaudii) paralarvae off South Africa: reviewing, testing and extending the ‘westward transport hypothesis.’. Fish. Oceanogr. 23, 116–131. doi: 10.1111/fog.12046
Merten, V., Christiansen, B., Javidpour, J., Piatkowski, U., Puebla, O., Gasca, R., et al. (2017). Diet and stable isotope analyses reveal the feeding ecology of the orangeback squid Sthenoteuthis pteropus (steenstrup 1855) (mollusca, ommastrephidae) in the eastern tropical Atlantic. PLoS One 12:e0189691. doi: 10.1371/journal.pone.0189691
Moiseev, S. I. (1991). Observation of the vertical distribution and behavior of nektonic squids using manned submersibles. Bull. Mar. Sci. 49, 446–456.
Moranta, J., Stefanescu, C., Massutí, E., Morales-Nin, B., and Lloris, D. (1998). Fish community structure and depth-related trends on the continental slope of the Balearic Islands (algerian basin, western mediterranean). Mar. Ecol. Prog. Ser. 171, 247–259.
Murali, A., Bhargava, A., and Wright, E. S. (2018). IDTAXA: a novel approach for accurate taxonomic classification of microbiome sequences. Microbiome 6:140. doi: 10.1186/s40168-018-0521-5
Nesis, K. N. (1987). Cephalopods of the World: Squids, Cuttlefishes, Octopuses and Allies. Neptune, NJ: TFH Publications.
Nesis, K. N., Nigmatullin, C. H. M., and Nikitina, I. V. (1998). Spent females of deepwater squid Galiteuthis glacialis under the ice at the surface of the weddell sea (Antarctic). J. Zool. 244, 185–200. doi: 10.1111/j.1469-7998.1998.tb00024.x
Oksanen, J. F., Blanchet, G., Friendly, M., Kindt, R., Legendre, P., McGlinn, D., et al. (2019). vegan: Community Ecology Package. R Package Version 25-6.
Okutani, T., and Satake, Y. (1978). Squids in the diet of 38 sperm whales caught in the pacific off northern Honshu, Japan, February 1977. Bull. Tokai Reg. Fish. Res. Lab. 93, 13–27.
Osterhage, D., MacIntosh, H., Althaus, F., and Ross, A. (2020). Multiple observations of bigfin squid (Magnapinna sp.) in the great australian bight reveal distribution patterns, morphological characteristics, and rarely seen behaviour. PLoS One 15:e0241066. doi: 10.1371/journal.pone.0241066
Papiol, V., Cartes, J. E., Fanelli, E., and Maynou, F. (2012). Influence of environmental variables on the spatio-temporal dynamics of bentho-pelagic assemblages in the middle slope of the Balearic Basin (NW mediterranean). Deep Sea Res. Part Oceanogr. Res. Pap. 61, 84–99. doi: 10.1016/j.dsr.2011.11.008
Pereira, J. G., Goncalves, J. M., and Clarke, M. R. (2016). Cephalopod identification keys to histioteuthidae, cranchiidae and octopodiformes of the azores, with an updated check-list. Arquipelago Life Mar. Sci. 33, 1–12.
Piatkowski, U., Pierce, G. J., and Morais da Cunha, M. (2001). Impact of cephalopods in the food chain and their interaction with the environment and fisheries: an overview. Fish. Res. 52, 5–10. doi: 10.1016/S0165-7836(01)00226-0
Pinfield, R., Dillane, E., Runge, A. K. W., Evans, A., Mirimin, L., Niemann, J., et al. (2019). False−negative detections from environmental DNA collected in the presence of large numbers of killer whales (Orcinus orca). Environ. DNA 1, 316–328. doi: 10.1002/edn3.32
Quetglas, A., Fliti, K., Massutí, E., Refes, W., Guijarro, B., and Zaghdoudi, S. (2006). First record of taningia danae (cephalopoda: octopoteuthidae) in the Mediterranean Sea. Sci. Mar. 70, 153–155. doi: 10.3989/scimar.2006.70n1153
R Core Team (2018). R: A Language and Environment for Statistical Computing. Vienna Austria: R Found. Stat. Comput.
Ramirez-Llodra, E., Brandt, A., Danovaro, R., De Mol, B., Escobar, E., German, C. R., et al. (2010). Deep, diverse and definitely different: unique attributes of the world’s largest ecosystem. Biogeosciences 7, 2851–2899. doi: 10.5194/bg-7-2851-2010
Roberts, M. J., and van den Berg, M. (2005). Currents along the tsitsikamma coast, South Africa, and potential transport of squid paralarvae and ichthyoplankton. Afr. J. Mar. Sci. 27, 375–388. doi: 10.2989/18142320509504096
Roberts, M. J., Zemlak, T., and Connell, A. (2011). Cyclonic eddies reveal oegopsida squid egg balloon masses in the Agulhas current, South Africa. Afr. J. Mar. Sci. 33, 239–246. doi: 10.2989/1814232X.2011.600294
Robison, B. (2009). Conservation of deep pelagic biodiversity. Conserv. Biol. 23, 847–858. doi: 10.1111/j.1523-1739.2009.01219.x
Robison, B. H., Reisenbichler, K. R., and Sherlock, R. E. (2017). The coevolution of midwater research and ROV technology at MBARI. Oceanography 30, 26–37.
Rodhouse, P. G. K., Pierce, G. J., Nichols, O. C., Sauer, W. H. H., Arkhipkin, A. I., Laptikhovsky, V. V., et al. (2014). Environmental effects on cephalopod population dynamics: implications for management of fisheries. Adv. Mar. Biol. 67, 99–233. doi: 10.1016/b978-0-12-800287-2.00002-0
Roper, C. F. E., and Vecchione, M. (1993). A geographic and taxonomic review of taningia danae Joubin, 1931 (cephalopoda: octopoteuthidae), with new records and observations on bioluminescence. Recent Adv. Cephalop. Fish. Biol. 1376, 441–456.
Roper, C. F. E., and Vecchione, M. (1996). In situ observations on Brachioteuthis beanii verrill: paired behavior, probably mating (cephalopoda, oegopsida). Am. Malacol. Bull. 13, 55–60.
Rosa, R., Dierssen, H. M., Gonzalez, L., and Seibel, B. A. (2008). Large-scale diversity patterns of cephalopods in the Atlantic open ocean and deep sea. Ecology 89, 3449–3461. doi: 10.1890/08-0638.1
Rosa, R., and Seibel, B. A. (2010). Metabolic physiology of the humboldt squid, Dosidicus gigas: implications for vertical migration in a pronounced oxygen minimum zone. clim. impacts ocean. top predat. Cliotop 86, 72–80. doi: 10.1016/j.pocean.2010.04.004
Rowell, T. W., Trites, R. W., and Dawe, E. G. (1985). Distribution of short-finned squid (Illex illecebrosus) larvae and juveniles in relation to the gulf stream frontal zone between florida and cape hatteras. NAFO Sci. Counc. Stud. 9, 77–92.
Roxburgh, S. H., Shea, K., and Wilson, J. B. (2004). The intermediate disturbance hypothesis: patch dynamics and mechanisms of species coexistence. Ecology 85, 359–371. doi: 10.1890/03-0266
Saito, H., and Kubodera, T. (1993). “Distribution of Ommastrephid rhynchoteuthion paralarvae (mollusca, cephalopoda) in the kuroshio region,” in Recent Advances in Fisheries Biology, eds T. Okutani, R. K. O’Dor, and T. Kubodera (Tokyo: Tokai University Press), 457–466.
Sampaio, E., Barreiros, J. P., and Rosa, R. (2018). A potential new endemism: speciation of the common octopus, Octopus vulgaris, in the desertas Islands, cabo verde? Zool. Caboverdiana 7, 39–47. doi: 10.7934/P3289
Schlining, B., and Jacobsen, S. N. (2006). MBARI’s video annotation and reference system. Proc. Mar. Technol. Soc. Electr. Electron. Eng. Oceans Conf. Boston Mass. 2006, 1–5.
Schmidtko, S., Stramma, L., and Visbeck, M. (2017). Decline in global oceanic oxygen content during the past five decades. Nature 542, 335–339. doi: 10.1038/nature21399
Sigsgaard, E. E., Nielsen, I. B., Carl, H., Krag, M. A., Knudsen, S. W., Xing, Y., et al. (2017). Seawater environmental DNA reflects seasonality of a coastal fish community. Mar. Biol. 164:128. doi: 10.1007/s00227-017-3147-4
Smale, M. J., and Clarke, M. R. (1996). Cephalopods as prey. IV. Fishes. Philos. Trans. R. Soc. Lond. B. Biol. Sci. 351, 1067–1081. doi: 10.1098/rstb.1996.0094
Smith, K. F., and Brown, J. H. (2002). Patterns of diversity, depth range and body size among pelagic fishes along a gradient of depth. Glob. Ecol. Biogeogr. 11, 313–322. doi: 10.1046/j.1466-822X.2002.00286.x
Stenvers, V. I., Hauss, H., Osborn, K. J., Neitzel, P., Merten, V., Scheer, S., et al. (2021). Distribution, associations and role in the biological carbon pump of Pyrosoma atlanticum (tunicata, thaliacea) off cabo verde, NE Atlantic. Sci. Rep. 11:9231. doi: 10.1038/s41598-021-88208-5
Stockton, W. L., and DeLaca, T. E. (1982). Food falls in the deep sea: occurrence, quality, and significance. Deep Sea Res. Part Oceanogr. Res. Pap. 29, 157–169. doi: 10.1016/0198-0149(82)90106-6
Sutton, T. T. (2013). Vertical ecology of the pelagic ocean: classical patterns and new perspecitves. J. Fish Biol. 83, 1508–1527. doi: 10.1111/jfb.12263
Sweeney, M. J., and Roper, C. F. E. (1998). “Classification, type localities, and type repositories of recent cephalopoda,” in Smithsonian Contributions to Zoology Systematics and Biogeography of Cephalopods, eds N. A. Voss, M. Vecchione, R. B. Toll, and M. J. Sweeney (Washington, DC: Smithsonian Institution Press), 561–599.
Taberlet, P., Coissac, E., Hajibabaei, M., and Rieseberg, L. H. (2012). Environmental DNA. Mol. Ecol. 21, 1789–1793. doi: 10.1111/j.1365-294X.2012.05542.x
Terashima, H., Sato, M., Kawasaki, H., and Thiam, D. (2007). Quantitative biological assessment of a newly installed artifical reef in Yenne, Senegal. Zool. Stud. 46, 69–82.
Thomsen, P. F., Kielgast, J., Iversen, L. L., Møller, P. R., Rasmussen, M., and Willerslev, E. (2012). Detection of a diverse marine fish fauna using environmental DNA from seawater samples. PLoS One 7:e41732. doi: 10.1371/journal.pone.0041732
Thurber, A. R., Sweetman, A. K., Narayanaswamy, B. E., Jones, D. O. B., Ingels, J., and Hansman, R. L. (2014). Ecosystem function and services provided by the deep sea. Biogeosciences 11, 3941–3963. doi: 10.5194/bg-11-3941-2014
Türkay, M. (1982). Marine crustacea decapoda von den kapverdischen inseln mit bemerkungen zur zoogeographie des gebietes. Cour. Forsch Inst. Senckenberg 52, 91–129.
Untergasser, A., Cutcutache, I., Koressaar, T., Ye, J., Faircloth, B. C., Remm, M., et al. (2012). Primer3—new capabilities and interfaces. Nucleic Acids Res. 40:e115. doi: 10.1093/nar/gks596
Vecchione, M. (2019). ROV observations on reproduction by deep-sea cephalopods in the central pacific ocean. Front. Mar. Sci. 6:403. doi: 10.3389/fmars.2019.00403
Vecchione, M., and Young, R. E. (1998). The magnapinnidae, a newly discovered family of oceanic squid (cephalopoda: oegopsida). South Afr. J. Mar. Sci. 20, 429–437.
Vecchione, M., Young, R. E., Guerra, A., Lindsay, D. J., Clague, D. A., Bernhard, J. M., et al. (2001). Worldwide observations of remarkable deep-sea squids. Science 294:2505. doi: 10.1126/science.294.5551.2505
Villanueva, R., Perricone, V., and Fiorito, G. (2017). Cephalopods as predators: a short journey among behavioral flexibilities, adaptions, and feeding habits. Front. Physiol. 8:598. doi: 10.3389/fphys.2017.00598
Villanueva, R., Vidal, E. A. G., Fernández-Álvarez, F. Á, and Nabhitabhata, J. (2016). Early mode of life and hatchling size in cephalopod molluscs: influence on the species distributional ranges. PLoS One 11:e0165334. doi: 10.1371/journal.pone.0165334
Visser, F., Merten, V. J., Bayer, T., Oudejans, M. G., de Jonge, D. S. W., Puebla, O., et al. (2021). Deep-sea predator niche segregation revealed by combined cetacean biologging and eDNA analysis of cephalopod prey. Sci. Adv. 7:eabf5908. doi: 10.1126/sciadv.abf5908
Voss, N. A., Vecchione, M., Toll, R. B., and Sweeney, M. J. (1998). Systematics and Biogeography of Cephalopods. Washington, DC: Smithsonian Institution Press - Smithsonian Contribution to Zoology.
Wada, T., Doi, H., Togaki, D., Kaida, R., Nagano, M., Katano, I., et al. (2020). Exploring a legendary giant squid: an environmental DNA approach. Mar. Biol. 167:160. doi: 10.1007/s00227-020-03773-z
Wirtz, P., Brito, A., Falcón, J., Freitas, R., Fricke, R., Monteiro, V., et al. (2013). The coastal fishes of the cape verde Islands - new records and an annotated check-list: (Pisces). Spixiana 36, 113–142.
Worm, B., Lotze, H. K., and Myers, R. A. (2003). Predator diversity hotspots in the blue ocean. Proc. Natl. Acad. Sci. 100, 9884–9888. doi: 10.1073/pnas.1333941100
Wormuth, J. H., and Roper, C. F. E. (1983). Quantitative sampling of oceanic cephalopods by nets: problems and recommendations. Biol. Oceanogr. 2, 357–377.
Yamamoto, S., Masuda, R., Sato, Y., Sado, T., Araki, H., Kondoh, M., et al. (2017). Environmental DNA metabarcoding reveals local fish communities in a species-rich coastal sea. Sci. Rep. 7:40368. doi: 10.1038/srep40368
Young, R. E., and Harman, R. F. (1985). Early life history stages of enoploteuthid squids (cephalopoda: teuthoidea: enoploteuthidae) from Hawaiian waters. Vie Milieu 35, 181–201.
Zuev, G. V., and Nikolsky, V. N. (1993). Ecological mechanisms related to intraspecific structure of the nektonic squid Sthenoteuthis pteropus (steenstrup). Recent Adv. Fish Biol. 1993, 653–664.
Keywords: environmental DNA (eDNA), cephalopods, biodiversity, Macaronesia, Cabo Verde, metabarcoding, deep sea, in situ observation
Citation: Merten V, Bayer T, Reusch TBH, Puebla O, Fuss J, Stefanschitz J, Lischka A, Hauss H, Neitzel P, Piatkowski U, Czudaj S, Christiansen B, Denda A and Hoving HJT (2021) An Integrative Assessment Combining Deep-Sea Net Sampling, in situ Observations and Environmental DNA Analysis Identifies Cabo Verde as a Cephalopod Biodiversity Hotspot in the Atlantic Ocean. Front. Mar. Sci. 8:760108. doi: 10.3389/fmars.2021.760108
Received: 17 August 2021; Accepted: 04 November 2021;
Published: 29 November 2021.
Edited by:
Jose Angel Alvarez Perez, Universidade do Vale do Itajaí, BrazilReviewed by:
Ian David Tuck, Fisheries New Zealand, Ministry for Primary Industries, New ZealandDavid Stanković, National Institute of Biology, Slovenia
Copyright © 2021 Merten, Bayer, Reusch, Puebla, Fuss, Stefanschitz, Lischka, Hauss, Neitzel, Piatkowski, Czudaj, Christiansen, Denda and Hoving. This is an open-access article distributed under the terms of the Creative Commons Attribution License (CC BY). The use, distribution or reproduction in other forums is permitted, provided the original author(s) and the copyright owner(s) are credited and that the original publication in this journal is cited, in accordance with accepted academic practice. No use, distribution or reproduction is permitted which does not comply with these terms.
*Correspondence: Véronique Merten, dm1lcnRlbkBnZW9tYXIuZGU=