- Marine Ecology and Systematics (MarES), Department of Biology, University of the Balearic Islands, Palma, Spain
The increasing occurrence of heat waves and water turbidity are threats to the persistence of seagrass meadows. Their effects on the productivity of seagrasses and the functioning of their associated microorganisms have not been studied extensively. The purpose of this study is to assess the effects of different light levels and temperatures on Posidonia oceanica; the endemic seagrass species in the Mediterranean Sea, and their N2-fixing community, which contributes importantly to the nitrogen requirements and high productivity of the plants. Aquarium experiments were conducted in winter, when the plants are more vulnerable to changes in temperature, subjecting them to short-term exposures to an ambient (15.5°C) and elevated temperatures (ambient+5.5°C), and at limited (13 μmol photons m−2 s−1) and saturating light conditions (124 μmol photons m−2 s−1). Primary production, chlorophyll content, reactive oxygen species production, polyphenols content, the nifH gene expression, N2 fixation, and alkaline phosphatase activities were measured in different plant tissues. Plants incubated at ambient temperature and high light exhibited an enhanced total chlorophyll production. Significantly higher gross and net primary production rates were also recorded under ambient temperature and high light conditions, which were approximately two-fold compared to the rest of the treatments. The oxidative stress analyses revealed an increased production of reactive oxygen species in young leaves incubated at ambient temperature and saturating light, while the polyphenols content in top leaves was considerably higher under elevated temperatures. In contrast, the N2 fixation and alkaline phosphatase rates were significantly higher under elevated temperature and low light levels. The presence of the N2-fixing phylotypes UCYN-A, -B, and -C was detected through genetic analyses, with UCYN-B demonstrating the highest nifH gene transcription levels at elevated temperatures. These findings emphasize the significant role of irradiance on the productivity of P. oceanica and the temperature dependence of the N2 fixation process in winter.
Introduction
Posidonia oceanica (L.) Delile is an endemic and dominant seagrass species in the Mediterranean Sea, where it forms extensive meadows from the surface down to a maximum depth of about 45 m (Procaccini et al., 2003; Boudouresque et al., 2006). Seagrass meadows play major ecological roles by enhancing biodiversity, supporting high productivity, protecting the geomorphology of the coastline, sequestering global oceanic carbon, and providing a buffering effect against ocean acidification (Duarte et al., 2005; Barbier et al., 2011; Fourqurean et al., 2017; Chou et al., 2018). However, seagrass ecosystems are currently suffering from a worldwide regression in response to several environmental stressors (Orth et al., 2006; Waycott et al., 2009; Marbà and Duarte, 2010). For instance, the reduction in the surface coverage of P. oceanica has been reported to be 34% in the past 50 years (Telesca et al., 2015). Considering that P. oceanica is a climax, slow-growing seagrass species, the natural and anthropogenic perturbations can be particularly critical, as their recovery from perturbations can be very slow or may not even recover at all (Serrano et al., 2011). Among the threats that these ecosystems are facing, eutrophication from the waste waters and aquaculture, shoreline constructions, anchoring and trawling, dredging, introduced species, and climate change (warming and rising of sea-level) are considered the major causes of the decline of P. oceanica meadows over the last decades (Boudouresque et al., 2009; Champenois and Borges, 2018). Most of these impacts potentially or ultimately reduce water transparency and, therefore, the quality and quantity of the irradiance reaching the seagrass canopy (Duarte et al., 2004; Orth et al., 2006). Epiphytic and planktonic algal accumulations from excess anthropogenic nutrients, increased sediment run-off, and resuspension of bottom sediments are primary causes of reduced underwater irradiance in coastal areas (Orth and Moore, 1983; Cambridge et al., 1986; Onuf, 1994). Ocean warming is also regarded as one of the most severe factors of global climate change, expected to cause, under the A1FI (extreme greenhouse gas emissions) scenario, the rise of the ocean surface temperatures between 2.4 and 6.4°C by the year 2100 (IPCC, 2007), along with an increased amplitude and duration of heat waves (abnormally warm seawater episodes reaching up to ~5°C above the mean temperatures recorded) (Marbà and Duarte, 2010; IPCC, 2014; Galli et al., 2017). These changes are predicted to have serious repercussions in the Mediterranean Sea given its confined nature, which makes it more susceptible to temperature increases, with the warming occurring at significantly higher rates compared to open oceans (Diffenbaugh et al., 2007; Vargas-Yáñez et al., 2008; Calvo et al., 2011).
Generally, light and nutrients comprise the energy source and the matter needed for the growth of seagrasses, while the temperature regulates biochemical processes involved in photosynthesis and respiration, thus, predominantly controlling the annual and seasonal production patterns of seagrasses (Lee and Dunton, 1996; Zupo et al., 1997; Lee et al., 2005, 2007). Water temperature and irradiance are usually correlated and display similar seasonal trends, making it difficult to isolate both environmental parameters in relation to seagrass growth and production (Kaldy and Dunton, 2000; Kaldy, 2006). Light requirements for seagrasses are unusually high, being approximately 10–37% of the surface irradiance, compared to the 0.1–1% needed for most of the other marine macrophytes, which is partially attributed to the inefficient carbon concentrating mechanisms for photosynthesis (Invers et al., 2001; Larkum et al., 2006; Zimmerman, 2006). In view of this, seagrasses are highly vulnerable to the deterioration of water clarity, which is evidenced through the reported large-scale losses of meadows worldwide (Dennison et al., 1993; Onuf, 1994; Short and Wyllie-Echeverria, 1996; Erftemeijer et al., 2006). Hence, understanding the light thresholds for seagrass survival is fundamental for the effective management of these valuable habitats (York et al., 2013). Elevated temperatures entail a grand risk of local extinction for cold-adapted plants, such as Mediterranean seagrasses, as they have visibly manifested physiological symptoms of heat stress and of reduced fitness (Beca-Carretero et al., 2018; Marín-Guirao et al., 2018). The consequences of heat waves can be notably damaging on seagrass meadows, promoting shoot mortality and population decline when critical temperature thresholds are surpassed (Díaz-Almela et al., 2009; Marbà and Duarte, 2010; Jordà et al., 2012). In addition, thermal stress can induce the acceleration of the respiration over the photosynthesis rates (Collier and Waycott, 2014; Marín-Guirao et al., 2018), and affect important life-history events, like reproduction, through an increased flowering intensity (Ruiz et al., 2018; Marín-Guirao et al., 2019).
Several studies have been conducted to determine the effects of light reduction on P. oceanica, comparing its response along bathymetric/spatial gradients (Alcoverro et al., 2001; Ruiz and Romero, 2003; Dattolo et al., 2014) and experimentally through the modification of the light environment with shading screens (Ruiz and Romero, 2001; Mazzuca et al., 2009; Serrano et al., 2011; Gacia et al., 2012). Investigations on the impacts of the sea warming, considering the climate change scenarios, have focused on its isolated effects (Marbà and Duarte, 2010; Guerrero-Meseguer et al., 2017; Hernán et al., 2017; Ruiz et al., 2018; Traboni et al., 2018) and the interaction with other stressors (Hendriks et al., 2017; Ontoria et al., 2019; Agawin et al., 2021). However, research on the combined effects of ocean warming and water turbidity in P. oceanica meadows remains relatively scarce, and understanding them is crucial for the future management of these coastal ecosystems. Moreover, no studies have been done, so far, on the effects of these factors on the microorganisms associated with P. oceanica, such as the nitrogen (N2) fixers.
Biological N2 fixation, defined as the enzymatic reduction of atmospheric N2 to ammonium equivalents, takes part in significantly sustaining the high productivity of P. oceanica in the oligotrophic Mediterranean Sea (Garcias-Bonet et al., 2019). Seagrasses harbor diverse communities of epi- and endophytic bacteria associated with their leaves, roots, and rhizomes, that can enhance plant growth through an increased nutrient availability, for instance, via N2 fixation or by mineralizing organic compounds (Uku et al., 2007; Cole and McGlathery, 2012; Garcias-Bonet et al., 2016). In particular, it has been reported that the N2 fixation processes associated with the phyllosphere of P. oceanica could potentially supply the total nitrogen demand of the plant (Agawin et al., 2016, 2017). The enzyme complex that catalyzes the biological N2 fixation process is the nitrogenase, which is composed of two proteins: conventionally, the iron protein or nitrogenase reductase, and the molybdenum iron protein or nitrogenase (Hamisi, 2010). The nifH gene encodes the iron protein, while the nifDK genes encode the molybdenum iron protein (Rubio and Ludden, 2002). Sequencing the nif genes, with nifH being the most sequenced and the marker gene of choice, has allowed the studying of the phylogeny, diversity, and abundance of N2-fixing microorganisms (Gaby and Buckley, 2012). A significant diversity has been identified among marine diazotrophs, with filamentous organisms primarily including the Trichodesmium sp. and the diatom symbiont Richelia intracellularis, and highly diverse unicellular diazotrophs that comprise Cyanobacteria, Proteobacteria, and Archaea (Moal et al., 2011). At present, three groups of unicellular N2-fixing cyanobacteria (UCYN) have been described: UCYN-A, B, and C (Zehr et al., 2001; Foster et al., 2007). Groups B and C are nanoplanktonic cells (2–10 μm), which are closely related to the cultivated strains Crocosphaera watsonii and Cyanothece sp., respectively (Church et al., 2005; Foster et al., 2007); whereas, members of Group A are of picoplanktonic size (0.7–1.5 μm) and uncultivated up until now (Biegala and Raimbault, 2008; Goebel et al., 2008).
Nitrogenase activity is influenced by a combination of environmental factors that differ depending on the geographic region and the diazotroph community composition (Mahaffey et al., 2005). Several studies have suggested a strong temperature dependence of the N2 fixation process at the enzymatic level, and a positive correlation to irradiance (Welsh, 2000; Brauer et al., 2013; Agawin et al., 2017; Garcias-Bonet et al., 2019). Furthermore, as the nitrogenase proteins require iron (Fe), the availability of both phosphorus (P) and Fe are factors that could limit or co-limit the N2 fixation process in some areas of the oceans (Sañudo-Wilhelmy et al., 2001; Karl et al., 2002; Mills et al., 2004). Primary aquatic producers usually contain external alkaline phosphates; enzymes capable of hydrolyzing organic phosphorus compounds (monoester phosphates), which liberate inorganic phosphorus and increase the availability of this nutrient for growth (Kuenzler and Perras, 1965; Martínez-Crego et al., 2006). Thus, the measurement of alkaline phosphatase activity has been employed as an indicator of the limitation of the phosphorus and the deficiency in algae and seagrasses (Pérez and Romero, 1993; Invers et al., 1995; Steinhart et al., 2002; Fernández-Juárez et al., 2019).
This study assesses the response of P. oceanica and its N2-fixing community to different combinations of temperature and light levels, in terms of primary production and respiration rates, chlorophyll content, alkaline phosphatase activity, oxidative stress indicators, and N2 fixation activities of the diazotrophs associated with different plant tissues.
Materials and Methods
Sampling and Experimental Design
To assess the effects of warming and deteriorating light conditions on Posidonia oceanica, as well as their N2 fixing community, aquarium experiments were conducted in winter, simulating combinations of present and future temperatures (IPCC, 2007) with two light conditions. The experiment was performed during winter, when the plants could be thermally more vulnerable to temperature increases (Agawin et al., 2021). The limited (13 μmol photons m−2 s−1) and saturating (124 μmol photons m−2 s−1) light levels, based on the photosynthesis-irradiance parameters documented in the literature for shallow P. oceanica meadows during winter (Alcoverro et al., 1998; Lee et al., 2007), were factorially combined with the ambient temperature corresponding to the average annual temperature in the Balearic Sea at the time of the collection (~15.5°C; SOCIB, 2020) and 5.5°C warmer (21°C). The applied temperature treatment was within the estimated temperature increase range under the A1FI high emissions scenario, with a likely range from 2.4 to 6.4°C (IPCC, 2007; Collins et al., 2013), and may simulate an abnormally warm seawater episode as projected for future heat waves in the Mediterranean Sea (IPCC, 2014; Darmaraki et al., 2019). In December 2020, plants were carefully collected from the coast of Llucmajor (2°44′22.65″E, 39°27′2.36″N; Majorca, Spain; Figure 1), through SCUBA diving at a depth between 4 to 6 m. Seawater was also collected and was immediately prefiltered through a 10 μm nylon Nitex filter, of which 8 L were added to each of 12 aquaria with 9 L of capacity. Three replicate aquaria were employed per treatment and 8–10 shoots of P. oceanica, with roots and part of the rhizome attached, were placed in each aquarium without sediments. The cut end of the horizontal rhizome of each plant was sealed using a non-toxic underwater D-D AquaScape epoxy to maintain the gas pressure inside the rhizome. The experiment was performed in a temperature-controlled room, with a duration of 18 days, in between which the seawater was replaced once on the eighth day to avoid nutrient limitation in the aquaria. The temperature treatments were achieved by respectively connecting each aquarium to water chillers (HAILEA HC-130A), with a continuous circuit of water and heaters (Aquael EasyHeater 25 W), with the desired temperature previously configured in the devices. Aquaria were illuminated by diode lamps (Aquael Leddy Slim Sunny 5 W) installed above, set to 11:13 h light: dark cycles and delivering incident photosynthetically active radiation (PAR) light levels at the seagrass canopy according to the treatments assigned. The partial pressure of the carbon dioxide (CO2) was adjusted through bubbling with an air-CO2 mixture. Atmospheric air was first scrubbed by soda lime to remove all CO2, then mixed with pure CO2 from a bottle using mass flow controllers (Aalborg). To achieve the present-day pCO2 levels, gases were mixed to 435 ppm pCO2 in mixing the bottles filled with marbles to assure the homogenization of gases. In each aquarium, the resulting mixture was regulated by a flow meter with a volume of 2.5 L min−1, and a flux diffuser was placed at the extremes of each tube to release the gases in diffused form.
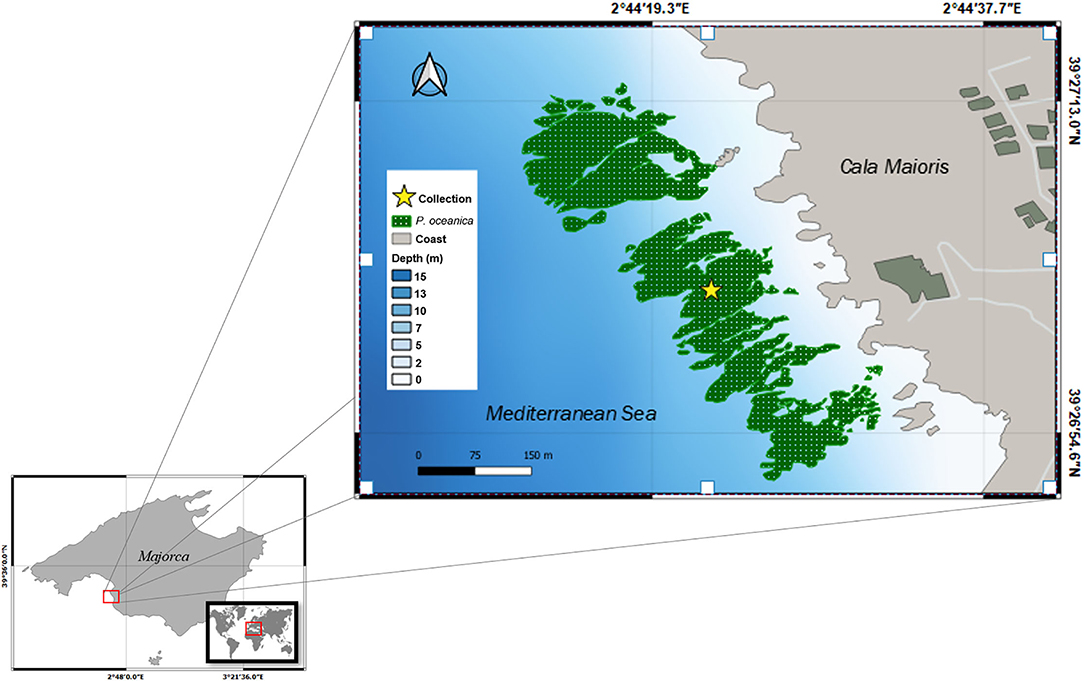
Figure 1. Geographical location of the collection site of Posidonia oceanica in the coast of Llucmajor, Majorca, Spain.
Physicochemical Parameters
The temperature and the pH were continuously monitored and recorded at 30-min intervals using sensors (IKS-Aquastar and ENV-40-pH calibrated with 4.0 and 7.0 pH NBS standards, respectively), connected to a D130 data logger (Consort) and a computer. The daily average PAR was monitored with light loggers (HOBO), which were positioned in the surface water of the aquaria. Due to a limited number of sensors and light loggers, only two replicates per treatment could be simultaneously measured for the indicated parameters. Initially, during and after the incubations, water samples from each aquarium were taken for the determination of nitrite (), nitrate (), ammonium (), phosphate (), and the total dissolved phosphorus (TDP) concentrations. The samples were filtered through sterile polypropylene filter holders (0.2 μm), using a peristaltic pump (Geotech Geopump), and kept frozen until analyzed. The inorganic nutrients samples (, , , and ) were stored in polypropylene tubes, while samples for TDP were deposited in the borosilicate Scott bottles. The concentrations were quantified following the spectrophotometric method of Strickland and Parsons (1972); while a modified protocol, based on Knap et al. (1997) and Weber-Shirk et al. (2001), was applied for determination. The TDP concentrations were also analyzed using the latter method after the persulfate digestion (Bronk et al., 2000). The content was determined by the flow injection analysis, as described by Diamond (2003), and the was measured according to the modified fluorometric method of Horstkotte and Duarte (2012).
Estimation of Primary Production and Respiration Rates
Dissolved oxygen (DO) concentrations were determined spectrophotometrically by the modified Winkler method, according to the protocol described by Labasque et al. (2004). For each aquarium, four Exetainer vials (initial values, n = 4) (12 ml) and two light and dark 125 ml Winkler bottles were filled with water from the aquarium filtered through sterile polypropylene filter holders (0.2 μm) using the peristaltic pump, taking care to avoid bubbles or turbulence when filling. The second youngest leaf of each of four independent shoots per aquarium was selected, cut into a 5 cm segment from the top, and, if necessary, epiphytes were scraped off. Each leaf segment was inserted into the light and dark Winkler bottles to incubate for 3 h inside their respective aquariums. For the phyllosphere measurements, two Erlenmeyer flasks were filled with 480 ml of filtered water from each aquarium and autoclaved, then a P. oceanica shoot, without roots and rhizomes, was introduced per flask and incubated as previously mentioned. After the incubation period, Exetainers were filled with the water from the Winkler bottles and the flasks until they overflowed, using the syringe with the attached tube to avoid gas exchange as much as possible. Immediately, 80 μL of MnCl2 (3 M), 80 μL of NaOH (8 M), and NaI (4 M) were added to the vials. Exetainers were tightly closed, agitated, and kept in cold and dark conditions until DO determination (between 24 and 48 h). The DO concentrations were estimated spectrophotometrically at 466 nm after adding 80 μL H2SO4 (10 M). The increase or the decrease of DO concentrations during the incubation period provided measures of net primary production (NPP) and respiration (R) in the light and dark bottles, respectively. Subsequently, the gross primary production (GPP) was calculated by summing the net photosynthetic rates obtained with the rate of dark respiration (GPP = NPP+R). The estimated changes in DO from the Erlenmeyer flasks provided the NPP of the P. oceanica phyllosphere. These values were normalized to the incubation time, volume of water, and the dry weight of the incubated tissue (μmg O2 g DW−1 h−1).
Determination of Chlorophyll Concentrations
Leaf chlorophyll concentrations in duplicate P. oceanica shoots from each aquarium were measured following Agawin et al. (1996). The extraction of chlorophyll a and b from the seagrass leaves was done by grinding about 0.1 to 0.3 g wet weight of the second youngest leaf per shoot, with a mortar and pestle in 96% ethanol. After extraction in the dark for 12 h, the suspensions were centrifuged at 2,800 × g for 10 min. Absorbances were measured at 665 and 649 nm using a Cary-50 Conc-UV Visible spectrophotometer. Afterward, chlorophyll a and b concentrations were determined using the formula of Wintermans and De Mots (1965).
Quantification of Alkaline Phosphatase Activity
Alkaline phosphatase activity (APA) was evaluated through a fluorometric assay, in which the hydrolysis of the fluorogenic substrate (S) 4-methylumbelliferyl phosphate (MUF-P, Sigma-Aldrich Química S.L.U./Merck Life Science S.L.U., Madrid, Spain) to 4-methylumbelliferyl (MUF) was measured (Fernández-Juárez et al., 2019). The second oldest and youngest leaf of each of two independent shoots per aquarium were selected and cut into a 5 cm segment from the top. From each of the two independent shoots, 5 cm pieces of unrinsed rhizomes and roots were also extracted. The leaf and root segments were inserted into 15 ml centrifuge tubes with 10 ml of filtered and autoclaved water from their respective aquariums, while the rhizomes were introduced into 50 ml Falcon centrifuge tubes with 40 ml of the water. Then, the MUF-P reagent at 2 μM of final concentration was added to each tube. After 1 h incubation in darkness at room temperature, APA was measured in a microtiter plate that contained borate buffer at pH 10 (3:1 of sample: buffer). The MUF production (μM MUF g DW−1 h−1) was measured with a Cary Eclipse spectrofluorometer (FL0902M009, Agilent Technologies Spain, S.L., Madrid, Spain) at 359 nm (excitation) and 449 nm (emission), and also using a calibration standard curve with commercial MUF (Sigma-Aldrich Química S.L.U./Merck Life Science S.L.U., Madrid, Spain).
Reactive Oxygen Species Production
Prior to biochemical analysis, P. oceanica leaves were carefully separated from the epiphytes. The leaf segments per aquarium were washed with distilled water to eliminate salt residues, then triturated in a mortar with a pestle in the presence of liquid nitrogen. The samples were homogenized in five volumes (w/v) of 50 mM Tris-HCl buffer, 1 mM EDTA, pH 7.5. Next, the solutions were homogenized in ice by employing a homogenizer, with a velocity set between 4-6, for a few minutes. Homogenates were centrifuged at 9000 × g at 4°C for 4 min to remove cell debris, nuclei, and mitochondria, and the supernatants were used for biochemical assays. All biochemical analyses were expressed per mg protein, measured by using the colorimetric Thermo Scientific Coomassie (Bradford), Illinois, United States Protein Assay Kit, with Bovine Serum Albumin (BSA) as a standard. The reactive oxygen species (ROS) production was measured using the molecular probe 2′,7′-dichlorofluorescein diacetate (DCFH-DA; Sigma-Aldrich Química S.L.U./Merck Life Science S.L.U., Madrid, Spain) in a filtered and autoclaved seawater, which was added to a 96-well microplate (Thermo Scientific, Fisher Scientific S.L., Madrid, Spain) containing the supernatant samples (final concentration of probe at 15 μg ml−1). This compound is intracellularly hydrolyzed by esterases to a non-fluorescent 2′,7′-dichlorodihydrofluorescin (DCFH), which is subsequently oxidized by ROS to a highly green-fluorescent 2′,7′-dichlorodihydrofluorescein (DCF) (Kumar et al., 2018). The fluorescence was measured at 25 °C in an FLx800 Microplate Fluorescence Reader (BioTek Instruments, Inc., Fisher Scientific S.L., Madrid, Spain) for 1 h, with an excitation of 480 nm and an emission of 530 nm. The measurements were obtained from the slope of the linear regression between the fluorescence readings and time, and expressed as arbitrary units (AU). The DCFH-DA was added in filtered and autoclaved seawater without sample as blanks under the same conditions stated above.
Phenolic Compounds Quantification
The total phenolic content of the P. oceanica extracts was estimated by the Folin-Ciocalteau colorimetric assay (Singleton et al., 1999). Briefly, 10 μl of the extracted sample was mixed with 10 μl of 2 N Folin-Ciocalteu reagent, 50 μl of 20% (w/v) sodium carbonate (Na2CO3), and 250 μl of distilled water. After incubation at room temperature for 90 min, absorbance was measured at 760 nm (UV-visible spectrophotometer Cary 100 Conc, Varian, Agilent Technologies Spain, S.L., Madrid, Spain). A calibration curve was built by using tyrosine as the standard, while the total phenolic content was expressed as mg of tyrosine/mg of protein. All determinations were carried out in duplicate per aquarium.
Measurement of N2 Fixation Rates
The N2 fixation rates were measured in the different plant tissues of P. oceanica using the acetylene reduction assay (ARA) (Stal, 1988; Capone, 1993; Agawin et al., 2014). The second oldest leaf, rhizomes, and roots of each of two independent shoots per aquarium were selected and cut into 5 cm segments. Additional 5 cm pieces of roots were also extracted from independent shoots for surface-sterilization by a series of sterilization steps [(i.e., 99% ethanol 1 min; 3.125% NaOCl 6 min; 99% ethanol 30 s; autoclaved GF/F filtered seawater final washing) (Coombs and Franco, 2003)], to measure the root endophyte N2 fixation rates. Each plant tissue was inserted into its respective incubation vial. Leaves and roots were inserted into 10 ml gas chromatograph (GC) vials, as well as the rhizomes into 50 ml Falcon centrifuge tubes. Each incubation vial or tube was humidified with 1 ml (for the GC vials) and with 2.5 ml (for the Falcon tubes) of sterilized GF/F filtered seawater. All vials and tubes were capped with gas-tight septum ports. Vials and tubes containing the rhizomes and roots were flushed with helium gas for 1 min to obtain anoxic conditions. Each incubation vial or tube was injected with the volume of acetylene gas at 20% (v/v) using gas-tight Hamilton syringes and then incubated for 3 h in their respective aquarium. Immediately after the incubation time, 10 ml of headspace was taken using a gas-tight Hamilton syringe from the incubation vials or tubes, transferred to Hungate tubes, and sealed with hot melted glue (SALKI, ref. 0430308, Grupodesa Fasteners, S.A.U., Barcelona, Spain) to avoid possible gas losses as much as possible (Agawin et al., 2014). Ethylene and acetylene were determined using a gas chromatograph (7890A, Agilent Technologies Spain, S.L., Madrid, Spain), equipped with a flame ionization detector. The column was a Varian wide-bore column (ref. CP7584) packed with CP-PoraPLOT U (27.5 m length, 0.53 mm inside diameter, 0.70 mm outside diameter, and 20 μm film thickness). Helium was used as the carrier gas at a flow rate of 30 ml min−1. Hydrogen and airflow rates were set at 30 ml min−1 and 365 ml min−1, respectively. The split flow was used so that the carrier gas flowing through the column was 4 ml min−1 at a pressure of 5 psi. The oven, injection, and detector temperatures were set at 52, 120, and 170°C, respectively. The amount of ethylene produced was obtained following the equations in Stal (1988). The acetylene reduction rates were converted to N2 fixation rates using a factor of 4:1 (C2H4:N2 reduced; Jensen and Cox, 1983) and reported per g dry weight of incubated plant biomass. The dry weight of the plant parts was determined by drying the plant parts at 60°C for 24 h (Short and Duarte, 2001).
Quantification of the nifH Gene Expression in the Phyllosphere of Posidonia oceanica
After the incubations, for the extraction of the epiphytic community in P. oceanica, the leaf segments from each aquarium were placed onto clean glass slides, then scraped on both sides with new sterile disposable scalpel blades (#10). The epiphytes obtained per aquarium were transferred into Eppendorf tubes with 1 ml of phosphate-buffered saline (PBS) solution, to remove salt residues that could interfere during the RNA extraction process, and then centrifuged at 13,000 × g for 15 min. The RNA extraction and purification were done with the Plant/Fungi Total RNA Purification Kit (Norgen, Cat. 25800, 31350, 25850, Norgen Biotek Corp., Ontario, Canada), following the manufactures protocol. The quality and quantity of the extractions (absence of DNA and protein contaminations) were assessed using NanoDrop (Thermo Fisher Scientific, Fisher Scientific S.L., Madrid, Spain). The expression of the nifH gene was assessed by a Reverse Transcription-quantitative Polymerase Chain Reaction (RT-qPCR), as described by Goebel et al. (2010), Moisander et al. (2010), and Turk-Kubo et al. (2012), considering primer sets designed for N2 fixing communities belonging to the Groups A, B, and C of unicellular cyanobacteria, the filamentous cyanobacteria genera Trichodesmium, and alpha-proteobacteria. The assays were performed in the LightCycler 480 Instrument II - Roche Life Science (Roche Diagnostics, S.L., Barcelona, Spain), using the Luna Universal One-Step RT-qPCR Kit (New England Biolabs, Inc., Massachusetts, United States). All the RT-qPCR reactions were carried out in triplicates to capture the intra-assay variability, and each assay included three no-template negative controls for each pair of primer. The cycle threshold (CT) values were used to calculate the number of gene copies per sample, based on the standard curves for each primer set, and normalized to the total RNA content.
Data and Statistical Analyses
Data are presented as mean ± standard deviation of the replicates from the treatments (n = 3). Prior to the statistical analyses, data were tested for normality using the Shapiro-Wilk (n <50) and Kolmogorov-Smirnov (n > 50) goodness of fit tests, while the homoscedasticity was assessed with Levene's test, and then log-transformed if necessary. One-way ANOVA was used to test the hypothesis that GPP, NPP, and respiration rates of P. oceanica vary among the different treatments. The effect of the treatments on chlorophyll content was examined through linear mixed models (LMM), including the aquaria as a random factor. For the remaining biological parameters (APA rates, ROS production, polyphenols content, N2 fixation, and nifH expression), LMM were also executed to evaluate possible differences among treatments and plant tissues, with the aquaria as a random factor, while considering the interaction between fixed factors. Finally, post-hoc analyses were performed with Tukey's test for multiple comparisons of means. The statistical analyses were performed using the R package, version 4.0.3.
Results
Physicochemical Parameters
The mean temperature of the aquaria at ambient and elevated temperature corresponded to 15.70 ± 0.47 and 21.48 ± 0.57°C, respectively. On average, the low and high light treatments differed, although not significantly, at ambient temperature with 0.86 ± 0.07 °C, and 0.82 ± 0.17 °C under elevated temperature, with the high light treatments reaching slightly higher values in both cases (Figure 2A). The pH of tanks receiving the low light exhibited a lower mean (7.82 ± 0.08) compared to those subjected to high light conditions (8.04 ± 0.11). The temporal fluctuations of the pH in all treatments are shown in Figure 2B, with diurnal changes of approximately 0.09 to 0.42 units. Regarding PAR values, the low and high light treatments were daily exposed to an average of 11.69 ± 2.45 and 126.29 ± 8.77 μmol photons m−2 s−1, respectively (Figure 2C). In the performed nutrient analyses, a decrease in the and concentrations of all treatments was evidenced toward the end of the experiment (Table 1), while the , , and TDP values were lower compared to the initial phase only before the water replacement. Furthermore, at the final stage of the incubations, concentrations were higher in all treatments and the was higher in aquaria under saturating light conditions, in comparison to the values obtained at the intermediate water replacement. A slight decrease in the concentrations was observed before the water replacement in the ambient temperature only with the high light treatment, while the TDP concentrations increased toward the end of the experiment in tanks under ambient temperature with low light and elevated temperature with high light. Nonetheless, the differences between treatments in the concentrations of all the nutrients analyzed at the end of the experiment were not significant (p > 0.05).
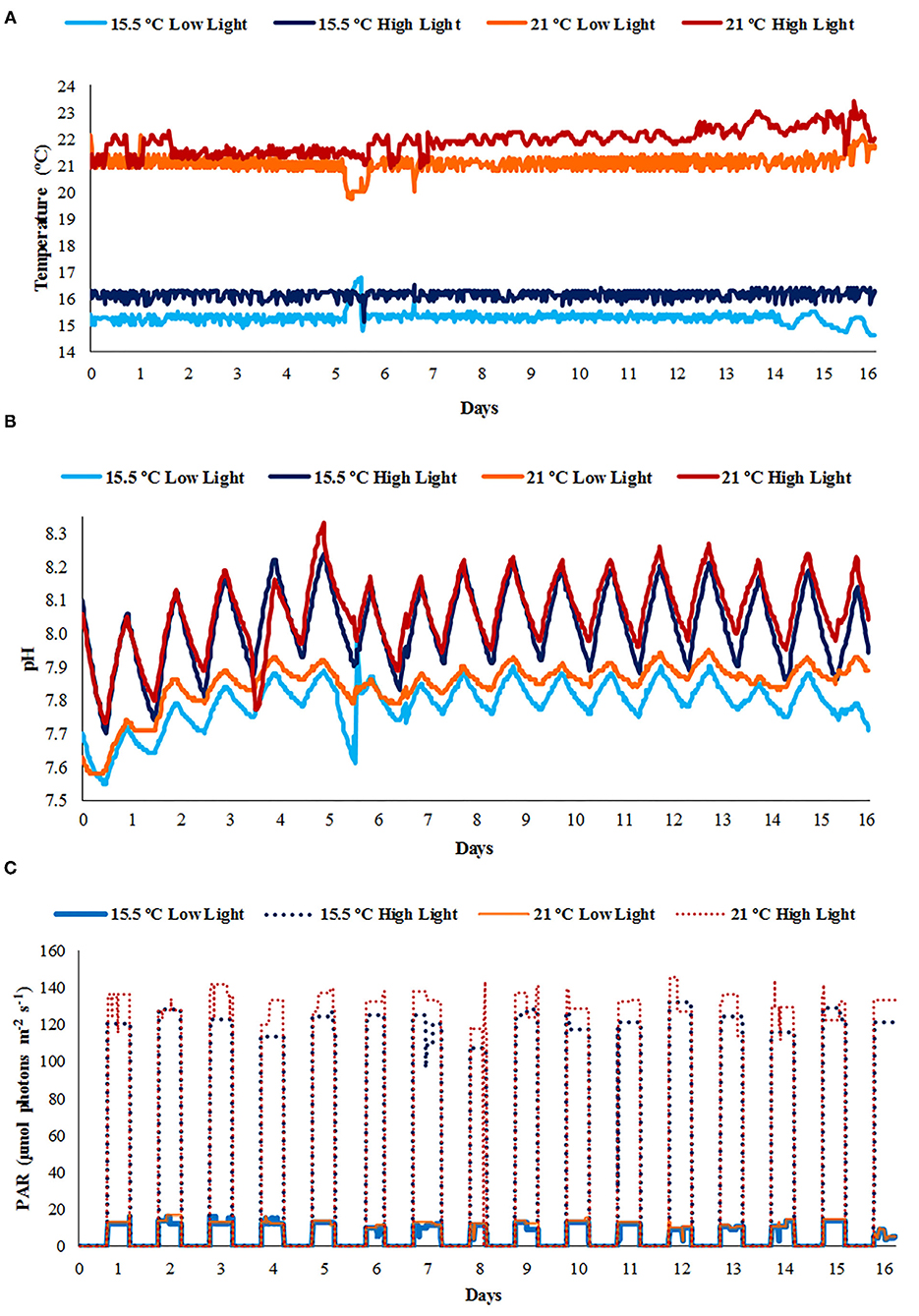
Figure 2. The diurnal variations of (A) temperature, (B) pH, and (C) photosynthetically active radiation (PAR) values measured during the experiment.
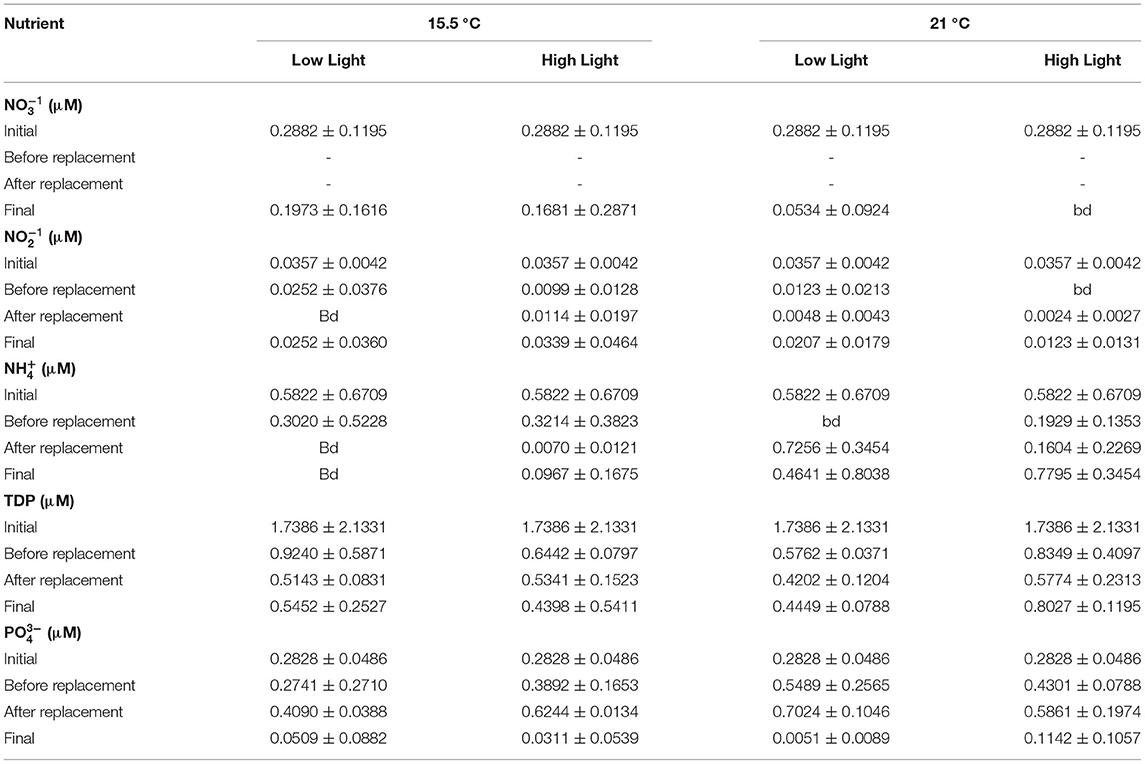
Table 1. The average (± SD, n = 3) concentrations of nitrate (), nitrite (), ammonium (), total dissolved phosphorus (TDP), and phosphate () during the course of the experiment, including the initial at day zero, before and after water replacement at day eight, and final at day 18 (bd: below detection).
Primary Production and Respiration Rates
The average GPP rates of the cut leaf segments were significantly higher (p < 0.05; Supplementary Table 1) under high light conditions at ambient temperature (9.61 ± 1.62 mg O2 g DW−1 h−1), compared with the low light treatments (15.5 °C = 5.32 ± 1.84 mg O2 g DW−1 h−1; 21 °C = 5.77 ± 0.59 mg O2 g DW−1 h−1) (Figure 3A). Similar results were obtained for the whole phyllosphere, where the mean NPP rates were significantly higher (p < 0.01) at saturating light conditions (21°C = 0.90 ± 0.60 mg O2 g DW−1 h−1; 15.5°C = 0.50 ± 0.23 mg O2 g DW−1 h−1), compared to the limited light treatment at an elevated temperature (0.11 ± 0.09 mg O2 g DW−1 h−1) (Figure 3B). The leaves incubated at an ambient temperature and a high light reached the highest NPP rates (p < 0.05), with a mean value of 6.07 ± 1.42 mg O2 g DW−1 h−1, which is approximately two-fold in comparison to the remaining treatments. Although the high light treatments exhibited the highest respiration rates, at elevated (−3.64 ± 2.13 mg O2 g DW−1 h−1) and ambient temperatures (-3.54 ± 3.03 mg O2 g DW−1 h−1), respectively, these values did not differ significantly from the remaining treatments (p > 0.05).
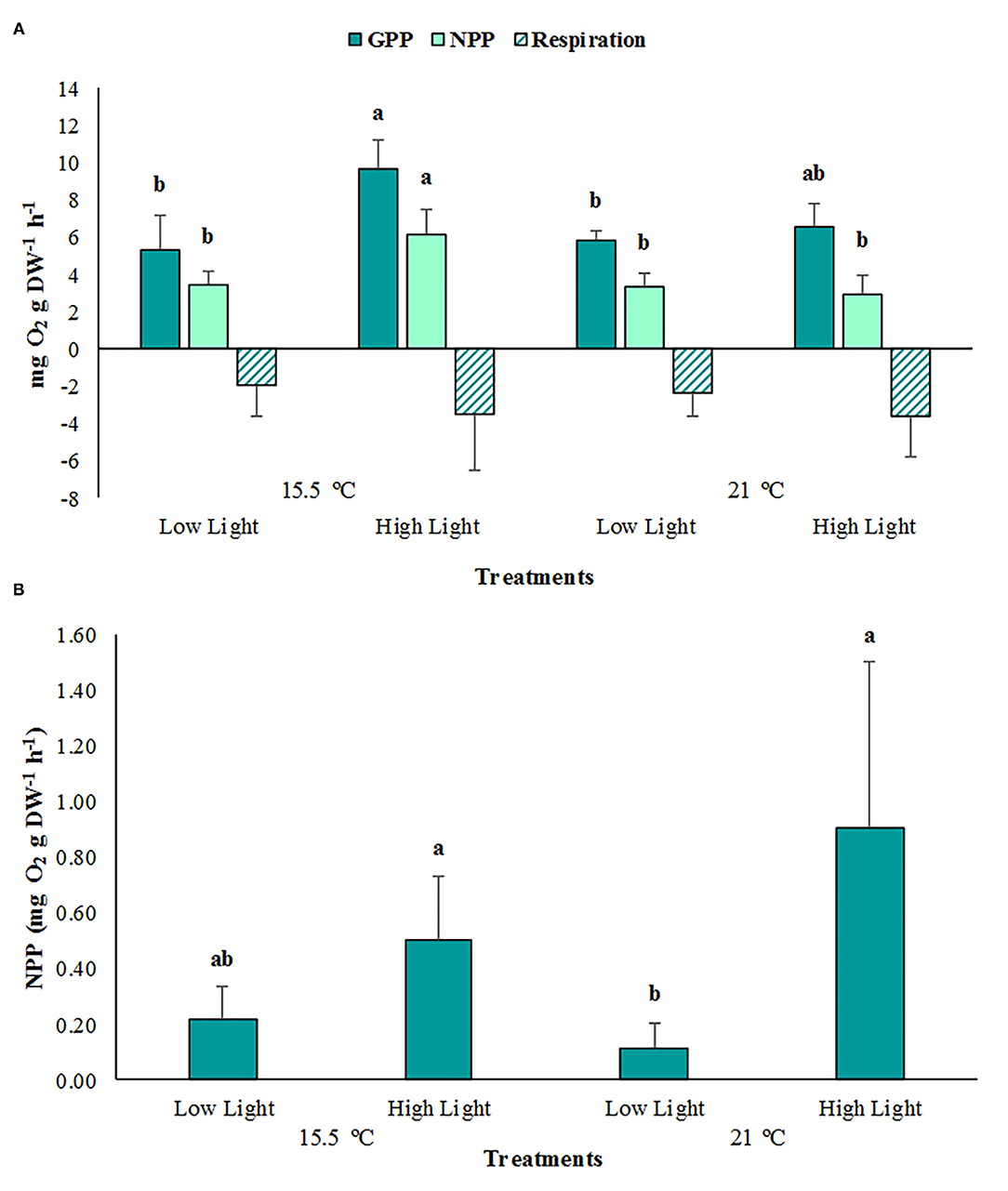
Figure 3. (A) The average gross primary production (GPP), net primary production (NPP), and respiration rates of Posidonia oceanica cut leaf segments in the different treatments (n = 3). (B) The average NPP rates of the P. oceanica phyllosphere in the different treatments (n = 3). The error bars represent ± SD. Different letters denote significant differences (p < 0.05) among treatments (Tukey's post-hoc test following the respective analysis of variance/deviance in Supplementary Table 1).
Chlorophyll Concentrations
Total chlorophyll concentrations, as well as chlorophyll a and b, demonstrated a corresponding trend with primary production, with considerably enhanced mean values (p < 0.001; Supplementary Table 2), under high light conditions at ambient (Total Chl = 329,67 ± 47.91; Chl a = 194.46 ± 22.91; Chl b = 135.21 ± 27.83 μg Ch WW−1) and elevated (Total Chl = 259.98 ± 26.49; Chl a = 152.88 ± 13.98; Chl b = 107.10 ± 17.23 μg g WW−1) temperatures, respectively (Figure 4).
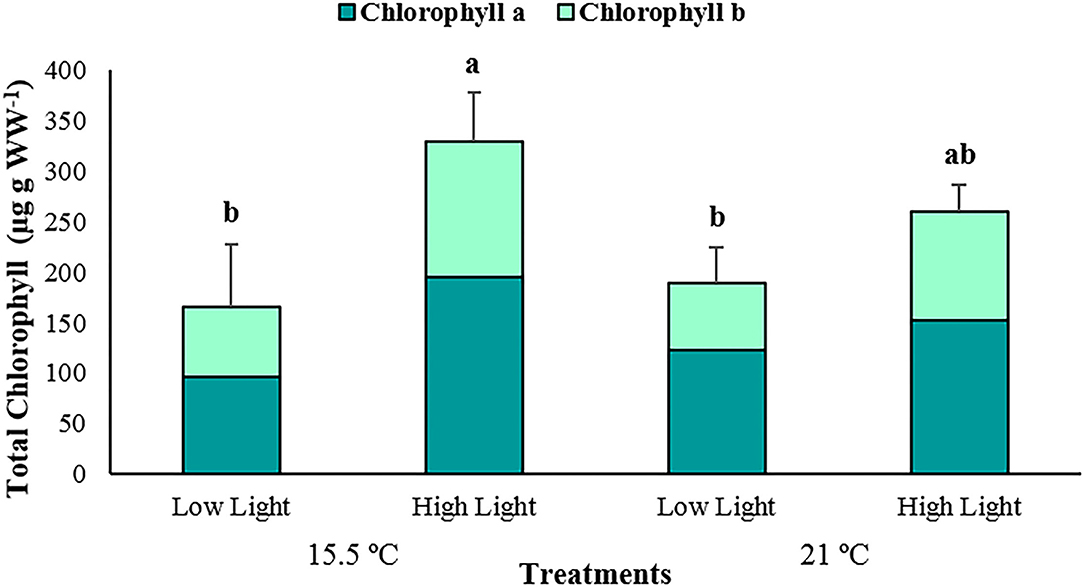
Figure 4. The average total chlorophyll content of Posidonia oceanica leaves in the different treatments (n = 5). The error bars represent ± SD. Different letters denote significant differences (p < 0.001) among treatments (Tukey's post-hoc test following the analysis of deviance in Supplementary Table 2).
Alkaline Phosphatase Activity
In general, APA rates differed significantly among treatments (p < 0.05) and plant tissues (p < 0.001), but were homogeneous between the interactions of these fixed factors (p > 0.05; Supplementary Table 3). The highest mean of APA was recorded at elevated temperature and low light conditions (5.36 ± 4.07 μM MUF g DW−1 h−1), while the lowest mean corresponded to the high light treatment under equal temperature (2.80 ± 2.26 μM MUF g DW−1 h−1). However, at the ambient temperature treatments, the values of this parameter did not significantly deviate from the rest (Figure 5). Regarding the plant tissues, the rhizomes showed considerably lower APA rates (0.46 ± 0.33 μM MUF g DW−1 h−1) in comparison to the leaves and roots.
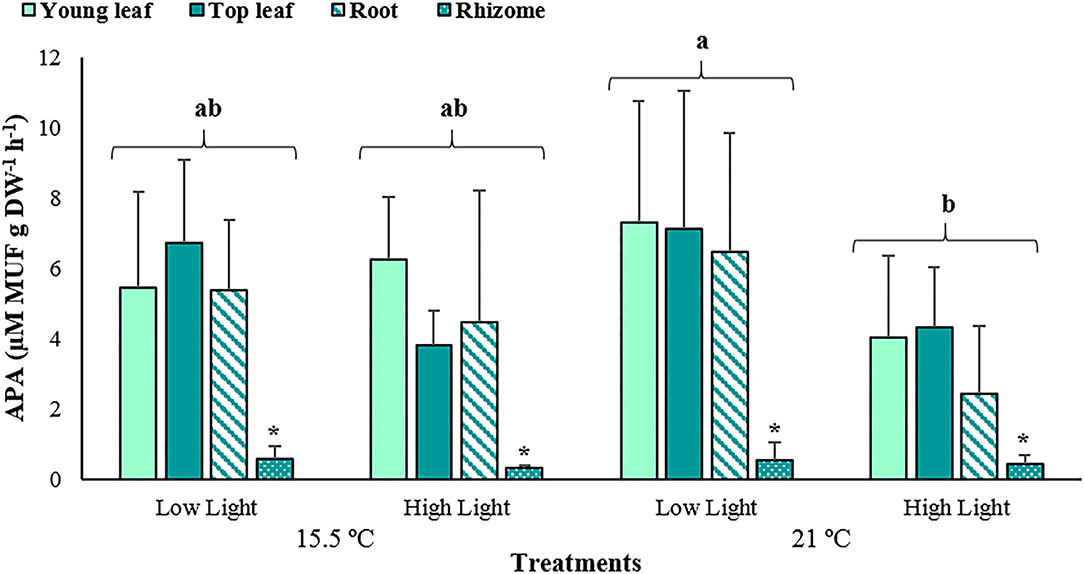
Figure 5. The average alkaline phosphatase activity (APA) associated with young leaves, top leaves, roots, and rhizomes of Posidonia oceanica incubated at the different treatments (n = 6). The error bars represent ± SD. Different letters denote significant differences (p < 0.05) among treatments, and the asterisk (*) between plant tissues (p < 0.001) (Tukey's post-hoc test following the analysis of deviance in Supplementary Table 3).
Reactive Oxygen Species and Phenolic Compounds
The reactive oxygen species (ROS) production varied significantly among the treatments depending on the plant tissue (p < 0.01; Supplementary Table 4), with increased values at ambient temperature and high light conditions for the young leaves only (87.87 ± 63.33 a.u. mg protein−1, Figure 6). Additionally, it can be observed that the top leaves produced greater quantities of ROS (137.54 ± 55.70 a.u. mg protein−1) than the young ones (44.02 ± 43.15 a.u. mg protein−1). Significant differences were also detected in the polyphenols content of P. oceanica between treatments depending on the plant tissue (p < 0.05; Supplementary Table 5), with the highest average amounts determined under the high light conditions, but at an elevated temperature and in the top leaves only (5.79 ± 0.39 mg tyrosine mg protein−1, Figure 7).
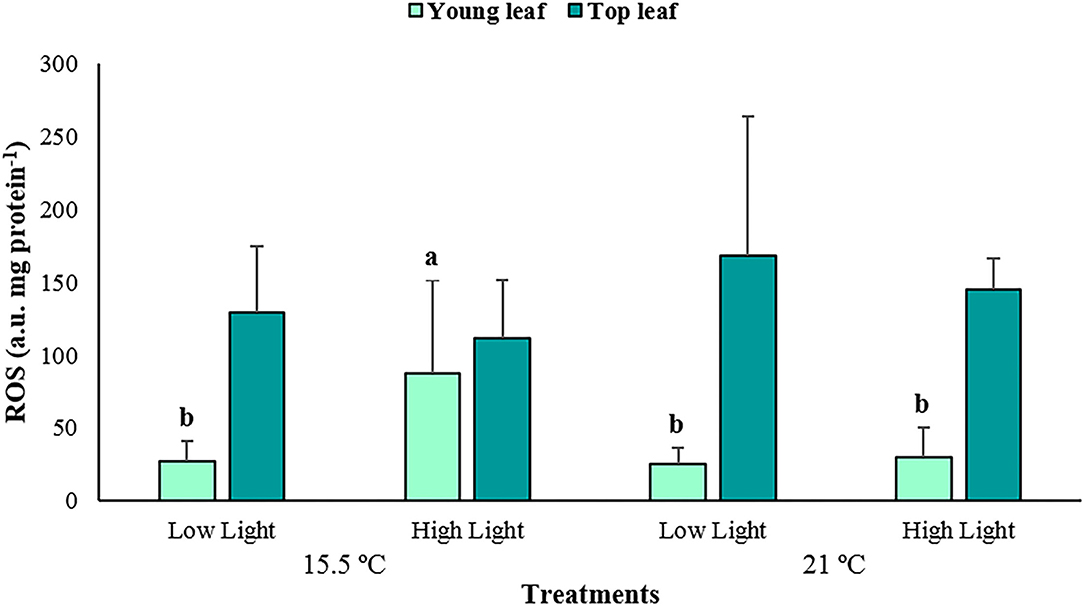
Figure 6. The average reactive oxygen species (ROS) production associated with young and top leaves of Posidonia oceanica in the different treatments (n = 6). The error bars represent ± SD. Different letters denote significant differences (p < 0.01) among treatments (Tukey's post-hoc test following the analysis of deviance in Supplementary Table 4).
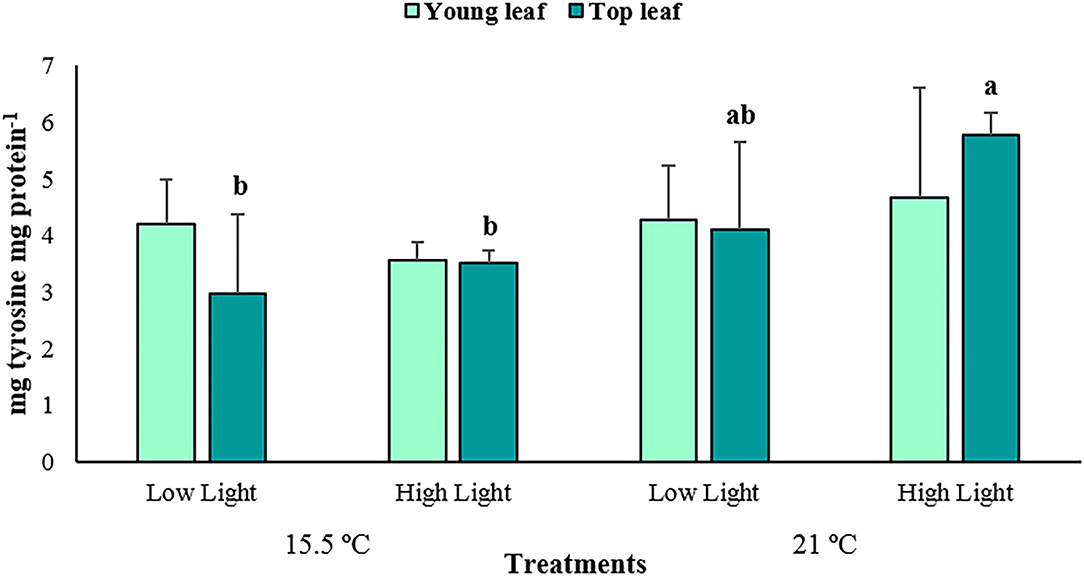
Figure 7. The average polyphenols content in young and top leaves of Posidonia oceanica in the different treatments (n = 4). The error bars represent ± SD. Different letters denote significant differences (p < 0.05) among treatments (Tukey's post-hoc test following the analysis of deviance in Supplementary Table 5).
N2 Fixation Rates and nifH Gene Expression
The estimated N2 fixation rates significantly differed among the treatments and plant tissues (p < 0.001; Supplementary Table 6), yet, the interactions between these factors did not influence the response (p > 0.05). For the most part, the results resembled those obtained for APA, with the highest fixation rates occurring at the elevated temperature and low light treatment (0.11 ± 0.06 nmol N2 g DW−1 h−1, Figure 8), and the lowest corresponding to the treatments of an ambient temperature at low light (0.02 ± 0.03 nmol N2 g DW−1 h−1) and an elevated temperature at high light (0.03 ± 0.05 nmol N2 g DW−1 h−1). As for the plant tissues, the sterilized roots exhibited the highest N2 fixation rates with an average of 0.10 ± 0.07 nmol N2 g DW−1 h−1, while the rhizomes demonstrated the lowest with a mean of 0.0026 ± 0.0024 nmol N2 g DW−1 h−1.
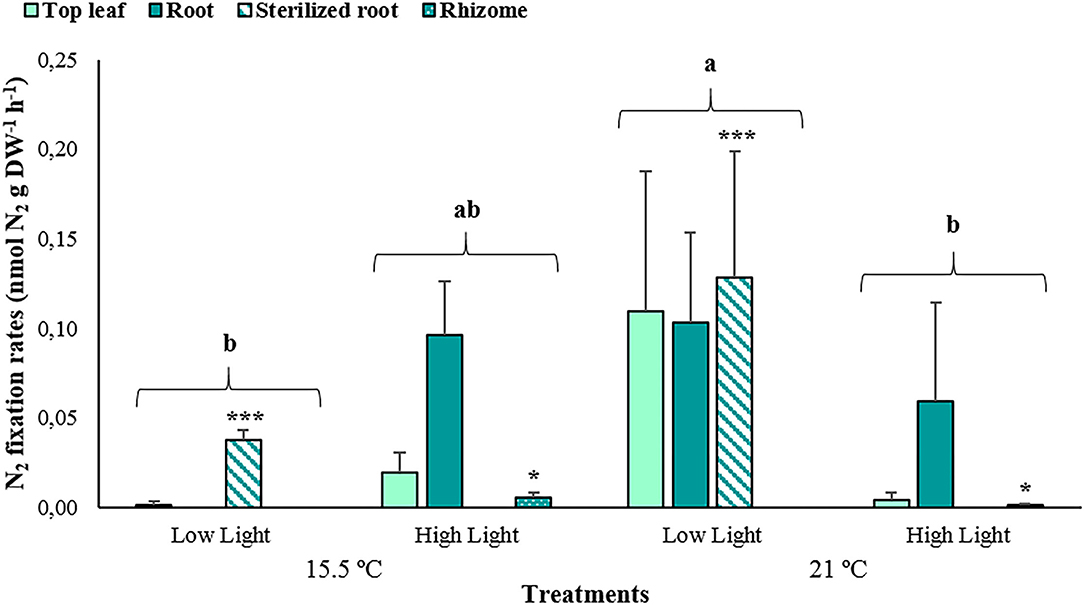
Figure 8. The average N2 fixation rates associated with young leaves, top leaves, roots, and sterilized roots of Posidonia oceanica incubated at the different treatments (n = 3). The error bars represent ± SD. Different letters denote significant differences among treatments, and the asterisk (*) between plant tissues (p < 0.001) (Tukey's post-hoc test following the analysis of deviance in Supplementary Table 6).
From the groups of N2 fixers examined through RT-qPCR, transcripts of the nifH gene were only detected for the cyanobacterial phylotypes UCYN-A, -B, and -C. It was determined that the total transcription of cyanobacterial groups was significantly conditioned by the type of treatment applied (p < 0.001; Supplementary Table 7). Overall, higher transcription values were obtained under elevated temperature, with UCYN-B contributing significantly with the greatest mean at low light conditions (8.46 ± 0.32 transcripts ng total RNA−1, p < 0.001, Figure 9). For the high light treatment under equal temperature, nifH expression was only perceived for UCYN-B and -C. In the ambient temperature treatments, although relatively higher values were estimated at high light compared to low light, the total transcription did not significantly differ among the cyanobacterial groups (p > 0.05). However, under low light conditions, the highest nifH expression was attained by UCYN-C, with an average of 5.65 ± 0.36 transcripts ng total RNA−1, which only deviated significantly (p < 0.01) from the mean exhibited by UCYN-A (1.22 ± 0.82 transcripts ng total RNA−1). In all the treatments, the expression levels of UCYN-A were lower in comparison to the remaining groups.
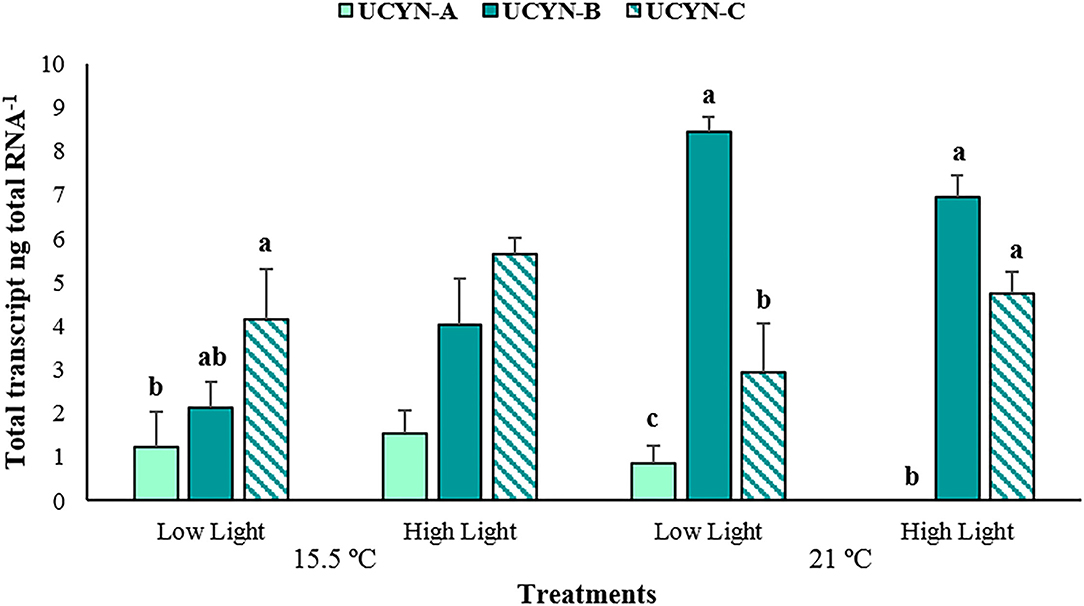
Figure 9. The average nifH gene expression of groups UCYN-A, -B, and -C determined with reverse transcription-quantitative polymerase chain reaction from the phyllosphere of Posidonia oceanica (n = 3). The error bars represent ± SD. Different letters denote significant differences (p < 0.001) (Tukey's post-hoc test following the analysis of deviance in Supplementary Table 7).
Discussion
The results obtained for primary productivity and chlorophyll content of Posidonia oceanica suggest an enhancement in these values under saturating light conditions for the plants during winter, which is in line with previous studies that emphasize light availability as the primary factor influencing the photosynthetic performance of this Mediterranean species (Pergent-Martini et al., 1994; Alcoverro et al., 1995). Higher average pH (i.e., more alkaline conditions), occurred in the aquaria under high light treatments (Figure 2B), possibly reflecting the greater buffering effect provided by the plants through increased photosynthesis (Hendriks et al., 2013). Generally, seagrass meadows can induce diurnal variations in the seawater carbon chemistry in relation to their productivity, through the uptake of CO2 during photosynthesis in the day and the release of CO2 with respiration at night (Chou et al., 2018; Howard et al., 2018). Hence, the more metabolically intense an ecosystem is, the greater its capacity to affect the seawater pH and its alkalinity (Duarte et al., 2013; Hendriks et al., 2013). In addition, greater chlorophyll production has been denoted as a photo-acclimation response of P. oceanica meadows thriving under high light conditions, which implies an increase in the number of reaction centers, and, consequently, in the capacity of photon absorption and of electron flow rate along the transport chain (Frost-Christensen and Sand-Jensen, 1992; Ruban, 2009; Dattolo et al., 2014). Higher chlorophyll content in P. oceanica leaves is generally related to greater photosynthetic rates (Alcoverro et al., 2001), as it was observed in this study. Although the elevated temperature conditions did not significantly affect the chlorophyll concentrations in this study, a past research done by Pirc (1986) reported that P. oceanica can also positively respond to the increasing temperatures with higher photosynthetic rates, as well as chlorophyll production in the winter and spring months. Similarly, Agawin et al. (2021) reported significantly greater chlorophyll a and b values for P. oceanica when incubated at elevated temperature (17°C) and CO2 conditions (1200 μmol CO2 mol−1) in winter.
During the incubations, elevated temperatures did not seem to negatively alter the photosynthetic response, nor promote higher respiration rates in P. oceanica, as opposed to previous findings that demonstrated the disruptive effect of this factor on the productivity of this seagrass species (Collier and Waycott, 2014; Marín-Guirao et al., 2018). This could be partially attributed to the fact that, although the elevated temperature treatment applied in the aquaria was 1°C above the optimum conditions recorded for this seagrass (17–20°C), it stays within its temperature comfort range (13–24°C) (Boudouresque and Meinesz, 1982). However, significantly higher leaf net photosynthetic rates were still exhibited by plants subjected to 15.5°C and high light treatment. On the other hand, in Agawin et al. (2021), positive responses to higher temperature were observed in the photosynthetic activity of P. oceanica, even though leaf respiration rates did not increase, suggesting that ocean warming scenarios may not necessarily have adverse effects on the carbon balance of the plants during winter. This agrees with the higher NPP rates estimated from the phyllosphere of P. oceanica at an elevated temperature and high light conditions during the experiment. Additionally, it should be considered that since the measurements were only made at the end of the experiment, it is possible that the biological parameters might have changed over the course of the incubations, and, thus, the results presented may not entirely address the response of P. oceanica to an abrupt increase of temperature.
The results of this study showed a positive response in the productivity of P. oceanica to light availability in winter. This suggests that anthropogenic activities that cause prolonged periods of a reduced surface irradiance will possibly have more destructive impacts in these ecosystems during winter, rather than an increasing occurrence of heat waves in the Mediterranean (Marbà and Duarte, 2010; Galli et al., 2017). Similarly, Hendriks et al. (2017) reported that low light availability had a negative effect on the photosynthetic performance of P. oceanica under the short-term experimental conditions for summer, while the temperature negatively affected the plants growth. Champenois and Borges (2018) also highlighted the strong association between the GPP of P. oceanica and the interannual variations of the light availability over a decade in the Bay of Revellata, France, as well as the positive correlation to temperature, given that the temperatures recorded had stayed within the comfort range of the seagrass species. Nevertheless, Serrano et al. (2011) demonstrated through in situ shading experiments in Portlligat Bay, Spain, that shallow P. oceanica meadows are more vulnerable to severe light limitation during spring-summer compared with autumn-winter, since it coincides with the plants' favorable growth season when they accumulate reserves for overwintering. The current research was conducted in winter, when the plants could be susceptible to the interaction between an increased seawater temperature and reduced light conditions, as they exhibit lower-upper thermal tolerance limits (Agawin et al., 2021), and the quantity of light they receive for this season is very limited in comparison with spring and summer. Moreover, the majority of experimental evidence on the impacts of elevated temperature on Mediterranean seagrasses have been carried out during the warmer months, hence, this study provides valuable information regarding the potential response of P. oceanica in winter.
The biochemical responses of P. oceanica showed that young leaves subjected to the ambient temperature and high light treatment have exhibited significantly higher ROS production, while top leaves demonstrated greater polyphenols content at an elevated temperature. These observations coincide with studies that reported how factors, such as high irradiance and temperature, may promote the production of ROS in photosynthetic organisms, that in excessive quantities leads to oxidative stress (Choo et al., 2004; Adams et al., 2006; Costa et al., 2015). In this study, the young leaves of P. oceanica that were still in the process of development might have been more vulnerable to the saturating light conditions, which possibly induced the elevated ROS concentrations detected. However, it should also be noted that the high primary production rates unavoidably prompt the production of ROS (Hajiboland, 2014), since these free oxygen radicals are liberated when the photolysis of water molecules by the photosystem II (PSII) occurs during photosynthesis (Lesser, 2006). This corresponds with the significantly higher NPP rates reported for the young leaves at the ambient temperature and high light treatment, hence, the increased amounts of ROS perceived in these tissues could also be related to their high photosynthetic activity. For the top leaves, the higher phenolic compounds measured could be a response of the plants to the elevated temperatures they were exposed to, considering they were not optimum for their functioning and seagrasses, especially for P. oceanica which are sensitive to the quality of environmental conditions (Orth et al., 2006). It may also reflect a thermal stress response of the plants, given the abrupt temperature increase applied in these treatments. Phenolic compounds demonstrate several biological functions that include antioxidant activity, and generally plants activate antioxidant mechanisms to detoxify the generated ROS and to avoid oxidative stress (Cheynier et al., 2013; Costa et al., 2015). The higher mean values of ROS calculated for top leaves under an elevated temperature compared to ambient treatments may support this notion, although the differences were not significant (p < 0.05).
The phosphatase activity in P. oceanica achieved its maximum values at an elevated temperature and low light conditions, which is consistent with the patterns described by Invers et al. (1995), who demonstrated that the increasing temperatures can positively affect APA rates in this seagrass until a certain threshold (24°C). The high activity of this enzyme under an elevated temperature and a low light also matches the significantly enhanced N2 fixation rates exhibited by this treatment, considering that the energy, in the form of adenosine triphosphate (ATP), to fuel N2 fixation is dependent on the presence of inorganic phosphorus; therefore, the demand for this nutrient is theoretically induced when the cells are fixing N2 (Romano et al., 2017; Fernández-Juárez et al., 2019). In contrast, the APA values were on average the lowest under equal temperature and saturating light, regardless of the more favorable pH values for the phosphatases in this treatment, as they were higher compared to the low light treatments. This result could be attributed to the fact that at the final stage of the experiment, the mean phosphate concentration for this treatment was several orders of magnitude higher than the rest (p > 0.05; Table 1), given that APA in seagrasses decreases under elevated phosphate content and, vice versa, increases with phosphorus limitation (Invers et al., 1995; Martínez-Crego et al., 2006; Agawin et al., 2021). As for the significantly lower APA rates demonstrated by the rhizomes, this may suggest that the metabolic activity occurring in this part of the plants is relatively low compared to the others, consequently, its inorganic phosphorus demand is low as well. Generally, the phosphatase activity tends to be greater in the leaves, partially due to the contribution of the epiphytes (Invers et al., 1995).
The estimated N2 fixation rates in the present study are relatively similar to those previously obtained by other works in P. oceanica meadows during winter (Agawin et al., 2017, 2019). The diazotrophic activity, being significantly higher at an elevated temperature and low light conditions, could be attributed to the strong temperature dependency of the nitrogenase enzyme (Brauer et al., 2013; Agawin et al., 2017; Garcias-Bonet et al., 2019). Further, the low nitrate concentrations exhibited in this treatment at the final stage of the incubations (Table 1) may suggest the existence of a dissolved inorganic nitrogen limitation, which possibly induces N2 fixing conditions in these aquaria. Nonetheless, the N2 fixation was considerably lower under an elevated temperature and saturating light conditions, and this could be related to the significantly high GPP values measured for the leaves and phyllosphere of P. oceanica in these tanks. It has been widely documented that the N2 fixation is an oxygen-sensitive process, since molecular oxygen (O2) is capable of inactivating the nitrogenase, then causing irreversible damage to the protein structure, as well as inhibiting the synthesis of the enzyme in many diazotrophs (Berman-Frank et al., 2003; Schoffman et al., 2016). Thus, an increased O2 evolution with an increased photosynthesis may negatively affect the N2 fixation activities associated with P. oceanica in treatments with increased GPP. The diazotrophic activity showed variability among plant parts, with the roots exhibiting higher average values, particularly the sterilized ones containing the root endophytes, in comparison to the leaves. These findings are consistent with the results of Lehnen et al. (2016) for P. oceanica and Hamisi et al. (2009) in tropical seagrass species. According to the latter authors, higher activities in the rhizosphere could be associated with a high occurrence of heterotrophic diazotrophs aside from the autotrophic bacteria in the phyllosphere. This pattern is also in agreement with what was reported by Agawin et al. (2019), who determined the presence of seasonality in the N2 fixation process related to P. oceanica meadows along the Mallorcan coast, with generally higher activities associated with the roots during winter.
The RT-qPCR analyses revealed the presence of the three groups of unicellular diazotrophic cyanobacteria in the phyllosphere of P. oceanica, with UCYN-B and -C displaying notably higher transcription levels of the nifH gene in comparison to UCYN-A. Past molecular analyses carried out by Agawin et al. (2017) indicated the presence of members of the UCYN-B: Crocosphaera, and UCYN-C, like Cyanothece, in addition to other genera of the phyla Cyanobacteria, Proteobacteria, Firmicutes, Bacteroidetes, and Archaea in the phyllosphere of P. oceanica. The UCYN-A (Candidatus Atelocyanobacterium thalassa) is regarded as one of the most abundant and widespread N2-fixing groups in the ocean, proven to live attached or in symbiosis with larger single-celled prymnesiophytes, given that they lack important biosynthetic pathways genes, including oxygenic photosynthesis and carbon fixation (Zehr et al., 2008; Thompson et al., 2012). Considering its association with larger algae and the frequent symbioses between cyanobacteria and multicellular plants as evidenced in terrestrial habitats (Thompson et al., 2012), the presence of this N2-fixing group in P. oceanica is also plausible. The identified diazotrophic communities showcased varied responses to the treatments, with UCYN-B demonstrating the maximum average of nifH expression at an elevated temperature and a limited light, while UCYN-C had significantly higher values compared to the rest under an ambient temperature and low light conditions, although the highest means for this latter group alone were both under high light treatments. The UCYN-B group, having enhanced activities at elevated temperatures, is consistent based on the literature (Brauer et al., 2013; Agawin et al., 2017), with the generally positive correlation recorded between the temperature and the N2 fixation. Moreover, past studies have evidenced the thermophilic habit of Crocosphaera (UCYN-B), with warmer sea surface temperatures (26–29 °C) primarily determining its distribution across the oceans (Church et al., 2008; Moisander et al., 2010). The results obtained for UCYN-C might be due to the different light and temperature requirements its representatives possess; consequently, the species have optimum N2 fixation rates under distinct conditions. On the other hand, although it has been denoted that UCYN-A exhibits a broad temperature range, more associated with cooler waters and a lower temperature optimum than the remaining cyanobacterial groups (Moisander et al., 2010; Cabello et al., 2020), the results obtained from the experiment do not reflect a clear pattern. However, these results should be interpreted with caution, as they may not adequately represent the in situ microbial composition associated with P. oceanica given that the incubation in aquaria might have affected it to a certain extent. Taking into consideration how the different diazotrophic species are adapted to grow and to function under differing conditions, further investigation is required to achieve a better understanding of their potential response to the interaction between the climate change factors and the other stressors.
Data Availability Statement
The raw data supporting the conclusions of this article will be made available by the authors, without undue reservation.
Author Contributions
MG-M and NA designed the experiments. MG-M, VF-J, and JR-C conducted all experiments. All authors led the writing of the manuscript, reviewed, and supervised by the head of the laboratory, NA.
Funding
This research was part of the project CTM2016-75457-P, funded by the MINECO/AEI/10.13039/501100011033 and the ERDF.
Conflict of Interest
The authors declare that the research was conducted in the absence of any commercial or financial relationships that could be construed as a potential conflict of interest.
Publisher's Note
All claims expressed in this article are solely those of the authors and do not necessarily represent those of their affiliated organizations, or those of the publisher, the editors and the reviewers. Any product that may be evaluated in this article, or claim that may be made by its manufacturer, is not guaranteed or endorsed by the publisher.
Acknowledgments
We acknowledge the help of the Scientific Technical Service of the University of the Balearic Islands in the gas chromatography (Maria Trinidad Garcia Barceló), spectrofluorimetric (Biel Martorell Crespí), and flow injection analyses (Josep Agustí Pablo Cànaves). We also thank the support of Regino Martinez (Spanish Institute of Oceanography) in the ammonium analysis.
Supplementary Material
The Supplementary Material for this article can be found online at: https://www.frontiersin.org/articles/10.3389/fmars.2021.757572/full#supplementary-material
References
Adams, W. I., Zarter, C. R., Mueh, K. E., and Amiard, V. (2006). Energy dissipation and photoinhibition: a continuum of photoprotection. In: Photoprotection, photoinhibition, gene regulation, and environment, eds. Demmig-Adams, B., Adams, W. I., and Mattoo, A. K., (Amsterdam, The Netherlands: Springer) p. 49–64.
Agawin, N. S. R., Benavides, M., Busquets, A., Ferriol, P., Stal, L. J., and Arístegui, J. (2014). Dominance of unicellular cyanobacteria in the diazotrophic community in the Atlantic Ocean. Limnol. Ocean. 59, 623–637. doi: 10.4319/lo.2014.59.2.0623
Agawin, N. S. R., Duarte, C. M., and Fortes, M. D. (1996). Nutrient limitation of Philippine seagrasses (Cape Bolinao, NW Philippines): in situ experimental evidence. Mar. Ecol. Prog. Ser. 138, 233–243. doi: 10.3354/meps138233
Agawin, N. S. R., Ferriol, P., Cryer, C., Alcon, E., Busquets, A., Sintes, E., et al. (2016). Significant nitrogen fixation activity associated with the phyllosphere of Mediterranean seagrass Posidonia oceanica: first report. Mar. Ecol. Prog. Ser. 551, 53–62. doi: 10.3354/meps11755
Agawin, N. S. R., Ferriol, P., and Sintes, E. (2019). Simultaneous measurements of nitrogen fixation in different plant tissues of the seagrass Posidonia oceanica. Mar. Ecol. Prog. Ser. 611, 111–127. doi: 10.3354/meps12854
Agawin, N. S. R., Ferriol, P., Sintes, E., and Moyà, G. (2017). Temporal and spatial variability of in situ nitrogen fixation activities associated with the Mediterranean seagrass Posidonia oceanica meadows. Limnol. Ocean. 62, 2575–2592. doi: 10.1002/lno.10591
Agawin, N. S. R., Gil Atorrasagasti, M. G., Frank Comas, A., Fernández-Juárez, V., López-Alforja, X., and Hendriks, I. E. (2021). Response of the seagrass Posidonia oceanica and its associated N2 fixers to high business-as-usual climate change scenario in winter. Limnol. Ocean. 1, 1–14. doi: 10.1002/lno.11758
Alcoverro, T., Cerbian, E., and Ballesteros, E. (2001). The photosynthetic capacity of the seagrass Posidonia oceanica: Influence of nitrogen and light. J. Exp. Mar. Biol. Ecol. 261, 107–120. doi: 10.1016/S0022-0981(01)00267-2
Alcoverro, T., Duarte, C. M., and Romero, J. (1995). Annual growth dynamics of Posidonia oceanica: Contribution of large-scale versus local factors to seasonality. Mar. Ecol. Prog. Ser. 120, 203–210. doi: 10.3354/meps120203
Alcoverro, T., Manzanera, M., and Romero, J. (1998). Seasonal and age-dependent variability of Posidonia oceanica (L.) Delile photosynthetic parameters. J. Exp. Mar. Biol. Ecol. 230, 1–13. doi: 10.1016/S0022-0981(98)00022-7
Barbier, E. B., Hacker, S. D., Kennedy, C., Koch, E. W., Stier, A. C., and Silliman, B. R. (2011). The value of estuarine and coastal ecosystem services. Ecol. Monogr. 81, 169–193. doi: 10.1890/10-1510.1
Beca-Carretero, P., Guihéneuf, F., Marín-Guirao, L., Bernardeau-Esteller, J., García-Muñoz, R., et al. (2018). Effects of an experimental heat wave on fatty acid composition in two Mediterranean seagrass species. Mar. Pollut. Bull. 134, 27–37. doi: 10.1016/j.marpolbul.2017.12.057
Berman-Frank, I., Lundgren, P., and Falkowski, P. (2003). Nitrogen fixation and photosynthetic oxygen evolution in cyanobacteria. Res. Microbiol. 154, 157–164. doi: 10.1016/S0923-2508(03)00029-9
Biegala, I. C., and Raimbault, P. (2008). High abundance of diazotrophic picocyanobacteria (<3 μm) in a Southwest Pacific coral lagoon. Aquat. Microb. Ecol. 51, 45–53. doi: 10.3354/ame01185
Boudouresque, C. F., Bernard, G., Bonhomme, P., Charbonnel, E., Diviacco, G., Meinesz, A., et al. (2006). Préservation et Conservation des Herbiers à Posidonia Oceanica. Monaco: Ramoge publisher.
Boudouresque, C. F., and Meinesz, A. (1982). Découverte de l'herbier de Posidonie. Parc Natl. Port-Cros Cah. 4, 1–79.
Boudouresque, C. F., Shili, A., Verlaque, M., and Paoli, D. C. P. (2009). Regression of Mediterranean seagrasses caused by natural processes and anthropogenic disturbances and stress: a critical review. Bot. Mar. 52, 395–418. doi: 10.1515/BOT.2009.057
Brauer, V. S., Stomp, M., Rosso, C., van Beusekom, S. A. M., Emmerich, B., Stal, L. J., et al. (2013). Low temperature delays timing and enhances the cost of nitrogen fixation in the unicellular cyanobacterium Cyanothece. ISME J. 7, 2105–2115. doi: 10.1038/ismej.2013.103
Bronk, D. A., Lomas, M. W., Glibert, P. M., Schukert, K. J., and Sanderson, M. P. (2000). Total dissolved nitrogen analysis: Comparisons between persulfate, UV and high temperature oxidation methods. Mar. Chem. 69, 163–178. doi: 10.1016/S0304-4203(99)00103-6
Cabello, A. M., Turk-Kubo, K. A., Hayashi, K., Jacobs, L., Kudela, R. M., and Zehr, J. P. (2020). Unexpected presence of the nitrogen-fixing symbiotic cyanobacterium UCYN-A in Monterey Bay, California. J. Phycol. 56, 1521–1533. doi: 10.1111/jpy.13045
Calvo, E., Sim,ó, R., Coma, R., Ribes, M., Pascual, J., Sabatés, A., et al. (2011). Effects of climate change on Mediterranean marine ecosystems: the case of the Catalan Sea. Clim. Res. 50, 1–29. doi: 10.3354/cr01040
Cambridge, M. L., Chiffings, A. W., Brittan, C., Moore, L., and McComb, A. J. (1986). The loss of seagrass in Cockburn Sound, Western Australia. II. Possible causes of seagrass decline. Aquat. Bot. 24, 269–285. doi: 10.1016/0304-3770(86)90062-8
Capone, D. G.. (1993). Determination of nitrogenase activity in aquatic samples using the acetylene reduction procedure. In: Handbook of methods in aquatic microbial ecology, eds. Kemp, P., Sherr, B., Sherr, E., and Cole, J. (Boca Raton, FL: Lewis Publishers) p. 621–631. doi: 10.1201/9780203752746-74
Champenois, W., and Borges, A. V. (2018). Inter-annual variations over a decade of primary production of the seagrass Posidonia oceanica. Limnol. Ocean. 64, 32–45. doi: 10.1002/lno.11017
Cheynier, V., Comte, G., Davies, K. M., Lattanzio, V., and Martens, S. (2013). Plant phenolics: recent advances on their biosynthesis, genetics, and ecophysiology. Plant Physiol. Biochem. 72, 1–20. doi: 10.1016/j.plaphy.2013.05.009
Choo, K. S., Snoeijs, P., and Pedersen, M. (2004). Oxidative stress tolerance in the filamentous green algae Cladophora glomerata and Enteromorpha ahlneriana. J. Exp. Mar. Biol. Ecol. 298, 111–123. doi: 10.1016/j.jembe.2003.08.007
Chou, W.-C., Chu, H.-C., Chen, Y.-H., Syu, R.-W., Hung, C.-C., and Soong, K. (2018). Short-term variability of carbon chemistry in two contrasting seagrass meadows at Dongsha Island: Implications for pH buffering and CO2 sequestration. Estuar. Coast. Shelf Sci. 210, 36–44. doi: 10.1016/j.ecss.2018.06.006
Church, M. J., Björkman, K. M., Karl, D. M., Saito, M. A., and Zehr, J. P. (2008). Regional distributions of nitrogen-fixing bacteria in the Pacific Ocean. Limnol. Ocean. 53, 63–77. doi: 10.4319/lo.2008.53.1.0063
Church, M. J., Jenkins, B. D., Karl, D. M., and Zehr, J. P. (2005). Vertical distributions of nitrogen-fixing phylotypes at Stn ALOHA in the oligotrophic North Pacific Ocean. Aquat. Microb. Ecol. 38, 3–14. doi: 10.3354/ame038003
Cole, L. W., and McGlathery, K. J. (2012). Nitrogen fixation in restored eelgrass meadows. Mar. Ecol. Prog. Ser. 448, 235–246. doi: 10.3354/meps09512
Collier, C. J., and Waycott, M. (2014). Temperature extremes reduce seagrass growth and induce mortality. Mar. Pollut. Bull. 83, 483–490. doi: 10.1016/j.marpolbul.2014.03.050
Collins, M., Knutti, R., Arblaster, J., Dufresne, J.-L., Fichefet, T., Friedlingstein, P., et al. (2013). Long-term climate change: projections, commitments and irreversibility. In: Climate Change 2013: The Physical Science Basis. Contribution of Working Group I to the Fifth Assessment Report of the Intergovernmental Panel on Climate Change, eds. Stocker, T. F., Qin, D., Plattner, G.-K., Tignor, M., Allen, S. K., Boschung, J., et al. (Cambridge, United Kingdom and New York, NY, USA: Cambridge University Press) 1029–1136.
Coombs, J. T., and Franco, C. M. M. (2003). Isolation and identification of actinobacteria from surface-sterilized wheat roots. Appl. Environ. Microbiol. 69, 5603–5608. doi: 10.1128/AEM.69.9.5603-5608.2003
Costa, M. M., Barrote, I., Silva, J., Olivé, I., Alexandre, A., Albano, S., et al. (2015). Epiphytes modulate Posidonia oceanica photosynthetic production, energetic balance, antioxidant mechanisms, and oxidative damage. Front. Mar. Sci. 2, 111. doi: 10.3389/fmars.2015.00111
Darmaraki, S., Somot, S., Sevault, F., Nabat, P., Cabos Narvaez, W. D., Cavicchia, L., et al. (2019). Future evolution of marine heatwaves in the Mediterranean Sea. Clim. Dyn. 53, 1371–1392. doi: 10.1007/s00382-019-04661-z
Dattolo, E., Ruocco, M., Brunet, C., Lorenti, M., Lauritano, C., D'Esposito, D., et al. (2014). Response of the seagrass Posidonia oceanica to different light environments: insights from a combined molecular and photo-physiological study. Mar. Environ. Res. 101, 225–236. doi: 10.1016/j.marenvres.2014.07.010
Dennison, W. C., Orth, R. J., Moore, K. A., Stevenson, J. C., Carter, V., Kollar, S., et al. (1993). Assessing water quality with submersed aquatic vegetation. Bioscience 43, 86–94. doi: 10.2307/1311969
Diamond, D.. (2003). QuikChem® Method 31-107-04-1-A. Determination of nitrate/nitrite in brackish or seawater by flow injection analysis. ed. Bogren, K. Colorado, United States: Lachat Instruments.
Díaz-Almela, E., Marbà, N., Martínez, R., Santiago, R., and Duarte, C. M. (2009). Seasonal dynamics of Posidonia oceanica in Magalluf Bay (Mallorca, Spain): temperature effects on seagrass mortality. Limnol. Ocean. 54, 2170–2182. doi: 10.4319/lo.2009.54.6.2170
Diffenbaugh, N. S., Pal, J. S., Giorgi, F., and Gao, X. (2007). Heat stress intensification in the Mediterranean climate change hotspot. Geophys. Res. Lett. 34, 1–6. doi: 10.1029/2007GL030000
Duarte, C. M., Hendriks, I. E., Moore, T. S., Olsen, Y. S., Steckbauer, A., Ramajo, L., et al. (2013). Is ocean acidification an open-ocean syndrome? Understanding anthropogenic impacts on seawater pH. Estuar. Coast. 36, 221–236. doi: 10.1007/s12237-013-9594-3
Duarte, C. M., Marb,à, N., and Santos, R. (2004). What may cause loss of seagrasses? In: European seagrasses: an introduction to monitoring and management, eds. Borum, J., Duarte, C. M., Krause-Jensen, D., and Greve, T. M. (Copenhagen, Denmark: The MandMS project) 19–23.
Duarte, C. M., Middelburg, J. J., and Caraco, N. (2005). Major role of marine vegetation on the oceanic carbon cycle. Biogeosciences. 2, 1–8. doi: 10.5194/bg-2-1-2005
Erftemeijer, P. L., Lewis, I. I. I. R, and Roy, R. (2006). Environmental impacts of dredging on seagrasses: a review. Mar. Pollut. Bull. 52, 1553–1572. doi: 10.1016/j.marpolbul.2006.09.006
Fernández-Juárez, V., Bennasar-Figueras, A., Tovar-Sanchez, A., and Agawin, N. S. R. (2019). The role of iron in the P-acquisition mechanisms of the unicellular N2-fixing cyanobacteria Halothece sp., found in association with the Mediterranean seagrass Posidonia oceanica. Front. Microbiol. 10, 1–15. doi: 10.3389/fmicb.2019.01903
Foster, R. A., Subramaniam, A., Mahaffey, C., Carpenter, E. J., Capone, D. G., and Zehr, J. P. (2007). Influence of the Amazon river plume on distributions of free-living and symbiotic cyanobacteria in the western tropical North Atlantic Ocean. Limnol. Ocean. 52, 517–532. doi: 10.4319/lo.2007.52.2.0517
Fourqurean, J.W., Duarte, C.M., Kennedy, H., Marbà, N., Holmer, M., Mateo, M.A., et al. (2017). Seagrass ecosystems as a globally significant carbon stock. Nat. Geosci. 5, 505–509. doi: 10.1038/ngeo1477
Frost-Christensen, H., and Sand-Jensen, K. (1992). The quantum efficiency of photosynthesis in macroalgae and submerged angiosperms. Oecologia. 91, 377–384. doi: 10.1007/BF00317627
Gaby, J. C., and Buckley, D. H. (2012). A comprehensive evaluation of PCR primers to amplify the nifH gene of nitrogenase. PLoS ONE. 7, e42149. doi: 10.1371/journal.pone.0042149
Gacia, E., Marb,à, N., Cebrián, J., Vaquer-Sunyer, R., Garcias-Bonet, N., and Duarte, C. M. (2012). Thresholds of irradiance for seagrass Posidonia oceanica meadow metabolism. Mar. Ecol. Prog. Ser. 466, 69–79. doi: 10.3354/meps09928
Galli, G., Solidoro, C., and Lovato, T. (2017). Marine heat waves hazard 3D maps and the risk for low motility organisms in a warming Mediterranean Sea. Front. Mar. Sci. 4, 136. doi: 10.3389/fmars.2017.00136
Garcias-Bonet, N., Arrieta, J. M., Duarte, C. M., and Marb,à, N. (2016). Nitrogen-fixing bacteria in Mediterranean seagrass (Posidonia oceanica) roots. Aquat. Bot. 131, 57–60. doi: 10.1016/j.aquabot.2016.03.002
Garcias-Bonet, N., Vaquer-Sunyer, R., Duarte, C. M., and Marb,à, N. (2019). Warming effect on nitrogen fixation in Mediterranean macrophyte sediments. Biogeosciences. 16, 167–175. doi: 10.5194/bg-16-167-2019
Goebel, N. L., Edwards, C. A., Carter, B. J., Achilles, K. M., and Zehr, J. P. (2008). Growth and carbon content of three different-sized diazotrophic cyanobacteria observed in the subtropical North Pacific. J. Phycol. 44, 1212–1220. doi: 10.1111/j.1529-8817.2008.00581.x
Goebel, N. L., Turk, K. A., Achilles, K. M., Paerl, R., Hewson, I., Morrison, A. E., et al. (2010). Abundance and distribution of major groups of diazotrophic cyanobacteria and their potential contribution to N2 fixation in the tropical Atlantic Ocean. Environ. Microbiol. 12, 3272–3289. doi: 10.1111/j.1462-2920.2010.02303.x
Guerrero-Meseguer, L., Marín, A., and Sanz-Lázaro, C. (2017). Future heat waves due to climate change threaten the survival of P. oceanica seedlings. Environ. Pollut. 230, 40–45. doi: 10.1016/j.envpol.2017.06.039
Hajiboland, R.. (2014). Reactive oxygen species and photosynthesis. In: Oxidative damage to plants: antioxidant networks and signaling, ed. P. Ahmad (Academic Press), p. 1–63. doi: 10.1016/B978-0-12-799963-0.00001-0
Hamisi, M.. (2010). Genetic variability and nitrogenase activity of cyanobacterial communities associated with tropical seagrass meadows. (western Indian Ocean).
Hamisi, M. I., Lyimo, T. J., Muruke, M. H. S., and Bergman, B. (2009). Nitrogen fixation by epiphytic and epibenthic diazotrophs associated with seagrass meadows along the Tanzanian coast, Western Indian Ocean. Aquat. Microb. Ecol. 57, 33–42. doi: 10.3354/ame01323
Hendriks, I. E., Olsen, Y. S., and Duarte, C. M. (2017). Light availability and temperature, not increased CO2, will structure future meadows of Posidonia oceanica. Aquat. Bot. 139, 32–36. doi: 10.1016/j.aquabot.2017.02.004
Hendriks, I. E., Olsen, Y. S., Ramajo, L., Basso, L., Steckbauer, A., Moore, T. S., et al. (2013). Photosynthetic activity buffers ocean acidification in seagrass meadows. Biogeosci. Discuss. 10, 12313–12346. doi: 10.5194/bgd-10-12313-2013
Hernán, G., Ortega, M. J., Gándara, A. M., Castejón, I., Terrados, J., and Tomas, F. (2017). Future warmer seas: increased stress and susceptibility to grazing in seedlings of a marine habitat-forming species. Glob. Chang. Biol. 23, 4530–4543. doi: 10.1111/gcb.13768
Horstkotte, B., and Duarte, C. M. (2012). Análisis de amonio en aguas marinas y aguas dulces por fluorimetría. In Expedición de circunnavegación Malaspina 2010. Cambio global y exploración de la biodiversidad del océano. Libro blanco de métodos y técnicas de trabajo oceanográfico, ed. Moreno-Ostos, E. (Madrid, Spain: Consejo Superior de Investigaciones Científicas) p. 123–132.
Howard, J. L., Creed, J. C., Aguiar, M. V. P., and Fouqurean, J. W. (2018). CO2 released by carbonate sediment production in some coastal areas may offset the benefits of seagrass “Blue Carbon” storage. Limnol. Ocean. 63, 160–172. doi: 10.1002/lno.10621
Invers, O., Pérez, M., and Romero, J. (1995). Alkaline phosphatase activity as a tool for assessing nutritional conditions in the seagrass Posidonia oceanica. Sci. Mar. 59, 41–47.
Invers, O., Zimmerman, R. C., Alberte, R. S., Perez, M., and Romero, J. (2001). Inorganic carbon sources for seagrass photosynthesis: an experimental evaluation of bicarbonate use in species inhabiting temperate waters. J. Exp. Mar. Biol. Ecol. 265, 203–217. doi: 10.1016/S0022-0981(01)00332-X
IPCC (2007). Climate change 2007: The Physical Science Basis. eds. Solomon, S., Qin, D., Manning, M., Chen, Z., Marquis, M., Averyt, K. B. et al. Cambridge, United Kingdom and New York, NY, USA: Cambridge University Press.
IPCC (2014). Climate Change 2014: Impacts, Adaptation, and Vulnerability. Part A: Global and Sectoral Aspects. Contribution of Working Group II to the Fifth Assessment Report of the Intergovernmental Panel on Climate Change. Field, C. B., Barros, V. R., Dokken, D. J., Mach, K. J., Mastrandrea, M. D., Bilir, T. E., et al. Cambridge, United Kingdom and New York, NY, USA: Cambridge University Press.
Jensen, B. B., and Cox, R. P. (1983). Direct measurements of steady-state kinetics of cyanobacterial N2 uptake by membrane-leak mass spectrometry and comparisons between nitrogen fixation and acetylene reduction. Appl. Environ. Microbiol. 45, 1331–1337. doi: 10.1128/aem.45.4.1331-1337.1983
Jordà, G., Marb,à, N., and Duarte, C. M. (2012). Mediterranean seagrass vulnerable to regional climate warming. Nat. Clim. Chang. 2, 821–824. doi: 10.1038/nclimate1533
Kaldy, J. E.. (2006). Production ecology of the non-indigenous seagrass, dwarf eelgrass (Zostera japonica Ascher, and Graeb.), in a Pacific Northwest Estuary, USA. Hydrobiologia 553, 201–217. doi: 10.1007/s10750-005-5764-z
Kaldy, J. E., and Dunton, K. H. (2000). Above- and below-ground production, biomass and reproductive ecology of Thalassia testudinum (turtle grass) in a subtropical coastal lagoon. Mar. Ecol. Prog. Ser. 193, 271–283. doi: 10.3354/meps193271
Karl, D., Michaels, A., Bergman, B., Capone, D., Carpenter, E., Letelier, R., et al. (2002). Dinitrogen fixation in the world's oceans. Biogeochemistry 57, 47–98. doi: 10.1023/A:1015798105851
Knap, A. H., Michaels, A. F., Steinberg, D. K., Bahr, F., Bates, N. R., Bell, S., et al. (1997). The determination of phosphorus in sea water. In: BATS Methods Manual, Version 4 (Woods Hole, MA, USA: U.S. JGOFS Planning Office) p. 71–74.
Kuenzler, E. J., and Perras, J. P. (1965). Phosphatases of marine algae. Biol. Bull. 128, 271–284. doi: 10.2307/1539555
Kumar, M., Contreras-Porcia, L., Kumar, N. M., and Ralph, P. J. (2018). Quantification and localization of reactive oxygen species in marine macrophytes. In: Protocols for Macroalgae Research, eds. Charrier, B., Wichard, T., and Reddy, C. R. K. (Boca Raton, FL: CRC Press) p. 267–277. doi: 10.1201/b21460-17
Labasque, T., Chaumery, C., Aminot, A., and Kergoat, G. (2004). Spectrophotometric Winkler determination of dissolved oxygen: re-examination of critical factors and reliability. Mar. Chem. 88, 53–60. doi: 10.1016/j.marchem.2004.03.004
Larkum, A. W. D., Drew, E. A., and Ralph, P. J. (2006). Photosynthesis and metabolism in seagrasses at the cellular level. In: Seagrasses: Biology, Ecology and Conservation, eds. Larkum, A. W. D., Orth, R. J., and Duarte, C. M. (Dordrecht, The Netherlands: Springer Netherlands) p. 323–345. doi: 10.1007/1-4020-2983-7_14
Lee, K.-S., and Dunton, K. (1996). Production and carbon reserve dynamics of the seagrass Thalassia testudinum in Corpus Christi Bay, Texas, USA. Mar. Ecol. Prog. Ser. 143, 201–210. doi: 10.3354/meps143201
Lee, K.-S., Park, S. R., and Kim, J.-B. (2005). Production dynamics of the eelgrass, Zostera marina in two bay systems on the south coast of the Korean peninsula. Mar. Biol. 147, 1091–1108. doi: 10.1007/s00227-005-0011-8
Lee, K. S., Park, S. R., and Kim, Y. K. (2007). Effects of irradiance, temperature, and nutrients on growth dynamics of seagrasses: a review. J. Exp. Mar. Biol. Ecol. 350, 144–175. doi: 10.1016/j.jembe.2007.06.016
Lehnen, N., Marchant, H. K., Schwedt, A., Milucka, J., Lott, C., Weber, M., et al. (2016). High rates of microbial dinitrogen fixation and sulfate reduction associated with the Mediterranean seagrass Posidonia oceanica. Syst. Appl. Microbiol. 39, 476–483. doi: 10.1016/j.syapm.2016.08.004
Lesser, M. P.. (2006). Oxidative stress in marine environments: biochemistry and physiological ecology. Annu. Rev. Physiol. 68, 253–278. doi: 10.1146/annurev.physiol.68.040104.110001
Mahaffey, C., Michaels, A., and Capone, D. (2005). The conundrum of marine N2 fixation. Am. J. Sci. 305, 546–595. doi: 10.2475/ajs.305.6-8.546
Marbà, N., and Duarte, C. M. (2010). Mediterranean warming triggers seagrass (Posidonia oceanica) shoot mortality. Glob. Chang. Biol. 16, 2366–2375. doi: 10.1111/j.1365-2486.2009.02130.x
Marín-Guirao, L., Bernardeau-Esteller, J., Garcia-Munoz, R., Ramos, A., Ontoria, Y., Romero, J., et al. (2018). Carbon economy of Mediterranean seagrasses in response to thermal stress. Mar. Pollut. Bull. 135, 617–629. doi: 10.1016/j.marpolbul.2018.07.050
Marín-Guirao, L., Entrambasaguas, L., Ruiz, J. M., and Procaccini, G. (2019). Heat-stress induced flowering can be a potential adaptive response to ocean warming for the iconic seagrass Posidonia oceanica. Mol. Ecol. 28, 2486–2501. doi: 10.1111/mec.15089
Martínez-Crego, B., Romero, J., and Alcoverro, T. (2006). The use of surface alkaline phosphatase activity in the seagrass Posidonia oceanica as a biomarker of eutrophication. Mar. Ecol. 27, 381–387. doi: 10.1111/j.1439-0485.2006.00101.x
Mazzuca, S., Spadafora, A., Filadoro, D., Vannini, C., Marsoni, M., Cozza, R., et al. (2009). Seagrass light acclimation: 2-DE protein analysis in Posidonia leaves grown in chronic low light conditions. J. Exp. Mar. Biol. Ecol. 374, 113–122. doi: 10.1016/j.jembe.2009.04.010
Mills, M. M., Ridame, C., Davey, M., La Roche, J., and Geider, R. J. (2004). Iron and phosphorus co-limit nitrogen fixation in the eastern tropical North Atlantic. Nature. 429, 292–294. doi: 10.1038/nature02550
Moal, M., Le, C.ollin, H., and Biegala, I. C. (2011). Intriguing diversity among diazotrophic picoplankton along a Mediterranean transect: a dominance of rhizobia. Biogeosciences. 8, 827–840. doi: 10.5194/bg-8-827-2011
Moisander, P. H., Beinart, R. A., Hewson, I., White, A. E., Johnson, K. S., Carlson, C. A., et al. (2010). Unicellular cyanobacterial distributions broaden the oceanic N2 fixation domain. Science. 327, 1512–1514. doi: 10.1126/science.1185468
Ontoria, Y., Cuesta-Gracia, A., Ruiz, J. M., Romero, J., and Pérez, M. (2019). The negative effects of short-term extreme thermal events on the seagrass Posidonia oceanica are exacerbated by ammonium additions. PLoS ONE. 14, 1–19. doi: 10.1371/journal.pone.0222798
Onuf, C. P.. (1994). Seagrasses, dredging and light in Laguna Madre, Texas, U.S.A. Estuar. Coast. Shelf Sci. 39, 75–91. doi: 10.1006/ecss.1994.1050
Orth, R. J., Carruthers, T. J. B., Dennison, W. C., Duarte, C. M., Fourqurean, J. W., Heck, K. L., et al. (2006). A global crisis for seagrass ecosystems. Bioscience. 56, 987–996. doi: 10.1641/0006-3568(2006)56987:AGCFSE2.0.CO;2
Orth, R. J., and Moore, K. A. (1983). Chesapeake Bay: an unprecedented decline in submerged aquatic vegetation. Science. 222, 51–53. doi: 10.1126/science.222.4619.51
Pérez, M., and Romero, J. (1993). Preliminary data on alkaline phosphatase activity associated with Mediterranean seagrasses. Bot. Mar. 36, 499–502. doi: 10.1515/botm.1993.36.6.499
Pergent-Martini, C., Rico-Raimondino, V., and Pergent, G. (1994). Primary production of Posidonia oceanica in the Mediterranean Basin. Mar. Biol. 120, 9–15.
Pirc, H.. (1986). Seasonal aspects of photosynthesis in Posidonia oceanica: influence of depth, temperature and light intensity. Aquat. Bot. 26, 203–212. doi: 10.1016/0304-3770(86)90021-5
Procaccini, G., Buia, M. C., Gambi, M. C., Pérez, M., Pergent, G., Pergent-Martini, C., et al. (2003). The seagrasses of the Western Mediterranean. In: World atlas of seagrasses, Green, E. P., and Short, F. T. (eds.) (Berkeley, USA: University of California Press) p. 48–52.
Romano, S., Bondarev, V., Kölling, M., Dittmar, T., and Schulz-Vogt, H. N. (2017). Phosphate limitation triggers the dissolution of precipitated iron by the marine bacterium Pseudovibrio sp. FO-BEG1. Front. Microbiol. 8, 364. doi: 10.3389/fmicb.2017.00364
Rubio, L., and Ludden, P. (2002). “The gene products of the nif regulon,” in Nitrogen fixation at the millennium, ed. G. Leigh (Amsterdam, The Netherlands: Elsevier Science B.V.) 101–136. doi: 10.1016/B978-044450965-9/50004-5
Ruiz, J. M., Marín-Guirao, L., García-Muñoz, R., Ramos-Segura, A., Bernardeau-Esteller, J., Pérez, M., et al. (2018). Experimental evidence of warming-induced flowering in the Mediterranean seagrass Posidonia oceanica. Mar. Pollut. Bull. 134, 49–54. doi: 10.1016/j.marpolbul.2017.10.037
Ruiz, J. M., and Romero, J. (2001). Effects of in situ experimental shading on the Mediterranean seagrass Posidonia oceanica. Mar. Ecol. Prog. Ser. 215, 107–120. doi: 10.3354/meps215107
Ruiz, J. M., and Romero, J. (2003). Effects of disturbances caused by coastal constructions on spatial structure, growth dynamics and photosynthesis of the seagrass Posidonia oceanica. Mar. Pollut. Bull. 46, 1523–1533. doi: 10.1016/j.marpolbul.2003.08.021
Sañudo-Wilhelmy, S. A., Kustka, A. B., Gobler, C. J., Hutchins, D. A., Yang, M., Lwiza, K., et al. (2001). Phosphorus limitation of nitrogen fixation by Trichodesmium in the central Atlantic Ocean. Nature 411, 66–69. doi: 10.1038/35075041
Schoffman, H., Lis, H., Shaked, Y., and Keren, N. (2016). Iron-nutrient interactions within phytoplankton. Front. Plant Sci. 7, 1–12. doi: 10.3389/fpls.2016.01223
Serrano, O., Mateo, M. A., and Renom, P. (2011). Seasonal response of Posidonia oceanica to light disturbances. Mar. Ecol. Prog. Ser. 423, 29–38. doi: 10.3354/meps08955
Short, F., and Duarte, C. M. (2001). Methods for the measurement of seagrass growth and production. In: Global seagrass research methods, eds. Short, F., Short, C. A., and Coles, R. G. (Amsterdam: Elsevier) p. 155–182. doi: 10.1016/B978-044450891-1/50009-8
Short, F. T., and Wyllie-Echeverria, S. (1996). Natural and human-induced disturbance of seagrasses. Environ. Conserv. 23, 17–27. doi: 10.1017/S0376892900038212
Singleton, V. L., Orthofer, R., and Lamuela-Raventós, R. (1999). Analysis of total phenols and other oxidation substrates and antioxidants by means of Folin-Ciocalteu reagent. Methods Enzym. 299, 152–178. doi: 10.1016/S0076-6879(99)99017-1
SOCIB (2020). SOCIB/Ocean forecast. Model. Forecast. Facil. Available online at: https://www.socib.es/index.php?seccion=modellingandfacility=lw4nc. (accessed December 1 2020).
Stal, L. J.. (1988). Nitrogen fixation in cyanobacterial mats. Methods Enzym. 167, 474–484. doi: 10.1016/0076-6879(88)67052-2
Steinhart, G. S., Likens, G. E., and Soto, D. (2002). Physiological indicators of nutrient deficiency in phytoplankton in southern Chilean lakes. Hydrobiologia. 489, 21–27. doi: 10.1023/A:1023271331086
Strickland, J. D. H., and Parsons, T. R. (1972). A Practical Handbook of Seawater Analysis. 2nd ed. Ottawa, Canada: Fisheries Research Board of Canada, Bulletin p. 167.
Telesca, L., Belluscio, A., Criscoli, A., Ardizzone, G., Apostolaki, E. T., Fraschetti, S., et al. (2015). Seagrass meadows (Posidonia oceanica) distribution and trajectories of change. Sci. Rep. 5, 12505. doi: 10.1038/srep12505
Thompson, A. W., Foster, R. A., Krupke, A., Carter, B. J., Musat, N., Vaulot, D., et al. (2012). Unicellular cyanobacterium symbiotic with a single-celled eukaryotic alga. Science. 337, 1546–1550. doi: 10.1126/science.1222700
Traboni, C., Mammola, S. D., Ruocco, M., Ontoria, Y., Ruiz, J. M., Procaccini, G., et al. (2018). Investigating cellular stress response to heat stress in the seagrass Posidonia oceanica in a global change scenario. Mar. Environ. Res. 141, 12–23. doi: 10.1016/j.marenvres.2018.07.007
Turk-Kubo, K. A., Achilles, K. M., Serros, T. R. C., Ochiai, M., Montoya, J. P., and Zehr, J. P. (2012). Nitrogenase (nifH) gene expression in diazotrophic cyanobacteria in the Tropical North Atlantic in response to nutrient amendments. Front. Microbiol. 3, 1–17. doi: 10.3389/fmicb.2012.00386
Uku, J., Björk, M., Bergman, B., and Díez, B. (2007). Characterization and comparison of prokaryotic epiphytes associated with three East African Seagrasses. J. Phycol. 43, 768–779. doi: 10.1111/j.1529-8817.2007.00371.x
Vargas-Yáñez, M., García, M. J., Salat, J., García-Martínez, M. C., Pascual, J., and Moya, F. (2008). Warming trends and decadal variability in the Western Mediterranean shelf. Glob. Planet. Chang. 63, 177–184. doi: 10.1016/j.gloplacha.2007.09.001
Waycott, M., Duarte, C. M., Carruthers, T. J. B., Orth, R. J., Dennison, W. C., Olyarnik, S., et al. (2009). Accelerating loss of seagrasses across the globe threatens coastal ecosystems. Proc. Natl. Acad. Sci. U.S.A. 106, 12377–12381. doi: 10.1073/pnas.0905620106
Weber-Shirk, M. L., Lion, L. W., and Bisogni, J. J. J. (2001). Phosphorus determination using the colorimetric ascorbic acid technique. In: Laboratory Research in Environmental Engineering, Laboratory Manual (Ithaca, USA: Cornell University) p. 64–69.
Welsh, D. T.. (2000). Nitrogen fixation in seagrass meadows: regulation, plant-bacteria interactions and significance to primary productivity. Ecol. Lett. 3, 58–71. doi: 10.1046/j.1461-0248.2000.00111.x
Wintermans, J., and De Mots, A. (1965). Spectrophotometric characteristics of chlorophylls a and b and their pheophytins in ethanol. Biochim. Biophys. Acta 109, 448–453. doi: 10.1016/0926-6585(65)90170-6
York, P. H., Gruber, R. K., Hill, R., Ralph, P. J., Booth, D. J., and Macreadie, P. I. (2013). Physiological and morphological responses of the temperate seagrass Zostera muelleri to multiple stressors: investigating the interactive effects of light and temperature. PLoS ONE. 8, 1–12. doi: 10.1371/journal.pone.0076377
Zehr, J. P., Bench, S. R., Carter, B. J., Hewson, I., Niazi, F., Shi, T., et al. (2008). Globally distributed uncultivated oceanic N2-fixing cyanobacteria lack oxygenic photosystem II. Science. 322, 1110–1112. doi: 10.1126/science.1165340
Zehr, J. P., Waterbury, J. B., Turner, P. J., Montoya, J. P., Omoregie, E., Steward, G. F., et al. (2001). Unicellular cyanobacteria fix N2 in the subtropical North Pacific Ocean. Nature 412, 635–638. doi: 10.1038/35088063
Zimmerman, R.. (2006). Light and photosynthesis in seagrass meadows. In: Seagrasses: Biology, Ecology and Conservation, eds. Larkum, A. W. D., Orth, R. J., and Duarte, C. M. (Dordrecht, The Netherlands: Springer Netherlands) 303–321. doi: 10.1007/1-4020-2983-7_13
Keywords: N2 fixation, Mediterranean Sea, Posidonia oceanica, water turbidity, heat waves
Citation: García-Márquez MG, Fernández-Juárez V, Rodríguez-Castañeda JC and Agawin NSR (2022) Response of Posidonia oceanica (L.) Delile and Its Associated N2 Fixers to Different Combinations of Temperature and Light Levels. Front. Mar. Sci. 8:757572. doi: 10.3389/fmars.2021.757572
Received: 12 August 2021; Accepted: 06 December 2021;
Published: 18 January 2022.
Edited by:
Ana Isabel Lillebø, University of Aveiro, PortugalReviewed by:
Nestor Arandia-Gorostidi, Stanford University, United StatesAnna Maria Addamo, Joint Research Centre (JRC), Italy
Copyright © 2022 García-Márquez, Fernández-Juárez, Rodríguez-Castañeda and Agawin. This is an open-access article distributed under the terms of the Creative Commons Attribution License (CC BY). The use, distribution or reproduction in other forums is permitted, provided the original author(s) and the copyright owner(s) are credited and that the original publication in this journal is cited, in accordance with accepted academic practice. No use, distribution or reproduction is permitted which does not comply with these terms.
*Correspondence: Manuela Gertrudis García-Márquez, bWFudWVsYWcuZ2FyY2lhbUB1aWIuZXM=