- 1Marine Resources Center, Marine Biological Laboratory, Woods Hole, MA, United States
- 2Department of Psychology, University of Washington, Seattle, WA, United States
- 3Department of Integrative Biology, University of California, Berkeley, Berkeley, CA, United States
- 4Department of Biology and Institute of Neuroscience, University of Oregon, Eugene, OR, United States
- 5Department of Neuroscience, Johns Hopkins University, Baltimore, MD, United States
- 6Department of Ecology and Evolutionary Biology, Princeton University, Princeton, NJ, United States
Cephalopods have the potential to become useful experimental models in various fields of science, particularly in neuroscience, physiology, and behavior. Their complex nervous systems, intricate color- and texture-changing body patterns, and problem-solving abilities have attracted the attention of the biological research community, while the high growth rates and short life cycles of some species render them suitable for laboratory culture. Octopus chierchiae is a small octopus native to the central Pacific coast of North America whose predictable reproduction, short time to maturity, small adult size, and ability to lay multiple egg clutches (iteroparity) make this species ideally suited to laboratory culture. Here we describe novel methods for multigenerational culture of O. chierchiae, with emphasis on enclosure designs, feeding regimes, and breeding management. O. chierchiae bred in the laboratory grow from a 3.5 mm mantle length at hatching to an adult mantle length of approximately 20–30 mm in 250–300 days, with 15 and 14% survivorship to over 400 days of age in first and second generations, respectively. O. chierchiae sexually matures at around 6 months of age and, unlike most octopus species, can lay multiple clutches of large, direct-developing eggs every ∼30–90 days. Based on these results, we propose that O. chierchiae possesses both the practical and biological features needed for a model octopus that can be cultured repeatedly to address a wide range of biological questions.
Introduction
Cephalopods (octopuses, squid, cuttlefish, and nautiluses) are a diverse and wide-spread class of marine mollusks that have long been useful for scientific research. Unique amongst coleoid cephalopods, octopuses demonstrate higher-order cognitive capabilities commonly attributed to vertebrates, including tool use, problem solving, observational learning, and episodic memory (Hochner et al., 2006; Huffard, 2013; Hanlon and Messenger, 2018). Studies of octopus nervous systems (Young, 1971), dynamic skin patterns (Hanlon et al., 2011), and arm biomechanics (Gutfreund et al., 1996; Sumbre et al., 2005; Tramacere et al., 2014; Maiole et al., 2019), have yielded many foundational insights into physiology, developmental biology, and neurobiology (Shigeno and Ragsdale, 2015; Stubbs and Stubbs, 2016; Di Cosmo et al., 2018; Edsinger and Dölen, 2018; Geffeney et al., 2019; Wang and Ragsdale, 2019; van Giesen et al., 2020). Despite the immense potential for comparative biological research between octopuses and vertebrates and aquaculture advances from the husbandry of other cephalopod species (Van Heukelem, 1977; Hanlon and Forsythe, 1985; Iglesias et al., 2004; Domingues et al., 2012), a sustainable, multigenerational laboratory octopus model has yet to be attained. Several biological and technical reasons hinder the advancement of octopus culture in laboratory settings:
(1) Water quality: Octopuses have a rapid metabolism and a high rate of waste production compared to other marine organisms (Hanlon, 1987; O’Dor and Wells, 1987; Boucher-Rodoni and Mangold, 1995; Lee, 1995). Changes in water parameters, such as high ammonia or nitrogen levels, can greatly impact octopus development and health (Oestmann et al., 1997; Lee et al., 1998). Maintaining optimal water quality at all times is paramount to the success of octopus culture (Boletzky and Hanlon, 1983; Hanlon and Forsythe, 1985; Vidal and von Boletzky, 2014).
(2) Diet: Octopuses are active predators. They require a high protein, low lipid, and low carbohydrate diet (Lee, 1995; Miliou et al., 2005; Domingues et al., 2007; Rosas et al., 2011, 2013), especially to support rapid growth as hatchlings (DeRusha et al., 1989; Lee et al., 1998; Navarro et al., 2014; Vidal et al., 2014). Most laboratory culture of octopuses depend on the use of live or frozen natural food items (Villanueva et al., 2014).
(3) Safe containment: Octopuses are notoriously skilled at escaping containment (Wood and Anderson, 2004; Asada et al., 2021), therefore the design of octopus enclosures must include sufficient measures to prevent their escape. Additionally, cannibalism is common in many octopus species (Hanlon and Forsythe, 2008; Ibánez and Keyl, 2010; Miranda et al., 2011; Hernández-Urcera et al., 2014; Espinoza et al., 2021). Although some species of octopus have been reared in group tanks with moderate success (Domingues et al., 2012), individual enclosures remain the most ethical containment option to minimize the risk of cannibalism or stressful interactions with nearby octopuses. This increases the space, materials, and amount of seawater required to rear octopuses.
(4) Life history attributes: Most octopus species are semelparous and die after one reproductive event (Rocha et al., 2001): females of commonly studied octopus species, including Octopus maya, Octopus vulgaris, and Octopus bimaculoides, lay only one clutch of eggs in their lives (Mangold and Von Boletzky, 1973; Van Heukelem, 1977; Mangold, 1987; Forsythe and Hanlon, 1988). This greatly limits laboratory breeding efforts. Further, some octopus species, such as O. vulgaris, undergo indirect development: females lay large numbers of small eggs that develop into planktonic paralarvae before undergoing metamorphosis to achieve the benthic stage (Iglesias et al., 2004). The paralarval stage is challenging to accommodate in laboratory settings, and multigenerational culture of this species has not yet been achieved (Iglesias et al., 2007). Species undergoing direct development, such as O. bimaculoides and O. chierchiae, produce hatchlings that resemble miniature benthic octopuses when they emerge, with highly developed nervous systems (Yamazaki et al., 2002; Shigeno and Ragsdale, 2015) and complex behaviors, such as hunting and camouflaging (Forsythe and Hanlon, 1988). Thus, direct-developing octopus species may be more amenable to laboratory culture across the entire life cycle.
Here, we overcome these challenges by advancing the laboratory aquaculture of the lesser Pacific striped octopus, Octopus chierchiae (Figure 1). O. chierchiae is native to the central Pacific coast of the Americas (Voss, 1971) and lives in low intertidal zones at water depths up to 40 meters (Rodaniche, 1984). Several unique biological features make O. chierchiae particularly amenable for laboratory culture and scientific research. As adults, O. chierchiae are very small: the largest O. chierchiae adult mantle length previously reported was 25 millimeters (mm) (Rodaniche, 1984). Other octopus study species, such as O. bimaculoides, O. maya, and O. vulgaris, can reach a mantle length three to ten times larger than O. chierchiae (Jereb et al., 2016). Unlike most octopus species, O. chierchiae is iteroparous. Individuals can undergo multiple rounds of reproduction and lay successive clutches of direct-developing eggs before death, which is useful for planning and managing the reproductive outputs of a colony of research animals (Dahm and Geisler, 2006). Mature males and females are visually and behaviorally sexually dimorphic: the tip of the third right arm in male O. chierchiae lacks suckers and is modified with a hectocotylus, a smooth, hooked organ used to pass spermatophores to the female during mating (Voss, 1968; Rodaniche, 1984; Figure 1B). Males also exhibit a visually striking arm-twirling behavior in which they rapidly shake the tips of their arms, which we term “tasseling.” In addition, O. chierchiae are marked by sharply contrasting black and white striping patterns (Figure 1A), which aids in non-invasive identification of individuals.
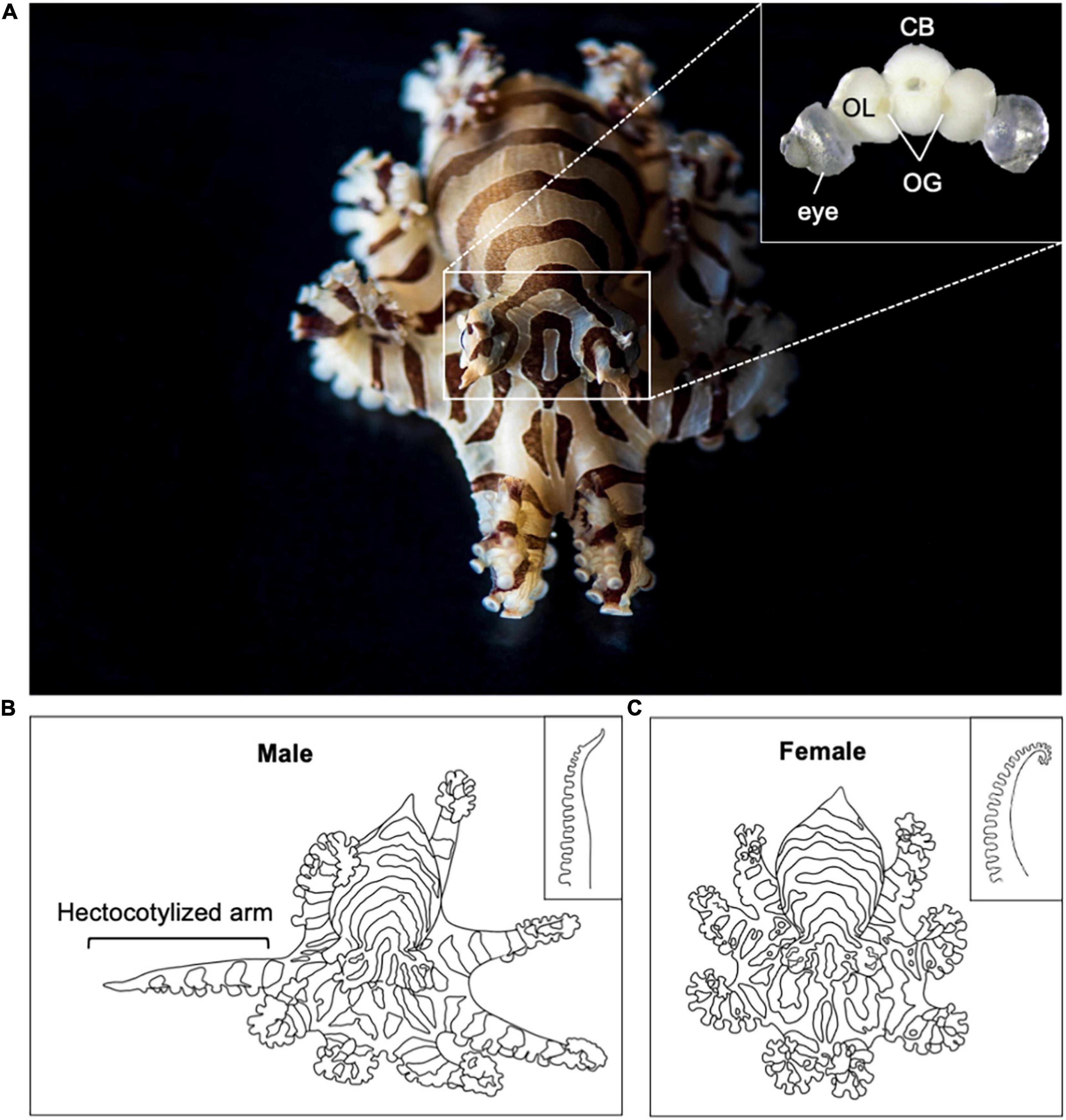
Figure 1. Adult Octopus chierchiae. (A) Dorsolateral view of an adult O. chierchiae, an iteroparous species of octopus native to the Pacific coast of North and Central America. Visual stripes are present 7–14 days after hatching and can be used to distinguish individuals. Inset depicts central nervous system with eyes attached. Two large, kidney-shaped optic lobes (OL) flank the central brain mass (CB; supraesophageal and subesophageal masses). The optic glands (OG) sit on top of the optic tract between the OL and the CB. (B) Illustration of a male O. chierchiae with the hectocotylized arm extended. Male adult O. chierchiae possess a specialized organ on the tip of the third right arm, the hectocotylus (depicted in inset), which has a smooth, sucker-less, hook-like appearance and is used to pass spermatophores to the female during mating. (C) Illustration of a female O. chierchiae, lacking a hectocotylized arm. Female adult O. chierchiae possess suckers along the full length of all arms (depicted in inset). Image A courtesy of Tim Briggs.
Although the small size, unique life history traits, and physical attributes of O. chierchiae make them particularly advantageous for development as a laboratory study organism, culture of O. chierchiae has been attempted multiple times since the 1970s without success. Apart from a single report on its reproductive patterns (Rodaniche, 1984; also see Hofmeister et al., 2011), research and breeding efforts in this species remain largely unreported. Collection of this species has been infrequent and challenging, so little is known about O. chierchiae behavioral ecology in its natural habitat. Ongoing culture of this species is therefore critical for research purposes and for direct observation of their behaviors. Here, we document the first successful multigenerational culture of O. chierchiae at the Marine Biological Laboratory in Woods Hole, MA. Through the creation of new low-cost enclosures using commonly sourced and commercially available materials, an amended diet, and rigorous cleaning and maintenance protocols, we successfully raised O. chierchiae in the laboratory for three generations. Octopuses grew from a 3.5-mm mantle length at hatching to an adult mantle length of approximately 20-30 mm in 250–300 days, with 15 and 14% survivorship to over 400 days of age in first and second generations, respectively. We estimate that O. chierchiae sexually mature at around 6 months of age, and can lay multiple clutches of eggs, approximately every 30–90 days. Based on these results, we propose that O. chierchiae possesses both the practical and biological features needed to become a model species of octopus for study across biological fields (see section “Discussion” for further details).
Materials and Methods
Water Chemistry
All water parameters were kept within optimal ranges as outlined in previous reports of cephalopod culture (Hanlon and Forsythe, 1985; Grasse, 2014). Temperature, salinity, pH, and dissolved oxygen levels were constantly monitored via automated systems (Apex Fusion Neptune System) and an additional water chemistry panel was conducted weekly. pH was tested using an Orion Star A221 meter and maintained between 8.0 and 8.3 using Two Little Fishes Kalkwasser to raise pH values when needed. Seawater temperature was tested using an Orion Star A221 meter and maintained between 22° and 24°C (72°–76°F; 295–297K) using 500w Finnex immersion heaters. Salinity was kept between 33 and 35 parts per thousand (33–35 practical salinity units) and tested using a Hach Hq40d Multi Meter. Salinity was lowered by adding reverse osmosis (RO)/deionized (DI) water or raised by adding hypersaline Marine Enterprise Crystal Sea Bioassay Laboratory Formula salt mix. Dissolved oxygen was tested using a Hach Hq40d and maintained at >80% through water changes and Bubblemac Industry air stones. Ammonia and nitrite levels were measured using a Hach DR3900 spectrophotometer and were maintained at 0 parts per million (ppm) and nitrate levels were kept at < 10 ppm, via water changes and water purification methods described below.
System Design and Tray Maintenance
Both closed and semi-closed seawater systems were successfully implemented for housing and culturing O. chierchiae (Figure 2). Closed systems had a reservoir of artificial seawater that recirculated continuously through multiple filtration steps (mechanical, chemical, and biological, see below) and ultraviolet (UV) sterilization. The artificial seawater was made by mixing Marine Enterprise Crystal Sea Bioassay Laboratory Formula with RO/DI water, and 10% water changes were performed weekly as needed depending on water quality. Closed systems can supply consistent water chemistry and culture conditions throughout the year.
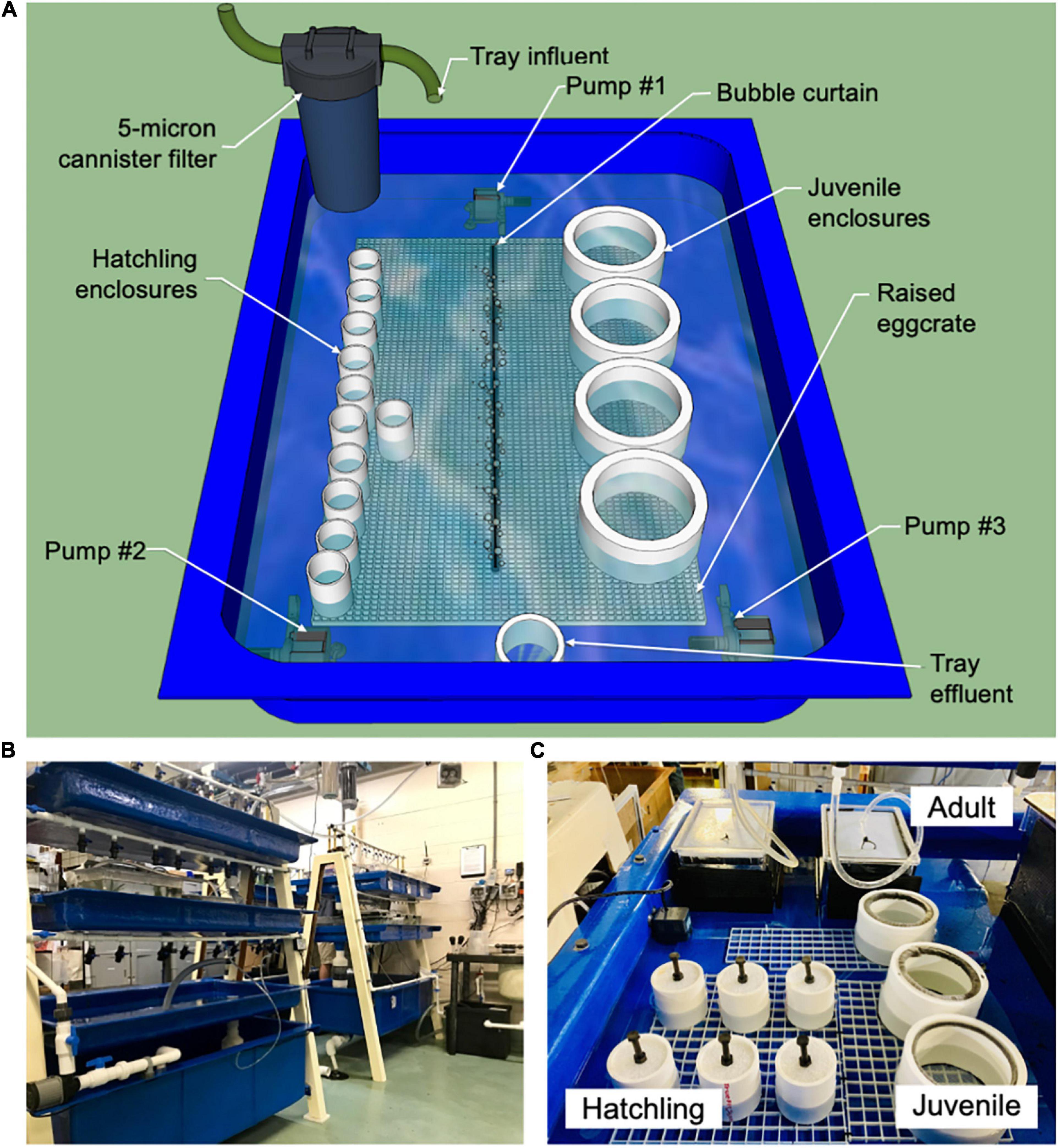
Figure 2. System and enclosure design. (A) Tray design used at the Marine Biological Laboratory. (B) A-frame system design made of fiberglass supports with a large fiberglass reservoir on the bottom and two shallow fiberglass trays above. Water circulates from the reservoir up to the trays via manifolds and discharges back down to the reservoir. Seawater recirculates through the appropriate filtration (mechanical, chemical, and biological) and ultraviolet (UV) sterilization. (C) Adult, juvenile, and hatchling enclosure designs.
Semi-closed seawater systems consisted of filtered seawater from Vineyard Sound, MA with a turnover rate of 200% per day. Prior to entering the systems, the seawater was filtered via a Stark horizontal sand filter consisting of three grades of Dryden Activated Filter Media® and topped with granulated activated carbon. Seawater was then directed through an 80W Emperor Aquatics SMART UV Sterilizer, 50-micron pleated cartridge filter, and granulated activated carbon cartridge and Seachem CuprisorbTM packet. Natural fluctuations in water chemistry in Vineyard Sound throughout the year may impact semi-closed seawater systems.
Both closed and semi-closed systems used aerated, submerged Kaldnes K1 media for biological filtration. Size and wattage of UV sterilizers were dictated by the water volume (100–150 gallons; 378.5–567.8 L) and flow rates of each specific system (∼90 ml/s in fiberglass trays). Submerged granulated activated carbon was used in each reservoir and replaced monthly. All seawater was mechanically filtered via 50-micron bag filters that were changed weekly. Protein skimmers were used in the reservoirs where appropriate and sized according to each system volume. Protein skimmers were cleaned two to three times weekly, the bag filters once weekly, the cartridge filters every 2 weeks, and the entire trays every 1–2 months. These were scrubbed and/or sprayed clean and rinsed with tap water to remove detritus, soaked in 10% bleach/water solution, then soaked in a mixture of 10% sodium thiosulfate (ProlineTM)/ water solution to neutralize the bleach, and finally rinsed thoroughly with DI water before re-use. All systems were kept on a 12-h light/dark cycle.
All systems utilized custom-built A-frame fiberglass supports with a large fiberglass reservoir on the bottom and two shallow fiberglass trays above (Figure 2B). Water circulated from the reservoir up to the trays via manifolds and discharged back down to the reservoir. All individuals were held within the shallow trays of each seawater system, with 7.62–10.16 cm (3–4 inches) of water depth (Figure 2A). In hatchling and juvenile enclosures (described in Section “Enclosure Design and Maintenance”), seawater was exchanged through a fine mesh bottom in each enclosure (Figure 2A). Hatchling and juvenile enclosures were elevated off the tray bottom using 1.27-cm by 1.27-cm (½-inch by ½-inch) square-cell polystyrene egg crate tables to support seawater exchange through each enclosure’s mesh bottom. Seawater exchange in enclosures was further increased by circulating the water in the tray with small submersible pumps (Rio) in each corner and a bubble curtain running lengthwise down the tray (Figure 2A). Adult enclosures received new seawater directly from valve-controlled supply manifolds on each system (Figure 2C). Displaced effluent water exited through a screened bulkhead back to the fiberglass tray. All seawater in contact with non-native organisms at Marine Biological Laboratory was sterilized with ozonation before returning to the ocean.
Enclosure Design and Maintenance
Three enclosure designs were used based on the age and size of the octopus (Figure 2C). After hatching, each O. chierchiae was housed in a separate enclosure. Once an octopus grew to about half the size of its enclosure (i.e., opposite arm tips reached approximately half of the enclosure diameter), it was transferred to a new enclosure of the next size (Table 1). The approximate ages at which we moved animals are indicated below. All enclosures were provided with one or two dens (see Supplementary Figure 1), and juvenile and adult enclosures also included shells and rocks of varying sizes for enrichment. Dirty enclosures, dens, and materials were scrubbed, cleaned, and rinsed using the cleaning methods described in Section “System Design and Tray Maintenance.”
Hatchling Enclosures
In our initial culture efforts, we observed that O. chierchiae hatchlings undergo a period of intense swimming, which has never been reported in this species or others. During this time, hatchlings readily escape through any air gaps in their enclosures and may die by getting lost in the aquaculture system or desiccation. We tested a variety of different materials and enclosure designs, including aversive materials such as Velcro strips, Astroturf, and window pile. We found that an enclosure design with soft, tight-fitting lids, as reported below, is most effective at reducing early hatchling mortality by escape and desiccation.
Hatchlings were housed in 3.18-cm (1¼-inch) polyvinyl chloride (PVC) enclosures with a 125-micron polyester mesh bottom and were provided 0.138-cm (⅛-inch) or 0.635-cm (¼-inch) barbed fittings as dens. Tight-fitting lids (the underside flush with the surface of the water) made of Styrofoam occluded the top opening of the PVC pipe. The lids were removed during feeding, and extra attention was taken to note the location of the octopus during feeds and to ensure the lid was carefully placed back onto the enclosure without crushing the octopus. Hatchlings are very sensitive to changes in water parameters, physical disturbances, or the growth of foreign organisms in their enclosures such as algae, hydroids, or cyanobacteria. To prevent unwanted growth, dirty enclosures, dens, and enrichment materials were replaced with clean ones once every 2 weeks.
Juvenile Enclosures
At approximately 3 months of age, octopuses were moved to 10.16-cm (4-inch) PVC enclosures with an 840-micron polyester mesh bottom and were provided with 90° PVC elbows as dens. To deter octopuses from escaping enclosures, we attached adhesive Velcro (rough hook side exposed) or adhesive weatherstrip window pile to the top-inner rim of the PVC tube. These materials are difficult for octopus suckers to adhere to and successfully prevented escape. Juvenile enclosures were replaced with clean ones once every 3 weeks.
Adult Enclosures
At approximately 6 months of age, octopuses were moved to 2.84 L (23.1-cm by 15.2-cm by 15.5-cm) plastic aquaria, each fitted with an opaque High-Density Polyethylene (HDPE)-weighted lid, seawater supply line, bulkhead, and filter screen allowing for water to flow directly into and out of the enclosure. We reduced potential stress via external visual stimulation by deploying PVC foam barriers, opaque Kydex plastic sheeting, or HDPE plastic sheeting between enclosures to prevent octopuses from seeing each other. A 1250-micron polyester mesh was placed within the filter screen of enclosures with egg-brooding females to prevent any hatchlings from escaping. Adult enclosures were placed directly in the trays as described in Section “System Design and Tray Maintenance” (Figure 2A) and contained various sizes of dens. Adult enclosures were replaced with clean ones once every 6–8 weeks.
Collection and Procurement of Wild Specimens
Historically, O. chierchiae have been spotted at several locations along the Pacific coast of Central America, with a few reported sightings as far North as the Gulf of California. For a full map of these locations, see Supplementary Figure 2. In 2018 and 2019, we purchased seven adult O. chierchiae (five females, two males) from a commercial wholesale vendor, Quality Marine (CA, United States), to establish cultures. These were wild collected from the Pacific intertidal zones of Nicaragua.
Estimating Sexual Maturation
We used indirect measures to determine sexual maturity and readiness for mating. We observed that males perform a “tasseling” behavior in the presence of a female, in which they vigorously twirl the distal tips of their arms (Hofmeister et al., 2011; Supplementary Video 1). A similar behavior has been reported by Caldwell et al. (2015) in mating contexts in the Larger Pacific Striped Octopus (LPSO, scientific name forthcoming), an octopus species that is closely related to O. chierchiae. As such, incidence of tasseling was used as a proxy for sexual maturity in males. In some cases, this behavior was also used to identify the individual as male. From a total of 10 males exhibiting tasseling in their home enclosures, we estimate that males become sexually mature at a mean age of 176 days (SEM: 8.60) (Table 2).
It is challenging to determine sexual maturity in unmated female O. chierchiae using only visual or non-invasive methods. Data from fourteen females who deposited unfertilized eggs suggests that females are sexually mature by a mean age of 232 days (SEM: 6.52) (Table 2). One female, who died at 134 days old, was found to have fully formed, unfertilized eggs in her mantle, which suggests that sexual maturity can occur much earlier. We estimated unmated females to be sexually mature when they surpassed the approximate age at which sibling males began to display the tasseling behavior, or when they began depositing unfertilized eggs (see Rodaniche, 1984).
Mating Trials and Monitoring Egg Development
To initiate mating, adult female O. chierchiae were presented with adult males of a similar or smaller size. Mating trials were performed two to four times per week in one of two methods: (1) introducing the male into the female’s enclosure, or (2) introducing the female followed by the male to a new adult enclosure. In both cases, water flow was turned off and all dens and enrichment materials were removed. No noticeable differences in mating behavior were observed with one method over the other and both were used interchangeably. Mating trials were monitored to ensure the male successfully inserted his hectocotylus into the female’s mantle opening. Once the male and the female separated, octopuses were returned to their home enclosures. If adults began to exhibit any signs of aggression toward each other, we promptly removed individuals from the mating enclosure and returned them to home enclosures. Females and males were paired based on age, size, how recently each individual had mated, and whether they had previously sired/laid a viable clutch of eggs. Individuals were mated repeatedly until they started showing signs of senescence (see section “Ethics Statement”). A mating trial was deemed unsuccessful if a female did not lay a viable clutch of eggs within a month of the mating trial, at which point the female could be mated once again, often with a different male.
After a mating trial, females’ dens were visually inspected during daily feeding for presence of eggs. Once a female laid a clutch of eggs, development of the eggs was monitored weekly until hatching and regular enclosure maintenance (described in Section “Enclosure Design and Maintenance”) was not resumed until after eggs had hatched. The total number of eggs laid was not counted to avoid causing undue stress or otherwise disturbing brooding females. The number of hatchlings produced per clutch of eggs laid per female was counted and the mean calculated for every generation. A one-way analysis of variance (ANOVA) followed by a pairwise Tukey’s multiple comparison test were performed to compare the means using GraphPad Prism version 8.0.0 for Windows (GraphPad Software, San Diego, CA, United States)1.
Feeding
Previous attempts at rearing O. chierchiae in captivity used live brine shrimp as the main food source, with little success (Rodaniche, 1984). We tested a variety of diets for O. chierchiae, including live copepods, frozen shrimp, frozen fish, live grass shrimp, and live crabs. In response to all but the last two food sources, O. chierchiae exhibited no interest in eating (i.e., rejected the food item) and showed increased mortality.
In our study, live-collected grass shrimp (Paelomnetes spp., ∼38 mm from head to tail) from marine estuaries and crabs (Hemigrapsus sanguineus, 35–40 mm in carapace width) from rocky beaches near Woods Hole, MA were offered to O. chierchiae to consume ad libitum. Hatchlings were fed 1/5th of a freshly killed grass shrimp twice daily (morning and afternoon). Individuals in juvenile enclosures were fed half of a freshly killed grass shrimp once daily in the afternoon and leftover food was removed the next morning. Adult males and non-egg-brooding females received a single live crab with the claws removed (to reduce the risk of the prey harming the octopus) once in the afternoon three to four times weekly. Egg-brooding females were hand-fed one whole grass shrimp three to four times weekly. Any leftover food, dead or alive, was removed at the subsequent feeding to minimize waste accumulation.
Measuring Mantle Length
To quantify growth in O. chierchiae, we measured mantle length (tip of mantle to midpoint between the eyes) of up to five hatchlings per clutch every 7 days from hatching until death or the end of the study (see Figure 3). Mantle length was measured by photographing the octopus in a clear Pyrex petri dish filled with filtered seawater resting atop a flat 15-cm (6-inch) ruler. To minimize stress, we tried to take the photo within a minute of moving the octopus to the dish. We collected data from a total of 66 individual octopuses from the first and second generations. Because few third-generation hatchlings were available, they were excluded from this analysis to reduce the risk of stress caused by increased handling. Images were imported to ImageJ for calibration and mantle measurement. Pooled averages of mantle length were taken at 7-day increments (i.e., age 0–7, 7–14, 14–21 days, and so on). In early 2020, the COVID-19 pandemic impeded the collection of growth data, and weekly measurements were terminated. At this point in time, an additional 40 adult octopuses (i.e., >280 days old) were randomly selected for mantle length measurement.
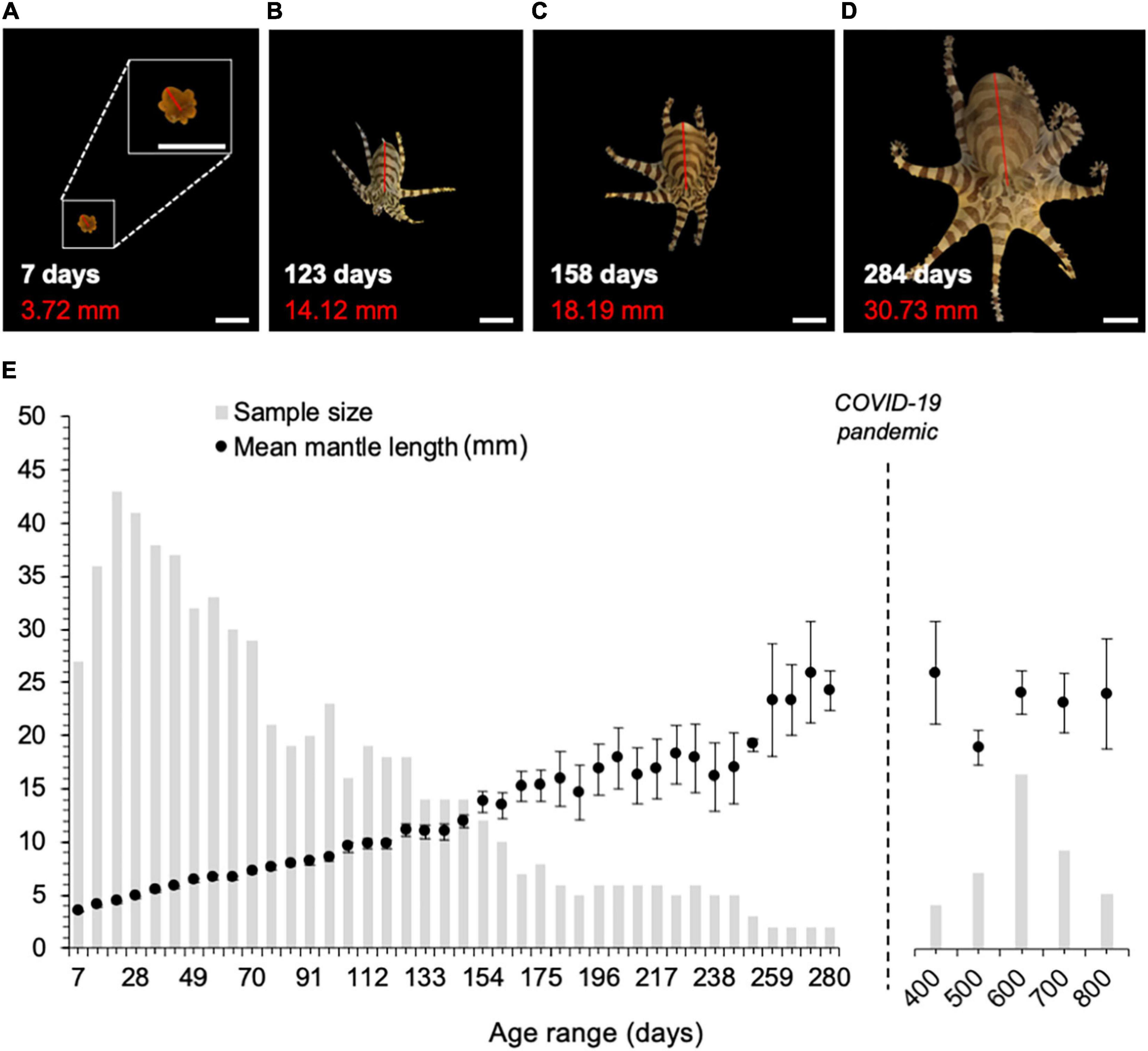
Figure 3. Growth of O. chierchiae over time. (A–D) Images of one individual at 7-, 123-, 158-, and 284-days of age (scale bar: 10 mm). White text is age (in days). Red text is mantle length (in millimeters), depicted by a red line. Inset in (A) shows image at 2x magnification. (E) Average measured mantle length ± SEM over time. For times < 280 days post-hatch, mean mantle lengths were measured for five randomly selected individuals from each clutch (if total cohort size permitted), measured every 7 days from birth. Data points represent pooled average mantle length measured at 7-day increments (i.e., age 0–7, 7–14, 14–21 days, and so on). For 280–800 days, mantle lengths are for 40 randomly selected adult individuals, where the pooled average was calculated for individuals between 280–400, 400–500, 500–600, 600–700, and 700–800 days of age. n, number of mantle length measurements; SEM, standard error of the mean.
Recording Mortalities and Survival Analysis
Cause of mortality and date were recorded at time of death. Natural deaths were defined as any mortality not known to be caused by direct human interference or error and included death due to old age or any unknown health-related issues. Non-natural causes of death included being crushed under enclosure lids (this only occurred with the smallest hatchlings in early containment designs), escape from enclosures, euthanasia with 2–5% ethanol after observing signs of senescence or compromised health, use in experimental diets or enclosures, stress caused by external environmental factors (such as poor water quality), aquaculture system problems, or other unknown causes (i.e., cause of death was not recorded at time of mortality). Available mortality data was pooled, grouped by generation, and used to plot a survival curve of the population by generation. Mean survival in days was calculated for each generation and a one-way ANOVA followed by a pairwise Tukey’s multiple comparison test were performed to compare the means using GraphPad Prism version 8.0.0 for Windows (GraphPad Software, San Diego, CA, United States, see footnote 1). The survival analysis was performed using mortality data from a total of nine first-generation clutches, 15 second-generation clutches, and one third-generation clutch. Individuals whose mortality data was lost or otherwise unavailable (n = 28) were not included in these analyses.
Results
Life History of Octopus chierchiae in Laboratory Culture
We cultured three consecutive generations of O. chierchiae under laboratory conditions from 2018 to 2020. From the original seven wild collected adults, a total of nine first-generation clutches, 15 second-generation clutches, and one third-generation clutches were raised to adulthood. These culture efforts produced a total of 771 octopuses: 350 first-generation, 396 second-generation, and 25 third-generation octopuses (Figures 4A, 5C). The overwhelming majority of lab-cultured individuals exhibited normal physical features, with the notable exception of an octopus with 16 arms from a third-generation clutch, which was found dead in its enclosure at 4 days of age (Supplementary Figure 3).
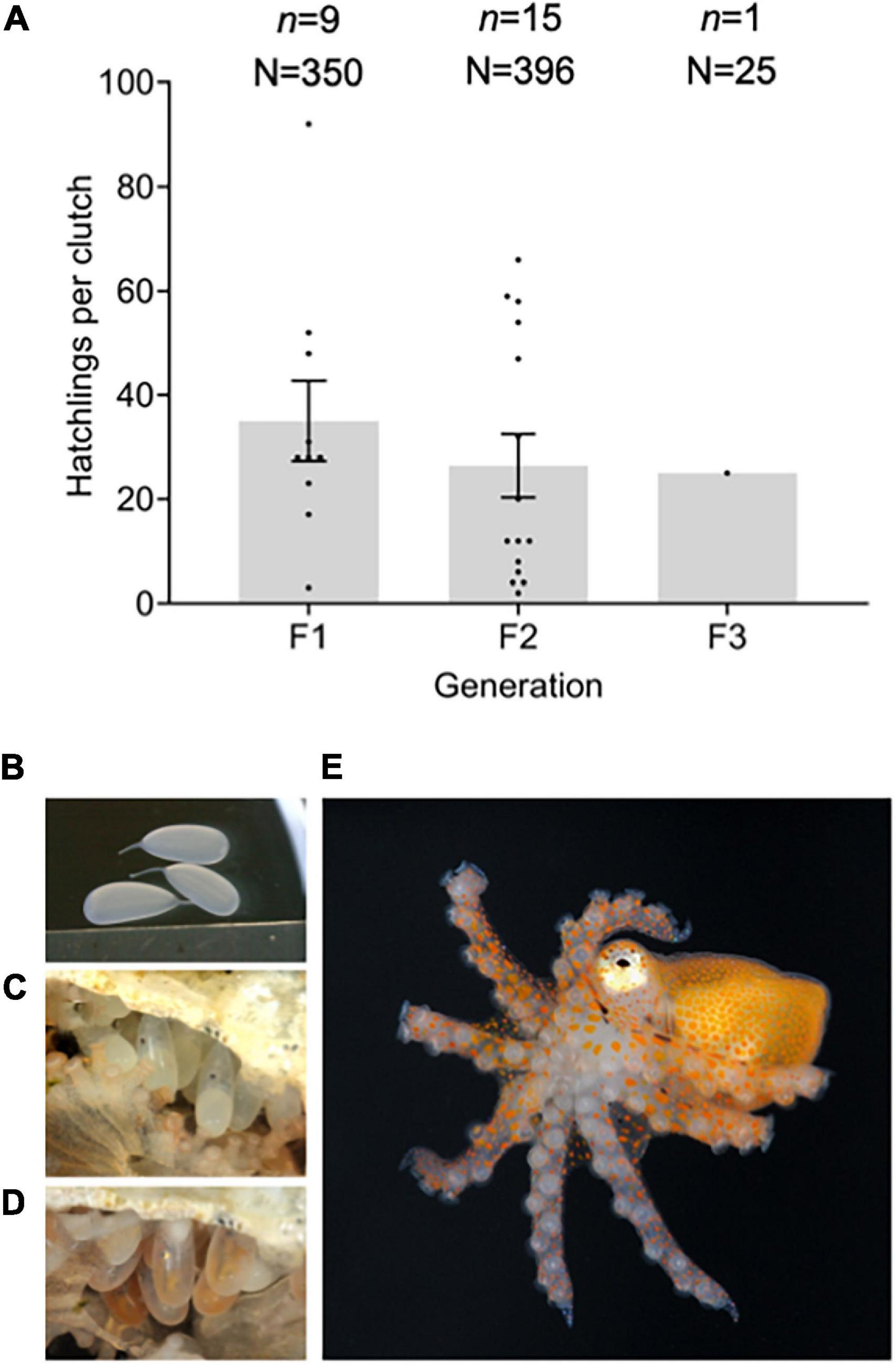
Figure 4. Octopus chierchiae eggs and hatchlings. (A) Hatchlings per clutch by generation. Mean (±SEM) hatchlings per clutch is reported by bars and number of hatchlings for each clutch is reported by black points. A one-way analysis of variance revealed no significant difference between the means of first and second generations (p > 0.05). The number of hatchlings for the one third-generation clutch is reported. (B) Translucent, freshly deposited eggs. (C) Eggs with visible eyespots and yolk sac at approximately 17 days post-laying. (D) Eggs with visible chromatophores at approximately 30 days post-laying. (E) O. chierchiae hatchling with bright orange pigmentation; F1, first generation; F2, second generation; F3, third generation; n, number of clutches; N, total number of hatchlings; SEM, standard error of the mean.
Both males and females exhibited typical signs of octopus senescence (Anderson et al., 2002) after reaching ages of over a year and a half (Supplementary Figure 4). No individuals reproduced after onset of senescence, although senescence did not appear to be triggered by first reproduction, as it is in other octopus species (Wodinsky, 1977; Rodhouse, 1998; Rocha et al., 2001; Anderson et al., 2002; Wang and Ragsdale, 2018).
Mating and Reproduction
We conducted a total of 88 mating trials and 22 octopuses (15 females and 7 males) reproduced. Typically, the female initiated mating by slowly approaching the male, who displayed arm tasseling. This was followed by the male pouncing on the female, grasping her by the mantle from the posterior end, and displaying a pale coloration while the female maintained her dark, banded coloration (Figure 5A; for videos of mating trials, see Supplementary Videos 2, 3). Beak-to-beak mating, a behavior previously seen in this species (Hofmeister et al., 2011) and reported in LPSO (Caldwell et al., 2015), was observed in rare instances and did not lead to egg-laying. Successful mating occurred when the male inserted his hectocotylus into the female’s mantle and delivered spermatophores (Figure 5B), which was visually confirmed in 38 of 88 mating trials. Occasionally, a female would eject a spermatophore during or after mating. The mean duration of time between introducing both octopuses in the mating arena and their separation post-mating was 56.6 min (n = 43 mating trials, SEM: 3.79), but ranged from 15 min to over an hour.
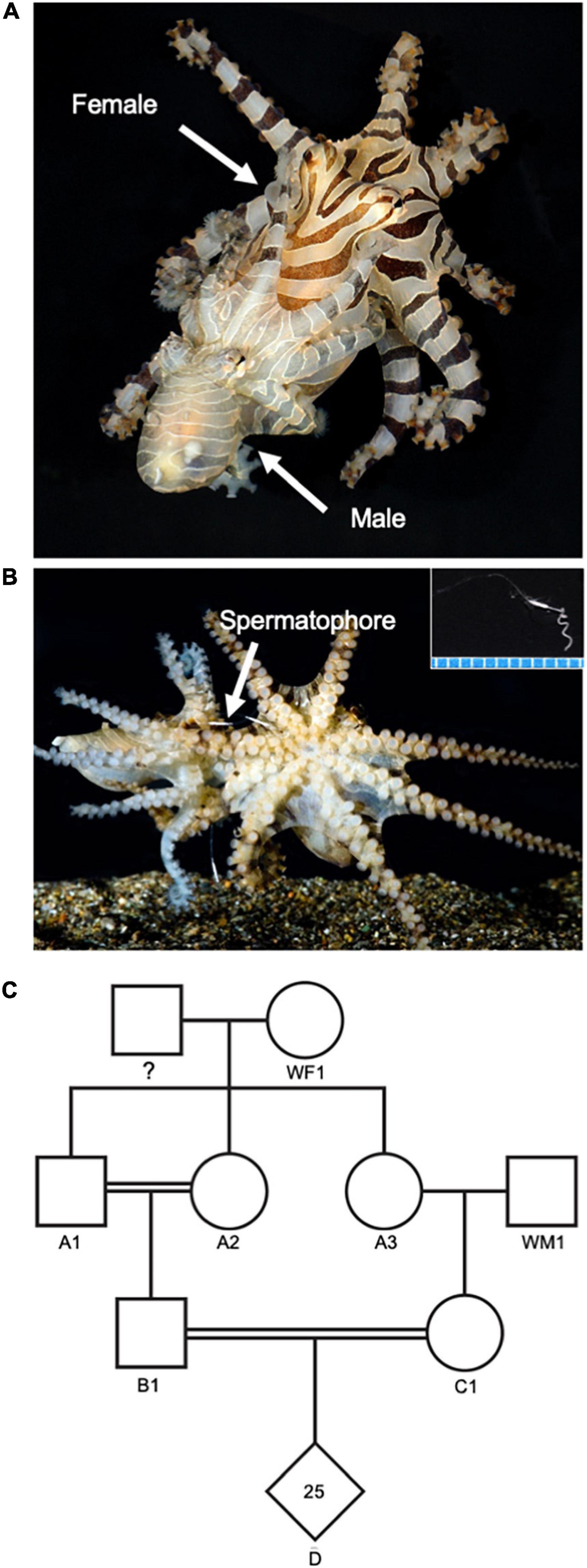
Figure 5. Octopus chierchiae mating and reproduction. (A) Male (smaller octopus with pale coloration) and female (larger octopus with dark banded coloration) mating (dorsolateral view). The male inserts the tip of his third right arm, called the hectocotylus, into the female’s mantle opening to deliver spermatophores. (B) Visible spermatophores during mating. Inset depicts a spermatophore (scale: millimeters). (C) Pedigree showing parental lineage of a third-generation clutch. Squares represent males, circles represent females, diamonds represent clutches, double solid lines highlight inbred pairs. Female WF1 was a wild-collected female. Prior to capture, she had mated unknown male(s) and produced Clutch A upon arrival in the lab. Two offspring from Clutch A (A1 and A2) were bred together and another offspring from Clutch A (A3) was outbred with a wild-collected male (WM1) to produce two second generation clutches. Two individuals from these second-generation clutches (B1 and C1) mated to produce a third-generation clutch (D) with 25 hatchlings. Note: for clarity, non-reproductive offspring of the first- and second-generation clutches are not represented in this pedigree.
Females deposited eggs in their dens between 14 and 30 days after successful mating. Before laying a clutch of eggs, a female’s mantle would swell and, in some cases, eggs could be seen through the partially translucent mantle epidermis by visual inspection. Thirty-one females laid their first clutch of eggs at a mean age of 241 days (SEM: 4.59) (Table 2). Eight females mated in the lab laid multiple clutches; four were wild-collected and four were from the first generation. Five of these females laid two clutches of eggs and the other three females laid three clutches of eggs. The time between laying subsequent clutches of eggs varied between 30 and 90 days. In one instance, a first-generation female laid two consecutive clutches of eggs after a single mating event, separated by 82 days. Four of the wild-caught females laid viable egg clutches before being mated in the laboratory (i.e., they mated with males before capture). One of these females laid two clutches of eggs without ever being introduced to a male in the laboratory, separated by 66 days. Our results align with Rodaniche’s (1984) observations, where a single female O. chierchiae mated twice and laid three viable clutches of eggs, the last of which was laid 83 days after mating. For full data on reproductive mating pairs, see Supplementary Table 1.
While brooding, females remained in their dens and positioned themselves below or next to the eggs. Brooding females continued to feed on shrimp, a behavior that has been previously reported in O. chierchiae (Rodaniche, 1984), and evocative of the first stages of maternal care in O. bimaculoides (Wang and Ragsdale, 2018). Deposited eggs were approximately 6.20 mm in length and were white/translucent in coloration (Figure 4B). Egg development progressed in a similar manner to that of LPSO (Caldwell et al., 2015). At approximately 17 days post-laying, a yolk sac and eyespots could be seen developing in the embryo as two dark spots near the center of the egg (Figure 4C). At approximately 30 days post-laying, bright orange chromatophores could be seen in the developing embryos (Figure 4D). Hatchling octopuses began emerging from eggs between 40 and 50 days after laying. The mean number of hatchlings that emerged per clutch was 35.0 (SEM: 7.7) for first-generation clutches (n = 9), 26.4 (SEM: 6.1) for second-generation clutches (n = 15), and the total hatchlings for the third-generation clutch was 25 (Figure 4A). A one-way ANOVA and Tukey’s multiple comparisons test revealed that there was no significant difference between the means for first and second generations (p > 0.05).
Occasionally, females discarded eggs from their den while brooding. Eggs that were discarded before development of visible embryonic chromatophores did not hatch, while most eggs discarded after formation of embryonic chromatophores hatched if they were moved out of the maternal enclosure to a separate 3.81-cm (1½-inch) PVC enclosure. In four instances, brooding females discarded between 10 and 20 eggs, and the resulting clutch yielded fewer than five hatchlings. Of these, two of the clutches were the second laid by a female while the other two were laid by 1-year-old females from the same first-generation clutch.
Five sexually mature males were mated several times without any observed negative effects to their health. One male in the first generation that survived to 402 days of age was mated eight times (with five different females) and sired eight clutches of eggs, producing a total of 214 viable hatchlings (Supplementary Table 1). Three of these females were siblings from the same clutch of eggs as the male.
Growth
Hatchlings were bright orange (Figure 4E) and stripes began to be visible after 7–14 days. Hatchlings had a mean mantle length of 3.5 mm (SEM: 0.07; n = 27) during the first week of life (Figure 3). Rodaniche (1984) reported a similar mantle length in laboratory-raised O. chierchiae hatchlings, but due to lack of appropriate food, growth remained static after 2 weeks, and mortality increased. On a diet of Palaemonetes shrimp and H. sanguineus crabs, our results suggest that mantle length growth is linear from birth until approximately 150–200 days of age (Supplementary Figure 5), after which it becomes asymptotic (Figure 3). Variability in average mantle length increased dramatically after ∼180 days post-hatch, as many individuals in the growth group died before reaching the end of the study and sample size decreased (Figure 3). Based on the data from 40 randomly sampled adults between 280 and 800 days of age, average mantle length did not increase with age after 280 days post-hatch (Figure 3). The maximum mantle length we observed was 40 mm in two individuals: one at 550 days post-hatch and the other at 799 days post-hatch, both of which were from the group of randomly sampled adults.
Survival
Across all three generations, mortality was highest during the first 30–60 days post-hatch. The largest drop in survival occurred in the first 5 days post-hatch in the third generation (Figure 6A). The mean survival for first, second, and third generations was 128.7 days (SEM: 9.9), 133.3 days (SEM: 8.5), and 25.4 days (SEM: 13.7) respectively (Figure 6B). Pair-wise multiple comparisons revealed that mean survival in the third generation was significantly lower than in first and second generations (p ≤ 0.05.) and there was no significant difference between survival in first and second generations (Figure 6B).
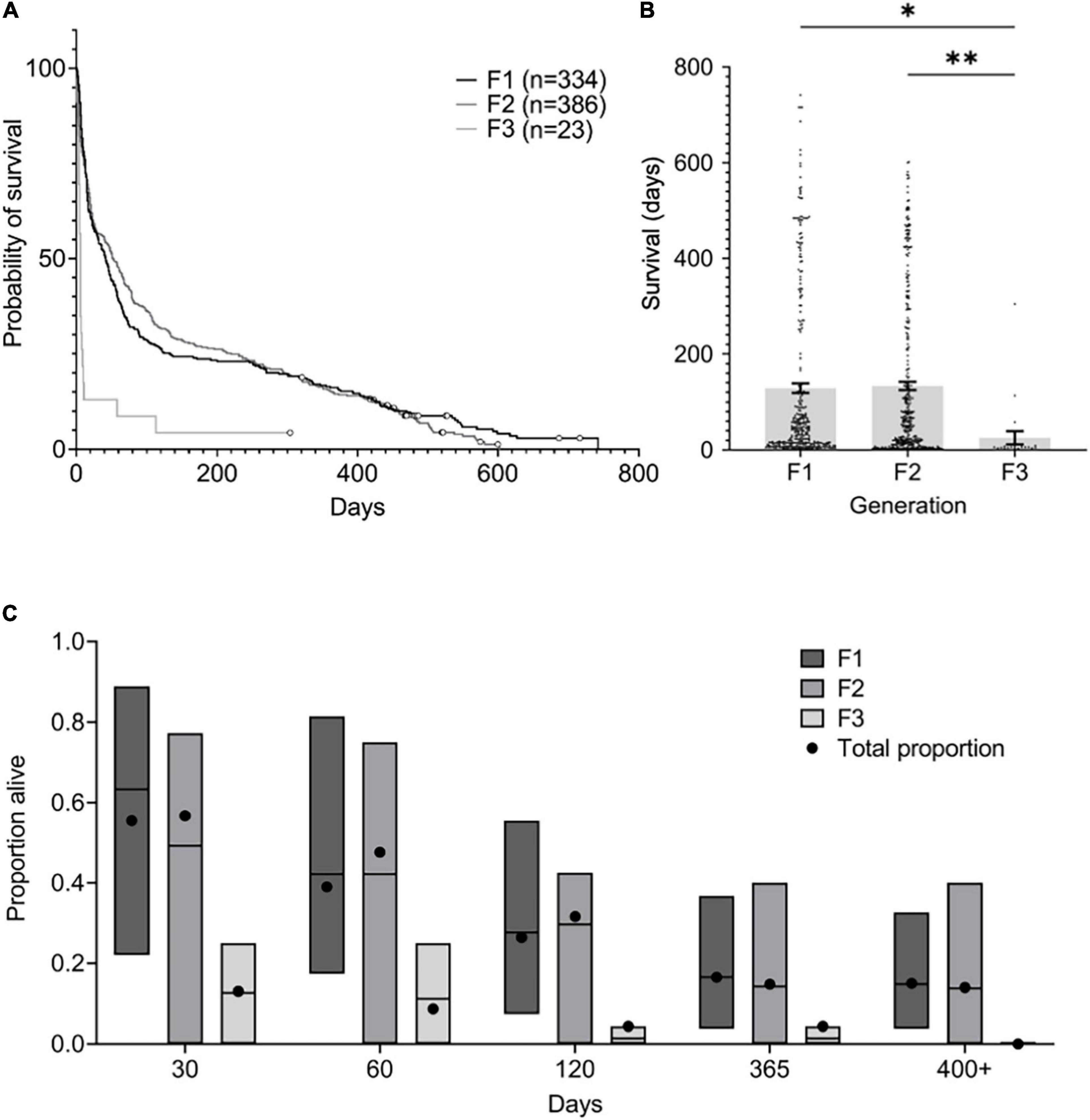
Figure 6. Octopus chierchiae survival by generation. Mortality data was pooled and grouped by generation. (A) Survival curve by generation. Individuals surviving to the end of the study were treated as censored observations, represented by circles. (B) Survival (in days) by generation. Mean survival (±SEM) is reported by bars and individual survival is reported as black points. A one-way analysis of variance followed by Tukey’s multiple comparison test revealed that mean survival in the first and second generations was significantly higher than in the third generation. (C) Proportion alive at five timepoints by generation. Mean proportion alive per clutch and spread (minimum and maximum proportion) are reported by bars. Total proportion of all individuals alive in each generation is reported as black circles. F1, first generation; F2, second generation; F3, third generation; n, number of individuals; SEM, standard error of the mean; *statistically significant at p ≤ 0.05; **statistically significant at p ≤ 0.005.
A total of 50 (15.0%) and 54 (14.0%) individuals survived to 400 days or older in the first- and second-generation clutches, respectively (Figure 6C). Of these, 11 (3.3%) survived to >500 days of age and three (0.9%) to >600 days of age in the first generation, while 13 (3.4%) survived to >500 days of age and one (0.3%) to >600 days of age in the second generation. A single individual from the third-generation clutch (4.3%) survived to 387 days of age. The oldest O. chierchiae we recorded in this study was a first-generation individual that survived to 742 days of age.
A total of 315, 367, and 22 mortalities were recorded for first, second, and third generations, respectively. Across all three generations, the largest proportion of mortalities were natural deaths: 54.9, 76.6, and 100% for first, second, and third generations, respectively (Table 3). The proportions of mortalities due to human error and desiccation were highest in the first generation and decreased in subsequent generations (Table 3).
Discussion
Octopuses offer a unique and exceptional opportunity to compare complex nervous systems, behaviors, and development across evolutionary distant and anatomically divergent invertebrate and vertebrate model organisms. Here, we leveraged the small size and unique life history traits of O. chierchiae to establish the first multigenerational laboratory aquaculture of the species. Over 2 years, we successfully reared three generations of O. chierchiae from an original seven wild-caught individuals, to establish a breeding population spanning all life stages.
Size and Growth Considerations
Octopus chierchiae hatchlings and juveniles grow rapidly during the first 150–200 days of life, reaching an adult mantle length of ∼25 mm around 280 days post-hatch (Figure 3). These results suggest that growth rates become asymptotic in adulthood, although this may be because all adults were fed a standard diet, regardless of body size or reproductive status. Evidence from other cultured octopus species demonstrates that diet impacts growth rate and size (García and Valverde, 2006; Domingues et al., 2007; Rosas et al., 2007; Pham and Isidro, 2009; Prato et al., 2010; Baeza-Rojano et al., 2013), and future studies should investigate the effects increasing diet on O. chierchiae growth rates, fecundity, and lifespan.
Given their small adult body size, O. chierchiae require significantly less physical space to house individually compared to other octopus species. The maximum recorded mantle length of O. chierchiae in culture to date (40 mm) is much smaller than that of other common octopus study systems, such as O. bimaculoides (85 mm mantle length), O. maya (120 mm), and O. vulgaris (250 mm) respectively (Jereb et al., 2016). Previous studies have housed individual O. maya in 45 L tanks (Walker et al., 1970), O. bimaculoides in 76 L tanks (Sinn et al., 2001), and O. vulgaris in 150 L tanks (Fiorito and Gherardi, 1999), while here we show that adult O. chierchiae can be housed in 2.84 L tanks. Larger species of octopus also require more food and consequently produce more nitrogenous wastes (Boucher-Rodoni and Mangold, 1995; Oestmann et al., 1997; Lee et al., 1998). Culturing smaller octopuses like O. chierchiae thus reduces the biological load per individual on the seawater system.
Survival and Lifespan
Octopus chierchiae mortality is highest in the first 30 days post-hatch across all generations, with hatchlings regularly surviving past 100 days (Figure 6), after which mortality decreases with age. Our data suggest that once O. chierchiae grow past a highly sensitive hatchling stage, survival to sexual maturity is highly likely. This is consistent with the low survival rate of paralarval octopus hatchlings in the wild (Laptikhovsky, 1998; Rocha et al., 2001) and a previous report of culturing O. chierchiae in which 4 out of 26 hatchlings reached a maximum age of 67 days before dying (Rodaniche, 1984). This differs from published accounts of culturing other octopus species, in which mortality rates are steady over the entire life cycle (Hanlon and Forsythe, 1985).
Additionally, ∼14% of individuals in first- and second-generation clutches survived to over 400 days of age (Figure 6). Survival is lowest in the third generation animals, likely due to a combination of (1) the shutdown of the Marine Biological Laboratory at the onset of the COVID-19 pandemic, (2) inbreeding depression (see below), and (3) seasonal parameters, such as changes in the incoming water source in semi-closed seawater systems or innate hormonal changes correlated to their reproductive biology. Additional studies should explore these possibilities in order to increase our understanding of O. chierchiae biology, survival, and lifespan. With further optimization of temperature and diet, cultured O. chierchiae may regularly live for up to 2 years and remain fertile for the majority of adulthood (Rodaniche, 1984). These results demonstrate that O. chierchiae has a comparable, if not longer, lifespan than many other octopus species commonly reared in culture, such as O. bimaculoides [1.5 years (Jereb et al., 2016) and O. maya (300 days, Van Heukelem, 1977)]. This would allow for observations and data collection on adult O. chierchiae for extensive periods of time while continuing to breed individuals to sustain the ongoing culture.
Reproductive Behaviors of Octopus chierchiae
Unlike most cephalopod species (Mangold, 1987; Cortez et al., 1995; Rocha et al., 2001), O. chierchiae are iteroparous (Rodaniche, 1984; Hofmeister et al., 2011), which is extremely beneficial for laboratory culture. We show that female O. chierchiae are capable of laying a clutch of 11–35 eggs approximately every 30–90 days, while males are capable of mating several times without any apparent negative physiological impact. Four of the wild-collected females laid one or more clutches of eggs before exposure to a male in the laboratory, suggesting that females can store sperm for extended periods of time. Sperm storage and sperm competition (Wigby and Chapman, 2004) has been previously reported in O. chierchiae (Rodaniche, 1984) and in other cephalopod species (Hanlon et al., 1999; Naud et al., 2005, 2016; Wada et al., 2005, 2010; Squires et al., 2015).
Our data show that estimating sexual maturity with appearance of tasseling behavior in males or first incidence of egg-depositing in females is sufficient to sustain an octopus breeding program that produces large numbers of hatchlings. Based on the results from this study, we estimate that O. chierchiae reach sexual maturity at approximately 6 months of age. Anecdotal necropsy observations from Rodaniche (1984) revealed the presence of eggs in females as young as 67 days of age. Similarly, we found eggs in a female that died at 134 days of age. It is possible that females become sexually mature much earlier than reported here, but do not lay eggs in the absence of sexually mature males. Future investigations are needed to determine how environmental factors, such as diet, temperature, and presence of conspecifics affect rate of sexual maturation.
Applications in Research
As Young (1964) outlined in his classic work A Model of the Brain, octopuses offer a unique opportunity to discover ‘the rules’ of how to build complex brain function by comparing solutions across invertebrate and vertebrate nervous systems. Toward this goal, the advances in octopus husbandry we report here make O. chierchiae an especially advantageous model organism for developmental, neurobiological, and behavioral study. Their predictable and repetitive reproduction provides a continuous supply of large, transparent eggs and direct-developing hatchlings. Transparent eggs permit access to the brain and other tissues with modern imaging techniques, such as calcium imaging, computed micro-tomography (micro-CT; Kerbl et al., 2013), ultrasound (King et al., 2005; Grimaldi et al., 2007; Margheri et al., 2011), and magnetic resonance imaging (MRI; Tramacere et al., 2012; Jiang et al., 2014). This makes them an excellent candidate for the study of embryological development and for pioneering CRISPR-Cas9 gene-editing technology, recently demonstrated in squid embryos (Crawford et al., 2020). Additionally, hatchlings exhibit many behavioral features as adults, such as hunting, evasion, and camouflage, all of which require exquisite control of the motor, chemotactile, and visual systems (Messenger, 1967, 2001; Mather, 1991; Yamazaki et al., 2002; Mäthger et al., 2012; Shigeno and Ragsdale, 2015; Wild et al., 2015; Chiao and Hanlon, 2019). However, little is known about the underlying neural circuits that process sensory information, or how the neurobiological strategies that underly these behaviors may change as the animal grows in size. A colony of O. chierchiae provides an opportunity to closely investigate the development and control of sensory systems across the entire lifespan. Thus, the promise of O. chierchiae as a standardized genetic model system plays a pivotal role toward a mechanistic neurobiological understanding of the octopus’s complex sensory systems and behaviors.
Iteroparity itself is interesting to study because it sets O. chierchiae apart from most other octopus species. In semelparous octopuses, reproduction triggers the signaling of the optic glands, the octopus analog of the vertebrate pituitary gland (Wells and Wells, 1969; Wang and Ragsdale, 2018). The optic gland signaling systems are responsible for post-mating behavioral changes, including maternal behaviors and death, and have recently been characterized in O. bimaculoides for the first time (Wang and Ragsdale, 2018). How the optic glands control reproduction in an iteroparous species is entirely unexplored. A lab-cultured colony of O. chierchiae would lay the foundation for investigating the interplay between reproduction and control of lifespan between iteroparous and semelparous octopus species, and accelerate the study of octopus reproductive neuroendocrinology, senescence, and aging.
Furthermore, O. chierchiae is closely related to the Larger Pacific Striped Octopus (LPSO), the only known social species of octopus (Caldwell et al., 2015). The LPSO is known to display unique behaviors such as beak-to-beak mating and mating pairs sharing a den (Caldwell et al., 2015). Importantly, some of the earliest insights into the brain mechanisms underlying social behaviors came from another such pair of asocial/social vertebrate sister species (montane and prairie voles) (Donaldson and Young, 2008). Unlike O. chierchiae, however, the LPSO deposits small eggs and hatchlings undergo indirect development (Caldwell et al., 2015; Jereb et al., 2016). The existence of another comparator pair in invertebrates presents an enticing opportunity to test evolutionary hypotheses with the ultimate goal of discovering universal synaptic and circuit motifs that govern sociality across species.
Current Challenges to Octopus chierchiae Culture
Octopus chierchiae culture faces several challenges. In addition to the careful attention to system design, feeding, and enclosure maintenance required to reduce mortality during the sensitive hatchling phase, our results suggest that inbreeding depression (Charlesworth and Charlesworth, 1987) begins to occur in the third generation. Although data from only one third-generation clutch was available, signs that point toward inbreeding depression in the third generation include: (1) significantly reduced survivorship, particularly in the first week of life (Figure 6) and (2) the discovery of a 16-armed octopus (Supplementary Figure 3), possibly due to genetic mutations from the small gene pool. Given that our study began with only seven wild-collected individuals, this observation is not surprising. These findings emphasize the need to periodically supplement the population with new genetics via wild-collected octopuses. Decreased fertility in later generations of laboratory culture has been demonstrated in several cephalopod species including the common cuttlefish, S. officinalis (Forsythe et al., 1994), the flamboyant cuttlefish, Metasepia pfefferi (Grasse, 2014), and the bigfin reef squid, Sepioteuthis lessoniana (Walsh et al., 2002). More research on inbreeding depression and fecundity in O. chierchiae is required to help identify genetic bottlenecks and modify protocols to optimize breeding efficiency. Additionally, due to the elusiveness of O. chierchiae, little is known about their behavior in situ. Future field studies that investigate O. chierchiae distribution patterns, preferred habitats, natural diets, and typical reproductive behaviors will greatly improve laboratory husbandry practices.
Conclusion
Octopus chierchiae is an ideal candidate model organism due to its small size, iteroparity, predictable reproduction, and direct-developing hatchlings. Various aspects of octopus behavior and biology, including a rapid metabolism that emphasizes the need for optimal water quality and frequent feeds, an affinity for escaping enclosures, and cannibalistic tendencies, make laboratory culture challenging. We have developed a culture program for O. chierchiae that optimizes reproductive success and survivorship to address these challenges. Starting with seven wild-collected individuals, we successfully reared three generations of O. chierchiae, generating large numbers of octopuses sufficient for maintaining a lab population spanning all life stages for over 2 years. In the long term, periodically supplementing an O. chierchiae culture with new genetic pools from wild-collected octopuses likely would reduce inbreeding-related defects and mortality, which will be important for maintaining a healthy, sustainable culture of O. chierchiae.
Data Availability Statement
The raw data supporting the conclusions of this article will be made available by the authors, without undue reservation.
Ethics Statement
Ethical review and approval was not required for the animal study because in the United States, cephalopods are not included in federal regulations that govern the use of animals in research laboratories, thus no protocol or approval number was required for this study. However, the Marine Biological Laboratory voluntarily upholds a Cephalopod Care Policy and the care of the animals in this study adhered to that policy. All animal care and handling followed methods established by the regulations of the Directive 2010/63/EU for cephalopods (Sykes et al., 2012; Smith et al., 2013; Fiorito et al., 2015) and institutional standards for cephalopod care.
Author Contributions
AG, AD, TS, and BG contributed to the conception and design of the study. AG, AD, TS, BG, and ZYW analyzed the data and wrote the manuscript. All the authors contributed to manuscript revision, read, and approved the submitted version.
Funding
The cephalopod program at the Marine Biological Laboratory (MBL) was supported by NSF 1827509 and NSF 1723141 grants. CN received funding from HFSP RGP0042. DG and DS received funding and research support from the University of Washington Friday Harbor Laboratories. ZYW was supported by funds from the Whitman Center at the MBL.
Conflict of Interest
The authors declare that the research was conducted in the absence of any commercial or financial relationships that could be construed as a potential conflict of interest.
Publisher’s Note
All claims expressed in this article are solely those of the authors and do not necessarily represent those of their affiliated organizations, or those of the publisher, the editors and the reviewers. Any product that may be evaluated in this article, or claim that may be made by its manufacturer, is not guaranteed or endorsed by the publisher.
Acknowledgments
We gratefully acknowledge the pioneering culture efforts of Rich Ross, Danielle Dallis, Travis Snyder, Benjamin Liu, Anna Ramji, Leo Song, and Saumitra Kelkar; Matthew Everett, Peter Kilian, and all former interns in the Cephalopod Program at the Marine Biological Laboratory (MBL) for assistance with data collection and ongoing O. chierchiae culture; and Philip Swiny, Carlos Carstens, Juan-chi, Vanessa Grasse, and William Wcislo (Smithsonian Tropical Research Institute) for support with field collection investigations. We thank Roger Hanlon, Jonathon Stone, David Welch, and the two reviewers for improving earlier versions of the manuscript, Tim Briggs for his exceptional photographs of the octopuses, and Caroline Albertin and Sarah Kocher for research support. We are grateful for Joshua Rosenthal’s support of the MBL’s cephalopod culture efforts, and for John Sigel and Sally Reid’s support of the Northeastern University Co-op Internship Program at the MBL.
Supplementary Material
The Supplementary Material for this article can be found online at: https://www.frontiersin.org/articles/10.3389/fmars.2021.753483/full#supplementary-material
Footnotes
References
Anderson, R. C., Wood, J. B., and Byrne, R. A. (2002). Octopus senescence: the beginning of the end. J. Appl. Anim. Welf. Sci. 5, 275–283. doi: 10.1207/S15327604JAWS0504_02
Asada, K., Nakajima, R., Nishibayashi, T., Ziadi-Künzli, F., Lajbner, Z., Miller, J., et al. (2021). Improving keeping for octopuses by testing different escape-proof designs on tanks for “Big Blue Octopus” (Octopus cyanea). Appl. Sci. 11:8547. doi: 10.3390/app11188547
Baeza-Rojano, E., Domingues, P., Guerra-García, J. M., Capella, S., Noreña-Barroso, E., Caamal-Monsreal, C., et al. (2013). Marine gammarids (Crustacea: Amphipoda): a new live prey to culture Octopus maya hatchlings. Aquac. Res. 44, 1602–1612. doi: 10.1111/j.1365-2109.2012.03169.x
Boletzky, S. V., and Hanlon, R. T. (1983). A review of the laboratory maintenance, rearing and culture of cephalopod molluscs. Mem. Natl. Mus. Vic. 44, 147–187. doi: 10.24199/j.mmv.1983.44.11
Boucher-Rodoni, R., and Mangold, K. (1995). Ammonia production in cephalopods, physiological and evolutionary aspects. Mar. Freshw. Behav. Physiol. 25, 53–60. doi: 10.1080/10236249409378907
Caldwell, R. L., Ross, R., Rodaniche, A., and Huffard, C. L. (2015). Behavior and body patterns of the larger Pacific striped octopus. PLoS One 10:e0134152. doi: 10.1371/journal.pone.0134152
Charlesworth, D., and Charlesworth, B. (1987). Inbreeding depression and its evolutionary consequences. Annu. Rev. Ecol. Syst. 18, 237–268. doi: 10.1146/annurev.es.18.110187.001321
Chiao, C., and Hanlon, R. (2019). “Rapid adaptive camouflage in cephalopods,” in Oxford Research Encyclopedia of Neuroscience. Available online at: https://oxfordre.com/neuroscience/view/10.1093/acrefore/9780190264086.001.0001/acrefore-9780190264086-e-182 (accessed November 12, 2021).
Cortez, T., Castro, B. G., and Guerra, A. (1995). Reproduction and condition of female Octopus mimus (Mollusca: Cephalopoda). Mar. Biol. 123, 505–510. doi: 10.1007/BF00349229
Crawford, K., Quiroz, J. F. D., Koenig, K. M., Ahuja, N., Albertin, C. B., and Rosenthal, J. J. (2020). Highly efficient knockout of a squid pigmentation gene. Curr. Biol. 30, 3484–3490. doi: 10.1016/j.cub.2020.06.099
Dahm, R., and Geisler, R. (2006). Learning from small fry: the zebrafish as a genetic model organism for aquaculture fish species. Mar. biotechnol. 8, 329–345. doi: 10.1007/s10126-006-5139-0
DeRusha, R. H., Forsythe, J. W., DiMarco, F. P., and Hanlon, R. T. (1989). Alternative diets for maintaining and rearing cephalopods in captivity. Lab. Anim. Sci. 39, 306–312.
Di Cosmo, A., Bertapelle, C., Porcellini, A., and Polese, G. (2018). Magnitude assessment of adult neurogenesis in the Octopus vulgaris brain using a flow cytometry-based technique. Front. Physiol. 9:1050. doi: 10.3389/fphys.2018.01050
Domingues, P., López, N., and Rosas, C. (2012). Preliminary trials on the use of large outdoor tanks for the ongrowing of Octopus maya juveniles. Aquac. Res. 43, 26–31. doi: 10.1111/j.1365-2109.2011.02797.x
Domingues, P. M., López, N., Muñoz, J. A., Maldonado, T., Gaxiola, G., and Rosas, C. (2007). Effects of a dry pelleted diet on growth and survival of the Yucatan octopus, Octopus maya. Aquac. Nutr. 13, 273–280. doi: 10.1111/j.1365-2095.2007.00474.x
Donaldson, Z. R., and Young, L. J. (2008). Oxytocin, vasopressin, and the neurogenetics of sociality. Science 322, 900–904. doi: 10.1126/science.1158668
Edsinger, E., and Dölen, G. (2018). A conserved role for serotonergic neurotransmission in mediating social behavior in octopus. Curr. Biol. 28, 3136–3142. doi: 10.1016/j.cub.2018.07.061
Espinoza, V., Farías, A., and Uriarte, I. (2021). Characterization of cannibalism in early paralarvae of Patagonian red octopus, Enteroctopus megalocyathus (Gould, 1852), grown in a controlled environment. J. World Aquac. Soc. 52, 475–481. doi: 10.1111/jwas.12738
Fiorito, G., Affuso, A., Basil, J., Cole, A., de Girolamo, P., D’angelo, L., et al. (2015). Guidelines for the care and welfare of cephalopods in research –a consensus based on an initiative by CephRes, FELASA and the boyd group. Lab. Anim. 49, 1–90. doi: 10.1177/0023677215580006
Fiorito, G., and Gherardi, F. (1999). Prey-handling behaviour of Octopus vulgaris (Mollusca, Cephalopoda) on bivalve preys. Behav. Process. 46, 75–88. doi: 10.1016/S0376-6357(99)00020-0
Forsythe, J. W., DeRusha, R. H., and Hanlon, R. T. (1994). Growth, reproduction and life span of Sepia officinalis (Cephalopoda: Mollusca) cultured through seven consecutive generations. J. Zool. 233, 175–192. doi: 10.1111/j.1469-7998.1994.tb08582.x
Forsythe, J. W., and Hanlon, R. T. (1988). Effect of temperature on laboratory growth, reproduction and life span of Octopus bimaculoides. Mar. Biol. 98, 369–379. doi: 10.1007/BF00391113
García, B. G., and Valverde, J. C. (2006). Optimal proportions of crabs and fish in diet for common octopus (Octopus vulgaris) ongrowing. Aquaculture 253, 502–511. doi: 10.1016/j.aquaculture.2005.04.055
Geffeney, S. L., Williams, B. L., Rosenthal, J. J., Birk, M. A., Felkins, J., Wisell, C. M., et al. (2019). Convergent and parallel evolution in a voltage-gated sodium channel underlies TTX-resistance in the greater blue-ringed octopus: Hapalochlaena lunulata. Toxicon 170, 77–84. doi: 10.1016/j.toxicon.2019.09.013
Grasse, B. (2014). The biological characteristics, life cycle, and system design for the flamboyant and paintpot cuttlefish, Metasepia sp., cultured through multiple generations. Drum Croaker 45, 58–71.
Grimaldi, A. M., Agnisola, C., and Fiorito, G. (2007). Using ultrasound to estimate brain size in the cephalopod Octopus vulgaris Cuvier in vivo. Brain Res. 1183, 66–73. doi: 10.1016/j.brainres.2007.09.032
Gutfreund, Y., Flash, T., Yarom, Y., Fiorito, G., Segev, I., and Hochner, B. (1996). Organization of octopus arm movements: a model system for studying the control of flexible arms. J. Neurosci. 16, 7297–7307. doi: 10.1523/JNEUROSCI.16-22-07297.1996
Hanlon, R., and Messenger, J. (2018). Cephalopod Behaviour, 2nd Edn. Cambridge: Cambridge University Press. doi: 10.1017/9780511843600
Hanlon, R. T. (1987). “Mariculture,” in Cephalopod Life Cycles, Volume II: Comparative Reviews, ed. P. R. Boyle (London: Academic Press), 291–305.
Hanlon, R. T., Ament, S. A., and Gabr, H. (1999). Behavioral aspects of sperm competition in cuttlefish, Sepia officinalis (Sepioidea: Cephalopoda). Mar. Biol. 134, 719–728. doi: 10.1007/s002270050588
Hanlon, R. T., Chiao, C. C. C., Mathger, L. M., Buresch, K. C., Barbosa, A., Allen, J. J., et al. (2011). “Rapid adaptive camouflage in cephalopods,” in Animal Camouflage, ed. S. Merilaita (Cambridge: University Press), 145–163. doi: 10.1017/cbo9780511852053.009
Hanlon, R. T., and Forsythe, J. W. (1985). Advances in the laboratory culture of octopuses for biomedical research. Lab. Anim. Sci. 35, 33–40.
Hanlon, R. T., and Forsythe, J. W. (2008). Sexual cannibalism by Octopus cyanea on a Pacific coral reef. Mar. Freshw. Behav. Physiol. 41, 19–28. doi: 10.1080/10236240701661123
Hernández-Urcera, J., Garci, M. E., Roura, Á, González, ÁF., Cabanellas-Reboredo, M., Morales-Nin, B., et al. (2014). Cannibalistic behavior of octopus (Octopus vulgaris) in the wild. J. Comp. Psychol. 128:427. doi: 10.1037/a0036883
Hochner, B., Shomrat, T., and Fiorito, G. (2006). The octopus: a model for a comparative analysis of the evolution of learning and memory mechanisms. Biol. Bull. 210, 308–317. doi: 10.2307/4134567
Hofmeister, J. K., Alupay, J. S., Ross, R., and Caldwell, R. L. (2011). Observations on mating behavior and development in the lesser Pacific striped octopus, Octopus chierchiae (Jatta, 1889). Integr. Comp. Biol. 51, E58–E58.
Huffard, C. L. (2013). Cephalopod neurobiology: an introduction for biologists working in other model systems. Invert. Neurosci. 13, 11–18. doi: 10.1007/s10158-013-0147-z
Ibánez, C. M., and Keyl, F. (2010). Cannibalism in cephalopods. Rev. Fish Biol. Fish. 20, 123–136. doi: 10.1007/s11160-009-9129-y
Iglesias, J., Otero, J. J., Moxica, C., Fuentes, L., and Sánchez, F. J. (2004). The completed life cycle of the octopus (Octopus vulgaris, Cuvier) under culture conditions: paralarval rearing using Artemia and zoeae, and first data on juvenile growth up to 8 months of age. Aquac. Int. 12, 481–487. doi: 10.1023/B:AQUI.0000042142.88449.bc
Iglesias, J., Sánchez, F. J., Bersano, J. G. F., Carrasco, J. F., Dhont, J., Fuentes, L., et al. (2007). Rearing of Octopus vulgaris paralarvae: present status, bottlenecks and trends. Aquaculture 266, 1–15. doi: 10.1016/j.aquaculture.2007.02.019
Jereb, P., Roper, C., Norman, M., and Finn, J. (2016). Cephalopods of the World. An Annotated and Illustrated Catalogue of Species Known to Date. Volume 3. Octopods and Vampire Squids. Roma: FAO, Food and Agriculture Organization of the United Nations.
Jiang, X., Lu, H., Shigeno, S., Tan, L. H., Yang, Y., Ragsdale, C. W., et al. (2014). Octopus visual system: a functional MRI model for detecting neuronal electric currents without a blood oxygen level dependent confound. Magn. Reson. Med. 72, 1311–1319. doi: 10.1002/mrm.25051
Kerbl, A., Handschuh, S., Nödl, M. T., Metscher, B., Walzl, M., and Wanninger, A. (2013). Micro-CT in cephalopod research: investigating the internal anatomy of a sepiolid squid using a non-destructive technique with special focus on the ganglionic system. J. Exp. Mar. Biol. Ecol. 447, 140–148. doi: 10.1016/j.jembe.2013.02.022
King, A. J., Henderson, S. M., Schmidt, M. H., Cole, A. G., and Adamo, S. A. (2005). Using ultrasound to understand vascular and mantle contributions to venous return in the cephalopod Sepia officinalis L. J. Exp. Biol. 208, 2071–2082. doi: 10.1242/jeb.01575
Laptikhovsky, V. V. (1998). Differentiation of reproductive strategies within a taxon, as exemplified by octopods. Ruthenica 8, 77–80.
Lee, P. G. (1995). Nutrition of cephalopods: fueling the system. Mar. Freshw. Behav. Physiol. 25, 35–51. doi: 10.1080/10236249409378906
Lee, P. G., Turk, P. E., Forsythe, J. W., and Dimarco, F. P. (1998). Cephalopod culture: physiological, behavioral and environmental requirements. Aquac. Sci. 46, 417–422. doi: 10.11233/aquaculturesci1953.46.417
Maiole, F., Giachero, S., Fossati, S. M., Rocchi, A., and Zullo, L. (2019). mTOR as a marker of exercise and fatigue in Octopus vulgaris arm. Front. Physiol. 10:1161. doi: 10.3389/fphys.2019.01161
Mangold, K. (1987). “Reproduction,” in Cephalopod Life Cycles, Volume II: Comparative Reviews, ed. P. R. Boyle (London: Academic Press), 157–200.
Mangold, K., and Von Boletzky, S. (1973). New data on reproductive biology and growth of Octopus vulgaris. Mar. Biol. 19, 7–12. doi: 10.1007/BF00355414
Margheri, L., Ponte, G., Mazzolai, B., Laschi, C., and Fiorito, G. (2011). Non-invasive study of Octopus vulgaris arm morphology using ultrasound. J. Exp. Biol. 214, 3727–3731. doi: 10.1242/jeb.057323
Mather, J. A. (1991). Foraging, feeding and prey remains in middens of juvenile Octopus vulgaris (Mollusca: Cephalopoda). J. Zool. 224, 27–39. doi: 10.1111/j.1469-7998.1991.tb04786.x
Mäthger, L. M., Bell, G. R., Kuzirian, A. M., Allen, J. J., and Hanlon, R. T. (2012). How does the blue-ringed octopus (Hapalochlaena lunulata) flash its blue rings? J. Exp. Biol. 215, 3752–3757. doi: 10.1242/jeb.076869
Messenger, J. B. (1967). The peduncle lobe: a visuo-motor centre in octopus. Proc. R. Soc. Lond. Ser. B Biol. Sci. 167, 225–251. doi: 10.1098/rspb.1967.0025
Messenger, J. B. (2001). Cephalopod chromatophores: neurobiology and natural history. Biol. Rev. 76, 473–528. doi: 10.1017/S1464793101005772
Miliou, H., Fintikaki, M., Kountouris, T., and Verriopoulos, G. (2005). Combined effects of temperature and body weight on growth and protein utilization of the common octopus, Octopus vulgaris. Aquaculture 249, 245–256. doi: 10.1016/j.aquaculture.2005.03.038
Miranda, R. M., Espinoza, V., Dörner, J., FarÍas, A., and Uriarte, I. (2011). Sibling cannibalism on the small octopus Robsonella fontaniana (d’Orbigny, 1834) paralarvae. Mar. Biol. Res. 7, 746–756. doi: 10.1080/17451000.2011.596543
Naud, M. J., Sauer, W. H., McKeown, N. J., and Shaw, P. W. (2016). Multiple mating, paternity and complex fertilisation patterns in the chokka squid Loligo reynaudii. PLoS One 11:e0146995. doi: 10.1371/journal.pone.0146995.t001
Naud, M. J., Shaw, P. W., Hanlon, R. T., and Havenhand, J. N. (2005). Evidence for biased use of sperm sources in wild female giant cuttlefish (Sepia apama). Proc. R. Soc. B Biol. Sci. 272, 1047–1051. doi: 10.1098/rspb.2004.3031
Navarro, J. C., and Monroig, Ó, and Sykes, A. V. (2014). “Nutrition as a key factor for cephalopod aquaculture,” in Cephalopod Culture, eds J. Iglesias, L. Fuentes, and R. Villanueva (Dordrecht: Springer), 77–95. doi: 10.1007/978-94-017-8648-5_5
O’Dor, R. K., and Wells, M. J. (1987). “Energy and nutrient flow,” in Cephalopod Life Cycles, Volume II: Comparative Reviews, ed. P. R. Boyle (London: Academic Press), 109–133.
Oestmann, D. J., Scimeca, J. M., Forsythe, J., Hanlon, R., and Lee, P. (1997). Special considerations for keeping cephalopods in laboratory facilities. J. Am. Assoc. Lab. Anim. Sci. 36, 89–93.
Pham, C. K., and Isidro, E. (2009). Growth and mortality of common octopus (Octopus vulgaris) fed a monospecific fish diet. J. Shellfish Res. 28, 617–623. doi: 10.2983/035.028.0326
Prato, E., Portacci, G., and Biandolino, F. (2010). Effect of diet on growth performance, feed efficiency and nutritional composition of Octopus vulgaris. Aquaculture 309, 203–211. doi: 10.1016/j.aquaculture.2010.09.036
Rocha, F., and Guerra, Á, and González, ÁF. (2001). A review of reproductive strategies in cephalopods. Biol. Rev. 76, 291–304. doi: 10.1017/S1464793101005681
Rodaniche, A. F. (1984). Iteroparity in the lesser Pacific striped octopus Octopus chierchiae (Jatta, 1889). Bull. Mar. Sci. 35, 99–104.
Rodhouse, P. G. (1998). Physiological progenesis in cephalopod molluscs. Biol. Bull. 195, 17–20. doi: 10.2307/1542771
Rosas, C., Cuzon, G., Pascual, C., Gaxiola, G., Chay, D., López, N., et al. (2007). Energy balance of Octopus maya fed crab or an artificial diet. Mar. Biol. 152, 371–381. doi: 10.1007/s00227-007-0692-2
Rosas, C., Sánchez, A., Pascual, C., Aguila, J., Maldonado, T., and Domingues, P. (2011). Effects of two dietary protein levels on energy balance and digestive capacity of Octopus maya. Aquac. Int. 19, 165–180. doi: 10.1007/s10499-010-9350-7
Rosas, C., Valero, A., Caamal-Monsreal, C., Uriarte, I., Farias, A., Gallardo, P., et al. (2013). Effects of dietary protein sources on growth, survival and digestive capacity of Octopus maya juveniles (Mollusca: Cephalopoda). Aquac. Res. 44, 1029–1044. doi: 10.1111/j.1365-2109.2012.03107.x
Shigeno, S., and Ragsdale, C. W. (2015). The gyri of the octopus vertical lobe have distinct neurochemical identities. J. Comp. Neurol. 523, 1297–1317. doi: 10.1002/cne.23755
Sinn, D. L., Perrin, N. A., Mather, J. A., and Anderson, R. C. (2001). Early temperamental traits in an octopus (Octopus bimaculoides). J. Comp. Psychol. 115:351. doi: 10.1037/0735-7036.115.4.351
Smith, J. A., Andrews, P. L. R., Hawkins, P., Louhimies, S., Ponte, G., and Dickel, L. (2013). Cephalopod research and EU Directive 2010/63/EU: requirements, impacts and ethical review. J. Exp. Mar. Biol. Ecol. 447, 31–45. doi: 10.1016/j.jembe.2013.02.009
Squires, Z. E., Wong, B. B., Norman, M. D., and Stuart-Fox, D. (2015). Last male sperm precedence in a polygamous squid. Biol. J. Linn. Soc. 116, 277–287. doi: 10.1111/bij.12590
Stubbs, A. L., and Stubbs, C. W. (2016). Spectral discrimination in color blind animals via chromatic aberration and pupil shape. Proc. Natl. Acad. Sci. U.S.A. 113, 8206–8211. doi: 10.1073/pnas.1524578113
Sumbre, G., Fiorito, G., Flash, T., and Hochner, B. (2005). Motor control of flexible octopus arms. Nature 433, 595–596. doi: 10.1038/433595a
Sykes, A. V., Baptista, F. D., Gonçalves, R. A., and Andrade, J. P. (2012). Directive 2010/63/EU on animal welfare: a review on the existing scientific knowledge and implications in cephalopod aquaculture research. Rev. Aquac. 4, 142–162. doi: 10.1111/j.1753-5131.2012.01070
Tramacere, F., Beccai, L., Mattioli, F., Sinibaldi, E., and Mazzolai, B. (2012). “Artificial adhesion mechanisms inspired by octopus suckers,” in Proceedings of the 2012 IEEE International Conference on Robotics and Automation, (Piscataway, NJ: IEEE), 3846–3851. doi: 10.1109/ICRA.2012.6225058
Tramacere, F., Kovalev, A., Kleinteich, T., Gorb, S. N., and Mazzolai, B. (2014). Structure and mechanical properties of Octopus vulgaris suckers. J. R. Soc. Interf. 11:20130816. doi: 10.1098/rsif.2013.0816
van Giesen, L., Kilian, P. B., Allard, C. A., and Bellono, N. W. (2020). Molecular basis of chemotactile sensation in octopus. Cell 183, 594–604. doi: 10.1016/j.cell.2020.09.008
Van Heukelem, W. F. (1977). Laboratory maintenance, breeding, rearing, and biomedical research potential of the Yucatan octopus (Octopus maya). Lab. Anim. Sci. 27(5 Pt 2), 852–859.
Vidal, E. A., Villanueva, R., Andrade, J. P., Gleadall, I. G., Iglesias, J., Koueta, N., et al. (2014). Cephalopod culture: current status of main biological models and research priorities. Adv. Mar. Biol. 67, 1–98. doi: 10.1016/B978-0-12-800287-2.00001-9
Vidal, E. A., and von Boletzky, S. (2014). “Loligo vulgaris and Doryteuthis opalescens,” in Cephalopod Culture, eds J. Iglesias, L. Fuentes, and R. Villanueva (Dordrecht: Springer), 271–313. doi: 10.1007/978-94-017-8648-5_16
Villanueva, R., Sykes, A. V., Vidal, E. A., Rosas, C., Nabhitabhata, J., Fuentes, L., et al. (2014). “Current status and future challenges in cephalopod culture,” in Cephalopod Culture, eds J. Iglesias, L. Fuentes, and R. Villanueva (Dordrecht: Springer), 479–489. doi: 10.1007/978-94-017-8648-5_26
Voss, G. L. (1968). Biological investigations of the deep sea. 39. Octopods from the R/V Pillsbury Southwestern Caribbean Cruise, 1966, with a description of a new species, Octopus zonatus. Bull. Mar. Sci. 18, 645–659.
Voss, G. L. (1971). Biological results of the university of miami deep-sea expeditions. 76. Cephalopods collected by the R/V John Elliott Pillsbury in the Gulf of Panama in 1967. Bull. Mar. Sci. 21, 1–34.
Wada, T., Takegaki, T., Mori, T., and Natsukari, Y. (2005). Sperm displacement behavior of the cuttlefish Sepia esculenta (Cephalopoda: Sepiidae). J. Ethol. 23, 85–92. doi: 10.1007/s10164-005-0146-6
Wada, T., Takegaki, T., Mori, T., and Natsukari, Y. (2010). Sperm removal, ejaculation and their behavioural interaction in male cuttlefish in response to female mating history. Anim. Behav. 79, 613–619. doi: 10.1016/j.anbehav.2009.12.004
Walker, J. J., Longo, N., and Bitterman, M. E. (1970). The octopus in the laboratory. Handling, maintenance, training. Behav. Res. Methods Instrum. 2, 15–18. doi: 10.3758/BF03205718
Walsh, L. S., Turk, P. E., Forsythe, J. W., and Lee, P. G. (2002). Mariculture of the loliginid squid Sepioteuthis lessoniana through seven successive generations. Aquaculture 212, 245–262. doi: 10.1016/S0044-8486(02)00126-6
Wang, Z. Y., and Ragsdale, C. W. (2018). Multiple optic gland signaling pathways implicated in octopus maternal behaviors and death. J. Exp. Biol. 221:jeb185751. doi: 10.1242/jeb.185751
Wang, Z., and Ragsdale, C. (2019). “Cephalopod nervous system organization,” in Oxford Research Encyclopedia of Neuroscience. Available online at: https://oxfordre.com/neuroscience/view/10.1093/acrefore/9780190264086.001.0001/acrefore-9780190264086-e-181 (accessed November 12, 2021).
Wells, M. J., and Wells, J. (1969). Pituitary analogue in the octopus. Nature 222, 293–294. doi: 10.1038/222293a0
Wigby, S., and Chapman, T. (2004). Sperm competition. Curr. Biol. 14, R100–R103. doi: 10.1016/j.cub.2004.01.013
Wild, E., Wollesen, T., Haszprunar, G., and Heß, M. (2015). Comparative 3D microanatomy and histology of the eyes and central nervous systems in coleoid cephalopod hatchlings. Org. Divers. Evol. 15, 37–64. doi: 10.1007/s13127-014-0184-4
Wodinsky, J. (1977). Hormonal inhibition of feeding and death in octopus: control by optic gland secretion. Science 198, 948–951. doi: 10.1126/science.198.4320.948
Wood, J. B., and Anderson, R. C. (2004). Interspecific evaluation of octopus escape behavior. J. Appl. Anim. Welf. Sci. 7, 95–106. doi: 10.1207/s15327604jaws0702_2
Yamazaki, A., Yoshida, M., and Uematsu, K. (2002). Post-hatching development of the brain in Octopus ocellatus. Zool. Sci. 19, 763–771. doi: 10.2108/zsj.19.763
Keywords: iteroparity, cephalopod, model organism, aquaculture, reproduction – mollusk, developmental biology, neurobiology, animal behavior
Citation: Grearson AG, Dugan A, Sakmar T, Sivitilli DM, Gire DH, Caldwell RL, Niell CM, Dölen G, Wang ZY and Grasse B (2021) The Lesser Pacific Striped Octopus, Octopus chierchiae: An Emerging Laboratory Model. Front. Mar. Sci. 8:753483. doi: 10.3389/fmars.2021.753483
Received: 04 August 2021; Accepted: 05 November 2021;
Published: 13 December 2021.
Edited by:
Thanos Dailianis, Institute of Marine Biology, Biotechnology & Aquaculture, Hellenic Centre for Marine Research, GreeceReviewed by:
Giovanna Ponte, Department of Biology and Evolution of Marine Organisms, Zoological Station Anton Dohrn, ItalyCarlos Rosas, National Autonomous University of Mexico, Mexico
Copyright © 2021 Grearson, Dugan, Sakmar, Sivitilli, Gire, Caldwell, Niell, Dölen, Wang and Grasse. This is an open-access article distributed under the terms of the Creative Commons Attribution License (CC BY). The use, distribution or reproduction in other forums is permitted, provided the original author(s) and the copyright owner(s) are credited and that the original publication in this journal is cited, in accordance with accepted academic practice. No use, distribution or reproduction is permitted which does not comply with these terms.
*Correspondence: Anik G. Grearson, YWdyZWFyc29uOTdAZ21haWwuY29t; Z. Yan Wang, enl3YW5nbGFiQGdtYWlsLmNvbQ==; Bret Grasse, YnJldC5ncmFzc2VAZ21haWwuY29t
†These authors share senior authorship