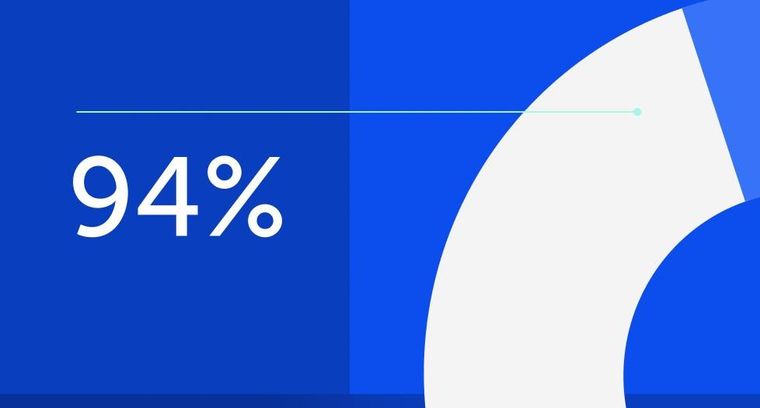
94% of researchers rate our articles as excellent or good
Learn more about the work of our research integrity team to safeguard the quality of each article we publish.
Find out more
ORIGINAL RESEARCH article
Front. Mar. Sci., 01 December 2021
Sec. Coral Reef Research
Volume 8 - 2021 | https://doi.org/10.3389/fmars.2021.750210
This article is part of the Research TopicCoral Reef Restoration in a Changing World: Science-based SolutionsView all 20 articles
Loss of foundation reef-corals is eroding the viability of reef communities and ecosystem function in many regions globally. Coral populations are naturally resilient but when breeding corals decline, larval supply becomes limiting and natural recruitment is insufficient for maintaining or restoring depleted populations. Passive management approaches are important but in some regions they are proving inadequate for protecting reefs, therefore active additional intervention and effective coral restoration techniques are needed. Coral spawning events produce trillions of embryos that can be used for mass larval rearing and settlement on degraded but recoverable reef areas. We supplied 4.6 million Acropora tenuis larvae contained in fine mesh enclosures in situ on three degraded reef plots in the northwestern Philippines during a five day settlement period to initiate restoration. Initial mean larval settlement was very high (210.2 ± 86.4 spat per tile) on natural coral skeleton settlement tiles in the larval-enhanced plots, whereas no larvae settled on tiles in control plots. High mortality occurred during early post-settlement life stages as expected, however, juvenile coral survivorship stabilised once colonies had grown into visible-sized recruits on the reef by 10 months. Most recruits survived and grew rapidly, resulting in significantly increased rates of coral recruitment and density in larval-enhanced plots. After two years growth, mean colony size reached 11.1 ± 0.61 cm mean diameter, and colonies larger than 13 cm mean diameter were gravid and spawned, the fastest growth to reproductive size recorded for broadcast spawning corals. After three years, mean colony size reached 17 ± 1.7 cm mean diameter, with a mean density of 5.7 ± 1.25 colonies per m–2, and most colonies were sexually reproductive. Coral cover increased significantly in larval plots compared with control plots, primarily from A. tenuis recruitment and growth. Total production cost for each of the 220 colonies within the restored breeding population after three years was United States $17.80 per colony. A small but significant increase in fish abundance occurred in larval plots in 2018, with higher abundance of pomacentrids and corallivore chaetodontids coinciding with growth of A. tenuis colonies. In addition, innovative techniques for capturing coral spawn slicks and larval culture in pools in situ were successfully developed that can be scaled-up for mass production of larvae on reefs in future. These results confirm that enhancing larval supply significantly increases settlement and coral recruitment on reefs, enabling rapid re-establishment of breeding coral populations and enhancing fish abundance, even on degraded reef areas.
Scleractinian hermatypic corals are foundation species on coral reefs (Bruno and Selig, 2007; Harrison and Booth, 2007), and function as ecosystem engineers with essential roles in calcification and reef accretion, creating crucial three-dimensional habitats for many other reef organisms (Birkeland, 1997; Burke et al., 2011; Hoegh-Guldberg et al., 2017). Healthy coral populations consist of highly fecund colonies that produce large numbers of gametes for broadcast spawning and planktonic larval development, or cyclic production of brooded planulae (Harrison, 2011; Randall et al., 2020). As with other marine invertebrates, the efficiency with which coral larval production results in successful settlement, survival, recruitment and growth into adult breeding colonies is unknown (Harrison and Wallace, 1990). Marine invertebrates have high intrinsic mortality, with losses from predation during their planktonic phase estimated to be up to 90–100% daily (Thorson, 1950; Rumrill, 1990; Pechenik, 1999), and pelagic larval dispersal results in many coral larvae being carried away from reefs in currents (Harrison and Wallace, 1990; Jones et al., 2009). Therefore, less than 0.1–0.001% of progeny may settle and survive to adult size on coral reefs. However, the low rate of larval settlement and recruitment is offset by production of vast quantities of coral spawn and billions or trillions of larvae, resulting in sufficient recruitment to maintain coral populations and enable recovery from most natural disturbances over decadal timescales (Connell et al., 1997; Gilmour et al., 2013; Gouezo et al., 2019).
Coral communities in many reef regions have been decimated by increasing anthropogenic disturbances including overfishing and destructive blast fishing (McManus et al., 1997; Wilkinson, 2008), coastal development, pollution and increasing predator and disease outbreaks (Bruno and Selig, 2007; Burke et al., 2011), which are exacerbated by climate change induced increased severity and frequency of marine heatwaves and mass coral bleaching (Hoegh-Guldberg et al., 2017; Hughes et al., 2018) and extreme storm events (De’ath et al., 2012; Jackson et al., 2014). The loss of large numbers of breeding corals significantly reduces gamete production, fertilisation rates and larval production (Harrison and Wallace, 1990; Randall et al., 2020), thereby impairing or preventing sufficient larval recruitment for natural recovery of coral populations and communities (Hughes et al., 2019). Reduced larval supply limits recruitment and accelerates decline in coral communities, creating opportunities for other reef invertebrates and algae to colonise, and can contribute to phase shifts from coral to algal dominated reef systems (Done, 1992; Bruno et al., 2009).
Passive reef management approaches are proving to be ineffective in enabling coral and reef communities to recover from chronic anthropogenic and natural disturbances in many coral reef regions around the world (Burke et al., 2011). Therefore, increasing attention and research has focussed on active coral restoration. Coral restoration can use either asexual fragmentation and cloning methods, or sexual production of corals to promote recovery, but current scales of restoration are limited (reviewed by Rinkevich, 1995; Edwards, 2010; Omori, 2019; Boström-Einarsson et al., 2020).
Asexual fragmentation and nursery outplanting methods can quickly increase clonal corals on high-value reef patches, but are inherently limited by high production costs and ongoing maintenance costs for managing nurseries (Edwards, 2010; Omori, 2019). Most early coral restoration projects relied on asexual coral fragmentation and transplantation to attempt to re-establish corals on degraded reef areas (Rinkevich, 1995; Edwards, 2010). Although conceptually simple, coral fragmentation and outplanting is often limited in scale, can be costly, and clonal fragments have limited genotypic diversity that reduces adaptive capacity and resilience of transplanted populations (Baums, 2008; Boström-Einarsson et al., 2020). More recent advances include the use of nurseries for enabling recovery of coral fragments before outplanting (“coral gardening”), which can lead to higher rates of survival and growth, but requires higher maintenance that increases production costs (Lirman and Schopmeyer, 2016; Rinkevich, 2019; Boström-Einarsson et al., 2020).
Sexual reproduction and mass culture of larvae enables enhanced genetic diversity and evolutionary potential among offspring derived from more tolerant surviving adult broodstock corals, and is potentially scalable to larger reef areas. However, broadcast spawning corals and other marine invertebrates with similar life histories have inherently high rates of mortality post-settlement due to strong environmental selective pressures operating on these invisible early stages of coral reproduction (Harrison and Wallace, 1990; Wilson and Harrison, 2005; Doropoulos et al., 2016; Randall et al., 2020).
Various forms of sexual propagation have been developed to overcome the inherent genetic limitations of asexual propagation for restoration programmes. These include settlement of cultured coral larvae on artificial substrata, and nursery rearing before transplantation and deployment on reefs (Omori, 2005; Petersen et al., 2005; Baria et al., 2012; Guest et al., 2014; Chamberland et al., 2017). Nursery rearing can sometimes increase survival rates of outplanted juvenile corals but also increases the production costs (Guest et al., 2014). Larval settlement in laboratory environments may also create artificial selection pressures and fitness consequences that result in ex situ settled juveniles being maladapted to environmental conditions on reefs when transplanted, leading to suboptimal survival and growth rates.
An alternative approach is larval enhancement whereby larvae are settled directly onto reef areas or artificial surfaces in situ, which increases the chances of successful settlement in preferred microhabitats and improved genotype-environment matching (Harrison et al., 2016; Harrison, 2021). However, relatively few studies have used this approach, and the initial studies were done on healthy reefs with naturally high larval supply and recruitment (Heyward et al., 2002; Suzuki et al., 2012; Edwards et al., 2015). Additionally, monitoring of settlers’ survival, growth and recruitment was performed for periods of six weeks (Heyward et al., 2002), six months (Suzuki et al., 2012) and approximately 13 months (Edwards et al., 2015). Therefore, these studies were unable to determine the longer-term restoration outcomes of the approach.
In 2013, dela Cruz and Harrison (2017) supplied ∼400,000 Acropora tenuis larvae into replicate 24 m2 reef plots on degraded reef areas in the northwestern Philippines and recorded high rates of initial larval settlement on natural settlement tiles in plots supplied with larvae and no settlement in control plots. Survival and growth of settled spat and recruits were monitored for three years, with significantly higher recruitment on larval enhancement plots compared with control plots. Larval enhancement resulted in an average of 2.3 colonies m–2 surviving on available reef substrata after three years, and re-established a breeding population on this degraded algal phase-shifted reef (dela Cruz and Harrison, 2017).
The present study builds on the successful pilot study of dela Cruz and Harrison (2017) and aimed to (1) quantify the effects of increased A. tenuis larval supply on initial larval settlement and recruitment rates on degraded reef substrata, and monitoring survival and growth of colonies in the restored population through to reproductive size, (2) assess longer-term restoration outcomes of larval enhancement on changes in coral and other benthic communities and fish assemblages over three years, and (3) develop new techniques for capturing larger volumes of coral spawn directly on the reef, for future larger-scale restoration.
Degraded reef areas at Magsaysay reef (16°31′36″ N, 120°02′01″ E) in the Magsaysay Marine Protected Area (MPA) in Northern Luzon, Philippines were used for this study. Magsaysay reef is part of the Bolinao-Anda Reef Complex (BARC) in the Lingayen Gulf located in the municipality of Anda, Pangasinan (Figure 1). This experiment sought to quantify the effects of supplying high densities of coral larvae onto replicate degraded reef plots during their settlement period, then monitoring initial larval settlement rates, subsequent survival and growth of recruits and adult corals over three years. Repeated monitoring compared the effects of larval restoration on benthic and fish assemblages, particularly fish abundance, species richness and trophic groups, between larval enhancement and control plots where no cultured larvae were supplied.
Figure 1. Location of coral restoration site at Magsaysay reef and Bolinao Marine Laboratory in Northern Luzon, Philippines.
Prior to the experiment in April–May 2016, six replicate 5 × 5 m plots were haphazardly selected on degraded reef areas at 3–4 m depth on Magsaysay reef. Plots were located at least 10 m apart, with three plots haphazardly assigned as larval enhancement plots and three plots as controls that did not receive larvae.
Newly settled coral polyps (spat) are microscopic and cryptic, hence are very difficult to detect on complex natural reef substrata (Harrison and Wallace, 1990). Therefore, initial rates of larval settlement were quantified using biologically conditioned 10 × 10 cm by 3–4 cm thick settlement tiles cut from dead tabulate Acropora skeletons collected from the intertidal zone beside Cory reef, near Magsaysay reef (Figure 1). Each tile was identified with a coded metal tag and biologically conditioned for four weeks in aerated flow-through seawater tanks at the Bolinao Marine Laboratory (BML) of The Marine Science Institute, University of the Philippines, prior to use on the reef plots.
Just prior to the experiment, tiles were examined to confirm that no coral recruits were present. Then ten tiles were deployed haphazardly in each of the three larval enhancement plots and in each of the three control plots, with each tile located on a separate small stainless steel post on a baseplate attached into the reef (after Mundy, 2000). A small 0.5–1 cm gap was left between each tile and its baseplate to allow larvae to settle on all tile surfaces. In addition, a total of 12 open reef control settlement tiles were also haphazardly deployed on posts near the corners of control plots (one open reef control tile at each corner of each of the three control plots), to monitor natural coral larval settlement patterns during the larval settlement period when the experimental plots were covered with fine mesh enclosures. The surface areas of the natural coral skeleton tiles were estimated to be about 360 ± 3.7 cm2 (SE) by 3D scanning thirty representative tiles (dela Cruz and Harrison, 2017). Minimal variation in surface areas among tiles allowed settlement rates to be standardised between tiles.
Four days prior to the full moon on 22 April 2016, colonies of Acropora tenuis were examined in situ on Magsaysay reef and Caniogan reef (16°30′26.8″ N, 120°0′47.7″ E; Figure 1) by carefully breaking a few branches to determine if colonies contained pink to red coloured mature eggs, indicating imminent spawning (Harrison et al., 1984). Twenty-three gravid colonies from Magsaysay reef and six gravid colonies from Caniogan reef were collected from ∼2–4 m depths and transferred to the BML aquaculture facility and maintained in an apparently healthy condition in large tanks with flow-through seawater and aeration. Coral colonies were monitored from ∼6 pm each evening during the crepuscular period to check for setting and spawning behaviours, and spawning of all 29 colonies occurred between 1830 and 1900 h on 23 April 2016 (1 night after full moon, nAFM, Table 1) in the BML tanks.
In addition, gravid three-year old A. tenuis F1 colonies grown from larvae that settled in larval restoration plots at Magsaysay reef in 2013 (dela Cruz and Harrison, 2017), were monitored during night dives to enable collection of spawned gametes from their first gametogenic cycle. At ∼1730 h each evening, spawn collection cones were carefully placed over gravid colonies until spawning occurred, then removed at ∼1930 h if spawning did not occur. The 40 cm diameter spawn collection cones were made from fine 180 μm organza cloth attached to a weighted metal ring, with the upper section connected to an inverted 800 mL clear plastic collection jar partially filled with air to keep the conical net floating upright. The first spawning of these three-year old restoration colonies occurred between 1830 and 1900 h on 24 April 2016 (2 nAFM, Table 1), and egg-sperm bundles were collected from 31 colonies. The plastic jars containing gametes were transported to the BML aquaculture facility and mixed together immediately in a 50 L container.
Millions of gametes from the spawned egg-sperm bundles were collected from the 29 collected colonies (ex situ) and the 31 three-year old F1 colonies (in situ), and each cohort was cultured separately in the BML aquaculture facility using standard methods as follows. Gamete bundles from all colonies that spawned together were collected and transferred into a fertilisation tank containing 20 L of 1 μm filtered seawater and gently mixed to facilitate cross-fertilisation (Harrison, 2006; dela Cruz and Harrison, 2017). After a fertilisation period of 1–1.5 h to optimise cross-fertilisation (dela Cruz and Harrison, 2020a), excess sperm was removed from the tank by siphoning water from beneath the floating eggs and embryos to prevent polyspermy and degraded water quality (Willis et al., 1997). The seawater with sperm was slowly replaced with new 1 μm filtered seawater, and the sperm washing process was repeated three times. After an hour, subsamples of eggs and embryos were removed and examined under a stereomicroscope to quantify percentage fertilisation.
Developing embryos were skimmed off the water surface and transferred to large rearing tanks (> 1,000 L and 500 L) at densities of 4–5 embryos cm–2 and gently agitated. After 24 h development when embryos had formed into more robust spheroidal planula larvae (Harrison and Wallace, 1990), gentle aeration was supplied and > 100 L of seawater was syphoned from the bottom of each tank and replaced with 1 μm filtered seawater each day to maintain water quality and healthy larval cultures. Larvae were cultured until they became competent to settle and were used for settlement trials.
On 29 April when F2 larval cohorts were 4.5 days old (from F1 corals that spawned in situ on the reef) and larvae from colonies that spawned ex situ at BML were 5.5 days old, samples of larvae were carefully filtered from culture tanks using fine plankton mesh sieves and counted under stereomicroscopes illuminated with fibre-optic and LED lights into five subsamples of 100 larvae from each cohort. Larvae were transferred in plastic jars to Magsaysay reef where each sample was placed inside a small plastic settlement cage with plankton mesh sides (after Ward and Harrison, 1997), with each settlement cage containing two biologically conditioned settlement tiles. These initial settlement trials showed that 12.4% ± 1.8 of the wild larvae and 18.4% ± 4.8 of the F2 cohort larvae settled within 5 days, confirming that larvae were healthy and competent to settle.
On 1 May, after seven days culture for the F2 cohort and eight days culture for the ex situ spawning cohort, competent swimming larvae were concentrated using fine plankton mesh sieves and combined into separate 160 L holding tanks for each cohort. Larvae were thoroughly mixed, then three 60 mL subsamples were taken from each tank and counted under stereomicroscopes to estimate total larval abundance in each cohort. A total of 4.6 million larvae were available from the cultures for the reef settlement experiment. These larvae were thoroughly mixed again to homogenise their distribution and then evenly distributed into fifteen 20 L plastic bags supplied with oxygen and sealed for transport to Magsaysay reef for the larval enhancement experiment (after dela Cruz and Harrison, 2017).
To retain larvae on the three larval enhancement plots during the settlement period, each treatment plot was enclosed in a purpose-designed 5 × 5 m by 60 cm deep square tent-like enclosure constructed from 180 μm plankton mesh net (Figure 2A). The net was reinforced with webbing sewn along the seams, and 50 × 50 cm larval supply portals made from vinyl with velcro sealing were located in each quadrant on the upper surface (Figure 2B). A PVC pipe frame around the perimeter and across the centre of the plot supported the integrity of the net shape. The net was secured onto the frame with webbing ties sewn at 1 m intervals along the perimeter, at each corner and in the centre. Steel reinforcing bars were driven into the reef at each corner and at intervals along the sides. Net sides were bordered with a 20 cm vinyl collar with a 10 mm rope sealed along the edge for increased strength. Regularly spaced 10 mm holes in the vinyl above the sealed rope allowed metal pegs to secure the base of the net to the seafloor. Additional concrete bricks and dead coral skeletons were placed along the vinyl collar to help seal the base of the net onto the reef. A small buoy was attached to the centre of the net to keep the upper surface of the net slightly above the reef to reduce abrasion of the net on corals and reef substrata (Figure 2A). The bottom of each mesh tent enclosure was therefore open to the reef benthic environment within each plot to enable larvae to settle on suitable dead coral substrata.
Figure 2. Larval mesh tent enclosure with frame attached onto reef (A), and divers adding concentrated A. tenuis larvae into a re-sealable portal on the mesh enclosure for settlement (B).
An estimated 1.54 million A. tenuis larvae were added into each of the three 5 × 5 m larval mesh nets on the larval enhancement plots by divers sequentially opening each of the larval portals and transferring the larvae in five plastic bags into the net (Figure 2B). The portal was then partly closed and a diver wafted the larvae further into the enclosure using a dive fin to increase the spread of larvae across the plot, then the portal was firmly resealed to prevent larvae drifting out of the net. Control plots were also covered with nets during the settlement period but no larvae were added.
On 5 May 2016 after four days larval settlement, strong winds and wave action were impacting the Magsaysay reef sites and the base of the larval mesh net in larval plot 3 had partly detached, so this net was removed to avoid damage to corals and the reef. On 6 May, the larval mesh enclosures were removed from the remaining two larval plots and the three control plots. The 60 settlement tiles from the six experimental plots (ten tiles in each of the three larval enhancement plots and in each of the three control plots) and the additional 12 settlement tiles deployed around the outside of control plots as open reef controls were carefully collected and transferred in seawater to a nearby temporary field station on Tanduyong Island (Figure 1). Tile surfaces were viewed while submerged in small trays under stereomicroscopes illuminated with fibre-optic and LED lights, and numbers of settled coral spat and their locations on tiles were recorded. After monitoring, tiles were returned to their specific locations on the reef plots and reattached onto the tile posts with the correct orientation, to enable repeated monitoring of growth and survival of spat on the tiles.
Initial survival on tiles was monitored after 1 and 2 months post-settlement by collecting the tiles and counting the surviving spat on each tile surface under stereomicroscopes at Tanduyong Island. Tiles were subsequently returned to the reef and reattached to their numbered tile posts. After 10 months, recruits on tiles had grown large enough to census underwater, so survival and growth were monitored in situ at 10, 12, 14, 17, 20, 25, 27, 29, 32, and 34 months post-settlement. The A. tenuis recruits that had settled on the reef surfaces within each of the larval enhancement plots were also visible underwater by 10 months, and their characteristic morphology and subsequent growth into recognisable A. tenuis colonies were used to identify them as originating from larvae that settled on the reef during the larval enhancement experiment. Each recruit and juvenile colony on the reef were mapped and a small, numbered metal tag was permanently attached nearby to facilitate monitoring at the same time as recruits on tiles were monitored.
Colony size was measured using vernier callipers to measure the length (l), width (w) and height (h) of each A. tenuis coral on the recruitment tiles and on the natural reef substrata. The mean planar diameter was calculated from the maximum and minimum diameters measured for each colony. The approximate volume was also measured following a spherical formula EV = πr2h, where r = (l + w)/4 (Shaish et al., 2010). In some cases, larvae settled in close proximity to others and colonies grew together, fusing into a larger chimeric colony. Individual colonies that fused together were still counted and measured separately when polyp demarcation or separation lines were still visible. Where individual corals were indistinct in a fused coral colony, the previous individual count was recorded for survivorship, but measured as a single fused colony for growth.
Evidence of sexual reproduction in all surviving recruits was assessed at 23 and 34 months after settlement, just prior to potential spawning periods after full moons when corals were 2 and 3 years old. Coral reproductive status was examined by carefully breaking up to three small branches to observe whether developing and pigmented oocytes and spermaries were present in broken sections of polyps (after Harrison et al., 1984). Branches were then gently wedged back into the colony to avoid loss of tissues and spawning biomass.
The costs of producing coral recruits including all materials, vessel hire and fuel, diving, labour, larval rearing, and capital costs for larval mesh tents were estimated following Edwards (2010). The average costs per colony were estimated by dividing the total costs by the total numbers of recruits alive at 10 months and at 3 years in the larval plots. Costs were initially calculated in Philippine Peso (PhP) and Australian Dollar (AUD), and converted to United States Dollar (USD) values.
Prior to the early 1980s, BARC reefs were characterised by relatively high 30–50% mean live coral cover and healthy reef status (Gomez et al., 1981). However, extensive blast fishing, aquaculture development and crown-of-thorns starfish outbreaks severely impacted coral and other reef communities in the Lingayen Gulf, resulting in degradation of these reefs during subsequent decades (Cruz-Trinidad et al., 2009; dela Cruz and Harrison, 2017). Blast fishing has since been banned and has now effectively ceased in local communities from Anda and nearby towns, hence these reefs are now potentially recoverable but are limited by low rates of natural coral recruitment (dela Cruz and Harrison, 2017).
To quantify benthic cover of corals, other benthos and the reef community status prior to larval enhancement, digital images of the experimental plots were taken in April 2016 using a Canon G1-X underwater camera and a 1 × 1 m quadrat frame, and images were analysed using CoralNet (Beijbom et al., 2015). Twenty-five random sampling points were generated for each image and the benthic category underlying each point was identified, resulting in 625 points per plot. Only one adult A. tenuis colony was present inside the reef plots prior to the experiment, growing in control plot 2. Some excess macroalgae within the plots was carefully removed by divers to reduce potential physical and allelopathic effects of algal biomass on larval settlement (Ceccarelli et al., 2018). Monitoring of the reef benthic community status was repeated in March 2019 to quantify changes over time in the experimental plots.
Modified Stationary Point-Counts (Bohnsack and Bannerot, 1986) were used to survey the reef fish assemblages in all plots. Ten-minute surveys were completed in each plot, and all non-cryptic fishes inside the plots were identified to species and recorded (Allen et al., 2005), and the total length of individual fishes was visually estimated (in cm). Monitoring of the reef fish assemblages in all plots was repeated in March 2017, 2018, and 2019 to quantify changes.
A further objective of this study was to develop new techniques for capturing large volumes of floating coral spawn slicks and culturing embryos and larvae in floating mesh net enclosures at sea for future similar experiments. Millions of spawned egg-sperm bundles were collected in situ on Magsaysay reef from large multispecific spawning events on 31 March 2016 (8 nAFM) and 1 April 2016 (9nAFM) and placed in a mesh rearing pool suspended from a 5 × 5 m floating bamboo frame. The rearing pool was 5 × 5 m square by 3.5 m deep with the upper part of the net system comprising a vinyl sheet extending from 0.5 m above the sea surface to 0.5 m below the surface (Figure 3A). All net systems for larval rearing or spawn slick collection (described below) feature vinyl sheeting above and below the waterline, as this smooth surface avoids abrasion of the delicate developing embryos on the plankton mesh. The lower portion of the larval rearing pool net comprised 180 μm plankton mesh net that extended down to the reef substratum where it was temporarily attached using small steel bars and pegs (Figure 3B). The net was located over a small patch of healthy reef dominated by large gravid Acropora hyacinthus and A. cytherea colonies, so that spawned egg-sperm bundles would float up and be retained within the net. Additional coral spawn was collected using coral spawn collection cones placed over other spawning Acropora spp. colonies observed on night dives, and by swimming spawn cones along the sea surface as neuston nets to collect samples of large spawn slicks that were then added into the larval rearing pool. Samples of developing embryos were taken 12 and 20 h after spawning to quantify development stages and health of the cultures.
Figure 3. A prototype 5 × 5 m floating bamboo frame larval rearing pool deployed on Magsaysay reef in 2016 (A), and the submerged mesh curtain attached to the bamboo frame on reef site (B). Spawn catcher with booms attached to bamboo frame in 2017 on Magsaysay reef (C), and 2018 larval rearing net deployed under a floating steel frame (D).
The spawn slick capture and larval culture pools concept was further developed by designing an innovative floating “spawn slick capture” net with two 15 m long booms that extended each side of a partly submerged net to funnel buoyant coral spawn slicks into the collector. A 2 × 2 m PVC pipe prototype semi-submersible frame was built in April 2016 and the design was further refined in 2017 during field trials on the reef. In March 2017, a 150 μm plankton mesh net with an upper vinyl panel extending 0.5 m above and below the sea surface, and paired 15 m inflatable spawn collector booms were attached to a 5 × 5 m floating bamboo frame which was positioned into the wind and down current from spawning corals on Magsaysay reef (Figure 3C). The booms were held apart at ∼90° using anchor ropes, and had a 30 cm weighted vinyl curtain submerged below the water surface to facilitate spawn slick collection (Figure 3C). Subsequently, the submerged 150 μm larval culture mesh nets were redesigned to be free-floating within a frame, and with tapered sides and a zipper opening at the base. These redesigned nets were attached to stronger 5 × 5 m floating steel frames to increase the success of spawn collection and larval culture on the reef in adverse weather conditions (Figure 3D). Large multispecific Acropora spp. spawning events occurred at Magsaysay reef on 20 and 21 March 2017 (8–9nAFM) and on the 12 and 13 March 2018 (10–11nAFM, Table 1), and spawned egg-sperm bundles were collected in the spawn catchers, and larvae were reared in the larval culture nets attached to the floating frames. In 2018, a solar powered seawater pump and aeration system was attached to the floating steel frames to maintain good water quality and increase the efficiency of larval cultures on the reef.
Environmental conditions on the Magsaysay reef site were monitored periodically throughout the study from January 2016 to March 2019. Sea temperatures were monitored using Stowaway temperature data loggers deployed at 3 m depth near the experimental plots periodically from January 2017 to April 2018. Additional sea temperature, salinity, dissolved oxygen (DO), and pH water quality parameters were monitored during field trips using a portable Horiba multiprobe instrument at depths of 3–4 m. Additional sea surface temperature (SST) data from January 2016 to March 2019 were obtained from Y29yYWxyZWVmd2F0Y2hAbm9hYS5nb3Y= (Supplementary Figure 1), and light intensity was measured using a LI-COR ®193SA spherical quantum sensor attached to a LI-COR ® LI-1400 data logger (Supplementary Figure 2). The ten to fifteen consecutive light readings were obtained at noon during monitoring field trips, and values averaged.
The three larval enhancement plots and the three control plots were used as statistical replicates (N = 3). Data from the groups of ten tiles within each plot were averaged to quantify mean initial settlement rates, and subsequent growth and number of surviving recruits at each monitoring period up to age 34 months. Data are reported as mean values ± standard error. Differences in larval settlement patterns on different tile surfaces from the larval-enhanced plots after five days of larval settlement were tested using one-way ANOVA. Tukey’s HSD test was conducted post hoc to determine any significant differences in settlement patterns among tile surfaces. The survivorship of coral recruits on different tile surfaces was analysed using non-parametric pairwise comparison survival tests, based on the Kaplan–Meier function (Lee and Wang, 2003). Significant differences in survival patterns of juvenile corals on natural substrata and on tiles from 10 to 34 months after larval settlement were also tested using the same analysis. Growth rates of juvenile corals on recruitment tiles versus growth rates on natural substrata were compared using one-way ANOVA, and significant increases in growth of juvenile corals through time were determined using repeated measures MANOVA. To determine if the assumptions of ANOVA were met, Shapiro-Wilk normality tests and Levene’s test of homoscedasticity were used on each independent variable.
Benthic community patterns within and among experimental plots were compared both before larval enhancement and three years after the larval restoration, using analysis of similarities (ANOSIM) and PERMANOVA (using PRIMER v6) to test for similarities and significant differences in the per cent benthic cover composition of major benthic categories including live corals, soft corals, sponges, other invertebrates, macroalgae, dead coral covered with turf algae, sand, dead coral and rubble.
Differences in fish species richness and fish abundance between larval enhancement and control plots were analysed using Mann-Whitney U-Test in Statistica ® software. Fish species composition between larval enhancement and control plots both before and three years after the larval restoration was graphically presented in two-dimensional ordination plots by non-metric multidimensional scaling (nMDS) using the Bray-Curtis measure of similarity (PRIMER v6). Data were transformed to fourth root so that each species contributed evenly to each analysis. Two-way ANOSIM (analysis of similarity) with pair-wise comparisons was conducted to formally test the significant differences between controls and each treatment. Similarity percentage procedure (SIMPER) was also employed to identify the fish species that contributed to the dissimilarities.
A total of 1,617,000 (± 124,910.20 SE) 8-day old larvae and 3,008,000 (± 244,767.50 SE) 7-day old F2 larvae were available from the cultures and these were divided equally into three groups for deployment in the three larval enhancement plots. High initial larval settlement rates were recorded on tiles in the three larval plots that had each been supplied with an estimated 1.54 million A. tenuis larvae. A total of 6,307 settled spat were recorded on the thirty tiles, with a mean of 210.2 ± 86.36 spat per tile (Figure 4A). Coral spat had well-developed primordial skeletons indicating rapid settlement after release onto the reef plots (Figure 4B). Mean larval settlement per tile was highest in larval plot 1 (382.2 ± 116.18), lower in plot 2 (138.2 ± 37), and lowest in plot 3 (110.3 ± 43). Highest mean settlement rates occurred on the sides and bottom surfaces of tiles with lower rates on the top surfaces, but these were not significantly different (F = 0.53, P = 0.61) (Figure 4C). No A. tenuis or other larvae settled on tiles in control plots covered in mesh enclosures (Figure 4A), and no larvae settled on the 12 tiles located around the outside of the mesh enclosures on the control plots, indicating there was no natural recruitment during the five-day larval settlement experiment.
Figure 4. Initial mean A. tenuis larval settlement on tiles in larval enhanced (N = 3) and control (N = 3) plots after 5 days larval settlement (A), three newly settled spat with well developed primordial skeletons (B), and mean settlement on different tile surfaces in the larval enhanced plots (C). Error bars are ± SE.
As expected, repeated monitoring of settled spat on tiles showed a Type III survivorship pattern characterised by high rates of mortality during the first two months after settlement and lower mortality from two to ten months (Figure 5A). Recruits grew large enough (1.9 ± 0.26 cm mean diameter) to be visible on tiles and on reef substrata after ten months, enabling in situ monitoring of survival and growth. On the settlement tiles, a total of 24 recruits survived to ten months, including 17 recruits on top surfaces, with an additional 261 A. tenuis recruits found on the natural reef substrata in the larval enhancement plots. Some of the larvae settled close together and after a few months some of these juveniles fused to form chimeras. After 25 and 27 months, a total of 14 fused colonies were recorded with each fused colony comprising of two to seven settlers.
Figure 5. Kaplan-Meier survivorship over 34 months for settled A. tenuis polyps, juveniles and recruits in (A) larval enhanced plots on tiles (orange) and for visible recruits on natural reef substrata (green) starting at 10 months after larval settlement, and (B) survivorship of recruits on different tile surfaces in the larval enhanced plots. Asterisk denotes significant difference between tile surfaces.
From 10–34 months after settlement, the number of recruits on tiles declined slowly with 13 recruits alive on top surfaces after 25 months, and eight of those colonies surviving at 34 months (log-rank test, χ2 = −255.24, P = 0.00, top > sides = bottom; Figure 5B). The mean number of surviving colonies on tiles in each of the three larval plots after 34 months was 2.7 ± 0.67 per plot. Higher numbers of juvenile colonies resulting from larval settlement directly on reef substrata were found, with a total of 212 colonies alive in the three larval enhancement plots after 34 months. The mean number of surviving colonies on natural reef substrata in each larval enhancement plot at 34 months was 70.7 ± 16.83, equivalent to ∼5.7 colonies surviving per m2 from larval settlement onto available reef areas in each larval enhancement plot (Figure 6).
Figure 6. High densities of A. tenuis colonies growing on a larval enhancement plot 34 months after larval settlement.
Growth of A. tenuis recruits, juveniles and colonies in the three larval enhancement plots was similar on recruitment tiles and natural reef substrata (Figure 7A), with no significant differences in average growth rates of colonies on tiles and reef surfaces (one-way ANOVA, F = 3.29, P = 0.14). Average growth rates of colonies on tiles from 10–34 months post-settlement were 90.8 ± 36.28 cm3 mo–1, and on reef substrata were 112.6 ± 17.55 cm3 mo–1. Repeated-measures MANOVA showed significant increases in coral volumes through time both on tiles and reef substrata in the larval enhancement plots (F = 11.14, P = 0.000), with no significant difference in volumes between substrata type (F = 0.92, P = 0.392). At 25 months after settlement (June 2018), average volumes of the fused colonies (2158.8 ± 333.02 cm3 mo–1) were 61% higher than those of individual colonies (824.1 ± 67.22 cm3 mo–1).
Figure 7. Mean growth of A. tenuis colonies over 34 months in the three larval enhanced plots, (A) mean volume and (B) mean diameter of colonies on settlement tiles (orange) and natural substrata (green). Error bars are ± SE.
At 10 months post-settlement when recruits were visible on tiles and on the reef, the 24 recruits on the settlement tiles had a mean diameter of 1.4 ± 0.0 cm, and the 261 recruits on the reef substrata had a mean diameter of 2.4 ± 0.07 cm. At 34 months, the eight recruits on the settlement tiles had a mean diameter of 15.9 ± 3.12 cm, and the 212 recruits on the reef substrata had a mean diameter of 18.1 ± 0.82 cm (Figure 7B), with a size range from less than 5 cm up to > 25 cm mean diameter.
Reproductive condition of all colonies was assessed at 23 months’ age, just prior to the potential first spawning period. Five colonies were gravid with pigmented eggs and well developed spermaries. These colonies ranged in size from 13.0 to 21.0 cm mean diameter (Figure 8A), and included one of the colonies growing on tiles, and four colonies growing on natural reef substrata in two of the larval plots. These colonies were observed spawning from 1830 to 1900 h on 1 and 2 May 2018 (1–2 nAFM, Table 1). Gametes collected from spawn cones placed over the gravid colonies were combined and transferred to the BML hatchery where high rates of fertilization were confirmed.
Figure 8. Size frequency plots of mean colony size of gravid and non-reproductive A. tenuis colonies in the three larval enhanced plots (A) in June 2018 and (B) March 2019, and (C) a three year old colony spawning in a larval restoration plot on 25 April 2019.
At 34 months, mean colony size for colonies on both tiles and reef substrata was 17.0 ± 1.86 cm and colony sizes ranged from less than 6 cm to more than 40 cm mean diameter (Figure 8B). A total of 77 colonies were sexually reproductive at this size and age, with gravid colonies ranging in size from 13.2 to 42.3 cm mean diameter (Figure 8B). These gravid colonies included four colonies growing on tiles and 73 colonies growing on natural reef substrata. Gravid colonies were observed spawning (Figure 8C) from 1830 to 1900 h on 23 and 24 May 2019 (4–5 nAFM, Table 1), and gametes were collected from the spawn cones and transferred into floating larval pool nets on the reef for larval culture.
The production cost for each of the 285 sexually derived A. tenuis coral colonies alive at 10 months was United States $13.73. At 34 months age, the production costs for each of the 220 colonies in the restored breeding population was United States $17.79 (Supplementary Table 1).
In 2016, reef benthic cover and coral community status were very similar in the three larval enhancement plots and the three control plots (Figure 9A) with no significant differences in community structure (ANOSIM, R: 0.04, P = 0.30). All plots were degraded and characterised by low mean cover of living scleractinian corals (18.5% ± 2.04%), with similar mean cover of macroalgae and very low cover of soft corals, sponges, other invertebrates and dead coral covered with turf algae, together comprising 21.4% ± 5.72% mean benthic cover. Mean cover of dead coral substrata and coral rubble surfaces potentially available for coral larval settlement was 50.9% ± 6.86%, which represents about 12.5 m2 of the reef area within each of the 25 m2 plots (Figure 9A).
Figure 9. Mean percentage cover of major benthic categories prior to larval enhancement in 2016 (A) and 35 months after larval enhancement in 2019 (B), also mean percentage cover of coral categories prior to larval enhancement in 2016 (C) and 35 months after larval enhancement in 2019 (D). Error bars are ± SE. N = 3 for both larval enhanced and control plots. Asterisk denotes significant difference in mean cover of A. tenuis between larval-enhanced and control plots.
The 2016 coral community had low mean cover of Acropora spp. and higher cover of encrusting Montipora spp., with low cover of Pocilloporidae, Poritidae, Merulinidae and other taxa (Figure 9B). There were no significant differences in mean cover of Acropora (R: −0.14, P = 0.10), Montipora (R: −0.14, P = 0.60), Porites (R: −0.14, P = 0.10), Pocilloporidae (R: −0.04, P = 0.80) or Merulinidae (R: −0.14, P = 0.10) between larval enhanced and control plots prior to the larval restoration experiment.
In March 2019, coral cover had increased 35 months after larval restoration (Figure 9C), with the larval enhancement plots having significantly higher mean cover than controls (univariate PERMANOVA: F = 14.16, P = 0.0001), primarily due to the 9.5% ± 1.30% increase in A. tenuis mean cover from the larval restoration. Total mean cover of reef corals increased from 19.6% ± 3.12% in 2016 to 40.5% ± 6.13% in 2019 in the larval enhancement plots, and from 17.3% ± 0.94% in 2016 to 26.2% ± 5.01% in 2019 in the control plots. Mean cover of non-scleractinian benthic categories were comparable to 2016 levels except for a reduction in macroalgae cover and hard substrata, corresponding with the increased cover of reef corals (Figure 9C).
Three years after larval restoration, A. tenuis cover had increased significantly in the larval enhancement plots compared with control plots (univariate PERMANOVA: F = 158.88, P = 0.0001) in which no new colonies of this species were recorded (Figure 9D). Mean cover of encrusting Montipora spp. had increased substantially in both larval enhancement (univariate PERMANOVA: F = 189.16, P = 0.0001) and control plots (univariate PERMANOVA: F = 3.73, P = 0.05) (Figure 9D). Mean cover of other reef corals was similar between surveys in 2016 and 2019 in both larval enhancement and control plots (Figures 9B,D).
Surveys of fish assemblages in 2016 showed similar low mean fish abundance and species richness in all experimental plots before the larval restoration experiment due to the degraded status of the reef. There were no significant differences between control and larval enhancement plots for fish abundance (Figure 10A; F = 2.7; P = 0.54) or fish species richness (Figure 10B; F = 1.86, P = 0.70) prior to the larval enhancement experiment. Pomacentridae and Labridae were the most abundant fish families, and had similar mean abundance in the larval enhanced and control plots in 2016, with low numbers of Chaetodontidae corallivores present (Figure 11).
Figure 10. Mean total fish abundance (A) and mean species richness (B) in larval enhanced (N = 3) and control (N = 3) plots before the larval restoration experiment in April 2016, and during annual surveys up to 2019. Error bars are ± SE. Asterisk denotes significant difference in mean fish abundance between larval-enhanced and control plots during 2018.
Figure 11. Mean abundance of three commonly occurring fish families (A) Chaetodontidae, (B) Pomacentridae, and (C) Labridae in larval enhanced (N = 3) and control (N = 3) plots before the larval restoration experiment in April 2016 and during annual monitoring surveys until 2019. Error bars are ± SE.
Mean fish abundance and species richness varied slightly between larval enhanced and control plots and among years. Mean fish abundance increased in the larval enhancement plots in 2018 (Figure 10A) and was significantly higher compared to mean abundance in control plots in 2018 (Mann-Whitney U-Test: Z = −1.9640, P = 0.0463). There were no significant differences in fish abundance between larval enhancement and control plots in 2016, 2017, or 2019. Mean fish species richness was slightly higher in larval restoration plots than in control plots in 2018 and 2019 but these differences were non-significant (Figure 10B). The slight increase in fish abundance and change in reef fish assemblages between monitoring years is partly attributable to the increase in Pomacentridae (Figure 11B), specifically the turf farmer pomacentrids such as Pomacentrus burroughi, P. chrysurus, and Plectroglyphidodon lacrymatus.
Reef fish assemblages were similar in all plots, although assemblages in larval enhancement plots were mostly clustered separately from the control plots in each of the monitoring years (Figure 12). However, reef fish assemblages recorded in 2016 were significantly different (R = 0.466, P = 0.001) from the assemblages recorded in 2017, 2018, and 2019 (Figure 12). Pairwise comparisons showed significant differences between 2016 and each of the subsequent years (P = 0.01), but no significant differences between the years 2017, 2018, and 2019. In addition to the turf farmer fishes, pomacentrids that take refuge within Acropora coral branches such as Dascyllus reticulatus, Chromis viridis, and Amblyglyphidodon curacao contributed to the differences in reef fish assemblages in 2016 and later years.
Figure 12. Non-metric multidimensional scaling (nMDS) plot showing patterns in fish assemblages in the larval-enhanced (N = 3) and control (N = 3) plots from 2016 to 2019.
A conceptual diagram summarising A. tenuis recruitment and growth, and changes in coral cover and reef fish assemblages during the three years following the 2016 larval restoration at Magsaysay reef is provided at Figure 13.
Figure 13. Conceptual diagram showing changes in coral cover and reef community assemblage in the three years following the 2016 larval restoration at Magsaysay reef.
Large scale multi-species synchronous coral spawning events were observed in situ on night dives around a remnant “coral garden” reef patch with high coral cover and species richness on Magsaysay reef in 2016, 2017, and 2018, with coral spawn slicks forming at the sea surface on peak spawning nights (Table 2).
The spawning events noted in Table 2 typically involved Acropora spp. colonies “setting” egg and sperm bundles under the inflated oral disc of polyps (sensu Harrison et al., 1984) from ∼1940 h, with buoyant bundles starting to be released synchronously from polyps by ∼2020 h, and spawning of some colonies occurring up to 2200 h and later. The largest coral spawn slicks developed on peak coral spawning nights (10–11 nAFM) that coincided with calm weather with low wind speeds and swell. As wind speed increased, spawned egg-sperm bundles, gametes and the slicks became dispersed across the sea surface.
These predictable large scale spawning events provided ready access to hundreds of millions of gametes from many colonies of diverse coral species, enabling development of new techniques and equipment for spawn collection and mass embryo and larval culture directly on reefs. In the 2016 pilot study, millions of gametes were collected in the 5 × 5 m net system that was attached to a floating bamboo frame deployed above healthy coral communities on 31 March (Figures 3A,B). Healthy developing embryos were recorded within the culture pool 12 and 20 h after spawning indicating that the system provided suitable environmental conditions for larval rearing on the reef. Additional coral spawn was added to the pool after spawning on 1 April before increasing wind dispersed the spawn at the sea surface preventing further collection. Strong winds and heavy wave action began to damage one corner of the larval culture pool’s bamboo frame early the next morning, causing most of the developing larvae to wash out of the net system. Consequently, later designs of the low-cost bamboo frames were cross-braced and strengthened and four bamboo frames and a prototype semi-submersed 2 × 2 m PVC pipe frame were stress-tested in sea trials while anchored near Magsaysay reef. These frames remained intact after three weeks including intermittent periods of strong winds and heavy wave action.
The at-sea larval collection and culture process were re-designed in 2017 (Figure 3C). Spawn slick samples were collected after the major spawning on 10 nAFM in March 2017, and an estimated 317,000 larvae were reared in the larval culture pool net enclosure supported within a 5 × 5 m steel frame with drum floats, which was temporarily moored adjacent to Magsaysay reef.
In 2018, the rearing pool net was further refined (Figure 3D), allowing for release of larvae directly from the net onto target reef sites by opening the zippered base. Coral spawn slicks were captured within the spawn catcher and larval pools after major spawning events on 12th and 13th March 2018, with > 90% fertilization rates recorded in samples.
Coral restoration should aim to re-establish breeding coral populations on damaged reefs using methods that are cost-effective and scalable, with restored populations capable of surviving and adapting to altered environmental conditions and stressors (Harrison, 2021). However, before restoration is attempted on any reef site it is important to use a decision framework to evaluate the need for such active interventions, and the likelihood of success using appropriate methods.
Initial baseline surveys are important to establish the status of reef communities, and determine the likelihood of natural recruitment enabling recovery within an appropriate timeframe without intervention. If the reef system is degraded and has very low natural recruitment and therefore unlikely to recover naturally, then active intervention is warranted if environmental conditions are potentially suitable for restoring coral populations in a cost-effective manner. Magsaysay reef, chosen for this study, is badly degraded with an algal phase-shifted reef community now characterised by low mean cover of live corals and few natural recruits, as it was in the 2013 larval restoration pilot study (dela Cruz and Harrison, 2017). That study also showed natural recruitment rates were low, and dominated by brooded pocilloporid spat with minimal Acropora recruits present on > 300 recruitment tiles deployed during a two-year period. Therefore, Magsaysay reef is unlikely to recover naturally without intervention.
The decision framework should also consider whether degraded reef systems are potentially recoverable, and the extent to which stressors and key threats that led to coral decline and reef degradation are still operating, or can be potentially managed or tolerated by new generations of restored corals. Previous key threats in the Bolinao-Anda Reef Complex (BARC) where this study was conducted included destructive fishing that is now controlled, a crown-of-thorns starfish corallivore outbreak in 2007 which is unlikely to re-occur given the low coral cover, and intermittent heat-stress and coral bleaching that had minimal impact on the 2013 restored A. tenuis population (dela Cruz and Harrison, 2017). Another key consideration for restoring degraded reefs is the extent to which the reef system is severely phase-shifted with low functional herbivory. Results from the 2013 pilot study showed that although the Magsaysay reef site is algal-dominated, enhancing larval supply significantly increased A. tenuis recruitment on larval restoration plots and restored a breeding population after three years (dela Cruz and Harrison, 2017). Therefore, Magsaysay reef is potentially recoverable, and restoring corals through increasing supply of sexually produced coral larvae can enhance genetic diversity and evolutionary potential, potentially improving environment-genotype matching and resilience of surviving corals (van Oppen et al., 2017).
Our results confirm that mass larval enhancement can significantly increase the initial settlement of corals even on degraded reefs where natural larval supply and coral recruitment have been compromised by loss of adult breeding corals and high turf and macroalgal cover. In this study, we used ∼3.7 times higher supply densities of coral larvae than in the 2013 larval enhancement pilot study (dela Cruz and Harrison, 2017), which resulted in about eight times higher mean initial larval settlement per tile in 2016 compared with 2013. This is consistent with Cameron and Harrison (2020), who found a strong positive relationship between increasing larval density and total larval settlement. Higher rates of settlement in the present study therefore reflect the higher larval supply densities in plots and may also have been enhanced by the use of 7–8 day old larvae that were more fully developed and potentially primed for rapid settlement when released onto the reef areas, compared with the 4 day old larvae used in 2013. Most of the newly settled spat in the 2016 study had well-developed skeletons visible through the translucent polyp tissues (Figure 4B), indicating that the larvae probably settled rapidly after being released into the mesh enclosures on the reef. In addition, the mesh tent enclosures may have allowed larvae to actively swim and search for suitable settlement sites more effectively than under the flat mesh sheets used in the 2013 study. No A. tenuis recruits settled on tiles in control plots covered in mesh enclosures or on the 12 tiles in open control areas without mesh, which confirms low natural larval supply during the larval settlement experiment, similar to previous pilot studies (dela Cruz and Harrison, 2017, 2020b).
Mortality of settled spat and juvenile colonies on tiles was highest during the first 10 months after settlement and then stabilised (Figure 5), consistent with the Type III survivorship curve reported for other broadcast spawning corals (Babcock, 1985; Wilson and Harrison, 2005; Vermeij and Sandin, 2008; Doropoulos et al., 2016; dela Cruz and Harrison, 2017, 2020b) and marine invertebrates (Keough and Downes, 1982; Roughgarden et al., 1985; Hunt and Scheibling, 1997). The causes of mortality of the microscopic newly settled polyps on tiles are not known, but previous studies have noted overgrowth and competition from other benthic biota including allelopathic effects of algae, predation, damage from herbivore grazing, and reduced water quality and runoff from nearby coastal communities contributing to juvenile mortality (Sammarco and Carleton, 1981; Harrington et al., 2004; Penin et al., 2010; Guest et al., 2014; dela Cruz and Harrison, 2017; Cameron and Harrison, 2020). High mortality during early post-settlement life stages may also be a consequence of newly metamorphosed settled polyps having insufficient energy reserves for survival after expending energy and resources for metamorphosis and the onset of skeletogenesis (Harrison and Wallace, 1990), prior to uptake of mutualistic Symbiodiniaceae.
Recruits were visible on tiles and on reef surfaces by 10 months and repeated monitoring showed high rates of survival up to 35 months, consistent with high survivorship patterns of visible Acropora spp. Recruits after colonies reached size-escape thresholds that were recorded in previous studies (Babcock, 1991; Raymundo and Maypa, 2004; Ritson-Williams et al., 2009; Doropoulos et al., 2012; dela Cruz and Harrison, 2017, 2020b). The survivorship pattern of A. tenuis recruits from larvae that settled directly on reef substrata in 2016 (Figure 5) was intermediate between the pattern recorded for A. tenuis recruits in 2013 (dela Cruz and Harrison, 2017) and for A. loripes recruits (dela Cruz and Harrison, 2020b), and substantially higher than for A. digitifera recruits on ceramic plugs outplanted onto reef areas in Palau after 5 and 11 months (Humanes et al., 2021).
Mean growth rates of A. tenuis recruits and juveniles were similar on tiles and on natural reef substrata, and both were higher than for recruits from the 2013 pilot study (dela Cruz and Harrison, 2017). These growth rates were higher than those recorded for A. tenuis settled in a hatchery and then outplanted onto reef areas after 18 months in Akajima, Japan (Iwao et al., 2010), and for A. millepora colonies grown in the BML hatchery or outplanted as sub-adults onto BARC reef areas in Northern Luzon, Philippines (Baria et al., 2012; Guest et al., 2014). Growth rates recorded during the present study were also higher than for A. loripes colonies growing from larvae settled directly in larval enhancement plots on Magsaysay reef (dela Cruz and Harrison, 2020b), and for A. digitifera colonies settled in an ex situ nursery and outplanted at five or 11 months (Humanes et al., 2021).
The rapid growth of most A. tenuis colonies resulting from direct larval settlement on reef areas indicates that environmental conditions are still suitable for survival and growth of this species on Magsaysay reef, despite its badly degraded status. Sea temperatures during this study ranged from 26.5 to 31°C (Supplementary Figure 1) and remained below the coral bleaching thresholds observed previously at Magsaysay reef (dela Cruz and Harrison, 2017). Seasonal changes were also evident in other environmental parameters including periods of reduced salinity and high turbidity from rainfall and runoff associated with monsoon conditions (Supplementary Figure 2). It is possible that elevated organic inputs and nutrients from seepage and runoff from adjacent coastal towns onto these nearshore reef systems are providing supplementary allochthonous food resources that are enhancing heterotrophic particulate feeding and dissolved organic matter uptake (Sorokin, 1993; Anthony, 2000), supplementing energy supplied from photosymbionts and predation on plankton. Larval settlement behaviour and suitable microhabitat selection directly on the reef may also have contributed to rapid growth of surviving colonies in comparison with other Acropora colonies reared from larvae that were settled in nurseries and subject to artificial environmental conditions and selection pressures during early life stages (Iwao et al., 2010; Baria et al., 2012; Guest et al., 2014).
The high densities of larvae supplied to the larval enhancement plots resulted in gregarious settlement of some larvae, and subsequent growth and fusion resulted in 14 chimeric colonies consisting of between two and seven individuals after two years. Chimerism has been reported among populations of Acropora species on reef areas (Puill-Stephan et al., 2009; Schweinsberg et al., 2015) and in experimental studies (dela Cruz and Harrison, 2017; Doropoulos et al., 2017; Cameron and Harrison, 2020; Sampayo et al., 2020), and may lead to enhanced early growth and survival to larger size refugia with potential for increased adaptive potential to changing environmental conditions.
Rapid colony growth also resulted in early onset of sexual reproduction in five colonies (13 to 21 cm mean diameter) that had mature gametes at two years after settlement, and therefore oogenesis was likely to have been initiated about 9 months prior to this at smaller colony sizes (Wallace, 1985; Harrison and Wallace, 1990; Randall et al., 2020). This is the fastest growth to sexual reproduction yet recorded in Acropora corals, and more rapid than predicted based on previous studies of this species (Wallace, 1985; Iwao et al., 2010; dela Cruz and Harrison, 2017) and other Acropora (Baria et al., 2012). Most colonies were sexually reproductive at three years of age with mean colony sizes above 13 cm diameter, thereby re-establishing a functional breeding population on the degraded reef system. The variability in colony sizes among breeding colonies is likely to reflect individual phenotype and holobiont responses to environmental conditions on Magsaysay reef, and highlights the complexity of predicting the age and size of sexual reproduction among reef corals (Wallace, 1985; Harrison and Wallace, 1990; Randall et al., 2020).
The timing of A. tenuis spawning was consistent among years and populations with all colonies recorded spawning during the crepuscular period around sunset, similar to spawning records from the Great Barrier Reef (Harrison et al., 1984; Willis et al., 1985; Babcock et al., 1986) and elsewhere (Baird et al., 2021). The lunar periodicity of spawning varied slightly among years and between the reef and BML culture facility (Table 1). Variability in the lunar night of spawning has been recorded among many Acropora populations in various reef regions, whereas some other taxa such as Merulinidae exhibit more consistent lunar periodicity of spawning, indicating that coral taxa may respond to proximate cues and ultimate selective pressures in different ways (Babcock et al., 1986; Harrison and Wallace, 1990; Hoadley et al., 2016; Randall et al., 2020).
The rapid re-establishment of breeding populations is important for initiating recovery of degraded reefs. Success at localised scales, such as achieved in this study, creates ongoing opportunities to expand larval restoration efforts to adjacent reef areas in future, using some of the millions of gametes now released annually by this population. These breeding populations also contribute to the depleted natural larval supply in the Lingayen Gulf, and some of these genetically diverse larvae are likely to disperse to other reefs and enhance recruitment and reef connectivity at larger scales over time (Harrison, 2006; Jones et al., 2009; Randall et al., 2020). As these breeding colonies grow and their spawning biomass increases, they become more fecund and hence their ecological value increases. In addition, the high densities of breeding colonies established during this reef trial are likely to enhance fertilisation rates on Magsaysay reef from high sperm and egg concentrations following synchronous spawning events (Oliver and Babcock, 1992; Levitan and Petersen, 1995; Yund, 2000). Remarkably, we know very little about the densities of breeding corals required to maximise fertilisation and cross-fertilisation rates on reefs, therefore future restoration trials should consider not only the overall abundance and spatial scales of restored colonies but also their densities for optimising breeding success (Teo and Todd, 2018).
Key issues for coral restoration that need to be resolved are production costs and scalability. The average production costs for each A. tenuis coral colony at 10 months was United States $13.70, and United States $17.80 for each of the 220 colonies in the restored breeding population at 34 months age. These costs were lower than for the 2013 pilot study United States $21.00 per colony at 35 months (dela Cruz and Harrison, 2017) and for A. loripes United States $35.00 at 35 months (dela Cruz and Harrison, 2020b), largely as a result of significantly increased larval supply and higher numbers of recruits and adult colonies surviving in the present study. This indicates that the cost-effectiveness of larval restoration should increase as mass larval production increases for larger-scale delivery onto damaged reefs, as long as long as settlement and recruitment density is optimised to avoid negative density-dependent mortality effects (Doropoulos et al., 2017; Cameron and Harrison, 2020). These production costs per colony for direct larval settlement onto degraded reef areas are substantially lower than for colonies reared in nurseries for extended periods prior to outplanting on reefs. For example, the production costs for 2.5 year old A. millepora colonies initially settled and held in the BML nursery for 7–19 months before outplanting onto reef areas in Northern Luzon, Philippines ranged from United States $284 to $61, respectively (Guest et al., 2014), and for 2.5 year old A. digitifera recruits settled and held in a nursery in Palau for 5 and 11 months before outplanting (United States $227 and $49, respectively; Humanes et al., 2021). These simple production cost metrics do not take into account the growing ecological and socio-economic values of these restored breeding colonies, which provide critical habitats for fish and other reef organisms, and annual increases in fecundity and production of millions of larvae.
Baseline reef community surveys in 2016 showed that the Magsaysay reef experimental plots were characterised by low mean live cover of reef corals and high cover of algae, and reduced abundance and diversity of reef fish, consistent with other degraded algal phase-shifted reefs (Bruno et al., 2009; Cheal et al., 2010; Ceccarelli et al., 2018). Three years after larval restoration, mean coral cover had doubled in the restoration plots to 40% primarily due to the restored A. tenuis population and growth of encrusting Montipora colonies present in the plots prior to larval enhancement. Coral cover also increased in the control plots due to growth of encrusting Montipora, but Acropora cover and growth of other colonies was negligible, and no additional A. tenuis colonies recruited onto the control plots over the three years of monitoring. These results indicate low natural larval supply and recruitment, therefore reef recovery will require active intervention through increased larval supply to catalyse the recovery of the foundation coral communities.
The significant increase in coral cover resulting from coral larval restoration corresponded with, and likely influenced, some changes in reef fish assemblages through time. There was a small increase in pomacentrids that shelter within coral branches (Coker et al., 2014) and an increase in chaetodontids that mainly feed on coral polyps (Cole and Pratchett, 2011) in the larval restoration plots; trends not evident in the control plots. This suggests that the larval restoration treatment enhanced the availability of suitable coral habitats for some fish on these reef areas. Similarly, increased abundance and diversity of reef fish and macroinvertebrates have been reported on other BARC reef areas following outplanting of coral fragments to increase coral biomass and reef structure (Cabaitan et al., 2008; dela Cruz et al., 2014).
Overall, fish assemblages in all plots were characterised by relatively low abundance and diversity, and dominated by small bodied individuals, reflecting the degraded status of the reef site (Jones et al., 2004; Nash and Graham, 2016). Although there were no clear differences in abundance of common reef fish functional guilds and families between control and larval restoration plots, these assemblages varied through time, particularly between 2016 and subsequent monitoring years. As the larval restoration and control plots are located within 10–20 m of each other, the increase in branching coral cover in the former may have influenced the mobile fish assemblages in the control plots. In addition, although the Magsaysay reef plots are included in the designated Magsaysay MPA, there is no enforcement of no-take zones on the reef and some fishers continue to fish within the MPA, so ongoing fishing pressures are likely to affect the fish assemblages at the restoration sites. Further community engagement and education about the need to protect the Magsaysay MPA reef sites, combined with increased local management and enforcement of fishing restrictions, is needed to enable fish habitats and fish assemblages to more fully recover and provide increased fish resources to other reef areas nearby (McCook et al., 2010; Russ et al., 2015).
To effectively scale up larval restoration and produce hundreds of millions or billions of coral larvae, we need to develop larger scale reef-based larval culture methods that are cost-effective and adaptable to different reef environments. Large-scale multispecies spawning events occur on many reefs around the world and often result in the formation of coral spawn slicks at the sea surface (Harrison et al., 1984; Babcock et al., 1986; Harrison and Wallace, 1990; Randall et al., 2020; Baird et al., 2021). These slicks provide ready access to billions or trillions of gametes, and enable collection of slick samples for larval rearing on reefs (Heyward et al., 2002; Omori, 2005; Doropoulos et al., 2019; Harrison, 2021).
The development of the integrated spawn catcher and larger larval culture pools in this study enables simple routine collection of coral spawn slicks containing hundreds of millions of egg-sperm bundles from diverse species, and mass culture of larvae from a diverse range of corals for mass larval supply over larger reef areas in future. These larval pools provide an effective method for mass larval production directly on reefs without the high costs of maintaining larvae and settlers in nurseries or on large vessels, and the floating frames can be produced at low cost ∼United States $200.00 each from bamboo, which is readily available throughout SE Asia and other major reef areas. The prototype bamboo frame was impacted by strong winds and rough seas, but subsequent cross-bracing strengthened the frame making it suitable for deployment in reef environments. The steel frames cost about United States $600.00 each but are more robust and have been used for six years, so these can provide a more cost effective approach if larval restoration is likely to be done over multiple years and in more exposed reef conditions.
The results of this study confirm that increasing larval supply and direct settlement on degraded reef areas can rapidly re-establish a breeding population of Acropora tenuis within two to three years and lead to significantly increased coral cover on restoration plots compared with control reef plots reliant on depleted natural larval supply. The higher densities of larval supply used in this study significantly increased larval settlement, recruitment and production of adult corals at higher densities on larval restoration plots, and at reduced cost compared with earlier studies. In addition, the increased cover of branching coral colonies corresponded with increased abundance of pomacentrids reliant on sheltering in branches, and increased chaetodontid corallivores on the larval restoration plots. Ongoing artisanal fishing pressures in the Magsaysay MPA will need to be managed in order to increase the abundance of larger fish and spawning stocks within the restoration areas, to enhance “spill-over” effects into nearby reef areas.
The new techniques for in situ spawn slick collection and larval culture on reefs developed in this study will enable more cost-effective mass larval production for increased scales of larval supply and restoration over larger reef areas in future. Use of natural spawn slicks will also enable multi-species cultures for restoring more diverse coral communities rather than single species populations. High post-settlement mortality bottlenecks that constrain recruitment in corals can be overcome by supplying higher densities of competent larvae. This allows natural selection pressures to operate on larger populations of settlers to select for genotypes that are better adapted to altered reef conditions. In addition, pre-settlement of competent larvae onto suitable natural dead coral or manufactured settlement surfaces with appropriate microtopography and microbial communities within the larval culture pools prior to deployment onto reef restoration areas should also significantly increase post-settlement survival, as would co-culturing larvae with more thermally tolerant Symbiodiniaceae to increase energy supply after settlement. Together, these approaches will increase coral recruitment success leading to faster and more efficient restoration of coral communities at larger scales.
The raw data supporting the conclusions of this article will be made available by the authors, without undue reservation.
PH obtained grant funding, designed the settlement nets and conceived the larval restoration, spawn catcher and larval pool methods, and wrote the draft manuscript. PH and DC designed the study and monitored larval settlement and interpreted data. DC collected and analysed benthic, recruit survival and growth data, and produced figures. PC co-ordinated monitoring fish assemblages and analysed fish data. KC contributed to larval rearing and collection of growth data and figures. All authors contributed to field work, manuscript revision, read, and approved the submitted version.
The authors declare that the research was conducted in the absence of any commercial or financial relationships that could be construed as a potential conflict of interest.
All claims expressed in this article are solely those of the authors and do not necessarily represent those of their affiliated organizations, or those of the publisher, the editors and the reviewers. Any product that may be evaluated in this article, or claim that may be made by its manufacturer, is not guaranteed or endorsed by the publisher.
We thank the Australian Centre for International Agricultural Research (ACIAR) for funding this research: grant ACIAR/FIS/2014/063 to PH, PC and J. Bennett. Thanks to ACIAR staff Chris Barlow, Ann Fleming, and Mai Alagcan for their ongoing support. Sincere thanks to the Galsim Family for use of Tanduyong Island as a field research base during the coral restoration fieldwork. We also thank staff and students at the Bolinao Marine Laboratory, Marine Science Institute, University of the Philippines, Diliman for their assistance with reef work: Elizabeth Gomez, Charlon Ligson, Rickdane Gomez and Fernando Castrence (including fish surveys), Marcos Ponce, Joey Cabasan, Sheldon Boco, Gabriel de Guzman, Albert Ponce, and Allan Abuan. We also thank Grant Cameron for field support and helping design, build and refine the prototype floating spawn catcher frames in 2016 and 2017.
The Supplementary Material for this article can be found online at: https://www.frontiersin.org/articles/10.3389/fmars.2021.750210/full#supplementary-material
Supplementary Figure 1 | Sea Surface Temperature record obtained from NOAA.
Supplementary Figure 2 | Environmental data in the experimental plots. * = no data.
Supplementary Table 1 | Summary of costs for larval enhancement and production of A. tenuis coral colonies.
Allen, G., Steene, R., Humann, P., and Deloach, N. (2005). Reef Fish Identification: Tropical Pacific Fishes. California, CA: New World Publications.
Anthony, K. R. N. (2000). Enhanced particle-feeding capacity of corals on turbid reefs (Great Barrier Reef. Australia). Coral Reefs 19, 59–67. doi: 10.1007/s003380050227
Babcock, R. C. (1985). “Growth and mortality in juvenile corals (Goniastrea, Platygyra, and Acropora): The first year,” in Proceedings of the 5th Int’l Coral Reef Congress, Vol. 4, ed. C. Gabrie (Tahiti: Antenne Museum-EPHE, Moorea, French Polynesia), 355–360.
Babcock, R. C. (1991). Comparative demography of three species of scleractinian corals using age- and size-dependent classifications. Ecol. Monogr. 61, 225–244.
Babcock, R. C., Bull, G. D., Harrison, P. L., Heyward, A. J., Oliver, J. K., Wallace, C. C., et al. (1986). Synchronous spawnings of 105 scleractinian coral species on the Great Barrier Reef. Mar. Biol. 90, 379–394.
Baird, A. H., Guest, J. R., Edwards, A. J., Bauman, A. G., Bouwmeester, J., Mera, H., et al. (2021). An Indo-Pacific coral spawning database. Sci. Data 8, 1–10. doi: 10.1038/s41597-020-00793-8
Baria, M. V. B., dela Cruz, D. W., Villanueva, R. D., and Guest, J. R. (2012). Spawning of three-year-old Acropora millepora corals reared from larvae in northwestern Philippines. Bull. Mar. Sci. 88, 61–62. doi: 10.5343/bms.2011.1075
Baums, I. B. (2008). A restoration genetics guide for coral reef conservation. Mol. Ecol. 17, 2796–2811. doi: 10.1111/j.1365-294X.2008.03787.x
Beijbom, O., Edmunds, P. J., Roelfsema, C., Smith, J., Kline, D. I., Neal, B. P., et al. (2015). Towards automated annotation of benthic survey images: variability of human experts and operational modes of automation. PLoS One 10:e0130312. doi: 10.1371/journal.pone.0130312
Birkeland, C. (1997). Life and Death of Coral Reefs, ed. C. Birkeland (New York, NY: Chapman and Hall).
Bohnsack, J. A., and Bannerot, S. P. (1986). A Stationary Visual Census Technique for Quantitatively Assessing Community Structure of Coral Reef Fishes. NOAA Technical Report NMFS 41. Washington, DC: NOAA, 21.
Boström-Einarsson, L., Babcock, R. C., Bayraktarov, E., Ceccarelli, D., Cook, N., Ferse, S. C. A., et al. (2020). Coral restoration – a systematic review of current methods, successes, failures and future directions. PLoS One 15:e0226631. doi: 10.1371/journal.pone.0226631
Bruno, J. F., and Selig, E. R. (2007). Regional decline of coral cover in the Indo-Pacific: timing, extent, and subregional comparisons. PLoS One 2:e0000711. doi: 10.1371/journal.pone.0000711
Bruno, J. F., Sweatman, H., Precht, W. H., Selig, E. R., and Schutte, V. G. W. (2009). Assessing evidence of phase shifts from coral to macroalgal dominance on coral reefs. Ecology 90, 1478–1484. doi: 10.1890/08-1781.1
Burke, L., Reytar, K., Spalding, M., and Perry, A. (2011). Reefs at Risk Revisited. Available online at: http://www.reefbase.org/resource_center/publication/main.aspx?refid=72882 (acesssed July 17, 2020).
Cabaitan, P. C., Gomez, E. D., and Aliño, P. M. (2008). Effects of coral transplantation and giant clam restocking on the structure of fish communities on degraded patch reefs. J. Exp. Mar. Biol. Ecol. 357, 85–98. doi: 10.1016/j.jembe.2008.01.001
Cameron, K. A., and Harrison, P. L. (2020). Density of coral larvae can influence settlement, post-settlement colony abundance and coral cover in larval restoration. Sci. Rep. 10, 1–11. doi: 10.1038/s41598-020-62366-4
Ceccarelli, D. M., Loffler, Z., Bourne, D. G., Al Moajil-Cole, G. S., Boström-Einarsson, L., Evans-Illidge, E., et al. (2018). Rehabilitation of coral reefs through removal of macroalgae: state of knowledge and considerations for management and implementation. Restor. Ecol. 26, 827–838. doi: 10.1111/rec.12852
Chamberland, V. F., Petersen, D., Guest, J. R., Petersen, U., Brittsan, M., and Vermeij, M. J. A. (2017). New seeding approach reduces costs and time to outplant sexually propagated corals for reef restoration. Sci. Rep. 7, 1–12. doi: 10.1038/s41598-017-17555-z
Cheal, A. J., MacNeil, M. A., Cripps, E., Emslie, M. J., Jonker, M., Schaffelke, B., et al. (2010). Coral-macroalgal phase shifts or reef resilience: links with diversity and functional roles of herbivorous fishes on the Great Barrier Reef. Coral Reefs 29, 1005–1015. doi: 10.1007/s00338-010-0661-y
Coker, D. J., Wilson, S. K., and Pratchett, M. S. (2014). Importance of live coral habitat for reef fishes. Rev. Fish Biol. Fish. 24, 89–126. doi: 10.1007/s11160-013-9319-5
Cole, A. J., and Pratchett, M. S. (2011). Effects of juvenile coral-feeding butterflyfishes on host corals. Coral Reefs 30, 623–630. doi: 10.1007/s00338-011-0746-2
Connell, J. H., Hughes, T. P., and Wallace, C. C. (1997). A 30-year study of coral abundance, recruitment, and disturbance at several scales in space and time. Ecol. Monogr. 67, 461–488. doi: 10.1890/0012-96151997067[0461:AYSOCA]2.0.CO;2
Cruz-Trinidad, A., Geronimo, R. C., and Aliño, P. M. (2009). Development trajectories and impacts on coral reef use in Lingayen Gulf, Philippines. Ocean Coast. Manag. 52, 173–180. doi: 10.1016/j.ocecoaman.2008.12.002
De’ath, G., Fabricius, K. E., Sweatman, H., and Puotinen, M. (2012). The 27-year decline of coral cover on the Great Barrier Reef and its causes. Proc. Natl. Acad. Sci. 109, 17995–17999. doi: 10.1073/pnas.1208909109
dela Cruz, D. W., and Harrison, P. L. (2017). Enhanced larval supply and recruitment can replenish reef corals on degraded reefs. Sci. Rep. 7, 1–13. doi: 10.1038/s41598-017-14546-y
dela Cruz, D. W., and Harrison, P. L. (2020a). Enhancing coral recruitment through assisted mass settlement of cultured coral larvae. PLoS One 15:e0242847. doi: 10.1371/journal.pone.0242847
dela Cruz, D. W., and Harrison, P. L. (2020b). Optimising conditions for in vitro fertilization success of Acropora tenuis, A. millepora and Favites colemani corals in Northwestern Philippines. J. Exp. Mar. Biol. Ecol. 524:151286. doi: 10.1016/j.jembe.2019.151286
dela Cruz, D. W., Villanueva, R. D., and Baria, M. V. B. (2014). Community-based, low-tech method of restoring a lost thicket of Acropora corals. ICES J. Mar. Sci. 71, 1866–1875. doi: 10.1093/icesjms/fst228
Done, T. J. (1992). Constancy and change in some Great Barrier Reef coral communities?: 1980-1990. Am. Zool. 32, 655–662.
Doropoulos, C., Elzinga, J., ter Hofstede, R., van Koningsveld, M., and Babcock, R. C. (2019). Optimizing industrial-scale coral reef restoration: comparing harvesting wild coral spawn slicks and transplanting gravid adult colonies. Restor. Ecol. 27, 758–767. doi: 10.1111/rec.12918
Doropoulos, C., Evensen, N. R., Gómez-Lemos, L. A., and Babcock, R. C. (2017). Density-dependent coral recruitment displays divergent responses during distinct early life-history stages. R. Soc. Open Sci. 4:170082. doi: 10.1098/rsos.170082
Doropoulos, C., Roff, G., Bozec, Y. M., Zupan, M., Werminghausen, J., and Mumby, P. J. (2016). Characterizing the ecological trade-offs throughout the early ontogeny of coral recruitment. Ecol. Monogr. 86, 20–44.
Doropoulos, C., Ward, S., Marshell, A., Diaz-Pulido, G., and Mumby, P. J. (2012). Interactions among chronic and acute impacts on coral recruits: the importance of size-escape thresholds. Ecology 93, 2131–2138. doi: 10.1002/ecy.1852
Edwards, A. J., (ed.) (2010). Reef Rehabilitation Manual. St Lucia: Coral Reef Targeted Research & Capacity Building or Management Program.
Edwards, A. J., Guest, J. R., Heyward, A. J., Villanueva, R. D., Baria, M. V., Bollozos, I. S. F., et al. (2015). Direct seeding of mass-cultured coral larvae is not an effective option for reef rehabilitation. Mar. Ecol. Prog. Ser. 525, 105–116. doi: 10.3354/meps11171
Gilmour, J. P., Smith, L. D., Heyward, A. J., Baird, A. H., and Pratchett, M. S. (2013). Recovery of an isolated coral reef system following severe disturbance. Science 340, 69–71. doi: 10.1126/science.1232310
Gomez, E. D., Alcala, A. C., and San Diego, A. C. (1981). “Status of Philippine coral reefs,” in Proceedings of the 4th Int’l Coral Reef Symp, Manila, ed. E. D. Gomez (Manila, Diliman: Marine Sciences Center, University of the Philippines), 275–282.
Gouezo, M., Golbuu, Y., Fabricius, K., Olsudong, D., Mereb, G., Nestor, V., et al. (2019). Drivers of recovery and reassembly of coral reef communities. Proc. R. Soc. B Biol. Sci. 286:20182908. doi: 10.1098/rspb.2018.2908
Guest, J. R., Baria, M. V., Gomez, E. D., Heyward, A. J., and Edwards, A. J. (2014). Closing the circle: is it feasible to rehabilitate reefs with sexually propagated corals? Coral Reefs 33, 45–55. doi: 10.1007/s00338-013-1114-1
Harrington, L., Fabricius, K., De’ath, G., and Negri, A. (2004). Recognition and selection of settlement substrata determine post-settlement survival in corals. Ecology 85, 3428–3437. doi: 10.1890/04-0298
Harrison, P. L. (2006). “Settlement competency periods and disersal potential of scleractinian reef coral larvae,” in Proceedings of the 10th Int’l Coral Reef Symposium, (Okinawa).
Harrison, P. L. (2011). “Sexual reproduction of scleractinian corals,” in Coral Reefs: An Ecosystem in Transition, eds Z. Dubinsky and N. Stambler (Dordrecht: Springer).
Harrison, P. L. (2021). More sex on the reef: can coral spawning help save reefs? Ocean Geogr. 56, 25–33.
Harrison, P. L., Babcock, R. C., Bull, G. D., Oliver, J. K., Wallace, C. C., and Willis, B. E. (1984). Mass spawning in tropical reef corals. Science 223, 1186–1189. doi: 10.1126/science.223.4641.1186
Harrison, P. L., and Booth, D. J. (2007). “Coral reefs: naturally dynamic and increasingly disturbed ecosystems,” in Marine Ecology, eds S. D. Connell and B. M. Gillanders (Melbourne: Oxford University Press), 316–377.
Harrison, P. L., Villanueva, R. D., and dela Cruz, D. W. (2016). Coral Reef Restoration Using Mass Coral Larval Reseeding. Final Report to Australian Centre for International Agricultural Research, Project SRA FIS/2011/031. Canberra: Australian Centre for International Agricultural Research.
Harrison, P. L., and Wallace, C. C. (1990). “Reproduction, dispersal and recruitment of scleractinian corals,” in Coral Reefs, ed. Z. Dubinsky (Amsterdam: Elsevier Science Publishers), 133–207.
Heyward, A. J., Smith, L. D., Field, S. N., and Rees, M. (2002). Enhancement of coral recruitment by in situ mass culture of coral larvae. Mar. Ecol. Prog. Ser. 230, 113–118. doi: 10.3354/meps230113
Hoadley, K. D., Vize, P. D., and Pyott, S. J. (2016). “Current understanding of the circadian clock within Cnidaria,” in The Cnidaria, Past, Present and Future, eds S. Goffredo and Z. Dubinsky (Berlin: Springer), 511–520. doi: 10.1016/j.margen.2014.01.003
Hoegh-Guldberg, O., Poloczanska, E. S., Skirving, W., and Dove, S. (2017). Coral reef ecosystems under climate change and ocean acidification. Front. Mar. Sci. 4:158. doi: 10.3389/fmars.2017.00158
Hughes, T. P., Kerry, J. T., Baird, A. H., Connolly, S. R., Chase, T. J., Dietzel, A., et al. (2019). Global warming impairs stock–recruitment dynamics of corals. Nature 568, 387–390. doi: 10.1038/s41586-019-1081-y
Hughes, T. P., Kerry, J. T., Baird, A. H., Connolly, S. R., Dietzel, A., Eakin, C. M., et al. (2018). Global warming transforms coral reef assemblages. Nature 556, 492–496. doi: 10.1038/s41586-018-0041-2
Humanes, A., Beauchamp, E. A., Bythell, J. C., Carl, M. K., Craggs, J. R., Edwards, A. J., et al. (2021). An experimental framework for selectively breeding corals for assisted evolution. Front. Mar. Sci. 8:669995. doi: 10.3389/fmars.2021.669995
Hunt, H. L., and Scheibling, R. E. (1997). Role of early post-settlement mortality in recruitment of benthic marine invertebrates. Mar. Ecol. Prog. Ser. 155, 269–301. doi: 10.3354/meps155269
Iwao, K., Omori, M., Taniguchi, H., and Tamura, M. (2010). Transplanted Acropora tenuis (Dana) spawned first in their life 4 years after culture from eggs. Galaxea, J. Coral Reef Stud. 12, 47–47. doi: 10.3755/galaxea.12.47
Jackson, J. B. C., Donovan, M. K., Cramer, K. L., and Lam, V. V. (2014). Status and Trends of Caribbean Coral Reefs- 1970-2012. Gland: IUCN.
Jones, G. P., Almany, G. R., Russ, G. R., Sale, P. F., Steneck, R. S., van Oppen, M. J. H., et al. (2009). Larval retention and connectivity among populations of corals and reef fishes: history, advances and challenges. Coral Reefs 28, 307–325. doi: 10.1007/s00338-009-0469-9
Jones, G. P., McCormick, M. I., Srinivasan, M., and Eagle, J. V. (2004). Coral decline threatens fish biodiversity in marine reserves. Proc. Natl. Acad. Sci. 109, 8251–8253. doi: 10.1073/pnas.0401277101
Keough, M. J., and Downes, B. J. (1982). Recruitment of marine invertebrates: the role of active larval choices and early mortality. Oecologia 54, 348–352. doi: 10.1007/BF00380003
Lee, E. T., and Wang, J. W. (2003). Statistical Methods for Survival Data Analysis. Hoboken, NJ: Wiley-Interscience.
Levitan, D. R., and Petersen, C. (1995). Sperm limitation in the sea. Trends Ecol. Evol. 10, 228–231.
Lirman, D., and Schopmeyer, S. (2016). Ecological solutions to reef degradation: optimizing coral reef restoration in the Caribbean and Western Atlantic. PeerJ 4, e2597. doi: 10.7717/peerj.2597
McCook, L. J., Ayling, T., Cappo, M., Choat, J. H., Evans, R. D., De Freitas, D. M., et al. (2010). Adaptive management of the Great Barrier Reef: a globally significant demonstration of the benefits of networks of marine reserves. Proc. Natl. Acad. Sci. U.S.A. 107, 18278–18285. doi: 10.1073/pnas.0909335107
McManus, J. W., Reyes, R. B. Jr., and Nanola, C. L. Jr. (1997). Effects of some destructive fishing methods on coral cover and potential rates of recovery. Environ. Manag. 21, 69–78. doi: 10.1007/s002679900006
Mundy, C. (2000). An appraisal of methods used in coral recruitment studies. Coral Reefs 19, 124–131. doi: 10.1007/s003380000081
Nash, K. L., and Graham, N. A. J. (2016). Ecological indicators for coral reef fisheries management. Fish Fish. 17, 1029–1054. doi: 10.1111/faf.12157
Oliver, J., and Babcock, R. C. (1992). Aspects of the fertilization ecology of broadcast spawning corals: sperm dilution effects and in situ measurements of fertilization. Biol. Bull. 183, 409–417. doi: 10.2307/1542017
Omori, M. (2005). Success of mass culture of acropora corals from egg to colony in open water. Coral Reefs 24, 563–563. doi: 10.1007/s00338-005-0030-4
Omori, M. (2019). Coral restoration research and technical developments: what we have learned so far. Mar. Biol. Res. 15, 377–409. doi: 10.1080/17451000.2019.1662050
Pechenik, J. A. (1999). On the advantages and disadvantages of larval stages in benthic marine invertebrate life cycles. Mar. Ecol. Prog. Ser. 177, 269–297. doi: 10.3354/meps177269
Penin, L., Michonneau, F., Baird, A. H., Connolly, S. R., Pratchett, M. S., Kayal, M., et al. (2010). Early post-settlement mortality and the structure of coral assemblages. Mar. Ecol. Prog. Ser. 408, 55–64. doi: 10.3354/meps08554
Petersen, D., Laterveer, M., and Schuhmacher, H. (2005). Innovative substrate tiles to spatially control larval settlement in coral culture. Mar. Biol. 146, 937–942. doi: 10.1007/s00227-004-1503-7
Puill-Stephan, E., Willis, B. L., van Herwerden, L., and van Oppen, M. J. H. (2009). Chimerism in wild adult populations of the broadcast spawning coral Acropora millepora on the Great Barrier Reef. PLoS One 4:e7751. doi: 10.1371/journal.pone.0007751
Randall, C. J., Negri, A. P., Quigley, K. M., Foster, T., Ricardo, G. F., Webster, N. S., et al. (2020). Sexual production of corals for reef restoration in the Anthropocene. Mar. Ecol. Prog. Ser. v 635, 203–232. doi: 10.3354/meps13206
Raymundo, L. J., and Maypa, A. P. (2004). Getting bigger faster: mediation of size-specific mortality via fusion in juvenile coral transplants. Ecol. Appl. 14, 281–295. doi: 10.1890/02-5373
Rinkevich, B. (1995). Restoration strategies for coral reefs damaged by recreational activities: the use of sexual and asexual recruits. Restor. Ecol. 3, 241–251. doi: 10.1111/j.1526-100X.1995.tb00091.x
Rinkevich, B. (2019). The active reef restoration toolbox is a vehicle for coral resilience and adaptation in a changing world. J. Mar. Sci. Eng. 7:201. doi: 10.3390/jmse7070201
Ritson-Williams, R., Arnold, S., Fogarty, N. D., Steneck, R. S., Vermeij, M., and Paul, V. J. (2009). New perspectives on ecological mechanisms affecting coral recruitment on reefs. Smiths. Contrib. Mar. Sci. 38, 437–457. doi: 10.5479/si.01960768.38.437
Roughgarden, J., Iwasa, Y., and Baxter, C. (1985). Demographic theory for an open marine population with space- limited recruitment. Ecology 66, 54–67. doi: 10.2307/1941306
Rumrill, S. S. (1990). Natural mortality of marine invertebrate larvae. Ophelia 32, 163–198. doi: 10.1080/00785236.1990.10422030
Russ, G. R., Miller, K. I., Rizzari, J. R., and Alcala, A. C. (2015). Long-term no-take marine reserve and benthic habitat effects on coral reef fishes. Mar. Ecol. Prog. Ser. 529, 233–248. doi: 10.3354/meps11246
Sammarco, P. W., and Carleton, J. H. (1981). “Damselfish territoriality and coral community structure: reduced grazing, coral recruitment, and effects on coral spat,” in Proceedings of the 4th International Coral Reef Symposium, Manila, 525–536.
Sampayo, E. M., Roff, G., Sims, C. A., Rachello-Dolmen, P. G., and Pandolfi, J. M. (2020). Patch size drives settlement success and spatial distribution of coral larvae under space limitation. Coral Reefs 39, 387–396. doi: 10.1007/s00338-020-01901-1
Schweinsberg, M., Weiss, L. C., Striewski, S., Tollrian, R., and Lampert, K. P. (2015). More than one genotype: how common is intracolonial genetic variability in scleractinian corals? Mol. Ecol. 24, 2673–2685. doi: 10.1111/mec.13200
Shaish, L., Levy, G., Katzir, G., and Rinkevich, B. (2010). Employing a highly fragmented, weedy coral species in reef restoration. Ecol. Eng. 36, 1424–1432. doi: 10.1016/j.ecoleng.2010.06.022
Suzuki, G., Arakaki, S., Suzuki, K., Iehisa, Y., and Hayashibara, T. (2012). What is the optimal density of larval seeding in Acropora corals? Fish. Sci. 78, 801–808. doi: 10.1007/s12562-012-0504-6
Teo, A., and Todd, P. A. (2018). Simulating the effects of colony density and intercolonial distance on fertilisation success in broadcast spawning scleractinian corals. Coral Reefs 37, 891–900. doi: 10.1007/s00338-018-1715-9
Thorson, G. (1950). Reproductive and larval ecology or marine bottom invertebrates. Biol. Rev. 25, 1–45. doi: 10.1111/j.1469-185X.1950.tb00585.x
van Oppen, M. J. H., Gates, R. D., Blackall, L. L., Cantin, N., Chakravarti, L. J., Chan, W. Y., et al. (2017). Shifting paradigms in restoration of the world’s coral reefs. Glob. Change Biol. 23, 3437–3448. doi: 10.1111/gcb.13647
Vermeij, M. J. A., and Sandin, S. A. (2008). Density-dependent settlement and mortality structure the earliest life phases of a coral population. Ecology 89, 1994–2004. doi: 10.1890/07-1296.1
Wallace, C. C. (1985). Reproduction, recruitment and fragmentation in nine sympatric species of the coral genus Acropora. Mar. Biol. 88, 217–233. doi: 10.1007/BF00392585
Ward, S., and Harrison, P. L. (1997). “The effects of elevated nutrient levels on settlement of coral larvae during the ENCORE experiment, Great Barrier Reef, Australia,” in Proceedings of the 8th Int’l Coral Reef Symposium, eds H. A. Lessios and I. G. Macintyre (Panama: Smithsonian Tropical Research Institute), 891–896. doi: 10.1016/s0025-326x(00)00181-8
Wilkinson, C. (2008). Status of Coral Reefs of the World: 2008. Townsville: Network and Reef and Rainforest Research Center.
Willis, B. L., Babcock, R. C., Harrison, P. L., Oliver, J. K., and Wallace, C. C. (1985). “Patterns in the mass spawning of corals on the Great Barrier Reef from 1981 to 1984,” in Proceedings of the 5th International Coral Reef Congress, Tahiti, 343–348.
Willis, B. L., Babcock, R. C., Harrison, P. L., and Wallace, C. C. (1997). Experimental hybridization and breeding incompatibilities within the mating systems of mass spawning reef corals. Coral Reefs 16, S53–S65.
Wilson, J., and Harrison, P. (2005). Post-settlement mortality and growth of newly settled reef corals in a subtropical environment. Coral Reefs 24, 418–421. doi: 10.1007/s00338-005-0033-1
Keywords: reef restoration, sexual reproduction, Acropora tenuis, larval settlement, coral recruitment, coral growth, survivorship, fish assemblages
Citation: Harrison PL, dela Cruz DW, Cameron KA and Cabaitan PC (2021) Increased Coral Larval Supply Enhances Recruitment for Coral and Fish Habitat Restoration. Front. Mar. Sci. 8:750210. doi: 10.3389/fmars.2021.750210
Received: 30 July 2021; Accepted: 09 November 2021;
Published: 01 December 2021.
Edited by:
Iliana B. Baums, The Pennsylvania State University (PSU), United StatesReviewed by:
Zac H. Forsman, University of Hawai’i at Mānoa, United StatesCopyright © 2021 Harrison, dela Cruz, Cameron and Cabaitan. This is an open-access article distributed under the terms of the Creative Commons Attribution License (CC BY). The use, distribution or reproduction in other forums is permitted, provided the original author(s) and the copyright owner(s) are credited and that the original publication in this journal is cited, in accordance with accepted academic practice. No use, distribution or reproduction is permitted which does not comply with these terms.
*Correspondence: Peter L. Harrison, cGV0ZXIuaGFycmlzb25Ac2N1LmVkdS5hdQ==
Disclaimer: All claims expressed in this article are solely those of the authors and do not necessarily represent those of their affiliated organizations, or those of the publisher, the editors and the reviewers. Any product that may be evaluated in this article or claim that may be made by its manufacturer is not guaranteed or endorsed by the publisher.
Research integrity at Frontiers
Learn more about the work of our research integrity team to safeguard the quality of each article we publish.