- 1International Research Center for Marine Biosciences at Shanghai Ocean University, Ministry of Science and Technology, Shanghai, China
- 2Key Laboratory of Exploration and Utilization of Aquatic Genetic Resources, Ministry of Education, Shanghai Ocean University, Shanghai, China
- 3Shanghai Engineering Research Center of Hadal Science and Technology, College of Marine Sciences, Shanghai Ocean University, Shanghai, China
- 4Department F.-A. Forel for Environmental and Aquatic Sciences, Environmental Biogeochemistry and Ecotoxicology, Faculty of Sciences, Earth and Environment Sciences, University of Geneva, Geneva, Switzerland
- 5Guangxi Key Laboratory of Aquatic Genetic Breeding and Healthy Aquaculture, Guangxi Academy of Fisheries Sciences, Nanning, China
- 6Department of Marine Biology, Institute of Biological Sciences, University of Rostock, Rostock, Germany
Marine hypoxia caused by nutrient enrichment in coastal waters has become a global problem for decades, especially diel-cycling hypoxia that occurs frequently in the summer season. On the contrary, sudden rainstorms, and freshwater discharge make salinity in estuarine and coastal ecosystems variable, which often occurs with hypoxia. We found mass mortality of the Hong Kong oyster Crassostrea hongkongensis in the field where hypoxia and salinity fluctuation co-occur in the summer season during the past several years. To investigate the effects of diel-cycling hypoxia and salinity changes on the hemocyte immune function of C. hongkongensis, oysters were exposed to a combined effect of two dissolved oxygen (DO) concentrations (24 h normal oxygen 6 mg/L, 12 h normal oxygen 6 mg/L, and 12 h hypoxia 2 mg/L) and three salinities (10, 25, and 35‰) for 14 days. Subsequently, all treatments were restored to constant normal oxygen (6 mg/L) and salinity under 25‰ for 3 days to study the recovery of hemocyte immune function from the combined stress. Hemocyte parameters were analyzed by flow cytometry, including hemocyte mortality (HM), total hemocyte count (THC), phagocytosis (PHA), esterase (EST) activity, reactive oxygen species (ROS), lysosomal content (LYSO), and mitochondrial number (MN). The experimental results showed that diel-cycling hypoxia and salinity changes have obvious interactive effects on various immune parameters. In detail, diel-cycling hypoxia and decreases in salinity led to increased HM, and low salinity caused heavier impacts. In addition, low salinity, and diel-cycling hypoxia also led to decreases in LYSO, EST, and THC, while the decrease of PHA only occurs in the early stage. On the contrary, ROS production increased significantly under low salinity and hypoxic conditions. After 3-day recovery, THC, PHA, EST, LYSO, and MN were basically restored to normal, while HM and ROS were still significantly affected by diel-cycling hypoxia and salinity change, indicating that the combined stress of diel-cycling hypoxia and salinity changes had latent effects on the immune function of C. hongkongensis. Our results highlight that diel-cycling hypoxia and salinity change may impair the health and survival of the Hong Kong oyster C. hongkongensis and may be the key factors for the mass mortality of this oyster in the field.
Highlights
- The combined effects of salinity change and hypoxia on hemocyte immune parameters of oysters with a shot-term recovery were investigated.
- Diel fluctuating hypoxia and salinity change had weak impact on the hemocyte immunity of oysters compared with constant exposure, apart from anti-oxidant stress and death of hemocytes which was difficult to recover.
- Oysters had higher hemocyte mortality and reactive oxygen species contents with lower total hemolymph count, esterase activity, and lysosome content under diel-cycling hypoxia and salinity change, compared with constant exposure.
- Oysters showed pretty recovery to diel-cycling hypoxia and water salinity change after 3-day recovery period.
Introduction
Given the global climate change, increasingly frequent rains, and typhoons have occurred in recent years, and the salinity in seawater is incrementally in a periodical change (IPCC, 2014). Therefore, the fauna in coastal and estuarine waters, like bivalves which have weak migration, are facing survival challenges from severe and frequent salinity change (Booij, 2005). Salinity in coastal and estuarine waters always varies from near-zero low salinity to high salinity depending on the amount of freshwater input (Montagna et al., 2018). Salinity in the oyster Crassostrea hongkongensis culture waters changes between 10 and 35‰ because of freshwater input and tides (Liu and Wang, 2013). Steady salinity is key for the survival of bivalves (McCarty et al., 2020), and environmental salinity change can cause internal changes in physical metabolism, ion concentrations, and enzymes because of osmotic stresses (Shekhar et al., 2013; Carregosa et al., 2014a,b). Most invertebrates like mollusks are osmoconformers, so that the osmolarity of the extra- and intracellular fluids changes according to the environmental salinity (Berger and Kharazova, 1997). Salinity change can affect bivalve hemocytes, for both immune and antioxidant functions. Study on the effect of salinity change and acidification on the thick shell mussels Mytilus coruscus showed that lower salinity shaved the immunity of hemocyte obviously rather than higher salinity (Wu et al., 2018b). Besides, salinity change could decrease phagocytosis (PHA) and induce more hemocyte mortality (HM) in the Pacific oyster Crassostrea gigas (Gagnaire et al., 2006). As for oysters, salinity change in combination with thermal stress can synergistically impact innate immune response and respiration in the Sydney rock oyster Saccostrea glomerata (Ertl et al., 2019).
Hypoxia, generally defined as dissolved oxygen (DO) <2.8 mg/L (Wu, 2002), usually appears at the bottom of coasts and estuaries, where it is closely related to the biological respiration and eutrophication, especially at night (Diaz and Rosenberg, 2008). Furthermore, anthropogenic activity impacts coasts and estuaries, causing a high frequency of eutrophication (Howarth et al., 2011) and forming hypoxic waters (Diaz and Rosenberg, 2008; Doney, 2010). With the change of tides, the DO range varies from 0.16 to 15.28 mg/L (0.8–87 kPa) (Legrand et al., 2018). Low DO can cause mortality, high migration, high risk of predation and infection, change of food resources, and decrease of immune responses in fish and aquatic invertebrates (Naqvi et al., 2000; Cheng et al., 2004; Yu et al., 2010; Vosloo et al., 2013); especially when DO is <2 mg/L, the immune function and growth can be impeded in aquatic fauna significantly, and individual mortality may increase (Diaz and Rosenberg, 2008).
The Hong Kong oyster C. hongkongensis grows mainly in the south of China with substantial nutrition and economic benefit and occupies an important place in the aquaculture industry of China (Qin et al., 2018). The Hong Kong oyster is also a vital species in the nearshore environmental health monitor, for the wide distribution, long lifecycle, large shape, and fixed growth habits (Foy et al., 2001; Guo et al., 2015). Its habitat is full of variety and extremely challenges the homeostasis (Pequeux, 1995; Przeslawski et al., 2015), but large amounts of researches focus on the effects of single environmental factors. Therefore, it is necessary to understand the complex effects of multiple stressors (Breitburg et al., 2015; Stevens and Gobler, 2018). As for the complex effects, the results in marine invertebrates mostly showed synergistic effects rather than additive or antagonistic effects (Huang et al., 2018b). For instance, the synergistic effect from salinity and temperature occupies 58.3% in the combined effect studies (Przeslawski et al., 2015). Therefore, exposure experiments with single factors cannot deduce the results of multiple factors studies, especially in the experiment on environmental and climatic change. At present, the research of combined environmental stress on bivalves is mostly focused on ocean acidification with other environmental factors (Sui et al., 2017; Wu et al., 2018b). The DO in the coastal intertidal zone alters easily with salinity change, and hypoxia often occurs at night, but the studies about the interaction between salinity and diel-cycling hypoxia on marine organisms are limited, especially on the marine mollusks living in such waters (Przeslawski et al., 2015). In this study, we evaluated the combined effects of salinity change and diel-cycling hypoxia on the immune parameters of the Hong Kong oyster C. hongkongensis, so as to more truly reflect the changes in the immune response of cultured shellfish. Hemocyte plays an important role in bivalve immune responses (Wang et al., 2012, 2014; Gomes et al., 2013), including swallowing and killing pathogens and antigens (Donaghy et al., 2009), while the previous study indicated that there were plenty of biological and non-biological factors that could change the hemocyte-dependent defense mechanisms in bivalves and decrease the immune functions (Matozzo et al., 2007).
We studied the changes in various immune parameters of Hong Kong oysters under the conditions of diel-cycling hypoxia and salinity change, and flow cytometry was used to determine the hemocyte parameters. We aim to clarify how the internal environment of bivalves responds to the stress of reduced DO and fluctuations in salinity in the coastal environment. This study provides reference information for solving actual shellfish aquaculture production and for assessing the health status of oysters under multiple environmental stressors.
Materials and Methods
Experimental Animals
Natural seeding of Hong Kong oyster C. hongkongensis (shell length 7.6 ± 0.8 cm and wet weight 74 ± 5.1 g) was collected from Shajing, Maowei Sea of Qinzhou, Beihai City, Guangxi Province, China (108° 50′45″-109° 47′28″ E, 20° 26′26″-21° 55′34″ N). Oysters with no shell damage and with even size were selected. The epibionts on the shell were softly removed, and then, these oysters were acclimated to laboratory conditions for 14 days. During the acclimation period, the condition was mimicking the sampling site at sampling: pH 8.1–8.2, temperature 24–25°C, and salinity 25.0‰. To reduce the effects of waste from oyster metabolism, we used a 240 L/h recirculating aquarium system. During the whole experimental period, including acclimation and experimentation, the oysters were fed with the microalgae Isochrysis galbana (concentration: 2.5 × 105 cells/mL) two times a day.
Experimental Design
To study the combined effect of salinity change and hypoxia on C. hongkongensis, we selected 10, 25, and 35‰ for low salinity, normal salinity, and high salinity, respectively. At each salinity, the normal DO group of 24 h 6 mg/L and the diel-cycling hypoxia group (12 h 2 mg/L from 20: 00 to 8: 00 and 12 h 6 mg/L from 08: 00 to 20: 00) were set, totally six treatments. The control group was 24 h of normal DO (6 mg/L) and normal salinity (25‰). A total of 360 oysters were randomly divided into six treatments with three replicates (tanks, 20 oysters per tank) per treatment. Same as the acclimation period, water was controlled under controlled conditions (temperature 24–25°C, pH 8.1) (Qin et al., 2018) with 12 h of light and 12 h of darkness except for salinity and DO in the aquaculture system, to analyze the physiological effects of C. hongkongensis. During the experiment, temperature, DO, pH, and salinity were measured by a multiparameter instrument (5200A, YSI Inc., Ohio, USA), and the results were shown in Table 1. During a 17-day experimental period, including a 14-day exposure period and a 3-day recovery period, hemocyte parameters were measured on days 1, 7, 14, and 17. Salinity and DO of the culture system were restored to normal (salinity 25‰ and DO 6 mg/L) after the 14-day exposure period. During the whole experimental period, hypoxia conditions were attained by ventilating nitrogen to the seawater. To obtain the hypoxic conditions, nitrogen was passed through the O2 regulator (Loligo Systems Inc., Viborg, Denmark) into the aquarium system. With the help of the DO control system connected with computer software, the DO in the water was controlled by aerating nitrogen (Sui et al., 2015). In the meantime, different salinity conditions (10, 25, and 35‰) were obtained by diluting seawater with freshwater (Wu et al., 2018b).
Hemolymph Collection
A fine slit was opened using a metal shell opener, and the hemolymph was collected from the adductor muscle with a sterile syringe equipped with 22G needles (2 mL). Three oysters were randomly taken from each tank for hemolymph collection, and three samples were pooled to reduce individual differences; the hemolymph was immediately stored on the ice to ensure the activity of hemocytes.
Flow Cytometry
BD Accuri TM C6 flow cytometer (BD Biosciences, New York, NY, USA) was used to analyze the hemocyte parameters. The cytometer was equipped with air-cooled argon capable of firing 448 nm lasers. Before the test, the FSC threshold level was set at 4,200 to eliminate cell debris and the effects of other impurities. The fluorescent channels were set according to the corresponding fluorescent markers. The HM was tested at FL2, while the other cell parameters were measured at FL1 (Wang et al., 2012; Gajbhiye and Khandeparker, 2017). Each hemolymph sample analysis included a total of 20,000 events, and the speed was adjusted as a total event <300/s. Data were analyzed by BD CellQuest TM Pro (BD Biosciences, USA).
Hemocyte Mortality
Hemocyte mortality was tested using propidium iodide (PI, 1.0 mg/mL, Sigma Aldrich, St. Louis, Missouri, USA) (Gajbhiye and Khandeparker, 2017). Briefly, 400 μL hemolymph of oysters and 10 μL of PI were mixed and incubated in darkness at room temperature for 30 min. The HM was calculated based on the percentage of PI fluorescence relative to the total hemocyte counts (THCs).
Total Hemocyte Counts
Prior to the experiment, 900 μL of dimethyl sulfoxide (DMSO) solution and 100 μL of SYBR Green solution were mixed in advance. The mixture was diluted 100 times after uniform oscillations. Before the detection, 44 μL of diluted SYBR Green solution was added to 400 μL of hemolymph and incubated at room temperature in the dark for 30 min (Donaghy et al., 2012).
Phagocytosis
Fluorospheres® carboxylate-modified microspheres (diameter: 10 μm) was used to measure PHA; 400 μL of hemolymph was incubated with 10 μl of a 1/10 dilution of Fluorospheres® carboxylate-modified microspheres (diameter 1.0 μm, yellow–green fluorescent, Invitrogen, Carlsbad, California, USA) for 1 h in the dark at ambient temperature (Huang et al., 2016). The PHA was measured by the percentage of cells that had engulfed at least three fluorescent beads relative to all cells (Gagnaire et al., 2006).
Esterase
Esterase (EST) was evaluated using fluorescein diacetate (FDA, Sigma, St. Louis, Missouri, USA). FDA (400 μM/L) was prepared by diluting a stock solution (1/10) with sterile seawater and stored at −20°C. Briefly, 400 μL hemolymph with 2 μL FDA was incubated at room temperature and darkness for 15 min (Huang et al., 2016). EST was measured as the percentage of fluorescent cells relative to all cells (Gagnaire et al., 2008).
Reactive Oxygen Species
Reactive oxygen species (ROS) was assessed by 2′7′-dichlorofluorescein diacetate (DCFH-DA, Sigma, St. Louis, Missouri, USA). The stock solution (10 mM/L) of quantitative DCFH-DA dissolved in DMSO reagent was diluted with filtered sterile seawater (1/10), and then, 4 μL of DCFH-DA was added to 400 μL of hemolymph at room temperature in the dark and incubated for 15 min for ROS detection. ROS was defined on the basis of fluorescent cells among all cells and expressed in arbitrary units (AU) (Delaporte et al., 2003).
Lysosomal Content
Intracellular lysosomal content (LYSO) was measured by a commercial kit (Lysotracker® Yellowhck-123, 1 mM in DMSO, Invitrogen, Carlsbad, California, USA) (Gagnaire et al., 2008). Briefly, 1 μL of LysoTracker solution was added to 400 μL of hemolymph and incubated in darkness at room temperature for 2 h. LYSO was expressed as the mean intensity of Lyso-Tracker fluorescence exhibited by all the hemocytes in arbitrary units (AU) (Gagnaire et al., 2008).
Mitochondrial Number
The MTG solution (50 nM) was mixed with 400 μL of hemolymph, and the mixture was slowly shaken until the mixture was evenly mixed. The solution was incubated at room temperature under dark conditions for 30 min (Ciacci et al., 2012). Mitochondrial number (MN) was expressed as the mean fluorescence intensity compared with the control group.
Condition Index
The calculation method of condition index (CI) was based on the previous article (Norman, 2005). In different treatments, 10 oysters were selected to measure shell length, width, height, and weight. The following equation was used for calculating the CI (Maguire et al., 1999).
Data Analysis
The data were mean ± standard deviation, and Kolmogorov–Smirnov test and Levene's test were used to perform the normal distribution and homogeneous ANOVA. The SPSS 21 (SPSS Inc., Chicago, IL, USA) software was used to conduct a two-way ANOVA on DO, salinity fluctuations, and their interactions in the water body. A three-factor ANOVA was used to evaluate the effects of DO, salinity, time, and their interactions. In addition, a one-way ANOVA using Tukey's HSD test was used to evaluate the impact of salinity changes at each fixed DO. The Student's t-test was used to analyze the influence of different DO levels on the biochemical indicators under each fixed salinity condition. The difference was significant with p < 0.05. Origin 2019 was used to perform the principal component analysis (PCA) on all biochemical parameters during the exposure period and recovery period, respectively.
Results
Condition Index
During the experiment, DO had no significant effect on the CI. CI was highest (45.1) in 25‰ salinity and lowest (43.1) in 10‰ salinity. Compared with 25‰ and 35‰ conditions, the CI was significantly lower in 10‰ salinity (Table 2).
Immune Parameters
The HM was significantly affected by time, DO, and salinity and all their interactions (p < 0.01) (Table 3). Low salinity (10‰) increased HM after the exposure of 1 day; HM after the 14-day exposure was even higher than the 1-day exposure, while high salinity (35‰) led to no obvious change on HM during the whole experimental period (Figure 1). Besides, hypoxia at night (2 mg/L) intensified HM during the exposure period after 7 days. Even under normal condition of salinity (25‰), hypoxia at night still increased HM (Figure 1). After the 3-day recovery, HM was still affected by the change of salinity and DO and their interaction significantly (p < 0.01) (Table 4). Although HM in the low salinity group and hypoxia compared with the normal salinity group tended to decrease, which was the same with the value in the early exposure period (within 7 days), HM was still higher than the normal group (Figure 1).
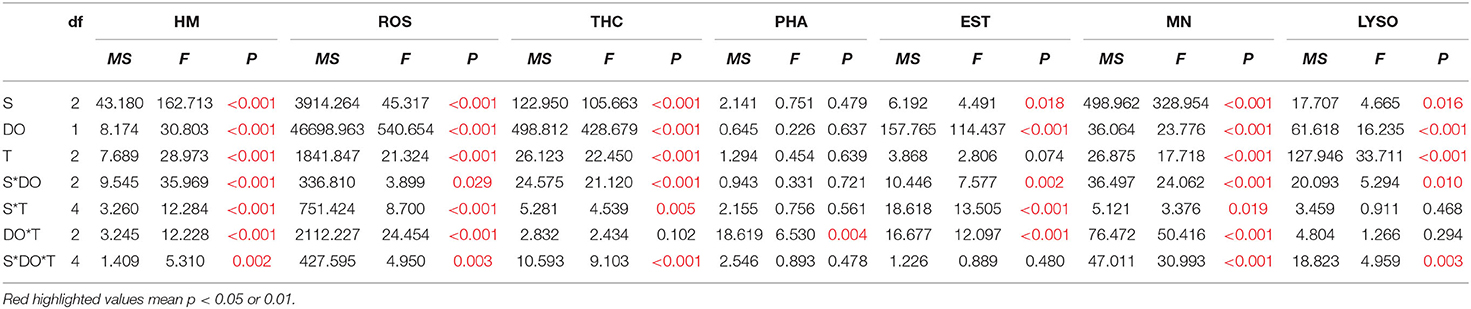
Table 3. Three-way ANOVA summary on the effects of salinity (S), dissolved oxygen (DO), and time (T) on hemocyte mortality (HM), reactive oxygen species (ROS), total hemocyte count (THC), phagocytosis (PHA), esterase (EST), mitochondrial number (MN), and lysosomal content (LYSO) of C. hongkongensis in experiments of the exposure period.
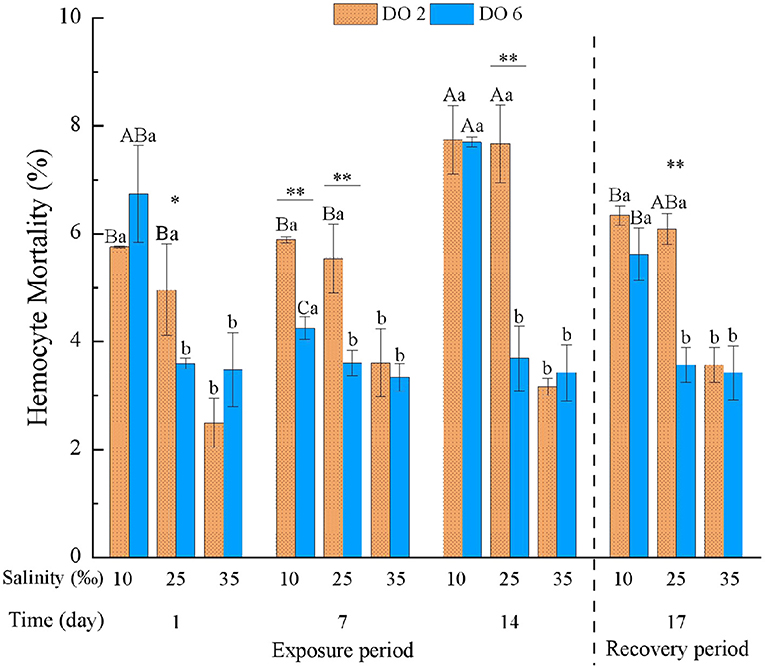
Figure 1. Hemocyte mortality (HM) of Crassostrea hongkongensis exposed to six combinations of salinity (10, 25, and 35‰) and DO (2 and 6 mg/L) at 1, 7, and 14 days during the exposure period and 3 days for the recovery period. Different capital letters indicate significant differences among time points within each salinity level in hypoxia level (2 mg/L) or control group (6 mg/L) (p < 0.05). Different small letters indicate significant differences between salinity within each time point in salinity level in hypoxia level (2 mg/L) or control group (6 mg/L) (p < 0.05). Asterisk indicates significant differences between DO within each time point and fixed salinity treatment, in which * represents significant difference (p < 0.05) and ** represents highly significant difference (p < 0.01).

Table 4. Two-way ANOVA summary on effects of salinity (S), dissolved oxygen (DO), and time (T) on hemocyte mortality (HM), reactive oxygen species (ROS), total hemocyte count (THC), phagocytosis (PHA), esterase (EST), mitochondrial number (MN), and lysosomal content (LYSO) of C. hongkongensis in experiments of the recovery period.
The ROS was significantly affected by time, DO, and salinity and all their interactions (Table 3). At day 1 of exposure, hypoxia at night and low salinity both induced the ROS of hemocyte, in which hypoxia caused the effects more obvious. Meanwhile, hypoxia at night induced ROS during the whole exposure period, and it intensified the induction of ROS at low salinity (Figure 2). Low salinity induced ROS, while high salinity caused no significant change on ROS (Figure 2). After the 3-day recovery, ROS was still affected by the change of salinity and DO significantly (p < 0.01) apart from the interactions (Table 4), in which ROS in the hypoxia group was still higher than the normal group although ROS showed a decreasing tendency; ROS in the low salinity group was still significantly higher compared with the normal and higher salinity group (Figure 2).
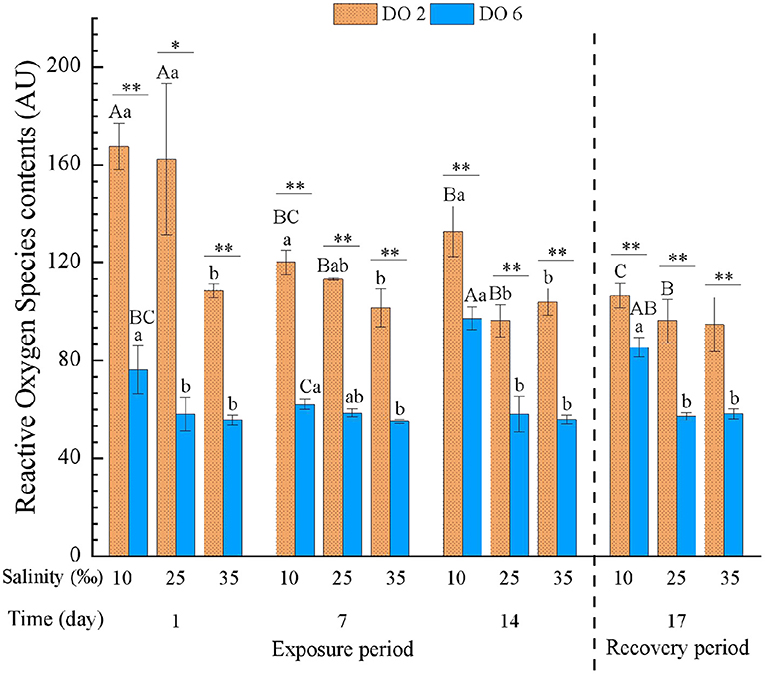
Figure 2. Reactive oxygen species (ROS) of C. hongkongensis exposed to six combinations of salinity (10, 25, and 35‰) and DO (2 and 6 mg/L) at 1, 7, and 14 days during the exposure period and 3 days for the recovery period. Different capital letters indicate significant differences among time points within each salinity level in hypoxia level (2 mg/L) or control group (6 mg/L) (p < 0.05). Different small letters indicate significant differences between salinity within each time point in salinity level in hypoxia level (2 mg/L) or control group (6 mg/L) (p < 0.05). Asterisk indicates significant differences between DO within each time point and fixed salinity treatment, in which * represents significant difference (p < 0.05) and ** represents highly significant difference (p < 0.01).
Apart from the interaction between DO and time, THC was significantly affected by time, DO, and salinity and all their interactions (Table 3). Hypoxia at night and the variety of salinity all decreased THC, and the hypoxia showed a more intensive effect than salinity, in which only low salinity deduced THC significantly at day 1 of exposure (Figure 3). Besides, the effects were strengthened under interactions. After the 3-day recovery, THC was still affected by the change of salinity and DO and their interaction significantly (p < 0.05) (Table 4). THC of most groups rebounded to the normal level, and THC in high salinity with hypoxia treatment showed higher values than any other hypoxia groups, in which there was no significant difference between hypoxia and no-hypoxia in the high salinity group (Figure 3).
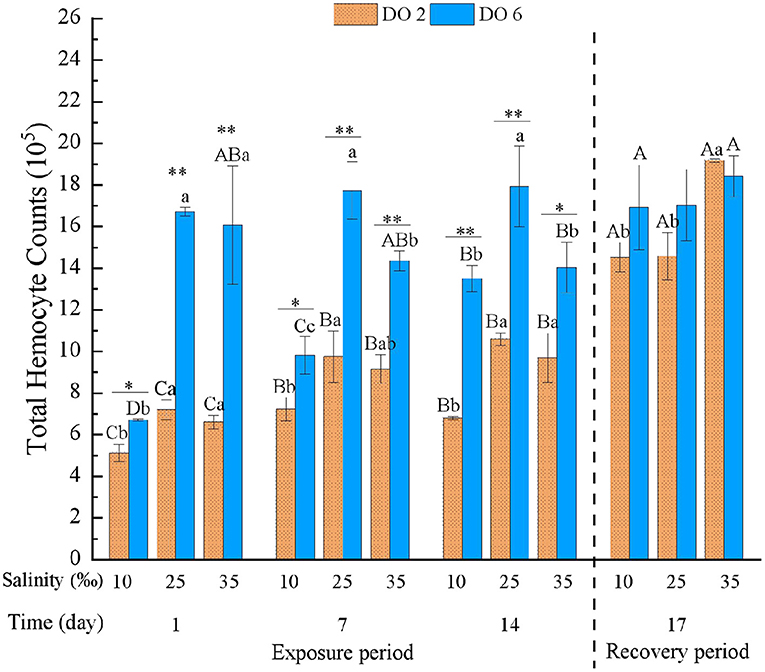
Figure 3. Total hemocyte count (THC) of C. hongkongensis exposed to six combinations of salinity (10, 25, and 35‰) and DO (2 and 6 mg/L) at 1, 7, and 14 days during the exposure period and 3 days for the recovery period. Different capital letters indicate significant differences among time points within each salinity level in hypoxia level (2 mg/L) or control group (6 mg/L) (p < 0.05). Different small letters indicate significant differences between salinity within each time point in salinity level in hypoxia level (2 mg/L) or control group (6 mg/L) (p < 0.05). Asterisk indicates significant differences between DO within each time point and fixed salinity treatment, in which * represents significant difference (p < 0.05) and ** represents highly significant difference (p < 0.01).
Phagocytosis was only affected by the interaction between DO and time (Table 3). For the first day of exposure, hypoxia at night had the potential to induce PHA while PHA then returned to normal status, in which PHA was not influenced significantly (Figure 4). Also, no significant effects turned out during the recovery period (Table 4).
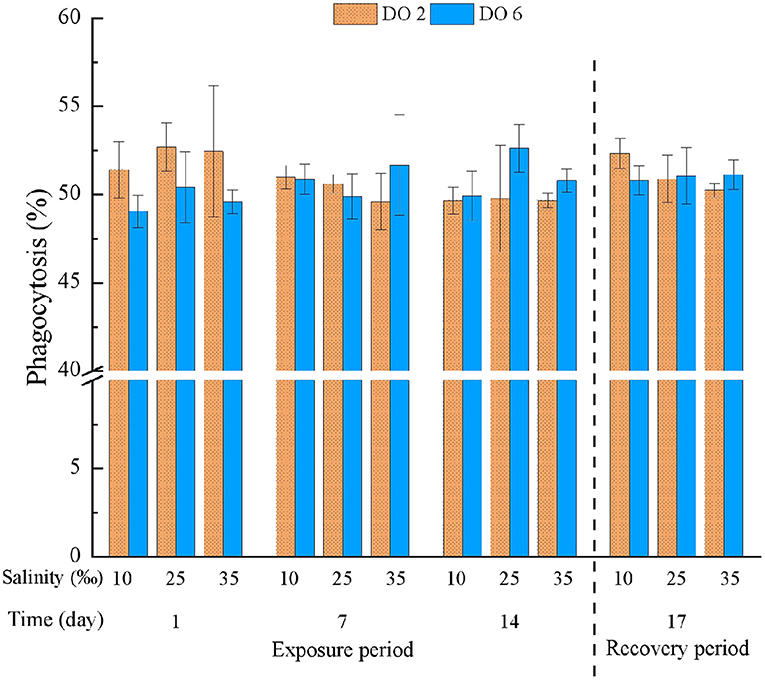
Figure 4. Phagocytosis (PHA) of C. hongkongensis exposed to six combinations of salinity (10, 25, and 35‰) and DO (2 and 6 mg/L) at 1, 7, and 14 days during the exposure period and 3 days for the recovery period. Different capital letters indicate significant differences among time points within each salinity level in hypoxia level (2 mg/L) or control group (6 mg/L) (p < 0.05). Different small letters indicate significant differences between salinity within each time point in salinity level in hypoxia level (2 mg/L) or control group (6 mg/L) (p < 0.05).
Esterase was significantly affected by salinity and DO, their interactions, and each of them with time (Table 3). In the high salinity group, hypoxia at night decreased EST after the 1-day exposure, and high salinity deduced EST along with the exposure time. EST in the low salinity group with hypoxia was always significantly lower than the normal group after the 1-day exposure, while EST showed no difference if DO were normal (Figure 5). Hypoxia at night caused more intensive alteration on EST than salinity change, apart from high salinity at the end of the exposure period which caused decreases in two treatment groups. After the 3-day recovery, there was no significant effect on EST by the change of salinity and DO and their interaction (p > 0.05) (Table 4), and EST in all treatment groups were restored with no obvious difference (Figure 5).
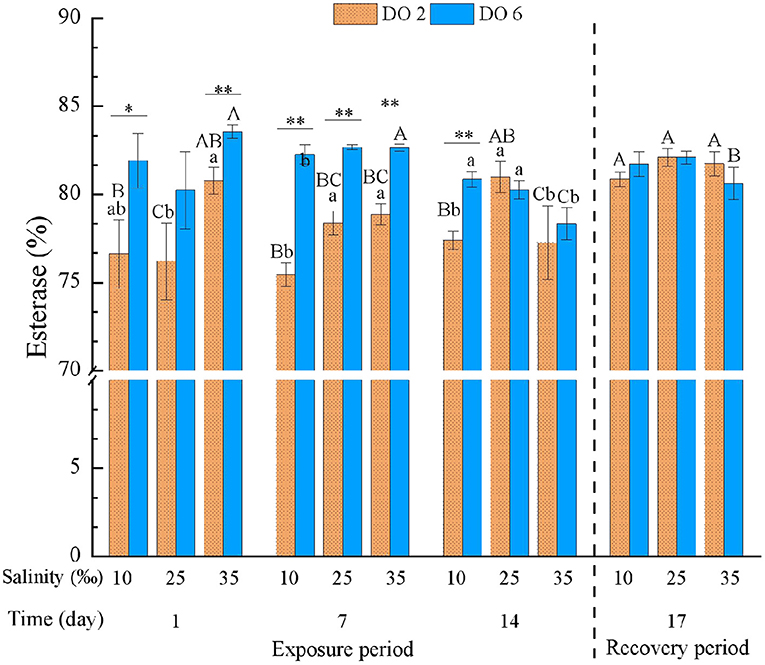
Figure 5. Esterase (EST) of C. hongkongensis exposed to six combinations of salinity (10, 25, and 35‰) and DO (2 and 6 mg/L) at 1, 7, and 14 days during the exposure period and 3 days for the recovery period. Different capital letters indicate significant differences among time points within each salinity level in hypoxia level (2 mg/L) or control group (6 mg/L) (p < 0.05). Different small letters indicate significant differences between salinity within each time point in salinity level in hypoxia level (2 mg/L) or control group (6 mg/L) (p < 0.05). Asterisk indicates significant differences between DO within each time point and fixed salinity treatment, in which * represents significant difference (p < 0.05) and ** represents highly significant difference (p < 0.01).
Mitochondrial number was significantly affected by time, DO, and salinity and all their interactions obviously (p < 0.01) (Table 3). During the exposure period, high salinity decreased MN obviously after the 1-day exposure, while low salinity increased MN at the end of the exposure period (14 days) (Figure 6). In the high salinity group, hypoxia induced MN gradually along with the exposure time, while MN decreased in the normal DO water (Figure 6), and there were significant differences between the two groups, showing antagonism (p < 0.01). After the 3-day recovery, there was no significant effect on MN by the change of salinity and DO and their interaction (p > 0.05) (Table 4), and MN in all treatment groups were restored with no obvious difference (Figure 6).
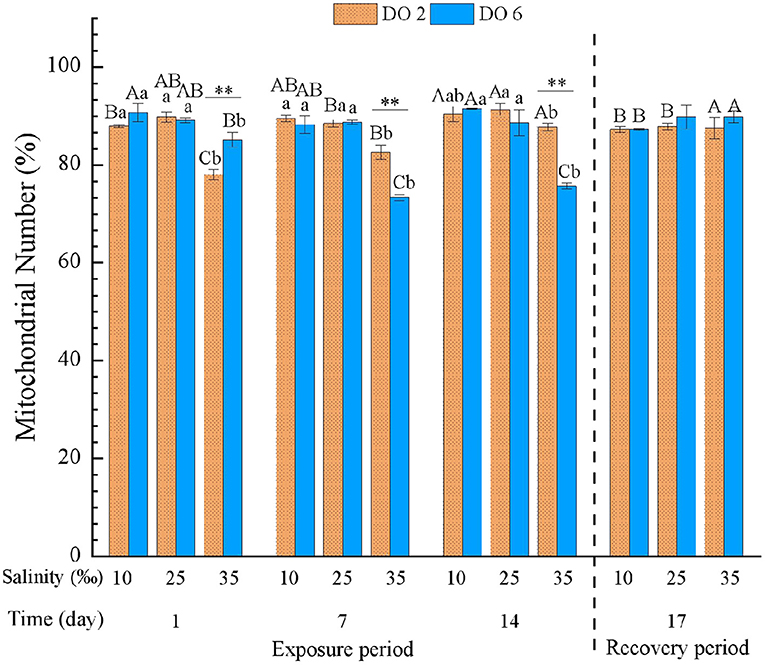
Figure 6. Mitochondrial number (MN) of C. hongkongensis exposed to six combinations of salinity (10, 25, and 35‰) and DO (2 and 6 mg/L) at 1, 7 and 14 days during the exposure period and 3 days for the recovery period. Different capital letters indicate significant differences among time points within each salinity level in hypoxia level (2 mg/L) or control group (6 mg/L) (p < 0.05). Different small letters indicate significant differences between salinity within each time point in salinity level in hypoxia level (2 mg/L) or control group (6 mg/L) (p < 0.05).
Apart from the interactions between salinity and time and between DO and time, the LYSO was significantly affected by time, DO and salinity, and other interactions (Table 3). With the exposure time, LYSO in all treatment groups decreased significantly (p < 0.01) (Figure 7). The change of salinity had no significant effects on LYSO at the first 7 days, apart from that the interaction between hypoxia and low salinity deduced LYSO significantly. However, LYSO in all treatment groups (only salinity change, only hypoxia at night, and salinity change & hypoxia) decreased significantly (p < 0.01), while no obvious intensified interaction was found between salinity and DO. After the 3-day recovery, there was no significant effect on LYSO by the change of salinity and DO and their interaction (p > 0.05) (Table 4), and LYSO in all treatment groups was restored to normal condition (Figure 7).
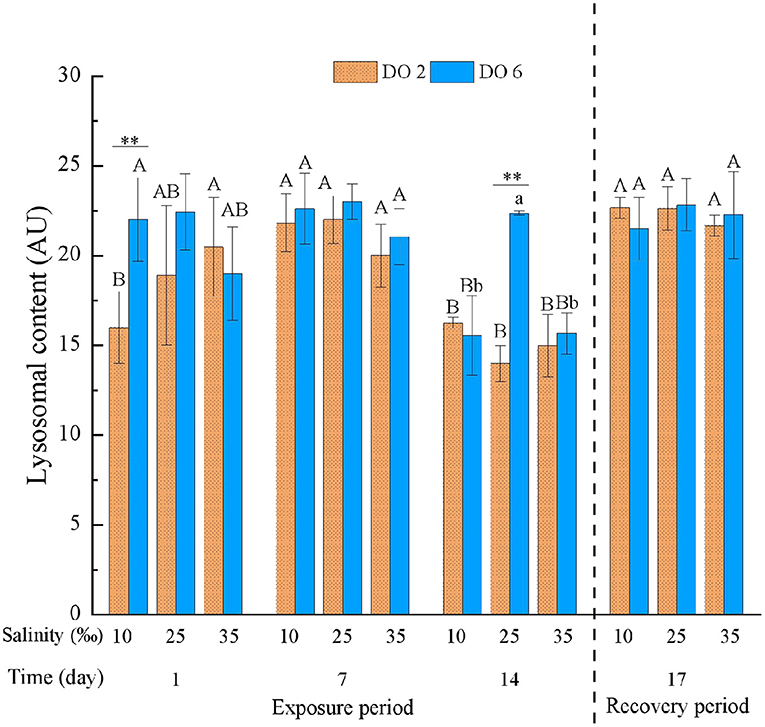
Figure 7. Lysosomal content (LYSO) of C. hongkongensis exposed to six combinations of salinity (10, 25, and 35‰) and DO (2 and 6 mg/L) at 1, 7, and 14 days during the exposure period and 3 days for the recovery period. Different capital letters indicate significant differences among time points within each salinity level in hypoxia level (2 mg/L) or control group (6 mg/L) (p < 0.05). Different small letters indicate significant differences between salinity within each time point in salinity level in hypoxia level (2 mg/L) or control group (6 mg/L) (p < 0.05).
Principal Component Analysis
The PCA revealed that 64.89 and 77.63% of overall variance were explicated for the exposure period and recovery period, respectively (Figure 8). During the exposure period, PC1 explained 39.17% of overall variance, showing the most significant result referred to the separation between hypoxia at night and normal DO treatment, in which the diel-cycling hypoxia increased MN, HM, and ROS with a bit induction on PHA at the meantime; while THC, EST, and LYSO were decreased (Figure 8A). Changes in NM, HM, and ROS were negatively correlated with the changes in THC, EST, and LYSO. PC2 explained 25.72% of overall variance, showing the most significant result referred to the separation between high salinity and low salinity treatment, in which low salinity caused higher MN and HM more easily (Figure 8A). During the recovery period, PC1 explained 39.23% of overall variance, showing the most significant result referred to the separation between low salinity treatments and normal salinity group, in which only HM and ROS still showed some differences under low salinity conditions after the short-time recovery. PC2 explained 38.40% of overall variance, showing the most significant result referred to the separation between the combined hypoxia and low salinity treatment and the normal group, in which HM and ROS still showed some differences in diel-cycling hypoxia and low salinity conditions after the short-time recovery (Figure 8B).
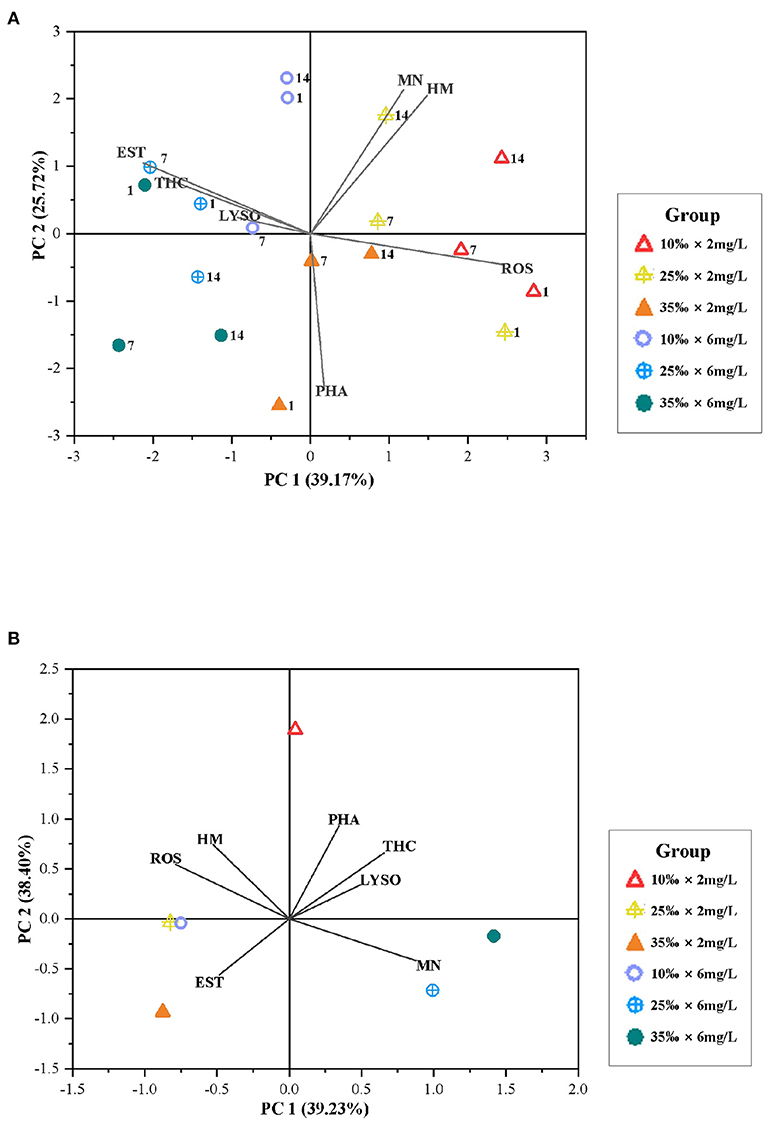
Figure 8. (A) Biplot originating from PCA integrating all measured variables (HM, PHA, EST, ROS, THC, LYSO, and MN) and three time points (days: 1, 7, and 14) at six different treatments during the exposure period. (B) Biplot originating from principal component analysis integrating all measured variables (HM, PHA, EST, ROS, THC, LYSO, and MN) at six different treatments after the 3-day recovery. The scores of the experimental conditions were shown.
Discussion
The circulating hemocytes of bivalves are susceptible to external factors, such as salinity fluctuations (Gajbhiye and Khandeparker, 2017), temperature changes (Gagnaire et al., 2006), reduced DO (Long et al., 2008), and organic pollutants (Gu et al., 2020). However, the combined effects of salinity change and DO reduction on marine species are limitedly studied nowadays rather than systematically and thoroughly (Wang et al., 2011). When salinity changed, effects could be explained by a decreased hemocyte activity as a result of osmotic effects (Pipe and Coles, 1995) or a physiological inhibition due to decreased respiration and increased activity of antioxidant enzymes (Matozzo et al., 2013). Under hypoxia conditions, bivalves need to open the valve to maximize oxygen absorption to meet physical needs (Sadok et al., 1997). There should be a balance between the two processes, so that the interaction between the change of salinity and DO can be more complicated. In this study, after the 2-week exposure period, the immune parameters of hemocytes were significantly changed, especially under the combined stress of low salinity and hypoxia at night, indicating that the short-term hypoxia and change in salinity have a negative impact on the health of oysters in the near-coastal water. In addition, results from the short-term recovery indicated that the negative impact cannot be improved right away.
During the whole experiment, the Hong Kong oysters did not suffer from death, suggesting tolerance to the change of salinity and hypoxia to some degree. According to the previous study, clam Scrobicularia plana and African cockle Cerastoderma edule also showed certain tolerance to salinity change in the short-term exposure, although a gradually increased mortality appeared in the long-term exposure (Verdelhos et al., 2015). Besides, bivalves can adapt to a hypoxic environment by decreasing the metabolic activity and energy utilization (Wang et al., 2011; Wu et al., 2018a).
Change in THC is considered to be caused by the proliferation or inflammation of the tissues around hemocytes (Yu et al., 2010). THC of marine bivalves is affected by exogenous factors, such as salinity and DO, and THC is also a reliable parameter for evaluating the health of bivalves (Cajaraville et al., 1996; Munari et al., 2011; Wang et al., 2014). In this study, change in salinity reduced THC significantly in the Hong Kong oysters, although high salinity showed a weaker effect than low salinity. The degree of influence of salinity change on hemocytes of oysters could also be observed from the result that the change in salinity weakened the cell respiration and protein synthesis (Ivanina et al., 2020). Some studies in thick shell mussel M. coruscus also showed that change in salinity decreased THC (Wu et al., 2018b), while others observed that high salinity would induce THC in green-lipped mussel Perna viridis (Wang et al., 2012) and Philippine clams Ruditapes philippinarum (Reid et al., 2003). Thus, we deduce the weaker adaptability of Hong Kong oyster to salinity changes. Apart from that, a decrease in DO can also damage hemocyte immunity in bivalves. In this study, hypoxia at night also caused lower THC easily, which is the same with the result in mussel P. viridis (Wang et al., 2014) and cockle Chamelea gallina (Matozzo et al., 2005). Changes in salinity and diel-cycling hypoxia can both lead to the reduction of THC, and hypoxia even aggravated the decrease of THC when salinity changed, which is similar to acidification that exacerbates the effect of salinity change on hemocytes (Wu et al., 2018b). Fortunately, the short-term recovery benefited to THC, but it had a negative impact on oyster in hypoxic water at night. Also, the THC of C. gallina can be recovered after 24 h of hypoxia, while it is out of reach of normal rank after 48 h hypoxic exposure (Matozzo et al., 2005). Therefore, stress caused by diel-cycling hypoxia seemed to be considered as hypoxia similar to the short-term hypoxia within 24 h, but the difference was that the long-term diel-cycling hypoxia would still deliver obvious adverse results on THC.
The HM can be used as a meaningful indicator in the immune response system (Wang et al., 2012). Results showed that salinity reduction significantly increased HM with an increasing trend during the long-term exposure, and increased HM also was indicated in the Pacific oyster C. gigas (Gagnaire et al., 2006). In addition, the increased HM under hypoxic water at night in the Hong Kong oyster was similar to the result of the short-term hypoxia affecting cockle C. gallina (Pampanin et al., 2002). In a word, the results of HM suggested that hemocyte in Hong Kong oyster is sensitive to hypoxia and low salinity. However, it should be highlighted that hypoxia in high salinity did not induce an increase in HM, which is speculated that high salinity stimulated an increase in the energy metabolism of oysters, thereby strengthening cell resistance and then preventing from induction of death (Ivanina et al., 2020). Results of the short-term recovery showed that the HM of Hong Kong oyster had not been well-alleviated, deducing weak adaptation to low salinity and hypoxia in Hong Kong oysters, but mortality or death of hemocytes actually is irreversible. Also, hypoxia seems to be an irreversible factor to affect HM according to the study of Pampanin et al. (2002) and Wang et al. (2012).
The ROS plays an indispensable role in the innate immune response in bivalves (Terahara and Takahashi, 2008). The release of ROS from hemocytes is considered as a key internal defense mechanism through which potential pathogens are killed (Lushchak, 2011; Gajbhiye and Khandeparker, 2017). But if the production of ROS exceeds the antioxidant capacity of bivalves, it will cause cell oxidative damage (Cheng et al., 2004; Gu et al., 2020), so that the reduction of THC may result from high ROS production (Wu et al., 2018b). In this study, THC at low salinity was indeed significantly reduced, and diel-cycling hypoxia also exacerbated the production of ROS in salinity changed water. Low salinity also increased ROS and decreased THC in thick shell mussel M. coruscus (Wu et al., 2018b). The increase of ROS can be explained as the induction of ROS in hemocyte or the inhibition of enzyme to release ROS (Lushchak, 2011). High salinity had no significant influence on ROS, which is similar to the study of Gajbhiye and Khandeparker (2017) who found no significant difference in ROS between 25 and 35‰. Hypoxia at night induced ROS even though salinity was normal. Hypoxia also induced ROS in mussels (Sui et al., 2016), proving hypoxia causes an increase of ROS in bivalves. Similar to HM, ROS was difficult to return to normal level after the short-term recovery. Excessive ROS production may destroy the scavenging activity of the antioxidant system of hemocyte in oyster, leading to a transition between oxidants and antioxidants. Therefore, high HM in the recovery period may also be relevant to ROS.
The EST is a kind of hydrolytic enzyme involved in a series of hydrolysis processes (Pretti and Cognetti-Varriale, 2001) and the intracellular degradation in hemocytes (Mottin et al., 2010). The antibacterial properties of hemocytes in the Hong Kong oyster originate from antibacterial substances such as EST and lysozyme (Li et al., 2018), but the EST of bivalves can be affected by pollutants and other environmental changes, such as change in DO or salinity (Pretti and Cognetti-Varriale, 2001; Wang et al., 2011; Xian et al., 2014). In this study, change in salinity reduced the EST of oysters. Low salinity reduced the EST in oyster C. gigas (Gagnaire et al., 2006), clam Paphia malabarica (Gajbhiye and Khandeparker, 2017), and mussel P. viridis (Wang et al., 2012). While high salinity caused no significant effect on EST until the long-term exposure led to a reduction of activity, showing a slighter effect on EST than low salinity (Wu et al., 2018b). Besides, when oysters were exposed to varying salinity, diel-cycling hypoxia exacerbated the reduction of EST to varying degrees during the 14-day exposure period, which was similar to the experimental results in mussel P. viridis (Wang et al., 2012). Single hypoxia also decreased EST significantly in the early exposure stage (Sui et al., 2016), and the significance is even more obvious if the time extended, indicating a decrease in antibacterial ability. The short-term recovery helped EST recover from the change in salinity and DO, deducing the reversibility of impact.
The PHA is part of the innate immune defense; its function is to recognize and remove foreign bodies (like bacteria) and dead cells (Flannagan et al., 2012). The results of this study show that changes in salinity had no influence on PHA, meaning reorganization and swallowing were normal in Hong Kong oyster. High salinity also had no effect on the percentage of phagocytes in mussel Mytilus galloprovinalis (Malagoli et al., 2007). Higher salinity cannot increase the PHA of the Pacific oyster until after 48 h exposure (Gagnaire et al., 2006). This study also indicates that the short-term hypoxia is beneficial to the increase of PHA in oyster, but PHA in mussel in the early stage of hypoxia is not affected (Malagoli and Ottaviani, 2005). PHA of Hong Kong oyster is connected with granulocytes (Li et al., 2018), and no massive death of granulocytes needs further research to support.
Lysosome takes part in invading microorganisms, so that LYSO can be considered as indicators of health status and defense system vitality in bivalves (Fulin et al., 1989). Lysosome has the ability to synthesize lysozyme in hemocytes of bivalves that is secreted into hemolymph during the PHA (Cheng et al., 1975), and therefore, the immunity can be impacted. In this study, high or low salinity significantly decreased LYSO exclusively with time. Gagnaire et al. (2006) indicated that LYSO had a negative correlation with time significantly. The long-term exposure to diel-cycling hypoxia decreased LYSO even when salinity was normal, and hypoxia can strengthen the impact of salinity. Hypoxia can also reduce LYSO in mussel M. coruscus after 12 h exposure (Wang et al., 2012). After the short-term recovery period, LYSO recovered in each group with no significant difference, indicating that changes in salinity and diel-cycling hypoxia may not cause irreversible damage to LYSO.
The MN is assessed by a specific fluorescent dye MTG, which is sensitive to changes in mitochondrial potential. Due to the photostability, MTG can produce a bright specific signal even at low concentrations (Gautam et al., 2018). MN is closely related to the aerobic capacity of individuals and tissues (Guderley, 2004). Therefore, the amount of MN can affect the aerobic respiration of hemocytes, which in turn affects energy metabolism. Interestingly, the results of this study showed that high salinity significantly reduced MN, indicating decreased respiration. In addition, mitochondria also play an important role in ion transport for adapting salinity change (Ballantyne and Storey, 1984). Changes of MN in hemocytes resulted from their adaptation to change of ion concentration in hemolymph under high salinity. Hypoxia at night significantly increased the MN of Hong Kong oysters in high-salinity waters after the long-term exposure, indicating a way to relieve hypoxia and adapt to diel-cycling short-term hypoxia.
To explain the difference in hemocyte parameters as Hong Kong oysters were exposed to diel-cycling hypoxic water with different salinity, PCA was utilized and it was found that DO was the main influencing factor. Hypoxia can easily lead to immunosuppression and increase susceptibility to pathogens in oysters (Barnett et al., 2020). During the whole exposure period, the two different DO treatments were separated by PC1, showing different or even opposite results in immune parameters, coinciding with the changing trend of the results of each parameter obtained previously in this study. Combining ANOVA and PCA, it is easy to observe the effect of diel-cycling hypoxia, that is, LYSO, EST, and THC are reduced, while ROS, MN, and HM are increased. When oysters were exposed to a stressful environment, negative correlations between the changes of ROS and HM and the changes of LYSO, EST, and THC were found (Huang et al., 2018a), and this is also confirmed from the study in mussels (Wu et al., 2018b). The changes of LYSO, THC, and EST in the hemocyte of Hong Kong oyster are positively correlated with DO changes, and this can be explained by the expression of Tβ-4 mRNA. The expression of Tβ-4 mRNA is related to the production of hemocytes and immunologically active substances (Barnett et al., 2020). When oysters are exposed to hypoxic water, THC is reduced, so that the corresponding lysozyme and EST related to bacteriostatic substances are also reduced. After the short-term recovery, through PCA, oysters under night hypoxia and low salinity were still quite different from those in other treatments in terms of HM and ROS. Through the analysis of the results in this study, we can know that HM and ROS did not recover well. As far as we are concerned, diel-cycling hypoxia has a bit stronger impact than the salinity, for some parameters cannot recover well after the recovery period as a result of hypoxia. Therefore, the short-term recovery has positive effects on the immunity of Hong Kong oyster populations, but living in unsuitable water bodies for a long time, especially in hypoxic water bodies, may threaten the survival of oysters and the development of the oyster farming industry. In addition, the better biomarkers for indicating the immune alteration, according to our results, are HM and MN because of the sensitive changes, especially the MN decrease in oyster under high salinity and diel-cycling hypoxia conditions (Figure 6).
Conclusion
Variable salinity and hypoxia are typical stressors for marine organisms inhabiting estuaries, coastal, and intertidal zones where natural or anthropogenic activity may result in large changes of environmental salinity and DO (Verdelhos et al., 2015). Under the stress of salinity changes and diel-cycling hypoxia, the hemocyte functions of Hong Kong oyster C. hongkongensis were impaired. In addition, low salinity did massive harm to the immunity of oysters than high salinity. Meanwhile, diel-cycling hypoxia shaved immune functions substantially, which was synergistic with low salinity. Although the long-term exposure to salinity changes with diel-cycling hypoxia was adverse, the short-term recovery could be helpful for oysters to recover, apart from HM and ROS. Our results provide useful information for the health assessment of oysters and bring new insights to oyster aquaculture industries.
Data Availability Statement
The original contributions presented in the study are included in the article/Supplementary Material, further inquiries can be directed to the corresponding author/s.
Author Contributions
HD did the assistance to both writing and experiments. QZ and HC contributed to the experiment. JP provided the resource of oysters. WL, IS, MH, and YW provided project funding and helped to modify the manuscript. All authors contributed to the article and approved the submitted version.
Funding
This research was supported by the Open Fund of Guangxi Key Laboratory of Aquatic Genetic Breeding and Healthy Aquaculture and the Science and Technology Major Project of Guangxi [Grant No: AA17204080-4] and the grants from the National Natural Science Foundation of China (31872587).
Conflict of Interest
The authors declare that the research was conducted in the absence of any commercial or financial relationships that could be construed as a potential conflict of interest.
Publisher's Note
All claims expressed in this article are solely those of the authors and do not necessarily represent those of their affiliated organizations, or those of the publisher, the editors and the reviewers. Any product that may be evaluated in this article, or claim that may be made by its manufacturer, is not guaranteed or endorsed by the publisher.
Supplementary Material
The Supplementary Material for this article can be found online at: https://www.frontiersin.org/articles/10.3389/fmars.2021.749623/full#supplementary-material
References
Ballantyne, J. S., and Storey, K. B. (1984). Mitochondria from the hepatopancreas of the marine clam Mercenaria mercenaria: substrate preferences and salt and pH effects on the oxidation of palmitoyl-L-carnitine and succinate. J. Exp. Zool. 230, 165–174. doi: 10.1002/jez.1402300202
Barnett, A. F., Gledhill, J. H., Griffitt, R. J., Slattery, M., Gochfeld, D. J., and Willett, K. L. (2020). Combined and independent effects of hypoxia and tributyltin on mRNA expression and physiology of the eastern oyster (Crassostrea virginica). Sci. Rep. 10:10605. doi: 10.1038/s41598-020-67650-x
Berger, V. J., and Kharazova, A. D. (1997). Mechanisms of salinity adaptations in marine molluscs. Hydrobiologia 355, 115–126. doi: 10.1023/A:1003023322263
Booij, M. J. (2005). Impact of climate change on river flooding assessed with different spatial model resolutions. J. Hydrol. 303, 176–198. doi: 10.1016/j.jhydrol.2004.07.013
Breitburg, D. L., Salisbury, J., Bernhard, J. M., Cai, W.-J., Dupont, S., Doney, S. C., et al. (2015). And on top of all that coping with ocean acidification in the midst of many stressors. Oceanography 28, 48–61. doi: 10.5670/oceanog.2015.31
Cajaraville, M. P., Olabarrieta, I., and Marigomez, I. (1996). In vitro activities in mussel hemocytes as biomarkers of environmental quality: a case study in the Abra Estuary (Biscay Bay). Ecotoxicol. Environ. Saf. 35, 253–260. doi: 10.1006/eesa.1996.0108
Carregosa, V., Figueira, E., Gil, A. M., Pereira, S., Pinto, J., Soares, A. M. V. M., et al. (2014a). Tolerance of Venerupis philippinarum to salinity: Osmotic and metabolic aspects. Comp. Biochem. Physiol. Part A Mol. Integr. Physiol. 171, 36–43. doi: 10.1016/j.cbpa.2014.02.009
Carregosa, V., Velez, C., Soares, A. M. V. M., Figueira, E., and Freitas, R. (2014b). Physiological and biochemical responses of three Veneridae clams exposed to salinity changes. Comp. Biochem. Physiol. B. Biochem. Mol. Biol. 177–178, 1–9. doi: 10.1016/j.cbpb.2014.08.001
Cheng, T. C., Rodrick, G. E., Foley, D. A., and Koehler, S. A. (1975). Release of lysozyme from hemolymph cells of Mercenaria mercenaria during phagocytosis. J. Invertebr. Pathol. 25, 261–265. doi: 10.1016/0022-2011(75)90076-2
Cheng, W., Liu, C., Cheng, S.-Y., and Chen, J.-C. (2004). Effect of dissolved oxygen on the acid-base balance and ion concentration of Taiwan abalone Haliotis diversicolor supertexta. Aquaculture 231, 573–586. doi: 10.1016/j.aquaculture.2003.10.030
Ciacci, C., Canonico, B., Bilanicova, D., Fabbri, R., Cortese, K., Gallo, G., et al. (2012). Immunomodulation by different types of N-Oxides in the hemocytes of marine bivalve Mytilus galloprovincialis. PLoS ONE 7:36937. doi: 10.1371/journal.pone.0036937
Delaporte, M., Soudant, P., Moal, J., Lambert, C., Quere, C., Miner, P., et al. (2003). Effect of a mono-specific algal diet on immune functions in two bivalve species - Crassostrea gigas and Ruditapes philippinarum. J. Exp. Biol. 206, 3053–3064. doi: 10.1242/jeb.00518
Diaz, R. J., and Rosenberg, R. (2008). Spreading dead zones and consequences for marine ecosystems. Science 321, 926–929. doi: 10.1126/science.1156401
Donaghy, L., Jauzein, C., and Volety, A. K. (2012). First report of severe hemocytopenia and immunodepression in the sunray venus clam, Macrocallista nimbosa, a potential new aquaculture species in Florida. Aquaculture 364, 247–251. doi: 10.1016/j.aquaculture.2012.08.045
Donaghy, L., Lambert, C., Choi, K.-S., and Soudant, P. (2009). Hemocytes of the carpet shell clam (Ruditapes decussatus) and the Manila clam (Ruditapes philippinarum): current knowledge and future prospects. Aquaculture 297, 10–24. doi: 10.1016/j.aquaculture.2009.09.003
Doney, S. C. (2010). The growing human footprint on coastal and open-ocean biogeochemistry. Science 328, 1512–1516. doi: 10.1126/science.1185198
Ertl, N. G., O'Connor, W. A., and Elizur, A. (2019). Molecular effects of a variable environment on Sydney rock oysters, Saccostrea glomerata: thermal and low salinity stress, and their synergistic effect. Mar. Genom. 43, 19–32. doi: 10.1016/j.margen.2018.10.003
Flannagan, R. S., Jaumouille, V., and Grinstein, S. (2012). “The cell biology of phagocytosis,” in Annual Review of Pathology: Mechanisms of Disease, Vol 7, eds A. K. Abbas, S. J. Galli and P. M. Howley (Chicago, IL: University of Chicago Libraries), 61–98. doi: 10.1146/annurev-pathol-011811-132445
Foy, R. H., Lennox, S. D., and Smith, R. V. (2001). Assessing the effectiveness of regulatory controls on farm pollution using chemical and biological indices of water quality and pollution statistics. Water Res. 35, 3004–3012. doi: 10.1016/S0043-1354(00)00587-X
Fulin, E., Chu, and Peyre, J. F. L. (1989). Effect of environmental factors and parasitism on hemolymph lysozyme and protein of American oysters (Crassostrea virginica). J. Invertebr. Pathol. 54, 224–232. doi: 10.1016/0022-2011(89)90032-3
Gagnaire, B., Duchemin, M., Auffret, M., Thomas-Guyon, H., and Renault, T. (2008). Comparison of hemocyte parameters in the pericardial cavity and the adductor muscle sinus in the Pacific oyster, Crassostrea gigas using two types of flow cytometers. Aquat. Biol. 21, 39–43. doi: 10.1051/alr:2008009
Gagnaire, B., Frouin, H., Moreau, K., Thomas-Guyon, H., and Renault, T. (2006). Effects of temperature and salinity on haemocyte activities of the Pacific oyster, Crassostrea gigas (Thunberg). Fish Shellfish Immunol. 20, 536–547. doi: 10.1016/j.fsi.2005.07.003
Gajbhiye, D. S., and Khandeparker, L. (2017). Immune response of the short neck clam Paphia malabarica to salinity stress using flow cytometry. Mar. Environ. Res. 129, 14–23. doi: 10.1016/j.marenvres.2017.04.009
Gautam, N., Sankaran, S., Yason, J. A., Tan, K. S. W., and Gascoigne, N. R. J. (2018). A high content imaging flow cytometry approach to study mitochondria in T cells: MitoTracker Green FM dye concentration optimization. Methods 134, 11–19. doi: 10.1016/j.ymeth.2017.11.015
Gomes, T., Araujo, O., Pereira, R., Almeida, A. C., Cravo, A., and Bebianno, M. J. (2013). Genotoxicity of copper oxide and silver nanoparticles in the mussel Mytilus galloprovincialis. Mar. Environ. Res. 84, 51–59. doi: 10.1016/j.marenvres.2012.11.009
Gu, H., Wei, S., Hu, M., Wei, H., Wang, X., Shang, Y., et al. (2020). Microplastics aggravate the adverse effects of BDE-47 on physiological and defense performance in mussels. J. Hazard. Mater. 398:122909. doi: 10.1016/j.jhazmat.2020.122909
Guderley, H. (2004). Metabolic responses to low temperature in fish muscle. Biol. Rev. 79, 409–427. doi: 10.1017/S1464793103006328
Guo, X., He, Y., Zhang, L., Lelong, C., and Jouaux, A. (2015). Immune and stress responses in oysters with insights on adaptation. Fish Shellfish Immunol. 46, 107–119. doi: 10.1016/j.fsi.2015.05.018
Howarth, R., Chan, F., Conley, D. J., Garnier, J., Doney, S. C., Marino, R., et al. (2011). Coupled biogeochemical cycles: eutrophication and hypoxia in temperate estuaries and coastal marine ecosystems. Front. Ecol. Environ. 9, 18–26. doi: 10.1890/100008
Huang, X., Jiang, X., Sun, M., Dupont, S., Huang, W., Hu, M., et al. (2018a). Effects of copper on hemocyte parameters in the estuarine oyster Crassostrea rivularis under low pH conditions. Aquat. Toxicol. 203, 61–68. doi: 10.1016/j.aquatox.2018.08.003
Huang, X., Lin, D., Ning, K., Sui, Y., Hu, M., Lu, W., et al. (2016). Hemocyte responses of the thick shell mussel Mytilus coruscus exposed to nano-TiO2 and seawater acidification. Aquat. Toxicol. 180, 1–10. doi: 10.1016/j.aquatox.2016.09.008
Huang, X., Liu, Z., Xie, Z., Dupont, S., Huang, W., Wu, F., et al. (2018b). Oxidative stress induced by titanium dioxide nanoparticles increases under seawater acidification in the thick shell mussel Mytilus coruscus. Mar. Environ. Res. 137, 49–59. doi: 10.1016/j.marenvres.2018.02.029
IPCC (2014). Climate change 2013: The Physical Science Basis: Intergovernmental Panel on Climate Change.
Ivanina, A. V., Jarrett, A., Bell, T., Rimkevicius, T., Beniash, E., and Sokolova, I. M. (2020). Effects of seawater salinity and pH on cellular metabolism and enzyme activities in biomineralizing tissues of marine bivalves. Comp. Biochem. Physiol. Part A Mol. Integr. Physiol. 248:110748. doi: 10.1016/j.cbpa.2020.110748
Legrand, E., Riera, P., Pouliquen, L., Bohner, O., Cariou, T., and Martin, S. (2018). Ecological characterization of intertidal rockpools: seasonal and diurnal monitoring of physico-chemical parameters. Reg. Stud. Mar. Sci. 17, 1–10. doi: 10.1016/j.rsma.2017.11.003
Li, J., Zhang, Y., Mao, F., Lin, Y., Xiao, S., Xiang, Z., et al. (2018). The first morphologic and functional characterization of hemocytes in Hong Kong oyster, Crassostrea hongkongensis. Fish Shellfish Immunol. 81, 423–429. doi: 10.1016/j.fsi.2018.05.062
Liu, F., and Wang, W.-X. (2013). Facilitated bioaccumulation of cadmium and copper in the oyster Crassostrea hongkongensis solely exposed to zinc. Environ. Sci. Technol. 47, 1670–1677. doi: 10.1021/es304198h
Long, W. C., Brylawski, B. J., and Seitz, R. D. (2008). Behavioral effects of low dissolved oxygen on the bivalve Macoma balthica. J. Exp. Mar. Biol. Ecol. 359, 34–39. doi: 10.1016/j.jembe.2008.02.013
Lushchak, V. I. (2011). Environmentally induced oxidative stress in aquatic animals. Aquat. Toxicol. 101, 13–30. doi: 10.1016/j.aquatox.2010.10.006
Maguire, J. A., Fleury, P. G., and Burnell, G. M. (1999). Some methods for quantifying quality in the scallop Pecten maximus (L.). J. Shellfish Res. 18, 59–66.
Malagoli, D., Casarini, L., Sacchi, S., and Ottaviani, E. (2007). Stress and immune response in the mussel Mytilus galloprovincialis. Fish Shellfish Immunol. 23, 171–177. doi: 10.1016/j.fsi.2006.10.004
Malagoli, D., and Ottaviani, E. (2005). Cytotoxicity as a marker of mussel health status. J. Mar. Biolog. Assoc. 85, 359–362. doi: 10.1017/S0025315405011276h
Matozzo, V., Chinellato, A., Munari, M., Bressan, M., and Marin, M. G. (2013). Can the combination of decreased pH and increased temperature values induce oxidative stress in the clam Chamelea gallina and the mussel Mytilus galloprovincialis? Mar. Pollut. Bull. 72, 34–40. doi: 10.1016/j.marpolbul.2013.05.004
Matozzo, V., Monari, M., Foschi, J., Papi, T., Cattani, O., and Marin, M. G. (2005). Exposure to anoxia of the clam Chamelea gallina I. Effects on immune responses. J. Exp. Mar. Biol. Ecol. 325, 163–174. doi: 10.1016/j.jembe.2005.04.030
Matozzo, V., Monari, M., Foschi, J., Serrazanetti, G. P., Cattani, O., and Marin, M. G. (2007). Effects of salinity on the clam Chamelea gallina. Part I: alterations in immune responses. Mar. Biol. 151, 1051–1058. doi: 10.1007/s00227-006-0543-6
McCarty, A. J., McFarland, K., Small, J., Allen, S. K. Jr., and Plough, L. V. (2020). Heritability of acute low salinity survival in the Eastern oyster (Crassostrea virginica). Aquaculture 529:735649. doi: 10.1016/j.aquaculture.2020.735649
Montagna, P. A., Hu, X., Palmer, T. A., and Wetz, M. (2018). Effect of hydrological variability on the biogeochemistry of estuaries across a regional climatic gradient. Limnol. Oceanogr. 63, 2465–2478. doi: 10.1002/lno.10953
Mottin, E., Caplat, C., Mahaut, M.-L., Costil, K., Barillier, D., Lebel, J.-M., et al. (2010). Effect of in vitro exposure to zinc on immunological parameters of haemocytes from the marine gastropod Haliotis tuberculata. Fish Shellfish Immunol. 29, 846–853. doi: 10.1016/j.fsi.2010.07.022
Munari, M., Matozzo, V., and Marin, M. G. (2011). Combined effects of temperature and salinity on functional responses of haemocytes and survival in air of the clam Ruditapes philippinarum. Fish Shellfish Immunol. 30, 1024–1030. doi: 10.1016/j.fsi.2011.01.025
Naqvi, S. W. A., Jayakumar, D. A., Narvekar, P. V., Naik, H., Sarma, V., D'Souza, W., et al. (2000). Increased marine production of N2O due to intensifying anoxia on the Indian continental shelf. Nature 408, 346–349. doi: 10.1038/35042551
Norman, M. (2005). Condition index of the eastern oyster, Crassostrea virginica (Gmelin 1791) in Sapelo Island Georgia-effects of site, position on bed and pea crab parasitism. J. Shellfish Res. 24, 121–126. doi: 10.2983/0730-8000(2005)24[121:CIOTEO]2.0.CO;2
Pampanin, D. M., Ballarin, L., Carotenuto, L., and Marin, M. G. (2002). Air exposure and functionality of Chamelea gallina haemocytes: effects on haematocrit, adhesion, phagocytosis and enzyme contents. Comp. Biochem. Phys. A Mol. Integr. Physiol. 133, 199–199. doi: 10.1016/S1095-6433(02)00114-9
Pequeux, A. (1995). Osmotic regulation in crustaceans. J. Crust. Biol. 15, 1–60. doi: 10.2307/1549010
Pipe, R. K., and Coles, J. A. (1995). Environmental contaminants influencing immunefunction in marine bivalve molluscs. Fish Shellfish Immunol. 5, 581–595. doi: 10.1016/S1050-4648(95)80043-3
Pretti, C., and Cognetti-Varriale, A. M. (2001). The use of biomarkers in aquatic biomonitoring: the example of esterases. Aquat. Conserv. 11, 299–303. doi: 10.1002/aqc.457
Przeslawski, R., Byrne, M., and Mellin, C. (2015). A review and meta-analysis of the effects of multiple abiotic stressors on marine embryos and larvae. Glob. Chang. Biol. 21, 2122–2140. doi: 10.1111/gcb.12833
Qin, Y., Xiao, S., Ma, H., Mo, R., Zhou, Z., Wu, X., et al. (2018). Effects of salinity and temperature on the timing of germinal vesicle breakdown and polar body release in diploid and triploid Hong Kong oysters, Crassostrea hongkongensis, in relation to tetraploid induction. Aquac. Res. 49, 3647–3657. doi: 10.1111/are.13833
Reid, H. I., Soudant, P., Lambert, C., Paillard, C., and Birkbeck, T. H. (2003). Salinity effects on immune parameters of Ruditapes philippinarum challenged with Vibrio tapetis. Dis. Aquat. Org. 56, 249–258. doi: 10.3354/dao056249
Sadok, S., Uglow, R. F., and Haswell, S. J. (1997). Haemolymph and mantle fluid ammonia and ninhydrin positive substances variations in salinity-challenged mussels (Mytilus edulis L). J. Exp. Mar. Biol. Ecol. 211, 195–212. doi: 10.1016/S0022-0981(96)02732-3
Shekhar, M. S., Kiruthika, J., and Ponniah, A. G. (2013). Identification and expression analysis of differentially expressed genes from shrimp (Penaeus monodon) in response to low salinity stress. Fish Shellfish Immunol. 35, 1957–1968. doi: 10.1016/j.fsi.2013.09.038
Stevens, A. M., and Gobler, C. J. (2018). Interactive effects of acidification, hypoxia, and thermal stress on growth, respiration, and survival of four North Atlantic bivalves. Mar. Ecol. Prog. Ser. 604, 143–161. doi: 10.3354/meps12725
Sui, Y., Hu, M., Huang, X., Wang, Y., and Lu, W. (2015). Anti-predatory responses of the thick shell mussel Mytilus coruscus exposed to seawater acidification and hypoxia. Mar. Environ. Res. 109, 159–167. doi: 10.1016/j.marenvres.2015.07.008
Sui, Y., Kong, H., Shang, Y., Huang, X., Wu, F., Hu, M., et al. (2016). Effects of short-term hypoxia and seawater acidification on hemocyte responses of the mussel Mytilus coruscus. Mar. Pollut. Bull. 108, 46–52. doi: 10.1016/j.marpolbul.2016.05.001
Sui, Y., Liu, Y., Zhao, X., Dupont, S., Hu, M., Wu, F., et al. (2017). Defense responses to short-term hypoxia and seawater acidification in the thick shell mussel Mytilus coruscus. Front. Physiol. 8:145. doi: 10.3389/fphys.2017.00145
Terahara, K., and Takahashi, K. G. (2008). Mechanisms and immunological roles of apoptosis in molluscs. Curr. Pharm. Des. 14, 131–137. doi: 10.2174/138161208783378725
Verdelhos, T., Marques, J. C., and Anastacio, P. (2015). The impact of estuarine salinity changes on the bivalves Scrobicularia plana and Cerastoderma edule, illustrated by behavioral and mortality responses on a laboratory assay. Ecol. Indic. 52, 96–104. doi: 10.1016/j.ecolind.2014.11.022
Vosloo, A., Laas, A., and Vosloo, D. (2013). Differential responses of juvenile and adult South African abalone (Haliotis midae Linnaeus) to low and high oxygen levels. Comp. Biochem. Physiol. Part A Mol. Integr. Physiol. 164, 192–199. doi: 10.1016/j.cbpa.2012.09.002
Wang, Y., Hu, M., Cheung, S. G., Shin, P. K. S., Lu, W., and Li, J. (2012). Immune parameter changes of hemocytes in green-lipped mussel Perna viridis exposure to hypoxia and hyposalinity. Aquaculture 356, 22–29. doi: 10.1016/j.aquaculture.2012.06.001
Wang, Y., Hu, M., Li, Q., Li, J., Lin, D., and Lu, W. (2014). Immune toxicity of TiO2 under hypoxia in the green-lipped mussel Perna viridis based on flow cytometric analysis of hemocyte parameters. Sci. Total Environ. 470, 791–799. doi: 10.1016/j.scitotenv.2013.09.060
Wang, Y., Hu, M., Wong, W. H., Shin, P. K. S., and Cheung, S. G. (2011). The combined effects of oxygen availability and salinity on physiological responses and scope for growth in the green-lipped mussel Perna viridis. Mar. Pollut. Bull. 63, 255–261. doi: 10.1016/j.marpolbul.2011.02.004
Wu, F., Cui, S., Sun, M., Xie, Z., Huang, W., Huang, X., et al. (2018a). Combined effects of ZnO NPs and seawater acidification on the haemocyte parameters of thick shell mussel Mytilus coruscus. Sci. Total Environ. 624, 820–830. doi: 10.1016/j.scitotenv.2017.12.168
Wu, F., Xie, Z., Lan, Y., Dupont, S., Sun, M., Cui, S., et al. (2018b). Short-term exposure of Mytilus coruscus to decreased pH and salinity change impacts immune parameters of their haemocytes. Front. Physiol. 9:166. doi: 10.3389/fphys.2018.00166
Wu, R. S. S. (2002). Hypoxia: from molecular responses to ecosystem responses. Mar. Pollut. Bull. 45, 35–45. doi: 10.1016/S0025-326X(02)00061-9
Xian, J., Li, B., Guo, H., Miao, Y., Ye, J., Feng, L., et al. (2014). Haemocyte apoptosis of the tiger shrimp Penaeus monodon exposed to cadmium. Bull. Environ. Contam. Toxicol. 92, 525–528. doi: 10.1007/s00128-013-1165-6
Keywords: hemocyte, immune response, oyster, flow cytometry, hypoxia, salinity
Citation: Xie Z, Wei S, Dong H, Chen H, Zhang Q, Liu W, Peng J, Sokolova IM, Hu M and Wang Y (2021) Hemocyte Responses of the Oyster Crassostrea hongkongensis Exposed to Diel-Cycling Hypoxia and Salinity Change. Front. Mar. Sci. 8:749623. doi: 10.3389/fmars.2021.749623
Received: 29 July 2021; Accepted: 18 August 2021;
Published: 16 September 2021.
Edited by:
Pengzhi Qi, Zhejiang Ocean University, ChinaCopyright © 2021 Xie, Wei, Dong, Chen, Zhang, Liu, Peng, Sokolova, Hu and Wang. This is an open-access article distributed under the terms of the Creative Commons Attribution License (CC BY). The use, distribution or reproduction in other forums is permitted, provided the original author(s) and the copyright owner(s) are credited and that the original publication in this journal is cited, in accordance with accepted academic practice. No use, distribution or reproduction is permitted which does not comply with these terms.
*Correspondence: Jinxia Peng, MjY5Njc5MjUwQHFxLmNvbQ==; Youji Wang, eW91aml3YW5nMkBnbWFpbC5jb20=
†These authors have contributed equally to this work