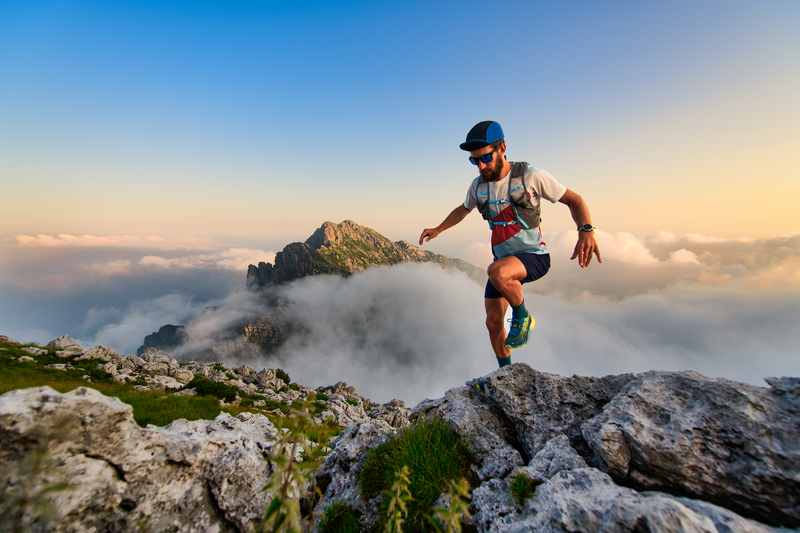
94% of researchers rate our articles as excellent or good
Learn more about the work of our research integrity team to safeguard the quality of each article we publish.
Find out more
ORIGINAL RESEARCH article
Front. Mar. Sci. , 02 November 2021
Sec. Marine Biology
Volume 8 - 2021 | https://doi.org/10.3389/fmars.2021.748389
To better understand the hydrodynamic and hydrographic conditions experienced by larvae in the nearshore (within 1 km of shore), and the role that larval behavior plays in mediating shoreward transport to adult benthic habitats, we examined the vertical distribution and concentration of barnacle cyprids in a shallow, nearshore region in southern California, United States. We collected high-resolution physical measurements of currents and temperature at 3 stations (8, 5, and 4 m depths), and high-frequency measurements of barnacle larvae at a 4 m deep station ∼300 m from shore. Larvae were sampled from distinct 1 m depth intervals between the surface and the bottom (0–1 m, 1–2 m, 2–3 m, 3 m-bottom), each hour for overnight periods that ranged between 13 to 24 h in five cruises during the summers of 2017 and 2018. Barnacle cyprids of Chthamalus fissus predominated in all samples. Thermal stratification decreased closer to shore, but when the nearshore-most station remained stratified (Δ°C m–1 ≥ 0.1), C. fissus cyprid concentrations were high to extremely abundant (exceeding 200 and 4,000 individuals m–3, respectively). There were significant positive correlations between thermal stratification and the log-transformed C. fissus concentration at cruise-to-cruise scales, and between stratification and vertical variability in the high-frequency cross-shore currents at 2-day scales. Additionally, estimated larval transport was relatively high and shoreward when nearshore thermal stratification was greatest. Significant, albeit small, diel differences in cyprid distributions were also observed, with the proportion of cyprids increasing near the surface at night, and concentrations greater during the day than at night. Collectively, these results suggest that thermal stratification increases larval supply to the nearshore, and may enhance onshore larval transport to augment chances of successful settlement and recruitment to the intertidal adult habitat.
Most benthic marine organisms settling in the intertidal zone have planktonic larvae that reside temporarily (days to weeks) in the water column before returning to shore to complete their life cycle. Therefore, the extent of settlement and recruitment of benthic organisms relies, in part, on successful larval transport. Larval transport, defined as a flux, or as the mean horizontal translocation of larvae between points along a specified one-dimensional axis per unit time (Pineda and Reyns, 2018), is a critical component of larval dispersal, defined as the spread of larvae from spawning to settlement sites (Pineda et al., 2007). Studying the mechanisms of larval transport is fundamental to conserve marine species, manage fisheries, improve modeling of population dynamics (Cowen and Sponaugle, 2009), and understand population connectivity.
The physical processes and biological mechanisms driving coastal larval transport have been extensively studied, and yet remain poorly understood in the nearshore (here defined as within 1 km from shore but excluding the surfzone; reviewed in Pineda and Reyns, 2018). This is partly because studies on intertidal species suggest that larval transport and dispersal can be episodic, and occur at smaller spatial scales than previously anticipated, with larvae often remaining within the nearshore close to settlement sites (Shanks et al., 2003; Tapia and Pineda, 2007; Morgan et al., 2009; Shanks and Shearman, 2009; Hagerty et al., 2018). The generally poor horizontal swimming capabilities of larvae (Chia et al., 1984) make them susceptible to being swept away due to the advective nature of the nearshore (Lentz and Fewings, 2012). However, ontogenetic changes in larval swimming efficiency (Wong et al., 2020) and larval behavioral responses may regulate dispersal and improve chances of returning to a settling site. These behaviors include altering vertical distribution through buoyancy control and vertical swimming (Daigle and Metaxas, 2011; DiBacco et al., 2011) that allow larvae to exploit vertically sheared flows and ultimately control horizontal transport (e.g., Thièbaut et al., 1992; Weidberg et al., 2019; Guillam et al., 2020). Moreover, larvae of benthic organisms also exhibit diel vertical distribution patterns (dos Santos et al., 2008; Bonicelli et al., 2016; Guillam et al., 2020), allowing them to evade visual predators during the day or reducing energy consumption by remaining in colder waters (e.g., Zaret and Suffern, 1976; Forward and Rittschof, 2000). Thus, the extent to which larvae can exploit onshore flow by altering their vertical position in the nearshore may have profound consequences on successful recruitment of benthic populations and should be characterized at fine temporal resolutions to be better understood.
The degree to which larvae regulate transport in the nearshore is complicated because hydrodynamic conditions can be unpredictable and are highly variable (Winant and Bratkovich, 1981; Bonicelli et al., 2016; Morgan et al., 2018). Physical processes in shallow coastal waters are affected by topographic features (e.g., Lerczak et al., 2003), surface and internal tides (e.g., Weidberg et al., 2019), wind-driven processes (e.g., Griffin and Middleton, 1992), surface gravity waves (e.g., Kumar and Feddersen, 2017), and other meso- and large-scale physical processes (e.g., Pineda et al., 2018) that impact the water column from scales of seconds to days to years, all of which have implications on larval transport and dispersal (Pineda et al., 2007). Recent findings in southern California indicate that increases in thermal stratification within the nearshore results in larval accumulation closer to shore, in a region just seaward of the surfzone (Hagerty et al., 2018). It is possible that higher stratification promotes the development of fronts, internal tidal bores, or other internal motions that aid onshore larval transport (Pineda, 1999; Shanks et al., 2003; Weidberg et al., 2019). For example, in shallow waters, these internal motions tend to result from tidal flows interacting with bathymetric features and can propagate toward shore along the thermocline (e.g., Winant and Olson, 1976). Furthermore, higher stratification correlates with vertical variability in two-way cross-shore flows (e.g., Winant and Bratkovich, 1981), potentially enhancing larval behavioral control of cross-shore transport (Hagerty et al., 2018), and promoting settlement. Moreover, stratification can also act as a barrier to vertical larval distribution, as some species maintain bottom distributions (sea scallops: Daigle and Metaxas, 2011), or remain below the thermocline (gastropod, bivalve and polychaete larvae: Lloyd et al., 2012) in stratified conditions. A number of studies have also documented the association of fish larvae and other zooplankton with the depth of the thermocline (e.g., Haney, 1988; Gray and Kingsford, 2003), suggesting that larvae exhibit behaviors and respond to water-column dynamics.
For barnacles in central Chile, vertical distribution in relation to the pycnocline depth varies by species and by larval stage, over several months (Bonicelli et al., 2016), but it is unclear how such distribution and abundance responds to high-frequency (<24 h), in addition to the monthly, variations in stratification. Understanding how larval distribution varies in response to stratification is particularly relevant in the nearshore, where internal waves and diurnal heating can drive changes to the water column stratification on daily, hourly, and shorter time scales (e.g., Winant, 1974; Sinnett et al., 2018; Grimes et al., 2020a). Barnacles have a typical marine invertebrate lifecycle, the early and final larval stages are easily distinguished, are very abundant, and knowledge about the vertical migration of their larvae can help to understand larval transport of other benthic species with pelagic larvae. In temperate eastern Pacific coastlines, barnacles tend to have six naupliar stages that develop offshore, and a final non-feeding cyprid larval stage which transports through the nearshore to reach intertidal juvenile and adult settlement habitats (Tapia and Pineda, 2007; Bonicelli et al., 2016; Hagerty et al., 2018). Barnacle larvae exhibit ontogenetic differences in vertical distribution (Tapia et al., 2010; Bonicelli et al., 2016; Hagerty et al., 2018), and may display changes in vertical position during diel cycles (dos Santos et al., 2007; Bonicelli et al., 2016). Changes in vertical distribution can also take place at small-spatial scales (100’s m) in response to frontal circulation (Pineda, 1999), and in laboratory conditions when downwelling flows are experienced (DiBacco et al., 2011).
This study aimed to provide insights on how behavior, vertical distribution, abundance, and onshore larval transport of barnacle larvae are driven by dynamic conditions at a shallow, nearshore site over a 24 h period. We focused on barnacle cyprids, the stage that must transport from the offshore larval pool to adult intertidal habitats to settle. We emphasized thermal stratification processes because previous studies revealed an association between stratification and (a) nearshore cross-shore larval distribution at cruise-to-cruise (∼days to few weeks) and seasonal scales (Hagerty et al., 2018), and (b) settlement at 2–3 day (Pineda and López, 2002) and interannual scales (Pineda et al., 2018). Here, we build on this work to determine how larval abundance and vertical larval distributions are impacted by shorter-scale (hourly) fluctuations in hydrodynamic and hydrographic conditions. We hypothesize that larval abundance in the nearshore is related to thermal stratification. We focused in the shallow nearshore zone, located in ∼4 m deep area just offshore of the surfzone, because this is an important region (Hagerty et al., 2018) connecting offshore larval development grounds to the surfzone and adult rocky intertidal habitat. Nearshore regions of many coastal areas remain understudied (but see Porri et al., 2006; Dudas et al., 2009b; Pfaff et al., 2015), yet quantifying the temporal scales at which nearshore hydrodynamic and hydrographic processes operate is critical for understanding larval transport of organisms residing in coastal and rocky intertidal habitats. In this study, we specifically addressed the following questions: (1) does nearshore larval abundance vary with thermal stratification, and if so, at what temporal scales? (2) Is onshore larval transport associated with thermal stratification? (3) What is the hourly and day-night variability in larval vertical distribution?
This study was conducted offshore of Bird Rock, La Jolla, CA, United States (Figure 1) within the South La Jolla State Marine Reserve, a marine protected area (MPA). This region hosts large populations of the numerically dominant barnacle species Chthamalus fissus, that has a larval duration of ca. 3 weeks (e.g., Miller et al., 1989), and larvae that recruit throughout the year (Pineda and López, 2002; Hargenrader, 2018; Pineda et al., 2018). Sampling occurred at the same 4 m deep station sampled by Hagerty et al. (2018) who observed relatively large (monthly and seasonal) temporal-scale variability in cross-shore larval distribution and thermal stratification. The purpose of this follow-up study was to examine high-frequency (hourly) variations of cyprid vertical distribution with respect to hydrodynamic and hydrographic conditions in the water column at a fixed station in a region seaward of the surfzone where cyprid accumulation was previously observed when offshore waters were stratified (Hagerty et al., 2018). Sampling was conducted during June and July (hereafter summer) 2017 and 2018, corresponding to periods of high barnacle settlement (Pineda, 1994; Pineda et al., 2018) and thermal stratification (Winant and Bratkovich, 1981; Hagerty et al., 2018; Sinnett and Feddersen, 2019).
Figure 1. Inset showing Bird Rock study site in La Jolla region (indicated by arrow), CA, United States. Map shows nearshore bathymetric isobaths from Mean Lower Low Water (MLLW) to 12 m depth at 2 m isobath intervals. Black ‘X’ represents the 4 m deep larval and CTD sampling station (∼280 m from shore); the two black circles represent the 5 m- and 8 m-deep temperature mooring stations (∼400 and ∼800 m from shore respectively); Nortek Aquadopp Profiler (ADCP) was also deployed near the 5 m deep temperature mooring at ∼6 m depth (circles overlap). Lidar bathymetry data from the 2013 NOAA Coastal California TopoBathy Merge Project https://data.noaa.gov/dataset/2013-noaa-coastal-california-topobathy-merge-project.
Samples were taken from a 7.6 m boat anchored at a fixed, shallow (average 4 m deep over a tidal cycle) nearshore station (Figure 1) during 5 cruises: Cruises 1 and 2 were conducted July 16–17 and July 25–26, 2017, respectively, while Cruises 3, 4 and 5 were conducted June 7–8, June 21–22, and July 16–17, 2018, respectively (Table 1). Cruise dates were not selected to replicate tidal or lunar cycles. Plankton profiles were sampled hourly using a Dominator submersible semivortex pump (Ebara 50DWXU6.4S) to filter 2 m3 of seawater from distinct 1 m depth intervals extending from the surface to the bottom [0–1 m, 1–2 m, 2–3 m and 3 m- to the seafloor bottom (∼4 m)], by oscillating the pump continuously throughout each 1 m depth interval. Seawater was filtered using a 118-μm mesh net to collect all stages of barnacle larvae, and samples were immediately preserved in 100% ethanol. All cruises were sampled during the morning and evening twilight and through the night. However, due to equipment failure, the number of plankton sampling hours differed for each cruise (Cruise 1 = 13 h; Cruise 2 = 14 h; Cruise 3 = 23 h; Cruise 4 = 19 h; and Cruise 5 = 24 h; Table 1). Plankton samples were quantitatively subsampled using a Folsom plankton splitter, and larvae were enumerated and identified using a dissecting microscope (Olympus SZX12-ILLD). Barnacle cyprids were identified to species based on existing morphological descriptions (Lewis, 1975; Vedder and Branscomb, 1982; Brown and Roughgarden, 1985; Miller et al., 1989; Miller and Roughgarden, 1994; Shanks, 2001), including those confirmed through molecular analyses by Hagerty et al. (2019). Six species of barnacle larvae were identified, including C. fissus (92% of counted individuals), Pollicipes polymerus (7%), and the remaining 1% of cyprids comprised of Balanus glandula, Balanus trigonus, Tetraclita rubescens, and Megabalanus californicus. Only two individual cyprids remained unidentified. Given the low concentration of other species, C. fissus cyprids will hereafter be the focus of this study.
Table 1. Cruise summaries with dates, start and end hours of CTD and plankton sampling (PDT), Chthamalus fissus cyprid concentrations (no. m–3) with minimum and maximum concentrations per cruise in parentheses, and average ± SE thermal stratification (Δ°C m–1) at 8 m- and 5 m-deep mooring stations (SBE 56 thermistor data) and 4 m-deep plankton station (CTD data).
The vertical center of distribution for C. fissus cyprids was determined by calculating their Mean Depth Distribution (MDD, Tapia et al., 2010) for every hour of sampling using the following equation:
A SonTek CastAway-CTD was used to measure temperature and depth profiles every ∼7 min throughout the plankton sampling period at the 4 m deep station (∼280 m from shore; Figure 1). Stratification in this region is primarily driven by thermal variation (Winant and Bratkovich, 1981; Sinnett et al., 2018; Grimes et al., 2020a). Two temperature moorings were deployed for the duration of summer (June 16 to August 31, 2017; May 18 to August 30, 2018) to provide longer temporal-scale context of offshore thermal stratification during periods of plankton sampling: one mooring at the 5 m-deep station (∼400 m from shore and ∼100 m from the 4 m station) and one at the 8 m-deep station (∼800 m from shore and ∼500 m from the 4 m station) during both years (Figure 1). SBE-56 thermistors were deployed at 1 m depth intervals on both moorings, such that the 5 and 8 m moorings had 4 and 6 instruments, respectively, programmed to record temperature every 5 s. Thermal stratification was measured as the change in temperature m–1 (Δ°C m–1) and calculated as follows:
Average stratification was calculated for each station by cruise. The CTD data were used to generate hourly-averaged time series of stratification and thermocline depth (calculated as the depth where the maximum change in temperature occurred) corresponding to each hour of plankton sampling. Additionally, daily-averaged stratification and thermocline depth were calculated from the 5 m-deep temperature mooring data to better understand the hydrographic conditions during the summer months when plankton sampling took place. The water column was considered stratified when Δ°C m–1 ≥ 0.1 (e.g., Sinnett and Feddersen, 2019).
A 1 Mhz Nortek Aquadopp acoustic Doppler current profiler (ADCP) was deployed ∼200 m away from the 4 m deep station at roughly 6 m depth (adjacent to 5 m temperature mooring) to measure current velocities every 90 s in 0.5 m depth intervals (Figure 1). Due to side lobe contamination and inherent instrument limitations, currents at the surface and bottom could not be measured, with the shallowest bin on average 0.26 m below the surface of the water, but sometimes up to 1 m below the surface, and the deepest bin starting at 1.22 m above the bottom (MAB). Current directions were rotated to align with the predominate cross-shore and alongshore directions and separated into cross-shore (u, positive onshore) and alongshore (v, positive southward) components. A 33 h low-pass filter was applied to each component to separate the low- and high-frequency currents. The high-frequency currents include signals from the semidiurnal surface tide and internal tidal variability. Given our goal in understanding how barnacle larvae are transported shoreward from offshore larval development areas to juvenile and adult rocky intertidal habitat, which typically involve vertically sheared flows, we also examined the variability of the cross-shore currents by calculating the standard deviation across depths of the high-frequency cross-shore currents (e.g., Hagerty et al., 2018; Pineda et al., 2018).
The hydrodynamic conditions barnacle cyprids experienced near the time of sampling were estimated with hourly-averages of currents that included the 30 min prior to plankton sampling as well as the ∼30 min duration of plankton sampling (t, the interval for larval flux calculations described below). Given that there was a ∼30 min interval before the next hour of plankton sampling commenced, this resulted in hourly-averaged currents that did not overlap between hours of plankton sampling.
To visualize the hydrodynamic and hydrographic conditions at each station, contour profiles were created from the current meter and temperature data using the contourf function in MATLAB R2021a with 5 contour levels for hourly averages of the currents, and 25 contour levels for the CTD data. The vertical distribution of C. fissus cyprid concentrations (reported as no. larvae m–3) were overlaid on the temperature contour profiles to examine patterns between the physical conditions of the water column and vertical position of cyprids over time.
To examine how cyprid vertical distribution and cross-shore currents influence shoreward larval transport, we calculated cross-shore larval flux. First, cross-shelf exchange flow (in m s–1), u’z,t, where z is the ADCP depth bin, and t is the time corresponding to plankton sampling (as described above), was calculated by subtracting the depth-averaged cross-shore currents from the cross-shore currents (as in Fewings et al., 2008). Then, for each hour of larval sampling, C. fissus cyprid concentration (no. larvae m–3) profiles were interpolated onto the ADCP depth bins, cz,t, to maximize vertical resolution using linear interpolation with the interp1 function in MATLAB R2021a. Finally, cross-shore larval flux (no. larvae m–2 s–1), used to estimate larval transport, was calculated for each hour of plankton sampling, as the depth-average of the product between the cross-shelf exchange flow and the larval concentration:
For each cruise, larval transport was estimated by integrating the hourly larval flux values over the duration of each cruise (as in Rowe and Epifanio, 1994), and dividing by the number of hours sampled for each cruise (reported in m h–1 given the varying cruise lengths). Positive values represent onshore (eastward) larval flux and transport.
To resolve diel vertical distribution patterns, sampling hours were separated into day and night based on the hours of civil twilight, defined as the time in the morning or evening when the geometric center of the sun is 6 degrees below the horizon as defined by the National Weather Service, NOAA. Given that day-night hours were not evenly sampled during each cruise, data from all cruises were combined for these analyses, with n = 48 h sampled during the day and 45 h sampled at night. We used a G–test of goodness-of-fit to determine if there was a significant difference in the vertical distribution of C. fissus cyprids during day vs. night periods using proportions of larvae collected by depth bin. Separate one-way analysis of variance (ANOVA) tests were used to determine if there were day-night differences in the C. fissus MDD, log-transformed concentration of cyprids, and thermocline depth. ANOVA assumptions of normality and homogeneity of variance were met. Day-night differences in larval flux were examined using Kruskal-Wallis tests because the data violated the assumptions of ANOVA.
We examined the degree to which C. fissus cyprid MDD, larval concentration, and thermocline depth were related to thermal stratification using correlation analysis. Visual inspection of the hourly time series revealed that low larval concentration values primarily occurred during cruises with low thermal stratification (e.g., Cruise 4), suggesting that hourly data were not independent. Moreover, the hourly stratification time series from the CTD data for each cruise contained significant autocorrelation. Thus, we focused on examining cruise-to-cruise variability by calculating the correlation between cruise-averaged larval MDD, concentration and stratification.
To understand the more general relationship between the hydrodynamic and hydrographic conditions, we used the time series generated by the ADCP data and the 5 m temperature mooring. The daily-averaged standard deviation of the high-frequency cross-shore currents, thermocline depth, and stratification were calculated from June 16 to July 31 in 2017, and June 1 to July 31 in 2018 to encompass the dates of larval sampling for each year. To remove the significant 1 d autocorrelation in the daily-averaged hydrodynamic and hydrographic records (confirmed by examination of the autocorrelation and partial autocorrelation functions), each time series was subsampled by taking points from every other day. These subsampled data were combined to examine the overall correlation between the daily-averaged standard deviation of the high-frequency cross-shore currents, thermocline depth, and thermal stratification.
While currents changed with depth and over time by cruise, variability was primarily driven by the high-frequency currents, as indicated by how closely the high-frequency currents reproduced the unfiltered current record (Figures 2–6A–F). Low-frequency currents were generally directed westward (offshore) and northward (Cruises 3-5) or with minimal cross-shore currents during Cruises 1 and 2 (Figures 2–6C,D). Alongshore current reversals were also prevalent in the unfiltered (panels ‘B’) and high-frequency (panels ‘F’) currents, with northward flowing (negative values) alternating with southward flowing (positive values) currents, every ∼4–6 h (Figures 2–6B,F). However, the phasing of the currents did not follow tidal sea level variations over time (compare Figures 2–6, with Figure 7). Furthermore, alongshore reversals (Figures 2–6B,F) were not consistently accompanied with cross-shore current reversals during all cruises (Figures 2–6A,E), although in Cruises 1 and 2 (Figures 2, 3), northward currents were generally associated with onshore (positive values) flow (e.g., 2:15 PDT on Figures 2A,B), and southward currents with offshore (negative values) flow (e.g., 2:00 PDT on Figures 3A,B).
Figure 2. Cruise 1 (July 16–17, 2017) currents, temperature and larval concentrations. Contour plots of hourly-averaged currents (ms–1), with (A) unfiltered cross-shore (u) component (positive values corresponding to onshore or eastward flow), (B) unfiltered alongshore (v) component (positive values corresponding to southward flow), (C) low-frequency cross-shore component, (D) low-frequency alongshore component, (E) high-frequency cross-shore component, and (F) high-frequency alongshore component. The thin gray horizontal lines indicate the depths above and below which ADCP data are missing. The black lines indicate the 0 ms–1 contours (G) temperature contour plot using CTD data at 4 m deep site with overlaid black circles representing Chthamalus fissus cyprid concentrations (no. m–3) in each sampling depth bin for each hour of sampling. The white area below the contour plot shows the changing water depth due to the tides, and the filled gray rectangle represents night-time sampling. Hours are in Pacific Daylight Time (PDT).
Figure 3. Cruise 2 (July 25–26, 2017) currents, temperature and C. fissus larval concentrations. Figure details are the same as Figure 2.
Figure 4. Cruise 3 (June 7–8, 2018) currents, temperature and larval concentrations. Figure details are the same as Figure 2. Note that C. fissus larval concentration circles are scaled differently than for Cruises 1, 2, and 4 due to high larval concentrations.
Figure 5. Cruise 4 (June 21–22, 2018) currents, temperature and larval concentrations. Figure details are the same as Figure 2.
Figure 6. Cruise 5 (July 16–17, 2018) currents, temperature and larval concentrations. Figure details are the same as Figure 2. Note that C. fissus larval concentration circles are scaled differently than for Cruises 1, 2, and 4 due to high larval concentrations.
Figure 7. Summary of physical conditions and larval parameters at the 4 m-deep plankton and CTD station during each cruise. Sea level (tidal height), thermal stratification from the CTD data, thermocline depth, C. fissus larval Mean Depth Distribution (MDD), larval concentrations by depth, and cross-shore larval flux (in rows) for all cruises (in columns). Black bars in sea level plots represent night-time sampling. Conditions are stratified when thermal stratification is ≥0.1 Δ°C m–1 (shown as horizontal reference line in stratification plots). Positive values represent onshore (eastward) larval flux.
Cruises during the summer of 2017 (Cruises 1 and 2; Figures 2, 3G) had overall warmer temperatures than those during summer 2018 (Cruises 3-5; Figures 4–6G). At the 4 m deep plankton station, temperatures during Cruises 1 and 2 were similar, between ∼ 22.5 and 24°C, while Cruises 3, 4, and 5 had cooler and more temporally variable temperatures (Figures 2–6G).
C. fissus cyprid concentrations also varied by cruise, with the lowest mean concentrations during Cruises 1, 2, and 4, and one order of magnitude greater concentrations during Cruises 3 and 5 (Table 1). While, cyprid distributions were also variable vertically and on hourly time scales, concentrations did not appear to fluctuate consistently with the currents or temperature (Figures 2–6). For example, during Cruise 1, cyprid concentrations were highest in northward flowing waters when examining the unfiltered and high-frequency currents (e.g., 17:15-19:15 PDT and 0:15-2:15 PDT, Figures 2B,F,G), but this pattern was not evident during Cruise 2 despite the strong alongshore current reversals in the unfiltered and high-frequency records (Figures 3B,F,G). In contrast, at the beginning of Cruise 3 (before 16:00 PDT), cyprid concentrations were highest in cooler temperature mid-depth to bottom waters when high-frequency currents were to the north and onshore (Figures 4E,F,G), but distributions shallowed at the onset of warmer surface waters ∼18:00 PDT near the time of an alongshore and cross-shore current reversal, but eventually resumed a deeper distribution near the end of the cruise when waters were cooler (Figure 4G). Likewise, during Cruise 4, there was an increase in cyprid concentrations after 5:15 PDT, as temperatures gradually cooled during the cruise (Figure 5G). Finally, during Cruise 5, cyprid concentrations increased near the surface ∼ 21:00-23:00 PDT, corresponding to an alongshore current reversal in the unfiltered and high-frequency currents, when flows shifted from southward to northward (Figures 6B,F,G). Cruise 5 temperatures were relatively warm, but experienced slight bottom cooling ∼ 7:00 PDT when we observed increasing cyprid concentrations at depth (Figure 6G). Over all of the cruises, one consistent pattern was that cyprid concentrations were typically greatest in the mid-depth (2–3 m) depth bins (Figure 7).
During all cruises, mean thermal stratification was highest at the 8 m deep station, and decreased closer to shore (5 and 4 m deep stations; Table 1). However, thermal stratification varied hourly at the 4 m deep station, with stratified waters (when values ≥0.1 Δ°Cm–1), occurring 31% of Cruise 1, 79% of Cruise 2, 96% of Cruise 3, 5% of Cruise 4, and 100% of Cruise 5 (Figure 7). Such fluctuations do not appear related to tidal sea level variations (Figure 7). Additionally, within-cruise temporal variations in thermocline depth, C. fissus MDD, cyprid vertical distribution, and cross-shore larval flux did not follow the hourly changes in thermal stratification (Figure 7).
Nonetheless, when data from all cruises were combined, general patterns emerged: there was a significant positive correlation between C. fissus cyprid concentrations and thermal stratification at the 4 m deep station (Pearson’s R = 0.89, p = 0.04; Figure 8). Dates with the highest mean thermal stratification (Cruises 3 and 5), had the largest C. fissus cyprid concentrations (Table 1 and Figure 8). In contrast, Cruise 4 had the lowest mean thermal stratification of all sampling dates and an order of magnitude lower cyprid concentration compared to Cruises 3 and 5 (Table 1 and Figure 8).
Figure 8. Correlation between mean (±SE) thermal stratification and log-transformed total (sum of all depths) C. fissus larval concentrations for each cruise (numbers 1–5).
Estimated cross-shore larval transport was greatest when mean thermal stratification was the highest (Figure 9). Cruises 3 and 5, with the highest stratification, had the highest onshore larval transport (eastward ∼ 0.5–1 m h–1). In fact, shoreward larval transport only resulted when conditions were stratified (Cruises 2, 3, and 5 when mean thermal stratification >0.1 Δ°Cm–1), and was minimal during the predominately unstratified conditions of Cruises 1 and 4 (Figure 9).
Figure 9. Relationship between mean (±SE) thermal stratification and estimated C. fissus cross-shore larval transport for each cruise (numbers 1–5). Positive values represent onshore (eastward) larval transport.
Vertical variations in the daily-averaged high-frequency cross-shore currents were positively correlated with thermal stratification (Pearson’s R = 0.87, p < 0.001; Figure 10). There were no significant correlations between thermocline depth and thermal stratification (p = 0.64), C. fissus MDD and thermocline depth (p = 0.28), MDD and thermal stratification (p = 0.81), and MDD or larval flux and sea level/tidal stage (see Supplementary Material).
Figure 10. Correlation between thermal stratification and the vertical variability of the high-frequency cross-shore currents (standard deviation across depths of the currents). Points are daily-averages sub-sampled every other day in June and July of 2017 and 2018.
The vertical distribution of C. fissus cyprids varied with depth, with the highest proportion found in the 2–3 m depth bin during both day and night periods over all combined cruises (Figure 11A). Despite similarities in this depth bin, there were significant day-night differences in overall depth distributions, with cyprid concentrations decreasing in the bottom-most depth bin, and increasing in the surface-most depth bin at night (G = 18,539.65, df = 3, p < 0.001; Figure 11A). C. fissus MDD day vs. night distributions were also significantly different, with the average ± SE MDD = 2.3 ± 0.07 m slightly deeper during the day, in comparison to 2.1 ± 0.06 m at night (one-way ANOVA: F1,91 = 1.324, p = 0.01). There was a significant difference in the log-transformed C. fissus concentration between day and night (one-way ANOVA: F1, 91 = 2.686, p = 0.008), with higher concentrations during the day than at night (Figure 11B). Overall, average ± SE thermal stratification was slightly higher (0.15 ± 0.01 Δ°C m–1) during the day than at night (0.11 ± 0.01 Δ°Cm–1). These data were not statistically analyzed because the high between-cruise variability in stratification and the larger number of day-time sampling hours during the higher stratification cruises (e.g., Cruises 3 and 5) may have biased the results. There were no significant day-night differences in thermocline depth (p = 0.24) or larval flux (p = 0.25).
Figure 11. Day-night variability in mean (±SE) C. fissus (A) larval vertical distribution, and (B) larval concentration.
We observed a positive relationship between Chthamalus fissus larval concentration and thermal stratification at cruise-to-cruise scales. Furthermore, onshore larval transport was associated with stratification, with little transport when the water column was weakly stratified. These results support our growing understanding of the central importance of thermal stratification on nearshore larval dynamics, as increased thermal stratification distributes cyprids closer to shore (Hagerty et al., 2018), increases nearshore larval abundances (this study), enhances onshore larval transport (this study), and settlement in rocky intertidal habitats (Pineda and López, 2002; Pineda et al., 2018).
Clear patterns between C. fissus cyprid concentrations and currents were not evident. However, in some cases (e.g., Cruises 1, 2, 5) increased larval concentrations appeared to be associated with high-frequency alongshore current reversals. Other studies have noted that alongshore currents can influence larval transport in the nearshore (Wing et al., 2003; Dudas et al., 2009a; Liévana MacTavish et al., 2016). It is possible that strong northward currents promote downwelling (onshore) flow (e.g., Lentz and Fewings, 2012), causing an increase in larval supply. Additionally, in open coastlines, alongshore currents tend to be more energetic and more relevant than anticipated to larval transport by impacting the cross-shore currents (Pineda, 2000; Lentz and Fewings, 2012). Another possibility that warrants future study is that alongshore current reversals at our study site may generate fronts that accumulate larvae on hourly time scales.
Most of the variability in the currents we observed was due to high-frequency (less than 33 h) processes such as surface and internal tides. There was no evidence that the phasing of reversals matched changes in sea level associated with the surface tide. Instead, these reversals might be related to the internal tide, a process that can cause significant cross-shore flows (e.g., Winant and Olson, 1976). Surface tidal currents are weak in southern California, and the high-frequency currents are dominated by the internal tidal variability (Lerczak et al., 2003) which tend to be decoupled in phase from the surface tide. Larvae may aggregate at the same depth where the internal tide causes shoreward flows (e.g., Weidberg et al., 2019), suggesting that larvae have the potential to use these features to mediate transport. A key consideration, however, is that internal wave and tide events near our study area are rare in the nearshore when thermal stratification is weak (e.g., Sinnett and Feddersen, 2019, and N.R and J.P. unpublished data). Thus, stratification may play a key role in the shoreward transport of larvae, as increases in thermal stratification could lead to more energetic cross-shore currents and internal tides that propagate shoreward enhancing larval transport and retention in the nearshore (Pineda, 1999; Weidberg et al., 2019).
Indeed, during periods of greatest thermal stratification, we observed the highest larval concentrations, and onshore larval transport, underlying the importance of understanding how stratification varies in the nearshore. Our results further the findings from a previous study that observed cross-shore cyprid distributions closer to shore when waters were more stratified (Hagerty et al., 2018). Moreover, both stratification and C. fissus larval settlement decreased during the 2016 El Niño (Pineda et al., 2018), indicating that reductions in stratification can disrupt larval supply to intertidal habitats. Increases in stratification limits vertical mixing and promotes sheared flows (Winant and Bratkovich, 1981), including two-way horizontal flows (e.g., Hagerty et al., 2018; Guillam et al., 2020). In fact, we also observed greater vertical variation in the cross-shore currents when the water column was stratified, suggesting more sheared flows occurred during these conditions. Changes in larval vertical distribution (Lloyd et al., 2012; Hagerty et al., 2018; Weidberg et al., 2019) in response to sheared flows could allow larvae to better regulate their horizontal distribution, and their distance from shore (Shanks and Shearman, 2009; Pineda and Reyns, 2018). Our larval flux calculations, which take into account the vertical positioning of cyprids and the cross-shore currents they experienced at each depth, varied on hourly scales, but net estimated larval transport was strongest in the shoreward direction when stratification was greatest. While we did not identify the physical transport mechanisms at play in this study, many nearshore cross-shelf exchange processes are dependent on stratification including internal waves and tides (e.g., Sinnett et al., 2018), and transient rip currents (e.g., Grimes and Feddersen, 2021), and cross-shore flows have the potential to shut down when conditions are unstratified (Lentz, 2001).
From previous work, we know that C. fissus cross-shore distributions were closer to shore, near 4 m depth, when nearshore stratification increased, and this area where cyprid concentrations increased can be thought as a zone of larval accumulation (Hagerty et al., 2018). By continuously sampling at a 4m depth station in the current study, we hypothesize that in addition to larval patchiness, some of the between-cruise variations in larval concentration might be due to shifting of the zone of larval accumulation relative to the position of our fixed sampling station, as nearshore stratification changed spatially. For instance, the very high larval concentrations we observed when stratification was highest throughout the nearshore suggests that our 4 m deep sampling station was within the larval accumulation zone. In contrast, the larval accumulation zone would be further offshore when the nearshore was unstratified (e.g., during Cruise 4 low stratification conditions extended to the 8 m deep station when compared to the other cruises), and could also help explain why larval concentrations were lower during this time. The seafloor offshore of the surfzone in Bird Rock is relatively rough, with scattered ledges. However, there are no large protruding features, with smaller features distributed throughout. Thus, it is unlikely that bottom bathymetry has a large influence on the position of the zone of accumulation compared to stratification. We speculate that higher offshore stratification allows the thermocline to penetrate into the 4 m deep station, thereby enabling larval transport closer to shore. A follow-up study should test the hypothesis that spatial variation in stratification influences where larvae accumulate in the nearshore.
Regardless of the time of day, ∼40% of cyprids remained within the 2–3 m depth bin. This pattern is consistent with those found in other studies where cyprids of intertidal species remained slightly above the bottom. For instance, Tapia et al. (2010) found that cyprids were usually most abundant between 15 and 25 m depth at a station that was 30 m deep, Bonicelli et al. (2016) found cyprids also remained near the bottom at a 20 m site, and Hagerty et al. (2018) observed cyprids distributed at depths near the bottom at stations along a transect extending from 4 to 12 m deep. Such depth distributions might allow cyprids to use cool, deep internal bores to transport closer to shore before reaching the intertidal zone (Pineda, 1991; Shanks et al., 2014; Fernández-Aldecoa et al., 2019). It is possible that deep waters are preferable for the non-feeding cyprids because cooler waters extend the lifespan of their lipid reserves, providing them with more time to reach intertidal habitats and increase their chances of successful settlement (see Satuito et al., 1996). However, cyprids of some barnacle subtidal species may occur in surface waters (e.g., Le Fèvre and Bourget, 1991) and vertical distribution might relate to adult habitat.
The significant day-night difference in C. fissus distribution was driven by concentration changes in the bottom and surface depth bins. At night, cyprids migrated away from the bottom and increased their distribution near the surface. While C. fissus MDD also shallowed slightly at night, the MDD calculations mask changes that occur at the tails of the cyprid distribution (near-bottom and near-surface). Nonetheless, chthamalid cyprid distributions were also slightly shallower at night off the coast of Chile (Bonicelli et al., 2016) and Portugal (dos Santos et al., 2007). Since cyprids are non-feeding, this migration is not to track prey but could be to avoid visual predators (reviewed in Haney, 1988), which is a common response of meroplankton in deeper waters (Zaret and Suffern, 1976; Forward and Rittschof, 2000). It is unclear whether the diel changes in cyprid distribution that we observed have implications for nearshore larval transport as there were no day-night differences in larval flux. In coastal locations off Brittany (France), brittle star larvae that undergo a nocturnal upward migration in a stratified embayment experience retention, as shoreward currents are generated at night by the stratification dynamics (Guillam et al., 2020).
At our study site, thermal stratification might break down at night due to diurnal cooling (e.g., Grimes et al., 2020b), but we could not test for day-night differences in stratification because the larger number of day-time sampling hours during the higher stratification cruises may have introduced bias. Possible day-night variations in thermal stratification, coupled with the significant positive relationship we observed between larval concentrations and stratification may help explain why larval concentrations were significantly higher during the day than at night. However, characterizing variations in thermal stratification in the nearshore is complicated because it is modulated by many physical processes (e.g., surface and internal waves and tides, diurnal heating/cooling, seasons, El Niño), operating on a range of temporal scales (Sinnett et al., 2018; Sinnett and Feddersen, 2019; Grimes and Feddersen, 2021). Therefore, describing the dependencies of larval transport on stratification, a unifying factor in the dynamics of many physical processes, may be one way to simplify understanding the complex problem of benthic recruitment.
We observed dynamic nearshore larval distributions, changing at the scale of hours, possibly as a response to varying physical conditions in the water column in addition to larval patchiness. Larval distributions did not change on hourly scales in response to thermal stratification; however, cruises with higher stratification yielded higher larval concentrations and onshore larval transport. This suggests that cruise-to-cruise variability in stratification is more important than within-cruise variability, and provides insight as to the relevant temporal scales (days rather than hours) over which stratification operates to influence larval dynamics in the nearshore. While variation in thermal stratification did not alter larval vertical distribution on hourly scales, the high-frequency, depth-resolved sampling undertaken in this study made it possible to calculate larval flux during each cruise, and identify the relationship between onshore larval transport and thermal stratification. Although a specific mechanism was not resolved, our results suggest that nearshore thermal stratification is key for onshore larval transport, likely driven in part by the high-frequency cross-shore currents. Finally, this study showed that C. fissus cyprid larvae undertake diel changes in their vertical distribution, with larvae generally slightly shallower at night. Both behavior and physical processes appear to play an important role in facilitating accumulation of barnacle cyprids in shallow waters and successful onshore transport, with implications for larval supply and recruitment to intertidal habitats.
The raw data supporting the conclusions of this article will be made available by the authors, without undue reservation.
GY contributed to field collections, sample processing, data analyses, and writing of the manuscript. NR and JP conceived of the study, and contributed to field collections, writing of the manuscript and funding. All authors contributed to manuscript revisions and approved the submitted version.
This study was funded by the National Science Foundation under grants OCE-1357290, OCE-1357327, OCE-1630459, and OCE-1630474.
The authors declare that the research was conducted in the absence of any commercial or financial relationships that could be construed as a potential conflict of interest.
All claims expressed in this article are solely those of the authors and do not necessarily represent those of their affiliated organizations, or those of the publisher, the editors and the reviewers. Any product that may be evaluated in this article, or claim that may be made by its manufacturer, is not guaranteed or endorsed by the publisher.
This material contributed to GY’s MS Thesis at the University of San Diego (Yamhure, 2020). We thank M. Swiderski, A. Basilio, R. Harrel, and D. Steward for help with 24 h sampling. Supplies and travel support were also provided by the University of San Diego and Woods Hole Oceanographic Institution. J. Prairie and S. Lentz gave valuable feedback on earlier drafts of the manuscript, and V. Starczak provided statistical guidance.
The Supplementary Material for this article can be found online at: https://www.frontiersin.org/articles/10.3389/fmars.2021.748389/full#supplementary-material
Bonicelli, J., Tyburczy, J., Tapia, F. J., Finke, G. R., Parragué, M., Dudas, S., et al. (2016). Diel vertical migration and cross-shore distribution of barnacle and bivalve larvae in the central Chile inner-shelf. J. Exp. Mar. Biol. Ecol. 485, 35–46. doi: 10.1016/j.jembe.2016.08.013
Brown, S. K., and Roughgarden, J. (1985). Growth, morphology, and laboratory culture or larvae of Balanus glandula (Cirripedia: Thoracica). J. Crustacean Biol. 5, 574–570. doi: 10.2307/1548236
Chia, F. S., Buckland-Nicks, J., and Young, C. M. (1984). Locomotion of marine invertebrate larvae: a review. Can. J. Zool. 62, 1205–1222. doi: 10.1139/z84-176
Cowen, R. K., and Sponaugle, S. (2009). Larval dispersal and marine population connectivity. Ann. Rev. Mar. Sci. 1, 44–66. doi: 10.1146/annurev.marine.010908.163757
Daigle, R. M., and Metaxas, A. (2011). Vertical distribution of marine invertebrate larvae in response to thermal stratification in the laboratory. J. Exp. Mar. Biol. Ecol. 409, 89–98. doi: 10.1016/j.jembe.2011.08.008
DiBacco, C., Fuchs, H., Pineda, J., and Helfrich, K. (2011). Swimming behavior and velocities of barnacle cyprids in a downwelling flume. Mar. Ecol. Prog. Ser. 433, 131–148. doi: 10.3354/meps09186
dos Santos, A., Santos, A. M. P., and Conway, D. V. P. (2007). Horizontal and vertical distribution of cirripede cyprid larvae in an upwelling system off the Portuguese coast. Mar. Ecol. Prog. Ser. 329, 145–155. doi: 10.3354/meps329145
dos Santos, A., Santos, A. M. P., Conway, D. V. P., Bartilotti, C., Lourenço, P., and Queiroga, H. (2008). Diel vertical migration of decapod larvae in the Portuguese coastal upwelling ecosystem: implications for offshore transport. Mar. Ecol. Prog Ser. 359, 171–183. doi: 10.3354/meps07341
Dudas, S. E., Rilov, G., Tyburczy, J. A., and Menge, B. A. (2009b). Linking larval abundance, onshore supply and settlement using instantaneous and integrated methods. Mar. Ecol. Prog. Ser. 387, 81–95.
Dudas, S. E., Grantham, B. A., Kirincich, A. R., Menge, B. A., Lubchenco, J., and Barth, J. A. (2009a). Current reversals as determinants of intertidal recruitment on the central Oregon coast. ICES J. Mar. Sci. 66, 396–407. doi: 10.1093/icesjms/fsn179
Fernández-Aldecoa, R. G., Ladah, L. B., Morgan, S. G., Dibble, C. D., Solana-Arellano, E., and Filonov, A. (2019). Delivery of zooplankton to the surf zone during strong internal tidal forcing and onshore winds in Baja California. Mar. Ecol. Prog. Ser. 625, 15–26.
Fewings, M., Lentz, S. J., and Fredericks, J. (2008). Observations of cross-shore flow driven by cross-shore winds on the inner continental shelf. J. Phys. Oceanogr. 38, 2358–2378. doi: 10.1175/2008JPO3990.1
Forward, R. B., and Rittschof, D. (2000). Alteration of photoresponses involved in diel vertical migration of a crab larva by fish mucus and degradation products of mucopolysaccharides. J. Exp. Mar. Biol. Ecol. 245, 277–292. doi: 10.1016/s0022-0981(99)00169-0
Gray, C. A., and Kingsford, M. J. (2003). Variability in thermocline depth and strength, and relationships with vertical distributions of fish larvae and mesozooplankton in dynamic coastal waters. Mar. Ecol. Prog. Ser. 247, 197–210. doi: 10.3354/meps247211
Griffin, D. A., and Middleton, J. H. (1992). Upwelling and internal tides over the inner New South Wales continental shelf. J. Geophys. Res. 97, 14389–14405. doi: 10.1029/92JC01294
Grimes, D. J., and Feddersen, F. (2021). The self-similar stratified inner-shelf response to transient rip-current-induced mixing. J. Fluid Mech. 915:A82. doi: 10.1017/jfm.2021.140
Grimes, D. J., Feddersen, F., and Kumar, N. (2020a). Tracer exchange across the stratified inner-shelf driven by transient rip-currents and diurnal surface heat fluxes. Geophys. Res. Lett. 47:e2019GL086501. doi: 10.1029/2019GL086501
Grimes, D. J., Feddersen, F., Giddings, S. N., and Pawlak, G. (2020b). Cross-shore deformation of a surfzone-released dye plume by an internal tide on the inner shelf. J. Phys. Oceanogr. 50, 35–54. doi: 10.1175/jpo-d-19-0046.1
Guillam, M., Bessin, C., Blanchet-Aurigny, A., Cugier, P., Nicolle, A., and Thiébaut, É, et al. (2020). Vertical distribution of brittle star larvae in two contrasting coastal embayments: implications for larval transport. Sci. Rep. 10:12033. doi: 10.1038/s41598-020-68750-4
Hagerty, M., Reyns, N., and Pineda, J. (2018). Constrained nearshore larval distributions and thermal stratification. Mar. Ecol. Prog. Ser. 595, 105–122. doi: 10.3354/meps12561
Hagerty, M., Reyns, N., Pineda, J., and Govindarajan, A. (2019). Diversity and distribution of nearshore barnacle cyprids in Southern California through the 2015-16 El Niño. PeerJ. 7:e7186. doi: 10.7717/peerj.7186
Hargenrader, C. (2018). The Temporal and Spatial Dynamics of Larval Supply, Settlement, and Adult Populations of Chthamalus Fissus Within the La Jolla, California Rocky Intertidal. M.S. thesis. San Diego: University of San Diego.
Kumar, N., and Feddersen, F. (2017). A new offshore transport mechanism for shoreline-released tracer induced by transient rip currents and stratification. Geophys. Res. Lett. 44, 2843–2851.
Le Fèvre, J., and Bourget, E. (1991). Neustonic niche for cirripede larvae as a possible adaptation to long-range dispersal. Mar. Ecol. Prog. Ser. 74, 184–194.
Lentz, S. J. (2001). The influence of stratification on the wind-driven cross-shelf circulation over the North Carolina shelf. J. Phys. Oceanogr. 31, 2749–2760. doi: 10.1175/1520-04852001031.2
Lentz, S. J., and Fewings, M. R. (2012). The wind and wave-driven inner-shelf circulation. Ann. Rev. Mar. Sci. 4, 317–343. doi: 10.1146/annurev-marine-120709-142745
Lerczak, J. A., Winant, C. D., and Hendershott, M. C. (2003). Observations of the semidiurnal internal tide on the southern California slope and shelf. J. Geophys. Res. 108, 9–20. doi: 10.1029/2001JC001128
Lewis, C. A. (1975). Development of the gooseneck barnacle Pollicipes polymerus (Cirripedia: Lepadomorpha): fertilization through settlement. Mar. Biol. 32, 141–153. doi: 10.1007/BF00388507
Liévana MacTavish, A., Ladah, L., Lavín, M., Filonov, A., Tapia, F. J., and Leichter, J. (2016). High frequency (hourly) variation in vertical distribution and abundance of meroplanktonic larvae in nearshore waters during strong internal tidal forcing. Cont. Shelf Res. 117, 92–99.
Lloyd, M. J., Metaxas, A., and deYoung, B. (2012). Patterns in vertical distribution and their potential effects on transport of larval benthic invertebrates in a shallow embayment. Mar. Ecol. Prog. Ser. 469, 37–52.
Miller, K. M., and Roughgarden, J. (1994). Description of the larvae of Tetraclita rubescens and Megabalanus californicus with a comparison of the common barnacle larvae of the central California coast. J. Crustacean Biol. 14, 579–600. doi: 10.2307/1549003
Miller, K. M., Blower, S. M., Hedgecock, D., and Roughgarden, J. (1989). Comparison of larval and adult stages of Chthamalus dalli and Chthamalus fissus. J. Crustacean Biol. 9, 242–256.
Morgan, S. G., Fisher, J. L., Miller, S. H., McAfee, S. T., and Largier, J. L. (2009). Nearshore larval retention in a region of strong upwelling and recruitment limitation. Ecology 90, 3489–3502. doi: 10.1890/08-1550.1
Morgan, S. G., Miller, S. H., Robart, M. J., and Largier, J. L. (2018). Nearshore larval retention and cross-shelf migration of benthic crustaceans at an upwelling center. Front. Mar. Sci. 5:161. doi: 10.3389/fmars.2018.00161
Pfaff, M. C., Branch, G. M., Fisher, J. L., Hoffmann, V., Ellis, A. G., and Largier, J. L. (2015). Delivery of marine larvae to shore requires multiple sequential transport mechanisms. Ecology 96, 1399–1410. doi: 10.1890/14-0229.1
Pineda, J. (1991). Predictable upwelling and shoreward transport of planktonic larvae by internal tidal bores. Science 253, 548–551.
Pineda, J. (1994). Spatial and temporal patterns in barnacle settlement along a southern California rocky shore. Mar. Ecol. Prog. Ser. 107, 125–138. doi: 10.3354/meps107125
Pineda, J. (1999). Circulation and larval distribution in internal tidal bore warm fronts. Limnol. Oceanogr. 44, 1400–1414.
Pineda, J. (2000). Linking larval settlement to larval transport: assumptions, potentials, and pitfalls. Oceanogr. East. Pac. 1, 84–105.
Pineda, J., and López, M. (2002). Temperature, stratification and barnacle larval settlement in two Californian sites. Cont. Shelf Res. 22, 1183–1198. doi: 10.1016/S0278-4343(01)00098-X
Pineda, J., and Reyns, N. (2018). “Larval transport in the coastal zone: biological and physical processes,” in Evolutionary Ecology of Marine Invertebrate Larvae, eds T. J. Carrier, A. M. Reitzel, and A. Heyland (Oxford: Oxford University Press), 141–159.
Pineda, J., Hare, J. A., and Sponaugle, S. (2007). Larval dispersal and transport in the coastal ocean and consequences for population connectivity. Oceanography 20, 22–39. doi: 10.5670/oceanog.2007.27
Pineda, J., Reyns, N., and Lentz, S. (2018). Reduced barnacle larval abundance and settlement in response to large-scale oceanic disturbances: temporal patterns, nearshore thermal stratification, and potential mechanisms. Limnol. Oceanogr. 63, 2618–2629. doi: 10.1002/lno.10964
Porri, F., McQuaid, C. D., and Radloff, S. (2006). Spatio-temporal variability of larval abundance and settlement of Perna perna: differential delivery of mussels. Mar. Ecol. Progr. Ser. 315, 141–150.
Rowe, P. M., and Epifanio, C. E. (1994). Flux and transport of larval weakfish in Delaware Bay, USA. Mar. Ecol. Progr. Ser. 110, 115–120.
Satuito, C. G., Shimizu, K., Natoyama, K., Yamazaki, M., and Fusetani, N. (1996). Age-related settlement success by cyprids of the barnacle Balanus amphitrite, with special reference to consumption of cyprid storage protein. Mar. Biol. 127, 125–130. doi: 10.1007/BF00993652
Shanks, A. L. (2001). An Identification Guide to the Larval Marine Invertebrates of the Pacific Northwest. Corvallis, OR: Oregon State University Press.
Shanks, A. L., and Shearman, R. K. (2009). Paradigm lost? Cross-shelf distributions of intertidal invertebrate larvae are unaffected by upwelling or downwelling. Mar. Ecol. Progr. Ser. 385, 189–204. doi: 10.3354/Meps08043
Shanks, A. L., McCulloch, A., and Miller, J. (2003). Topographically generated fronts, very nearshore oceanography and the distribution of larval invertebrates and holoplankters. J. Plankton Res. 25, 1251–1277. doi: 10.1093/plankt/fbg090
Shanks, A. L., Morgan, S. G., Macmahan, J., Reniers, A. J. H. M., Jarvis, M., Brown, J., et al. (2014). Onshore transport of plankton by internal tides and upwelling-relaxation events. Mar. Ecol. Progr. Ser. 502, 39–51. doi: 10.3354/meps10717
Sinnett, G., and Feddersen, F. (2019). The nearshore heat budget: effects of stratification and surfzone dynamics. J. Geophys. Res. 124, 8219–8240. doi: 10.1029/2019JC015494
Sinnett, G., Feddersen, F., Lucas, A. J., Pawlak, G., and Terrill, E. (2018). Observations of nonlinear internal wave runup to the surfzone. J. Phys. Oceanogr. 48, 531–554. doi: 10.1175/JPO-D-17-0210.1
Tapia, F., and Pineda, J. (2007). Stage-specific distribution of barnacle larvae in nearshore waters: potential for limited dispersal and high mortality rates. Mar. Ecol. Progr. Ser. 342, 177–190.
Tapia, F., DiBacco, C., Jarrett, J. N., and Pineda, J. (2010). Vertical distribution of barnacle larvae at a fixed nearshore station in southern California: stage-specific and diel patterns. Estuar. Coast. Shelf Sci. 86, 265–270. doi: 10.1016/j.ecss.2009.11.003
Thièbaut, E., Dauvin, J. C., and Lagadeuc, Y. (1992). Transport of Owenia fusiformis larvae (Annelida: Polychaeta) in the Bay of Seine. I. Vertical distrubution in relation to water column stratification and ontogenic vertical migration. Mar. Ecol. Progr. Ser. 80, 29–39.
Vedder, K., and Branscomb, E. S. (1982). A description of the naupliar stages of the barnacles, Balanus glandula Darwin, Balanus cariosus Pallas, and Balanus crena Tus Bruguière (Cirripedia, Thoracica). Crustaceana 42, 83–95. doi: 10.1163/156854082X00722
Weidberg, N., Goschen, W., Jackson, J. M., Pattrick, P., McQuaid, C. D., and Porri, F. (2019). Fine scale depth regulation of invertebrate larvae around coastal fronts. Limnol. Oceanogr. 64, 785–802. doi: 10.1002/lno.11074
Winant, C. D., and Bratkovich, A. (1981). Temperature and currents in the southern California shelf: a description of the variability. J. Phys. Oceanogr. 11, 71–86. doi: 10.1175/1520-048519810112
Winant, C. D., and Olson, J. R. (1976). The vertical structure of coastal currents. Deep-Sea Res. 23, 925–936.
Wing, S. R., Botsford, L. W., Morgan, L. E., Diehl, J. M., and Lundquist, C. J. (2003). Inter-annual variability in larval supply to populations of three invertebrate taxa in the northern California Current. Estuar. Coast. Shelf Sci. 57, 859–872.
Wong, J. Y., Chan, B. K. K., and Chan, K. Y. K. (2020). Swimming kinematics and hydrodynamics of barnacle larvae throughout development. Proc. R. Soc. 287:20201360. doi: 10.1098/rspb.2020.1360
Yamhure, G. (2020). Nearshore Vertical Distribution Of Barnacle Cyprids: Temporal Patterns and Hydrographic Variability. M.S. thesis. San Diego: University of San Diego.
Keywords: larval vertical distribution, thermocline, larval transport, Chthamalus fissus, diel cycles, nearshore
Citation: Yamhure GM, Reyns N and Pineda J (2021) High Larval Concentrations and Onshore Transport of Barnacle Cyprids Associated With Thermal Stratification. Front. Mar. Sci. 8:748389. doi: 10.3389/fmars.2021.748389
Received: 27 July 2021; Accepted: 04 October 2021;
Published: 02 November 2021.
Edited by:
Francesca Porri, South African Institute for Aquatic Biodiversity, South AfricaReviewed by:
Luis Gimenez, Bangor University, United KingdomCopyright © 2021 Yamhure, Reyns and Pineda. This is an open-access article distributed under the terms of the Creative Commons Attribution License (CC BY). The use, distribution or reproduction in other forums is permitted, provided the original author(s) and the copyright owner(s) are credited and that the original publication in this journal is cited, in accordance with accepted academic practice. No use, distribution or reproduction is permitted which does not comply with these terms.
*Correspondence: Nathalie Reyns, bnJleW5zQHNhbmRpZWdvLmVkdQ==
Disclaimer: All claims expressed in this article are solely those of the authors and do not necessarily represent those of their affiliated organizations, or those of the publisher, the editors and the reviewers. Any product that may be evaluated in this article or claim that may be made by its manufacturer is not guaranteed or endorsed by the publisher.
Research integrity at Frontiers
Learn more about the work of our research integrity team to safeguard the quality of each article we publish.