- 1Department of Geosciences, University of Padova, Padua, Italy
- 2Department of Environmental Sciences, Informatics, and Statistics, Ca’ Foscari University of Venice, Venice, Italy
- 3Department of Earth Sciences, University of Firenze, Firenze, Italy
- 4Unité Mixte de Recherche 7194 du Centre National de la Recherche Scientifique, Muséum National d’Histoire Naturelle, Department Homme et Environnement, Paris, France
- 5National Research Council of Italy (CNR), Institute of Geosciences and Earth Resources, Padua, Italy
- 6Swiss Federal Institute of Technology (ETH) Zürich, Geological Institute, Zurich, Switzerland
- 7Institute of Marine Science (ISMAR), National Research Council of Italy (CNR), Bologna, Italy
- 8National Institute for Nuclear Physics (INFN), Section of Firenze, Sesto Fiorentino, Italy
- 9Department of Chemistry Ugo Schiff, University of Firenze, Sesto Fiorentino, Italy
Many salt-marsh systems worldwide are currently threatened by drowning and lateral erosion that are not counteracted by sufficient sediment supply. Here we analyze the response of a salt-marsh system to changes in sediment availability and show that, contrary to what would have been expected, marsh dynamics in the vertical plane can be insensitive to large sediment supply. We integrate sedimentological, geochronological, paleoecological, geophysical, and chemical analyses of salt-marsh sediments accumulated over the past six centuries in the Southern Venice Lagoon (Italy), and suggest that a time lag exists between enhanced river-fed clastic sediment input and its signature in the salt-marsh succession. This time lag is likely caused by the stocking of the sediment along the margins of pre-existing marshes, which started to significantly expand horizontally – rather than accrete vertically – when sediment input increased. When sediment input drastically decreased, wind waves re-suspended the river-fed deposits and distributed them over the marsh platform, eventually allowing for vertical accretion. Understanding the response of salt-marsh systems to changes in sediment supply has important implications for the management of tidal landscapes and the prediction of their evolution under the effects of natural and anthropogenic forcings. Our results highlight that the study of ultra-recent sedimentary successions needs to be carried out on the basis of a deep understanding of specific depositional dynamics.
Introduction
Salt marshes are valuable ecosystems of great social, economical, ecological, and geomorphological importance (Barbier et al., 2011). The accumulation of inorganic and organic sediments (e.g., Morris et al., 2002; D’Alpaos et al., 2007; Mudd et al., 2009; Roner et al., 2016) allows salt marshes to face rates of relative sea-level rise (RSLR) up to a given threshold (Kirwan et al., 2010, 2016; D’Alpaos et al., 2011) and eventually to reach biogeomorphic equilibrium conditions (D’Alpaos, 2011; Kirwan and Megonigal, 2013; Marani et al., 2013; Roner et al., 2016). In particular, halophytic vegetation species colonizing tidal marshes contribute to marsh vertical accretion by enhancing mineral deposition, through direct capture of sediment particles (e.g., Leonard and Luther, 1995; Li and Yang, 2009) and via reduction of turbulence kinetic energy (e.g., Leonard and Croft, 2006; Mudd et al., 2010), as well as organic sedimentation due to root growth and litter deposition (e.g., Nyman et al., 2006; Neubauer, 2008). The elevation of marsh surface, in turn, affects vegetation productivity (e.g., Morris et al., 2002), in this way closing the bio-geomorphic feedback. Salt-marsh accretion is mainly driven by inorganic accumulation in more elevated and better-aerated soils typically found along the marsh edges (e.g., Marani et al., 2006; Boaga et al., 2014), while the organic component becomes important in the low-lying inner part of marshes (e.g., D’Alpaos, 2011; Roner et al., 2016).
The effects of natural changes and human interference on the subtle equilibrium between vertical accretion and rates of RSLR have often resulted in irreversible transformations, leading to a significant decrease in salt-marsh extent worldwide during the last century (Castillo et al., 2000; Carniello et al., 2009; Gedan et al., 2009; FitzGerald and Hughes, 2019). High rates of RSLR and the lack of clastic sediments are key factors driving salt-marsh drowning worldwide (Morris et al., 2002; Marani et al., 2007; Gedan et al., 2009; Valiela et al., 2009; Mudd, 2011; D’Alpaos and Marani, 2016), whereas the effect of wind-wave erosion on salt-marsh margins has been highlighted as the main process responsible for their lateral retreat (Mariotti and Fagherazzi, 2010, 2013; Marani et al., 2011; Leonardi and Fagherazzi, 2014; Leonardi et al., 2016; Finotello et al., 2020). Other second-order processes, such as for example the establishment and expansion of salt pans and ponds, can also lead to significant loss of marsh surfaces (e.g., Mariotti, 2016; Ortiz et al., 2017; Schepers et al., 2020; Wang et al., 2021).
Recent mathematical modeling suggests that salt-marsh topography, and the related effects on biological productivity and vertical accretion, could adjust to century-scale RSLR with a lag of several decades (Allen, 1995; Kirwan and Murray, 2008; D’Alpaos et al., 2011). Accordingly, salt-marsh elevation and accretion rates might currently be out of equilibrium with modern rates of RSLR, reflecting environmental conditions developed over previous decades (Kirwan and Murray, 2008). Variations in sediment supply are likely to have similar effects on the stability of marshlands (Kirwan and Temmerman, 2009; Kirwan et al., 2011). Therefore, a time lag is expected between changes in sediment availability and new salt-marsh equilibrium conditions in the vertical frame (D’Alpaos et al., 2011), though field shreds of evidence for such behavior are still missing. On the contrary, salt-marsh dynamics in the horizontal plane appears to respond faster to changes in external forcings, chief among which are wind waves and, yet again, external sediment supply (Marani et al., 2011; Leonardi et al., 2016; Ladd et al., 2019; Finotello et al., 2020). For instance, Kirwan et al. (2011) suggested that, during the European settlement, salt marshes along the North American coast underwent a marked and rapid expansion due to increased sediment supply triggered by human-made deforestation, before they started retreating toward a pre-settlement equilibrium.
Similarly to other coastal systems worldwide (Day et al., 2000; Gedan et al., 2009), the Venice Lagoon (Italy) is currently threatened by a severe loss of marshlands, with a decrease of natural salt marshes from ca. 255 km2 in AD 1611 to 43 km2 in AD 2010 (Carniello et al., 2009; D’Alpaos, 2010; Tommasini et al., 2019). Such a decrease was mainly observed in the Southern Lagoon due to a severe reduction in sediment supply following repeated man-made diversions of the Brenta River outside the Lagoon, carried out to avoid the siltation of the lagoonal basin. All these changes, which are recorded in historical documents (D’Alpaos, 2010; Bondesan and Furlanetto, 2012) and preserved in the stratigraphic record (Roner et al., 2017), make the Southern portion of the Venice Lagoon a unique laboratory to understand the evolution of salt marshes under the effects of changing sediment supply.
In this paper, we use a multi-proxy approach that combines sedimentological, geochronological, paleoecological, geophysical, and chemical analyses of salt-marsh deposits accumulated over the past six centuries to unravel the response of salt-marsh platforms to changes in sediment supply in the vertical and horizontal planes. The comparison between different datasets shows a substantial time lag between the increase in river-fed clastic sediment input and its signatures in salt-marsh sedimentary successions. Understanding the response of salt marshes to changes in external sediment supply has broad implications for managing tidal landscapes and predicting their evolution under the effects of natural and anthropogenic-induced morphodynamic changes.
Geomorphological Setting: The Southern Venice Lagoon and the Brenta River
The Venice Lagoon is an elongated waterbody located in the northwestern Adriatic Sea, characterized by an area of about 550 km2, a semi-diurnal micro-tidal regime (maximum water excursions of ±70 cm around mean sea level and average tidal range of about 1.0 m), and a mean water depth over tidal flats of about 1.5 m. The Lagoon is connected to the Adriatic Sea by the Lido, Malamocco, and Chioggia inlets from North to South. The study area (Punta Cane) is located in the Southern portion of the Lagoon, about 10 km SW of the Malamocco inlet (Figures 1A,B).
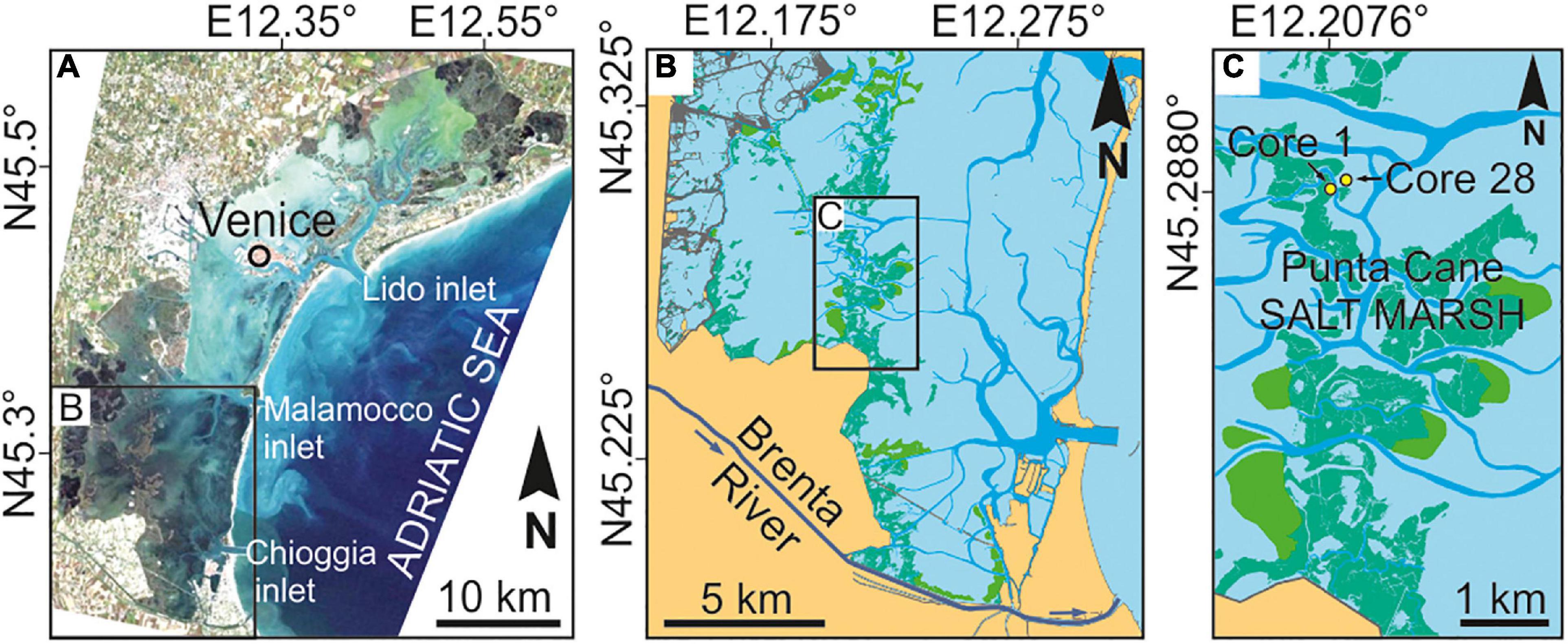
Figure 1. Geomoprohlogical setting and study area. (A) Location of the study area in the Southern Venice Lagoon. (B) Punta Cane salt-marsh area and the current path of the Brenta River. (C) Location of the two sedimentary cores analyzed in this study, located in the Punta Cane salt-marsh area.
The Southern Venice Lagoon hosts a ∼20 m thick Holocene sedimentary succession, which overlays Pleistocene alluvial deposits accumulated during the Last Glacial Maximum. Modern lagoonal sedimentation, associated with the evolution of tidal channels, tidal flats, subtidal platforms, and salt marshes, started to accumulate ca. 2,000 years ago (Zecchin et al., 2009). The volume of clastic sediments supplied to the Southern Lagoon during the last millennium was essentially controlled by the Brenta River, a 174 km long river draining the Dolomites (Southern Italian Alps). Carbonates (dolomite), gneiss, phyllite, granite, and volcanic rocks (e.g., andesite and rhyolite) are the predominant rocks exposed in the upper part of the Brenta River drainage basin, while carbonates are found in the lower basin (Surian and Cisotto, 2007). During the past centuries, the Brenta River was repeatedly diverted inside and outside the Venice Lagoon (Figure 2; see also D’Alpaos, 2010). Specifically, the river fed the Lagoon in the Punta Cane area during two time periods, hereinafter named BR1 and BR2, going (i) from AD 1457 to 1548 (BR1), when two different riverine inlets were sequentially activated, and (ii) from AD 1840 to 1896 (BR2). Given the proximity to the former outlet of the Brenta River (Figures 1B,C, 2), the salt-marsh sedimentary succession of the Punta Cane area represents a unique archive to investigate the effects of variations in clastic sediment supply on salt-marsh evolution.
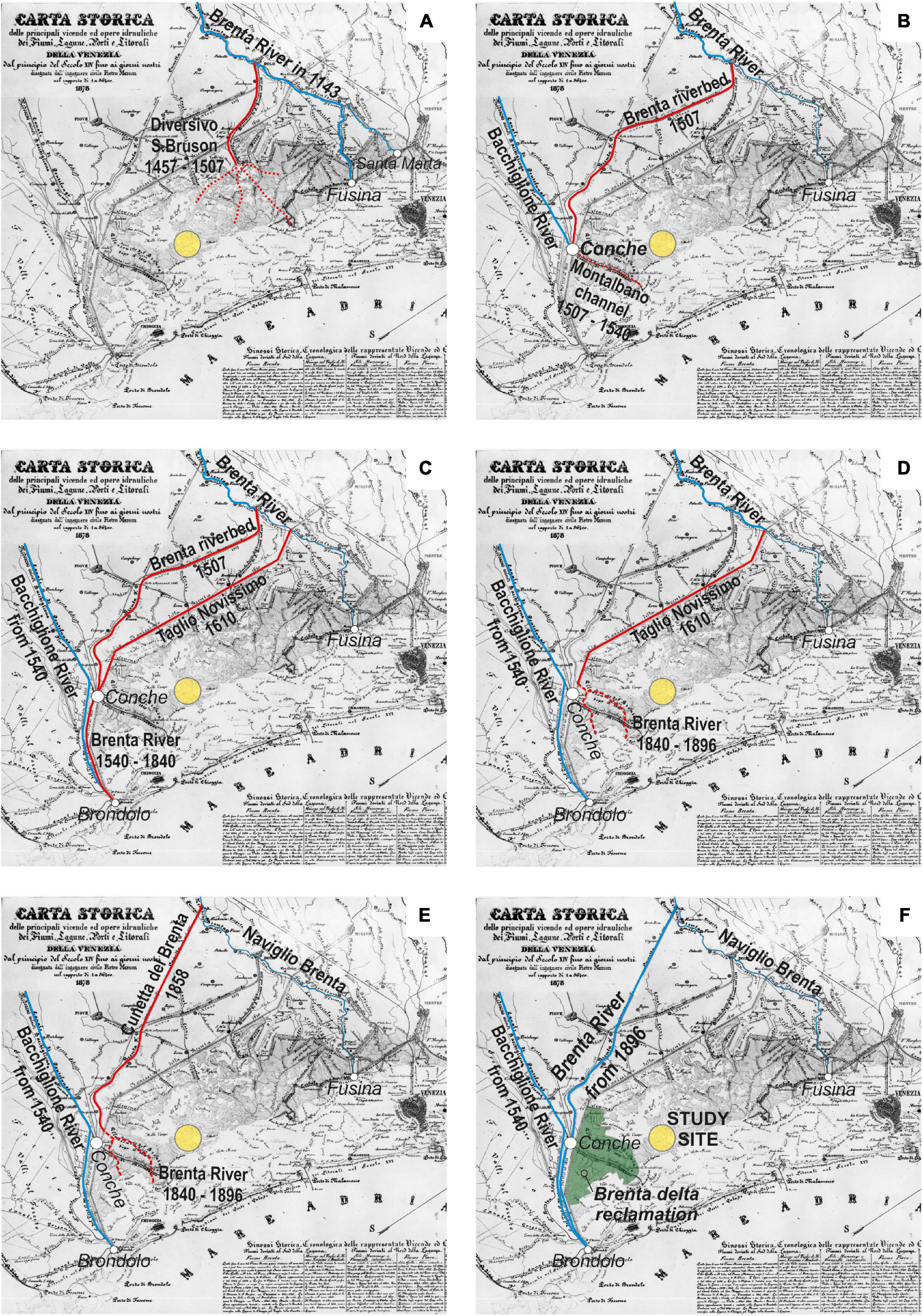
Figure 2. The six panels (A–F) show the evolution of the Brenta River over the past millennium, reconstructed from the available historical maps (adapted from D’Alpaos, 2010). Dates are reported in each individual panel, where red lines show the Brenta River diversions and the yellow dot indicates the Punta Cane area. In (F) the green area represents the extent of the Brenta River delta reclamation at the beginning of the 20th century after its last introduction into the Venice Lagoon (AD 1840–1896, see E).
Materials and Methods
In the Punta Cane area, salt-marsh deposits are about 1.80 m thick and cover a palustrine unit (minimum thickness 2 m) made up of peat with abundant reed fragments. A high-resolution (i.e., decadal-scale) age model for this succession was recently proposed by Roner et al. (2017) integrating radiocarbon dating and 210Pb and 137Cs analyses. The model revealed that salt-marsh deposition began around AD 1350, and allowed us to identify the BR1 phase between −155 and −115 cm below the present-day salt-marsh surface, as well as to highlight the signatures of the BR2 phase between −65 and −60 cm (Figure 3).
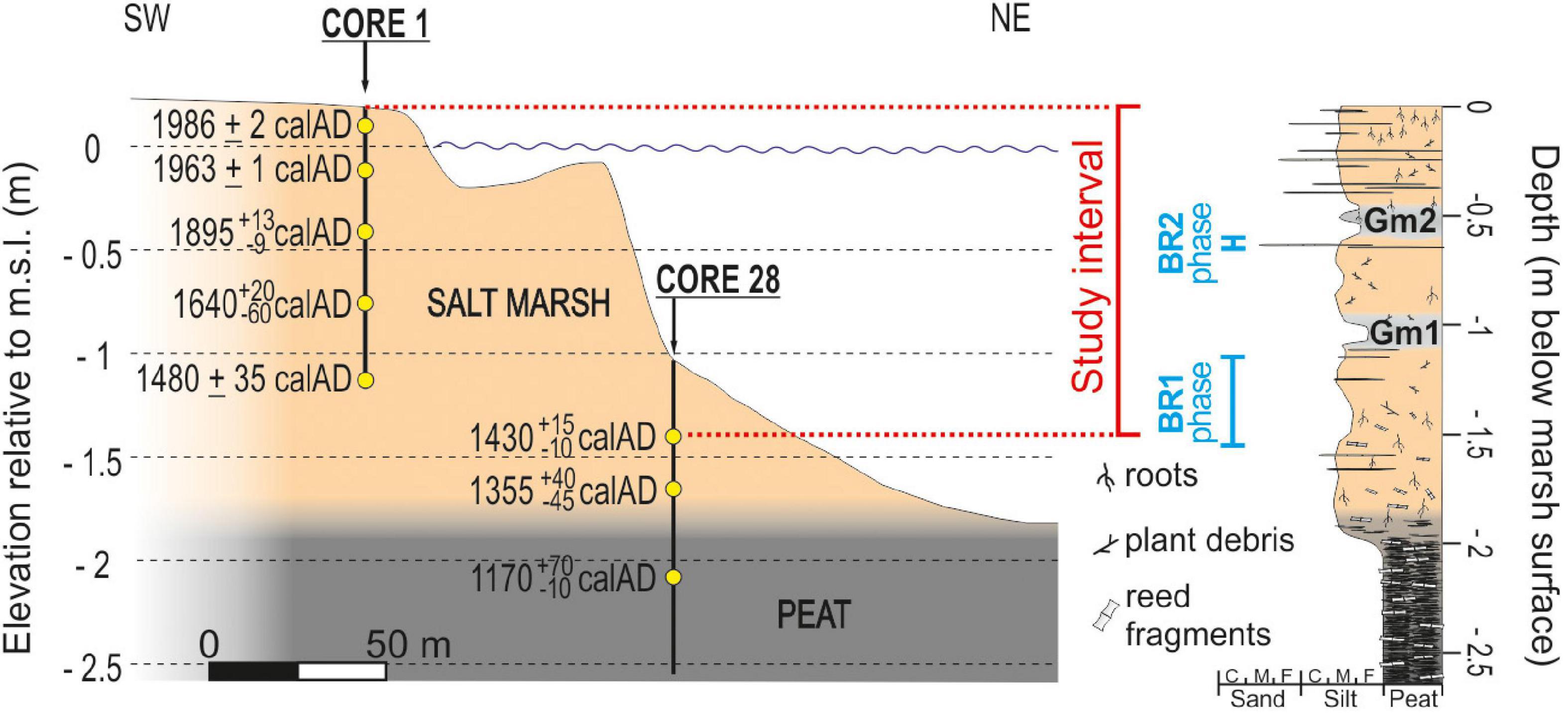
Figure 3. On the left, schematic representation of the Punta Cane sedimentary succession. Yellow dots represent the depths of the dated samples. Each age reports the mode value obtained from the age distribution, and the errors of the calibrated age interval at 68% of probability (after Roner et al., 2017). On the right, sedimentological log of the Punta Cane succession built up from cores 1 and 28. The upper 1.5 m salt-marsh deposits represent the study interval. BR1 and BR2 phases indicate the intervals of the Brenta River feeding the Venice Lagoon.
In this study, we adopted a multi-proxy approach aimed to detect the signature of sediment input sourced from the Brenta River in the Punta Cane succession. In particular, we investigated the upper 1.50 m thick marsh deposits (Figure 3) on the basis of an approach that couples sedimentological, paleoecological, geophysical, and chemical analyses. Results are here expressed as a function of time (Figure 4) based on the age model proposed by Roner et al. (2017). Sedimentological analyses include core description and measurements of organic content and inorganic fraction, as well as sediment grain size. We determined the organic content (3 cm sample spacing) through a Loss On Ignition process at 375∘C for 16 h (Ball, 1964; Morris et al., 2016; Roner et al., 2016) and we calculated it as weight loss after burning. We measured the grain size of the inorganic fraction (3 cm sample spacing) through laser diffraction analysis, after removal of organic components with hydrogen peroxide (H2O2; Gray et al., 2010). Paleoecological analyses involved palynology and foraminifera assemblages. We washed sediment samples through a 63 μm mesh sieve to remove the fine fraction to study foraminiferal assemblage characterizations, that have been analyzed in two sedimentary intervals accumulated across phases BR1 (9 samples, 3 cm spaced) and BR2 (15 samples, 3 cm spaced). We counted foraminifera under a stereomicroscope and classified them according to the taxonomic order of Loeblich and Tappan (1987). Assemblage composition used in this study has been compared with previous works on salt-marsh foraminifera in the Venice Lagoon (Petrucci et al., 1983; Albani et al., 1984; Serandrei-Barbero et al., 2004). For palynological investigations (3 cm sample spacing), following the addition of Lycopodium tablets to determine palynomorph concentration, we treated 38 pre-dried sediment samples with standard HCl, HF, and KOH procedures. We counted palynomorphs by transmitted light microscopy, at between 500x and 1,000x magnification. We measured volumetric specific susceptibility (k) by using a Bartington MS2C logging sensor with a spatial resolution of 2 cm. Finally, we carried out an X-Ray Fluorescence analysis on the salt-marsh succession using an Avaatech XRF Core Scanner at 10 kV and a resolution of 1 cm down-core.
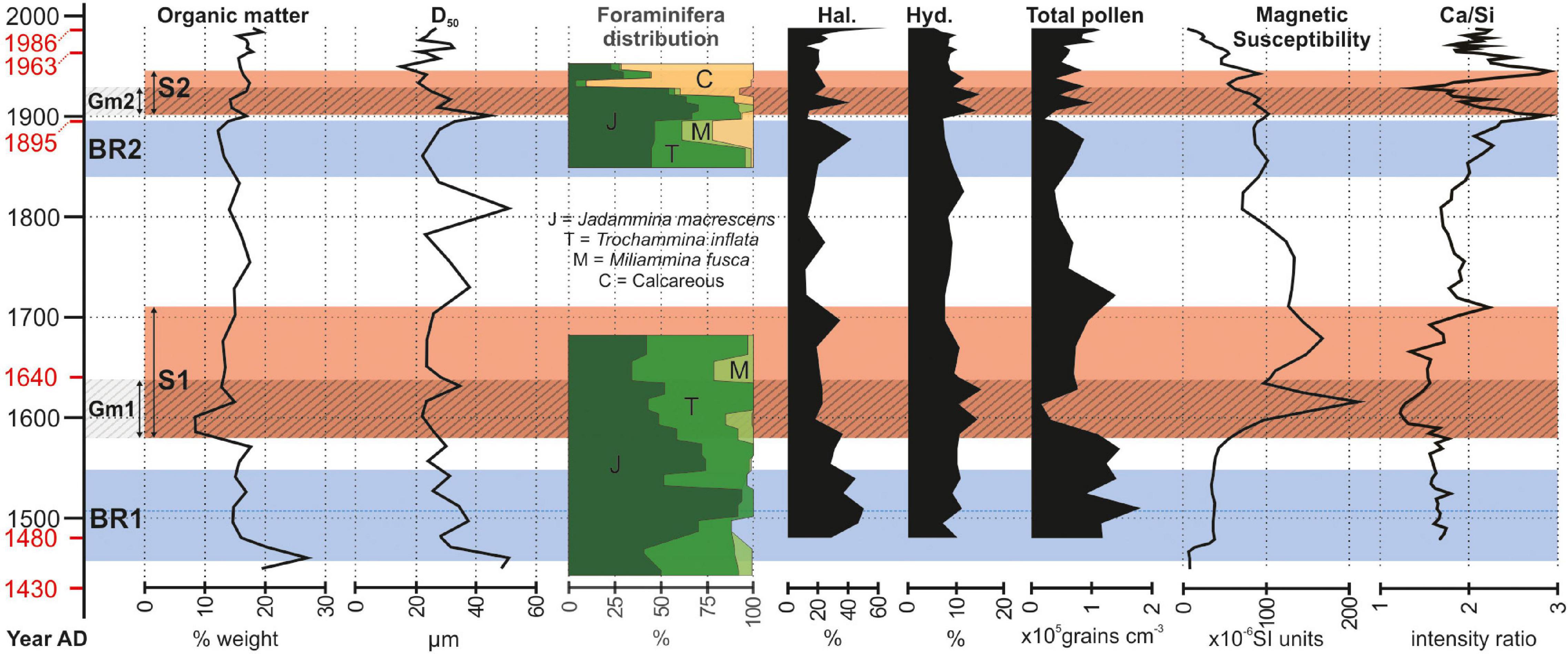
Figure 4. Results of the multi-proxy approach employed along the salt-marsh succession. From left to right, graphs represent the results of: sedimentological analysis (organic matter content and grain size distribution); paleoecological analysis (foraminifera distribution: J = Jadammina macrescens, T = Trochammina inflate, M = Miliammina fusca, C = calcareous species, i.e., Quinqueloculina seminulum, Ammonia beccarii, Haynesina paucilocula, Aubignyna perlucida; palynology: Halophytes, Hydrophytes, Total pollen); geophysical analysis (magnetic susceptibility); geochemical analysis by XRF. The vertical axis is the time expressed in years AD. Dates in red are those obtained from the chronological model proposed by Roner et al. (2017). Blue intervals BR1 and BR2 represent the two phases of the Brenta River feeding the Venice Lagoon in the Punta Cane area. Red stripes S1 and S2 have been detected from the signal of the different proxies and represent the signature of the Brenta River sedimentary input. Lined intervals Gm1 and Gm2 identify two gray mud intervals in the marsh deposit (see sedimentological log in Figure 3) and represent the onset of S1 and S2, respectively.
Results
The salt-marsh deposits (see sedimentological log in Figure 3) consist of horizontally laminated, sometimes bioturbated mud, with scattered millimeter-thick laminae of fine to very-fine grained sand. Mud is dominantly brownish and contains abundant plant debris and in situ root remains. The accumulation of salt-marsh deposits occurred in the upper part of the intertidal zone, where mud settled down around high-water slack, at the transition between flood and ebb tides. Sandy laminae were generated during storms, when waves re-suspended sand and mud from the tidal flats and subtidal platforms in front of the marsh and delivered them onto the salt-marsh platform (Carniello et al., 2009; Mariotti and Carr, 2014). The organic matter produced by halophytic vegetation contributed to salt-marsh accretion together with the inorganic component (Morris et al., 2002; Roner et al., 2016). Two main intervals of grayish mud with scarce plant debris, hereinafter Gm1 and Gm2 (Figures 3–5), occur within the salt-marsh succession. Following the proposed age model, Gm1 and Gm2 intervals date back to AD 1580–1640 and AD 1900–1930, respectively, (Figures 4, 5).
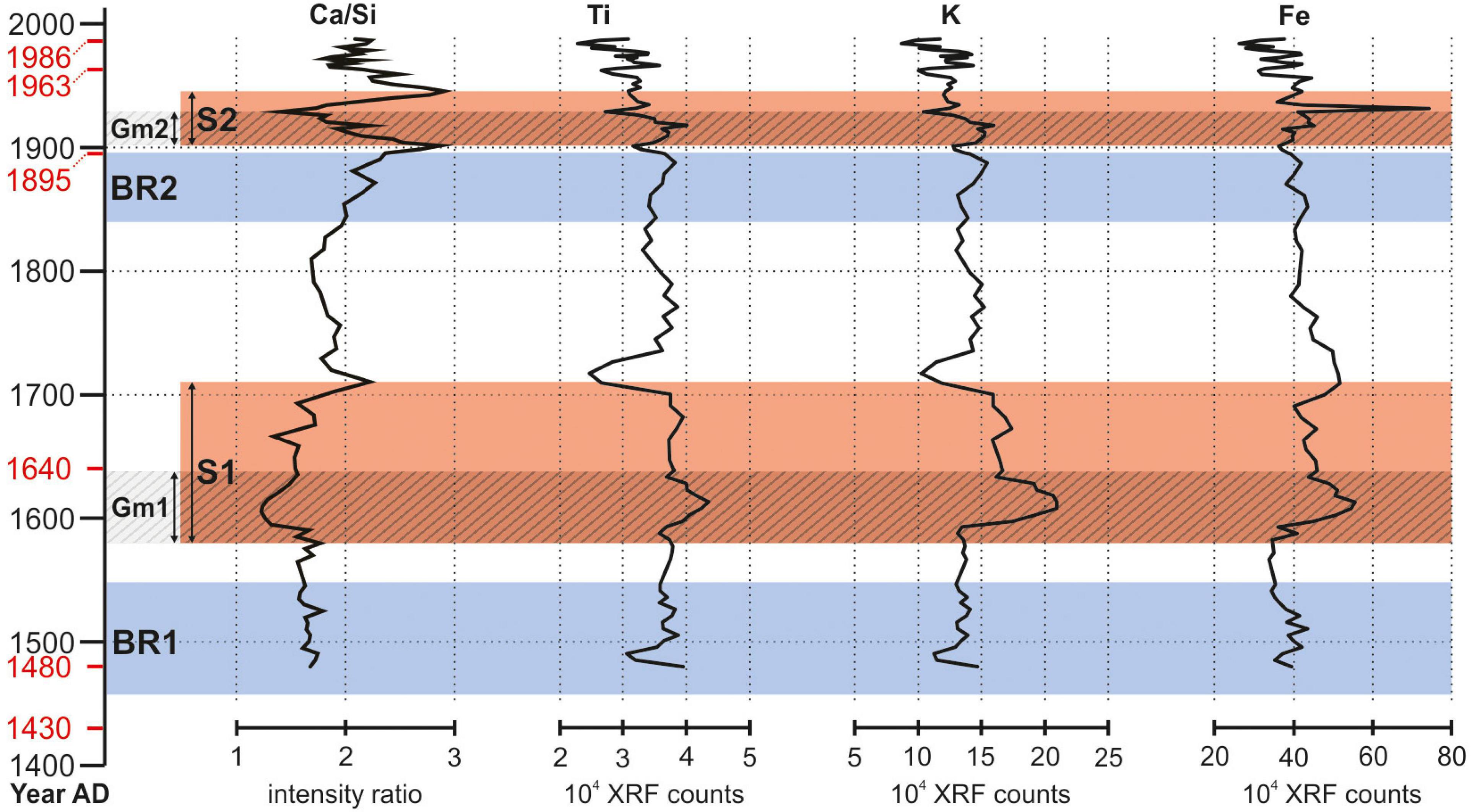
Figure 5. Ca/Si intensity ratio trend (see Figure 4), related to the signal of other detrital elements (titanium, potassium, and iron) to confirm the existence of the two intervals S1 and S2. For dates on the vertical axis, BR1, BR2, S1, S2, Gm1, and Gm2 intervals refer to Figure 4 caption.
The organic matter content (Figure 4) ranges between 8.5 and 27% and no significant trend or changes can be detected through the salt-marsh succession, except for a major decrease (occurring over a time span of ca. 15 year) observed at the base of the Gm1 interval (AD 1580–1640). The higher values at the bottom of the core (20–27%) can be ascribed to bioturbation, which mixed the underlying peat with salt-marsh mud.
The median grain size D50 (Figure 4) is in the range 15–50 μm and no significant trend or changes are detected through the study succession. The occurrence of small positive peaks corresponds to the presence of the sandy laminae.
Foraminifera assemblage (Figure 4) shows that the two studied intervals (ca. from AD 1450 to 1680 and from AD 1850 to 1950) accumulated in salt-marsh depositional settings. Up to ca. AD 1910, the dominance of Trochammina inflata, Jadammina macrescens, and Miliammina fusca points to a typical assemblage of upper-marsh in the Venice Lagoon (Petrucci et al., 1983). After AD 1910 to the 1950s, the foraminifera assemblage is dominated by calcareous species (predominantly Quinqueloculina seminulum, with secondary contributions from the lagoonal species Ammonia beccarii, Haynesina paucilocula, and Aubignyna perlucida) which, together with the salt-marsh species T. inflata and J. macrescens, suggest the presence of a middle-marsh environment (Levin et al., 1996; Horton and Edwards, 2006).
Palynological evidence (Figure 4) suggests the predominance of halophytic vegetation (mostly Amaranthaceae) and prominent growth of vegetated salt marshes at ca. AD 1500 and 1880, except for small-scale fluctuations in the retrieved signals and excluding major peak at the top of the core that corresponds to the present-day marsh. On the other hand, hydrophyte presence (especially Cyperaceae) increases in correspondence of Gm1 and Gm2 (i.e., at ca. AD 1600 and 1900), pointing to a freshwater riverine input. The most pronounced drops in pollen concentration mark the lower part of muddy Gm1 and Gm2 intervals.
Magnetic susceptibility (Figure 4) highlights quite constant and low values (about 40 × 10–6 SI units) for phase BR1 with an absolute minimum (5 × 10–6 SI units) in correspondence of the bottom of the study succession (AD 1450), where salt-marsh deposits are mixed with the basal peat by bioturbation. A clear peak (>200 × 10–6 SI units) marks interval Gm1 around AD 1620, consistently with the occurrence of detrital magnetic elements sourced from rocks (i.e., granitoids) exposed in the Brenta-River drainage basin. Moving upward, the susceptibility exhibits a decreasing trend that is possibly correlated with the slight increasing content in organic matter.
XRF analysis highlights two clear intervals where Ca/Si (Figure 4) and content of detrital elements like Ti, K, and Fe (Figure 5) show low and high values, respectively. The lower interval covers ca. 130 year (from ca. AD 1580 to 1710), while the more recent one corresponds to ca. 45 year (from ca. AD 1900 to 1945). Both intervals are floored by the two gray Gm1 and Gm2 mud layers, respectively. The negative excursion in Ca/Si intensity ratio and the increase in Ti, K, and Fe (Figure 5) is consistent with sediments deriving from dismantling of lithotypes (i.e., granitoids) exposed in the Brenta River drainage basin.
Discussion
The study succession documents the evolution of a salt-marsh depositional environment over a time period of 650 years, thus including the two historically documented time-spans of active fluvial input by the Brenta River (i.e., BR1 and BR2 periods). The dataset presented here shows that, despite the large volume of sediments delivered by the Brenta River during these stages – which determined during BR2 an expansion of the total marsh area of 24 km2 according to the available historical maps (Roner et al., 2017; Tommasini et al., 2019) – the ratio between organic and inorganic sedimentation, as well as the inorganic grain size, remained almost constant. Moreover, an initially stable upper salt-marsh environment is also documented by the foraminifera assemblage, whereas a middle-marsh environment was suggested to occur in the early 20th century (Figure 4). On the contrary, magnetic susceptibility, XRF, and palynology proxies consistently point at two distinct time intervals retaining the signatures of the Brenta River sedimentary input, i.e.,: (i) S1 (AD 1580–1710), and (ii) S2 (AD 1900–1945; Figure 4). Because the sedimentary signatures, S1 and S2, are younger than BR1 and BR2, respectively, it emerges that the active delivery of river sediments to the salt-marsh surface was delayed relative to the river input into the Lagoon. We suggest that this time lag is due to the temporary storage of river-fed deposits around the salt marshes in the Punta Cane area, which significantly expanded when the Brenta River was reintroduced into the Lagoon. The latter process is indeed supported by the widespread presence of halophytes around AD 1500 and AD 1880 (Figure 4). River-fed deposits were essentially stored around pre-existing salt marshes (Figure 6) promoting their further expansion, whereas only a minimum amount of sediment was accumulated over salt-marsh surfaces. This explains why neither a significant increase in salt-marsh elevation nor a decrease in organic matter production were observed.
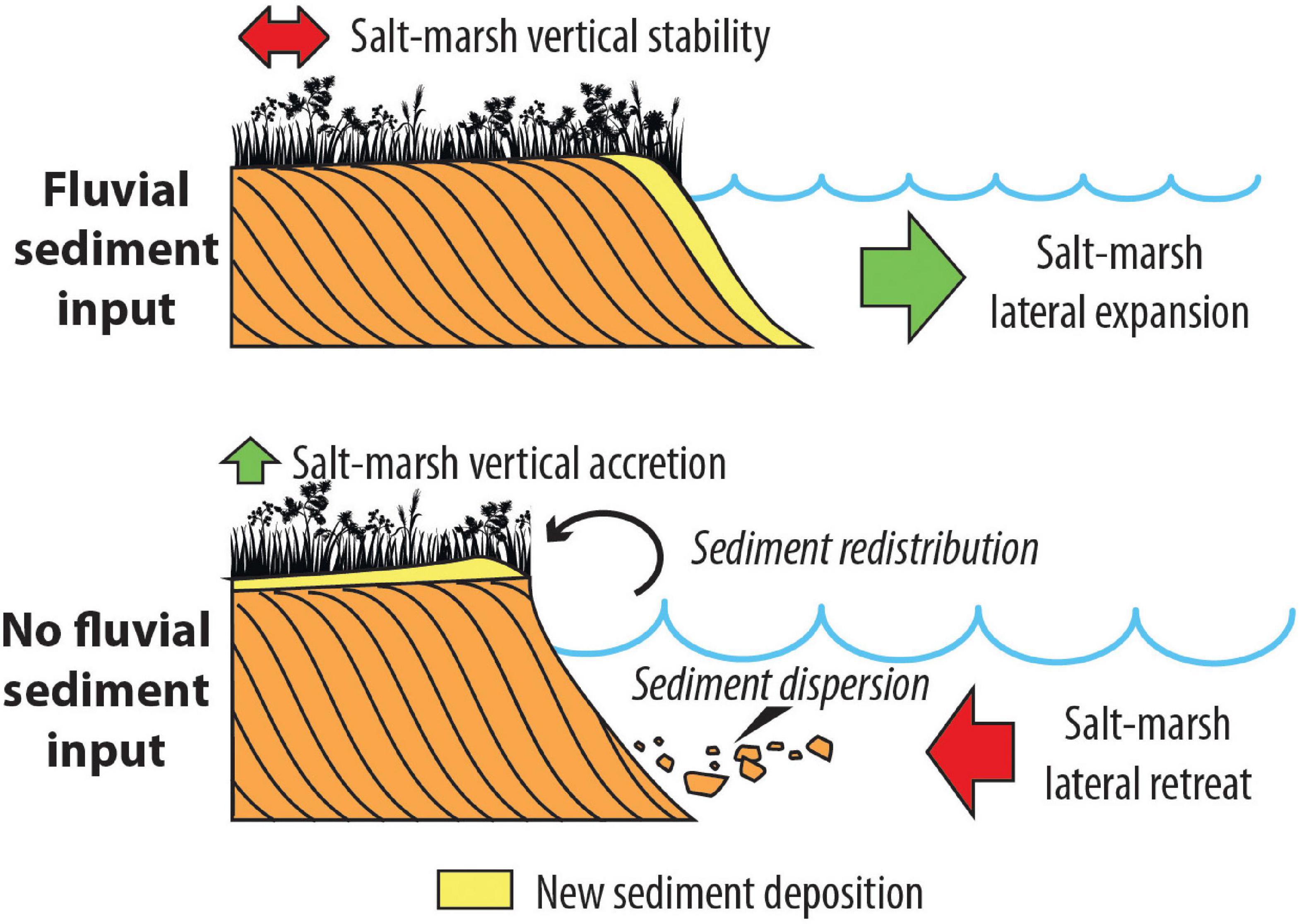
Figure 6. Sketch showing the effects of the presence (above) and of the absence (below) of a fluvial sediment input on tidal marshes, according to the conceptual model developed in the present study.
With respect to the BR2 event, this interpretation is strongly supported by Lanciani (1872), who documented an average accumulation of 25 cm of sediments above the intertidal surfaces when the Brenta River debouched into the Venice Lagoon between AD 1867 and 1870. When the Brenta River was diverted outside the Lagoon, the river-fed deposits were re-worked and re-suspended by wind waves and spread over the marshes by wind waves during periods of relatively high water levels (e.g., Mariotti and Fagherazzi, 2013; Figure 6). In the Punta Cane succession, this sediment pulse over the salt marshes clearly emerges from: (i) the accumulation of the grayish mud layers, Gm1 and Gm2; (ii) the dilution of the total pollen content; and (iii) the expansion of hydrophytes. These processes are also followed by the high values of the magnetic susceptibility and the detrital elements. Based on the age model proposed by Roner et al. (2017), the time lag between the onset of BR1 and S1 is about 120 years, while between the onset of BR2 and S2 the time lag appears to be about 60 years (Figure 4). The comparison of historical maps highlights changes in the position of salt-marsh margins: during the BR1 event, the marsh border was further away than during BR2 (D’Alpaos, 2010; Roner et al., 2017) and, consequently, also the source of the sediments was farther, requiring longer times to be redistributed on the inner portion of the salt marsh, where the study succession is currently located.
Conclusion
Our field observations confirm previous modeling results suggesting the existence of a lag between a perturbation in external forcings, i.e., a high sediment pulse for the study case at hand, and the response of a salt-marsh system to such perturbation (Kirwan and Murray, 2008; D’Alpaos et al., 2011). However, it is worth noting that previous modeling approaches (e.g., D’Alpaos et al., 2011) were typically based on a zero-dimensional approximation, i.e., models considered one point as representative of the whole marsh platform. Our study, on the contrary, highlights that the spatial dynamics of the salt-marsh system play a relevant role in the relaxation time required for the system to reach new equilibrium conditions. Our results clearly emphasize that investigations of ultra-recent sedimentary successions cannot preclude a deep understanding of specific depositional dynamics.
Data Availability Statement
All the data presented in this study have been collected and processed between 2015 and 2019, and are freely available at https://doi.org/10.5281/zenodo.5509123.
Author Contributions
MR and MG: conception of the work, field data collection, sedimentological data analyses, data interpretation, and wrote and reviewed the article. AD’A: conception of the work, field data collection, data interpretation, and wrote and reviewed the article. AF: field data collection and wrote and reviewed the article. AB, NC-N, and MV: palynological data analyses and article review. SD: foraminifera data analyses and article review. LV: geophysical data analyses and article review. AG: geochemical data analyses and article review. LB, MF, and LL: geochronological data analyses and article review. LT: field data collection. All authors contributed to the article and approved the submitted version.
Funding
This work was supported by the CARIPARO Project titled “Reading signatures of the past to predict the future: 1,000 years of stratigraphic record as a key for the future of the Venice Lagoon”; the project HYDROSEM (Progetti di Eccellenza CARIPARO 2017, Cassa di Risparmio di Padova e Rovigo): “Fluvial and tidal meanders of the Venetian-Po plain: From hydrodynamics to stratigraphy” project (PI. MG). This scientific activity was partially performed within by the Research Program Venezia 2021, with the contribution of the Provveditorato for the Public Works of Veneto, Trentino Alto Adige, and Friuli Venezia Giulia, provided through the concessionary of State Consorzio Venezia Nuova and coordinated by CORILA, Research Line 3.2 [AD’A (PI); MG; and AF], that is gratefully acknowledged.
Conflict of Interest
The authors declare that the research was conducted in the absence of any commercial or financial relationships that could be construed as a potential conflict of interest.
Publisher’s Note
All claims expressed in this article are solely those of the authors and do not necessarily represent those of their affiliated organizations, or those of the publisher, the editors and the reviewers. Any product that may be evaluated in this article, or claim that may be made by its manufacturer, is not guaranteed or endorsed by the publisher.
Acknowledgments
The authors acknowledge constructive reviews by two reviewers, which greatly helped to improve the manuscript.
References
Albani, A. D., Favero, V., and Serandrei Barbero, R. (1984). Benthonic foraminifera as indicators of intertidal environments. GeoMarine Lett. 4, 43–47. doi: 10.1007/BF02237973
Allen, J. R. L. (1995). Salt-marsh growth and fluctuating sea level: implications of a simulation model for Flandrian coastal stratigraphy and peat-based sea-level curves. Sediment. Geol. 100, 21–45. doi: 10.1016/0037-0738(95)00101-8
Ball, D. F. (1964). Loss-on-ignition as an estimate of organic matter and organic carbon in non-calcareous soils. J. Soil Sci. 15, 84–92. doi: 10.1111/j.1365-2389.1964.tb00247.x
Barbier, E. B., Hacker, S. D., Kennedy, C., Koch, E. W., Stier, A. C., and Silliman, B. R. (2011). The value of estuarine and coastal ecosystem services. Ecol. Monogr. 81, 169–193. doi: 10.1890/10-1510.1
Boaga, J., D’Alpaos, A., Cassiani, G., Marani, M., and Putti, M. (2014). Plant-soil interactions in salt marsh environments: experimental evidence from electrical resistivity tomography in the Venice Lagoon. Geophys. Res. Lett. 41, 6160–6166. doi: 10.1002/2014GL060983
Bondesan, A., and Furlanetto, P. (2012). Artificial fluvial diversions in the mainland of the Lagoon of Venice during the 16th and 17th centuries inferred by historical cartography analysis. Geomorphologie 2, 175–200.
Carniello, L., Defina, A., and D’Alpaos, L. (2009). Morphological evolution of the Venice lagoon: evidence from the past and trend for the future. J. Geophys. Res. Earth Surf. 114, 1–10. doi: 10.1029/2008JF001157
Castillo, J. M., Luque, C. J., Castellanos, E. M., and Figueroa, M. E. (2000). Causes and consequences of salt-marsh erosion in an Atlantic estuary in SW Spain. J. Coast. Conserv. 6, 89–96. doi: 10.1007/BF02730472
D’Alpaos, A. (2011). The mutual influence of biotic and abiotic components on the long-term ecomorphodynamic evolution of salt-marsh ecosystems. Geomorphology 126, 269–278. doi: 10.1016/j.geomorph.2010.04.027
D’Alpaos, A., and Marani, M. (2016). Reading the signatures of biologic-geomorphic feedbacks in salt-marsh landscapes. Adv. Water Resour. 93, 265–275. doi: 10.1016/j.advwatres.2015.09.004
D’Alpaos, A., Lanzoni, S., Marani, M., and Rinaldo, A. (2007). Landscape evolution in tidal embayments: modeling the interplay of erosion, sedimentation, and vegetation dynamics. J. Geophys. Res. Earth Surf. 112, 1–17. doi: 10.1029/2006JF000537
D’Alpaos, A., Mudd, S. M., and Carniello, L. (2011). Dynamic response of marshes to perturbations in suspended sediment concentrations and rates of relative sea level rise. J. Geophys. Res. Earth Surf. 116, 1–13. doi: 10.1029/2011JF002093
D’Alpaos, L. (2010). Fatti e misfatti di idraulica lagunare. La laguna di Venezia dalla diversione dei fiumi alle nuove opere delle bocche di porto. Venice: Istituto Veneto di Scienze, Lettere ed Arti.
Day, J. W., Britsch, L. D., Hawes, S. R., Shaffer, G. P., Reed, D. J., and Cahoon, D. R. (2000). Pattern and process of land loss in the mississippi delta: a spatial and temporal analysis of wetland habitat change. Estuaries 23, 425–438. doi: 10.2307/1353136
Finotello, A., Marani, M., Carniello, L., Pivato, M., Roner, M., Tommasini, L., et al. (2020). Control of wind-wave power on morphological shape of salt marsh margins. Water Sci. Eng. 13, 45–56. doi: 10.1016/j.wse.2020.03.006
FitzGerald, D. M., and Hughes, Z. (2019). Marsh processes and their response to climate change and sea-level rise. Annu. Rev. Earth Planet. Sci. 47, 481–517. doi: 10.1146/annurev-earth-082517-010255
Gedan, K. B., Silliman, B. R., and Bertness, M. D. (2009). Centuries of human-driven change in salt marsh ecosystems. Ann. Rev. Mar. Sci. 1, 117–141. doi: 10.1146/annurev.marine.010908.163930
Gray, A. B., Pasternack, G. B., and Watson, E. B. (2010). Hydrogen peroxide treatment effects on the particle size distribution of alluvial and marsh sediments. Holocene 20, 293–301. doi: 10.1177/0959683609350390
Horton, B., and Edwards, R. (2006). Quantifying holocene sea level change using intertidal foraminifera: lessons from the British Isles. Anuário do Inst. Geociências 29, 541–542.
Kirwan, M. L., and Megonigal, J. P. (2013). Tidal wetland stability in the face of human impacts and sea-level rise. Nature 504, 53–60. doi: 10.1038/nature12856
Kirwan, M. L., and Murray, A. B. (2008). Tidal marshes as disequilibrium landscapes? Lags between morphology and Holocene sea level change. Geophys. Res. Lett. 35, 1–5. doi: 10.1029/2008GL036050
Kirwan, M. L., Guntenspergen, G. R., D’Alpaos, A., Morris, J. T., Mudd, S. M., and Temmerman, S. (2010). Limits on the adaptability of coastal marshes to rising sea level. Geophys. Res. Lett. 37:L23401. doi: 10.1029/2010GL045489
Kirwan, M. L., Murray, A. B., Donnelly, J. P., and Corbett, D. R. (2011). Rapid wetland expansion during European settlement and its implication for marsh survival under modern sediment delivery rates. Geology 39, 507–510. doi: 10.1130/G31789.1
Kirwan, M. L., Temmerman, S., Skeehan, E. E., Guntenspergen, G. R., and Fagherazzi, S. (2016). Overestimation of marsh vulnerability to sea level rise. Nat. Clim. Chang. 6, 253–260. doi: 10.1038/nclimate2909
Kirwan, M., and Temmerman, S. (2009). Coastal marsh response to historical and future sea-level acceleration. Quat. Sci. Rev. 28, 1801–1808. doi: 10.1016/j.quascirev.2009.02.022
Ladd, C. J. T., Duggan-Edwards, M. F., Bouma, T. J., Pagès, J. F., and Skov, M. W. (2019). Sediment supply explains long-term and large-scale patterns in salt marsh lateral expansion and erosion. Geophys. Res. Lett. 46, 11178–11187. doi: 10.1029/2019GL083315
Lanciani, F. (1872). Sul Brenta e sul Novissimo Relazione alla Commissione Pel Miglioramento dei Porti e Lagune Venete. Firenze: Tip. e lit. del Giornale del genio civile.
Leonard, L. A., and Croft, A. L. (2006). The effect of standing biomass on flow velocity and turbulence in Spartina alterniflora canopies. Estuar. Coast. Shelf Sci. 69, 325–336. doi: 10.1016/j.ecss.2006.05.004
Leonard, L. A., and Luther, M. E. (1995). Flow hydrodynamics in tidal marsh canopies. Limnol. Oceanogr. 40, 1474–1484. doi: 10.4319/lo.1995.40.8.1474
Leonardi, N., and Fagherazzi, S. (2014). How waves shape salt marshes. Geology 42, 887–890. doi: 10.1130/G35751.1
Leonardi, N., Ganju, N. K., and Fagherazzi, S. (2016). A linear relationship between wave power and erosion determines salt-marsh resilience to violent storms and hurricanes. Proc. Natl. Acad. Sci. U.S.A. 113, 64–68. doi: 10.1073/pnas.1510095112
Levin, L. A., Talley, D., and Thayer, G. (1996). Succession of macrobenthos in a created salt marsh. Mar. Ecol. Prog. Ser. 141, 67–82. doi: 10.3354/meps141067
Li, H., and Yang, S. L. (2009). Trapping effect of tidal marsh vegetation on suspended sediment, Yangtze Delta. J. Coast. Res. 25, 915–936. doi: 10.2112/08-1010.1
Loeblich, A. R., and Tappan, H. (1987). Foraminiferal Genera and Their Classification. Boston, MA: Springer.
Marani, M., D’Alpaos, A., Lanzoni, S., and Santalucia, M. (2011). Understanding and predicting wave erosion of marsh edges. Geophys. Res. Lett. 38, 1–5. doi: 10.1029/2011GL048995
Marani, M., D’Alpaos, A., Lanzoni, S., Carniello, L., and Rinaldo, A. (2007). Biologically-controlled multiple equilibria of tidal landforms and the fate of the Venice lagoon. Geophys. Res. Lett. 34, 1–5. doi: 10.1029/2007GL030178
Marani, M., Da Lio, C., D’Alpaos, A., and D’Alpaos, A. (2013). Vegetation engineers marsh morphology through multiple competing stable states. Proc. Natl. Acad. Sci. U.S.A. 110, 3259–3263. doi: 10.1073/pnas.1218327110
Marani, M., Silvestri, S., Belluco, E., Ursino, N., Comerlati, A., Tosatto, O., et al. (2006). Spatial organization and ecohydrological interactions in oxygen-limited vegetation ecosystems. Water Resour. Res. 42:W06D06. doi: 10.1029/2005WR004582
Mariotti, G. (2016). Revisiting salt marsh resilience to sea level rise: are ponds responsible for permanent land loss? J. Geophys. Res. Eart 121, 1391–1407. doi: 10.1002/2013JF002871.Received
Mariotti, G., and Carr, J. (2014). Dual role of salt marsh retreat: long-term loss and short-term resilience. Water Resour. Res. 50, 2963–2974. doi: 10.1002/2013WR014676
Mariotti, G., and Fagherazzi, S. (2010). A numerical model for the coupled long-term evolution of salt marshes and tidal flats. J. Geophys. Res. Earth Surf. 115:F01004. doi: 10.1029/2009JF001326
Mariotti, G., and Fagherazzi, S. (2013). Critical width of tidal flats triggers marsh collapse in the absence of sea-level rise. Proc. Natl. Acad. Sci. U. S. A. 110, 5353–5356. doi: 10.1073/pnas.1219600110
Morris, J. T., Barber, D. C., Callaway, J. C., Chambers, R., Hagen, S. C., Hopkinson, C. S., et al. (2016). Contributions of organic and inorganic matter to sediment volume and accretion in tidal wetlands at steady state. Earth’s Futur. 4, 110–121. doi: 10.1002/2015EF000334
Morris, J. T., Sundareshwar, P. V. V. V., Nietch, C. T., Kjerfve, B. B., and Cahoon, D. R. (2002). Responses of coastal wetlands to rising sea leve. Ecology 83, 2869–2877. doi: 10.1890/0012-96582002083
Mudd, S. M. (2011). The life and death of salt marshes in response to anthropogenic disturbance of sediment supply. Geology 39, 511–512. doi: 10.1130/focus052011.1
Mudd, S. M., D’Alpaos, A., and Morris, J. T. (2010). How does vegetation affect sedimentation on tidal marshes? Investigating particle capture and hydrodynamic controls on biologically mediated sedimentation. J. Geophys. Res. Earth Surf. 115:F03029. doi: 10.1029/2009JF001566
Mudd, S. M., Howell, S. M., and Morris, J. T. (2009). Impact of dynamic feedbacks between sedimentation, sea-level rise, and biomass production on near-surface marsh stratigraphy and carbon accumulation. Estuar. Coast. Shelf Sci. 82, 377–389. doi: 10.1016/j.ecss.2009.01.028
Neubauer, S. C. (2008). Contributions of mineral and organic components to tidal freshwater marsh accretion. Estuar. Coast. Shelf Sci. 78, 78–88. doi: 10.1016/j.ecss.2007.11.011
Nyman, J. A., Walters, R. J., Delaune, R. D., and Patrick, W. H. (2006). Marsh vertical accretion via vegetative growth. Estuar. Coast. Shelf Sci. 69, 370–380. doi: 10.1016/j.ecss.2006.05.041
Ortiz, A. C., Roy, S., and Edmonds, D. A. (2017). Land loss by pond expansion on the Mississippi River Delta Plain. Geophys. Res. Lett. 44, 3635–3642. doi: 10.1002/2017GL073079
Petrucci, F., Medioli, F. S., Scott, D. B., Pianetti, F. A., and Cavazzini, R. (1983). Evaluation of the usefulness of foraminifera as sea-level indicators in the Venice lagoon (N. Italy). Ateneo Parm. Acta Nat. 19, 63–77.
Roner, M., D’Alpaos, A., Ghinassi, M., Marani, M., Silvestri, S., Franceschinis, E., et al. (2016). Spatial variation of salt-marsh organic and inorganic deposition and organic carbon accumulation: inferences from the Venice lagoon, Italy. Adv. Water Resour. 93, 276–287. doi: 10.1016/j.advwatres.2015.11.011
Roner, M., Ghinassi, M., Fedi, M., Liccioli, L., Bellucci, L. G., Brivio, L., et al. (2017). Latest holocene depositional history of the southern Venice Lagoon, Italy. Holocene 27, 1731–1744. doi: 10.1177/0959683617708450
Schepers, L., Brennand, P., Kirwan, M. L., Guntenspergen, G. R., and Temmerman, S. (2020). Coastal, marsh degradation into ponds induces irreversible elevation loss relative to sea level in a microtidal system. Geophys. Res. Lett. 47:e2020GL089121. doi: 10.1029/2020GL089121
Serandrei-Barbero, R., Albani, A., and Bonardi, M. (2004). Ancient and modern salt marshes in the Venetian Lagoon. Palaeogeogr. Palaeoclimatol. Palaeoecol. 202, 229–244.
Surian, N., and Cisotto, A. (2007). Channel adjustments, bedload transport and sediment sources in a gravel-bed river, Brenta River, Italy. Earth Surf. Process. Landforms 32, 1641–1656. doi: 10.1002/esp.1591
Tommasini, L., Carniello, L., Ghinassi, M., Roner, M., and D’Alpaos, A. (2019). Changes in the wind-wave field and related salt-marsh lateral erosion: inferences from the evolution of the Venice Lagoon in the last four centuries. Earth Surf. Process. Landforms 44, 1633–1646. doi: 10.1002/esp.4599
Valiela, I., Kinney, E., Culberston, J., Peacock, E., and Smith, S. (2009). “Global losses of mangroves and salt marshes,” in Global Loss of Coastal Habitats: Rates, Causes and Consequences, ed. C. M. Duarte (Bilbao:Fundación BBVA), 184.
Wang, C., Schepers, L., Kirwan, M. L., Belluco, E., D’Alpaos, A., Wang, Q., et al. (2021). Different coastal marsh sites reflect similar topographic conditions under which bare patches and vegetation recovery occur. Earth Surf. Dyn. 9, 71–88. doi: 10.5194/esurf-9-71-2021
Keywords: salt marsh, sediment supply, Venice Lagoon (Italy), coastal environment, vertical accretion rate
Citation: Roner M, Ghinassi M, Finotello A, Bertini A, Combourieu-Nebout N, Donnici S, Gilli A, Vannacci M, Vigliotti L, Bellucci LG, Fedi M, Liccioli L, Tommasini L and D’Alpaos A (2021) Detecting the Delayed Signatures of Changing Sediment Supply in Salt-Marsh Landscapes: The Case of the Venice Lagoon (Italy). Front. Mar. Sci. 8:742603. doi: 10.3389/fmars.2021.742603
Received: 16 July 2021; Accepted: 20 September 2021;
Published: 11 October 2021.
Edited by:
Nicoletta Leonardi, University of Liverpool, United KingdomReviewed by:
Christian Schwarz, University of Delaware, United StatesQinghua Ye, Deltares, Netherlands
Copyright © 2021 Roner, Ghinassi, Finotello, Bertini, Combourieu-Nebout, Donnici, Gilli, Vannacci, Vigliotti, Bellucci, Fedi, Liccioli, Tommasini and D’Alpaos. This is an open-access article distributed under the terms of the Creative Commons Attribution License (CC BY). The use, distribution or reproduction in other forums is permitted, provided the original author(s) and the copyright owner(s) are credited and that the original publication in this journal is cited, in accordance with accepted academic practice. No use, distribution or reproduction is permitted which does not comply with these terms.
*Correspondence: Marcella Roner, marcella.roner@unipd.it; Andrea D’Alpaos, andrea.dalpaos@unipd.it