- 1Ecology, Evolution, and Behavior, University of California, Santa Cruz, Santa Cruz, CA, United States
- 2Environmental Assessment Group, Korea Environment Institute, Sejong, South Korea
Climate change is rapidly altering the thermal environment in terrestrial and aquatic systems. Transgenerational thermal plasticity (TGP) – which occurs when the temperatures experienced by the parental generation prior to the fertilization of gametes results in a change in offspring reaction norms – may mitigate the effects of climate change. Although “maternal effects” have been widely studied, relatively little is known about TGP effects in vertebrates, particularly paternal contributions. We used artificial fertilization to cross sheepshead minnow (Cyprinodon variegatus) parents exposed to either low (26°C) or high (32°C) temperatures and measured growth rates of the offspring over the first 8 weeks of life at both low and high temperatures. A linear mixed effects model was employed to quantify the effects of maternal, paternal, and offspring temperatures on offspring growth and fecundity. We found that the offspring growth rate up to 63 days post-hatch was affected by both the temperature they experienced directly and parental temperatures prior to fertilization. Growth was lowest when neither parents’ temperature matched the offspring temperature, indicating a strong transgenerational effect. Notably, offspring growth was highest when all three (offspring, sire, and dam) temperatures matched [although the three-way interaction was found to be marginally non-significant (P = 0.155)], suggesting that TGP effects were additive across significant sire-offspring (P < 0.001) and dam-offspring interactions (P < 0.001). Transgenerational effects on fecundity (GSI) were suggestive for both maternal and paternal effects, but not significant. The finding that thermal TGP is contributed by both parents strongly suggests that it has an epigenetic basis.
Introduction
Global temperatures are predicted to rise by at least 2°C by 2050–2100, a rapid shift that is significantly more than that of the past 420,000 years (Hoegh-Guldberg et al., 2007). Increased ocean temperatures can negatively affect marine organisms, e.g., shifts in metabolic rate (Gillooly et al., 2001), decreased body size at each key developmental stages (Atkinson, 1995), and declines in fish population size (Clark et al., 2003). With accelerating change, potential mismatches between the expected and realized thermal environment are more likely to occur and can substantially affect key physiological traits across life stages such as reduced growth, maturation, fecundity, and ultimately survival (Kingsolver and Huey, 1998; Pörtner et al., 2001; Hani et al., 2019). However, the ramifications of rapid climate change can be buffered by transgenerational plasticity (TGP), a carryover effect that is characterized by changes in the reaction norms of offspring that are closely correlated to the environment experienced by the parents (Mousseau and Fox, 1998; Donelson et al., 2018). Parental contributions to offspring can therefore alter the strength of selection on organisms during early life stages in response to climate change, allowing for population persistence and adaptation in populations with high genetic diversity (Heckwolf et al., 2018).
Transgenerational plasticity is often described as a generalization of well-studied maternal effects in which the current state of the mother modifies the offspring phenotype. Maternal effects are found in a wide range of both invertebrate and vertebrate taxa (Mousseau and Dingle, 1991; Mousseau and Fox, 1998; Galloway and Etterson, 2007; Shama, 2015; Ruebel and Latham, 2020; McGhee et al., 2021) and arise through a variety of mechanisms, e.g., provisioning of energy or nutrients (Berkeley et al., 2004), hormones (Groothuis and Schwabl, 2008), and antibodies (Grindstaff et al., 2003), or epigenetic mechanisms such as DNA methylation (Cooney et al., 2002) and histone modification (Upadhyaya et al., 2017).
In contrast, paternal effects were initially thought to be minimal or non-existent (Roach and Wulff, 1987). However, increased interest on paternal effects in recent years (Rutkowska et al., 2020) has revealed evidence that non-genetic paternal effects can arise in both mammals and fish through changes in the sperm epigenome and transmission of the paternal DNA methylome that directly affect resulting offspring through DNA methylation (Jiang et al., 2013; Baxter and Drake, 2019; Skvortsova et al., 2019). Effects of epigenetic transfer can be wide ranging, for example, male rats conditioned to fear the smell of acetophenone paired with naive females had produced offspring that also showed an aversion to the same odor (Dias and Ressler, 2014). Offspring sired by male tunicates from low density populations developed faster, a higher hatching success rate, and were more likely to survive when the environment of the offspring matched the environment of the sire (Crean et al., 2013).
With parental effects found to be stronger early in life (Wilson and Reale, 2006), non-genetic paternal effects can play a role in offspring survival across a myriad of taxa that can have long-lasting consequences (Uller, 2008). Importantly, because the mechanisms for non-genetic paternal effects are limited, evidence for paternal effects in TGP provides strong, albeit indirect, evidence that TGP is epigenetic (Curley et al., 2011). Despite growing literature, relatively little is known about non-genetic paternal contributions to thermal performance, particularly in ectothermic vertebrates (but see Shama and Wegner, 2014). Several studies have found that performance is best when the parent and offspring environments match (Shama and Wegner, 2014; Donelson et al., 2018). But when both parents contribute to TGP, which parents’ environment is most relevant? Given the more numerous pathways for maternal effects to manifest, we might hypothesize that the maternal environment will have the greatest impact on TGP. Alternatively, since TGP has ostensibly evolved to increase offspring fitness, perhaps TGP is driven by the environment that matches best (or worst). Or more simply, perhaps the contributions of sire and dam are additive, such that TGP is driven by the average parental environment. Distinguishing between these hypotheses would be most relevant for species that have sex-specific aggregations and hence parents with distinct thermal histories, or otherwise display sex differences in life history and behavior before breeding (Tarka et al., 2018). In this situation, to effectively predict transgenerational effects on key offspring fitness traits such as growth rate or reproductive allocation and their implications for population responses to climate change, we would need to determine the interplay between non-genetic maternal and paternal effects. In addition, although the age-specificity of maternal effects is fairly well-studied (e.g., Monteleone and Houde, 1990; Marteinsdottir and Steinarsson, 1998; Gao and Munch, 2013), very little is known about how paternal contributions change over the ontogeny of their offspring.
To fill these gaps, we evaluated the effect of parental temperature on offspring thermal performance, explicitly partitioning sire and dam contributions to growth rate and reproductive allocation using sheepshead minnows (Cyprinodon variegatus) as our model organism. Sheepshead minnows are ectothermic fish found on the US East Coast from Massachusetts to Florida and into the Caribbean across a wide range of thermal regimes. They tolerate temperatures between −1.5 and 41.6°C (Bennett and Beitinger, 1997), grow rapidly and mature in under a year (Lee et al., 2017). Moreover, they grow well in individual housings making it possible to eliminate competition for food and social stress as factors influencing growth. Critically, previous studies have found that sheepshead minnows exhibit thermal TGP across multiple generations (Salinas and Munch, 2012; Lee et al., 2020). After 30 days of parental exposure to different temperatures, the fastest growing offspring tend to be individuals whose parents were at the same temperature (Salinas and Munch, 2012; Munch et al., 2021). To control for the possibility of selection on larval survival at different temperatures, the experiment was repeated using the same rearing protocols, but reducing the parental temperature treatment to 7 days. With this brief parental temperature treatment, no effect of the parent temperature was observed. As the only difference between these experiments was the duration of the parent temperature exposure, Salinas and Munch (2012) attributed differences in offspring growth to TGP. However, they used mass-spawning to produce large numbers of fertilized eggs and were thus unable to rigorously control for the possibility of fecundity selection and unable to distinguish between paternal and maternal contributions to phenotype. To resolve these issues, we used artificial fertilization to test for distinct maternal and paternal contributions to offspring growth rate and gonadosomatic index (GSI), key indicators for survival and fecundity that could also be subject to growth-fecundity tradeoffs. Although all husbandry was kept identical to Salinas and Munch (2012), we narrowed the range of acclimation and test temperatures from 21–34°C down to 24–32°C to reduce concerns about temperature stress and subsequently exposed offspring to parental reproductive temperatures.
Materials and Methods
In August 2014, wild juvenile sheepshead minnows (C. variegatus) were collected from tidal ponds in South Carolina, United States (32°45′2″ N, 79°53′50″ W) where they typically experienced daily average temperatures between 2.5 and 32.5°C (median temperature = 21.5°C). The fish were acclimated in aquaria held at 24°C at the Southwest Fisheries Science Center, Santa Cruz, California. As sheepshead minnows exhibit grandparental effects (Lee et al., 2020) we maintained breeding populations in the lab under constant temperature (24°C) for three generations, to reduce uncontrolled environmental effects and used F3 fish as the parents in this experiment.
Fish Crosses
In 2016, we separated F3 females (n = 40) and males (n = 40) into individual nets for 30 days before the collection of gametes to ensure that eggs were not fertilized before collection. We randomly subdivided these into two groups and acclimated them to experimental temperatures of 26 and 32°C for 30 days which is sufficient to elicit a transgenerational response (Salinas and Munch, 2012). No parents died over this interval.
To examine the degree to which maternal and paternal temperatures affect TGP in F4 offspring, we artificially crossed males and females from each temperature group in a full factorial design. We anesthetized female fish with MS-222 to reduce handling stress, and conducted strip spawning to collect unfertilized eggs. To obtain sperm, we dissected testes from males euthanized with concentrated MS-222 solution, pulverized the testes in a petri dish and diluted with seawater. Eggs from each female were divided into two petri dishes, with half artificially fertilized with sperm/seawater solution from a randomly selected 26°C male, and the other half by a 32°C male. To ensure fertilization success, the egg-sperm mixture was allowed to sit for ∼30 min. We then subdivided the fertilized eggs from each into separate mesh cages (34.3 × 43.9 × 5.1 cm) for hatching and growth at 26 and 32°C. All subsequent acclimation, feeding, and daily care followed protocols described in Salinas and Munch (2012) which did not generate detectable selection on larvae and juveniles.
One week post-hatch, we randomly selected four (F4) larvae from each family-temperature combination and placed them into individual growth chambers (8.5 cm diameter × 20.0 cm height) with mesh walls and a solid clear base to allow for tracking of individual growth trajectories and elimination of social interactions. Immediately upon hatching, larvae were fed Rotifera until around 5 mm. Larvae between 5 and 10 mm were fed Artemia nauplii and then switched to crushed TetraMin (Tetra Holding, Blacksburg, VA, United States) flakes. All feeding for larvae was ad libitum four times a day. Seawater was maintained at 20 ppt and photoperiod set at 14:10. Water changes were conducted daily at 10% of the total volume of water in the aquarium table to maintain high water quality. We photographed the fish weekly over 9 weeks with a Canon 40D digital camera (3888 × 2592 pixels; Canon, Japan). At this time all fish within a temperature group were randomly redistributed across aquarium tables to homogenize any table effects. After 9 weeks, we euthanized specimens with concentrated MS-222 solution and dissected them to measure wet body mass (g) and wet gonad mass (g).
Data Analysis
We measured standard length with Image J (Schneider et al., 2012) from weekly photographs. Growth rate (mm/d) was calculated from the change in body length between weeks, starting with weeks 2 and 3. GSI was calculated as the ratio of gonad wet weight to gutted wet weight. Three-way ANOVA tests and post hoc Tukey tests were then used to assess the significance of parent and offspring temperature on growth rate and GSI with offspring, sire and dam temperatures as fixed effects and maternal and paternal identity as random effects. Note that aquarium table was not modeled as a random effect, since offspring within a temperature rearing group were redistributed randomly at weekly intervals across tanks throughout the rearing period. To evaluate whether differences in GSI were driven by growth, we repeated the GSI analysis with growth as a covariate as maturation can be growth or size dependent in fish (Ernande et al., 2004). All of the analyses were performed with R Studio (version 1.3.1056) and the package “lmer4” (Bates et al., 2015).
Results
Offspring growth rates assessed at mean parental temperature were consistent with previous trends found in Salinas and Munch (2012) in which the fastest growth occurs when parent and offspring temperatures match (Figure 1, Supplementary Figure 1, Table 1). Sire and dam identity were not significant for offspring growth rate (estimated variance contributed by parent ID was <0.001). For GSI, random effects were not significant for dam (variance = 0.0572) or sire (variance = 0.042). We therefore dropped parent IDs as random effects from subsequent models.
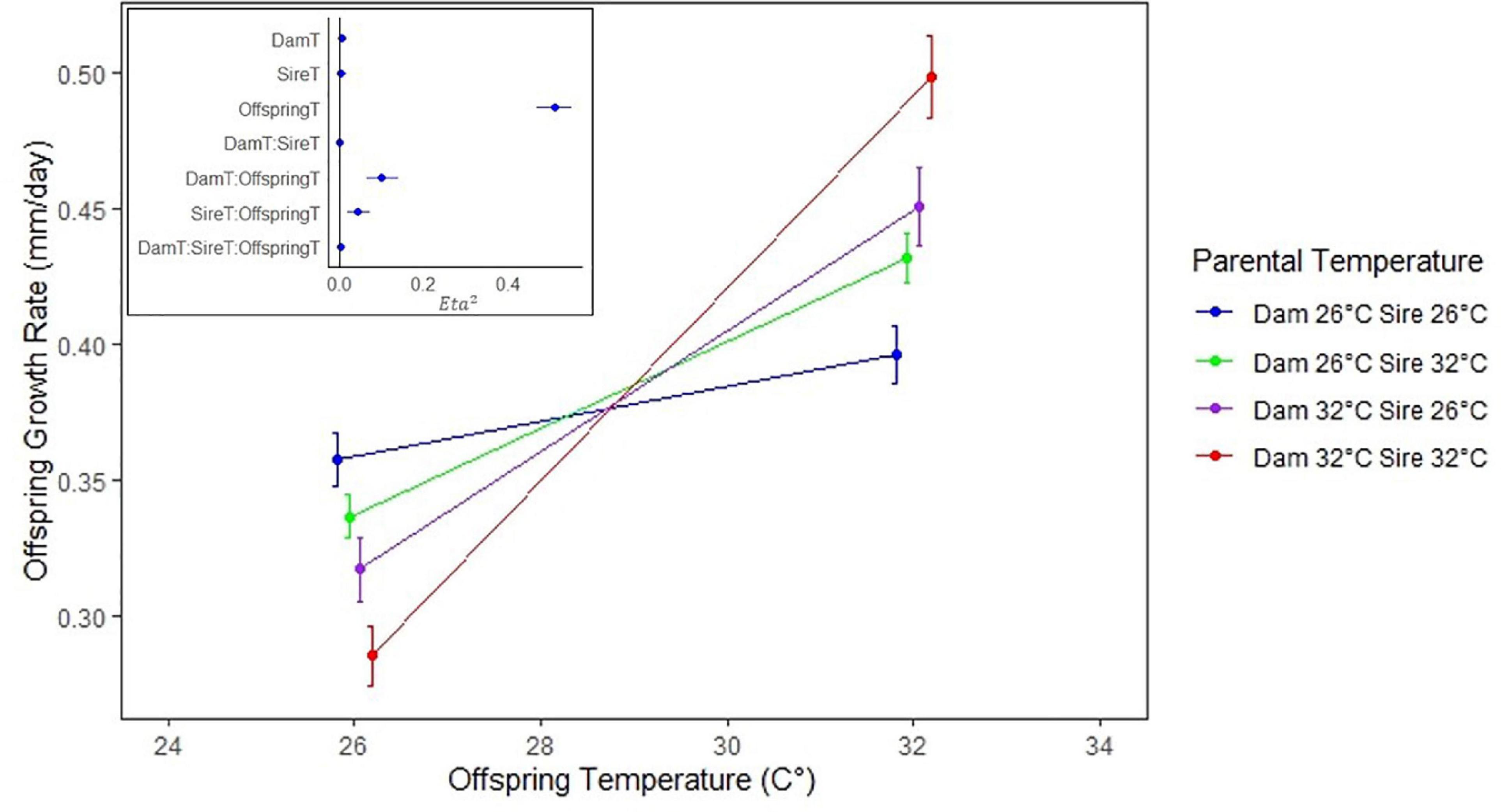
Figure 1. Average growth rate (mm/d) over the first 63 days versus offspring temperature (°C). The red line indicates growth of offspring whose sire and dam were both at 32 (°C). Similarly, the purple, green, and blue lines correspond to dam/sire temperatures of 32/26, 26/32, and 26/26, respectively. Note that the fastest growing offspring at each temperature were those whose parents were both at the same temperature. Average growth rates were not significantly different between sire temperatures 26 and 32 within the 26 offspring group, or between dam/sire temperatures 26/32 and 32/26 in either offspring temperature group. Error bars denote 95% confidence intervals. Inset: Eta2 for ANOVA results over 63 days of growth, where offspring, dam-offspring, and sire-offspring interactions were found to be significant.
Not surprisingly, offspring growth rate was significantly correlated with offspring rearing temperature (P < 0.001) at all days of growth (Table 2 and Supplementary Tables 1, 2). More interestingly, we found significant interactions between Dam °C × Offspring °C (P < 0.001, interaction coefficient = 0.095) and Sire °C × Offspring °C (P < 0.001, interaction coefficient = 0.057), but we did not find a significant three-way interaction at any time period (Dam °C × Sire °C × Offspring °C, P = 0.155) (Table 2). Consequently, TGP results from the additive sum of Dam °C × Offspring °C and Sire °C × Offspring °C interactions (Figure 1 and Supplementary Figure 1), whereas a significant three-way interaction would have resulted in a multiplicative or exponential influence on varied growth trajectories. Additionally, we found that average growth rates were not significantly different between sire temperatures 26 and 32°C within the 26°C offspring group, or between dam/sire temperatures 26/32 and 32/26 in either offspring temperature group (Supplementary Figure 2).
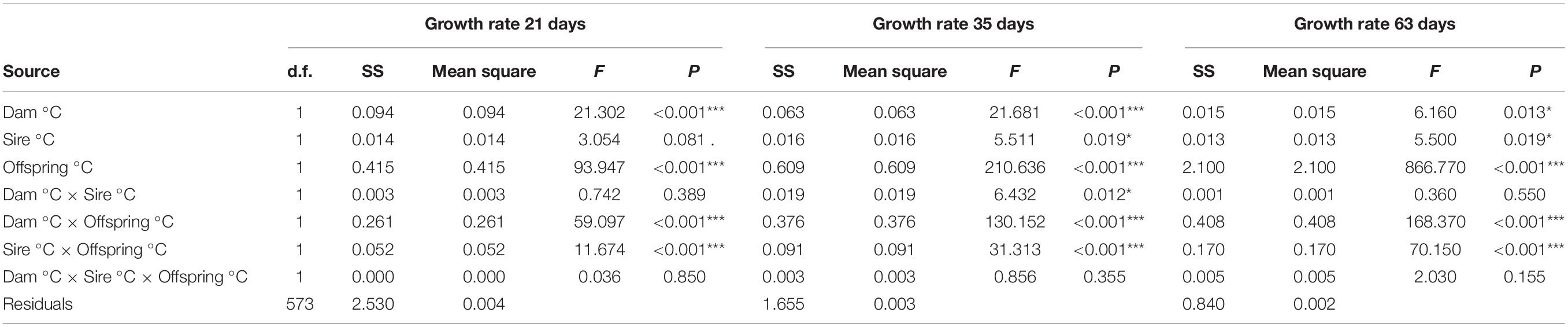
Table 2. 3-way ANOVA with regression results for growth rate (mm day–1) after 30 days of parental temperature exposure at 21, 35, and 63 days of offspring growth (Significance codes: “***” 0.001; “*” 0.05; “.” 0.1).
After separation of offspring by sex, we found that both female and male offspring exhibited the same trends, with significant two-way interactions between both Dam °C × Offspring °C (P < 0.001) and Sire °C × Offspring °C (P < 0.05) on growth rate after 21 days of growth (Supplementary Tables 1, 2). However, among female offspring, we found a significant three-way interaction by 63 days of growth (Dam °C × Sire °C × Offspring °C, P = 0.006) (Supplementary Table 1).
For both female and male offspring, GSI was most significantly affected by offspring temperature (P < 0.001) (Table 3 and Figure 2). Additionally, the trends in GSI across parent temperature treatments paralleled that for growth rate, such that the highest GSI for both offspring sexes was observed when sires, dams, and offspring were at 32°C (Figures 2A,B). However, we found that the effects of parental temperature were generally not significant when compared to the effects of offspring temperature, owing to a larger variance in GSI. Only the interaction between Dam °C × Offspring °C was significant for male offspring (P < 0.001) (Table 3). Among female offspring, only the Offspring °C effect was significant (P < 0.001); with no significant interaction between Dam °C × Offspring °C (P = 0.099) or Sire °C × Offspring °C (P = 0.309) (Table 3 and Figure 2B). In addition, growth rate did not affect GSI for either sex of offspring, as inclusion in the model did not significantly improve model fit and ANOVA with growth rate as a covariate for GSI did not alter results. Overall, we found trends of high parental contribution during the earliest weeks of growth that decreased over the first 5 weeks of age (Figure 3).
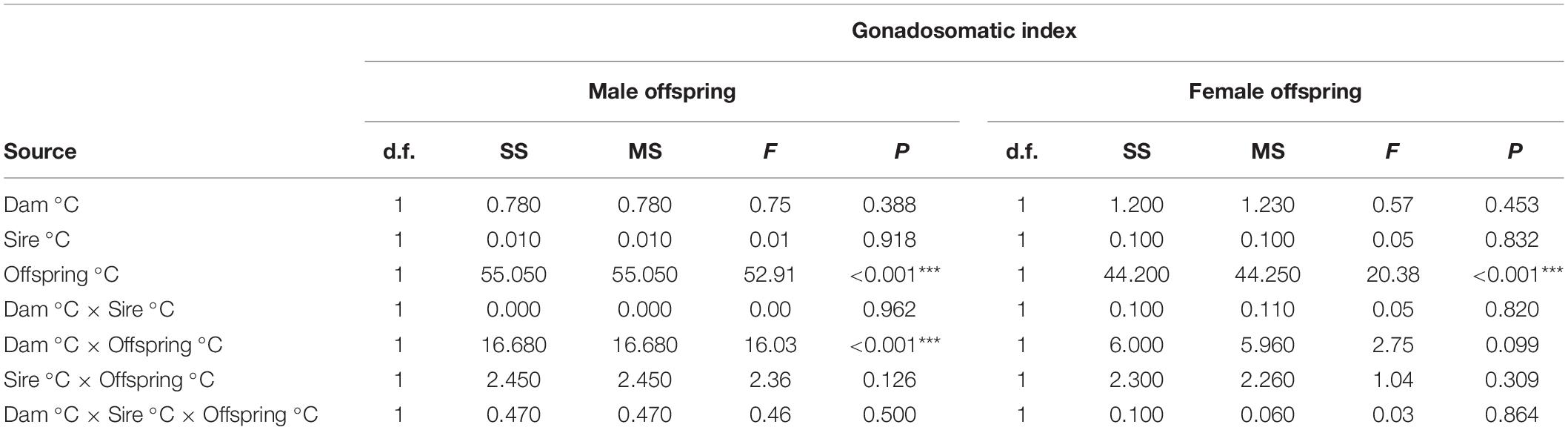
Table 3. ANOVA results for GSI after 30 days of parental temperature exposure and 63 days of offspring growth (Significance codes: “***” 0.001).
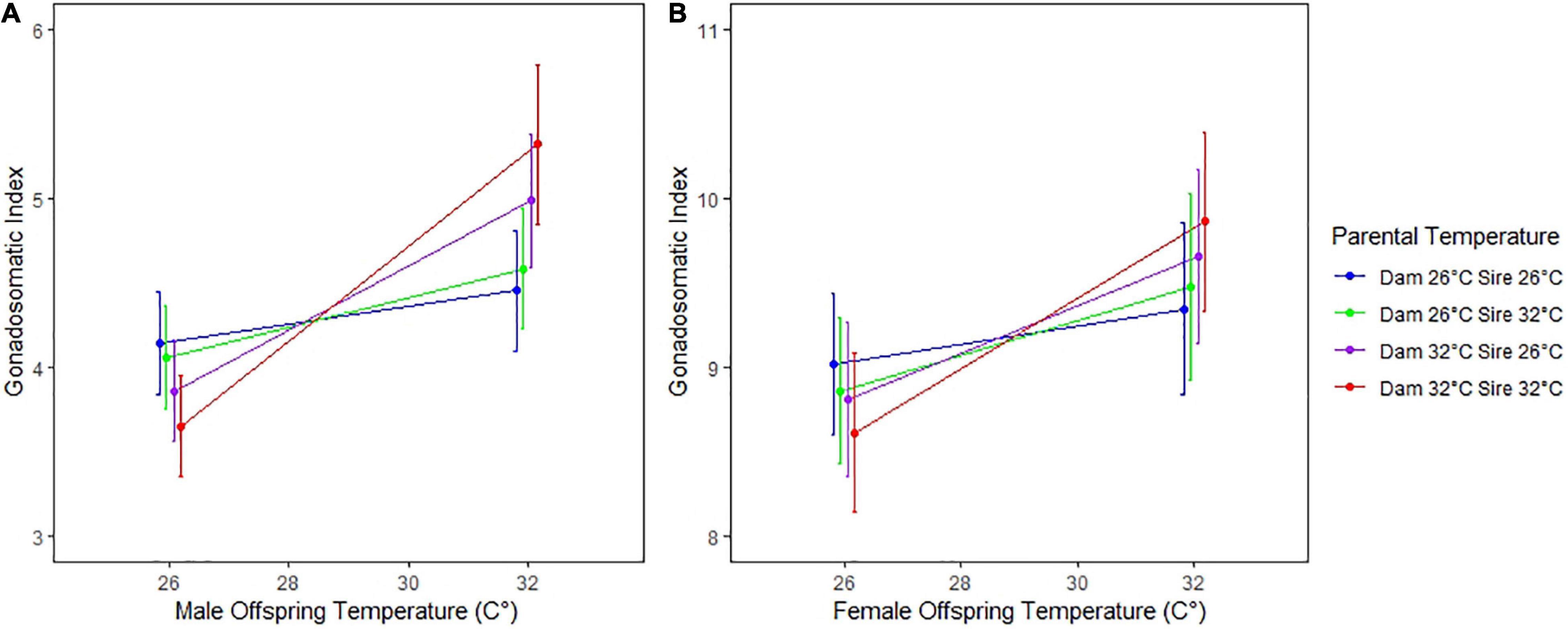
Figure 2. (A) Gonosomatic index for male offspring versus offspring temperature (°C). (B) Gonosomatic index for female offspring versus offspring temperature (°C). The red line indicates GSI for offspring whose sire and dam were both at 32 (°C). Similarly, the purple, green, and blue lines correspond to dam/sire temperatures of 32/26, 26/32, and 26/26, respectively. Note that the GSI is largest (hence maturation fastest) for offspring whose parents were both at the same temperature. Error bars denote 95% confidence intervals.
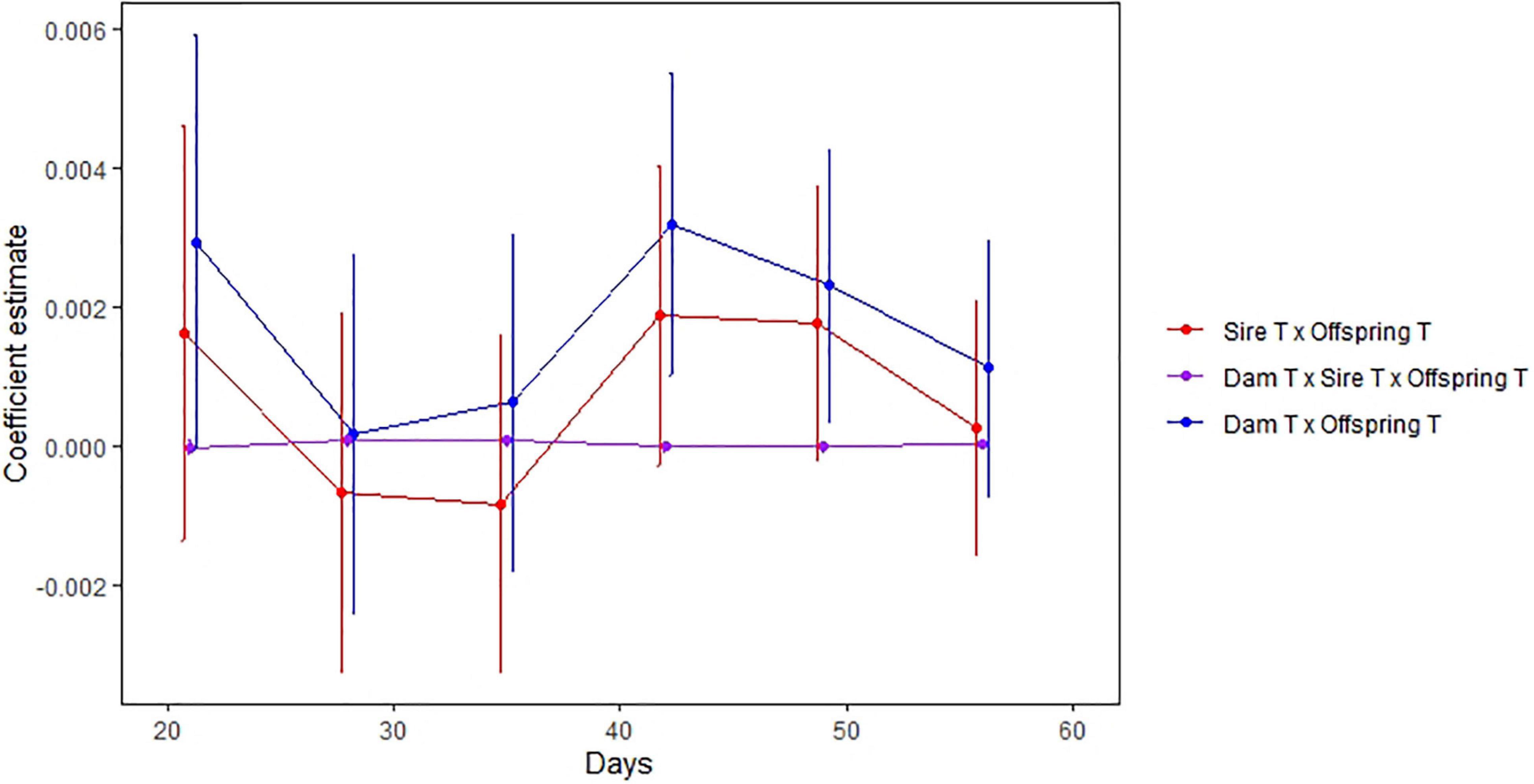
Figure 3. Degree of TGP versus offspring age. The horizontal axis is the age (days) of offspring and the vertical axis is the parent temperature x offspring temperature interaction effect on offspring growth over the previous week. The interaction measures the change in the slope of the growth v. temperature reaction norm attributed to dam (blue) and sire (red) temperature treatments. Effects for dams and sires are largely parallel, with peaks at 20 and 42 days. Error bars denote residual standard error.
Discussion
Our results are consistent with prior research examining thermal TGP effects on growth rate in fish including, but not limited to: sheepshead minnows, sticklebacks, and tropical reef fish (Donelson et al., 2012; Salinas and Munch, 2012; Shama and Wegner, 2014; Donelson et al., 2018). Our study extends results from previous studies in two ways. First, we found that that TGP effects on growth rate clearly comes from both sires and dams. Importantly, no three-way interaction between dam, sire, and offspring temperature was found affecting offspring growth rate, indicating that the contribution from the parents is additive rather than multiplicative. Moreover, as sire and dam both contribute to TGP effects, the mean parent environment may be sufficient to predict TGP. To evaluate this idea, we repeated the ANOVA using the average parent temperature instead of independent sire and dam temperatures. This reduced model fits the data nearly as well (R2 = 0.65, compared with R2 = 0.66 for the model with distinct parent temperatures). Hence, at least for sheepshead minnows, the mean parent temperature is sufficient for predicting offspring thermal performance.
Secondly, we found that TGP depends on the sex of the offspring; although offspring growth was affected by both parents regardless of sex, male offspring had no significant three-way interaction at any time point, whereas for female offspring growth, the three-way interaction between sire, dam, and offspring temperature effects were significant at 63 days of growth (P < 0.05) and may indicate that TGP can differentially impact growth rate (Figure 2 and Supplementary Tables 1, 2). This suggests a sex-linked epigenetic mark for certain traits. Existing research on differentiated epigenetic inheritance by sex of the parent is largely focused on maternal impacts (Dunn et al., 2011) thus far, though both paternal and maternal methylation imprints have been observed in mice (Heard and Martienssen, 2014). Recent research on paternal influences in zebrafish have revealed that patterns in paternal DNA methylation are found to be maintained throughout early development of the embryo, allowing for paternal epigenetic signatures to be passed on to offspring (Jiang et al., 2013; Skvortsova et al., 2019).
Previous studies have found a significant interaction between parental treatment temperature and offspring rearing temperature on growth rate (Salinas and Munch, 2012; Shama et al., 2014; Lee et al., 2020; Munch et al., 2021). Here, we found that transgenerational effects on growth rate extend out to 63 days post-hatch, indicating that thermal TGP persists throughout the entire juvenile stage. Consistent with previous studies on maternal effects (e.g., Gao and Munch, 2013), parental contributions were greatest early in life and decreased over the first 5 weeks of age (Figure 3). TGP then increased with offspring age to a maximum around 42 days. This may be due to a shift in maturation stages between seven to 8 weeks of growth (Lee et al., 2017). In contrast, other TGP studies in marine sticklebacks have found significant 3-way interactions after 90 days (Shama and Wegner, 2014).
In many fishes, mortality in larval and juvenile stages is extremely size selective (Perez and Munch, 2010) suggesting that TGP should increase offspring survival to maturity. However, we recognize that rapid juvenile growth may have trade-offs with other aspects of performance in fishes, such as swimming speed, behavioral aggression, and somatic energy storage (Schultz and Conover, 1997; dos Santos Schmidt et al., 2021; Mengistu et al., 2021; Monnet et al., 2021). In light of possible growth-fecundity trade-offs, we also examined the impacts of TGP on GSI. We found that GSI significantly increased with offspring temperature and varied with parent temperature in a manner strongly consistent with TGP, although this effect was not significant. This is consistent with the results of Munch et al. (2021), which did find significant TGP in GSI in sheepshead minnows. Hence, although recent meta-analyses have questioned the adaptive value of transgenerational effects (Sánchez-Tójar et al., 2020), the evidence presented here suggests that thermal TGP is adaptive whenever the average parental environment is positively correlated with temperatures their offspring encounter.
Kielland et al. (2017) did not find evidence for TGP in the zooplankton Daphnia pulex and suggested that much prior evidence for TGP resulted from experimental artifacts. In light of this, it is worth evaluating potential confounds in our results. The most obvious potential problems are: (a) genetic differences among parents assigned to each temperature treatment; (b) selection on parents during temperature treatment; and (c) selection on offspring during spawning and subsequent rearing. Since the random effects of sire and dam are minimal and non-significant, there is little evidence for substantial genetic differences among parents. Moreover, since no parents died during the temperature treatment and fecundity was equalized by artificial breeding, there is no opportunity for selection on parents to affect the results. Finally, previous work on sheepshead minnows demonstrated that rearing protocols are not responsible for the differences in offspring growth associated with different parent temperature treatments (i.e., the same protocols do not produce TGP when the parent exposure time is short) (Salinas and Munch, 2012; Munch et al., 2021). Therefore, we are reasonably confident that TGP in sheepshead minnows is not an experimental artifact. Moreover, the fact that sire-driven TGP is nearly equal to that contributed by dams after 3 weeks of growth and persists to grand-offspring (Lee et al., 2020), strongly suggest that thermal TGP in offspring growth is epigenetic, though further work is needed to elucidate a specific mechanism, e.g., methylation (Jiang et al., 2013; Skvortsova et al., 2019).
Environmental conditions are changing rapidly in both marine and freshwater environments (Scavia et al., 2002) and TGP may dampen selection resulting from a change in environment (Donelson et al., 2018). As such, TGP may mitigate population decline by alleviating a “phenotype-environment mismatch,” ultimately buying time for evolutionary rescue (DeWitt et al., 1998; Marshall et al., 2010; Harmon and Pfennig, 2021). However, although plasticity represents an organismal capacity for extremely rapid phenotypic changes over a single generation, it is unclear how thermal TGP will affect species responses over the long term. For instance, multiple traits may be affected in addition to growth, such as the skewing of offspring gender ratios (Donelson and Munday, 2015), thermosensory behavioral adjustments (Abram et al., 2017), migration pattern (Merlin and Liedvogel, 2019), disease resistance (Moghadam et al., 2015), transgenerational immune priming (Freitak et al., 2009), and hatching success (Stillwell and Fox, 2005).
In addition, although theoretical studies abound (Gomulkiewicz and Holt, 1995; Lande, 2009; McCaw et al., 2020) we know next to nothing about how TGP evolves in natural populations. Walsh et al. (2016) compared the degree of TGP in response to predator cues among locally adapted populations and found that the degree of TGP depended on the predictability of predator presence in the native habitat. Moreover, Munch et al. (2021) found that the degree of TGP in sheepshead minnows from different latitudes changes in proportion to the predictability of the thermal environment. Although these geographic patterns are consistent with TGP evolving in response to environmental gradients, they do not tell us how fast TGP will evolve - a critical issue for the impact of a changing climate. In addition, climate change involves multiple drivers and future research should investigate the extent at which TGP could buffer populations with multiple environmental stressors such as hypoxia and ocean acidification (Gobler et al., 2018; Roman et al., 2019). In light of increasingly severe climatic shifts and decreasing environmental predictability, studies elucidating the extent of TGP effects on key physiological and behavioral traits critical to population persistence are an important next step.
Data Availability Statement
The raw data supporting the conclusions of this article are available on the Open Science Forum repository (Identifier: doi: 10.17605/OSF.IO/X2U6H).
Ethics Statement
The animal study was reviewed and approved by IACUC, Office of Research Compliance, UCSC.
Author Contributions
SM conceived and designed the study. SC and W-SL performed the research and conducted analyses. All authors contributed to the manuscript.
Funding
This research was generously supported by a Pew Marine Conservation Fellowship to SM and NSF OCE grant #1130483. W-SL was partly supported by the research, “Development of an integrated disease-ecology assessment system” (GP2021-15), funded by the Korea Environment Institute (KEI).
Conflict of Interest
The authors declare that the research was conducted in the absence of any commercial or financial relationships that could be construed as a potential conflict of interest.
Publisher’s Note
All claims expressed in this article are solely those of the authors and do not necessarily represent those of their affiliated organizations, or those of the publisher, the editors and the reviewers. Any product that may be evaluated in this article, or claim that may be made by its manufacturer, is not guaranteed or endorsed by the publisher.
Acknowledgments
We thank Jamie Bongard for experimental assistance.
Supplementary Material
The Supplementary Material for this article can be found online at: https://www.frontiersin.org/articles/10.3389/fmars.2021.734318/full#supplementary-material
Supplementary Figure 1 | Interaction plot of average offspring growth rate (mm/d) over the first 63 days versus offspring temperature (°C). The red line indicates growth of offspring at 32, and blue line growth of offspring at 26. Note that the fastest growing offspring at each temperature were those whose parents were both at the same temperature. Error bars denote 95% confidence intervals.
Supplementary Figure 2 | Tukey test for analysis of significance in growth rate between temperature treatment groups. Statistically significant groups did not have the 95% confidence bar overlap with zero difference in mean levels.
References
Abram, P. K., Boivin, G., Moiroux, J., and Brodeur, J. (2017). Behavioural effects of temperature on ectothermic animals: unifying thermal physiology and behavioural plasticity. Biol. Rev. 92, 1859–1876. doi: 10.1111/brv.12312
Atkinson, D. (1995). Effects of temperature on the size of aquatic ectotherms: exceptions to the general rule. J. Therm. Biol. 20, 61–74. doi: 10.1016/0306-4565(94)00028-H
Bates, D., Mächler, M., Bolker, B., and Walker, S. (2015). Fitting linear mixed-effects models using lme4. J. Stat. Softw. 67, 1–48. doi: 10.18637/jss.v067.i01
Baxter, F. A., and Drake, A. J. (2019). Non-genetic inheritance via the male germline in mammals. Philos. Trans. R. Soc. B Biol. Sci. 374:20180118. doi: 10.1098/rstb.2018.0118
Bennett, W. A., and Beitinger, T. L. (1997). Temperature tolerance of the sheepshead minnow, cyprinodon variegatus. Copeia 1997:77. doi: 10.2307/1447842
Berkeley, S. A., Chapman, C., and Sogard, S. M. (2004). Maternal age as a determinant of larval growth and survival in a marine fish, sebastes melanops. Ecology 85, 1258–1264. doi: 10.1890/03-0706
Clark, R. A., Fox, C. J., Viner, D., and Livermore, M. (2003). North Sea cod and climate change – modelling the effects of temperature on population dynamics. Glob. Change Biol. 9, 1669–1680. doi: 10.1046/j.1365-2486.2003.00685.x
Cooney, C. A., Dave, A. A., and Wolff, G. L. (2002). Maternal methyl supplements in mice affect epigenetic variation and DNA methylation of offspring. J. Nutr. 132(8 Suppl), 2393S–2400S. doi: 10.1093/jn/132.8.2393S
Crean, A. J., Dwyer, J. M., and Marshall, D. J. (2013). Adaptive paternal effects? Experimental evidence that the paternal environment affects offspring performance. Ecology 94, 2575–2582. doi: 10.1890/13-0184.1
Curley, J. P., Mashoodh, R., and Champagne, F. A. (2011). Epigenetics and the origins of paternal effects. Horm. Behav. 59, 306–314. doi: 10.1016/j.yhbeh.2010.06.018
DeWitt, T. J., Sih, A., and Wilson, D. S. (1998). Costs and limits of phenotypic plasticity. Trends Ecol. Evol. 13, 77–81. doi: 10.1016/S0169-5347(97)01274-3
Dias, B. G., and Ressler, K. J. (2014). Parental olfactory experience influences behavior and neural structure in subsequent generations. Nat. Neurosci. 17, 89–96. doi: 10.1038/nn.3594
Donelson, J. M., and Munday, P. L. (2015). Transgenerational plasticity mitigates the impact of global warming to offspring sex ratios. Glob. Change Biol. 21, 2954–2962. doi: 10.1111/gcb.12912
Donelson, J. M., Munday, P. L., McCormick, M. I., and Pitcher, C. R. (2012). Rapid transgenerational acclimation of a tropical reef fish to climate change. Nat. Clim. Change 2, 30–32. doi: 10.1038/nclimate1323
Donelson, J. M., Salinas, S., Munday, P. L., and Shama, L. N. S. (2018). Transgenerational plasticity and climate change experiments: Where do we go from here? Glob. Change Biol. 24, 13–34. doi: 10.1111/gcb.13903
dos Santos Schmidt, T. C., Hay, D. E., Sundby, S., Devine, J. A., Óskarsson, G. J., Slotte, A., et al. (2021). Adult body growth and reproductive investment vary markedly within and across Atlantic and Pacific herring: a meta-analysis and review of 26 stocks. Rev. Fish Biol. Fish. 31, 685–708. doi: 10.1007/s11160-021-09665-9
Dunn, G. A., Morgan, C. P., and Bale, T. L. (2011). Sex-specificity in transgenerational epigenetic programming. Horm. Behav. 59, 290–295. doi: 10.1016/j.yhbeh.2010.05.004
Ernande, B., Dieckmann, U., and Heino, M. (2004). Adaptive changes in harvested populations: plasticity and evolution of age and size at maturation. Proc. Biol. Sci. 271, 415–423.
Freitak, D., Heckel, D. G., and Vogel, H. (2009). Dietary-dependent trans-generational immune priming in an insect herbivore. Proc. Biol. Sci. 276, 2617–2624. doi: 10.1098/rspb.2009.0323
Galloway, L. F., and Etterson, J. R. (2007). Transgenerational plasticity is adaptive in the wild. Science 318, 1134–1136. doi: 10.1126/science.1148766
Gao, J., and Munch, S. (2013). Genetic and maternal variation in early growth in the Atlantic silverside Menidia menidia. Mar. Ecol. Prog. Ser. 485, 211–222. doi: 10.3354/meps10333
Gillooly, J. F., Brown, J. H., West, G. B., Savage, V. M., and Charnov, E. L. (2001). Effects of size and temperature on metabolic rate. Science 293, 2248–2251. doi: 10.1126/science.1061967
Gobler, C. J., Merlo, L. R., Morrell, B. K., and Griffith, A. W. (2018). Temperature, acidification, and food supply interact to negatively affect the growth and survival of the forage fish, Menidia beryllina (Inland Silverside), and Cyprinodon variegatus (Sheepshead Minnow). Front. Mar. Sci 5:86. doi: 10.3389/fmars.2018.00086
Gomulkiewicz, R., and Holt, R. D. (1995). When does evolution by natural selection prevent extinction? Evolution 49, 201–207. doi: 10.2307/2410305
Grindstaff, J. L., Brodie, E. D., and Ketterson, E. D. (2003). Immune function across generations: integrating mechanism and evolutionary process in maternal antibody transmission. Proc. Biol. Sci. 270, 2309–2319. doi: 10.1098/rspb.2003.2485
Groothuis, T. G. G., and Schwabl, H. (2008). Hormone-mediated maternal effects in birds: mechanisms matter but what do we know of them? Philos. Trans. R. Soc. Lond. B. Biol. Sci. 363, 1647–1661. doi: 10.1098/rstb.2007.0007
Hani, Y. M. I., Turies, C., Palluel, O., Delahaut, L., Bado-Nilles, A., Geffard, A., et al. (2019). Effects of a chronic exposure to different water temperatures and/or to an environmental cadmium concentration on the reproduction of the threespine stickleback (Gasterosteus aculeatus). Ecotoxicol. Environ. Saf. 174, 48–57. doi: 10.1016/j.ecoenv.2019.02.032
Harmon, E. A., and Pfennig, D. W. (2021). Evolutionary rescue via transgenerational plasticity: evidence and implications for conservation. Evol. Dev. 23, 292–307. doi: 10.1111/ede.12373
Heard, E., and Martienssen, R. A. (2014). Transgenerational epigenetic inheritance: myths and mechanisms. Cell 157, 95–109. doi: 10.1016/j.cell.2014.02.045
Heckwolf, M. J., Meyer, B. S., Döring, T., Eizaguirre, C., and Reusch, T. B. H. (2018). Transgenerational plasticity and selection shape the adaptive potential of sticklebacks to salinity change. Evol. Appl. 11, 1873–1885. doi: 10.1111/eva.12688
Hoegh-Guldberg, O., Mumby, P. J., Hooten, A. J., Steneck, R. S., Greenfield, P., Gomez, E., et al. (2007). Coral reefs under rapid climate change and ocean acidification. Science 318, 1737–1742. doi: 10.1126/science.1152509
Jiang, L., Zhang, J., Wang, J.-J., Wang, L., Zhang, L., Li, G., et al. (2013). Sperm, but not oocyte, DNA methylome is inherited by zebrafish early embryos. Cell 153, 773–784. doi: 10.1016/j.cell.2013.04.041
Kielland, Ø. N., Bech, C., and Einum, S. (2017). No evidence for thermal transgenerational plasticity in metabolism when minimizing the potential for confounding effects. Proc. Biol. Sci. 284:20162494. doi: 10.1098/rspb.2016.2494
Kingsolver, J. G., and Huey, R. B. (1998). Evolutionary analyses of morphological and physiological plasticity in thermally variable environments. Am. Zool. 38, 545–560. doi: 10.1093/icb/38.3.545
Lande, R. (2009). Adaptation to an extraordinary environment by evolution of phenotypic plasticity and genetic assimilation. J. Evol. Biol. 22, 1435–1446. doi: 10.1111/j.1420-9101.2009.01754.x
Lee, W., Salinas, S., Lee, Y., Siskidis, J. A., Mangel, M., and Munch, S. B. (2020). Thermal transgenerational effects remain after two generations. Ecol. Evol. 10, 11296–11303. doi: 10.1002/ece3.6767
Lee, W.-S., Mangel, M., and Munch, S. B. (2017). Developmental order of a secondary sexual trait reflects gonadal development in male sheepshead minnows (Cyprinodon variegatus). Evol. Ecol. Res. 18, 531–538.
Marshall, D. J., Monro, K., Bode, M., Keough, M. J., and Swearer, S. (2010). Phenotype–environment mismatches reduce connectivity in the sea. Ecol. Lett. 13, 128–140. doi: 10.1111/j.1461-0248.2009.01408.x
Marteinsdottir, G., and Steinarsson, A. (1998). Maternal influence on the size and viability of Iceland cod Gadus morhua eggs and larvae. J. Fish Biol. 52, 1241–1258. doi: 10.1111/j.1095-8649.1998.tb00969.x
McCaw, B. A., Stevenson, T. J., and Lancaster, L. T. (2020). Epigenetic responses to temperature and climate. Integr. Comp. Biol. 60, 1469–1480. doi: 10.1093/icb/icaa049
McGhee, K. E., Barbosa, A. J., Bissell, K., Darby, N. A., and Foshee, S. (2021). Maternal stress during pregnancy affects activity, exploration and potential dispersal of daughters in an invasive fish. Anim. Behav. 171, 41–50. doi: 10.1016/j.anbehav.2020.11.003
Mengistu, S. B., Palstra, A. P., Mulder, H. A., Benzie, J. A. H., Trinh, T. Q., Roozeboom, C., et al. (2021). Heritable variation in swimming performance in Nile tilapia (Oreochromis niloticus) and negative genetic correlations with growth and harvest weight. Sci. Rep. 11:11018. doi: 10.1038/s41598-021-90418-w
Merlin, C., and Liedvogel, M. (2019). The genetics and epigenetics of animal migration and orientation: birds, butterflies and beyond. J. Exp. Biol. 222(Pt Suppl 1):jeb191890. doi: 10.1242/jeb.191890
Moghadam, H., Mørkøre, T., and Robinson, N. (2015). Epigenetics—potential for programming fish for aquaculture? J. Mar. Sci. Eng. 3, 175–192. doi: 10.3390/jmse3020175
Monnet, G., Rosenfeld, J. S., and Richards, J. G. (2021). Behavioural variation between piscivore and insectivore rainbow trout Oncorhynchus mykiss. J. Fish Biol. 99, 955–963. doi: 10.1111/jfb.14781
Monteleone, D. M., and Houde, E. D. (1990). Influence of maternal size on survival and growth of striped bass Morone saxatilis Walbaum eggs and larvae. J. Exp. Mar. Biol. Ecol. 140, 1–11. doi: 10.1016/0022-0981(90)90076-O
Mousseau, T. A., and Dingle, H. (1991). Maternal effects in insect life histories. Annu. Rev. Entomol. 36, 511–534. doi: 10.1146/annurev.en.36.010191.002455
Mousseau, T. A., and Fox, C. W. (1998). The adaptive significance of maternal effects. Trends Ecol. Evol. 13, 403–407. doi: 10.1016/S0169-5347(98)01472-4
Munch, S. B., Lee, W. S., Walsh, M., Hurst, T., Wasserman, B. A., Mangel, M., et al. (2021). A latitudinal gradient in thermal transgenerational plasticity and a test of theory. Proc. Biol. Sci. 288:20210797.
Perez, K. O., and Munch, S. B. (2010). Extreme selection on size in the early lives of fish. Evolution 64, 2450–2457. doi: 10.1111/j.1558-5646.2010.00994.x
Pörtner, H. O., Berdal, B., Blust, R., Brix, O., Colosimo, A., De Wachter, B., et al. (2001). Climate induced temperature effects on growth performance, fecundity and recruitment in marine fish: developing a hypothesis for cause and effect relationships in Atlantic cod (Gadus morhua) and common eelpout (Zoarces viviparus). Cont. Shelf Res. 21, 1975–1997. doi: 10.1016/S0278-4343(01)00038-3
Roach, D. A., and Wulff, R. D. (1987). Maternal effects in plants. Annu. Rev. Ecol. Syst. 18, 209–235. doi: 10.1146/annurev.es.18.110187.001233
Roman, M. R., Brandt, S. B., Houde, E. D., and Pierson, J. J. (2019). Interactive effects of hypoxia and temperature on coastal pelagic zooplankton and fish. Front. Mar. Sci 6:139. doi: 10.3389/fmars.2019.00139
Ruebel, M. L., and Latham, K. E. (2020). Listening to mother: long-term maternal effects in mammalian development. Mol. Reprod. Dev. 87, 399–408. doi: 10.1002/mrd.23336
Rutkowska, J., Lagisz, M., Bonduriansky, R., and Nakagawa, S. (2020). Mapping the past, present and future research landscape of paternal effects. BMC Biol. 18:183. doi: 10.1186/s12915-020-00892-3
Salinas, S., and Munch, S. B. (2012). Thermal legacies: transgenerational effects of temperature on growth in a vertebrate. Ecol. Lett. 15, 159–163. doi: 10.1111/j.1461-0248.2011.01721.x
Sánchez-Tójar, A., Lagisz, M., Moran, N. P., Nakagawa, S., Noble, D. W. A., and Reinhold, K. (2020). The jury is still out regarding the generality of adaptive ‘transgenerational’ effects. Ecol. Lett. 23, 1715–1718. doi: 10.1111/ele.13479
Scavia, D., Field, J. C., Boesch, D. F., Buddemeier, R. W., Burkett, V., Cayan, D. R., et al. (2002). Climate change impacts on U.S. coastal and marine ecosystems. Estuaries 25, 149–164. doi: 10.1007/BF02691304
Schneider, C. A., Rasband, W. S., and Eliceiri, K. W. (2012). NIH Image to ImageJ: 25 years of image analysis. Nat. Methods 9, 671–675. doi: 10.1038/nmeth.2089
Schultz, E. T., and Conover, D. O. (1997). Latitudinal differences in somatic energy storage: adaptive responses to seasonality in an estuarine fish (Atherinidae: Menidia menidia). Oecologia 109, 516–529. doi: 10.1007/s004420050112
Shama, L. N. S. (2015). Bet hedging in a warming ocean: predictability of maternal environment shapes offspring size variation in marine sticklebacks. Glob. Change Biol. 21, 4387–4400. doi: 10.1111/gcb.13041
Shama, L. N. S., Strobel, A., Mark, F. C., and Wegner, K. M. (2014). Transgenerational plasticity in marine sticklebacks: maternal effects mediate impacts of a warming ocean. Funct. Ecol. 28, 1482–1493. doi: 10.1111/1365-2435.12280
Shama, L. N. S., and Wegner, K. M. (2014). Grandparental effects in marine sticklebacks: transgenerational plasticity across multiple generations. J. Evol. Biol. 27, 2297–2307. doi: 10.1111/jeb.12490
Skvortsova, K., Tarbashevich, K., Stehling, M., Lister, R., Irimia, M., Raz, E., et al. (2019). Retention of paternal DNA methylome in the developing zebrafish germline. Nat. Commun. 10:3054. doi: 10.1038/s41467-019-10895-6
Stillwell, R. C., and Fox, C. W. (2005). Complex patterns of phenotypic plasticity: interactive effects of temperature during rearing and oviposition. Ecology 86, 924–934. doi: 10.1890/04-0547
Tarka, M., Guenther, A., Niemelä, P. T., Nakagawa, S., and Noble, D. W. A. (2018). Sex differences in life history, behavior, and physiology along a slow-fast continuum: a meta-analysis. Behav. Ecol. Sociobiol. 72:132. doi: 10.1007/s00265-018-2534-2
Uller, T. (2008). Developmental plasticity and the evolution of parental effects. Trends Ecol. Evol. 23, 432–438. doi: 10.1016/j.tree.2008.04.005
Upadhyaya, B., Larsen, T., Barwari, S., Louwagie, E. J., Baack, M. L., and Dey, M. (2017). Prenatal exposure to a maternal high-fat diet affects histone modification of cardiometabolic genes in newborn rats. Nutrients 9:407. doi: 10.3390/nu9040407
Walsh, M. R., Castoe, T., Holmes, J., Packer, M., Biles, K., Walsh, M., et al. (2016). Local adaptation in transgenerational responses to predators. Proc. R. Soc. B Biol. Sci. 283:20152271. doi: 10.1098/rspb.2015.2271
Keywords: transgenerational plasticity, paternal effects, maternal effects, Cyprinodon variegatus, temperature, growth rate, fecundity
Citation: Chang SL, Lee W-S and Munch SB (2021) Separating Paternal and Maternal Contributions to Thermal Transgenerational Plasticity. Front. Mar. Sci. 8:734318. doi: 10.3389/fmars.2021.734318
Received: 30 June 2021; Accepted: 22 September 2021;
Published: 12 October 2021.
Edited by:
Lisa N. S. Shama, Wadden Sea Station Sylt, Alfred Wegener Institute Helmholtz Centre for Polar and Marine Research (AWI), GermanyReviewed by:
Anne Beemelmanns, Memorial University of Newfoundland, CanadaHeather Diana Veilleux, University of Alberta, Canada
Copyright © 2021 Chang, Lee and Munch. This is an open-access article distributed under the terms of the Creative Commons Attribution License (CC BY). The use, distribution or reproduction in other forums is permitted, provided the original author(s) and the copyright owner(s) are credited and that the original publication in this journal is cited, in accordance with accepted academic practice. No use, distribution or reproduction is permitted which does not comply with these terms.
*Correspondence: Sarah L. Chang, Y2hhbmcuc2FyYWhsQGdtYWlsLmNvbQ==