- 1United States Geological Survey, Maine Cooperative Fish and Wildlife Research Unit, Department of Wildlife, Fisheries, and Conservation, University of Maine, Orono, ME, United States
- 2Biology Department, SUNY College at Oneonta, Oneonta, NY, United States
- 3Senator George J. Mitchell Center for Sustainability Solutions, University of Maine, Orono, ME, United States
- 4U.S. Fish and Wildlife Service, Falls Church, VA, United States
- 5National Marine Fisheries Service, Northeast Fisheries Science Center, Woods Hole, MA, United States
- 6Connecticut River Fish and Wildlife Conservation Office, U.S. Fish and Wildlife Service, Sunderland, MA, United States
American shad (Alosa sapidissima) are native to the east coast of North America from the St. Johns River, Florida, to the St. Lawrence River region in Canada. Since the 1800s, dams have reduced access to spawning habitat. To assess the impact of dams, we estimated the historically accessed spawning habitat in coastal rivers (485,618 river segments with 21,113 current dams) based on (i) width, (ii) distance from seawater, and (iii) slope (to exclude natural barriers to migration) combined with local knowledge. Estimated habitat available prior to dam construction (2,752 km2) was 41% greater than current fully accessible habitat (1,639 km2). River-specific population models were developed using habitat estimates and latitudinally appropriate life history parameters (e.g., size at age, maturity, iteroparity). Estimated coast-wide annual production potential was 69.1 million spawners compared with a dammed scenario (41.8 million spawners). Even with optimistic fish passage performance assumed for all dams (even if passage is completely absent), the dam-imposed deficit was alleviated by fewer than 3 million spawners. We estimate that in rivers modeled without dams, 98,000 metric tons of marine sourced biomass and nutrients were annually delivered, 60% of which was retained through carcasses, gametes and metabolic waste. Damming is estimated to have reduced this by more than one third. Based on our results, dams represent a significant and acute constraint to the population and, with other human impacts, reduce the fishery potential and ecological services attributed to the species.
Introduction
The migration of animals remains one of the most recognizable and ecologically spectacular occurrences in nature. Animals from diverse evolutionary lineages share a behavioral solution to the seasonal and ephemeral nature of habitat suitability for different phases of their life histories (Dingle and Drake, 2007). These may occur across wide expanses of aerial, terrestrial and aquatic habitat, and the flux of these organisms spans and inextricably links disparate and sometimes distant ecosystems (Weaver et al., 2018). Organisms transport biomass and nutrients (Kelt and Van Vuren, 2001), sometimes over great distance and large geographic scales. The timing of movements, the food-web function and life-history strategies may all influence ecological significance (Rosenzweig, 1971). These phenomena fundamentally shift interlinked systems from marine to inland ecosystems with annual regularity (Doughty et al., 2016).
Migration in sea-run or diadromous fishes is illustrative of seasonal movements that links the ocean with fresh water habitat. The global patterns of diadromy have been well described (McDowall, 1987) and the general trends have been linked to productivity differences between inland and marine habitats (Gross, 1987) in complex ways (Dodson et al., 2009). These fish species have been important to humans prior to colonization of North America, through to the present day.
Wilcove (2010) describes the four great threats to migratory animals, each of which is a result of human population growth: habitat destruction, overexploitation, climate change and barriers to migration. In coastal regions, all of these threats are evident, but impoundments represent a conservation challenge that results from both complementary and contradictory socioeconomic tradeoffs that directly influence fish populations (Roy et al., 2018; Song et al., 2019; Roy et al., 2020). Migratory fish have declined, somewhat predictably, through the loss of connectivity to habitat critical for the expression of their life history. Damming fundamentally alters the longitudinal connectivity of freshwater ecosystems, particularly for anadromous fish (Hall et al., 2011; Liermann et al., 2012), a fact that has been effectively revealed through the removal of dams in coastal systems (e.g., Watson et al., 2018; Wippelhauser, 2021).
American shad (Alosa sapidissima) are native to the east coast of North America and demonstrate the human toll on migratory organisms. This anadromous clupeid has an extensive native range, from the St. Johns River, Florida, to the St. Lawrence River region in Canada. Adults must enter freshwater to spawn as a critical part of their life history (Zydlewski and McCormick, 1997b; Zydlewski and Wilkie, 2012). While some populations have flourished outside of their native range (e.g., the Columbia River; Petersen et al., 2003), native populations have been, and remain, depleted (Atlantic States Marine Fisheries Commission (ASMFC), 2020). Since the late 1800s, the four great threats to migratory animals have driven precipitous population declines range wide (Bilkovic et al., 2002; Limburg and Waldman, 2009; Hasselman and Limburg, 2012) and remain continuing and persistent threats to this fish (Burdick, 1954; Talbot, 1954; Bradley, 1959; Chittenden, 1969).
The development of American shad fisheries in the 1700s grew to a pattern of over exploitation by the late 19th century that, combined with habitat loss into the 20th century, resulted in a functional collapse of fisheries and fishery closures in the late 20th and early 21st centuries. Historically abundant, this species supported commercial fisheries with coast-wide landings that exceeded 20,000 metric tons (MT) in the late 1890s (Walburg and Nichols, 1967; Hightower et al., 1996; Limburg et al., 2003, Atlantic States Marine Fisheries Commission (ASMFC), 2007). The ability to access upriver habitat permitted great population potential in river systems and provided sustainable human value (Limburg et al., 2003). Early settlers of Cooperstown, New York, were said to have avoided starvation by American shad reaching Otsego Lake, the Susquehanna River’s source, more than 1,000 km from the coast (Taylor, 1995). In the Gulf of Saint Lawrence, American shad reached the Ottawa River, a distance of over 1,100 km (Provost, 1987). Modern fishery impacts are mollified by stricter harvest regulations such as moratoria (Olney and Hoenig, 2001; Atlantic States Marine Fisheries Commission (ASMFC), 2007) but much of the historic human value (economic, recreational and cultural) for this species has been lost with its absence or reduction in coastal rivers due to persistent anthropogenic influences.
Most notably, dam construction greatly restricted access of American shad to spawning and rearing habitat, directly limiting the scope for population and spawner abundance (Rulifson, 1994; Limburg et al., 2003). Dams may provide critical societal functions to meet needs for electricity, water supply, and flood control (Roy et al., 2018) but these dams, their operations, and impoundments may also conflict with fish conservation goals (Song et al., 2019). Fish passage is often a requested and implemented strategy through the Federal Energy Regulatory Commission (FERC) in the United States, but implementation levels can vary widely for these multi decade permits (Vogel and Jansujwicz, 2021).
Latitudinal differences in the life histories of American shad have shaped the influence imposed by dams through their range. Early and influential assessments of these clinal variations in life history traits (i.e., Carscadden and Leggett, 1975; Leggett and Carscadden, 1978; Glebe and Leggett, 1981a) remain heavily relied upon by both researchers and managers. American shad are entirely semelparous (and more fecund) in the southern rivers with a lower length at age (Leggett and Carscadden, 1978; Gilligan-Lunda et al., 2021) than in northerly populations. North of the Cape Fear River in North Carolina, some level of repeat spawning is observed, and the proportion increases in rivers to the north. Northern American shad are larger and are observed to spawn in as many as five (Grote et al., 2014; McBride et al., 2016) and even seven seasons (Provost, 1987). The degree to which this clinal life history variation reflects phenotypic plasticity (versus genotypic differences) remains an important management interest.
American shad exhibit spawning fidelity to their natal rivers (Talbot, 1954; Hill, 1959; Nichols, 1966; Carscadden and Leggett, 1975) with moderate levels of straying (Mansueti and Kolb, 1953; Williams and Daborn, 1984; Melvin et al., 1986). While divergence among rivers in Canada is notable (Hasselman et al., 2010), human mediated transfers among rivers are likely causal to diminished apparent divergence among some populations within the United States (Hasselman et al., 2013). Thus, while genetic discrimination at a river level may be most appropriate, categorization of three main eco-regions (Northern Iteroparous [NI], Southern Iteroparous [SI] and Semelparous [SM]) provides a convenient and logistically functional division of American shad rivers (Figure 1; Atlantic States Marine Fisheries Commission (ASMFC), 2020).
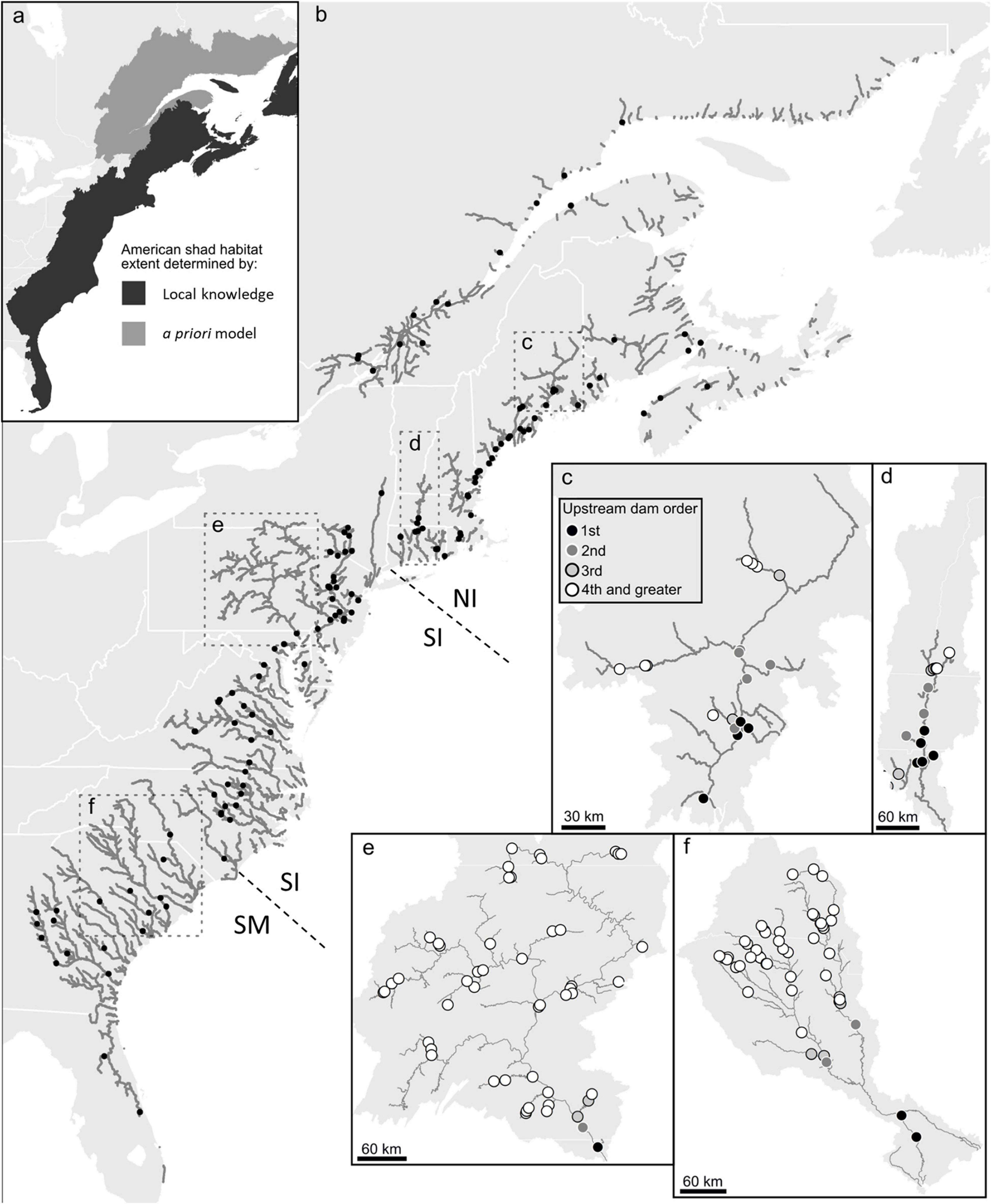
Figure 1. Estimated American shad habitat through a combination of local knowledge and a priori modeling along the eastern coast of North America (a), the full extent of their historic range. In the full panel (b), gray lines indicate suitable riverine habitat. Only the first dam on each river or tributary is shown (black circles) for clarity. Dashed lines indicate divisions between northern iteroparous (NI), southern iteroparous (SI) and semelparous SM) eco-regions Insets provide greater detail for four rivers; Penobscot River (c), Connecticut River (d), Susquehanna River (e), Cooper River and (f) the Santee River, indicating the numerous impoundments that constrain the migration of American shad in these watersheds.
Habitat obstruction or inadequate dam passage may influence populations in these three eco-regions differently. For all eco-regions, access to spawning habitat represents a significant outcome of effective upstream fish passage through dams. The survival of both juveniles and adult migrants during downstream migration is important for population dynamics (Castro-Santos and Letcher, 2010; Stich et al., 2019), albeit to varying degrees for each eco-region. In the SM eco-region, downstream adult passage is not required as they will have made their terminal migration. For the SI and NI eco-regions, the importance of downstream passage for adults is of obvious importance. Failure to provide downstream passage results in an ecological trap that truncates the age distribution of the population (Stich et al., 2019). This is important as repeat spawners have increased reproductive potential (higher fecundity) and provide a buffer against poor recruitment years (Carscadden and Leggett, 1975). The progeny of adult spawners that successfully ascend a dam in all eco-regions, also must all pass downstream as juveniles to reach the ocean.
Together, differences in the life histories among eco-regions may differentially influence the delivery of biomass and nutrients to a river. Semelparous Pacific Salmon (Oncorhynchus spp.; Cederholm et al., 1999; Naiman et al., 2002; and Schindler et al., 2003) and sea lamprey (Petromyzon marinus; Weaver et al., 2016) deliver significant nutrients subsidies through the decay of carcasses. With northern populations displaying lower spawning mortality rates and a greater instance of repeat spawning than southern populations, nutrient delivery through excretion and spawning (gametes) may be most important. American shad generally do not feed upon entering rivers, but their mass loss during the run can be considerable (Glebe and Leggett, 1981a,b; Leonard and McCormick, 1999; Walter and Olney, 2003). Regardless of the source, the level to which the historical delivery of marine nutrients, and the seaward return of nutrients by juveniles, has shifted because of dams.
Conservation efforts on the Gulf of Maine’s Penobscot River resulted in the removal of two main-stem dams (Opperman et al., 2011) and spurred interest in understanding the restoration potential of American shad for this river which historically had supported an abundant population. Annual landings of over 2 million adults were reported in the 1860s (Foster and Atkins, 1867) and American shad had been a critical fishery for the Penobscot Nation. Trinko Lake et al. (2012) defined the spatial extent of American shad habitat in this system and characterized connectivity. The development of a simple population model provided a means of assessing the influence of dam removals on the production potential of a newly opened river (Bailey and Zydlewski, 2013) but fell short in providing a tool that incorporated fish passage. Stich et al. (2019) developed a generalizable model platform that could be applied to any river system based on management needs. This approach allowed for the assessment of upstream and downstream passage on river-specific productivity. The importance of understanding fish passage in light of both upstream and downstream efficiencies and survival was made evident. This approach was then applied to a series of rivers, range wide, based on informed habitat assessments (Gilligan-Lunda et al., 2021). Similar work by Barber et al. (2018) used this population modeling approach to characterize the delivery of nutrient by another alosine, the alewife (Alosa pseudoharengus), by employing estimates of nutrient loss, delivery, and exodus.
In this paper we aspire to implement these complementary approaches of spatial analysis, river specific population modeling and assessment of nutrient dynamics to address the lament of Limburg et al. (2003):
We can only imagine today what full Atlantic coastal ecosystems (rivers, estuaries, and coastal marine areas) looked like, but one thing is clear, shad played a far more important role then than they do today. To plan for a sustainable future for American shad, we should reenvision those systems and strive to balance fisheries demands with their ecological function. To do this will require better modeling, better data, and above all, renewed commitment.
To estimate the opportunity cost realized by this species through dam construction throughout its range, we sought to first estimate habitat that was historically and currently exploited by American shad for spawning in Atlantic coastal rivers. This was accomplished by characterizing 485,618 river reaches and 21,113 dams. We identified potential spawning habitat based on criteria of (i) river width, (ii) distance from seawater intrusion and (iii) slope (to exclude natural barriers to migration) combined with local knowledge. The areas of potential spawning habitat were aggregated to estimate historic habitat available prior to the construction of dams and impoundments for each coastal river. Each river was then assessed using a life history-based population model incorporating latitudinally appropriate life history parameters (e.g., clines in size at age, maturity rates, iteroparity, and maximum age). In aggregate this approach allowed a direct assessment of the theoretical spawning potential lost coast-wide to the construction of dams and allowed us to estimate the historic and current capacities for biomass and nutrient delivery.
Methods
Characterization of American Shad Habitat Through Their Native Range
We estimated American shad habitat based on available knowledge of habitat extent, area, and accessibility. These data were collected for the entire historic geographic extent of American shad, spanning eastern United States and Canada using a two-step approach. First, we used the United States National Hydrography Dataset (USGS, 2019) and Canadian National Hydrographic Network (Natural Resources Canada, 2019) to determine the potential freshwater networks available for migration, spawning, and rearing. The data were organized as a series of flowline segments, representing interconnected stream and river reach segments (Figure 2). To simplify this analysis, we assumed initially that American shad would not migrate to reach segments with a mean channel width of less than 15 m, in accordance with pre-existing habitat suitability models (Stier and Crance, 1985; Harris and Hightower, 2012). Second, we validated our a priori assessment of historic habitat extent of American shad with the help of local experts from each state or province in our study region (see section “ACKNOWLEDGMENTS”). The experts modified historic extents based on the presence of natural barriers (e.g., steep rapids, waterfalls) or environmental conditions (e.g., temperature, salinity, and reach segment width) that are unsuitable for American shad spawning.
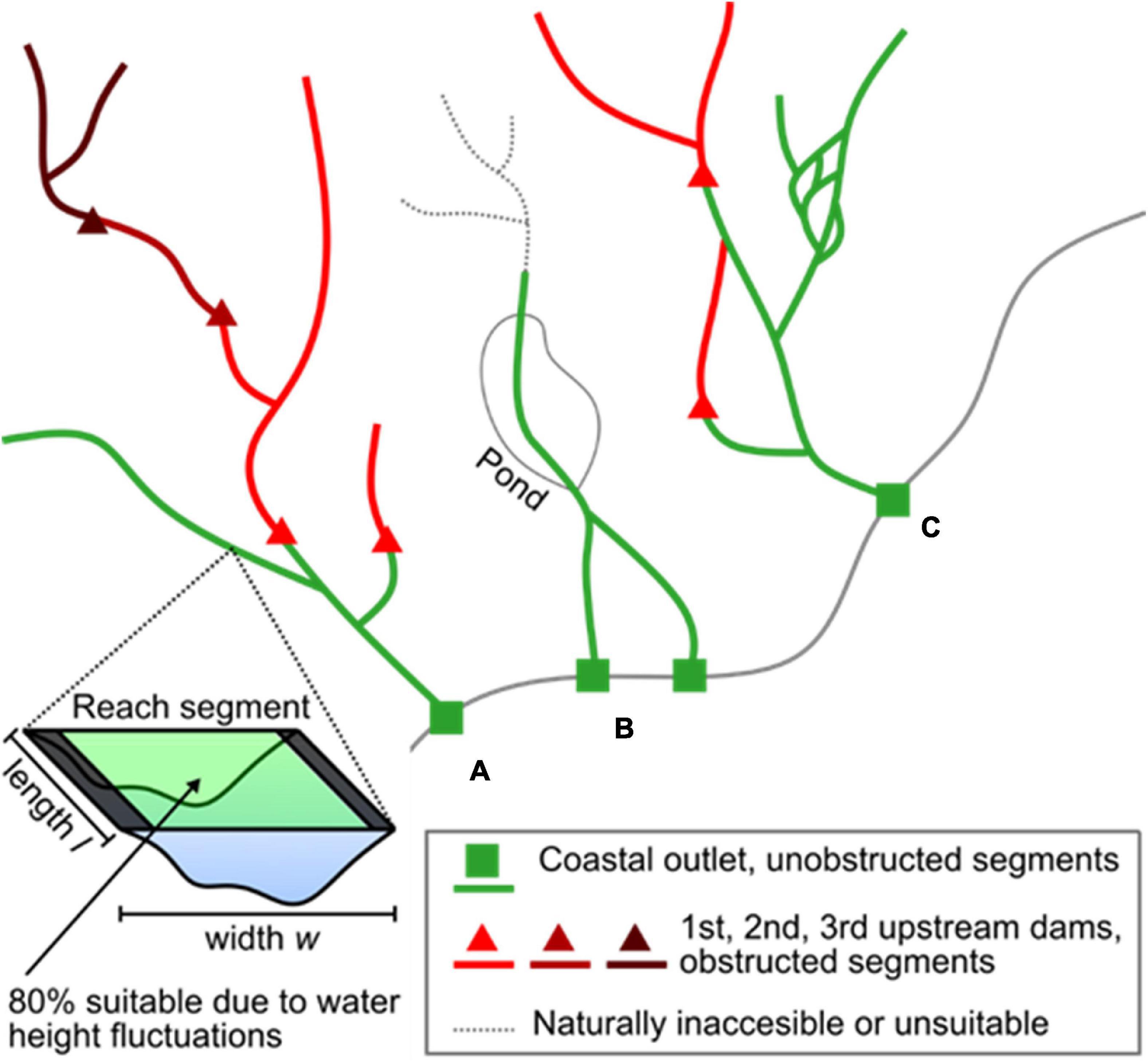
Figure 2. Example of our approach to habitat area calculation. (A) Habitat was calculated for all reach segments. We then summed habitat groups that occurred between point features (e.g., habitat between a coastal outlet point and the first upstream dams in that river, habitat between a dam and other dams immediately upstream). (B) Rivers that bifurcated along the coast were summed as a single habitat group due to their upstream connection. Reach segments deemed inaccessible or unsuitable by experts were excluded from the habitat calculation, in addition to pond and lake areas. (C) We included downstream bifurcations in our habitat group calculations. Dams located on a bifurcation obstructed habitat only to the end of the bifurcation when other dominant flow paths were unobstructed.
Empirically determined stream discharge-width relationships were used to calculate potential habitat area from the flowline data (e.g., Leopold and Maddock, 1953). This approach allowed for the estimation of horizontal surface area at any reach segment based on drainage area, geographic location, and nearby stream gage data. We first used the enhanced unit runoff method (EROM; McKay et al., 2012) to estimate mean annual discharge, then estimated mean reach segment width (w) using the power law equation:
where Q is discharge, k was a derived width coefficient, and b was a derived exponent. Values for k and b vary by region but are typically close to 10 and 0.5, respectively (Bray, 1982; Sweet and Geratz, 2003; Dudley, 2004; Mohamoud and Parmar, 2006; Bent and Waite, 2013). Horizontal surface area was then calculated as:
where l is segment reach length. We assumed that fluctuations in discharge cause 20% of this area to be periodically dry along the shorelines and therefore inadequate for migration and spawning (sensu NOAA, 2009). This approach allowed us to specifically exclude dam impoundments from any analyses. Additionally we excluded lake and pond areas in the watershed assessments as American shad avoid lacustrine habitat (Stier and Crance, 1985).
We then calculated cumulative habitat areas segmented by the presence of dams by combining our habitat flowline data with a congruent dam geodatabase compiled from multiple sources (Martin and Apse, 2011; Martin, 2013, 2019; Natural Resources Canada, 2019). We summed habitat area for all reach segments upstream of each dam point, in addition to each coastal outlet point of streams and rivers (Figure 1). These point data were also vetted by local experts. Sums were taken iteratively by starting at the reach segment where the point was located, then adding upstream neighbor reaches until upstream dam points or headwater points were found. This search was recursive to avoid summing overestimations due to downstream flow bifurcations (Figure 2). Finally, we tagged individual streams and rivers with common names used by the Atlantic States Marine Fisheries Commission (Atlantic States Marine Fisheries Commission (ASMFC), 2007) for United States rivers and best available names for Canadian Rivers (Natural Resources Canada, 2019). In the end, this analysis produced a range wide assessment of putative American shad habitat as influenced by each impoundment found within the identified area. Data are compiled for the three eco-regions based on regional spawning strategies (Northern Iteroparous [NI], Southern Iteroparous [SI], and Semelparous [SM]) as delineated by Hasselman et al. (2013) and adopted by Atlantic States Marine Fisheries Commission (ASMFC) (2020).
Population Model
In order to assess the theoretical impact of dams on American shad range wide, we simulated the population potential for each of 164 identified systems. This approach allowed us to compare three broad scale scenarios (i) historical or intact “Undammed” rivers, (ii) “Dammed”, with current dams in place with no fish passage, and (iii) dams with favorable upstream and downstream passage to reflect the “Current” condition (Figure 3). All modeling routines were implemented in the ‘anadrofish’ package (Stich et al., 2020) for R (R Core Team, 2019) to provide a user interface for reproducibility and further exploration.
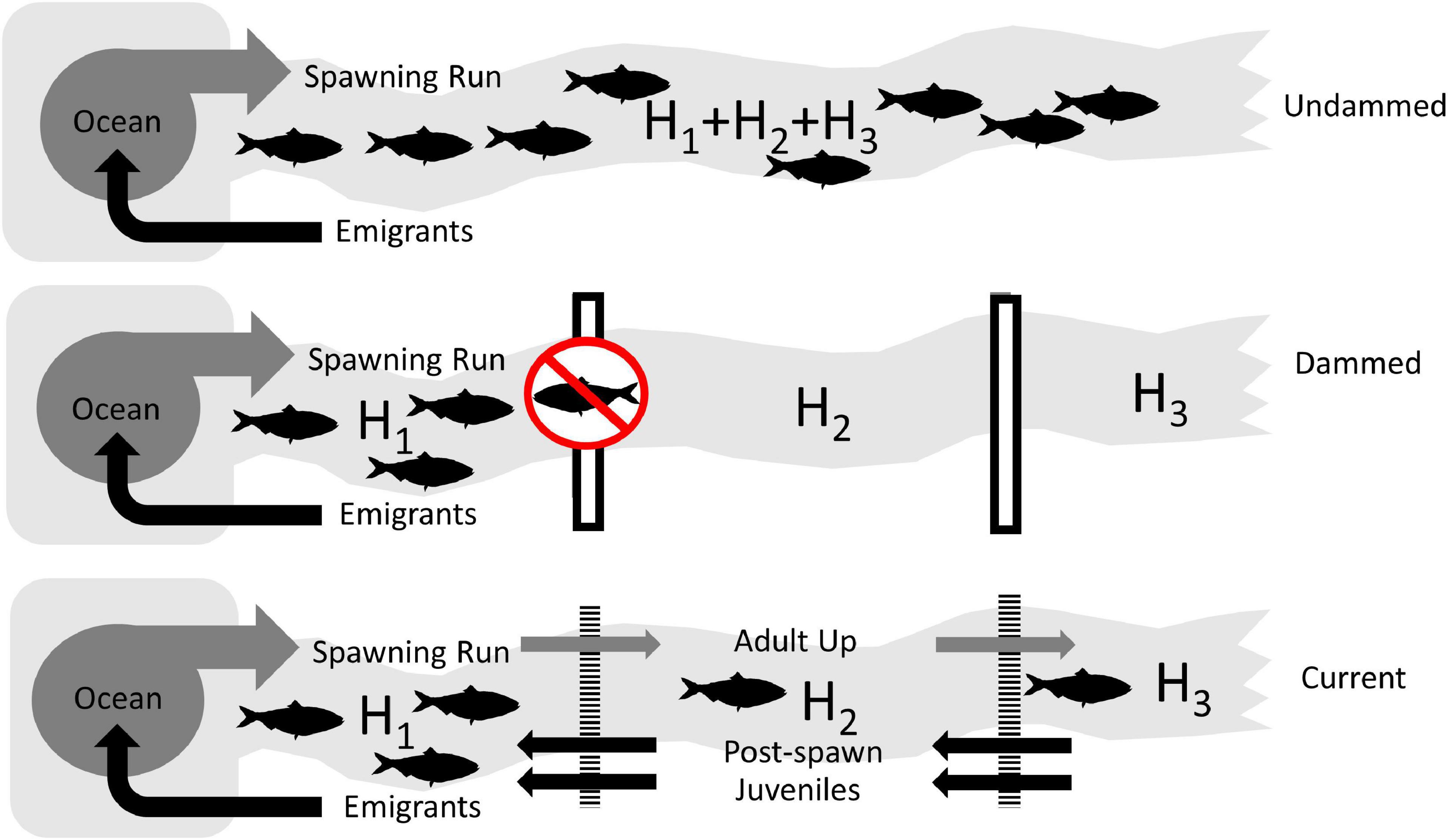
Figure 3. Conceptual diagrams of the three scenarios used to assess the impact of lost habitat and reduced connectivity on the coast-wide production potential of American shad due to dams. The Undammed scenario represents habitat accessibility in the historically undammed rivers. The Dammed scenario depicts the access to habitat that is not impacted by extant impoundments (assuming worst case, non-existing passage). The Current scenario depicts the status quo, where the current array of dams is in place, but upstream and downstream passage is applied at levels that represent the “best case” for American shad.
The river specific population modeling effort was based on Stich et al. (2019), who applied a stochastic life-history based simulation model to assess the theoretical effects of dam passage and migratory delay on abundance, population demographics, and spatial distribution of spawning adults over time. However, we followed Harris and Hightower (2012) and Bailey and Zydlewski (2013) in adopting an age-structured (rather than individual) approach to migration dynamics to generalize the modeling framework across the known range for American shad. This general model structure facilitated the incorporation of geographically appropriate life history parameters. Because these fish are iteroparous in the northern extent of their range and semelparous in the southern extent, downstream migration of both juveniles and adults was considered important for coast-wide population dynamics. Each river was identified as being in the NI, SI or SM region and assigned life history parameters estimated for each eco-region (Atlantic States Marine Fisheries Commission (ASMFC), 2020). As this population model has been described previously, the description and equations are included as Supplementary Material 1 and available as open source code1.
Dam passage probabilities were assigned based on each of the passage scenarios. For the Undammed scenario, the dams exerted no influence on passage (upstream and downstream passage probabilities were 1.00), whereas for the Dammed scenario, upstream passage probability was set to zero. For the Current scenario, upstream passage was assigned based on available estimates (Table 1), assuming a passage probability of 0.40 (the unweighted mean of reported values). Separate downstream survival probabilities were used for adults (SDA = 0.80) and juveniles (SDJ = 0.95) and were based on available estimates or recent modeling efforts (e.g., USFWS, 2019). These values reflect reportedly “excellent” upstream passage rates for American shad (Haro and Castro-Santos, 2012) and are intended to represent the most optimistic assessment of American shad passage at all dams (even when there are fish ways). Downstream values are averages of reported values from sources in Table 1.
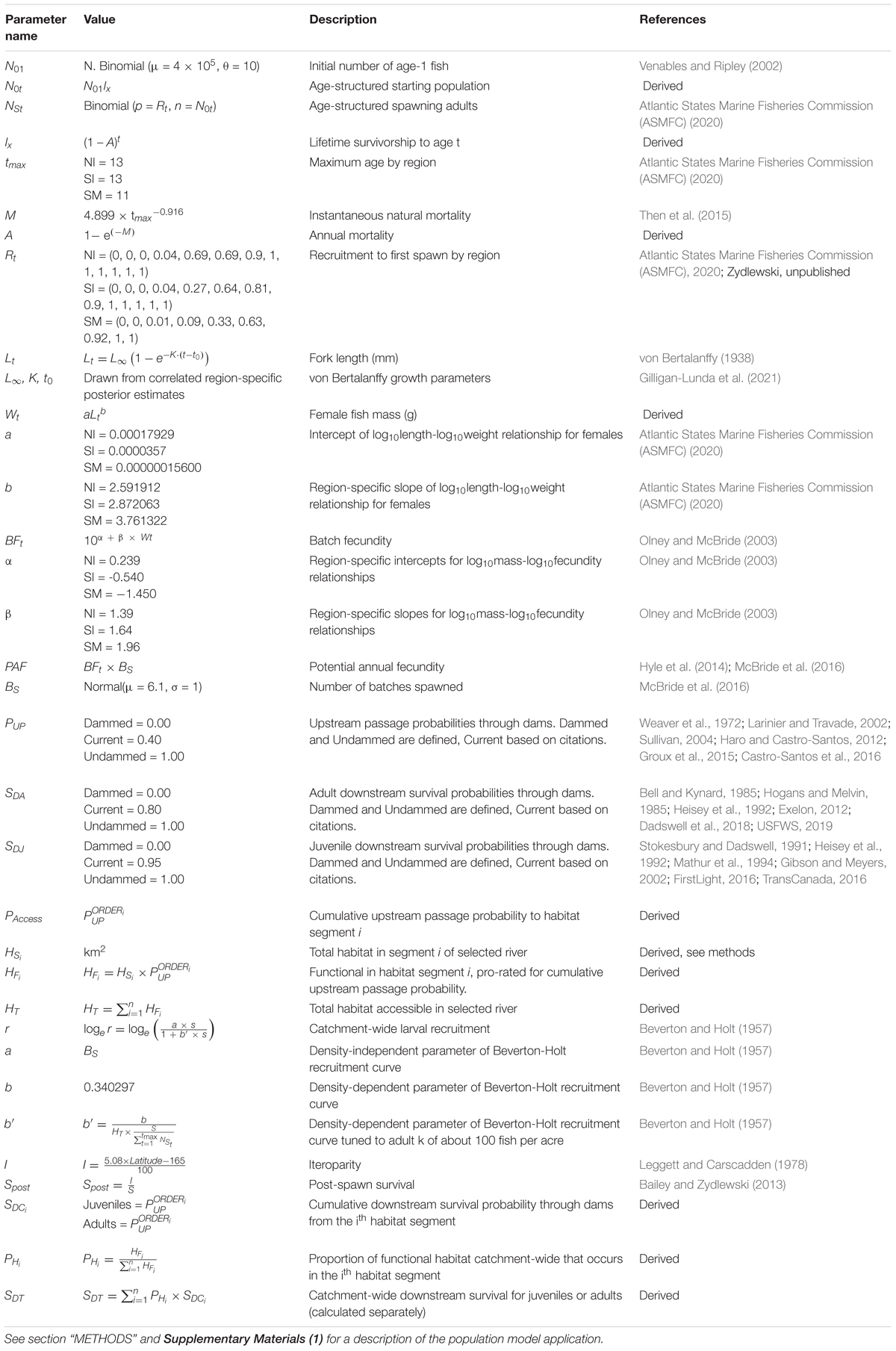
Table 1. Parameter values for shad population model used to estimate the potential spawning run size for each river in the natural range of American shad.
For each scenario, upstream and downstream passage probabilities were fixed across all dams. In all cases, upstream passage probabilities restricted the number of adults reaching spawning areas while downstream passage rates were applied as cumulative, catchment-wide mortality risks for juveniles and adults based on their locations above dams. The probability of fish reaching each habitat segment (PAccess) was calculated as the cumulative product of PUP through the number of dams (ORDER) downstream of that segment (). The amount of available habitat (km2) in each ith habitat segment (HS) was pro-rated based on this product, and the resulting “functional” habitat (HF) was summed throughout the catchment to yield over n habitat units an estimate of total habitat (HT):
The available habitat for returning adults and the simulated population of spawning fish were used to develop a river- and scenario-specific life history-based population model. Survival of adult fish to spawn was randomly drawn for each simulation. We used a Beta distribution (a = 90 and b = 10) to achieve a distribution of pre-spawn survival (mean of 0.90, standard deviation of 0.025, constrained on the interval [0, 1]). Based on the scenario-specific habitat (area) available in each river, we applied carrying capacity (k) to catchment-wide larval production (r) from a vector of age-structured spawners (s) using a Beverton and Holt (1957) recruitment curve with density dependence and a multiplicative error structure:
The density dependent parameter (b′) was tuned to impose a k of 24,711 adult fish per square kilometer (100 adult fish/acre), an often used production potential for stock assessment (Atlantic States Marine Fisheries Commission (ASMFC), 2020) based on Stevenson (1899). This resulted in b = 0.340297 when mean fecundity was used for the value of a (density-independent parameter, specified as Ft):
Because HT was summed across all units, this approach includes the implicit assumption that spawning fish distribute in the river according to proportional availability of habitat at the reach scale. Likewise, this assumption is also implicit in density-dependent recruitment due to the inclusion of HT in the denominator of the function describing b′ (density dependence in larval recruitment at the catchment level).
We assigned the larval-to-outmigrant survival (SJ) as a random draw from a normal distribution with a mean of 0.007535 and a standard deviation of 0.04 [70-d survival based on daily mortality rates reported by Crecco et al. (1983)] to estimate juvenile migrants from each river (Njuv) for each habitat unit. This quantity was simulated on the loge-scale and back-transformed to avoid negative values. Adults incurred post-spawn mortality based on the difference between natural mortality (M) and the projected degree of iteroparity as derived from Leggett and Carscadden (1978) and applied by Bailey and Zydlewski (2013). Iteroparity (I) was simulated from river-specific latitude using the equation developed by Leggett and Carscadden (1978):
We assumed that natural annual survival (S = 1 – A) was the joint product of surviving the spawning period (Spost) and surviving the duration of the year. We assumed there was no fishing mortality in all systems. Therefore, we simulated post-spawn survival as the quotient of I and S:
For populations in which I was predicted to be negative based on the equation from Leggett and Carscadden (1978) we set I = 0, and therefore Spost was likewise zero (semelparous populations in extreme southern extent). Likewise, if the value of Spost was greater than one, we set Spost = 1 (iteroparous populations in extreme northern extent).
For an assumed 2-month residence time in freshwater (based on Leggett, 1972 [40-100 days] and Chittenden, 1976 [> 60 days]; Table 1), survival in freshwater during the spawning run (Sspawn) was calculated as the product of two components:
Where S2month is 2-month interval survival (S) applied as an instantaneous rate of mortality over 2 months and calculated by using the relationship between annual interval mortality (A) and instantaneous mortality Z12 (for a 12-month period; Miranda et al., 2007) to calculate Z for a 2-month interval (Z2) as:
So that monthly interval survival is calculated as:
For semelparous populations, Sspawn is determined by Spost (which is set to zero), while for the northern iteroparous populations, in river mortality is determined by natural annual survival (S2month) that is the same survival as for fish remaining in the ocean. Mortalities were imposed at the end of this period and therefore did not influence spawning.
Downstream mortality of juveniles or adults through dams was based on proportional distribution of available habitat as appropriate to each scenario. The total mortality incurred through downstream dam passage was estimated as a function of the imposed downstream survival probability through dams (for both juvenile [SDJ] and adults [SDA]) and the proportional distribution of available habitat with respect to dam order. First, downstream survival from each ith river segment to the ocean was calculated as the cumulative probability of downstream dam survival (SDCi) separately for juveniles (SDCJ) and adults (SDCA) based on the number of dams downstream of each segment. Next, the total proportion of accessible habitat (PHi) in each ith river segment was calculated as the quotient of prorated habitat in each segment (HS–P) and the sum of all available habitat:
We then calculated the catchment-wide survival during downstream migration for adults,
or juveniles as the weighted sum of SDCi (sum of the products of PHi and SDCi):
Age-structured out-migrants were then added back into the non-spawning (ocean) population, the population was projected one time-step based on instantaneous mortality (M in the absence of fishery impacts or Z otherwise) and the simulation continued for a total of 50 years to ensure stabilization of abundance estimates within rivers. We repeated this simulation for each river and each passage scenario by randomly sampling the river, passage scenario (Dammed, Undammed, Current) and each of the stochastic parameters during each simulation. This resulted in approximately 2,000 simulations per passage scenario per river. For each of the 164 river and scenario combinations, the output for the last year (50) was collected for the number of age specific spawners and juvenile emigrants.
Biomass and Nutrient Delivery
To estimate changes in biomass, total N and total P delivery to the freshwater environment (by adults) and to the marine environment (by juveniles), we used the average outputs from the simulations from each river. The average age specific numbers of spawners returning to each river and passage scenario combination were used to estimate the total spawner biomass seasonally entering each river:
where P is the proportion of male (M) or females (F), N is the average number of spawners of age t and Wt,F or Wt,M is the sex specific mass at age t. Sex-specific mass for an individual fish was calculated by calculating average length at age and mass as a function of length described in Supplementary Equations 4, 5, using region-specific parameters.
Juvenile biomass leaving each river was calculated as the summation of the number of juveniles produced from all habitat units in each river (NJUV) multiplied by the average, weighted survival downstream to the ocean (SDTJ, Equation 13).
The total river output (JuvenileBiomassRiver) was simply the sum of juvenile biomass reaching the ocean from each habitat unit. The estimated mass of a juvenile emigrant, WJUV, was based on reported juvenile masses ranging from 2.0 to 4.5 g (Zydlewski and McCormick, 1997a; Haskell, 2018); we applied an average WJUV of 3.36 g (Table 2).
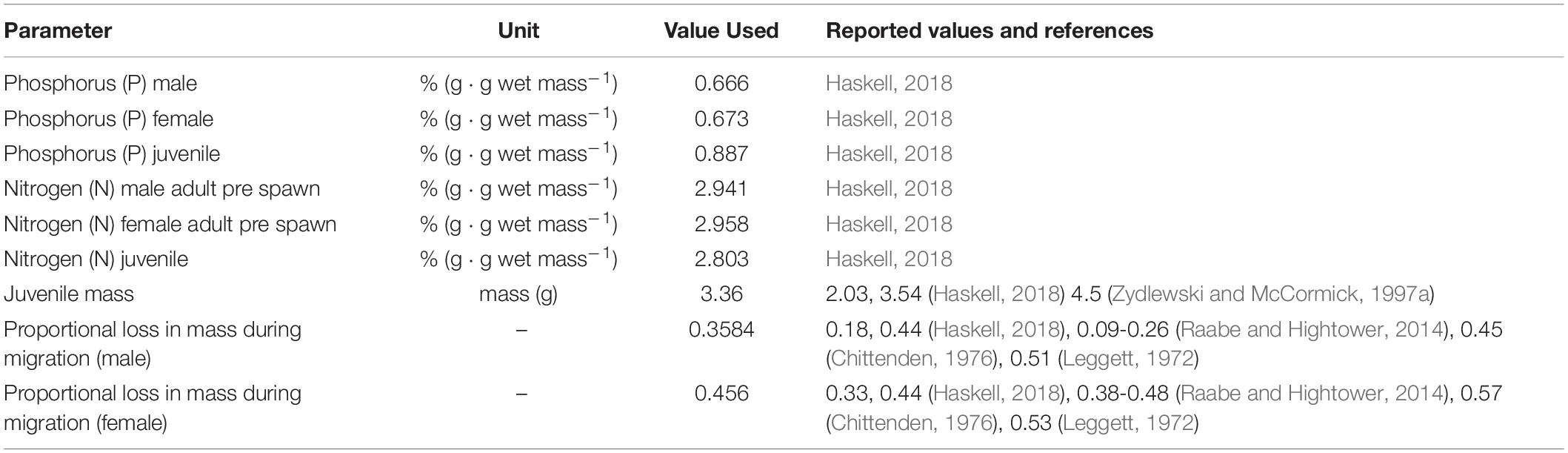
Table 2. Parameter used to estimate annual, river specific nutrient input (via adult carcasses, spawning, and metabolism) and nutrient output (juvenile emigration).
The delivery (and retention) of marine derived biomass (Biomassdelivered) and nutrients into each river during the spawning migration was estimated as a function of: (i) the initial biomass of adults arriving in freshwater (SpawnerBiomassRiver), (ii) the probability of death during spawning, and (iii) if surviving and returning to the ocean (for rivers in the NI and SI regions), the estimated biomass lost during the spawning migration through spawning and metabolic loss.
Biomasscarcass represents the overall biomass of presumptive spawners and successful spawners that die in the river (this includes the contribution from fish carcasses, gametes and metabolism of fish that ultimately die in fresh water). Biomass carcass is calculated as:
Where Sspawn is probability of survival through the spawning migration (Equation 8), N is the number of spawners at age t, P is the proportion of males (M) or females (F) in a given river, and W is the mass of an individual returning male (or female) adult at age t.
Biomassmetabolic is the sum of metabolism expenditures in freshwater and shed biomass in the form of gametes for spawners who successfully enter a river as a presumptive spawner and return to the ocean. This biomass lost in river by surviving fish (gonadal and metabolic) is calculated as:
All parameters are defined as described in Equation 17. Biomass lost during the spawning run for surviving migrants (Δ) is the proportional sex specific loss of mass due to metabolic expenditure and gametic release. These values are based on empirical data reported by Leggett (1972), Chittenden (1976), Raabe and Hightower (2014), and Haskell (2018) that range 18–51% observed mass loss for males and 33–57% for females. We applied average values of 36 % and 46% mass loss for males (ΔM) and females (ΔF) respectively (Table 2). We acknowledge this as a simplifying assumption as it is well-known that distance traveled (Leonard and McCormick, 1999), temperature experience (Glebe and Leggett, 1981b; Raabe and Hightower, 2014) and residence time (Raabe and Hightower, 2014) all influence the extent of individual mass lost in freshwater.
Marine derived nutrient transport (phosphorus or nitrogen) was calculated using estimated nutrient density (grams of nutrient per gram of fish wet mass) applied to estimated of Biomassdelivered. Several simplifying assumptions were applied. We used nutrient density values 0.670 g/g wet mass for phosphorus and 2.950 g/g wet mass for nitrogen (based on averaged male and female data from Haskell, 2018). We assumed nutrient density was the same for males and females, and across tissues (i.e., nutrient density for gonads, metabolic tissue loss and whole-body densities did not differ). Thus, pre-spawn estimates of nutrient density are assumed to be representative of the nutrients shed through gonadal and metabolic loss. This is generally the case as whole body nutrient densities differ by < 1% from male to female (Haskell, 2018). For the gonads, however, we note that the testes are enriched in both N and P (∼10 % over other tissues) while the ovaries are enriched in N (∼10%) but depleted in P (∼50%), resulting in an over estimate for P. However, because female gonadal mass represents approximately 10 % of the mass of a pre-spawn female (Leonard and McCormick, 1999), and the applied mass loss based on observations is more than four-fold greater (46% mass loss during spawning), the influence on phosphorus estimates is minimal.
Results
Habitat
Historic American shad habitat was estimated to be 2,287 km2 for the entire range and comprised 164 rivers that were identified to have suitable habitat based on the identified criteria. This assessment was based on a combination of a priori assumptions and informed by local knowledge through most of the United States and part of Canada. For some of the assessment in Canada, estimates are based solely on the a priori model and our inability to locate appropriate experts. The habitat was divided fairly evenly among eco-regions with 30, 38, and 32% identified in the NI, SI and SM eco-regions, respectively (Figure 4). In the NI eco-region, 18% of the habitat was found in Canada while 12% was found in the United States (see also Table in Supplementary Material 2).
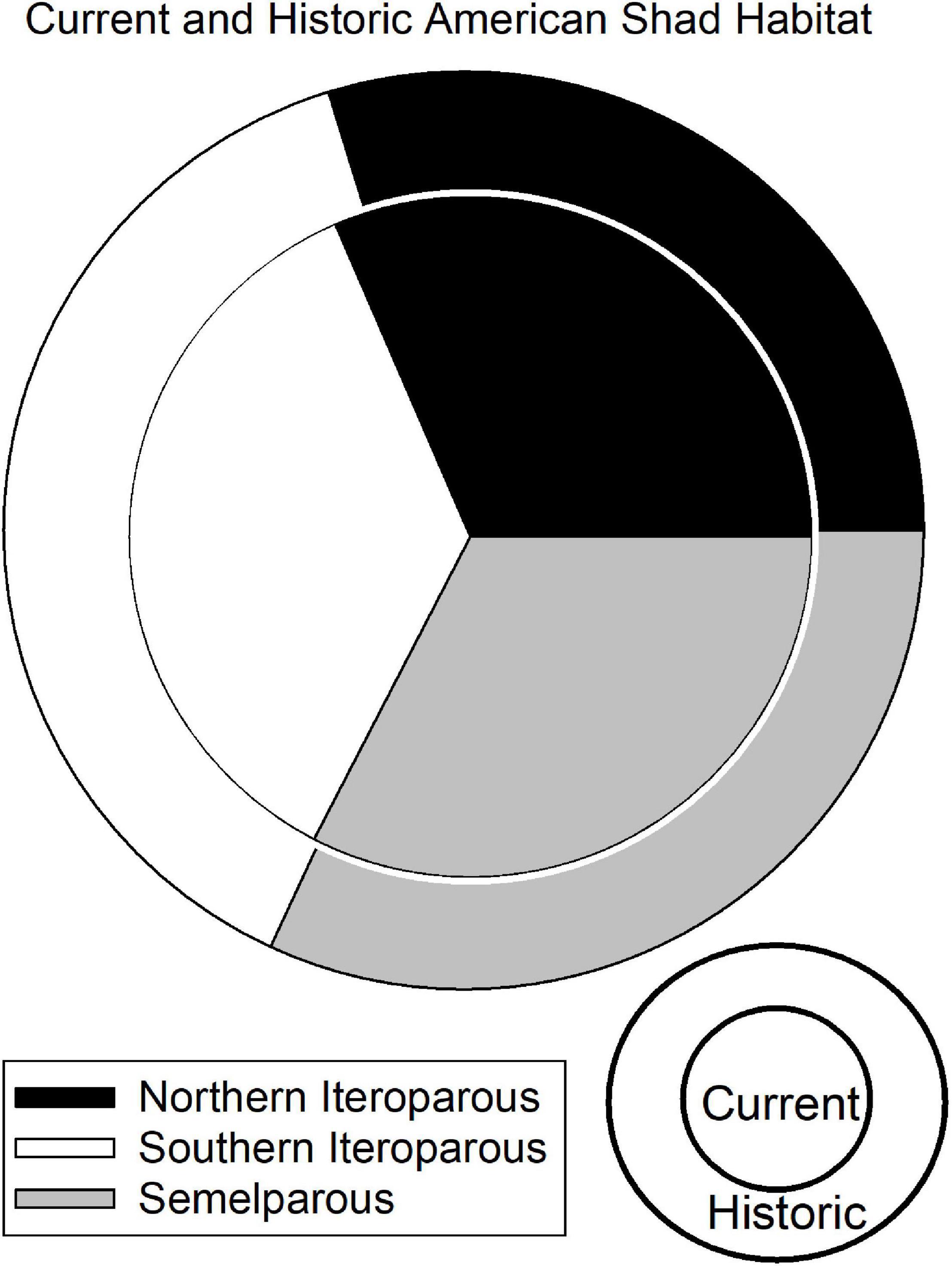
Figure 4. Graphic representation of the Undammed (outer) and Dammed (inner) scenario freshwater spawning habitat available to American shad throughout their native range. The size of the circles is proportional to the area of historic unimpounded habitat (2,752 km2) and current habitat access (1,639 km2), indicating that American shad are impeded from reaching nearly 41% of their historic habitat.
The presence of dams on 68 rivers throughout the range of American shad fully or partially blocks access to an estimated 41% of their coast-wide habitat (Figure 4). The constraint of access to habitat found upstream of at least one barrier is comparably high for all eco-regions, with 37, 44, and 39% loss of habitat due to first main-stem dams for NI, SI and SM, respectively. It is notable that habitat in the Canadian jurisdiction of the NI eco-region has remained comparably intact with an estimated 19% loss. In contrast, the United States NI region is heavily impounded, with a loss of connectivity to 65% of the habitat.
Throughout their range, ten rivers account for more than half (52%) of riverine habitat of American shad (Susquehanna [11.0%], St. Lawrence [8.3%], Altamaha [6.5%], Delaware [4.7%], Savannah [4.3%], James [4.3%], Roanoke [3.4%], Cape Fear [3.0%], Connecticut [3.0%], Congaree [2.9%]; Figure 5; Table in Supplementary Material 2). Dams on these rivers have resulted in the reduction of habitat access from a minimum of 14% loss in the Delaware River to complete habitat blockage in the Congaree River. Dam blockage at these ten rivers accounts for the loss of 575 km2 of habitat, nearly 62% of the coast-wide loss in habitat for this species. On the Susquehanna River alone, dams block 243 km2 of habitat, 10.6% of total historically accessible habitat coast-wide.
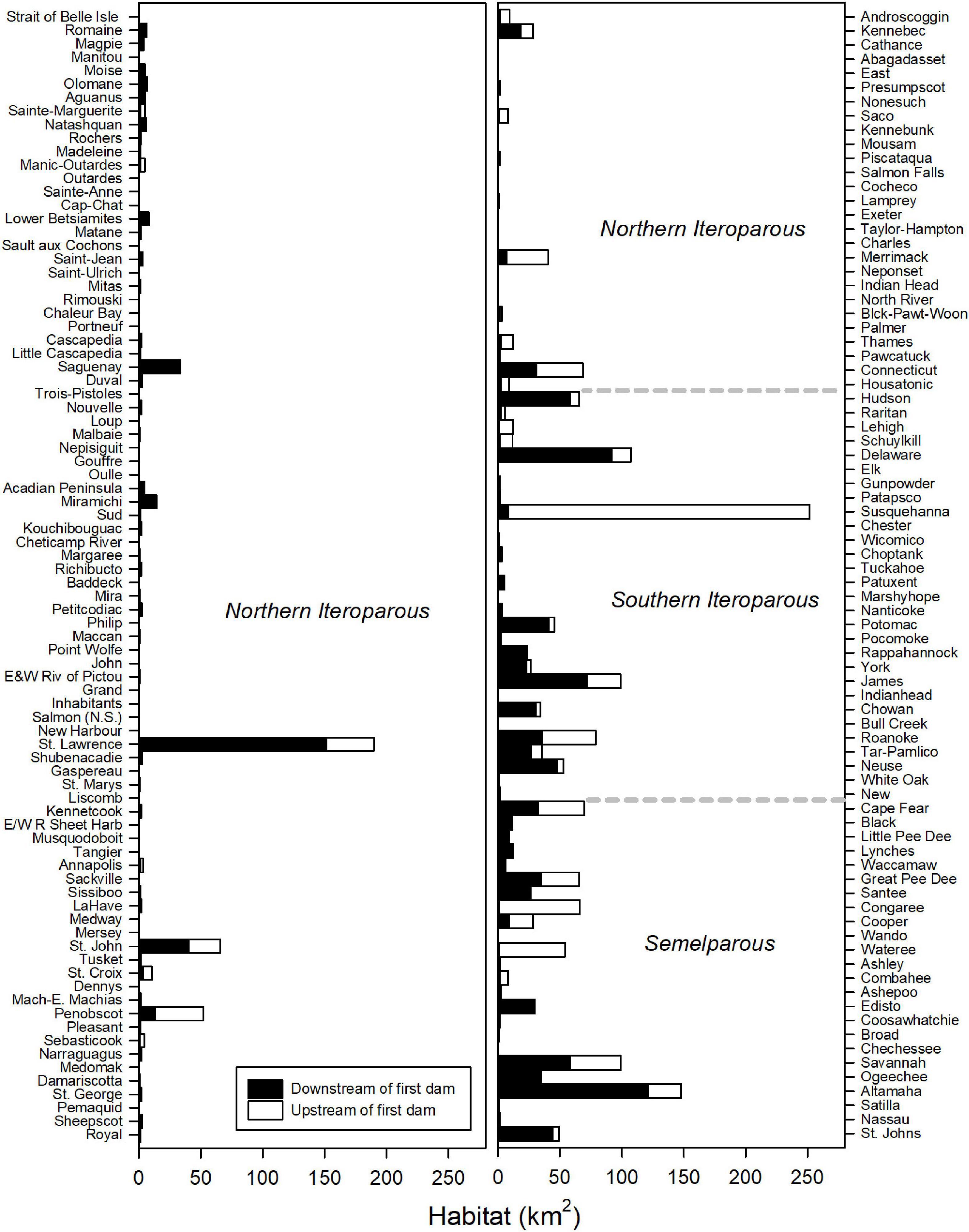
Figure 5. Total habitat available to American shad upstream and downstream of the first dam by river throughout their native range. Dotted lines indicate transitions between eco-regions. Rivers (y-axis) are ordered in descending latitude.
Population
Historic American shad population potential was estimated to be 69.1 million adults for the entire range. While historic habitat area is roughly equal for each of the eco-regions, the modeled spawner density is heavily skewed to the NI and SI eco-regions. We calculated an estimated historic potential of 37.5 million (54%) and 27.2 million (39%) spawners for the NI and SI regions, respectively (Figure 6). Historical abundance in the Canadian NI ecoregion was estimated at 24.0 million fish (35% of the coastal run) while the United States portion of the NI eco-region was 13.6 million (20% of the coastal run; See also Supplementary Material 2). The entire SM was estimated at only 4.3 million spawning fish for the entire region, accounting for only 6% of the spawner potential. When considering the potential for all 164 rivers to support spawning runs of adult American shad, estimates of spawner potential are directly linked to estimates of available habitat (Figure 7). However, latitudinal variation in growth, maturation, and post-spawn survival (Leggett and Carscadden, 1978; Gilligan-Lunda et al., 2021) all influence the relative differences observed in population potential. On average, a square km of habitat results in 55,200 spawners in the NI eco-region but only 31,000 in the SI eco-region. Because of the lack of additive age classes in the SM eco-region (i.e., no repeat spawners), a square kilometer of area is modeled to support only 5,900 spawners.
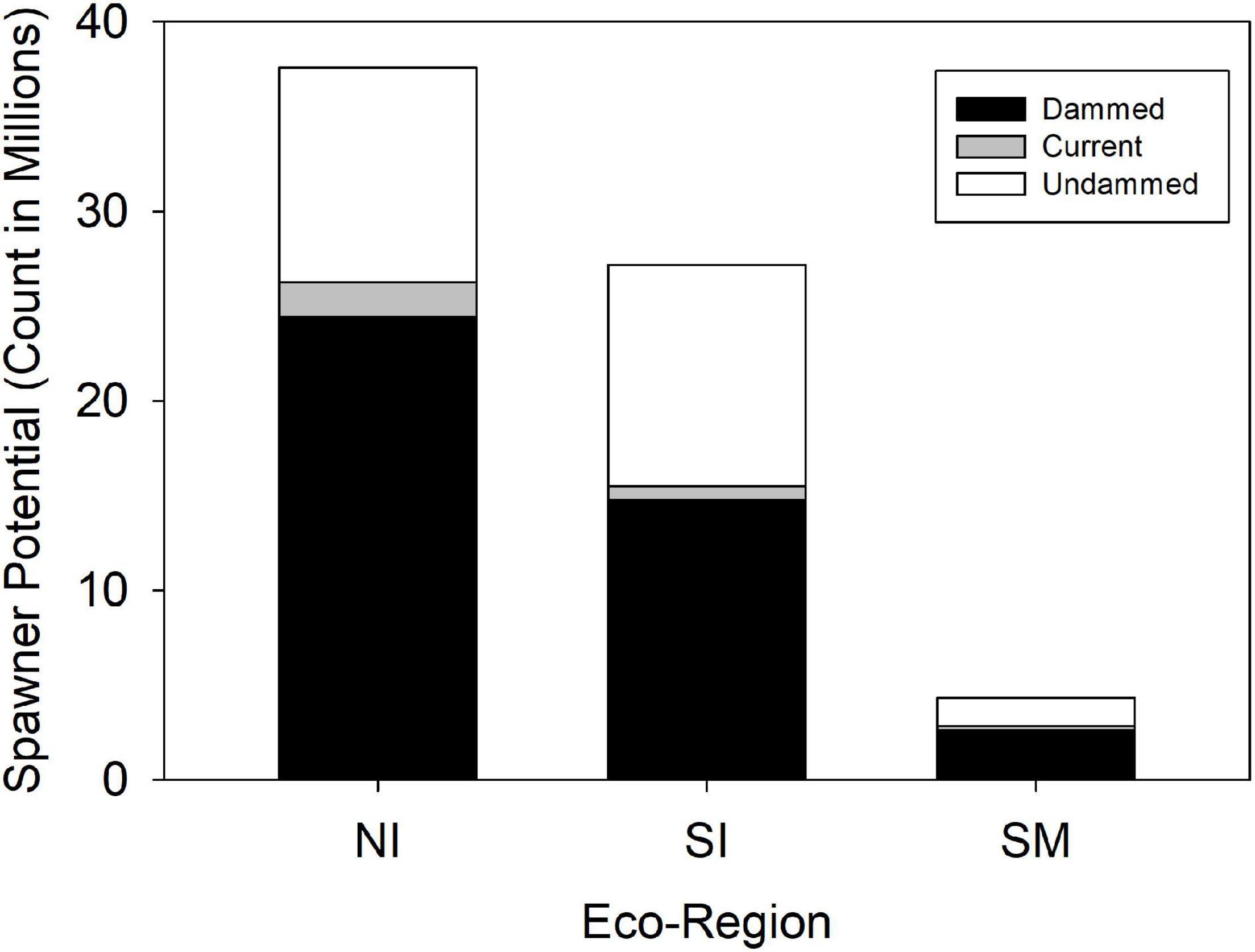
Figure 6. Modeled spawner abundance potential of American shad under the no passage (“Dammed”), status quo (“Current”), and no dam (“Undammed”) passage scenarios by eco-region through their native range from Canada to Florida.
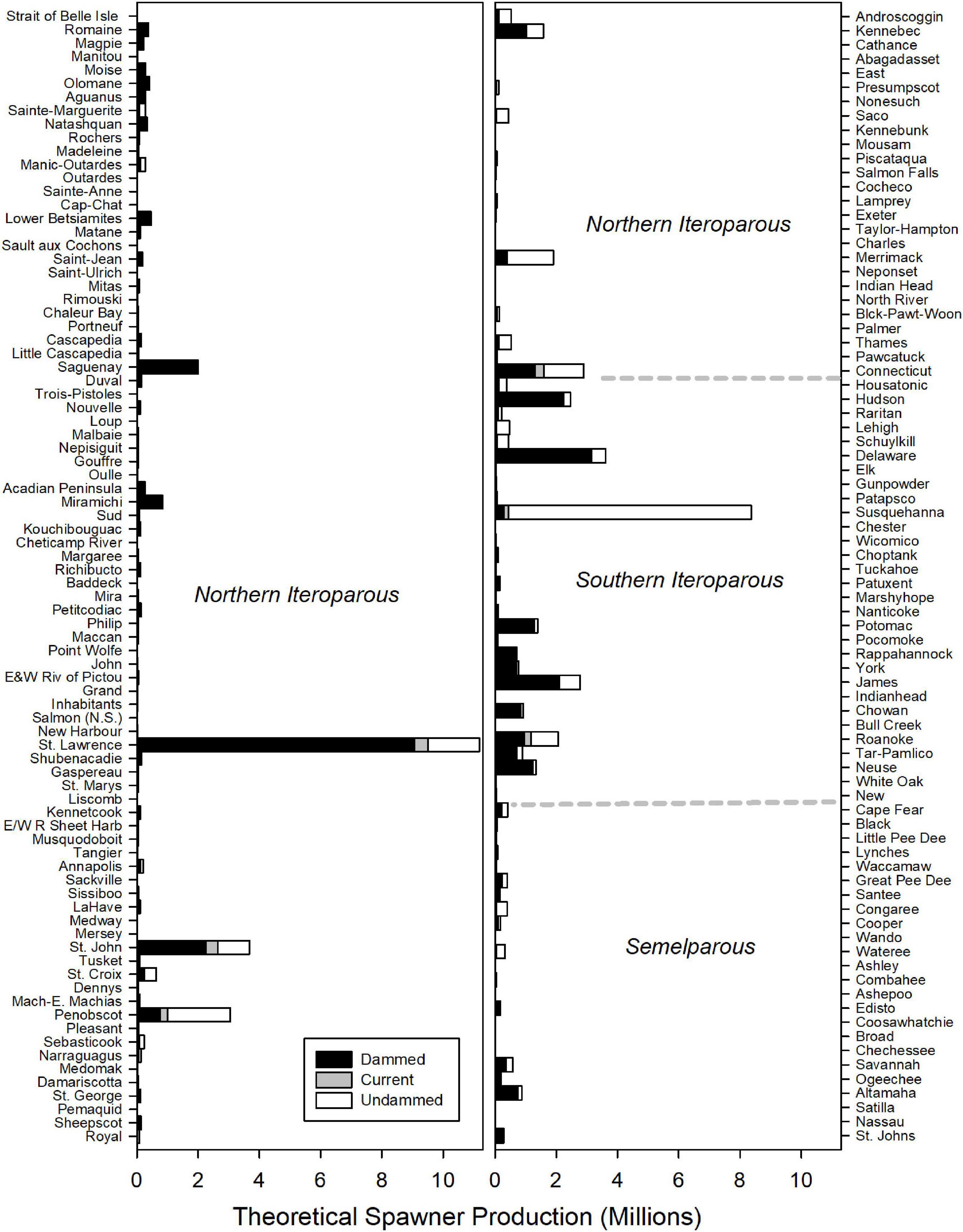
Figure 7. Estimated spawning potential of American shad for each river through their native range from Canada to Florida. Dotted lines indicate transitions between Eco-regions regions. Rivers (y-axes) are ordered in descending latitude. The stacked bars depict estimated potential for the No Passage, Current and Undammed scenarios.
Coast-wide, there is an estimated 39% loss in population potential, directly attributable to lost habitat between the Undammed and Dammed scenarios (Figure 8). Regional population potential is diminished by 35, 46, and 40% reductions in abundance for NI, SI and SM regions, respectively. Population potential loss in the Canadian jurisdiction of the NI eco-region is 18% while the United States NI region is reduced by 65%.
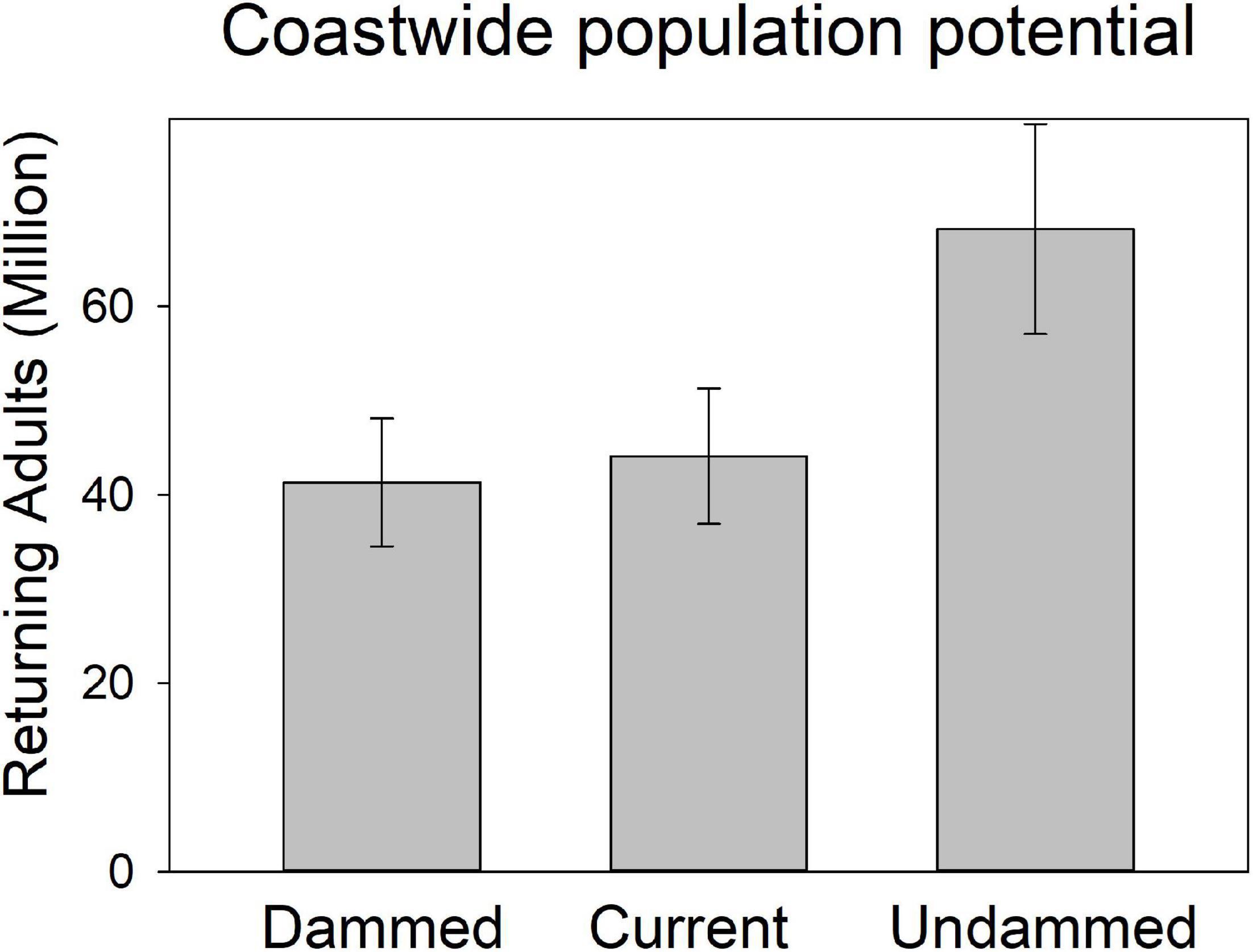
Figure 8. Coast-wide spawning potential of American shad under the no passage (“Dammed”), status quo (“Current”), and no dam (“Undammed”) passage scenarios. Boxes indicate 25th to 75th interquartile range and whiskers indicate 95% CI.
Under the Current scenario, where we applied upstream passage probabilities that represent some of the most favorable conditions reported in the literature, there was limited increase in spawning potential of 9% coast-wide from the Dammed scenario (from 41.8 to 44.6 million). Thus, the most optimistic application of dam passage parameters to each of the hundreds of dams that block access to American spawning habitat provides a theoretical benefit of restoring only 4% of the historic spawning potential that is diminished by 39% due to dams. This limited alleviation of population potential influence is lowest in the SI eco-region (2.7% increase), and is comparable in the NI (4.9%) and SM (5.4%). Passage mediated alleviation of population potential in the Canadian jurisdiction of the NI eco-region is 4.1% while the United States NI region is 6.2%, suggesting a greater potential for recovery.
Biomass and Nutrients
Historic American shad populations supported an estimated average of 69.1 million spawners coast-wide, delivering 98,000 MT of biomass to undammed freshwater coastal systems on an annual basis. We estimate that 59,100 MT (more than 60%) of the delivered biomass remained in the river systems in the form of carcasses, tissues shed during spawning, and metabolic loss. Historic biomass delivered to the NI was 51,500 MT (with 25,800 MT retained) and 41,200 MT delivered to the SI eco-region (with 35,500 MT retained; Figure 9). For the SM eco-region, a much smaller 5,200 MT biomass was delivered, but all would be retained as part of the semelparous life history in the region. Differences among eco-regions are driven by competing trends of lower potential spawner numbers in the southern latitudes, greater spawner number due to iteroparity in the north and reduced individual spawner sizes (due to a truncated age distribution as repeat spawning declines in the SI and the SM eco-regions).
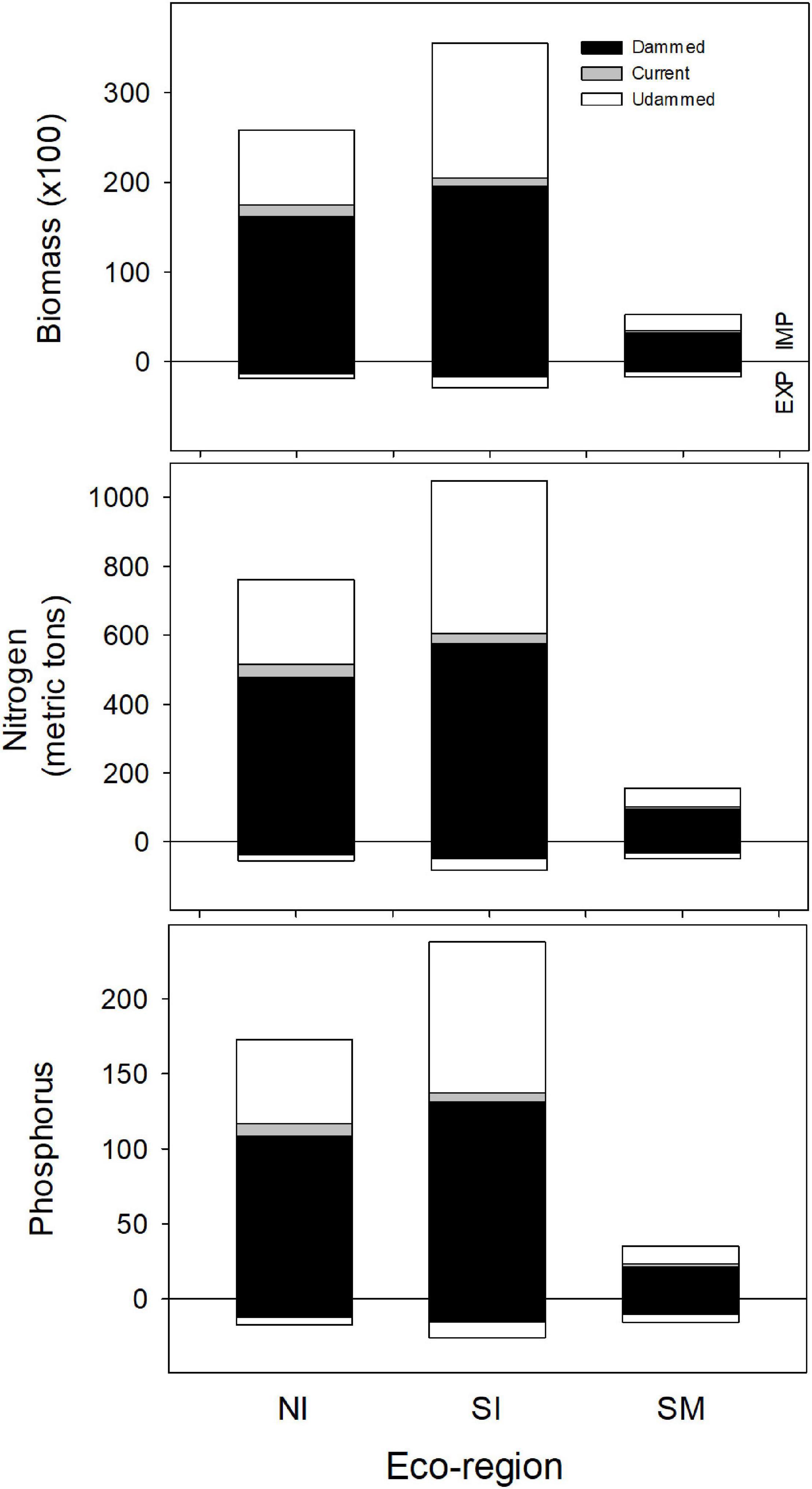
Figure 9. Estimates of annual import (IMP: positive from the zero line) and export (EXP: negative from the zero line) of biomass, nitrogen and phosphorus delivered to each eco-region by American shad through their native range from Canada to Florida under the no passage (“Dammed”), status quo (“Current”), and no dam (“Undammed”) passage scenarios.
Under an Undammed scenario, juvenile migration from fresh water transports an estimated 6,600 MT of biomass to the ocean coast-wide, with comparable transport from each of the eco-regions (1,900, 2,900 and 1,800 MT respectively for NI, SI and SM eco-regions). Estimated biomass export to the ocean is considerably lower than the biomass import into freshwater. We estimate 13-fold greater import in the NI eco-region, 12-fold in the SI, but only 3-fold greater in the SM (Figure 9).
For the Dammed and the Current scenarios, the relative relations between import and export remain similar among eco-regions and coast-wide, however the magnitudes of both import and export reflect the reduced population under habitat access restriction. Coast-wide, retained biomass is reduced from 59,100 MT for the No Dam scenario to 38,900 MT for the No Passage scenario. Under the Current Scenario, biomass delivered is slightly increased to 41,400 MT. These represent a 34% and 30% reduction in biomass delivered for the No Passage and Current scenarios respectively, compared to the No Dam scenario. Similar reductions in retained biomass import for the Dammed (37, 45, and 40%) and Current scenarios (32, 42, and 34%) were observed in each of the three eco-regions (NI, SI, and SM). Juvenile nutrient export was reduced from 6,600 to 3,500 MT for the Dammed scenario, and modestly recovered to 3,900 MT under the Current scenario (37 and 42% reductions, respectively).
Because the inputs we used for nitrogen and phosphorus densities are not markedly different between males, females and juveniles (Table 2) patterns of nutrient delivery match the trends observed for biomass by eco-region. Under an Undammed scenario, coast-wide retained nitrogen is estimated at 1,963 MT (761, 1,048 and 154 MT for NI, SI and SM) and retained phosphorus is estimated as 446 MT (173, 238 and 35 MT for NI, SI and SM). Under an Undammed scenario, juvenile migration from fresh water exports an estimated 186 MT of nitrogen (54, 82, and 49 MT for NI, SI and SM) and 59 MT of phosphorus (17, 26 and 16 MT for NI, SI and SM). The patterns of reduced nutrient delivery under No Passage and Current Conditions match the trends observed for biomass by eco-region.
Coast-wide, retained N delivery is reduced from 1,963 MT to 1,147 for the Dammed scenario (478, 576, and 93 MT for NI, SI and SM), and modestly recovered to 1,221 MT under the Current scenarios (515, 605, and 102 MT for NI, SI and SM; Figure 9). Parallel reduction in retained P delivery is reduced from 446 to 260 MT for the Dammed scenario (108, 131, and 21 MT for NI, SI and SM), and boosted to 277 MT under the Current scenarios (117, 137, and 23 MT for NI, SI and SM; Figure 9). Juvenile nitrogen export was reduced from 186 to 110 for the Dammed scenario (34, 46, and 30 for NI, SI and SM), and grew to 119 MT under the Current scenario (38, 48, and 33 for NI, SI and SM). Juvenile phosphorus export was reduced from 59 to 35 MT for the Dammed scenario (11, 14, and 9 for NI, SI and SM), and grew to 37 MT under the Current scenario (12, 15, 10 for NI, SI and SM).
Discussion
Based on a priori modeling and local knowledge, the historic habitat availability for American shad spanning the east coast of North America approached 2,300 km2. While our estimation was derived from 164 coastal rivers, it is likely that American shad may spawn in additional smaller systems, though these would contribute minimally to the overall coastal population. The estimate of 41% loss of habitat connectivity through damming of coastal systems corroborates assertions that loss of spawning habitat access is likely the major cause of population declines in this species (Limburg et al., 2003; Atlantic States Marine Fisheries Commission (ASMFC), 2020). The proportional distribution of accessible habitat is relatively constant among the three eco-regions (when governmental borders are not considered). This indicates that, while impacts are regionally variable, dam construction has negatively influenced habitat access by American shad, similarly, throughout their range.
Because the recruitment function we used incorporates habitat area directly (see Stich et al., 2019), it is unsurprising that theoretical capacity to support runs of adult American shad into rivers mirrors this assumption. Despite the fact that total available habitat was divided fairly evenly among eco-regions, the relative scope for population size is substantially greater in the NI, decreased in the SI and lowest in the SM eco-region due to differences in life history. While this is intuitive based on the degree of iteroparity, growth rates, and maximum sizes (Gilligan-Lunda et al., 2021), it highlights the difference between relative and absolute losses of spawner potential due to dams. The SM eco-region’s lost scope of 1.74 million fish is dwarfed by the loss of more than 13 million in the NI eco-region, suggesting that parallel differences in fisheries potential also exist. While the historic spawner production potential is higher in the north and lower to the south, it is notable that each of the regions generally lost comparably high proportions of production habitat. Thus, the fish in southern rivers may be viewed as having the highest conservation vulnerability, particularly at the southern end of the range where climate change may impose additional ecological constraints. Based on this modeling exercise, coast-wide production potential is more than 69.1 million spawners per year without dams compared with the Dammed scenario of just under 41.8 million spawners, a reduction of 39 %.
The intent is of this modeling exercise has been to provide a realistic quantification of the lost potential for American shad that has altered coastal ecosystems and reduced commercial and recreational fisheries opportunities over the species’ native range. We paint a clear picture of the relative loss of potential coast-wide, but a cautionary note is warranted. Population estimation is a challenge even when the input parameters are well characterized, and that is not the case here. We are aware that American shad select areas for spawning based on temperature, water velocity, depth and substrate (Hightower et al., 2012), evidence that some habitats are more suitable than others. Additionally, juvenile alosine recruitment from reaches of a watershed likely differs based on migratory distance and conditions (Tommasi et al., 2015), yet we have made the implicit assumption of equal habitat quality. Models such as the ones presented here are built with considerable uncertainty, and assumptions in the model parameters. This has been well highlighted here, and elsewhere (Castro-Santos and Letcher, 2010; Bailey and Zydlewski, 2013; Stich et al., 2019). For American shad, there remain many fundamental relations that are poorly characterized (e.g., passage, reproduction, survival and juvenile recruitment), a condition common in such model efforts (Goethel et al., 2011).
It is self-evident that the parameterization of the system we imposed defined the boundaries of our outcomes, and we anticipate that continued research efforts will aid in refining our estimates. However, our estimates are likely to be conservatively low based on model construction, parameter values applied, and assumptions made. Specifically, the Beverton-Holt recruitment curve we have employed is derived from Stevenson (1899), an approach that may grossly under estimate capacity. These cautions withstanding, the values of spawners we generated are realistic in magnitude and represent the best available information. Our estimate of 98,000 MT of spawners returning to all rivers is congruent with historic commercial fisheries landings that exceeded 20,000 MT annually (Walburg and Nichols, 1967; Limburg et al., 2003). Undammed potentials for specific rivers (Supplementary Material 2) are generally consistent with historic annual landings harvest from Walburg and Nichols (1967), the data suggest a high fisheries exploitation rate of nearly 20% of the average entire run in the Neuse, James, and Delaware Rivers, 50% in the Potomac and 70% in the York River. A reported harvest of over 2 million adults in the Penobscot River in the 1860s (Foster and Atkins, 1867) would represent a harvest of 65% (of our average estimated run of 3.1 million fish). Harvest data from other rivers indicate that our model may significantly undervalue spawner potential. Reported annual harvest in the Nanticote and Choptank Rivers (Walburg and Nichols, 1967) were two- and three-fold greater than our average undammed population projections. Such difference are undoubtedly due to both population stochasticity and model underestimation. Thus, the most robust value of this exercise is the relative influence of dams which provides an unambiguous relative index of the impact of impaired habitat access.
The inability for current realistic fish passage measures to restore any more than 9% of the estimated spawning potential coast-wide is troubling news for the restoration of these fish. Our results indicate that the application of current upstream and downstream passage rates at all dams affords a remarkably small increase in the theoretical production potential relative to rivers that are wholly inaccessible upstream of the first dam. We estimate that fishway passage coast-wide at dams represents a fixed constraint of about 35% on the spawning run potential of American shad. It is possible that as advances in fish passage engineering, other protective measures, and understanding of fish behavior continue to evolve, passage efficacy may improve beyond our optimistic estimates. The use of fish passage performance criteria (e.g., Stich et al., 2019; CRASC, 2020) may also facilitate fish passage improvements by providing biologically relevant targets. These potential advances withstanding, the low theoretical return on investment of fishways is heavily influenced by the presence of multiple dams on rivers, resulting in a compounding influence on passage and survival.
It is notable that this significant imposition on theoretical spawning potential results from a model that estimates contemporary passage values considered to be “excellent” compared to those typically achieved (Haro and Castro-Santos, 2012). True impacts of dams in systems with poor fish passage may be – and are likely to be – greater. Even when significant engineering efforts are made to construct fishways to allow upstream migration, effective connection to upstream habitat can be illusory (Noonan et al., 2012; Bunt et al., 2012, 2016). It is notoriously challenging to provide safe, effective and timely upstream passage past dams for American shad, and the poor passage performance of this species has long been noted (Stevenson, 1899). Challenges associated with dams (beyond passage and survival) have not been incorporated in our model. Flow disruptions, temperature, and changed community composition could further increase the negative impacts of dams on American shad populations. Loss through coastal commercial and recreational fisheries continues in states where Fisheries Management Plans (or alternatives) are in place (Atlantic States Marine Fisheries Commission (ASMFC), 2020). Lastly, other factors (e.g., habitat degradation) may impose further loss of production potential on river- and region-specific levels.
Fishway design continues to be a critical interface of biology and engineering with significant advances in concepts and application (Silva et al., 2018). However, though fishways have been constructed at some of the numerous dams that many American shad encounter in their migration, many, if not most, are largely or wholly ineffective (Haro and Castro-Santos, 2012). Other dams simply have no specific passage accommodations (e.g., Roanoke River; Hightower and Sparks, 2003), yet for this exercise we attributed optimistic values of fishway performance. Because the time course for hydropower relicensing through the FERC process is 40 years or more (Vogel and Jansujwicz, 2021) the current state of fish passage has a long sustained effect. Managers may only experience one opportunity to shape passage at a dam once during their professional careers. For these reasons, poor utilization of fishways is likely to remain a substantial, if not dominant, culprit for diminishing American shad spawning runs (Atlantic States Marine Fisheries Commission (ASMFC), 2020).
Fish passage is often assessed as a binary event, but even successful dam passage may still exact a toll that is not revealed through modeling. Even when fish passage opportunities are available, dams restrict the timing of access to spawning habitat (e.g., Grote et al., 2014). Dams may therefore increase a migrant’s vulnerability to predation (Andrews et al., 2018) or a fishery (Atlantic States Marine Fisheries Commission (ASMFC), 2020), as well as depleting energy stores by imposing delay. The sustained delay below a dam may, however, may preclude successfully passing fish from reaching their reproductive potential (Leonard and McCormick, 1999). Among teleosts, American shad display an elevated basal metabolic rate that is likely causal to their upstream migration being more energetically costly than anadromous salmon (Leonard and McCormick, 1999). Metabolism is determined by water temperature through its effects on enzymatic, metabolic, and cardiac processes (Lennox et al., 2018) so that accelerated energetic depletion occurs in the warmed water near dams (Martin et al., 2015).
Reduction in energy reserves may result in reduced individual fitness (Nadeau et al., 2010) by impairing spawning behaviors and gamete production (Brett, 1962; Rand and Hinch, 1998; Martin et al., 2015). Migrating adults passing a dam do experience an advantage in gaining access to habitat with fewer conspecific competitors. This may afford an advantage in cases where the juvenile rearing carrying capacity is reached, resulting in greater per capita recruitment to the juvenile stage (Walters and Korman, 1999). This advantage, if realized, comes at a significant cost for American shad in the iteroparous eco-regions. In order for spawning adults to spawn again, they must retain enough energy to reach the ocean. Fish must not only reach their spawning habitat in time to spawn, but also must maintain energy stores sufficient to return to the marine environment where they will recommence feeding and growth (Doucett et al., 1999). After spawning, adults leave spawning areas and move into the estuary (Grote et al., 2014) to feed and recondition (Walter and Olney, 2003). Delays at dams are commonly observed in downstream migrating adult shad (Weaver et al., 2019) and undoubtedly exacerbate energy loss at a time when stores are at their lowest. Impediments to the resumption of feeding may therefore increase mortality through prolonged energy loss (Castro-Santos and Letcher, 2010) or reduced performance (Leggett, 1972; Chittenden, 1976; Raabe and Hightower, 2014). Based on comparisons of iteroparous and semelparous populations, it has been suggested that a depletion of greater than 60% of energy reserves may serve as a constraint to an iteroparous life history (Glebe and Leggett, 1981b), while the threshold for post-spawn survival may be as low as 30-40% (Leonard and McCormick, 1999). In addition to a physiological constraint, these fish face high risks of mortality passing dams, particularly if passing through a turbine or other unsafe route (Bell and Kynard, 1985; Hogans and Melvin, 1985; Haro and Castro-Santos, 2012).
The population impact of an impoundment may be amplified by a sequence of dams that must be navigated twice by iteroparous adults to reach spawning habitat, thereby imposing compounding mortality risk on spawners. It is useful to differentiate between the latitudinal cline that is observed in American shad (semelparity in the south to iteroparity in the north) from reduced post-spawn adult survival in the southern and northern iteroparous eco-regions. The degree to which these life history differences reflect population level characteristics versus phenotypic plasticity is poorly characterized. Populations accessing suitable spawning habitat through anthropogenic facilities are subject to an adult mortality akin to an intercept fishery on size and age distributions and results in the systematic loss of “big old fat female fish” (Hixon et al., 2014). This has been well characterized in the Connecticut River where the proportion of repeat spawners declined from 49% in the late 1950s (Walburg and Nichols, 1967; Carscadden and Leggett, 1975; Limburg et al., 2003), to a mean of 5 % for the period 2006-2015 (Atlantic States Marine Fisheries Commission (ASMFC), 2020). Failure to recognize the important role of passage has led to the unlikely assertion that upstream passage may be detrimental to a population because of energetic constraints (Leggett et al., 2004) rather than poor downstream passage. In order to repeat spawn, adults in the SI and NI eco-regions need both access to habitat and an effective exit strategy or “forced semelparity” may result.
Whether parents are semelparous or iteroparous, the progeny of American shad spawned upstream of dams suffer the risk of mortality when moving downstream. Young American shad generally remain in freshwater until migrating downstream in the fall associated with declining river temperature (Leggett and Whitney, 1972; O’Leary and Kynard, 1986). Downstream passage at dams may entail delay, confinement and turbulence during a period of time when these fish exhibit a heightened sensitivity to stress (Shrimpton et al., 2001) and an environmentally influenced loss of ion regulatory ability in freshwater (Zydlewski and McCormick, 1997b). Juvenile shad, being far smaller than adults, are generally not effectively excluded from turbines. These fish have less individual risk than entrained adults (Heisey et al., 1992; Mathur et al., 1994) though it is biologically significant. The impacts of dams through injury or delay may shape the disposition of migrants. Their success (or failure) depends on phenology of their physiological development (Zydlewski et al., 2003) in conjunction with biotic and abiotic environmental factors (McCormick et al., 1998; Limburg, 2001).
Prior to the construction of dams range wide, American shad delivered considerable biomass and nutrient loads to freshwater systems (Limburg et al., 2003; Figure 9). It is difficult to fully appreciate the ecological significance or magnitude of delivery of 1,963 MT of nitrogen and 446 MT of phosphorus on an annual basis. The seasonal influx driven by the carcasses, gametic release and metabolism would undoubtedly change the freshwater system as has been observed for other species (Weaver et al., 2018). The estimated 34% reduction in nutrient delivery associated with dams is significant. As Haskell (2018) asserts, for American shad, spatial context is of particular importance because shad are broadcast spawners using open water rather than being associated with substrate as is the case for sea lamprey (Weaver et al., 2018) and salmon (Rex and Petticrew, 2008). Spawner distribution in a natural river results in distribution that is punctuated over time and space. Therefore the reduction in spawner potential associated with dams is not only a reduction in the magnitude of nutrient delivered, but a curtailment of the spatial distribution of marine derived nutrient incursions.
In the context of nutrient spiraling theory, in streams and rivers, downstream nutrient changes are driven by delivery, up-take and flushing (Newbold et al., 1982). As such, American shad represent only one broken link in the chain that has historically connected the marine and freshwater ecosystems through the seasons (Limburg et al., 2003). The interruption of ecological connectivity is common for many coastal ecosystems; in the Pacific Northwest nutrient delivery by Pacific salmon is estimated to be only 6-7 % of historical levels (Gresh et al., 2000). The nature of these connections likely changes over the range of the American shad, as anadromous species have a greater scope for influence in freshwater systems with nutrient limitation (nitrogen, phosphorus or co-limited) that influences the degree of nutrient incorporation (Cederholm et al., 1999; Chaloner et al., 2002; Bellmore et al., 2014; Samways and Cunjak, 2015). Increased N and P availability leads to increased primary production, but these effects may be swamped by nutrients generated from anthropogenic land use practices (Twining et al., 2013). The nutrient dynamics of American shad are less comprehensively explored than other alosines (Durbin et al., 1979; Post and Walters, 2009; Walters et al., 2009; West et al., 2010) offering an important direction of future research.
The presence of dams greatly curtails estimated nutrient transport (both import and export) and, in parallel with spawner estimates, fish passage provides a low level of restoration of this ecological function. The dynamics and magnitude of nutrient exchange are strongly influenced by population size and life history differences among the three eco-regions in the natural range. Our models project that in the NI and SI ranges, where iteroparity results in greater spawner potential, delivery is tilted towards import. Haskell (2018) also assessed American shad to be net importers of N and P in the Columbia River where iteroparity levels are 32% (Petersen et al., 2003). In the SM eco-region, despite the higher proportion of carcasses delivered during migration, we estimate that the net flux of nutrients in the southern part of their range to be significantly lower than the northern regions. The nature of delivery among regions differs as well. Carcasses of migrating fish can break down over days and weeks (Garman, 1992; Weaver et al., 2015) so nutrients are not immediately liberated whereas nutrients released via gametes and metabolic processes are more readily available.
Our presentation of average spawner escapement and juvenile transport likely obscures a more nuanced story of nutrient delivery that has been altered by the imposition of dams on the landscape. The complex role of nutrients delivered by alosine species is largely driven by levels of escapement (Barber et al., 2018), allowing locally disparate net nutrient balance. At high levels of spawner escapement, as occurs in our stabilized population models, biomass delivery (and resulting nitrogen and phosphorus delivery) are markedly skewed to import, particularly in the NI and SI regions (Figure 9) where spawner numbers are higher (Figure 6). In cases where adult dam passage is poor, the juvenile recruits produced per spawner can be expected to be higher than average, resulting in a more equitable exchange, or even a net export (Barber et al., 2018). In such cases, with reduced competition, larger migrating juveniles may further shift the net balance in favor of export (Moore and Schindler, 2004; Moore et al., 2011). Such a localized patchwork of nutrient balance may also occur in natural systems with impediments to migration (e.g., natural falls) or simply due to the attrition of spawners over the length of a river (Meixler et al., 2009; Hall et al., 2011; Pess et al., 2014). Even when a river is producing spawners, failure to connect these fish to their habitat can result in a lower import or net export in nutrient limited systems.
As juvenile American shad develop and grow, they are directly embedded into pelagic, littoral, benthic, and terrestrial systems (Limburg et al., 2003). Feeding – or being preyed upon – directly links these fish to food webs. As is the case for many anadromous species, juvenile American shad (and adults) may be directly consumed through aquatic (Willson and Halupka, 1995; Jaecks and Quinn, 2014), terrestrial and avian predators (Dalton et al., 2009; DeBruyne et al., 2012). Aquatic macroinvertebrates also actively feed on carcasses during their freshwater residency, serving as further conduit of nutrients between the terrestrial and aquatic environments (Polis et al., 1997; Vanni, 2002; Hocking and Reimchen, 2009). Both bottom-up and top-down pathways of nutrient incorporation may result from the influence that American shad likely have in the freshwater ecosystem.
The restriction of American shad to lower reaches of the coastal rivers is part of a fundamental shift in the riverine communities due to impoundment (Kiraly et al., 2015; Watson et al., 2018). Fish communities in these altered systems have both winners and losers, often favoring native and non-native “invaders” (sensu Carey et al., 2012) such as black bass (Micropterus spp.). By presenting an obstacle to migrations, dams may make American shad and other alosines increasingly vulnerable to novel predators (such blue catfish and [Ictalurus furcatus] and flathead catfish [Pylodictis olivarisast] in the Chesapeake Bay region; Schmitt et al., 2017).
In addition to the impacts on the freshwater environment, the dam mediated loss of connectivity also affects estuarine and coastal systems. The presence of adult and juvenile American shad in coastal systems benefit estuarine and marine organisms. The exodus of juvenile shad from freshwater is protracted from the summer into the fall (Williams and Bruger, 1972; O’Leary and Kynard, 1986; Zydlewski and McCormick, 1997b), overlapping with the migration of other alosines. Such migrations attract predators (Davis et al., 2012; McDermott et al., 2015), but at the same time reduce individual risk. Many predatory species depend on the seasonal pulses of prey species (Willson and Womble, 2006; Richardson et al., 2014; Furey and Hinch, 2017). As such, patterns in alosine migration may shape both current and historic distributions of marine fish species (Baird, 1883; Ames, 2004). Specialized detection of relatively high frequency sounds by American shad may also indicate evolutionary predatory pressures, suggesting that changes in abundance may influence the foraging success of echolocating marine mammals (Mann et al., 1998).
We have considered the influence of dams on American shad through their coastal range and used the best available data to quantify the dam-mediated impact on habitat loss, spawner production potential and nutrient transport range wide. The ecological potential and human value of these fish has been markedly reduced through the partial and complete occlusion of access to spawning and juvenile rearing habitat. Historically these fish have linked freshwater systems to the marine environment, from the mangrove estuaries of Florida to the boreal forests of Canada. More than 100 years ago Stevenson (1899) asserted that “There is no species of fish more important to residents of the Atlantic seaboard than the shad.” The fisheries, and the human connections to the fish, have diminished in spite of fisheries closures and extensive passage efforts (Atlantic States Marine Fisheries Commission (ASMFC), 2020). The data suggest that dams remain the most significant impediment to restoration of the “founding fish” (McPhee, 2003). While contemporary passage rates fail to achieve even modest population recovery, dam removal appears to remain a viable solution (Raabe and Hightower, 2014; Izzo et al., 2016; Moser and Paradis, 2017; Watson et al., 2018). The recolonization of newly accessible habitat by migratory fish is well-documented (e.g., Burdick and Hightower, 2006; Hogg et al., 2013). While decision-making at dams involves a wide range of stakeholders with diverse and sometimes conflicting objectives (Roy et al., 2018), “active restoration” has been framed as a balanced approach that integrates both values and science (Hart et al., 2002). There is a growing appreciation for the biological and economic benefits of restoring coastal connectivity (Dias et al., 2019), thereby regaining that which we have lost.
Data Availability Statement
The original contributions presented in the study are included in the article/Supplementary Material, further inquiries can be directed to the corresponding author/s.
Author Contributions
JZ led in the framing of the manuscript and compilation of data and led the writing. DS led the population modeling and was a major contributor to writing. SR led the GIS analysis and was a major contributor to writing. All authors contributed to the framing of the manuscript, interpretation and editorial review.
Conflict of Interest
The authors declare that the research was conducted in the absence of any commercial or financial relationships that could be construed as a potential conflict of interest.
Publisher’s Note
All claims expressed in this article are solely those of the authors and do not necessarily represent those of their affiliated organizations, or those of the publisher, the editors and the reviewers. Any product that may be evaluated in this article, or claim that may be made by its manufacturer, is not guaranteed or endorsed by the publisher.
Acknowledgments
Logistical support was provided by the United States Geological Survey Maine Cooperative Fish and Wildlife Research Unit. Any use of trade, firm, or product names is for descriptive purposes only and does not imply endorsement by the United States Government. The findings and conclusions in this article are those of the authors and do not necessarily represent the views of the U.S. Fish and Wildlife Service. All data generated or analyzed during this study are included in the main text or as Supplementary Information for this publication. We thank the Atlantic States Marine Fisheries Commission (ASMFC) and individuals from the ASMFC American Shad Technical Committee and Stock Assessment Subcommittee (SAS) members who contributed data and knowledge that has informed our efforts. We are exceptionally grateful to the dozens of biologists from both the United States and Canada who generously shared their local knowledge of the landscape, habitat use and history of American shad in their region. Only fear of accidental exclusion prevents the authors’ attempt at listing the names of those who spoke for the American shad.
Supplementary Material
The Supplementary Material for this article can be found online at: https://www.frontiersin.org/articles/10.3389/fmars.2021.734213/full#supplementary-material
Footnotes
References
Ames, E. P. (2004). Atlantic cod stock structure in the Gulf of Maine. Fisheries 29, 10–28. doi: 10.1577/1548-8446(2004)29[10:acssit]2.0.co;2
Andrews, S. N., Zelman, K., Ellis, T., Linnansaari, T., and Curry, R. A. (2018). Diet of striped bass and muskellunge downstream of a large hydroelectric dam: a preliminary investigation into suspected Atlantic salmon smolt predation. North Am. J. Fish. Manag. 38, 734–746. doi: 10.1002/nafm.10074
Atlantic States Marine Fisheries Commission (ASMFC) (2007). American Shad Stock Assessment Report for Peer Review: Volume I - Stock Assessment Overview. Arlington, VA: ASMFC.
Atlantic States Marine Fisheries Commission (ASMFC) (2020). American shad Benchmark Stock Assessment and Peer Review. Arlington, VA: ASMFC.
Bailey, M. M., and Zydlewski, J. D. (2013). To stock or not to stock? Assessing the restoration potential of a remnant American Shad spawning run with hatchery supplementation. North Am. J. Fish. Manag. 33, 459–467. doi: 10.1080/02755947.2013.763874
Baird, S. (1883). U.S. Commissioner of Fish and Fisheries Report of 1883. Washington, DC: NOAA, xi–xiv.
Barber, B. L., Gibson, A. J., O’Malley, A. J., and Zydlewski, J. (2018). Does what goes up also come down? Using a recruitment model to balance alewife nutrient import and export. Mar. Coast. Fish. 10, 236–254. doi: 10.1002/mcf2.10021
Bell, C. E., and Kynard, B. (1985). Mortality of adult American shad passing through a 17 megawatt Kaplan turbine at a low-head hydroelectric dam. North Am. J. Fish. Manag. 5, 33–38. doi: 10.1577/1548-8659(1985)5<33:moaasp>2.0.co;2
Bellmore, J. R., Fremier, A. K., Mejia, F., and Newsom, M. (2014). The response of stream periphyton to Pacific salmon: using a model to understand the role of environmental context. Freshw. Biol. 59, 1437–1451. doi: 10.1111/fwb.12356
Bent, G. C., and Waite, A. M. (2013). Equations for Estimating Bankfull Channel Geometry and Discharge for Streams in Massachusetts. U.S. Geological Survey Scientific Investigations Report 2013–5155. Reston, VA: U.S. Geological Survey, doi: 10.3133/sir20135155
Beverton, R. J. J., and Holt, S. J. (1957). On the dynamics of exploited fish populations. United Kingdom Ministry of Agriculture and Fisheries. Fisheries Investigations, Vol. 19. London: H.M. Stationery Office.
Bilkovic, D. M., Olney, J. E., and Hershner, C. H. (2002). Spawning of American shad (Alosa sapidissima) and striped bass (Morone saxatilis) in the Mattaponi and Pamunkey Rivers, Virginia. Fish. Bull. 100:632.
Bradley, M. C. (1959). An Ecological Survey Of The Potomac And Anacostia Rivers With Special Emphasis On Pollution. Washington, DC: Catholic University of America Press.
Bray, D. I. (1982). “Regime equations for gravel-bed rivers,” in Gravel-Bed Rivers, eds R. D. Hey, J. C. Bathurst and C. R. Thome (Chichester: Wiley), 517–542.
Brett, J. R. (1962). Some considerations in the study of respiratory metabolism in fish, particularly salmon. J. Fish. Board Can. 19, 1025–1038. doi: 10.1139/f62-067
Bunt, C. M., Castro-Santos, T., and Haro, A. (2012). Performance of fish passage structures at upstream barriers to migration. River Res. Applic. 28, 457–478. doi: 10.1002/rra.1565
Bunt, C. M., Castro-Santos, T., and Haro, A. (2016). Reinforcement and validation of the analyses and conclusions related to fishway evaluation data from Bunt ‘performance of fish passage structures at upstream barriers to migration. River Res. Applic. 32, 2125–2137. doi: 10.1002/rra.3095
Burdick, G. E. (1954). An analysis of the factors, including pollution, having possible influence on the abundance of shad in the Hudson River. N. Y. Fish Game J. 1, 188–205.
Burdick, S. M., and Hightower, J. E. (2006). Distribution of spawning activity by anadromous fishes in an Atlantic slope drainage after removal of a low-head dam. Trans. Am. Fish. Soc. 135, 1290–1300. doi: 10.1577/t05-190.1
Carey, M. P., Sanderson, B. L., Barnas, K. A., and Olden, J. D. (2012). Native invaders–challenges for science, management, policy, and society. Front. Ecol. Environ. 10:373–381. doi: 10.1890/110060
Carscadden, J. E., and Leggett, W. C. (1975). Meristic differences in spawning populations of American shad, Alosa sapidissima: evidence for homing to tributaries in the St. John River, New Brunswick. J. Fish. Board Can. 32, 653–660. doi: 10.1139/f75-084
Castro-Santos, T., and Letcher, B. H. (2010). Modeling migratory energetics of Connecticut River American shad (Alosa sapidissima): implications for the conservation of an iteroparous anadromous fish. Can. J. Fish. Aquat. Sci. 67, 806–830. doi: 10.1139/f10-026
Castro-Santos, T., Sprankle, K., and Perry, R. (2016). “Passage performance and migration delay of American Shad and the Holyoke Fishlifts. Fish Passage 2016,” in Proceedings of the International Conference on River Connectivity, University of Massachusetts, Amherst, MA. June 20-22, 2016, (Amherst, MA),Google Scholar
Cederholm, C. J., Kunze, M. D., Murota, T., and Sibatani, A. (1999). Pacific salmon carcasses: essential contributions of nutrients and energy for aquatic and terrestrial ecosystems. Fisheries 24, 6–15. doi: 10.1577/1548-8446(1999)024<0006:psc>2.0.co;2
Chaloner, D. T., Martin, K. M., Wipfli, M. S., Ostrom, P. H., and Lamberti, G. A. (2002). Marine carbon and nitrogen in southeastern Alaska stream food webs: evidence from artificial and natural streams. Can. J. Fish. Aquat. Sci. 59, 1257–1265.
Chittenden, M. E. Jr. (1969). Life History and Ecology of the American shad, Alosa sapidissima, in the Delaware River. Doctoral Dissertation. New Brunswick, NJ: Rutgers University.
Chittenden, M. E. (1976). Weight loss, mortality, feeding, and duration of residence of adult American shad, Alosa sapidissima, in fresh water. Fish. Bull. 74, 151–157.
CRASC (2020). Addendum on American Shad Passage Performance Criteria, for the Connecticut River American Shad Management Plan. Sunderland, MA: CRASC.
Crecco, V., Savoy, T., and Gunn, L. (1983). Daily mortality rates of larval and juvenile American shad (Alosa sapidissima) in the Connecticut River with changes in year-class strength. Can. J. Fish. Aquat. Sci. 40, 1719–1728.
Dadswell, M. J., Spares, A. D., Mclean, M. F., Harris, P. J., and Rulifson, R. A. (2018). Long-term effects of tidal hydroelectric propeller turbine on the populations of three anadromous fish species. J. Fish. Biol. 93, 192–206. doi: 10.1111/jfb.13755
Dalton, C. M., Ellis, D., and Post, D. M. (2009). The impact of double-crested cormorant (Phalacrocorax auritus) predation on anadromous alewife (Alosa pseudoharengus) in south-central Connecticut, USA. Can. J. Fish. Aquat. Sci. 66, 177–186. doi: 10.1139/f08-198
Davis, J. P., Schultz, E. T., and Vokoun, J. C. (2012). Striped Bass consumption of Blueback Herring during vernal riverine migrations: does relaxing harvest restrictions on a predator help conserve a prey species of concern? Mar. Coast. Fish. 4, 239–251. doi: 10.1080/19425120.2012.675972
DeBruyne, R. L., DeVault, T. L., and Duerr, A. E. (2012). Spatial and temporal comparisons of double-crested cormorant diets following the establishment of Alewife in Lake Champlain, USA. J. Great Lakes Res. 38, 123–130. doi: 10.1016/j.jglr.2011.05.001
Dias, B. S., Frisk, M. G., and Jordaan, A. (2019). Opening the tap: Increased riverine connectivity strengthens marine food web pathways. PLoS One 14:e0217008. doi: 10.1371/journal.pone.0217008
Dodson, J. J., Laroche, J., and Lecomte, F. (2009). Contrasting evolutionary pathways of anadromy in euteleostean fishes. Am. Fish. Soc. Symp. 69, 63–77.
Doucett, R. R., Hooper, W., and Power, G. (1999). Identification of anadromous and nonanadromous adult brook trout and their progeny in the Tabusintac River, New Brunswick, by means of multiple-stable-isotope analysis. Trans. Am. Fish. Soc. 128, 278–288. doi: 10.1577/1548-8659(1999)128<0278:ioaana>2.0.co;2
Doughty, C. E., Roman, J., Faurby, S., Wolf, A., Haque, A., Bakker, E. S., et al. (2016). Global nutrient transport in a world of giants. Proc. Natl. Acad. Sci. U.S.A. 113, 868–873. doi: 10.1073/pnas.1502549112
Dudley, R. W. (2004). Hydraulic-Geometry Relations for Rivers in Coastal and Central Maine. Scientific Investigations Report 2004-5042. Reston, VA: U.S. Geological Survey.
Durbin, A. G., Nixon, S. W., and Oviatt, C. A. (1979). Effects of the spawning migration of the Alewife, Alosa pseudoharengus, on freshwater ecosystems. Ecology 60, 8–17. doi: 10.2307/1936461
Exelon. (2012). Estimation of Survival of Adult American Shad Passed Through Francis and Kaplan Turbines. RSP 3.2 Conowingo Hydroelectric Project. FERC # 405. Prepared by Normandeau Associates and Gomez and Sullivan Engingeers. Utica, NY: New Hampshire and Gomez and Sullivan Engineers, P.C. Available online at: https://mde.state.md.us/programs/Water/WetlandsandWaterways/Documents/ExelonMD/FERC/Conowingo-FRSP-3.02.pdf (accessed October 5, 2021).
FirstLight (2016). Evaluate Downstream Passage of Juvenile American Shad, Interim Study Report. Washington, DC: FERC Relicensing.
Foster, N. W., and Atkins, C. G. (1867). Report of Commission on Fisheries. In Twelfth annual report of the Secretary of the Maine Board of Agriculture. Stevens and Sayward Printers to the State. Augusta, ME: Secretary of the Maine Board of Agriculture.
Furey, N. B., and Hinch, S. G. (2017). Bull trout movements match the life history of sockeye salmon: consumers can exploit seasonally distinct resource pulses. 2017. Trans. Am. Fish. Soc. 146, 450–461. doi: 10.1080/00028487.2017.1285353
Garman, G. C. (1992). Fate and potential significance of post spawning anadromous fish carcasses in an Atlantic coastal river. Trans. Am. Fish. Soc. 121, 390–394. doi: 10.1577/1548-8659(1992)121<0390:fapsop>2.3.co;2
Gibson, A. J. F., and Meyers, R. A. (2002). A logistic regression model for estimating turbine mortality at hydroelectric generating stations. Trans. Am. Fish. Soc. 131, 623–633. doi: 10.1577/1548-8659(2002)131<0623:alrmfe>2.0.co;2
Gilligan-Lunda, E. K., Stich, D. S., Mills, K. E., Bailey, M. M., and Zydlewski, J. D. (2021). Climate change may cause shifts in growth and instantaneous natural mortality of American Shad throughout their native range. Trans. Am. Fish. Soc. 150, 407–421. doi: 10.1002/tafs.10299
Glebe, B. D., and Leggett, W. C. (1981a). Latitudinal differences in energy allocation and use during the freshwater migrations of American shad (Alosa sapidissima) and their life history consequences. Can. J. Fish. Aquat. Sci. 38, 806–820. doi: 10.1139/f81-109
Glebe, B. D., and Leggett, W. C. (1981b). Temporal, intra-population differences in energy allocation and use by American shad (Alosa sapidissima) during the spawning migration. Can. J. Fish. Aquat. Sci. 38, 795–805. doi: 10.1139/f81-108
Goethel, D. R., Quinn, T. J., and Cadrin, S. X. (2011). Incorporating spatial structure in stock assessment: movement modeling in marine fish population dynamics. Rev. Fish. Sci. 19, 119–136. doi: 10.1080/10641262.2011.557451
Gresh, T., Lichatowich, J., and Schoonmaker, P. (2000). An estimation of historic and current levels of salmon production in the Northeast Pacific ecosystem: evidence of a nutrient deficit in the freshwater systems of the Pacific Northwest. Fisheries 25, 15–21. doi: 10.1577/1548-8446(2000)025<0015:aeohac>2.0.co;2
Grote, A. B., Bailey, M. M., and Zydlewski, J. D. (2014). Movements and demography of spawning American shad in the Penobscot River, Maine, prior to dam removal. Trans. Am. Fish. Soc. 143, 552–563. doi: 10.1080/00028487.2013.864705
Groux, F., Therrien, J., Chanseau, M., Courret, D., and Tetard, S. (2015). Knowledge Update on Shad Upstream Migration Fishway Design and Efficiency – Project LIFE09 NAT/DE/000008 – Conservation and Restoration of the Allis Shad in the Gironde and Rhine watersheds – Action A1. Report from WSP to ONEMA. 81.
Hall, C. J., Jordaan, A., and Frisk, M. G. (2011). The historic influence of dams on diadromous fish habitat with a focus on river herring and hydrologic longitudinal connectivity. Landsc. Ecol. 26, 95–107. doi: 10.1007/s10980-010-9539-1
Haro, A., and Castro-Santos, T. (2012). Passage of American Shad: paradigms and realities. Mar. Coast. Fish. 4, 252–261. doi: 10.1080/19425120.2012.675975
Harris, J. E., and Hightower, J. E. (2012). Demographic population model for american shad: will access to additional habitat upstream of dams increase population sizes? Mar. Coast. Fish. 4, 262–283. doi: 10.1080/19425120.2012.675969
Hart, D. D., Johnson, T. E., Bushaw-Newton, K. L., Horwitz, R. J., Bednarek, A. T., Charles, D. F., et al. (2002). Dam removal: challenges and opportunities for ecological research and river restoration: we develop a risk assessment framework for understanding how potential responses to dam removal vary with dam and watershed characteristics, which can lead to more effective use of this restoration method. BioScience 52, 669–682.
Haskell, C. A. (2018). From salmon to shad: Shifting sources of marine-derived nutrients in the Columbia River Basin. Ecol. Freshw. Fish. 27, 310–322. doi: 10.1111/eff.12348
Hasselman, D. J., Bradford, R. G., and Bentzen, P. (2010). Taking stock: defining populations of American shad (Alosa sapidissima) in Canada using neutral genetic markers. Can. J. Fish. Aquat. Sci. 67, 1021–1039. doi: 10.1139/f10-031
Hasselman, D. J., and Limburg, K. E. (2012). Alosine restoration in the 21st century: challenging the status quo. Mar. Coast. Fish. 4, 174–187. doi: 10.1080/19425120.2012.675968
Hasselman, D. J., Ricard, D., and Bentzen, P. (2013). Genetic diversity and differentiation in a wide ranging anadromous fish, American shad (Alosa sapidissima), is correlated with latitude. Mol. Ecol. 22, 1558–1573. doi: 10.1111/mec.12197
Heisey, P. G., Mathur, D., and Rineer, T. (1992). A reliable tag-recapture technique for estimating turbine passage survival: application to young-of-the-year American Shad (Alosa sapidissima). Can. J. Fish. Aquat. Sci. 49, 1826–1834. doi: 10.1139/f92-202
Hightower, J. E., Harris, J. E., Raabe, J. K., Brownell, P., and Drew, C. A. (2012). A Bayesian spawning habitat suitability model for American Shad in southeastern United States Rivers. J. Fish. Wildlife Manag. 3, 184–198. doi: 10.3996/082011-jfwm-047
Hightower, J. E., and Sparks, K. L. (2003). “Migration and spawning habitat of American shad in the Roanoke river, North Carolina,” in Biodiversity, Status, and Conservation Of The World’s Shads, eds K. E. Limburg and J. R. Waldman (Bethesda: American Fisheries Society Symposium), 193–199.
Hightower, J. E., Wicker, A. M., and Endres, K. M. (1996). Historical trends in abundance of American shad and river herring in Albemarle Sound, North Carolina. North Am. J. Fish. Manag. 16, 257–271. doi: 10.1577/1548-8675(1996)016<0257:htiaoa>2.3.co;2
Hill, D. R. (1959). Some Uses of Statistical Analysis in Classifying Races of American Shad (Alosa sapidissima). Washington, DC: US Government Printing Office.
Hixon, M. A., Johnson, D. W., and Sogard, S. M. (2014). BOFFFs: on the importance of conserving old- growth age structure in fishery populations. ICES J. Mar. Sci. 71, 2171–2185. doi: 10.1093/icesjms/fst200
Hocking, M. D., and Reimchen, T. E. (2009). Salmon species, density and watershed size predict magnitude of marine enrichment in riparian food webs. Oikos 118, 1307–1318. doi: 10.1111/j.1600-0706.2009.17302.x
Hogans, W. E., and Melvin, G. D. (1985). Mortality of Adult American Shad (Alosa sapidissima) Passed Through a Stratflo Turbine at the low Head Tidal Power Generating Station at Annapolis Royal, Nova Scotia. Wolfville, NS: T. P. H. Applied Fisheries Research, Inc.
Hogg, R., Coghlan, S. M. Jr., and Zydlewski, J. (2013). Anadromous sea lampreys recolonize a Maine coastal river tributary after dam removal. Trans. Am. Fish. Soc. 142, 1381–1394. doi: 10.1080/00028487.2013.811103
Hyle, A. R., McBride, R. S., and Olney, J. E. (2014). Determinate versus indeterminate fecundity in American shad, an anadromous clupeid. Trans. Am. Fisher. Soc. 143, 618–633. doi: 10.1080/00028487.2013.862178
Izzo, L. K., Maynard, G. A., and Zydlewski, J. (2016). Upstream movements of Atlantic salmon in the lower Penobscot River, Maine following two dam removals and fish passage modifications. Mar. Coast. Fish. 8, 448–461. doi: 10.1080/19425120.2016.1185063
Jaecks, T., and Quinn, T. P. (2014). Ontogenetic shift to dependence on salmon-derived nutrients in Dolly Varden char from the Iliamna River, Alaska. Environ. Biol. Fish. 97, 1323–1333. doi: 10.1007/s10641-014-0221-3
Kelt, D. A., and Van Vuren, D. H. (2001). The ecology and macroecology of mammalian home range area. Am. Natural. 157, 637–645. doi: 10.2307/3079304
Kiraly, I. A., Coghlan, S. M. Jr., Zydlewski, J., and Hayes, D. (2015). An assessment of fish assemblage structure in a large river. River Res. Applic. 31, 301–312. doi: 10.1002/rra.2738
Larinier, M., and Travade, F. (2002). The design of fishways for shad. Bull. Francais Peche Piscicul. 364, 135–146. doi: 10.1051/kmae/2002098
Leggett, W. C. (1972). Weight loss in American shad (Alosa sapidissima), Wilson) during the freshwater migration. Trans. Am. Fish. Soc. 101, 549–552. doi: 10.1577/1548-8659(1972)101<549:wliasa>2.0.co;2
Leggett, W. C., and Carscadden, J. E. (1978). Latitudinal variation in reproductive characteristics of American Shad (Alosa sapidissima): Evidence for population specific life history strategies in fish. J. Fish. Res. Board Can. 35, 1469–1478. doi: 10.1139/f78-230
Leggett, W. C., Savoy, T. F., and Tomichek, C. A. (2004). “The impact of enhancement initiatives on the structure and dynamics of the Connecticut River population of American shad,” in The Connecticut River Ecological Study (1965–1973) Revisited: Ecology of the Lower Connecticut River, 1973–2003. American Fisheries Society, Monograph 9, eds P. M. Jacobson, D. A. Dixon, W. C. Leggett, and B. C. Marcy Jr., and R. R. Massengill (Bethesda, MD: American Fisheries Society), 391–405.
Leggett, W. C., and Whitney, R. R. (1972). Water temperature and the migrations of American shad. Fish. Bull. 70, 659–670.
Lennox, R. J., Eliason, E. J., Havn, T. B., Johansen, M. R., Thorstad, E. B., Cooke, S. J., et al. (2018). Bioenergetic consequences of warming rivers to adult Atlantic salmon Salmo salar during their spawning migration. Freshw. Biol. 63, 1381–1393. doi: 10.1111/fwb.13166
Leonard, J. B., and McCormick, S. D. (1999). Effects of migration distance on whole-body and tissue-specific energy use in American shad (Alosa sapidissima). Can. J. Fish. Aquat. Sci. 56, 1159–1171. doi: 10.1139/f99-041
Leopold, L., and Maddock, T. (1953). The hydraulic geometry of stream channels and some physiographic implications. U.S. Geol. Survey Prof. Pap. 252, 57.
Liermann, C. R., Nilsson, C., Robertson, J., and Ng, R. Y. (2012). Implications of dam obstruction for global freshwater fish diversity. BioScience 62, 539–548. doi: 10.1525/bio.2012.62.6.5
Limburg, K., Hattala, K., and Kahnle, A. (2003). American Shad in its Native Range. Biodivers. Status Conserv. Worlds Shads. 125–140.
Limburg, K. E. (2001). Through the gauntlet again: demographic restructuring of American shad by migration. Ecology 82, 1584–1596. doi: 10.1890/0012-9658(2001)082[1584:ttgadr]2.0.co;2
Limburg, K. E., and Waldman, J. R. (2009). Dramatic declines in North Atlantic diadromous fishes. BioScience 59, 955–965. doi: 10.1525/bio.2009.59.11.7
Mann, D. A., Lu, Z., Hastings, M. C., and Popper, A. N. (1998). Detection of ultrasonic tones and simulated dolphin echolocation clicks by a teleost fish, the American shad (Alosa sapidissima). J. Acoustical Soc. Am. 104, 562–568. doi: 10.1121/1.423255
Mansueti, R., and Kolb, H. (1953). A Historical Review of the Shad Fisheries of North America. Solomons, MD: Chesapeake Biological Laboratory.
Martin, B. T., Nisbet, R. M., Pike, A., Michel, C. J., and Danner, E. M. (2015). Sport science for salmon and other species: ecological consequences of metabolic power constraints. Ecol. Lett. 18, 535–544. doi: 10.1111/ele.12433
Martin, E. (2013). Chesapeake Fish Passage Prioritization. Available online at: http://maps.tnc.org/EROF_ChesapeakeFPP/ (accessed October 5, 2021).
Martin, E. H. (2019). Assessing and prioritizing barriers to aquatic connectivity in the Eastern United States. J. Am. Water Resour. Assoc. 55, 401–412. doi: 10.1111/1752-1688.12694
Martin, E. H., and Apse, C. D. (2011). Northeast Aquatic Connectivity: An Assessment of Dams on Northeastern Rivers. The Nature Conservancy, Eastern Freshwater Program. Arlington, VA: The Nature Conservancy.
Mathur, D., Heisey, P. G., and Robinson, D. A. (1994). Turbine passage mortality of juvenile American shad at a low head hydroelectric dam. Trans. Am. Fish. Soc. 123, 108–111. doi: 10.1577/1548-8659(1994)123<0108:ntpmoj>2.3.co;2
McBride, R. S., Ferreri, R., Towle, E. K., Boucher, J. M., and Basilone, G. (2016). Yolked oocyte dynamics support agreement between determinate- and indeterminate-method estimates of annual fecundity for a northeastern United States population of American shad. PLoS One 11:e0164203. doi: 10.1371/journal.pone.0164203
McCormick, S. D., Hansen, L. P., Quinn, T. P., and Saunders, R. L. (1998). Movement, migration, and smolting of Atlantic salmon (Salmo salar). Can. J. Fish. Aquat. Sci. 55, 77–92. doi: 10.1139/d98-011
McDermott, S. P., Bransome, N. C., Sutton, S. E., Smith, B. E., Link, J. S., and Miller, T. J. (2015). Quantifying alosine prey in the diets of marine piscivores in the Gulf of Maine. J. Fish. Biol. 86, 1811–1829. doi: 10.1111/jfb.12692
McDowall, R. M. (1987). “The occurrence and distribution of diadromy among fishes, Vol. 1,” in Common Strategies of Anadromous and Catadromous Fishes, eds M. J. Dadswell, R. J. Klauda, C. M. Moffit, R. L. Saunders, R. A. Rulifson, and J. E. Cooper (Bathesda, MD: American Fisheries Society Symposium), 1–13.
McKay, L., Bondelid, T., Dewald, T., Johnston, J., Moore, R., and Rea, A. (2012). NHDPlus Version 2: User Guide. Washington, DC: US Environmental Protection Agency.
Meixler, M. S., Bain, M. B., and Walter, M. T. (2009). Predicting barrier passage and habitat suitability for migratory fish species. Ecol. Modell. 220, 2782–2791. doi: 10.1016/j.ecolmodel.2009.07.014
Melvin, G. D., Dadswell, M. J., and Martin, J. D. (1986). Fidelity of American shad, Alosa sapidissima (Clupeidae), to its river of previous spawning. Can. J. Fish. Aquat. Sci. 43, 640–646. doi: 10.1139/f86-077
Miranda, L. E., Bettoli, P. W., and Brown, M. L. (2007). in Analysis and Interpretation of Freshwater Fisheries Data, eds C. S. Guy and M. L. Brown (Bethesda MD: American Fisheries Society), 229–277. doi: 10.47886/9781888569773.ch6
Mohamoud, Y. M., and Parmar, R. S. (2006). Estimating streamflow and associated hydraulic geometry, the mid-atlantic region, USA 1. JAWRA J. Am. Water Resour. Assoc. 42, 755–768. doi: 10.1111/j.1752-1688.2006.tb04490.x
Moore, J. W., Hayes, S. A., Duffy, W., Gallagher, S., Michel, C. J., and Wright, D. (2011). Nutrient fluxes and the recent collapse of coastal California salmon populations. Can. J. Fish. Aquat. Sci. 68, 1161–1170. doi: 10.1139/f2011-054
Moore, J. W., and Schindler, D. E. (2004). Nutrient export from freshwater ecosystems by anadromous Sockeye Salmon (Oncorhynchus nerka). Can. J. Fish. Aquat. Sci. 61, 1582–1589. doi: 10.1139/f04-103
Moser, M. L., and Paradis, R. L. (2017). Pacific lamprey restoration in the Elwha River drainage following dam removals. Am. Curr. 42, 3–8.
Nadeau, P. S., Hinch, S. G., Hruska, K. A., Pon, L. B., and Patterson, D. A. (2010). The effects of experimental energy depletion on the physiological condition and survival of adult sockeye salmon (Oncorhynchus nerka) during spawning migration. Environ. Biol. Fish. 88, 241–251. doi: 10.1007/s10641-010-9635-8
Naiman, R. J., Bilby, R. E., Schindler, D. E., and Helfield, J. M. (2002). Pacific salmon, nutrients, and the dynamics of freshwater and riparian ecosystems. Ecosystems 5, 399–417. doi: 10.1007/s10021-001-0083-3
Natural Resources Canada. (2019). National Hydrographic Network (NHN). Available online at: http://ftp.maps.canada.ca/pub/nrcan_rncan/vector/geobase_nhn_rhn/ (accessed October 5, 2021).
Newbold, J. D., Mulholland, P. J., Elwood, J. W., and O’neill, R. V. (1982). Organic carbon spiralling in stream ecosystems. Oikos 38, 266–272. doi: 10.2307/3544663
Nichols, P. R. (1966). Comparative Study of Juvenile American Shad Populations by Finray and Scute Counts (No. 525). US Department of the Interior, Bureau of Commercial Fisheries. Washington, DC: US Department of the Interior.
NOAA (2009). Biological Valuation of Atlantic Salmon Habitat Within the Gulf of Maine Distinct Population Segment. Gloucester, MA: NOAA National Marine Fisheries Service.
Noonan, M. J., Grant, J. W., and Jackson, C. D. (2012). A quantitative assessment of fish passage efficiency. Fish Fish. 13, 450–464. doi: 10.1111/j.1467-2979.2011.00445.x
O’Leary, J. A., and Kynard, B. (1986). Behavior, length, and sex ratio of seaward-migrating juvenile American shad and blueback herring in the Connecticut River. Trans. Am. Fish. Soc. 115, 529–536. doi: 10.1577/1548-8659(1986)115<529:blasro>2.0.co;2
Olney, J. E., and Hoenig, J. M. (2001). Managing a fishery under moratorium: assessment opportunities for Virginia’s stocks of American shad. Fisheries 26, 6–12. doi: 10.1577/1548-8446(2001)026<0006:mafuma>2.0.co;2
Olney, J. E., and McBride, R. S. (2003). Intraspecific variation in batch fecundity of American shad: revisiting the paradigm of reciprocal latitudinal trends in reproductive traits. Am. Fish. Soc. Symp. 35, 185–192.
Opperman, J. J., Royte, J., Banks, J., Day, L. R., and Apse, C. (2011). The Penobscot River, Maine, USA: a basin-scale approach to balancing power generation and ecosystem restoration. Ecol. Soc. 16:7.
Pess, G. R., Quinn, T. P., Gephard, S. R., and Saunders, R. (2014). Re-colonization of Atlantic and Pacific rivers by anadromous fishes: linkages between life history and the benefits of barrier removal. Rev. Fish Biol. Fish. 24, 881–900. doi: 10.1007/s11160-013-9339-1
Petersen, J. H., Hinrichsen, R. A., Gadomski, D. M., Feil, D. H., and Rondorf, D. W. (2003). “American shad in the Columbia River,” in Biodiversity, Status, and Conservation of the World’s Shads, Vol. 35, eds K. E. Limburg and J. R. Waldman (Bethesda, MD: American Fisheries Society, Symposium), 141–155.
Polis, G. A., Anderson, W. B., and Holt, T. D. (1997). Toward an integration of landscape and food web ecology: the dynamics of spatially subsidized food webs. Annu. Rev. Ecol. Evol. Syst. 28, 289–316. doi: 10.1146/annurev.ecolsys.28.1.289
Post, D. M., and Walters, A. W. (2009). Nutrient excretion rates of anadromous Alewives during their spawning migration. Trans. Am. Fish. Soc. 138, 264–268. doi: 10.1577/t08-111.1
Provost, J. (1987). L’alose Savoureuse (Alosa sapidissima, Wilson) du Fleuve Saint-Laurent: Etude Comparative des Phenotypes Morphologiques et de Certains Aspects de la Biologie de Quelques Populations. Master’s Thesis. Quebec: University of Quebec. (In French.).
R Core Team (2019). R: A Language and Environment for Statistical Computing. Vienna: R Foundation for Statistical Computing.
Raabe, J. K., and Hightower, J. E. (2014). American Shad migratory behavior, weight loss, survival, and abundance in a North Carolina river following dam removals. Trans. Am. Fish. Soc. 143, 673–688. doi: 10.1080/00028487.2014.882410
Rand, P. S., and Hinch, S. G. (1998). Swim speeds and energy use of upriver-migrating sockeye salmon (Oncorhynchus nerka): simulating metabolic power and assessing risk of energy depletion. Can. J. Fish. Aquat. Sci. 55, 1832–1841. doi: 10.1139/f98-068
Rex, J. F., and Petticrew, E. L. (2008). Delivery of marine-derived nutrients to streambeds by Pacific salmon. Nat. Geosci. 1, 840–843. doi: 10.1038/ngeo364
Richardson, D. E., Palmer, M. C., and Smith, B. E. (2014). The influence of forage fish abundance on the aggregation of Gulf of Maine Atlantic cod (Gadus morhua) and their catchability in the fishery, Cooper A, editor. Can. J. Fish. Aquat. Sci. 71, 1349–1362. doi: 10.1139/cjfas-2013-0489
Rosenzweig, M. L. (1971). Paradox of enrichment: destabilization of exploitation ecosystems in ecological time. Science 171, 385–387. doi: 10.1126/science.171.3969.385
Roy, S., Daignault, A., Zydlewski, J., Truhlar, A., Smith, S., Jain, S., et al. (2020). Coordinated river infrastructure decisions enhance social-ecological resilience. Environ. Res. Lett. 15:104054. doi: 10.1088/1748-9326/abad58
Roy, S., Uchida, E., Souza, S., Blachly, B., Fox, E., Gardner, K., et al. (2018). Damming decisions: a multi-scale approach to balance trade-offs among dam infrastructure, river restoration, and cost. Proc. Natl. Acad. Sci. U.S.A. 115, 12069–12074. doi: 10.1073/pnas.1807437115
Rulifson, R. A. (1994). “Status of anadromous Alosa along the east coast of North American,” in Anadromous Alosa Symposium: Proceedings of a Symposium held at the Seventh Annual meeting of the Tidewater Chapter in Virginia Beach, Virginia, 14-15 January 1993, eds J. E. Cooper, R. T. Eades, R. J. Klauda, and J. G. Loesch (Bethesda, MD: American Fisheries Society), 134–158.
Samways, K. M., and Cunjak, R. A. (2015). Increases in benthic community production and metabolism in response to marine-derived nutrients from spawning Atlantic Salmon (Salmo salar). Freshw. Biol. 60, 1647–1658. doi: 10.1111/fwb.12597
Schindler, D. E., Scheuerell, M. D., Moore, J. W., Gende, S. M., Francis, T. B., and Palen, W. J. (2003). Pacific salmon and the ecology of coastal ecosystems. Front. Ecol. Environ. 1:31–37.
Schmitt, J. D., Hallerman, E. M., Bunch, A., Moran, Z., Emmel, J. A., and Orth, D. J. (2017). Predation and prey selectivity by nonnative catfish on migrating alosines in an Atlantic slope estuary. Mar. Coast. Fish. 9, 108–125. doi: 10.1080/19425120.2016.1271844
Shrimpton, J. M., Zydlewski, J. D., and McCormick, S. D. (2001). The stress response of juvenile American shad to handling and confinement is greater during migration in freshwater than in seawater. Trans. Am. Fish. Soc. 130, 1203–1210. doi: 10.1577/1548-8659(2001)130<1203:tsroja>2.0.co;2
Silva, A. T., Lucas, M. C., Castro-Santos, T., Katopodis, C., Baumgartner, L. J., Thiem, J. D., et al. (2018). The future of fish passage science, engineering, and practice. Fish Fish. 19, 340–362. doi: 10.1111/faf.12258
Song, C., O’Malley, A., Roy, S., Zydlewski, J., Barber, B., and Mo, W. (2019). Managing dams for energy and fish tradeoffs: what does a win-win solution take? Sci. Total Environ. 669, 833–843. doi: 10.1016/j.scitotenv.2019.03.042
Stevenson, C. H. (1899). The Shad Fisheries of the Atlantic Coast of the United States. Pages 101–269 in U.S. Commission of Fish and Fisheries, part24, Report of the Commissioner for the Year Ending June 30, 1898. Washington, DC: U.S. Commission of Fish and Fisheries.
Stich, D. S., Roy, S. G., and Zydlewski, J. D. (2020). Anadrofish: Anadromous Fish Population Responses to Habitat Changes. R Package Version 1.1.0. Available online at: https://github.com/danStich/anadrofish (accessed October 5, 2021).
Stich, D. S., Sheehan, T. F., and Zydlewski, J. D. (2019). A dam passage performance standard model for American shad. Can. J. Fish. Aquat. Sci. 76, 762–779. doi: 10.1139/cjfas-2018-0008
Stier, D. J., and Crance, J. H. (1985). Habitat Suitability Index Models and Instream Flow Suitability Curves: American Shad. Washington, DC: U.S. Fish and Wildlife Service.
Stokesbury, K. D., and Dadswell, M. J. (1991). Mortality of juvenile clupeids during passage through a tidal, low head hydroelectric turbine at Annaplis Royal, Nova Scotia. North Am. J. Fish. Manag. 11, 149–154. doi: 10.1577/1548-8675(1991)011<0149:mojcdp>2.3.co;2
Sullivan, T. (2004). Evaluation of the Turners Falls Fishway Complex and Potential Improvements for Passing American Shad. Master’s Thesis. Boston, MA: University of Massachusetts.
Sweet, W. V., and Geratz, J. W. (2003). Bankfull Hydraulic Geometry Relationships and Recurrence Intervals for North Carolina’s Coastal Plain. J. Am. Water Resour. Assoc. 39, 861–871. doi: 10.1111/j.1752-1688.2003.tb04411.x
Talbot, G. B. (1954). Factors associated with fluctuations in abundance of Hudson River shad. U.S. Fish Wildlife Serv. Fish. Bull. 56, 373–413.
Taylor, A. (1995). William Cooper’s Town: Power and Persuasion on the Frontier of the Early American Republic. New York, NY: A. A. Knopf.
Then, A. Y., Hoenig, J. M., Hall, N. G., and Hewitt, D. A. (2015). Evaluating the predictive performance of empirical estimators of natural mortality rate using information on over 200 fish species. ICES J. Mar. Sci. 72, 82–92. doi: 10.1093/icesjms/fsu136
Tommasi, D., Nye, J., Stock, C., Hare, J. A., Alexander, M., and Drew, K. (2015). Effect of environmental conditions on juvenile recruitment of alewife (Alosa pseudoharengus) and blueback herring (Alosa aestivalis) in fresh water: a coastwide perspective. Can. J. Fish. Aquat. Sci. 72, 1037–1047. doi: 10.1139/cjfas-2014-0259
TransCanada. (2016). ILP Study 22 Downstream migration of juvenile American shad at Vernon. Study report. Submitted to FERC 2016. Prepared by Normandeau Associates, NH.
Trinko Lake, T. R., Ravana, K. R., and Saunders, R. (2012). Evaluating changes in diadromous species distributions and habitat accessibility following the Penobscot River Restoration Project. Mar. Coast. Fish. 4, 284–293. doi: 10.1080/19425120.2012.675971
Twining, C. W., West, D. C., and Post, D. M. (2013). Historical changes in nutrient inputs from humans and anadromous fishes in New England’s coastal watersheds. Limnol. Oceanogr. 58, 1286–1300. doi: 10.4319/lo.2013.58.4.1286
USGS (2019). National Hydrography Dataset (n.d.) Plus, Version 2. U.S. Environmental Protection Agency and U.S. Geological Survey. Reston, VA: USGS.
Vanni, M. J. (2002). Nutrient cycling by animals in freshwater ecosystems. Annu. Rev. Ecol. Evol. Syst. 33, 341–370. doi: 10.1146/annurev.ecolsys.33.010802.150519
Venables, W. N., and Ripley, B. D. (2002). “Random and mixed effects,” in Modern Applied Statistics With S. Statistics and Computing, (New York, NY: Springer).
Vogel, S., and Jansujwicz, J. (2021). Fish passage is an upstream battle during FERC relicensing in Maine. Fish. Manag. Ecol. doi: 10.1111/fme.12513
von Bertalanffy, L. (1938). A quantitative theory of organic growth (inquiries on growth laws II). Hum. Biol. 10, 181–213.
Walburg, C. H., and Nichols, P. R. (1967). Biology and Management of the American Shad and Status of the Fisheries, Atlantic Coast of the United States, 1960. U.S. Fish and Wildlife Service Special Scientific Report–Fisheries 550. Washington, DC: NOAA.
Walter, J. F. III, and Olney, J. E. (2003). Feeding behavior of American shad during spawning migration in the York River, Virginia. Am. Fish. Soc. Symp. 35, 201–209.
Walters, A. W., Barnes, R. T., and Post, D. M. (2009). Anadromous Alewives (Alosa pseudoharengus) contribute marine-derived nutrients to coastal stream food webs. Can. J. Fish. Aquat. Sci. 66, 439–448. doi: 10.1139/F09-008
Walters, C., and Korman, J. (1999). Linking recruitment to trophic factors: revisiting the Beverton-Holt recruitment model from a life history and multispecies perspective. Rev. Fish Biol. Fish. 9, 187–202. doi: 10.1023/A:1008991021305
Watson, J. M., Coghlan, S. M. Jr., Zydlewski, J., Hayes, D. B., and Kiraly, I. A. (2018). Dam removal and fish passage improvement influence fish assemblages in the Penobscot River, Maine. Trans. Am. Fish. Soc. 147, 525–540. doi: 10.1002/tafs.10053
Weaver, C. R., Thompson, C. S., and Ossiander, F. J. (1972). Evaluation of Fish Passage in the Vertical Slot Regulating Section of the South Shore Ladder at the John Day Dam. National Marine Fisheries Service, Final Report to the Army Corps. Of Engingeers. Portland: Portland District.
Weaver, D. M., Brown, M., and Zydlewski, J. D. (2019). Observations of American Shad Alosa sapidissima approaching and using a vertical slot fishway at the head-of-tide Brunswick Dam on the Androscoggin River, Maine. North Am. J. Fisher. Manag. 39, 989–998.
Weaver, D. M., Coghlan, S. M. Jr., Greig, H. S., Klemmer, A. J., Perkins, L. B., and Zydlewski, J. (2018). Subsidies from anadromous sea lamprey (Petromyzon marinus) carcasses function as a reciprocal nutrient exchange between marine and freshwaters. River Res. Applic. 34, 824–833. doi: 10.1002/rra.3291
Weaver, D. M., Coghlan, S. M. Jr., and Zydlewski, J. (2016). Sea lamprey carcasses exert local and variable food web effects in a nutrient-limited Atlantic coastal stream. Can. J. Fish. Aquat. Sci. 73, 1616–1625. doi: 10.1139/cjfas-2015-0506
Weaver, D. M., Coghlan, S. M., Zydlewski, J., Hogg, R. S., and Canton, M. (2015). Decomposition of sea lamprey Petromyzon marinus carcasses: temperature effects, nutrient dynamics, and implications for stream food webs. Hydrobiologia 760, 57–67. doi: 10.1007/s10750-015-2302-5
West, D. C., Walters, A. W., Gephard, S., and Post, D. M. (2010). Nutrient loading by anadromous Alewife (Alosa pseudoharengus): contemporary patterns and predictions for restoration efforts. Can. J. Fish. Aquat. Sci. 67, 1211–1220. doi: 10.1139/F10-059
Wilcove, D. S. (2010). No Way Home: The Decline of the World’s Great Animal Migrations. Washington, DC: Island Press.
Williams, R. O., and Bruger, G. E. (1972). Investigations on American Shad in the St. Johns River. Florida Department of Natural Resources, Marine Research Laboratory, Technical Series, Vol. 66, 1–49.
Williams, R. R., and Daborn, G. R. (1984). Spawning of the American shad (Alosa sapidissima) in the Annapolis River, Nova Scotia. Proc. Nova Scotian Instit. Sci. 34, 9–14.
Willson, M. F., and Halupka, K. C. (1995). Anadromous fish as keystone species in vertebrate communities. Conserv. Biol. 9, 489–497. doi: 10.1046/j.1523-1739.1995.09030489.x
Willson, M. F., and Womble, J. N. (2006). Vertebrate exploitation of pulsed marine prey: a review and the example of spawning herring (2006). Rev. Fish. Biol. Fish. 16, 183–200. doi: 10.1007/s11160-006-9009-7
Wippelhauser, G. (2021). Recovery of diadromous fishes: a kennebec river case study. Trans. Am. Fish. Soc. 150, 277–290. doi: 10.1002/tafs.10292
Zydlewski, J., and McCormick, S. D. (1997b). The loss of hyperosmoregulatory ability in migrating juvenile American shad, Alosa sapidissima. Can. J. Fish. Aquat. Sci. 54, 2377–2387. doi: 10.1139/f97-144
Zydlewski, J., and McCormick, S. D. (1997a). The ontogeny of salinity tolerance in the American shad, Alosa sapidissima. Can. J. Fish. Aquat. Sci. 54, 182–189. doi: 10.1139/f96-251
Zydlewski, J., McCormick, S. D., and Kunkel, J. G. (2003). Late migration and seawater entry is physiologically disadvantageous for American shad juveniles. J. Fish Biol. 63, 1521–1537. doi: 10.1111/j.1095-8649.2003.00264.x
Keywords: American shad, Alosa sapidissima, diadromous fish, migration, dam, fish passage, marine derived nutrients
Citation: Zydlewski J, Stich DS, Roy S, Bailey M, Sheehan T and Sprankle K (2021) What Have We Lost? Modeling Dam Impacts on American Shad Populations Through Their Native Range. Front. Mar. Sci. 8:734213. doi: 10.3389/fmars.2021.734213
Received: 30 June 2021; Accepted: 20 September 2021;
Published: 25 October 2021.
Edited by:
Mark J. Henderson, United States Geological Survey, United StatesReviewed by:
Joshua Raabe, University of Wisconsin–Stevens Point, United StatesAndrew M. Fischer, University of Tasmania, Australia
Copyright © 2021 Zydlewski, Stich, Roy, Bailey, Sheehan and Sprankle. This is an open-access article distributed under the terms of the Creative Commons Attribution License (CC BY). The use, distribution or reproduction in other forums is permitted, provided the original author(s) and the copyright owner(s) are credited and that the original publication in this journal is cited, in accordance with accepted academic practice. No use, distribution or reproduction is permitted which does not comply with these terms.
*Correspondence: Joseph Zydlewski, am9zZXBoekBtYWluZS5lZHU=