- National Centre for Polar and Ocean Research (NCPOR), Ministry of Earth Sciences, Vasco da Gama, India
High saline and cold Antarctic Bottom Water (AABW) forms around the continental margin of Antarctica that ventilates into the global ocean. To study the recent changes in AABW, we have analyzed the in situ observations collected from Indian Ocean expeditions to the Southern Ocean during 2010, 2011, 2017, 2018, and 2020. A comprehensive analysis of these observations indicated recent freshening, warming, and contraction in the layer thickness of the AABW. Even though the AABW depicted inter-annual variability, it changed to moderately fresher and lighter water mass at the end of the recent decade. The characteristics of AABW exhibited a contraction in its layer thickness (∼50–120 m) during recent years. The water mass showed its freshening (∼0.002) and warming (∼0.04°C) tendency from 2018 to 2020. The recent warming (∼0.3°C) of Circumpolar Deep Water (CDW) near the Prydz Bay suggests enhanced melting of ice shelves. It is hypothesized that the combined influences of onshore intrusion of warm CDW, upper ocean warming, sea ice decline, wind forcing, polynya, and calving events possibly caused the freshening and reduction in the thickness of AABW. The continued changes in the ocean-atmospheric environmental conditions and the subsequent changes in the bottom water characteristics likely influence the global climate, overturning circulation, and the biogeochemical cycle.
Introduction
The Antarctic Bottom Water (AABW) forms in the Southern Ocean which is the coldest and high saline water mass that travels throughout the global ocean. Characterization of the AABW is not always consistently followed considering the variety of its source region. The major known source regions of AABW are the Weddell Sea, the Cape Darnley polynya, the Ross Sea, and the Adélie Coast (Rintoul, 2007; Ohshima et al., 2013). The physical characteristics of AABW vary according to their formation region. The bottom water in the Ross Sea is characterized by a potential temperature (θ) from −0.3 to −0.6°C and salinity of more than 34.72. The coldest and freshest variety of bottom water forms along the Weddell Sea (Orsi et al., 1999). The type of AABW along the Adélie coast is characterized by low saline-cold water with high oxygen (van Wijk and Rintoul, 2014). The bottom water observed in the Australian-Antarctic Basin (AAB) and the Princess Elizabeth Trough (PET) is sourced and advected from its eastern region (Rintoul, 2007; van Wijk and Rintoul, 2014). The advection of high dense bottom water occurs by the boundary currents from the Pacific to the Indian sector of the Southern Ocean (ISSO) (Orsi et al., 1999; Aoki et al., 2008; van Wijk and Rintoul, 2014). Advection of slightly less dense AABW with a neutral density of more than 28.27 kg m–3 also occurs from the western region that is sourced from the Weddell-Enderby Basin (Orsi et al., 1999).
Mixing of various water masses such as the dense shelf water (DSW) and Circumpolar Deep Water (CDW) influences the formation of AABW (Orsi et al., 2002). The dominant production of AABW occurs in the coastal polynyas through increased sea-ice production and salt ejection associated with various dynamic and thermodynamic processes. For example, few of the known sources are reported in Cape Darnley, Adélie Land, and Vincennes Bay (Ohshima et al., 2013; Rintoul, 2013; Kitade et al., 2014). More recently, the formation of AABW in the Ross Sea has been linked to sea-ice changes through various processes associated with different modes of climatic variability such as Southern Annular Mode (SAM) and El Niño–Southern Oscillation (ENSO) (Silvano et al., 2020).
Many investigations revealed the asymmetrical modifications of AABW due to the influence of the formation of polynyas and enhanced melting in the glaciers. The variability in the fresh water budget along the Antarctica coast influences the formation of dense water and the signals get propagated into the deep ocean through ventilation of AABW (Rintoul, 2007). The global-scale contraction of AABW is reported by Purkey and Johnson (2012). The variations in the characteristics and volume of AABW are attributed to the freshening of source waters, not to the disparities in the formation rate alone (Johnson et al., 2014; van Wijk and Rintoul, 2014). A decline of AABW production in the Cape Darnley region due to enhanced ice shelf melting appears to be linked with global climate warming. Increased oceanic heat input shall lead to the irrevocable loss of ice sheets, intensified ocean/ice shelf interaction, and may further decrease the production of AABW in the future (Williams et al., 2016). Rapid collision of iceberg glaciers and associated calving events at the bottom water source regions may lead to enhanced freshening that results in the formation and spreading of fresh and less dense AABW (Menezes et al., 2017). Enhanced advection of CDW toward the ice shelf also leads to the decline of the shelf water density and subsequently influences the formation of AABW (Guo et al., 2019). The modifications of the AABW in the AAB in a decadal time scale showed asymmetrical variations (Aoki et al., 2020b).
In the present investigation, we focus on the repetitive profiling made at four stations between 55 and 64°S along the meridional transect of 57°30’E in the ISSO (Figure 1). The observed freshening and shrinkage of the AABW layer shall be linked to the alteration in characteristics or a variation in the formation rate of the source waters. Furthermore, we discussed the freshening and subduction of the AABW layer during the past decade. We linked these modifications of AABW with the shoaling and intrusion of CDW in the Prydz Bay region, variations in the wind, sea-ice, and calving events.
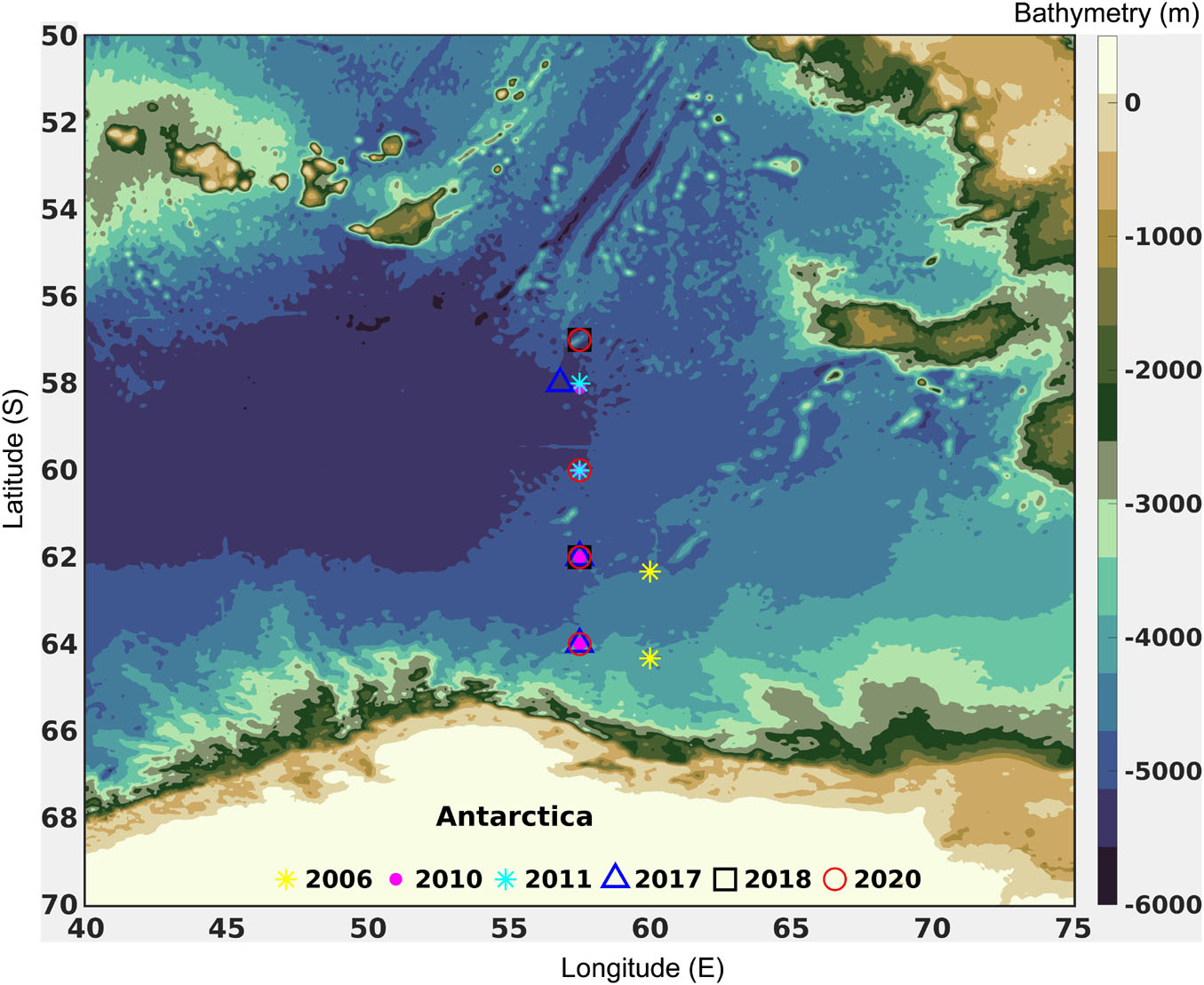
Figure 1. The Conductivity, Temperature, Depth (CTD) station locations for different years from 2006 to 2020 are overlaid on the ETOPO1 bathymetric map (Amante and Eakins, 2009).
Materials and Methods
Temperature and salinity data collected from the various Southern Ocean expeditions (SOE) during SOE 2010 (January 31, 2010, to March 1, 2010), SOE 2011 (February 1, 2011, to March 2, 2011), SOE 2017 (January 6 to February 28, 2017), SOE 2018 (December 8, 2017, to February 4, 2018), and SOE 2020 (January 22 to March 8, 2020) were used to characterize the AABW and its modification (Supplementary Table 1). Except for SOE 2010, the Conductivity, Temperature, Depth (CTD) instrument used during the cruises was SBE 911plus, with the temperature and conductivity accuracy of ± 0.001°C and ± 0.0001 Sm–1, respectively. During SOE 2010, a portable SBE 19plus CTD was used to collect the temperature and salinity data. The location and CTD model used during different cruises are given in Supplementary Table 1. The CTD data were processed using the standard protocol of manufacture supplied software downcast data. The temperature (ITS90) and salinity (PSS78) data from CTD were later converted, respectively, to conservative temperature (θ)-absolute salinity (SA) using the Gibbs-Sea-Water toolbox1. Field calibration of CTD salinity data was carried out against the laboratory determination of salinity using a salinometer (Guideline 8400B). We have used the underway conductivity temperature depth (UCTD) (Rudnick and Klinke, 2007) during December 2017 (referred to as austral summer 2018) and the expendable conductivity temperature depth (XCTD) during January 2017 for the collection of temperature and salinity along a section from 60 to 67°S between 70 and 74°E. The XCTD profiles were quality controlled by following the guidelines in the Uchida et al. (2011). The temperature accuracy of XCTD and UCTD probes is ± 0.02°C. We have used these probes in the shallow depth regions where the range of variability is much larger (>0.5°C) than the accuracy of these probes. Additionally, we have used World Ocean Circulation Experiment (WOCE) data at 62.33°S 60°E and 64.33°S 60°E from the WOCE S041 line 2006 for our study. The instrumented seal data set (Treasure et al., 2017) of the Marine Mammals from the shelf regions off Cape Darnley for the period 2015–2017 has been used to check the variability of the CDW in recent years.
The sea-ice concentration (SIC) and area from multiple passive microwave satellite observations were acquired from the National Snow and Ice Data Center (NSIDC) (data ID G0192, version 3). The brightness temperature data for different microwave bands were converted to SIC using the National Aeronautics and Space Administration (NASA) Team algorithm with a spatial resolution of a 25 km grid. The satellite sensor characteristics, sea-ice retrieval, and validation of passive microwave sensors are well described (Fetterer et al., 2016). The time series of satellite-based sea-ice data used in this study spanned from 1979 to 2020. To understand the recent variability of AABW in the ISSO, we have considered ECMWF ERA-5 based sea-ice and wind observations in and around the polynyas located along the Weddell Sea, ISSO, Western Pacific, and the Ross Sea. The polynya locations were identified from sea-ice maps and the nomenclatures have been referred to using earlier studies (Nihashi and Ohshima, 2015; Jena et al., 2019). The different seasons are defined as follows: winter (June, July, and August) and summer (December, January, and February). The seasonal variations of sea-ice were computed by averaging the corresponding monthly mean data. The seasonal computation of the SAM index was carried out using the monthly mean observational SAM index (Marshall, 2003).
Results
Observations on Freshening and Warming of Antarctic Bottom Water
The present study provided new evidence showing that the AABW (SA > 34.80; −0.2 ≤ θ ≤−0.7) has become fresher and warmer during 2020 compared to 2017–18, along the meridional transect of 57°30’E in the ISSO. Figure 2 shows the changes in the θ-SA relationship at four locations. From 2006 to 2020, the maximum freshening occurred in 2010. Earlier studies showed the AABW became fresher (0.01) and warmer (∼0.1°C) from 2006 to 2010 (Anilkumar et al., 2015). Nevertheless from 2010 to 2018, AABW regained its salinity (Figure 2B). This is consistent with the observations by Aoki et al. (2020b) who reported reversal of freshening of AABW during 2018–19 in comparison with the previous decades. The observed freshening is pronounced at 80°E, in contrast to the increase in salinity to the east of 115°E (Aoki et al., 2020b). Furthermore from 2018 to 2020, the AABW lost its salinity by ∼0.002 and became warmer by ∼0.04°C suggesting re-freshening and warming from 2018 to 2020, though this freshening was not as intense as that in 2010.
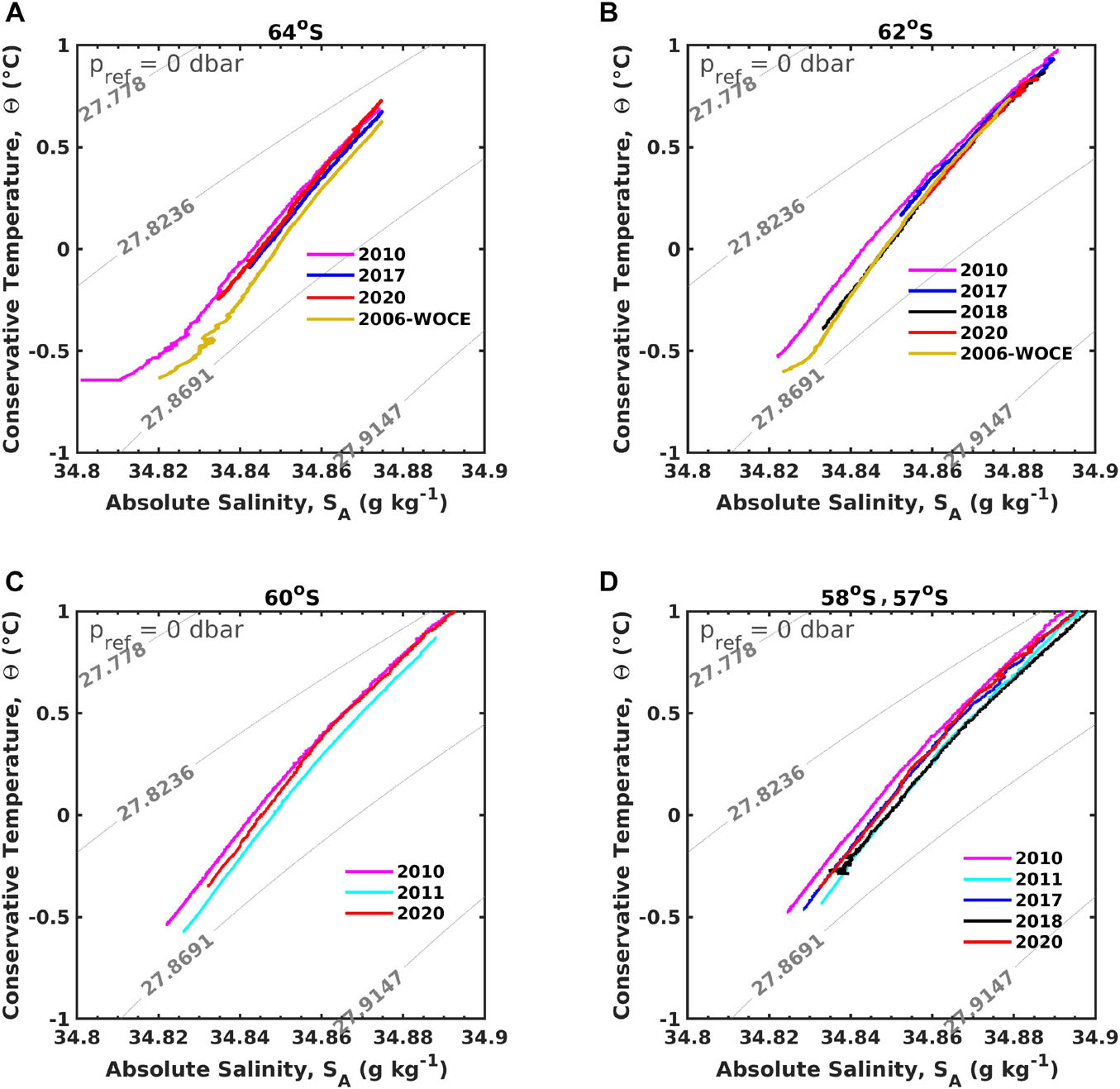
Figure 2. The conservative temperature (θ)-absolute salinity (SA) relationship at (A) 64°S, (B) 62°S, (C) 60°S, (D) 58°S, 57°S along ∼57.5°E. Refer to Supplementary Table 1 for the exact date and location of CTD stations.
The thickness of the AABW in the ISSO with the features, SA > 34.80; −0.2 ≤ θ ≤ −0.7, and yn > 28.27, is depicted in Figure 3A. At 64°S, an enhanced freshening was observed in 2010, however, the thickness of AABW is reduced by ∼50 m from 2010 to 2020. Similarly, a reduction in thickness (∼120 m) is observed at 58°S from 2010 to 2017. We note in this study that the observations for 2018 and 2020 are not available at this location. An exception was observed at 57°S, with a minimum thickness of AABW during 2018–2020. The inclusion of WOCE data into our analysis showed an enhanced reduction in thickness (∼120 m) from 2006 to 2020. However, the locations for WOCE are slightly different from the station locations of Indian expeditions to the Southern Ocean. Our results suggest that the freshening was higher during 2010 compared to 2020. However, a reduction in thickness was more pronounced in recent years consisting of heterogeneous variability at few locations (Figure 1). This indicates that the supply of AABW has been reduced in the study region during 2020 compared to 2010. The observed reduction of AABW is likely driven either by reduced supply or increased consumption, which can be outflow or mixing to lighter density water mass. We provided a detailed discussion on the source and production of AABW in the coastal waters of Antarctica.
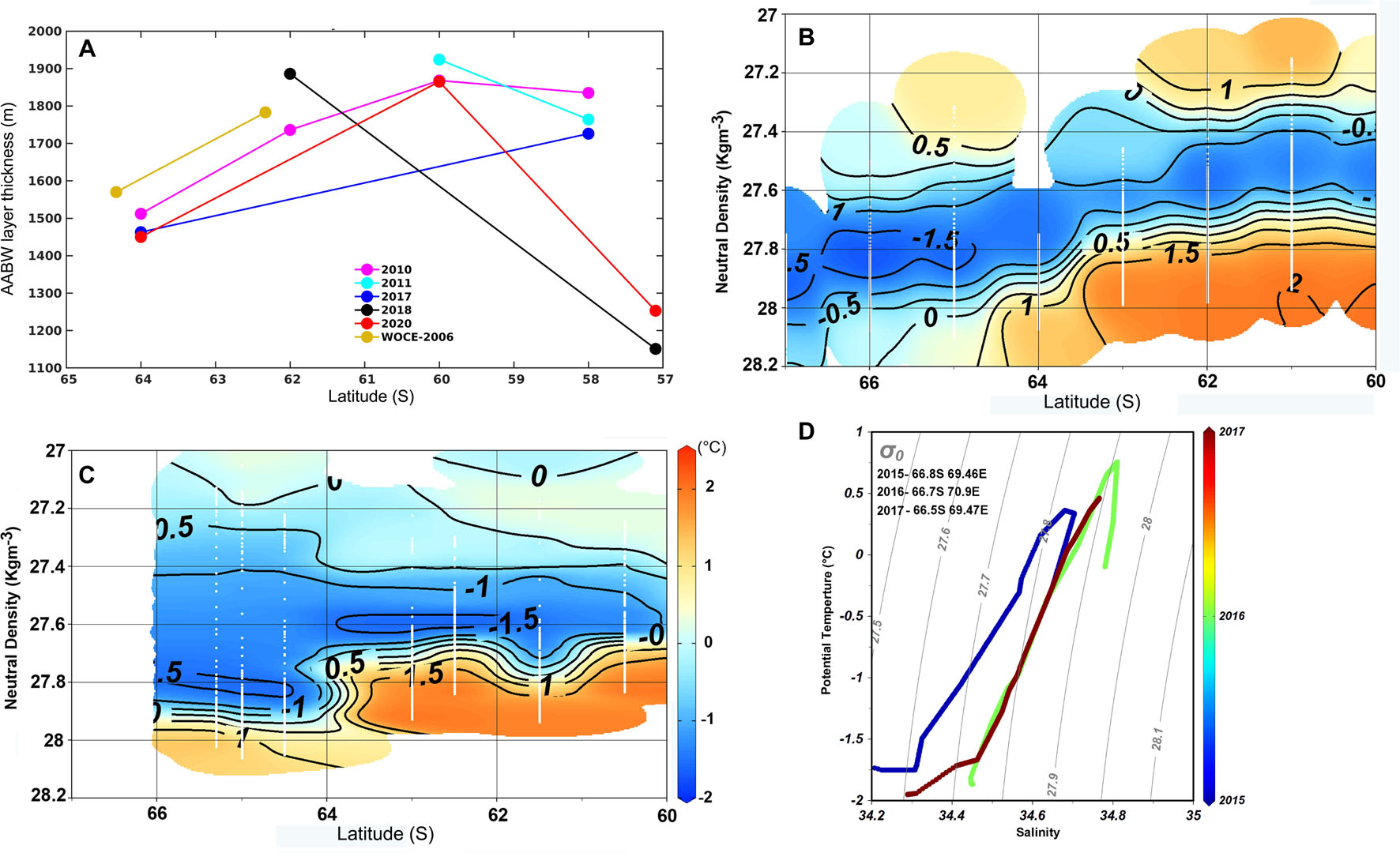
Figure 3. (A) The thickness of the Antarctic Bottom Water (AABW) in the Indian sector of the Southern Ocean (ISSO), (B,C) Vertical distribution of temperature along a meridional section (between 68 and 75°E) showing the propagation of warm Circumpolar Deep Water (CDW) toward the shelf region of the Prydz Bay during (B) 2017, (C) 2018 (Neutral density > 28.05 Kg m– 3 has used to demarcate the CDW) and (D) TS diagram depicts the CDW variability for the period 2015–2017 off Cape Darnley region from the seal data.
Causes for the Freshening of Antarctic Bottom Water
Role of Circumpolar Deep Water
We provided new evidence representing the contraction in the layer thickness of the AABW during 2020. In the Southern Ocean region, modifications of AABW due to the ice shelf melting and coastal polynya formation for more than a decade from the 1990s have been documented in the earlier literature (Jacobs, 2004; Rintoul, 2007; Johnson et al., 2008; Purkey and Johnson, 2012, 2013; Shimada et al., 2012; van Wijk and Rintoul, 2014; Aoki et al., 2020a, b). The CDW plays a key role in influencing the formation of AABW. For example, enhanced basal melting of the ice shelf was reported due to the intrusion of warm CDW that diminishes the buttressing and hastens glacier flow; in turn, freshens the bottom water (Rignot and Jacobs, 2002; Pritchard et al., 2012; Schmidtko et al., 2014). We analyzed the changes in the property indicators depicted by the CDW in the Prydz Bay region that encompasses Cape Darnley. The Cape Darnley region in Prydz Bay is one of the major sources for AABW near the present study area. The intrusion of warmer CDW across the continental shelf break is attributed to the embrace of the inflow of the Prydz Bay gyre to the eastern rim of the bay (Heil et al., 1996). The denser CDW was produced by the mixing of the salty-warm CDW, advecting on the shelf break, with cold Shelf Water (SW) over the continental shelf (Yabuki et al., 2006). The onshore intrusions of CDW could considerably augment the basal melt rate of the ice shelf (Dansereau et al., 2013).
We characterized the CDW in the Prydz Bay based on the available hydrographic observations (Figure 3B). Our analysis indicated a warming of the CDW by ∼0.3°C during the recent years (Figures 3C,D). The intrusion of this warm CDW into the ice shelf may result in enhanced basal melting in the ice shelf. It has been reported that the Prydz Bay gyre encompassing within the Bay to the Antarctic convergence is connected from a southern boundary of Prydz Bay with a coastal current that intensifies (>1 m s–1) along the MacRobertson shelf, further a part of the current flows offshore at 63°E, while other branch flows toward the west (Nunes Vaz and Lennon, 1996). We suggest that the intrusion and shoaling of warm CDW in the Prydz Bay and the subsequent ice shelf melting likely play a role in the freshening trend and descent of AABW encountered in the present study region. The intrusion of CDW into the shelf break and its thermal structure may be generally characterized in two ways either upward sloping or downward sloping (Schmidtko et al., 2014). The weakening of easterly wind over the coastal Prydz Bay (Cape Darnley and Barrier polynyas) and all along the coast between 90 and 160°E, occurred during 2016–2020 could cause upward tilting CDW and improved onshore intrusions (Stewart and Thompson, 2012; Dutrieux et al., 2013; Figure 4 and Supplementary Figure 5). This may cause enhanced melting in the ice shelf and likely hinders the formation of a significant volume of AABW. It is known that the CDW is warming and shoaling all over the coastal regions of Antarctica. However, the strong warming and shoaling of CDW occurs where it has more access to the shelf break. Hence, in the areas where CDW slopes upward, shoaling over time may lead to warming of the bottom waters in the shelf region. However, in the regions where CDW experiences the downward slope constrains the shoaling of CDW toward the shelf break and controls the associated warming and subsequent melting of the ice shelf. This provides a shield from the offshore changes in CDW (Schmidtko et al., 2014). Nevertheless, the present observations nurture an apprehension in the stability of the bottom water temperature of the Prydz Bay region due to the intrusion of warm CDW. The Cape Darnley region is one of the origins of AABW as reported by Aoki et al. (2020a). The changes encountered with CDW in one of the regions near the continental shelf of Cape Darnley are shown in Figure 3D. A clear warming trend of CDW has been observed in this region from 2015 to 2017. This warm CDW may trigger the ice shelf melting. The significance of surface forcing on acquaintances between offshore and onshore properties has been emphasized by the current dynamical approaches. As reported elsewhere (Stewart and Thompson, 2012), the onshore movement of CDW and the specific slope of CDW toward the shelf break are controlled by polar easterlies. The thermal gradient athwart the continental shelf and beneath the ice shelf is modified by the offshore atmospheric anomalies (Dutrieux et al., 2013).
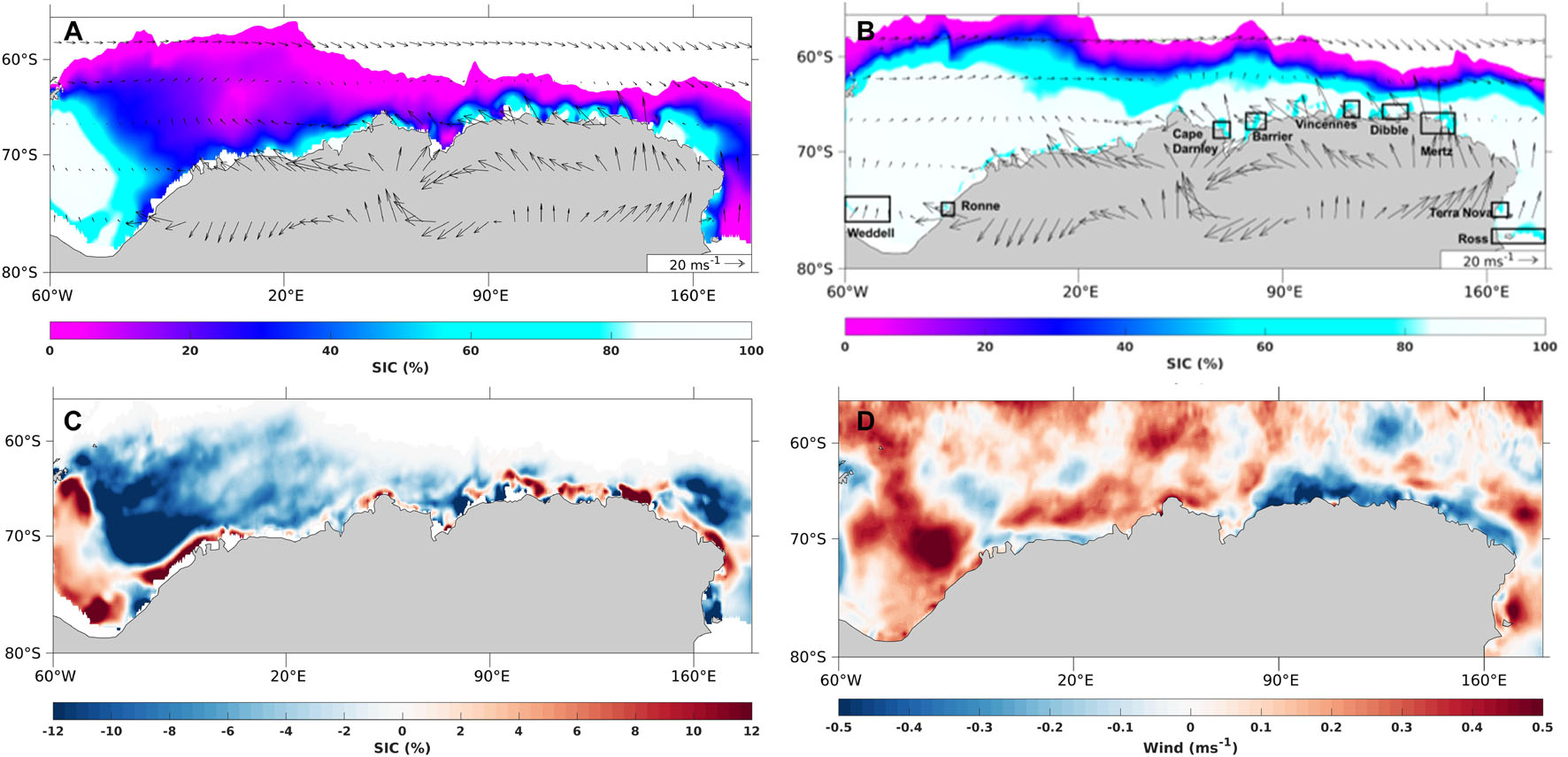
Figure 4. Climatological sea-ice concentration (SIC) (1979–2015) shows nine coastal polynyas with the prevailing pattern of south-easterly wind anomaly during (A) summer and (B) winter. (C) Summer sea-ice anomaly for 2016–2020. (D) Summer wind anomaly for 2016–2020. The anomalies were computed relative to a 37-year climatology (1979–2015).
Role of Wind and Sea-Ice Variability
The properties of AABW are primarily influenced by atmospheric forcing, sea-ice dynamics, freshwater inputs from shelf ice/continental discharge, and mixing with surrounding water masses. We have analyzed the variability of wind and sea-ice during summer and winter periods. This is because the ocean-atmospheric preconditioning in the polynya likely occurs in summer, followed by the formation of AABW in winter owing to salt ejected during sea-ice formation.
Analysis of sea-ice area in the entire Southern Ocean showed an overall expansion during summer (0.02 × 103 km2 yr–1, p < 0.05) and winter (0.03 × 103 km2 yr–1, p < 0.05) of 1979–2020 (Supplementary Figure 1). However, in the last decade from 2010 to 2020, the area declined at a significant rate (p < 0.05) of −0.26 × 103 km2 yr–1 and −0.29 × 103 km2 yr–1 during summer and winter, respectively. Spatial trend maps of SIC from 2010 to 2020 showed the declining trends (p < 0.05) for all the sectors of the Southern Ocean invariably during both seasons (Supplementary Figure 2). However, exceptions of increasing trends were observed along the coastal region of Amundsen–Bellingshausen Seas during summer and marginal ice zone of Weddell Sea during winter. There was an unprecedented decline in the sea-ice area with the record low values observed between 2016 and 2020, considering the entire time series of satellite data starting from 1979 (Turner et al., 2017; Jena and Pillai, 2020; Supplementary Figure 1). To understand its likely impact on AABW, we have analyzed the wind and SIC for nine major coastal polynyas along the Weddell Sea (Weddell and Ronne Polynya), ISSO (Cape Darnley and Barrier Polynya), Western Pacific (Vincennes, Dibble, and Mertz polynya), and Ross Sea (Terra Nova and Ross Polynya). The climatological maps (1979–2015) of SIC showed nine coastal polynyas with a prevailing pattern of south-easterly wind (Figures 4A,B). Wide-spread negative anomaly in SIC was a dominant feature during summer except for some regional heterogeneity of positive and negative anomaly along the coast (Figure 4C). The negative anomaly of sea-ice in summer influences the upper ocean thermal structure through radiative heating with enhanced shortwave radiation into the ocean. The upper ocean warming in summer plays an important role in the hindrance of sea-ice growth in the subsequent winter season that can influence the formation of AABW. Analysis of Argo data based upper ocean thermal structure in the Prydz Bay provided evidence of warming trend from 2006 through 2020 (Supplementary Figures 3, 4).
Our analysis on the wind during summer (2016–2020) indicated regional heterogeneity of positive and negative anomaly, with the dominant anomalous weakening of easterly wind along the coast between 90 and 160°E, corresponding to an overall positive phase of SAM (Figure 4D and Supplementary Figure 5A). The positive phase of SAM plays a key role with the poleward movement of antarctic circumpolar current (ACC) which leads to warmer temperature results in increased glacier melting. Hence, the positive phase of SAM in summer was allied to the slowdown of AABW formation (Purkey and Johnson, 2012). The time series analysis of wind for all polynya regions also showed an anomalous weakening of easterly wind except for Ronne and Ross Ice shelf Polynya (Supplementary Figure 5). The weakening of easterly wind has been identified as an important factor for upper ocean warming and enhanced intrusion of warm CDW toward the Antarctic ice shelves (Dinniman et al., 2012; Schmidtko et al., 2014). Reduction of winter sea-ice production was also reported in a polynya in the Prydz Bay due to intrusion of warmer CDW onto the continental shelf that minimized the formation of AABW (Guo et al., 2019). The process of heat entrainment to the surface occurred through deep convection in winter. Subsequently, the freshwater discharge from the enhanced melting of ice shelves induced by wind anomalies and ocean warming associated with climate modes likely influenced the freshening of AABW.
Discussion
Freshening (∼0.002) and warming (∼0.04°C) were shown by AABW toward the end of the last decade in the ISSO. In this study, we encompass the modifications of AABW off the Enderby basin to the last decade and consider changes in temperature, salinity, and AABW layer thickness. By using measurements from the same location and season, we minimize the discrepancies in spatial and seasonal variability. The previous study over the same region reported a fresher (∼0.01) and warmer (0.05°C) AABW in 2010 compared to 2006 (Anilkumar et al., 2015). However, the latest observations during 2020 exhibit moderate modifications in the properties of AABW compared to the previous decade in the ISSO. The earlier studies in the AAB reported that the cause for the freshening of bottom waters in this basin may be due to the freshening of the source of the dense water (van Wijk and Rintoul, 2014). The prevalent warming, contraction of bottom waters and variations in the mixing as well as stratification detected downstream are attributed to the freshening of the sources (Purkey and Johnson, 2010; Shimada et al., 2012). During the last decade, we have observed a 50 to 120 m reduction in the layer thickness of AABW (Figure 3A). A descending rate concurrent to a previous study by Purkey and Johnson (2012) that showed a decrease of 81 m per decade for AABW with θ ≤ 0°C, was divulged in the present study.
Instead of a decline in the formation rate of Weddell Sea Bottom Water (WSBW), the robust incursion of older Warm Deep Water rather was reported by Heuzé et al. (2013). Between 1984 and 2008, the reduction in the area of cross-section of the WSBW at the Prime Meridian caused variations in the Weddell gyre wind-driven circulation instead of its formation rate (Fahrbach et al., 2011; van Wijk and Rintoul, 2014). These studies have suggested that in the AAB, the variations observed along the AABW export pathway are attributed to the formation of lighter varieties of source waters and the contraction of AABW is compensated by expansion of lower and upper CDW and surface waters. Another reason for the observed freshening of AABW could be due to the anomalous decline in sea-ice cover during recent years. Silvano et al. (2020) have reported the regaining of the properties in the salinity, density, and thickness of AABW in the Ross Sea in 2018–2019, similar to those observed in the 1990s, due to the sea ice formation caused by the rare unification of SAM and El Niño conditions between 2015 and 2018. This favors the formation of AABW.
One of the major events we would like to discuss in this study might have an influence on the modification of AABW observed during the present study. For example, the calving event at the Mertz Glacier Tongue (MGT) during January-February 2010, influenced the AABW formation in coastal polynyas over the Mertz Depression and Adélie Depression located east and west of the MGT (Williams et al., 2010). This reduced sea-ice production up to 20% and the outflow of DSWs to nearly 23% (Kusahara et al., 2011; Tamura et al., 2012). The MGT calving resulted in a decrease in salinity (∼0.15) of DSWs from 2008 to 2012. This high rate of freshening is compatible with 50 years of freshening observed in the Ross Sea (Shadwick et al., 2013). The effect of the MGT calving event through various oceanic processes influences the AABW on the decadal time scale (Kusahara et al., 2011).
The time span required for AABW to get transported from its formation area to the present study region is unknown. The speed of the mean abyssal currents carrying the freshening signal would be higher than 2 cm s–1 as reported in earlier studies (Menezes et al., 2017). Strong boundary current transporting AABW westward was reported in this region (Orsi et al., 1999). The MGT calving event occurred in 2010, however, the freshening signal was observed during 2020 in the present study region (Figure 2). Considering the distance between Adélie coast (146°E; 67°S) and 64°S 57.5°E, the required traveling time of AABW was computed. We suggest a meager probability to link the MGT calving in 2010 with the freshening observed during 2020. Due to this event, unusual hastening in bottom water freshening was reported during 2016 (Menezes et al., 2017).
Williams et al. (2016) and Silvano et al. (2018) have reported the freshening of AABW due to the influence of ice shelf melting. Kusahara et al. (2011) has suggested that the effect of the calving event at the MGT influences the AABW on a decadal time scale through various oceanic processes. However, our study area is away from the abovementioned regions, and hence, actual cause for the changes observed for AABW shall be difficult to be traced. Hence, we suggest that the influence of the AABW formed in the Cape Darnley and the calving event at MGT might have also triggered the changes of AABW observed in the present study region. Nevertheless, Aoki et al. (2020a) have reported that the recently revealed bottom water off Cape Darnley is transporting an enhanced quantity of freshwater and feeding the surplus freshwater into the abyssal Weddell Sea. This suggests that the bottom water originated from Cape Darnley has influenced the changes experienced at the Weddell Sea. Hence, one of the causes for the changes observed with the AABW in our study area could be due to the influence of the AABW transported from the Cape Darnley region. Furthermore from 1974 to 2016, freshening of the bottom water closer to the present study region (65°S 61°E; 64°S 59°E and 58°S 53E) were also reported by the previous investigators (Aoki et al., 2020a).
We reported upward tilting of CDW and its onshore intrusions in the Prydz Bay, causing the freshening and subduction of AABW, possibly triggered by the weakening of easterly winds between 2016 and 2020. Our findings on modifications of AABW properties due to the penetration of warm CDW into the Prydz Bay region are supported by the results from earlier studies (Rintoul, 2007; Purkey and Johnson, 2013). The Cape Darnley polynya was identified as one of the origins of AABW contributing around 13–30% of bottom water for the Atlantic sector (Williams et al., 2016; Aoki et al., 2020a). Vibrant warming of CDW was encountered in the Cape Darnley region as portrayed in Figure 3D. This transportation of AABW occurs through the Indian sector, hence we suggest that one of the sources for the modifications of AABW observed in the present study region is likely from Cape Darnley origin.
Conclusion
Our study reveals that the AABW became fresher (∼0.002), warmer (0.04°C), and subducted by ∼50–120 m toward the end of the past decade in the ISSO. Various reasons discussed for the freshening and subduction of AABW are the upward tilting of CDW and its onshore intrusions in the Prydz Bay, possibly induced by the weakening of easterly winds between 2016 and 2020. The CDW experienced warming of ∼0.3°C and its onshore intrusion likely enhances melting of the ice shelves. Freshwater discharge from the enhanced melting of ice shelves induced by the onshore intrusion of warm CDW, ocean warming, and calving events likely influenced the freshening and contraction of AABW. This result postulates that more observations are required for a comprehensive understanding of the modifications of AABW properties. The latest initiation such as Deep Argo technology has facilitated bottom water sampling at a higher temporal resolution (Kobayashi, 2018). The amalgamations of sustained Eulerian and Lagrangian data collections, as well as modeling studies, are the more reliable methods for illustrating the long-term differential modifications of the AABW.
Data Availability Statement
The in situ data collected during the Indian Scientific Expeditions to the Southern Ocean are available at https://data.mendeley.com/datasets/vtm8wh3933/1. Mendeley Data, V1, doi: 10.17632/vtm8wh3933.1.
Author Contributions
NA formulated the idea and led the study and wrote the manuscript. All other authors contributed to the writing, review, and editing of the manuscripts and carried out data analysis. All authors contributed to the article and approved the submitted version.
Funding
This research was supported by the Ministry of Earth Sciences and NCPOR (Government of India).
Conflict of Interest
The authors declare that the research was conducted in the absence of any commercial or financial relationships that could be construed as a potential conflict of interest.
Publisher’s Note
All claims expressed in this article are solely those of the authors and do not necessarily represent those of their affiliated organizations, or those of the publisher, the editors and the reviewers. Any product that may be evaluated in this article, or claim that may be made by its manufacturer, is not guaranteed or endorsed by the publisher.
Acknowledgments
We acknowledge the Ministry of Earth Sciences (MoES) for all the support extended for undertaking this study. We acknowledge Aswathi, Junior Research Fellow, NCPOR for processing the seal data. We acknowledge organizations such as the National Snow and Ice Data Center (NSIDC), National Oceanic and Atmospheric Administration (NOAA), European Centre for Medium Range Weather Forecast (ECMWF), National Aeronautics and Space Administration (NASA), and their data processing teams for providing the dataset. This is NCPOR contribution number: J-51/2021-22.
Supplementary Material
The Supplementary Material for this article can be found online at: https://www.frontiersin.org/articles/10.3389/fmars.2021.730630/full#supplementary-material
Supplementary Figure 1 | Inter-annual variability of sea-ice area (× 106 km2) and SAM index during (A) summer and (B) winter for the Southern Ocean from 1979 to 2020.
Supplementary Figure 2 | The trend of sea-ice concentration (SIC) (% year–1) in the entire Southern Ocean during the last decade from 2010 to 2020 for (A) summer and (B) winter.
Supplementary Figure 3 | Depth-latitude cross-section of potential temperature from marine Argo atlas for February (A) 2006, (B) 2017, (C) 2018, and (D) 2020 at 71.7°E transect near the Prydz Bay. The data are available at https://argo.ucsd.edu/data/data-visualizations/marine-atlas/.
Supplementary Figure 4 | Variability of potential temperature during summer (February) from 2006 to 2020 showed recent upper ocean warming in the Prydz Bay at 71.7°E and 64°S.
Supplementary Figure 5 | Time series of Southern Annular Mode (SAM) index, SIC, and wind anomaly for nine major coastal polynyas along the Weddell Sea (Weddell and Ronne Polynya), Indian Ocean sector of the Southern Ocean (Cape Darnley and Barrier Polynya), Western Pacific (Vincennes, Dibble, and Mertz polynya), and Ross Sea (Terra Nova and Ross Polynya).
Supplementary Table 1 | The date and locations of Conductivity, Temperature, Depth (CTD) stations occupied during different cruises.
Footnotes
References
Amante, C., and Eakins, B. W. (2009). ETOPO1 1 arc-minute global relief model: procedures, data sources and analysis. NOAA Tech. Memo. NESDIS NGDC 24:19. doi: 10.1594/PANGAEA.769615
Anilkumar, N., Chacko, R., Sabu, P., and George, J. V. (2015). Freshening of Antarctic bottom water in the Indian Ocean sector of Southern Ocean. Deep Sea Res. 2 Top. Stud. Oceanogr. 118, 162–169. doi: 10.1016/j.dsr2.2015.03.009
Aoki, S., Fujii, N., Ushio, S., Yoshikawa, Y., Watanabe, S., Mizuta, G., et al. (2008). Deep western boundary current and southern frontal systems of the Antarctic Circumpolar Current southeast of the Kerguelen Plateau. J. Geophys. Res. Oceans 113, 1–14. doi: 10.1029/2007JC004627
Aoki, S., Yamazaki, K., Hirano, D., Katsumata, K., Shimada, K., Kitade, Y., et al. (2020b). Reversal of freshening trend of Antarctic bottom water in the Australian-Antarctic Basin during 2010s. Sci. Rep. 10:14415. doi: 10.1038/s41598-020-71290-6
Aoki, S., Katsumata, K., Hamaguchi, M., Noda, A., Kitade, Y., Shimada, K., et al. (2020a). Freshening of Antarctic bottom water off Cape Darnley, East Antarctica. J. Geophys. Res. Oceans 125:e2020JC016374. doi: 10.1029/2020JC016374
Dansereau, V., Heimback, P., and Losch, M. (2013). Simulation of subice shelf melt rates in a general circulation model: velocity-dependent transfer and the role of friction. J. Geophys. Res. Oceans 119, 1765–90.
Dinniman, M. S., Klinck, J. M., and Hofmann, E. E. (2012). Sensitivity of circumpolar deep water transport and ice shelf basal melt along the west antarctic peninsula to changes in the winds. J. Clim. 25, 4799–4816. doi: 10.1175/JCLI-D-11-00307.1
Dutrieux, A. P., De Rydt, J., Jenkins, A., Holland, P. R., and Ha, H. K. (2013). Strong sensitivity of Pine Island ice shelf melting to climatic variability. Science 343, 174–8.
Fahrbach, E., Hoppema, M., Rohardt, G., Boebel, O., Klatt, O., and Wisotzki, A. (2011). Warming of deep and abyssal water masses along the Greenwich meridian on decadal time scales: the Weddell gyre as a heat buffer. Deep Sea Res. 2 Top. Stud. Oceanogr. 58, 2509–2523. doi: 10.1016/j.dsr2.2011.06.007
Fetterer, F., Knowles, K., Meier, W., and Savoie, M. (2016). Sea Ice Index, Version 2 (updated daily). Boulder: National Snow and Ice Data Center.
Guo, G., Shi, J., Gao, L., Tamura, T., and Williams, G. D. (2019). Reduced sea ice production due to upwelled oceanic heat flux in Prydz Bay, East Antarctica. Geophys. Res. Lett. 46, 4782–4789. doi: 10.1029/2018GL081463
Heil, P., Allison, I., and Lytle, V. (1996). Seasonal and interannual variations of the oceanic heat flux under a landfast Antarctic sea ice cover. J. Geophys. Res. 101, 25741–25752.
Heuzé, C., Heywood, K. J., Stevens, D. P., and Ridley, J. K. (2013). Southern Ocean bottom water characteristics in CMIP5 models. Geophys. Res. Lett. 40, 1409–1414. doi: 10.1002/grl.50287
Jacobs, S. S. (2004). Bottom water production and its links with the thermohaline circulation. Antarct. Sci. 16, 427–437. doi: 10.1017/S095410200400224X
Jena, B., and Pillai, A. N. (2020). Satellite observations of unprecedented phytoplankton blooms in the Maud Rise polynya, Southern Ocean. Cryosphere 14, 1385–1398. doi: 10.5194/tc-14-1385-2020
Jena, B., Ravichandran, M., and Turner, J. (2019). Recent reoccurrence of large open-ocean polynya on the Maud Rise Seamount. Geophys. Res. Lett. 46, 4320–4329. doi: 10.1029/2018GL081482
Johnson, G. C., McTaggart, K. E., and Wanninkhof, R. (2014). Antarctic Bottom Water temperature changes in the westernSouth Atlantic from 1989 to 2014. J. Geophys. Res. Oceans 119, 8567–8577. doi: 10.1002/2014JC010367
Johnson, G. C., Purkey, S. G., and Bullister, J. L. (2008). Warming and freshening in the abyssal southeastern Indian Ocean. J. Clim. 21, 5351–5363. doi: 10.1175/2008JCLI2384.1
Kitade, Y., Shimada, K., Tamura, T., Williams, G. D., Aoki, S., Fukamachi, Y., et al. (2014). Antarctic bottom water production from the Vincennes Bay Polynya, East Antarctica. Geophys. Res. Lett. 41, 3528–3534. doi: 10.1002/2014GL059971
Kobayashi, T. (2018). Rapid volume reduction in Antarctic Bottom Water off the Adélie/George V Land coast observed by deep floats. Deep Sea Res. I Oceanogr. Res. Pap. 140, 95–117. doi: 10.1016/j.dsr.2018.07.014
Kusahara, K., Hasumi, H., and Williams, G. D. (2011). Impact of the Mertz Glacier Tongue calving on dense water formation and export. Nat. Commun. 2:159. doi: 10.1038/ncomms1156
Marshall, G. J. (2003). Trends in the Southern Annular Mode from observations and reanalyses. J. Clim. 16, 4134–4143. doi: 10.1175/1520-04422003016
Menezes, V. V., Macdonald, A. M., and Schatzman, C. (2017). Accelerated freshening of Antarctic Bottom Water over the last decade in the Southern Indian Ocean. Sci. Adv. 3, 1–10. doi: 10.1126/sciadv.1601426
Nihashi, S., and Ohshima, K. I. (2015). Circumpolar mapping of antarctic coastal polynyas and landfast sea ice: relationship and variability. J. Clim. 28, 3650–3670. doi: 10.1175/JCLI-D-14-00369.1
Nunes Vaz, R. A., and Lennon, G. W. (1996). Physical oceanography of the Prydz Bay region of Antarctic waters. Deep Sea Res. I Oceanogr. Res. Pap. 43, 603–641. doi: 10.1016/0967-0637(96)00028-3
Ohshima, K. I., Fukamachi, Y., Williams, G. D., Nihashi, S., Roquet, F., Kitade, Y., et al. (2013). Antarctic Bottom Water production by intense sea-ice formation in the Cape Darnley polynya. Nat. Geosci. 6, 235–240. doi: 10.1038/ngeo1738
Orsi, A. H., Johnson, G. C., and Bullister, J. L. (1999). Circulation, mixing, and production of Antarctic Bottom Water. Progr. Oceanogr. 43, 55–109. doi: 10.1016/S0079-6611(99)00004-X
Orsi, A. H., Smethie, W. M., and Bullister, J. L. (2002). On the total input of Antarctic waters to the deep ocean: a preliminary estimate from chlorofluorocarbon measurements. J. Geophys. Res. Oceans 107:3122. doi: 10.1029/2001jc000976
Pritchard, H. D., Ligtenberg, S. R. M., Fricker, H. A., Vaughan, D. G., Van Den Broeke, M. R., and Padman, L. (2012). Antarctic ice-sheet loss driven by basal melting of ice shelves. Nature 484, 502–505. doi: 10.1038/nature10968
Purkey, S. G., and Johnson, G. C. (2010). Warming of global abyssal and deep Southern Ocean waters between the 1990s and 2000s: contributions to global heat and sea level rise budgets. J. Clim. 23, 6336–6351. doi: 10.1175/2010JCLI3682.1
Purkey, S. G., and Johnson, G. C. (2012). Global contraction of Antarctic Bottom Water between the 1980s and 2000s. J. Clim. 25, 5830–5844. doi: 10.1175/JCLI-D-11-00612.1
Purkey, S. G., and Johnson, G. C. (2013). Antarctic bottom water warming and freshening: contributions to sea level rise, ocean freshwater budgets, and global heat gain. J. Clim. 26, 6105–6122. doi: 10.1175/JCLI-D-12-00834.1
Rignot, E., and Jacobs, S. S. (2002). Rapid bottom melting widespread near antarctic ice sheet grounding lines. Science 296, 2020–2023. doi: 10.1126/science.1070942
Rintoul, S. R. (2007). Rapid freshening of Antarctic Bottom Water formed in the Indian and Pacific oceans. Geophys. Res. Lett. 34:L06606. doi: 10.1029/2006GL028550
Rintoul, S. R. (2013). On the origin and influence of Adélie land bottom water. Antarct. Res. Ser. 75, 151–171. doi: 10.1029/ar075p0151
Rudnick, D. L., and Klinke, J. (2007). The underway conductivity-temperature-depth instrument. J. Atmos. Ocean. Technol. 24, 1910–1923. doi: 10.1175/JTECH2100.1
Schmidtko, S., Heywood, K. J., Thompson, A. F., and Aoki, S. (2014). Multidecadal warming of Antarctic waters. Science 346, 1227–1231. doi: 10.1126/science.1256117
Shadwick, E. H., Rintoul, S. R., Tilbrook, B., Williams, G. D., Young, N., Fraser, A. D., et al. (2013). Glacier tongue calving reduced dense water formation and enhanced carbon uptake. Geophys. Res. Lett. 40, 904–909. doi: 10.1002/grl.50178
Shimada, K., Aoki, S., Ohshima, K. I., and Rintoul, S. R. (2012). Influence of Ross Sea Bottom Water changes on the warming and freshening of the Antarctic Bottom Water in the Australian-Antarctic Basin. Ocean Sci. 8, 419–432. doi: 10.5194/os-8-419-2012
Silvano, A., Rintoul, S. R., Peña-Molino, B., Hobbs, W. R., van Wijk, E., Aoki, S., et al. (2018). Freshening by glacial meltwater enhances melting of ice shelves and reduces formation of antarctic bottom water. Sci. Adv. 4. doi: 10.1126/sciadv.aap9467
Silvano, A., Foppert, A., Rintoul, S. R., Holland, P. R., Tamura, T., Kimura, N., et al. (2020). Recent recovery of Antarctic Bottom Water formation in the Ross Sea driven by climate anomalies. Nat. Geosci. 13, 780–786. doi: 10.1038/s41561-020-00655-3
Stewart, A. L., and Thompson, A. F. (2012). Sensitivity of the ocean’s deep overturning circulation to easterly Antarctic winds. Geophys. Res. Lett. 39:L18604. doi: 10.1029/2012GL053099
Tamura, T., Williams, G. D., Fraser, A. D., and Ohshima, K. I. (2012). Potential regime shift in decreased sea ice production after the Mertz Glacier calving. Nat. Commun. 3:826. doi: 10.1038/ncomms1820
Treasure, A. M., Roquet, F., Ansorge, I. J., Bester, M. N., Boehme, L., Bornemann, H., et al. (2017). Marine mammals exploring the oceans pole to pole: a review of the MEOP consortium. Oceanography 30, 132–138.
Turner, J., Phillips, T., Marshall, G. J., Hosking, J. S., Pope, J. O., Bracegirdle, T. J., et al. (2017). Unprecedented springtime retreat of Antarctic sea ice in 2016. Geophys. Res. Lett. 44, 6868–6875. doi: 10.1002/2017GL073656
Uchida, H., Shimada, K., and Kawano, T. (2011). A method for data processing to obtain high-quality XCTD data. J. Atmos. Ocean. Technol. 28, 816–826. doi: 10.1175/2011JTECHO795.1
van Wijk, E. M., and Rintoul, S. R. (2014). Freshening drives contraction of Antarctic Bottom Water in the Australian Antarctic Basin. Geophys. Res. Lett. 41, 1657–1664. doi: 10.1002/2013GL058921
Williams, G. D., Aoki, S., Jacobs, S. S., Rintoul, S. R., Tamura, T., and Bindoff, N. L. (2010). Antarctic bottom water from the adélie and George v Land Coast, East Antarctica (140-149°E). J. Geophys. Res. Oceans 115, 1–29. doi: 10.1029/2009JC005812
Williams, G. D., Herraiz-Borreguero, L., Roquet, F., Tamura, T., Ohshima, K. I., Fukamachi, Y., et al. (2016). The suppression of Antarctic bottom water formation by melting ice shelves in Prydz Bay. Nat. Commun. 7:12577. doi: 10.1038/ncomms12577
Keywords: Antarctic Bottom Water (AABW), freshening, contraction, Circumpolar Deep Water (CDW), sea ice
Citation: Anilkumar N, Jena B, George JV, P S, S K and Ravichandran M (2021) Recent Freshening, Warming, and Contraction of the Antarctic Bottom Water in the Indian Sector of the Southern Ocean. Front. Mar. Sci. 8:730630. doi: 10.3389/fmars.2021.730630
Received: 25 June 2021; Accepted: 01 October 2021;
Published: 18 November 2021.
Edited by:
Gilles Reverdin, Centre National de la Recherche Scientifique (CNRS), FranceReviewed by:
Ebenezer Nyadjro, Mississippi State University, United StatesD. Swain, Indian Institute of Technology Bhubaneswar, India
Copyright © 2021 Anilkumar, Jena, George, P, S and Ravichandran. This is an open-access article distributed under the terms of the Creative Commons Attribution License (CC BY). The use, distribution or reproduction in other forums is permitted, provided the original author(s) and the copyright owner(s) are credited and that the original publication in this journal is cited, in accordance with accepted academic practice. No use, distribution or reproduction is permitted which does not comply with these terms.
*Correspondence: N Anilkumar, YW5pbEBuY3Bvci5yZXMuaW4=