- 1Instituto de Estudos do Mar Almirante Paulo Moreira, Arraial do Cabo, Brazil
- 2Departamento de Biologia Marinha, Instituto de Biologia, Universidade Federal Fluminense (UFF), Rio de Janeiro, Brazil
- 3Departamento de Química Analítica, Universidade Federal Fluminense, Rio de Janeiro, Brazil
- 4Instituto de Botânica, Núcleo de Pesquisa em Ficologia, São Paulo, Brazil
Introduction
Phenotypic plasticity is an adaptive mechanism used by different organisms to cope with spatial and temporal variation in the environment (Murren et al., 2015). It can be defined as the set of responses of a single genotype to different environments (Padilla and Savedo, 2013), or as the ability of a given specimen to modify its phenotype in response to changes in environmental conditions (Kelly et al., 2012). Phenotypic plasticity is recognized as an evolutionary strategy to potentially adapt to a wide range of environmental conditions (Whitman and Agrawal, 2009).
In general, phenotypic plasticity is an expression of physiological conditions, and it is well-documented in terrestrial systems (Whitman and Agrawal, 2009). It can encompass a variety of manifestations induced by environmental conditions, such as morphological (Stewart, 2006), physiological (Padilla-Gamiño et al., 2016), behavioral (Snell-Rood, 2013), and phenological (Lanna et al., 2012) differences, among others, either permanent or not, and throughout life. In the marine environment, morphological plasticity is considered more frequent in invertebrates, whereas chemical plasticity occurs more frequently in seaweeds (Padilla and Savedo, 2013). There is also broad evidence for morphological and physiological plasticity in macroalgal species (Flukes et al., 2015).
Inducible chemical defense is a common and pronounced type of phenotypic plasticity expression in marine macroalgae, which appears to be promoted by herbivore pressure on the brown macroalga Fucus vesiculosus (Peckol et al., 1996) or the presence of epibionts on the red macroalga Laurencia dendroidea (Pereira et al., 2017). However, a decrease in chemical defense levels and the consequent increase in susceptibility to herbivory can also occur in the brown macroalgae Ascophyllum nodosum under ultraviolet-B radiation (UVBR) light (Pavia and Brock, 2000) and increased salinity (Pedersen, 1984), in L. dendroidea at a higher temperature and salinity (Sudatti et al., 2011), and Padina gymnospora subjected to high desiccation (Renaud et al., 1990). Furthermore, some authors have suggested that quantitative variation in chemical defense against herbivory can be driven by genetic differences between specimens of the red macroalga Delisea pulchra (Wright et al., 2000), with genetic control determining chemotypes or chemical races in specimens of the red macroalga Laurencia nipponica (Abe et al., 1999). Reproduction experiments in D. pulchra have demonstrated the influence of heritability in the variation of chemical defense production (Wright et al., 2004).
Nonetheless, how genetic and environmental factors interact to drive phenotypic plasticity of defensive chemicals in marine macroalgae remains unclear. For example, there is a significant correlation between genotype and concentrations of furanones, a heterocyclic organic compound found in the red seaweed D. pulchra. This correlation supports the existence of a genetic basis, although the environment could also be partially responsible for this variation (Wright et al., 2004). Moreover, concentrations of phlorotannins (Jormalainen and Honkanen, 2004), fouling inhibition (Honkanen and Jormalainen, 2005), and susceptibility to herbivory (Jormalainen et al., 2008) in the brown macroalga F. vesiculosus have considerable variability of genetic origin.
Research that considers the influence of genetic and environmental factors on the variability of chemical defenses under controlled conditions might help to understand the abundance patterns of secondary metabolites in marine macroalgae. For example, controlled experiments could help clarify the effect of these factors on the phenotypic plasticity of a defensive chemical since the variation in the production of secondary metabolites could arise both from the genetic divergence between populations and from differential responses to environmental changes (e.g., Ballhorn et al., 2011). Thus, it is essential to keep organisms from different habitats in common garden conditions to reveal genetic importance for phenotypic expressions, since they would be in absence of environmental influences (Patterson et al., 2018).
The red macroalga L. dendroidea J. Agardh is distributed along the Brazilian coast, and it exhibits considerable phenotypic plasticity in terms of morphological, anatomical, and cytological responses to environmental conditions (Machín-Sáncheza et al., 2014), and qualitative (Machado et al., 2016) and quantitative variability (Oliveira et al., 2013) in the production of chemical defenses. For example, the sesquiterpene elatol is a common and often important metabolite in Brazilian populations of L. dendroidea and plays key ecological roles as a chemical defense against herbivory (Pereira et al., 2003) and fouling (Da Gama et al., 2002). However, elatol concentrations vary between different populations (Oliveira et al., 2013) and even between individuals of the same population (Sudatti et al., 2006). Temperature (Sudatti et al., 2011) and simulated herbivory (Sudatti et al., 2018) have been shown to affect chemical defenses in L. dendroidea and probably contribute to the variation in elatol levels. Still, hitherto no experiments have addressed this plasticity within and among populations. This study assessed the variability in elatol production of L. dendroidea specimens collected from four populations along the Brazilian coast (Southwestern Atlantic) using common garden-controlled conditions. First, we evaluated how chemical defense production can vary between individuals (intra and interpopulation), comparing elatol levels between field individuals and their clones kept under common garden experimental conditions (four specimens/genotypes per population). Second, we manipulated temperature conditions to verify the intrapopulation growth variability and chemical defense under temperature stress, promoting trade-offs in macroalgal metabolism.
Materials and Methods
Collection and Clonal Culture Conditions
To obtain unialgal and clonal laboratory cultures, we collected specimens from four populations of L. dendroidea along the Brazilian coast: Vilas do Atlântico Beach, Bahia state—BA (12°53′27″S, 38°17′02″'W); Castelhanos Beach, Espírito Santo state—ES (20°51′40″S, 40°37′00″W); Forno Inlet, Northern Rio de Janeiro state—NRJ (22°46′40″S, 41°52′57″W); and Velho Beach, Southern Rio de Janeiro state—SRJ (23°02′50″S, 44°23′52″W) in January (summer), 2008. Voucher specimens of L. dendroidea from the four populations were deposited in the herbarium of the Instituto de Botânica, São Paulo state, Brazil: SP399793, BA; SP400151, ES; SP399790, NRJ; and SP399792, SRJ.
We considered each collected specimen as an individual, that is, supposedly as a distinct genotype. We used the term clone to refer to all harvested macroalgal parts obtained from an L. dendroidea specimen to produce laboratory cultures.
Clonal propagation was performed from the apical segments (5–10 mm) obtained from specimens (n = 4) of each of the L. dendroidea populations. Thus, four distinct genotypes from each population were used to evaluate the response to the experimental conditions. Epiphytic organisms were removed from the surfaces of each segment with a soft brush followed by several washes in sterilized seawater and drying with absorbent paper. Apical segments were transferred to sterile glass vials (80 ml capacity) with sterile seawater enriched with 25% Provasoli solution [polyethersulfone (PES)/4; corresponding to one-fourth of the original PES formulation] and maintained at 22 (±2°C), 32 ± 1 practical salinity unit (PSU) and 60–80 μmol photons m−2 s−1 irradiance, under cool-white fluorescent lamps (14:10 h light: dark photoperiod), without aeration. Irradiance was measured with a quantum photometer (LI-250A; Li-COR, Lincoln, NE, USA) equipped with an underwater quantum sensor (LI-192 SA; Li-COR, Lincoln, NE, USA). Initial clones propagated in the present study were excised from the same corresponding specimens from the previous study (Oliveira et al., 2013), replicated in culture media, and renewed weekly to obtain a unialgal culture (free of micro and other macroalgae). When necessary, germanium dioxide (1 mg L−1) was used to suppress diatomaceous growth (Kawai et al., 2005). Clones from each specimen per population of L. dendroidea were kept separately under culture laboratory conditions.
Common Garden Experiment
After establishing unialgal cultures (ca. 8 months), the intra and interpopulation variability of elatol in response to common garden laboratory conditions were evaluated for 1 month in four genotypes of each population of L. dendroidea propagated as clones. A total of 16 clones (excised from four individuals of each collection site) with the same biomass (6.0 mg) were maintained under the same conditions described above for clonal culture. Every week, we replaced the culture medium and reoriented the positions of the vials containing the clones to avoid shadow or other location effects. The clones were then weighed and subjected to extraction in hexane [high-performance liquid chromatography (HPLC) grade, TEDIA Company Inc., Rio de Janeiro, Brazil] to measure the amount of elatol. To compare the chemical defense levels produced under common garden conditions and field-collected specimens, we used previous field data of elatol contents in L. dendroidea from the four collection sites (refer to Oliveira et al., 2013) that gave rise to the clonal culture propagated in the present study. The dry weight biomass data obtained from field specimens of L. dendroidea (Oliveira et al., 2013) were converted to wet weight for comparison with the elatol concentrations obtained of the L. dendroidea clones obtained in our experiments.
Phenotypic Response to Temperature
We experimentally changed the temperature in growth chambers to assess its impact (at inter and intrapopulation levels) on the growth of L. dendroidea specimens. We chose this abiotic factor because it alters growth and elatol levels in L. dendroidea (Sudatti et al., 2011). We assessed the optimal temperature for development and elatol production (25°C), and another temperature (15°C) that is known to promote physiological stress on L. dendroidea (Sudatti et al., 2011).
We measured and compared the physiological responses of primary (growth) and secondary (elatol levels) metabolism between the four L. dendroidea populations and genotypes. For each treatment, clones of L. dendroidea with equivalent biomass (6.0 mg) were incubated in sterile clear glass vials containing 60 ml of sterilized seawater enriched with PES/2 (corresponding to half of the original Provasoli—PES formulation). Culture medium exchange and biomass measurements (wet weight) were conducted weekly for 1 month. Biomass was measured initially (time week 0) and every week (1–4). Biomass was expressed as % variation according to [(Final–Initial Biomass) × 100/Final Biomass] and interpreted as seaweed growth. At the end of the experiment (week 4), the L. dendroidea clones from all treatments were subjected to exhaustive extraction in hexane (HPLC grade, TEDIA Company Inc., Rio de Janeiro, Brazil).
For each experimental condition (15 and 25°C), we maintained four genotypes of the sterile sporophyte lifecycle phase of L. dendroidea in triplicates (clones) for each studied population, totaling 48 experimental units for each temperature tested.
Elatol Extraction and Quantification
Crude extracts were obtained from laboratory genotypes of L. dendroidea assayed using 5 ml of hexane (HPLC grade, TEDIA, Rio de Janeiro, Brazil) for each 0.01 g of fresh algal biomass incubated for 1 day at room temperature. This procedure was repeated two times. In each extract, elatol quantification in each extract was performed by gas chromatography coupled to electron capture detector (GC-ECD, Chrompack, Middelburg, the Netherlands) using the external standardization method (Sudatti et al., 2006). Elatol was isolated by precoated preparative thin-layer chromatography (TLC) plates, detected by TLC, identified by 1H NMR (nuclear magnetic resonance), and the results were compared with data available in the literature (König and Wright, 1997). The GC-ECD (Chrompack, Middelburg, the Netherlands) was fitted with an RTX-5 capillary column (30.0 m × 0.32 mm, 5% phenyl, 95% dimethylpolysiloxane; Restek, State College, PA, USA). The oven temperature program was: 80°C (1 min), 10°C min−1 up to 300°C (for 8 min), and finally −15°C min−1 down to 80°C. Nitrogen (N2 99.999%; White Martins, Rio de Janeiro, Brazil) was used as the carrier gas (28 cm s−1), make-up (35 ml min−1), and purge (15 ml min−1). The detector temperature was 320°C. We used manual injection system and on-column mode with a 0.5-μl syringe (full-volume injection). Concentrations of elatol were expressed as mg of elatol per g of L. dendroidea wet weight.
Statistical Analyses
Variations in elatol concentrations in L. dendroidea specimens from the four populations under common experimental (present study) and field conditions (data from Oliveira et al., 2013) from populations were compared with a two-way ANOVA. Chemical defense phenotypic variations (i.e., elatol levels) in response to temperature, source locality, and genotype (locality) were evaluated using nested ANOVA. A nested ANOVA was also performed to evaluate the effects of temperature, source locality, and genotype on the growth of L. dendroidea. Differences were significant when p < 0.05 (α = 5%). For nested ANOVA, elatol and biomass data were log and logit transformed, respectively, in order to meet ANOVA assumptions. If appropriate, a posteriori tests were performed [the Student-Newman-Keuls test (SNK)].
Results
Laboratory Experiment
Common garden conditions promoted more than the 2-fold increase of elatol levels in the four L. dendroidea populations when compared to field-collected specimens of this red seaweed from the field (data from Oliveira et al., 2013) (ANOVA/SNK, p = 0.00015). Specimens from SRJ state population showed higher levels of elatol compared to the remaining three populations [Bahia (BA); Espírito Santo (ES) and Northern Rio de Janeiro (NRJ); Figure 1], both in specimens maintained under common garden (ANOVA/SNK, p < 0.0002) and specimens from field conditions (Oliveira et al., 2013). In contrast, elatol levels did not differ between the other populations of L. dendroidea (BA = ES = NRJ) kept either under the common garden or from the field conditions (Figure 1). Thus, the conditions and locality significantly affected the levels of chemical defense (elatol levels) in L. dendroidea (Table 1A).
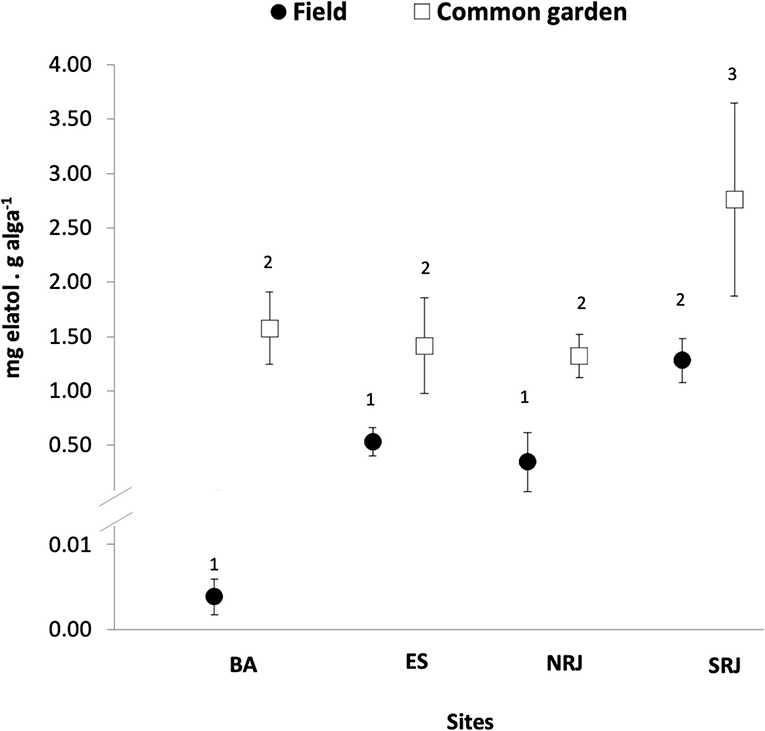
Figure 1. Elatol levels between Laurencia dendroidea populations were maintained under common garden conditions. Values are similar except for the SRJ population and are higher than field-collected specimens. Values are mean ± SD. BA, Bahia; ES, Espírito Santo; NRJ, Northern Rio de Janeiro; SRJ, Southern Rio de Janeiro. Experimental conditions with the same numbers are not significantly different (p < 0.05, ANOVA/SKN test, n = 4).
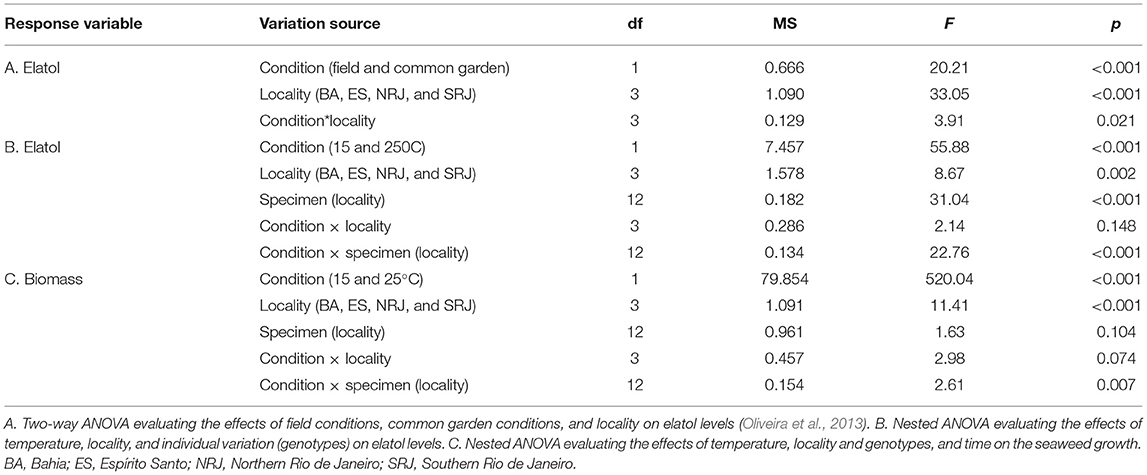
Table 1. ANOVA results were performed on data from experiments examining phenotypic responses of Laurencia dendroidea (=elatol production and biomass).
Temperature Effects on Chemical Defenses and Growth
Both temperatures (15°C and 25°C) influenced elatol concentrations. At 25°C, we observed a higher concentration of this chemical in genotypes/specimens from all populations (Figure 2A). However, locality and specimens also contributed to the observed variations, with evident interactions between these factors (Table 1B), as previously reported by Oliveira et al. (2013). The genotype influenced the elatol levels between populations and within the same population (intrapopulation variation), highlighting the ES specimens (4 ≠ 1 = 2 = 3) and SRJ (1 = 4 ≠ 2 = 3) specimens (Figure 2B; ANOVA/SKN, p < 0.01).
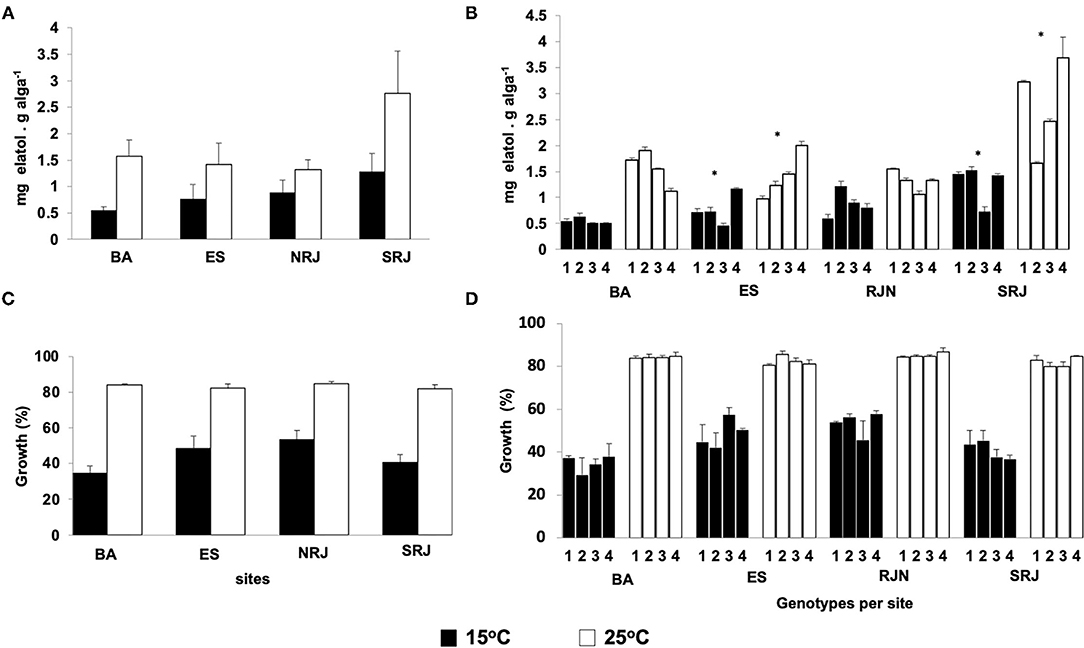
Figure 2. Effect of temperature on the chemical defense and growth of L. dendroidea specimens under laboratory-controlled conditions. At 25°C, elatol levels (A) and growth (C) were higher than at 15°C. Elatol levels differed between genotypes (n = 3) despite similar growth conditions for ES and SRJ populations (B). The mean growth (±SD) of each genotype (n = 3) was similar at 15 and 25°C (D). Numbers 1, 2, 3, and 4 correspond to the distinct genotypes of the unfertile sporophyte lifecycle phase. *Genotypes are significantly different. Sites and statistical notation as in Figure 1.
Biomass increased in response to temperature (15 and 25°C) throughout the experimental period and according to the locality, but not according to the specimen (Table 1C). As shown in Figure 2C, at week 4, we observed faster growth in specimens kept at a water temperature of 25°C (ca. 83% increase) than at 15°C (from 35 to 50% of the increase) (Figure 2C). Similar interpopulation growth was observed only between BA and SRJ (BA ≠ ES, RJN, and ES ≠ RJN ≠ RJS; Figure 2C; ANOVA/SKN, p < 0.01). All four specimens from the same population had similar growth patterns at 15°C and 25°C (Figure 2D).
Discussion
Contrary to our expectations, all individuals from the four populations of L. dendroidea showed higher levels of elatol under laboratory common garden conditions than the corresponding field-collected individuals. According to the literature, we expected a decrease in elatol levels under laboratory conditions due to the cultivation of L. dendroidea specimens in the absence of herbivores, fouling, and competitors, known as inducers of chemical defense in seaweeds (Paul and Van Alstyne, 1992; Pereira et al., 2017; Sudatti et al., 2018). However, elatol is a constitutive defense-type (Pereira et al., 2003), always present in the studied specimens of L. dendroidea.
The production of chemical defenses has a supposedly high metabolic cost, which would promote a trade-off between chemical precursors, substrates, and cofactors in the primary and secondary metabolism under an environmental gradient of abiotic stresses, competition, and predation pressures (Cronin, 2001). Greater allocation of resources for chemical defense would be presumable under intense herbivory pressure. In contrast, without herbivory risk, organisms would allocate energy toward further resource acquisition and growth, becoming superior competitors (Cronin, 2001). Both, the storage of auto-toxic substances in specialized structures (Salgado et al., 2008), or the storage of inactive biogenetic precursors that can be converted into active metabolites when appropriate Paul and Van Alstyne (1992) and the trade-off between chemical defense production and growth Pavia and Toth (1999) are evidence of seaweed chemical defense cost. Therefore, we can hypothesize that even in the absence of herbivory pressure and competition, but under optimal or non-stressful laboratory conditions (e.g., such as sufficient nutrient provision and light), there was an allocation of energy to produce elatol at the expense of other aspects such as growth itself. This small terpenoid seems to act as a constitutive defense, able to be induced by external signals, whose efficiency depends on dose and kind of ecological interaction (Sudatti et al., 2018; Sudattti et al., 2020).
Despite the decrease in environmental stress (nutrients uptake and herbivory), the inter and intrapopulation variation in elatol production observed under field conditions was also maintained in the laboratory, indicating that, in addition to environmental factors, intrinsic aspects (e.g., genetic control) act on the production of secondary metabolites. However, our results did not determine the regulatory mechanisms involved.
The four populations of L. dendroidea could be distinct considering that several seaweed species exhibit reduced gene flow (Santelices, 1990) and that Laurencia spores usually settle close to their parents (Abe et al., 1999). Furthermore, the distances between our L. dendroidea collection sites (ranging from ca. 300 to 1,800 km) are likely sufficient to facilitate differentiation between the four studied populations. Given the lack of information on the genetic structure and breeding experiments of populations to verify cross-generational inheritance of chemical defense, we can only suggest that the observed geographic interpopulation could be due to phenotypic plasticity or local adaptation and could be under genetic control. However, despite the geographic and biological characteristics of seaweeds, we can assume that the four studied populations of L. dendroidea maintain some level of genetic connectivity/reproductive compatibility and phenotypically appear to be members of the same species. Furthermore, the qualitative and quantitative variability of secondary metabolites previously found in six populations of L. dendroidea along the Southeast Brazilian coast is unequivocal evidence of the phenotypic plasticity in producing these chemicals by this species (refer to Machado et al., 2016).
In addition, each specimen (= genotype) showed a variable response in elatol production within the L. dendroidea population regardless if the clones were grown under optimal (25°C) or stressful (15°C) laboratory conditions. This result reinforces our assumption that since they were different genotypes, each specimen collected reacted in a particular way. In a previous study, we analyzed 70 field individuals of L. dendroidea (i.e., subjected to environmental factors) from Cabo Frio Island (North Rio de Janeiro) and found distinct elatol amounts (Sudatti et al., 2006). In agreement with this previous study, we also found intrapopulation variability in the ES and SRJ populations, although the sample size was small (n = 4 per population). Since the four individuals from each population of L. dendroidea produced different levels of elatol even when maintained under similar conditions in the laboratory, our data highlight that the genotype potentially controls the quantitative production of chemical defenses.
Mean growth was consistently higher in populations of L. dendroidea kept at 25°C than at 15°C. Still, in most instances, growth in the studied populations was similar over time and in the specimens maintained at either 15 or 25°C, indicating that growth is a less variable character than chemical defense. Indeed, most seaweeds can acclimate growth and photosynthesis in response to temperature variation (Eggert, 2012). An increase in temperature can accelerate photosynthesis and growth (Padilla-Gamiño and Carpenter, 2007), and nutrient uptake rates (Nishihara et al., 2005), justifying the faster growth at 25°C.
However, studies on phenotypic plasticity in marine organisms do not report the induction time for new phenotypes (Padilla and Savedo, 2013), making it difficult to ascertain whether certain traits have longer lag times than others or establish their real adaptive value. This study showed that individuals from four populations of L. dendroidea had similar growth but differed in elatol production during a 1-month experiment at the same temperature. This result indicates that changes in elatol production had a faster response than the biomass. Consequently, changes in elatol production seem to have a greater adaptive value for this seaweed species. Indeed, in the context of herbivory induction defense, elatol production can be rapidly deployed in 2 days (Sudatti et al., 2018). Given the broad defensive properties of elatol, individuals with higher elatol concentrations could have a selective advantage. Therefore, the SRJ population of L. dendroidea could show better fitness than the other populations studied. Further research covering genetic aspects could help elucidate if this variation results from a local adaptation. In this case, the SRJ population can be considered an ecotype, or simply the result of the phenotypic plasticity of the species.
Data from common garden experiments suggest the interaction between genes and environmental factors in modulating the production of secondary metabolites, resulting in wide variation in chemical defense levels observed in natural habitats. Changes in seaweed chemical traits are often attributed to herbivore induction, while abiotic factors are associated with changes in invertebrates (Padilla and Savedo, 2013). In a previous study, we also described great variability in the elatol levels in 70 specimens from a single population (Sudatti et al., 2006). Since then, we have performed some studies to help elucidate if this variability is associated with abiotic factors (Sudatti et al., 2011), herbivory (Sudatti et al., 2018), and epibiosis (Paradas et al., 2010; Sudatti et al., 2018). This article analyzed the transplantation potential of individuals from different populations in a common indoor garden design. We conclude that chemical defense is a more plastic trait than growth since populations and some genotypes differed in elatol production but not in biomass gain. However, further studies should also consider some additional factors, such as the cultivation of L. dendroidea under a broader temperature range. We also highlight the importance of considering clonal replication of different individuals from natural populations or a higher number of replicates in studies of seaweed chemical ecology since the intrapopulation variation is quite broad and can mask the effect of a variable response. In an ideal scenario, a suitable number of replicates obtained from diverse populations could reach a representative variation both within and among populations and avoid misinterpretation.
Finally, differences in elatol concentrations between and within populations are essential aspects that shape chemically mediated interactions and presumably affect seaweed fitness. Further research on the genetic structure of populations and breeding experiments to verify transgenerational inheritance are essential to confirm the influence of genotypes on elatol production and could help to understand/interpreting the variability in secondary metabolite production in seaweeds.
Data Availability Statement
The raw data supporting the conclusions of this article will be made available by the authors, without undue reservation.
Author Contributions
DS, AO, MF, and RP conceived and designed the experiments. DS and AO performed the experiments. SR, DS, and AO conducted chemical analysis. DS, AO, BG, and RP analyzed the data and wrote the manuscript. All authors contributed to the article and approved the submitted version.
Conflict of Interest
The authors declare that the research was conducted in the absence of any commercial or financial relationships that could be construed as a potential conflict of interest.
Publisher's Note
All claims expressed in this article are solely those of the authors and do not necessarily represent those of their affiliated organizations, or those of the publisher, the editors and the reviewers. Any product that may be evaluated in this article, or claim that may be made by its manufacturer, is not guaranteed or endorsed by the publisher.
Acknowledgments
We are grateful to the Conselho Nacional de Desenvolvimento Científico e Tecnológico (CNPq, 310464/2016-1) and the Fundação de Amparo á Pesquisa do Estado do Rio de Janeiro (FAPERJ, E-26/202.689/2019) for financial support. RP and MF thank CNPq for their Research Productivity Fellowship.
References
Abe, T., Masuda, M., Suzuki, T., and Suzuki, M. (1999). Chemical races in the red alga Laurencia nipponica (Rhodomelaceae, Ceramiales). Phycol. Res. 47, 87–95. doi: 10.1111/j.1440-1835.1999.tb00288.x
Ballhorn, D. J., Kautz, S, Jensen, M., Schmitt, I., Heil, M., and Heheman, A. D. (2011). Genetic and environmental interactions determine plant defences against herbivores. J. Ecol. 99, 313–326. doi: 10.1111/j.1365-2745.2010.01747.x
Cronin, G. (2001). “Resource allocation in seaweeds and marine invertebrates: chemical defense patterns in relation to defense theories,” in Marine Chemical Ecology, eds J. B. McClintock and B. J. Baker (Boca Raton, FL: CRC Press). doi: 10.1201/9781420036602.ch9
Da Gama, B. A. P., Pereira, R. C., Carvalho, A. G. V., Coutinho, R., and Yoneshigue-Valentin, Y. (2002). The effects of seaweed secondary metabolites on biofouling. Biofouling 18, 13–20. doi: 10.1080/08927010290017680
Eggert, A. (2012). “Seaweed responses to temperature,” in Seaweed Biology: Novel Insights Into Ecophysiology, Ecology and Utilization, eds C. Wiencke and K. Bischof (New York, NY: Springer). doi: 10.1007/978-3-642-28451-9_3
Flukes, E. B., Wright, J. T., and Johnson, C. R. (2015). Phenotypic plasticity and biogeographic variation in physiology of habitat-forming seaweed: response to temperature and nitrate. J. Phycol. 51, 896–909. doi: 10.1111/jpy.12330
Honkanen, T., and Jormalainen, V. (2005). Genotypic variation in tolerance and resistance to fouling in the brown alga Fucus vesiculosus. Oecologia 144, 196–205. doi: 10.1007/s00442-005-0053-0
Jormalainen, V., and Honkanen, T. (2004). Variation in natural selection for growth and phlorotannins in the brown alga Fucus vesiculosus. J. Evolut. Biol. 17, 807–820. doi: 10.1111/j.1420-9101.2004.00715.x
Jormalainen, V., Wikström, S. A., and Honkanen, T. (2008). Fouling mediates grazing: intertwining of resistances to multiple enemies in the brown alga Fucus vesiculosus. Oecologia 155, 559–569. doi: 10.1007/s00442-007-0939-0
Kawai, H., Motomura, T., and Okuda, K. (2005). “Isolation and purification techniques for macroalgae,” in Algal Culturing Techniques, ed R. A. Andersen (London: Academic Press). doi: 10.1016/B978-012088426-1/50010-X
Kelly, S. A., Panhuis, T. M., and Stoehr, A. M. (2012). Phenotypic plasticity: molecular mechanisms and adaptive significance. Compr. Physiol. 2, 1417–1439. doi: 10.1002/cphy.c110008
König, G. M., and Wright, A. D. (1997). Laurencia rigida: chemical investigations of its antifouling dichloromethane extract. J. Nat. Prod. 60, 967–970. doi: 10.1021/np970181r
Lanna, K. L., Connana, S., and Stiger-Pouvreau, V. (2012). Phenology, TPC and size-fractioning phenolics variability in temperate Sargassaceae (Phaeophyceae, Fucales) from Western Brittany: Native versus introduced species. Mar. Environ. Res. 80, 1–11. doi: 10.1016/j.marenvres.2012.05.011
Machado, F. L. S., Duarte, H. M., Gestinari, L. M. S., Cassano, V., Kaiser, C. R., and Soares, A. R. (2016). Geographic distribution of natural products produced by the red alga Laurencia dendroidea. J. Agardh. Chem. Biodiv. 13, 845–851. doi: 10.1002/cbdv.201500246
Machín-Sáncheza, M., Gall, L. L., Neto, A. I., Rousseau, F., Cassano, V., Sentíes, A., et al. (2014). A combined barcode and morphological approach to the systematics and biogeography of Laurencia pyramidalis and Laurenciella marilzae (Rhodophyta). Eur. J. Phycol. 49, 115–127. doi: 10.1080/09670262.2014.893017
Murren, C. J., Auld, J. R., Callahan, H., Ghalambor, C. K., Handelsman, C. A., Heskel, M. A., et al. (2015). Constraints on the evolution of phenotypic plasticity: limits and costs of phenotype and plasticity. Heredity 15, 293–301. doi: 10.1038/hdy.2015.8
Nishihara, G. N., Terada, R., and Noro, T. (2005). Effect of temperature and irradiance on the uptake of ammonium and nitrate by Laurencia brongniartii (Rhodophyta, Ceramiales). J Appl Phycol 17, 371–377. doi: 10.1007/s10811-005-5519-2
Oliveira, A. S., Sudatti, D. B., Fujii, M. T., Rodrigues, S. V., and Pereira, R. C. (2013). Inter- and intrapopulation variation in the defensive chemistry of the red seaweed Laurencia dendroidea (Ceramiales, Rhodophyta). Phycologia 52, 130–136. doi: 10.2216/12-058.1
Padilla, D. K., and Savedo, M. M. (2013). A systematic review of phenotypic plasticity in marine invertebrate and plant systems. Adv. Mar. Biol. 65, 67–94. doi: 10.1016/B978-0-12-410498-3.00002-1
Padilla-Gamiño, J. L., and Carpenter, R. C. (2007). Thermal ecophysiology of Laurencia pacifica and Laurencia nidifica (Ceramiales, Rhodophyta) from tropical and warm-temperate regions. J. Phycol. 43, 686–692. doi: 10.1111/j.1529-8817.2007.00362.x
Padilla-Gamiño, J. L., Gaitán-Espitia, J. D., Kelly, M. W., and Hofmann, G. E. (2016). Physiological plasticity and local adaptation to elevated pCO2 in calcareous algae: an ontogenetic and geographic approach. Evol. Appl. 9, 1043–1053. doi: 10.1111/eva.12411
Paradas, W. C., Salgado, L. T., Sudatti, D. B., Crapez, M. A., Fuji, M. T., et al. (2010). Induction of halogenated vesicle transport in cells of the red seaweed Laurencia obtusa. Biofouling 26, 277–286. doi: 10.1080/08927010903515122
Patterson, A., Flores-Renttería, L., Wipple, A., Whitham, A. T., and Gehring, C. (2018). Common garden experiments disentangle plant genetic and environmental contributions to ectomycorrhizal fungal community structure. New Phytol. 221, 493–502. doi: 10.1111/nph.15352
Paul, V. J., and Van Alstyne, K. (1992). Activation of chemical defense in the tropical green algae Halimeda spp. J. Exp. Mar. Biol. Ecol. 160:191–203. doi: 10.1016/0022-0981(92)90237-5
Pavia, H., and Brock, E. (2000). Extrinsic factors influencing phlorotannin production in the brown alga Ascophyllum nodosum. Mar. Ecol. Prog. Ser. 193, 285–294. doi: 10.3354/meps193285
Pavia, H., Toth, G., and Aberg, P. A. (1999). Trade-offs between phlorotannin production and annual growth in natural populations of the brown seaweed Ascophyllum nodosum. J. Ecol. 87:761–771.
Peckol, P., Krane, J. M., and Yates, J. L. (1996). Interactive effects of inducible defense and resource availability on phlorotannins in the north Atlantic brown alga Fucus vesiculosus. Mar. Ecol. Prog. Ser. 138, 209–217. doi: 10.3354/meps138209
Pedersen, A. (1984). Studies on phenol content and heavy metal uptake in fucoids. Hydrobiologia 116, 498–504. doi: 10.1007/978-94-009-6560-7_101
Pereira, R. C., Costa, E. S., Sudatti, D. B., and Da Gama, B. A. P. (2017). Inducible defenses against herbivory and fouling in seaweeds. J. Sea Res. 122, 25–33. doi: 10.1016/j.seares.2017.03.002
Pereira, R. C., da Gama, B. A. P., Teixeira, V. L., and Yoneshigue-Valentin, Y. (2003). Ecological roles of natural products from the Brazilian red seaweed Laurencia obtusa. Braz. J. Biol. 63, 665–672. doi: 10.1590/S1519-69842003000400013
Renaud, P. E., Hay, M. E., and Schmitt, T. M. (1990). Interactions of plant stress and herbivory: intraspecific variation in the susceptibility of a palatable versus an unpalatable seaweed to sea urchin grazing. Oecologia 82, 217–226. doi: 10.1007/BF00323538
Salgado, L. T., Viana, N. B., Andrade, L. R., Leal, R. N., Da Gama, B. A. P., et al. (2008). Intra-cellular storage, transport and exocytosis of halogenated compounds in marine red alga Laurencia obtusa. J. Struct. Biol. 162, 345–355. doi: 10.1016/j.jsb.2008.01.015
Santelices, B. (1990). Patterns of reproduction, dispersal and recruitment in seaweeds. Oceanogr. Mar. Biol. Ann. Rev. 28, 177–276.
Snell-Rood, E. C. (2013). An overview of the evolutionary causes and consequences of behavioural plasticity. Anim. Behav. 85, 1004–1011. doi: 10.1016/j.anbehav.2012.12.031
Stewart, H. L. (2006). Morphological variation and phenotypic plasticity of buoyancy in the macroalga Turbinaria ornata across a barrier reef. Mar. Biol. 149, 721–730. doi: 10.1007/s00227-005-0186-z
Sudatti, D. B., Fujii, M. T., Rodrigues, S. V., Turra, A., and Pereira, R. C. (2011). Effects of abiotic factors on growth and chemical defenses in cultivated clones of Laurencia dendroidea. J. Agardh. Mar. Biol. 158, 1439–1446. doi: 10.1007/s00227-011-1660-4
Sudatti, D. B., Fujii, M. T., Rodrigues, S. V., Turra, A., and Pereira, R. C. (2018). Prompt induction of chemical defenses in the red seaweed Laurencia dendroidea: the role of herbivory and epibiosis. J. Sea Res. 138, 48–55. doi: 10.1016/j.seares.2018.04.007
Sudatti, D. B., Rodrigues, S. V., and Pereira, R. C. (2006). Quantitative GC-ECD analysis of halogenated metabolites: determination of surface and within-thallus elatol of Laurencia obtusa. J. Chem. Ecol. 23, 835–843. doi: 10.1007/s10886-006-9033-z
Sudattti, D. B., Duarte, H. M., Soares, A. R., Salgado, L. T., and Pereira, R. C. (2020). New ecological role of seaweed secondary metabolites as autotoxic and allelopathic. Front. Plant Sci. 11:347. doi: 10.3389/fpls.2020.00347
Whitman, D. W., and Agrawal, A. A. (2009). “What is phenotypic plasticity and why is it important?,” in Phenotypic Plasticity of Insects: Mechanisms and Consequences., eds D. W. Whitman and T. N. Ananthakrishna (Enfield, NH: Science Publishers Inc.). doi: 10.1201/b10201-2
Wright, J. T., de Nys, R., and Poore, A. G. B. (2004). Chemical defense in a marine alga: heritability and the potential for selection by herbivores. Ecology 58, 2946–2959. doi: 10.1890/03-4041
Keywords: seaweed plasticity, common garden, laboratory cultivation, secondary metabolite, chemical defense
Citation: Sudatti DB, Oliveira AS, da Gama BAP, Fujii MT, Rodrigues SV and Pereira RC (2021) Variability in Seaweed Chemical Defense and Growth Under Common Garden Conditions. Front. Mar. Sci. 8:720711. doi: 10.3389/fmars.2021.720711
Received: 04 June 2021; Accepted: 22 September 2021;
Published: 25 October 2021.
Edited by:
Francesca Porri, South African Institute for Aquatic Biodiversity, South AfricaReviewed by:
Pamela A. Fernández, Universidad de Los Lagos, ChileTim Rawlings, Cape Breton University, Canada
Izabela Michalak, Wrocław University of Science and Technology, Poland
Copyright © 2021 Sudatti, Oliveira, da Gama, Fujii, Rodrigues and Pereira. This is an open-access article distributed under the terms of the Creative Commons Attribution License (CC BY). The use, distribution or reproduction in other forums is permitted, provided the original author(s) and the copyright owner(s) are credited and that the original publication in this journal is cited, in accordance with accepted academic practice. No use, distribution or reproduction is permitted which does not comply with these terms.
*Correspondence: Renato Crespo Pereira, cmNyZXNwbyYjeDAwMDQwO2lkLnVmZi5icg==
†Present address: Renato Crespo Pereira, Instituto de Pesquisas Jardim Botânico do Rio de Janeiro, Rio de Janeiro, Brazil