- 1Department of Natural Sciences, University of Alaska Southeast, Juneau, AK, United States
- 2Department of Agronomy, Horticulture and Plant Science, South Dakota State University, Brookings, SD, United States
- 3College of Fisheries and Ocean Sciences, University of Alaska Fairbanks, Juneau, AK, United States
- 4Gund Institute for Environment, University of Vermont, Burlington, VT, United States
- 5School of Marine and Atmospheric Sciences, Stony Brook University, Southampton, NY, United States
Primary producers are the foundation of marine food webs and require reliable nutrient sources to maintain their important role with ecosystems. While marine mammals and seabirds can play critical roles in marine nutrient cycling, their contributions are often overlooked. Southeast Alaska’s marine environment supports abundant marine mammal and seabird populations in addition to valuable fisheries. Nonetheless, there is still relatively little known about nutrient sources and fluxes in this region which is a critical component of fisheries management. The goal of our study was to advance knowledge of the role of mammals and seabirds in marine nutrient cycling and to understand how changing marine mammal and seabird populations may alter ecosystem dynamics. We utilized qualitative network models (QNMs) to examine how a simulated Southeast Alaska ecosystem would respond to an increase in marine mammals, seabirds, and nutrients. Researchers are increasingly utilizing QNMs as a first step in the development of ecosystem-based fisheries management plans as their adaptable nature is well suited to address rapidly changing climatic conditions. Our results indicate that marine mammals and seabirds make important contributions to marine nutrient concentrations in the region and that these valuable ecosystem services should not be overlooked.
Introduction
Large mammals and birds have a profound impact on nutrient cycling in terrestrial systems (e.g., McNaughton et al., 1997). For example, elephants (Loxodonta africanus) are ecosystem engineers that facilitate nutrient cycling through their high dietary diversity and excavation of termite mounds and salt licks, which release nutrients such as nitrogen, sodium, potassium, calcium, and magnesium into the soil (summarized in Poulsen et al., 2017). Black bears (Ursus americanus) and brown bears (U. arctos) in coastal Alaskan rainforest ecosystems feed on Pacific salmon (Oncorhynchus spp.) and release nitrogen and phosphorus into nutrient-limited soil (Sidle and Shaw, 1983) via discarded carcasses and salmon-enriched waste (Willson et al., 1988; Helfield and Naiman, 2006; Gende et al., 2007; Quinn et al., 2009). Many birds, through their vast migratory routes, transport nutrients between diverse ecosystems and across large spatial scales (Whelan et al., 2008; Bauer and Hoye, 2014; Gaston et al., 2018). In contrast, there is little understanding of the role of mammals and birds in nutrient cycling within marine systems and further, they are seldom considered in management strategies.
A growing body of research describes the importance of marine mammal scat (hereafter referred to as scat) to marine nutrient inputs in the mixed layer of marine waters. Due to the physiological dive response, which decreases metabolism and restricts non-vital (e.g., digestive) processes while diving (Kooyman et al., 1981), cetaceans are likely to defecate exclusively in the photic zone, producing buoyant, nutrient-rich fecal plumes that can stimulate primary productivity (Roman and McCarthy, 2010; Smith et al., 2013; Lavery et al., 2014; Roman et al., 2016). Roman and McCarthy (2010) showed that nitrogen input to the Gulf of Maine from cetacean and seal scat contributes more than all rivers in the region combined. Lavery et al. (2010) demonstrated how sperm whales (Physeter macrocephalus) have the potential to stimulate new primary productivity by transporting allochthonous iron from depth (via digested squid) to the photic zone in the Southern Ocean. A suite of studies (Nicol et al., 2010; Lavery et al., 2014; Ratnarajah et al., 2014, 2016; Roman et al., 2014) has also demonstrated the potential for baleen whale defecation to aid in recycling of autochthonous nutrients and stimulation of primary productivity. Despite this mounting evidence ascribing the importance of marine mammals to marine productivity, questions remain regarding the generalizability of these findings as all but two (Roman and McCarthy, 2010; Roman et al., 2016) of the aforementioned studies occurred in the pelagic waters of the Southern Ocean.
Seabird guano (hereafter referred to as guano) enhances marine nutrient levels in nearshore environments. Wing et al. (2014) showed that iron concentrations were eight times higher in seabird guano than in the sub-Antarctic mixed layer. Further, Wainright et al. (1998) reported that seabird breeding colonies in the Bering Sea were significant sources of recycled nitrogen for nearshore phytoplankton. In the Baltic Sea, guano derived from great cormorants (Phalacrocorax carbo) significantly enriched nitrogen and phosphorus levels in benthic communities near breeding colonies (Kolb et al., 2010; Gagnon et al., 2013) while guano leached from common murre (Uria aalge) breeding colonies increased ammonium (NH4+) and phosphate (PO43–) levels in the coastal marine environment by > 150% (Hentati-Sundberg et al., 2020). Guano-derived nitrogen and phosphorus are also important nutrient sources for Pacific coral reefs (Honig and Mahoney, 2016; Lorrain et al., 2017). The diverse locations of the aforementioned studies indicates that guano-based enrichment of nearshore waters persists across a latitudinal gradient.
Primary producers require reliable nutrient sources for photosynthesis and cellular function (Raven, 1988), but nutrients have a heterogeneous spatiotemporal distribution. In the Northeast Pacific, nutrient input depends on proximity to the continental shelf, with multiple mechanisms allowing for biologically available nutrients to enter both nearshore and pelagic systems (Stabeno et al., 2004; Weingartner et al., 2009). Nutrient availability in nearshore waters is determined by the watershed properties of the land they abut (Sugai and Burrell, 1984) and the interaction of water masses on the continental shelf, such as localized upwelling and mixing events (Stabeno et al., 2004). Nearshore nutrient limitation exhibits high geographic variability, and these complex, often generalized, dynamics require examination on a geographically finer scale to more fully understand nearshore nutrient fluxes.
Having a thorough understanding of ecosystem dynamics is necessary for the successful management of marine resources. Marine fisheries management in the US has historically followed a single species approach with little consideration for how broader ecosystem dynamics influence harvested species. Whereas it has become commonplace for fisheries policy makers and resource managers to consider physically derived nutrient sources in developing management strategies (Zador et al., 2019; Harvey et al., 2020), there are currently limited pathways to incorporate biologically derived nutrient sources. Moreover, these limitations cause marine mammals and seabirds rarely to be considered as anything but direct competitors for fishery resources (see Peterson et al., 2014; Chenoweth and Criddle, 2019; Hanselman et al., 2019). However, in recent years, there has been a shift toward more sustainable, holistic approaches to resource management via ecosystem-based fisheries management (EBFM; Marasco et al., 2007). The shift to the EBFM has created a platform allowing for an examination of traditionally overlooked ecosystem components and user groups (Levin et al., 2009; Zador et al., 2017; Rosellon-Druker et al., 2020). In particular, the push toward EBFM presents an opportunity to gain a thorough understanding of how marine mammal and seabird nutrient cycling dynamics can be incorporated into ecosystem management plans.
Situated in the Northeast Pacific, Southeast Alaska is comprised of the Alexander Archipelago, a region characterized by a narrow continental shelf; deep fjords; long, narrow channels; nearshore bays; rugged mountains; and tidewater glaciers (Weingartner et al., 2009). This region has a temperate marine climate with significant annual precipitation. In addition to precipitation events, Southeast Alaska retains substantial glacial coverage with glacial runoff that contributes to the volume of freshwater that is discharged into the region (Weingartner et al., 2009). Little is known of the marine nutrient profiles across Southeast Alaska, however, as past research has been geographically localized (Sugai and Burrell, 1984; Etherington et al., 2007; Hood and Scott, 2008; Hood and Berner, 2009; Fellman et al., 2010; Arimitsu et al., 2016). As a result, information on nutrient fluxes in the region is typically generalized from the small pool of existing research in the area in addition to other geographically similar regions (i.e., Norway; Hop et al., 2002; Öztürk and Bizsel, 2003). Further, there is no published research examining the nutrient inputs of marine mammals and seabirds to Southeast Alaska’s marine ecosystems, which is surprising given the increasing abundance of many species in the region (Marston et al., 2002; Dahlheim et al., 2009; National Oceanic and Atmospheric Administration (NOAA), 2016; Dragoo et al., 2019; Muto et al., 2020).
As a first step in addressing this information gap, we developed a qualitative network model (QNM) to examine how a simulated Southeast Alaska system would respond to multiple marine mammal and seabird perturbation scenarios. There has been a growing interest in the use of QNMs in EBFM, particularly as a first step within the National Marine Fisheries Service Integrated Ecosystem Assessment (IEA) framework (Dambacher et al., 2015; Harvey et al., 2016; Zador et al., 2017; Szymkowiak and Rhodes-Reese, 2020). QNMs require minimal data and allow researchers to holistically explore how an ecosystem will respond to a perturbation, while being accessible to scientists and managers alike (Puccia and Levins, 1985; Melbourne-Thomas et al., 2012). By operationalizing conceptual models, QNMs rely only on the sign of the interacting variables within the system (+, −, 0) to construct a sign directed graph (digraph). The digraph is then reconstructed into a community matrix, allowing for a probabilistic interpretation of the simulated community response to a perturbation (Melbourne-Thomas et al., 2012; Reum et al., 2015). Easily adaptable and iterative in nature, QNMs allow researchers to reevaluate variable interactions as processes, like the IEA, progress and new information becomes available (Harvey et al., 2016). Because of this, QNMs are increasingly useful in adaptive management scenarios that may otherwise require considerable amounts of data. Like any modeling technique, they are not without their limitations, but serve as a useful first step in understanding ecosystem dynamics by providing a qualitative assessment to aid in the development of EBFM.
The goal of this study is to advance understanding of the ecological significance of marine mammals and seabirds to ecosystem functioning in Southeast Alaska by examining their role in nutrient cycling. Utilizing QNMs, we model the contributions of marine mammals and seabirds to the ecosystem and discuss how our results may be used to inform EFBM.
Materials and Methods
Southeast Alaska Conceptual Model
We examined the ecological role of marine mammals and seabirds within the inside waters of a generalized Southeast Alaska fjord system during the spring and summer while taking into account their nutrient contributions (Figure 1). We first developed a conceptual model to depict the key ecological interactions within this ecosystem. The conceptual model was then operationalized as a QNM, providing a qualitative prediction of how the system would respond to increases in nutrient inputs from upper and lower trophic levels (Table 1).
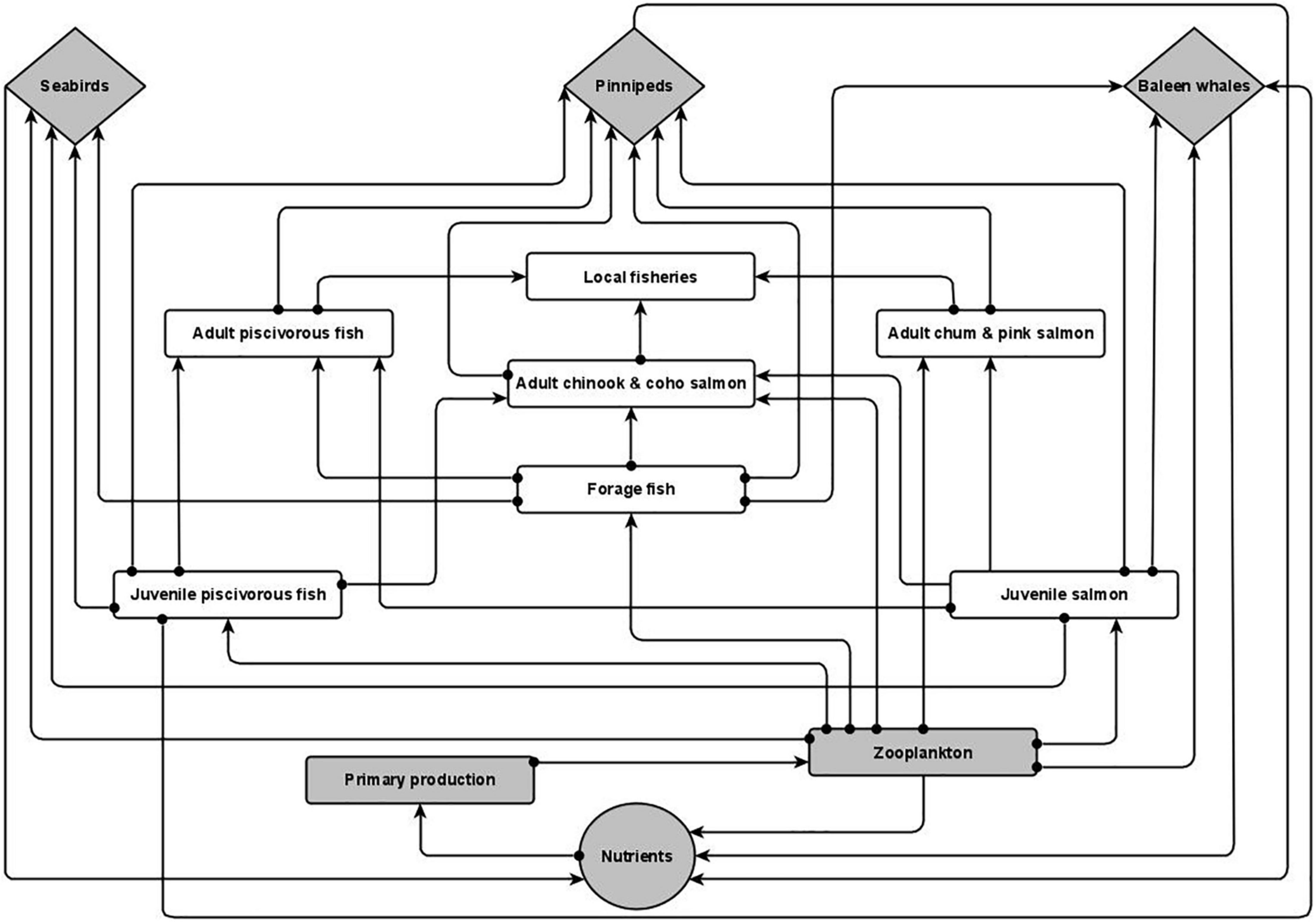
Figure 1. Conceptual model of the Southeast Alaska system. Lines that terminate with an arrow (→) indicate a positive relationship and lines that terminate with a circle (•) indicate a negative relationship. All variables have negative self-effect (not pictured). Detailed descriptions of node interactions can be found in Supplementary Table 1. Diamonds: Marine mammal and seabirds; rectangles: ecosystem variables; circle: marine nutrients. Gray nodes denote variables that were increased in the eight perturbation scenarios. Figure prepared using R version 3.4.0 (R Core Team, 2019) packages “ggplot2” (Wickham, 2016).
Relevant ecosystem variables and their linkages were determined from a literature review that included peer-reviewed literature and agency reports. Links were considered sufficient if a minimum of one paper documented the relationship. Literature searches were initially restricted to Southeast Alaska and expanded to the closest geographical or geographically comparable area if literature on Southeast Alaska was unavailable. Because of the limited number of publications specifically addressing the influence of marine mammals and seabirds on nutrient dynamics, literature searches on publications specific to this topic did not have any geographic restrictions (see Supplementary Table 1 for variable linkages and their supported references). Our model reflects a generalized depiction of the ecological interactions that occur within Southeast Alaska. Biota that occupied similar ecological niches were grouped together to enhance overall model stability. Scientific names for species included in model variables are listed in Table 1.
Marine mammals and seabirds are depicted as three separate variables (Figure 1). Seabirds often congregate in high numbers in areas where their prey (e.g., forage fish, zooplankton) concentrate (Marston et al., 2002; Arimitsu et al., 2016). We considered the species most commonly seen in the inside waters of Southeast Alaska during the spring and summer—gulls, Arctic terns, common murres, pigeon guillemots, marbled murrelets, and pelagic cormorants (Alaska Department of Fish and Game [ADFG], 2015; Table 1). Pinnipeds, specifically Steller sea lions and harbor seals, occur in Southeast Alaska year round. Steller sea lions primarily consume forage fish such as Pacific herring, and there have been instances where > 900 individuals congregated in a single bay (Berners Bay) to feed on spawning eulachon (Marston et al., 2002; Sigler et al., 2004; Womble and Sigler, 2006). The most common Baleen whale species, the humpback whale, occurs in Southeast Alaska from late spring to fall (Dahlheim et al., 2009) to consume zooplankton and small schooling fish (Jurasz and Jurasz, 1979; Marston et al., 2002; Boswell et al., 2016; Straley et al., 2018). Although the role of sperm whales in marine nutrient cycling has been examined (Lavery et al., 2010), this species rarely occurs in the inside waters of Southeast Alaska. Additionally, Dall’s porpoise (Phocoenoides dalli), harbor porpoise (Phocoena phocoena), and killer whales (Orcinus orca) occur in Southeast Alaska (Pearson, unpubl. data; Dahlheim et al., 2009) but their role in marine nutrient cycling has not been fully explored. While sea otters (Enhydra lutris) occur in Southeast Alaska, their presence in inside waters is still relatively limited (Tinker et al., 2019) and further, their role in marine nutrient cycling has not been fully explored. Therefore, we did not include odontocetes (toothed whales) or sea otters in our model.
Macronutrients (Nutrients) are primarily sourced from freshwater runoff as well as episodic mixing events within the region (Weingartner et al., 2009; Arimitsu et al., 2016). The guano and scat from seabirds, pinnipeds, and baleen whales have also been shown to contain high levels of limiting nutrients that positively influence phytoplankton (Primary production) growth (Theobald et al., 2006; Roman and McCarthy, 2010; Shatova et al., 2016), acting as localized fertilization events. Primary production in the region exhibits a large spring bloom, triggered by the combination of increasing solar radiation following the winter months and physical mixing events that enhance nutrient levels in the surface layer (Ziemann et al., 1991; Weingartner et al., 2009). Primary production biomass precipitously drops once nutrients are depleted. Subsequent smaller blooms occur throughout the summer following periodic weather-related mixing events (Ziemann et al., 1991). Ultimately, phytoplankton serves as the link between nutrients, regardless of source, and all of the other ecosystem components.
Zooplankton includes a variety of taxa that includes euphausiids, copepods, and larval fish. Primary production in the area is known to have a positive impact on euphausiid reproduction with spawning periods overlapping with the spring phytoplankton bloom (Szabo and Batchelder, 2014). Zooplankton is the primary prey item for the majority of the model variables but relatively little research has been done in the region to examine detailed species compositions. However, Szabo and Batchelder (2014) reported the presence of four euphausiid species (Thysanoessa raschii, T. longipes, T. spinifera, and Euphausia pacifica) in Frederick Sound and lower Stephens Passage during the late spring and summer. Overall, zooplankton is a key prey item for juvenile salmon, forage fish, juvenile piscivorous fish, and humpback whales (Dolphin, 1987; Burrows et al., 2016; Zador et al., 2019; Fergusson et al., 2020).
Forage fish are primarily comprised of Pacific herring and eulachon. Both species form large spawning aggregations in the spring and early summer that attract predators from throughout Southeast Alaska (Marston et al., 2002; Womble and Sigler, 2006). In particular, Pacific herring has been observed to make up more than 80% of the diet of humpback whales in the area (Straley et al., 2018).
Juvenile salmon fill a similar niche as forage fish, and their size and schooling tendencies make them susceptible to being consumed by animals such as humpback whales (Chenoweth et al., 2017). Wild and hatchery- raised Pacific salmon are abundant in Southeast Alaska and are consumed by a variety of predators (Sturdevant et al., 2012; Duncan and Beaudreau, 2019). The salmon hatcheries throughout the region rear juvenile salmon in saltwater net pens in nearshore areas to imprint them for release into the wild. When salmon are released, humpback whales are often observed feeding along shore in areas adjacent to release sites, presumably on hatchery salmon (Pearson, pers. obsv.). Adult chum and pink salmon are dependent upon zooplankton for the entirety of their marine residence (Sturdevant et al., 2012; Zador et al., 2019).
Juvenile piscivorous fish primarily comprises Pacific cod, pollock, and flatfish species. They are readily consumed by pinnipeds when Pacific herring is not available and are a common prey item for adult chinook and coho salmon (Sturdevant et al., 2012). Adult chinook and coho salmon have been shown to consume zooplankton, but fish comprise their primary prey items (Sturdevant et al., 2012). Juvenile and adult piscivorous fish inhabit deeper waters and do not school in the same manner as forage fish and juvenile salmon, making them a secondary prey item to pinnipeds in most cases, though valuable when preferred prey is not available (Womble and Sigler, 2006; Sturdevant et al., 2012).
Lastly, multiple local fisheries occur in the region throughout the year. In the summer months, commercial and recreational salmon fisheries harvest salmon species throughout Southeast Alaska. In addition to salmon fisheries, the Pacific halibut and sablefish longline fisheries occur from March to November throughout the region.
Qualitative Network Models
To gain a greater understanding of how marine mammals and seabirds interact with the ecosystem variables described above, we examined eight separate scenarios to demonstrate how the variables within our generalized Southeast Alaska ecosystem will respond to change. The eight separate scenarios increase (perturb) unique variables (Table 2) with the QNM that was developed from our conceptual model. These eight scenarios were considered direct ecosystem responses to increases in either higher (i.e., marine mammals and seabirds) or lower (i.e., nutrients, primary production, and zooplankton) trophic level components. This allowed for the simulated ecosystem response to increasing marine mammals and seabird populations to be placed in a broader ecological context.
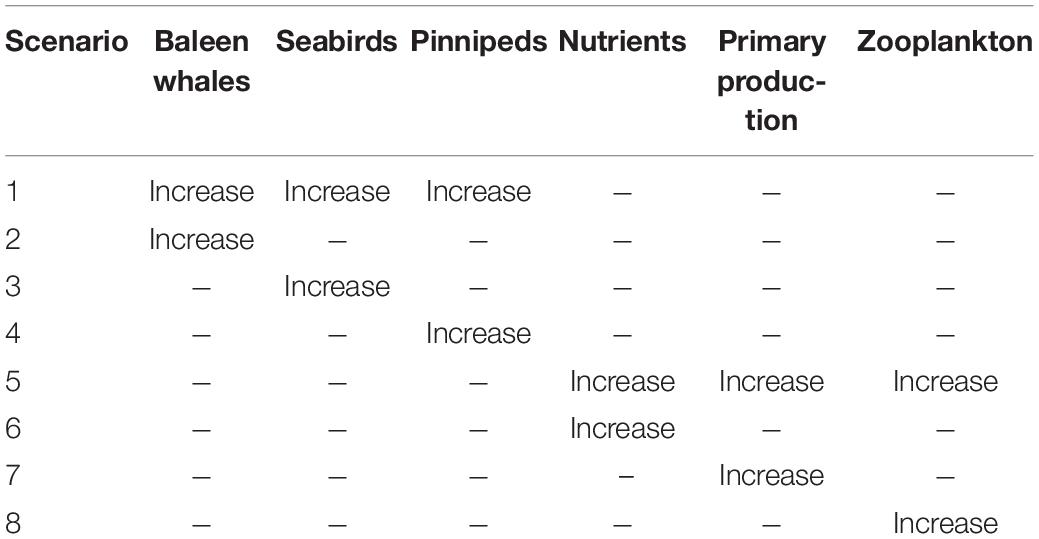
Table 2. Qualitative network modeling was used to simulate eight scenarios to assess the impact of increases in upper and lower trophic levels in Southeast Alaska.
QNMs provide a qualitative prediction of community response to a sustained (press) or short (pulse) perturbation and are effective in gaining a preliminary understanding of community interactions in data-limited systems (Dambacher et al., 2009; Melbourne-Thomas et al., 2012). Press perturbation experiments assess the long-term consequences of direct and indirect effects at a new equilibrium. Conversely, pulse perturbation experiments provoke an instantaneous alteration of a species abundance and examine the return of the community to a previous equilibrium state (Bender et al., 1984). Press and pulse perturbation experiments are frequently used together to operationalize conceptual models by creating a signed digraph of community variables with the node (variable) interaction strength represented as either positive (→) or negative (•) (Melbourne-Thomas et al., 2012).
A signed digraph was constructed from our conceptual model for Southeast Alaska (described below) in Dia (ver. 0.97.2)1. The R package QPress (Melbourne-Thomas et al., 2012; R Core Team, 2019) was used to analyze the QNMs constructed from our conceptual model. The QNM framework creates a community matrix from the signed digraph and expresses matrix elements as the directionality of the variable interactions (→ = 1, • = −1, no link = 0).
To assess how a simulated community will respond to a sustained press perturbation, a community matrix was created by assigning random variable interaction strengths (community matrix elements: 0, 1) while retaining each known link direction (positive or negative). Negative self-limitations (negative self-effects) were included in all variables to account for external processes not included in the system and to enhance overall model stability (Puccia and Levins, 1985; Raymond et al., 2011; Melbourne-Thomas et al., 2012). The stability of the simulated community matrix was tested against known criteria following the methods outlined in Melbourne-Thomas et al. (2012).
Only stable matrices were retained, and the inverse of these matrices was used to determine the predicted response of the community to a press perturbation. This process was repeated 10,000 times, from which we summarized the distribution of positive or negative responses of each variable to the perturbation. The impact of the perturbations was thus examined for each variable across the set of stable matrices. The response of the individual nodes was expressed as the probability of a given outcome (Melbourne-Thomas et al., 2012). A response was considered consistent if it was positive or negative ≥ 70% of the time, while responses < 70% were considered equivocal (Raymond et al., 2011; Harvey et al., 2016).
Results
The ecosystem response to the marine mammal and seabird scenarios was equivocal overall with 59% of the variables responding with uncertain results (not clearly positive or negative; Figure 2). Scenario 1 (baleen whale, pinniped, and seabird increase) elicited a positive response in primary production (75%) and nutrients (78%) and a negative response in local fisheries (79%), adult piscivorous fish (77%) and juvenile salmon (77%). Scenario 2 (baleen whale increase) elicited an equivocal response from all variables with the exception of pinnipeds (78%) and seabirds (79%) where the response was negative. Scenario 3 (seabird-only increase) elicited no positive responses amongst any group; however, baleen whales (79%) and pinnipeds (78%) responded negatively to this scenario. Lastly, Scenario 4 (pinniped-only increase) elicited a positive response in zooplankton (79%) and nutrients (84%) and a negative response in adult piscivorous fish (79%) and local fisheries (86%).
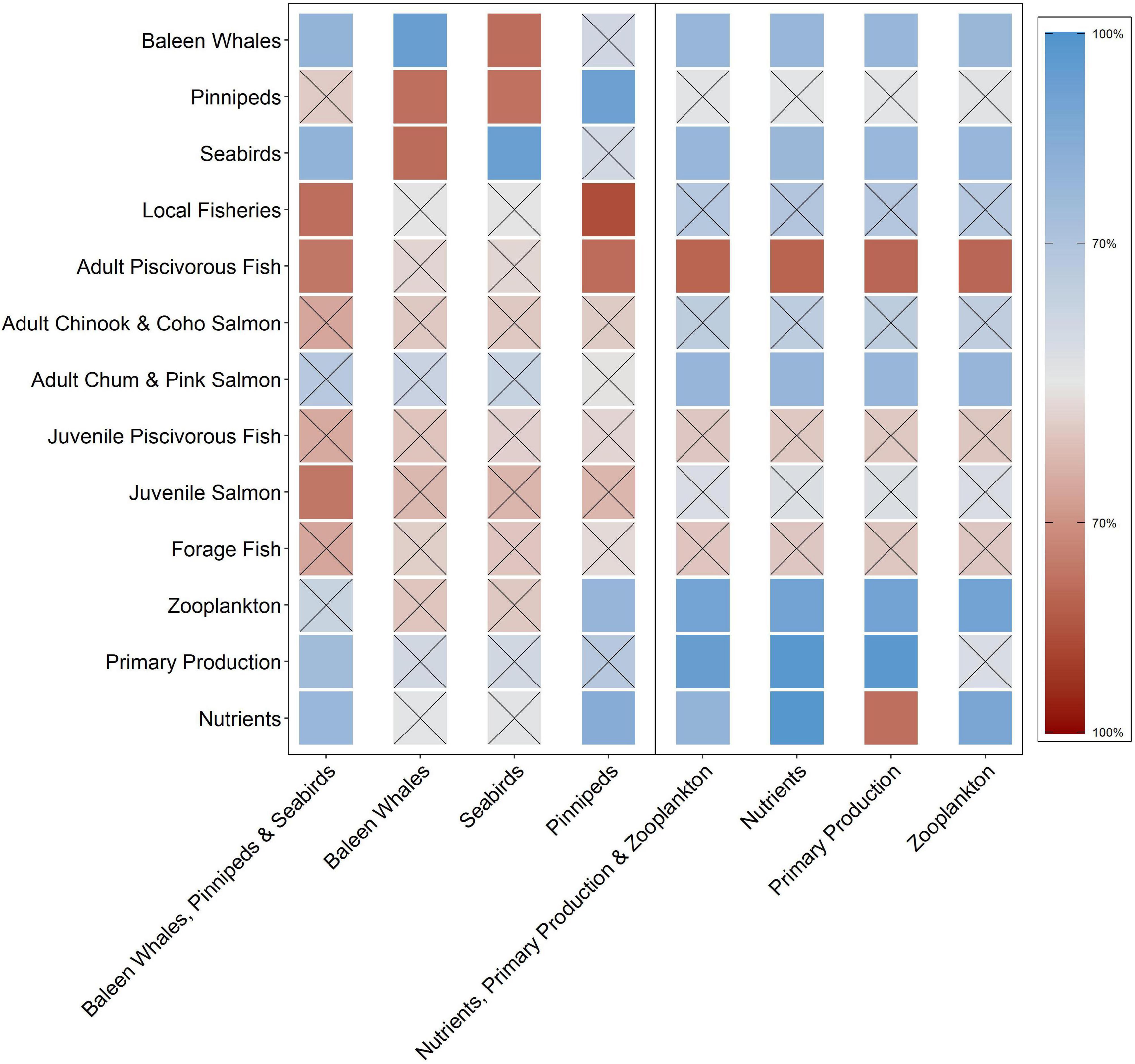
Figure 2. Qualitative network model simulation results for eight perturbation scenarios reflecting direct ecosystem responses to increases in either higher (baleen whales, pinnipeds, and/or seabirds) or lower (nutrients, primary production, and/or zooplankton) trophic level components. The squares represent the probability of a positive (blue) or negative (red) response of the ecosystem variables (y-axis) within each scenario (x-axis). Square shading intensity corresponds with an increase (darker) or decrease (lighter) in sign consistency. Squares with an “x” denote equivocal results that are less than the 70% threshold established for a response to be considered consistent (i.e., positive or negative).
The overall response to increases in the lower trophic level variables was equivocal, with 52% of the ecosystem components responding ambiguously (Figure 2). In particular, baleen whales, seabirds, and adult chum and pink salmon responded positively to each scenario. Scenario 5 (nutrient, primary production, and zooplankton increase) elicited a positive response in baleen whales (78%), seabirds (79%), and adult chum and pink salmon (79%) and a negative response in adult piscivorous fish (81%). Scenario 6 (nutrient-only increase) elicited a positive response in baleen whales (78%), seabirds (78%), adult chum and pink salmon (79%), zooplankton (90%), and primary production (98%) and a negative response to adult piscivorous fish (81%). Scenario 7 (primary production-only increase) elicited a positive response in baleen whales (79%), seabirds (78%), adult chum and pink salmon (78%), and zooplankton (90%) and a negative response in adult piscivorous fish (81%) and nutrients (78%). Finally, Scenario 8 (zooplankton-only increase) elicited a positive response in baleen whales (78%), seabirds (79%), adult chum and pink salmon (79%), and nutrients (88%) and a negative response in adult piscivorous fish (81%).
Discussion
Air-breathing vertebrates are essential to maintaining a nutrient balance in the marine environment, and their role in maintaining this balance has been largely overlooked. Researchers have recently begun to underscore the important role that these animals play in supporting this balance both in the past and present (Doughty et al., 2013, 2016; Roman et al., 2014). This study fills an important gap in the literature by presenting a broad-scale assessment of the contribution of marine mammals and seabirds to nutrient levels in Southeast Alaska. Our QNM results reveal that marine mammals and seabirds can make significant contributions to nutrient cycling and overall ecosystem functioning within Southeast Alaska. However, we caution that due to the inherently descriptive, exploratory, and simplistic nature of QNMs, these qualitative results should be substantiated with empirical data collection and rigorous quantitative modeling.
Our results revealed that increasing all three upper trophic level components (Scenario 1) resulted in an increase in nutrients in our simulated ecosystem. At the same time, however, the overall uncertain results from the individual increases in upper trophic levels (Scenarios 2–4) demonstrate the adaptability and resiliency of a diverse and healthy ecosystem in response to a perturbation (Apollonio, 2002; Worm et al., 2006). In contrast, the overall positive responses to increases in lower trophic levels (Scenarios 5–8) demonstrate the importance of nutrients, primary production, and zooplankton in maintaining ecosystem function and highlight their key role in regulating ecosystem productivity.
Work in the Southern Ocean has shown that nutrient inputs from sperm whale, blue whale (Balaenoptera musculus), and fin whale (B. physalus) fecal plumes can create positive feedback loops leading to enhanced ecosystem productivity (Lavery et al., 2010, 2014; Nicol et al., 2010). As the Northeast Pacific and the Southern Ocean have similar nutrient limitations (Fung et al., 2000), this positive feedback loop is also likely to occur in Alaskan waters. However, we caution that there is a difference between nutrient availability and input mechanisms between offshore areas (e.g., central Gulf of Alaska) and the fjord systems of Southeast Alaska.
Our QNM results revealed some surprising relationships. First, increasing baleen whales (Scenario 2) or seabirds (Scenario 3) did not increase nutrient levels. It is possible that the many sources of nutrient inputs of the fjord systems of Southeast Alaska (e.g., localized glacial-driven upwelling and mixing events; Weingartner et al., 2009), as compared to the pelagic systems in which the whale pump and similar processes have been previously investigated (Lavery et al., 2010, 2014; Nicol et al., 2010; Roman and McCarthy, 2010; Ratnarajah et al., 2014; Roman et al., 2014, 2016; Wing et al., 2014), require synergistic interactions among top marine predators to elevate nutrient levels. We also note that guano-derived nutrient enrichment tends to occur at localized scales, even in the presence of high seabird densities (Wainright et al., 1998; Kolb et al., 2010; Gagnon et al., 2013; Honig and Mahoney, 2016; Hentati-Sundberg et al., 2020). While the spatial scale of scat-derived nutrient enrichment remains unexplored, it is possible that this is also limited to localized scales. Empirical testing of these relationships is a fruitful avenue for future research. Second, zooplankton responded positively to pinnipeds, which consume other planktivores, such as forage fish, juvenile piscivorous fish, and juvenile salmon (Sigler et al., 2004; Womble and Sigler, 2006), thus releasing zooplankton from predation pressure. Finally, seabirds and pinnipeds were the only variables to respond negatively to an increase in baleen whales (Scenario 2), likely resulting from competition for similar prey sources in this region.
QNMs are a valuable tool for analyzing ecosystem interactions in data-limited systems and provide a means to guide future studies by bridging information gaps and highlighting relationships that warrant quantitative analysis. Nevertheless, this method is not without limitations. QNMs are generalized representations of ecosystems and often require the grouping or omission of variables to achieve model stability. In our study, some key ecological interactions were omitted for model stability. For example, we did not consider benthic interactions and the valuable role that benthic organisms play in ecosystem functioning. Fish such as Pacific halibut, walleye pollock, and Pacific cod are known to prey on benthic macroinvertebrates (Lang et al., 2003; Stoner, 2009; Pirtle et al., 2012; IPHC, 2014), and benthic species such as juvenile king crab (Paralithodes camtschaticus) have been identified in diet studies (Stoner, 2009; Pirtle et al., 2012). Further, we did not consider the influence of body size in our QNMs. Whereas pinnipeds elicited the strongest response in nutrients among the upper trophic level scenarios, the average mass of adult male and female Steller sea lions (273 and 1,000 kg, respectively; Jefferson et al., 2015) encompasses the mass of a newborn humpback whale calf (680 kg; Jefferson et al., 2015), which are both orders of magnitude larger than gulls (1.2 kg for glaucous-winged gulls, Larus glaucescens; Schreiber and Burger, 2001). Additionally, QNMs in their current forms are not able to weigh model components and thus our model did not consider the influence of spatiotemporal variability or weigh nutrient inputs according to defecation location (e.g., baleen whales always defecate at sea while pinnipeds and seabirds defecate at sea and on land). Finally, QNMs are currently unable to account for time-scale differences (i.e., actual food web mechanisms) in terms of how populations at different trophic levels will react over time to increases in nutrient inputs or lower trophic level biomass increases. As such, QNMs are a valuable exploratory tool but these constraints and limitations should be considered when interpreting the results.
Further, the simplistic nature of QNMs required the exclusion of many components that would benefit from additional research. For example, while we excluded odontocetes from our model, a greater understanding of their ecosystem role is critical, especially in regions where interactions between odontocetes and fisheries are increasing. Anecdotal reports have documented interactions between longline fishing vessels and odontocetes in Alaska since the 1960s (Dahlheim, 1988). In the mid-1990s, the Alaska Pacific halibut and sablefish longline fisheries transitioned from derby-style fishery with short, sometimes 1–2 day openers a year, to a catch share program with a 9-month season. This dramatic extension in the fishing season provided greater opportunities for killer whales and sperm whales to take advantage of easy prey waiting to be hauled in by fishermen (Hill et al., 1999; Sigler et al., 2008; Peterson and Carothers, 2013). The urgency of these conflicts has made it more difficult to examine interactions that are not as easily quantifiable in economic terms (Hanselman et al., 2018) as fishermen are required to adjust their harvesting strategies to avoid depredation (Peterson et al., 2014; Szymkowiak and Rhodes-Reese, 2020). Nevertheless, it has been shown that the recruitment of many commercial fish species, such as sablefish, is positively correlated with chlorophyll-a (Yasumiishi et al., 2014), a common proxy for primary production. Since primary production is dependent on reliable nutrient sources, and following our QNM results, it warrants further examination if the ecosystem services provided by whales outweighs the negative effects incurred by depredation.
The few studies that have assessed competition between whales and fisheries often do not consider the interplay between whales and lower trophic levels (Croll and Kudela, 2006; Essington, 2006; Surma and Pitcher, 2015). When considering ecosystem dynamics, marine mammals are oftentimes represented as analogous to fisheries with both groups depicted as a predator that removes an element from the community (Essington, 2006; Surma and Pitcher, 2015); however, this perspective neglects the positive feedback interactions between whales, other large marine vertebrate taxa, and primary production. Furthermore, researchers commonly assess the relative importance of particular taxa by estimating and comparing the total primary production that must be consumed along preceding trophic pathways before reaching the consumer of interest. These depictions often fail to examine the mechanisms that support primary production itself (Croll and Kudela, 2006; Essington, 2006). Previous studies comparing the primary production required to support whales and commercial fisheries conveyed that baleen whales repeatedly required less than commercial fisheries, with odontocetes requiring quantities nearer to that of commercial fisheries (Morissette et al., 2012; Ruzicka et al., 2013).
Based on the results of the present study, we advocate for an EBFM approach that also considers the bottom-up trophic effects of marine mammals and seabirds in enhancing primary production. The growing momentum for EBFM has propelled researchers and managers to examine ecosystem dynamics that may have otherwise been overlooked. Conceptual models and ultimately, QNMs, have become useful tools in the development of EBFM frameworks and provide a mechanism for overlooked ecosystem dynamics to be evaluated. The utilization of QNMs in data limited systems allows researchers to elucidate relationships that may have been otherwise unnoticed and are easily adapted as new information becomes available. The development of adaptive management strategies allow resource managers and policy makers to make informed decisions that support all user groups and aid in the long-term sustainability of marine resources.
Historically, marine mammals were heavily exploited (Ivashchenko et al., 2011; Ivashchenko and Clapham, 2014) with some great whale populations, such as the blue whale, having been reduced by more than 90% by commercial whaling (Branch et al., 2007). Similarly, seabirds were historically exploited for food, guano, and the millinery trade, which reduced some species (e.g., short-tailed albatross, Phoebastria albatrus) to < 50 individuals (Boersma et al., 2001). The function of marine mammals and seabirds in nutrient cycling has long been overlooked, in part because substantially depleted populations could be perceived as the status quo (Springer et al., 2003; Ivashchenko et al., 2011; Ivashchenko and Clapham, 2014; Roman et al., 2014). As many of these populations recover, understanding their role in ecosystem functioning will become increasingly important.
Conclusion
This study contributes to the growing body of literature revealing that marine mammals and seabirds are significant contributors to marine nutrient levels and highlights their critical but sometimes overlooked functional role within the ecosystem. Ecosystem management plans oftentimes consider these taxa only as charismatic megafauna from a tourism perspective (O’Connor et al., 2009) or as direct competitors from a fisheries perspective (Peterson et al., 2014). We recommend a detachment from this dichotomy through application of innovative methods that will facilitate integration of the ecosystem role of these top predators into successful, holistic, and sustainable ecosystem-based management practices.
Data Availability Statement
The original contributions presented in the study are included in the article/Supplementary Material, further inquiries can be directed to the corresponding author/s.
Author Contributions
MR-R in collaboration with and under the supervision of HP, conceived and designed the study, and collected, analyzed, and interpreted data. DC, JM-M, CR, JR, and JW contributed to project development and data acquisition and interpretation. CC contributed to project development and data interpretation. All authors contributed to drafting and revision of the manuscript and have approved the submitted version.
Conflict of Interest
The authors declare that the research was conducted in the absence of any commercial or financial relationships that could be construed as a potential conflict of interest.
Publisher’s Note
All claims expressed in this article are solely those of the authors and do not necessarily represent those of their affiliated organizations, or those of the publisher, the editors and the reviewers. Any product that may be evaluated in this article, or claim that may be made by its manufacturer, is not guaranteed or endorsed by the publisher.
Acknowledgments
We sincerely thank the reviewers for their suggestions and feedback.
Supplementary Material
The Supplementary Material for this article can be found online at: https://www.frontiersin.org/articles/10.3389/fmars.2021.720277/full#supplementary-material
Footnotes
References
Alaska Department of Fish and Game [ADFG] (2015). Common Marine Birds of Southeast Alaska. Juneau, AK: Alaska Department of Fish and Game, 28.
Apollonio, S. (2002). Hierarchical Perspectives in Marine Complexities: Searching for Systems in the Gulf of Maine. New York, NY: University of Columbia Press.
Arimitsu, M. L., Piatt, J. F., and Mueter, F. (2016). Influence of glacier runoff on ecosystem structure in Gulf of Alaska fjords. Mar. Ecol. Prog. Ser. 560, 19–40. doi: 10.3354/meps11888
Bauer, S., and Hoye, B. J. (2014). Migratory animals couple biodiversity and ecosystem functioning worldwide. Science 344:1242552. doi: 10.1126/science.1242552
Bender, E. A., Case, T. J., and Gilpin, M. E. (1984). Perturbation experiments in community ecology: theory and practice. Ecology 65, 1–13.
Boersma, P. D., Clark, J. A., and Hillgarth, N. (2001). “Seabird conservation,” in Biology of Marine Birds, eds E. A. Schreiber and J. Burger (Boca Raton, FL: CRC Press), 559–580.
Boswell, K. M., Rieucau, G., Vollenweider, J. J., Moran, J. R., Heintz, R. A., Blackburn, J. K., et al. (2016). Are spatial and temporal patterns in Lynn Canal overwintering Pacific herring related to top predator activity? Can. J. Fish. Aquat. Sci. 73, 1307–1318. doi: 10.1139/cjfas-2015-0192
Branch, T. A., Stafford, K. M., Palacios, D. M., Allison, C., Bannister, J. L., Burton, C. L. K., et al. (2007). Past and present distribution, densities and movements of blue whales Balaenoptera musculus in the Southern Hemisphere and northern Indian Ocean. Mamm. Rev. 37, 116–175.
Burrows, J. A., Johnston, D. W., Straley, J. M., Chenoweth, E. M., Ware, C., Curtice, C., et al. (2016). Prey density and depth affect the fine-scale foraging behavior of humpback whales Megaptera novaeangliae in Sitka Sound, Alaska, USA. Mar. Ecol. Prog. Ser. 561, 245–260. doi: 10.3354/meps11906
Carlson, H. R. (1980). Seasonal distribution and environment of Pacific herring near Auke Bay, Lynn Canal, southeastern Alaska. Trans. Am. Fish. Soc. 109, 71–78.
Chenoweth, E. M., and Criddle, K. R. (2019). The economic impacts of humpback whale depredation on hatchery-released juvenile pacific salmon in Southeast Alaska. Mar. Coast. Fish. Dyn. Manag. Ecosyst. Sci. 11, 62–75. doi: 10.1002/mcf2.10061
Chenoweth, E. M., Straley, J. M., McPhee, M. V., Atkinson, S., and Reifenstuhl, R. (2017). Humpback whales feed on hatchery-released juvenile salmon. R. Soc. Open Sci. 4:170180. doi: 10.1098/rsos.170180
Coutré, K. M., Beaudreau, A. H., and Malecha, P. W. (2015). Temporal variation in diet composition and use of pulsed resource subsidies by juvenile sablefish. Trans. Am. Fish. Soc. 144, 807–819. doi: 10.1080/00028487.2015.1037015
Croll, D. A., and Kudela, R. (2006). “Ecosystem impact of the decline of large whales in the North Pacific,” in Whales, Whaling, and Ocean Ecosystems, eds J. A. Estes, D. P. DeMaster, D. F. Doak, T. M. Williams, and R. L. Brownell Jr. (Berkeley, CA: University of California Press), 202–214. doi: 10.1371/journal.pone.0245409
Dahlheim, M. (1988). Killer Whale (Orcinus orca) Depredation on Longline Catches of Sablefish (Anoplopoma fimbria) in Alaskan Waters. Northwest and Alaska Fisheries Center Processed Report 88-14. Washington, DC: NOAA, 31.
Dahlheim, M. E., White, P. A., and Waite, J. M. (2009). Cetaceans of Southeast Alaska: distribution and seasonal occurrence. J. Biogeogr. 36, 410–426. doi: 10.1371/journal.pone.0155841
Dambacher, J. M., Gaughan, D. J., Rochet, M.-J., Rossignol, P. A., and Trenkel, V. M. (2009). Qualitative modelling and indicators of exploited ecosystems. Fish Fish. 10, 305–322. doi: 10.1111/j.1467-2979.2008.00323.x
Dambacher, J. M., Rothlisberg, P. C., and Loneragan, N. R. (2015). Qualitative mathematical models to support ecosystem-based management of Australia’s Northern Prawn Fishery. Ecol. Appl. 25, 278–298. doi: 10.1890/13-2030.1
Dolphin, W. F. (1987). Prey densities and foraging of humpback whales,Megaptera novaeangliae. Experientia 43, 468–471.
Doughty, C. E., Roman, J., Faurby, S., Wolf, A., Haque, A., Bakker, E. S., et al. (2016). Global nutrient transport in a world of giants. Proc. Natl. Acad. Sci. U.S.A. 113, 868–873. doi: 10.1073/pnas.1502549112
Doughty, C. E., Wolf, A., and Malhi, Y. (2013). The legacy of the Pleistocene megafauna extinctions on nutrient availability in Amazonia. Nat. Geosci. 6, 761–764. doi: 10.1038/ngeo1895
Dragoo, D. E., Renner, H. M., and Kaler, R. S. A. (2019). Breeding Status and Population Trends of Seabirds in Alaska, 2018, Fish and Wildlife Service Report AMNWR 2019/03. Homer, AK: U.S. Fish and Wildlife Service.
Duncan, D. H., and Beaudreau, A. H. (2019). Spatiotemporal variation and size-selective predation on hatchery- and wild-born juvenile chum salmon at marine entry by nearshore fishes in Southeast Alaska. Mar. Coast. Fish. 11, 372–390.
Essington, T. E. (2006). “Pelagic ecosystem response to a century of commercial fishing and whaling,” in Whales, Whaling, and Ocean Ecosystems, eds J. A. Estes, D. P. DeMaster, D. F. Doak, T. M. Williams, and R. L. Brownell Jr. (Berkeley, CA: University of California Press), 38–49. doi: 10.1525/9780520933200-009
Etherington, L. L., Hooge, P. N., Hooge, E. R., and Hill, D. F. (2007). Oceanography of Glacier Bay, Alaska: implications for biological patterns in a glacial fjord estuary. Estuaries Coasts 30, 927–944.
Fellman, J. B., Spencer, R. G. M., Hernes, P. J., Edwards, R. T., D’Amore, D. V., and Hood, E. (2010). The impact of glacier runoff on the biodegradability and biochemical composition of terrigenous dissolved organic matter in near-shore marine ecosystems. Mar. Chem. 121, 112–122. doi: 10.1016/j.marchem.2010.03.009
Fergusson, E. A., Watson, J., Gray, A., and Murphy, J. (2018). Annual Survey of Juvenile Salmon, Ecologically-Related Species, and Biophysical Factors in the Marine Waters of Southeastern Alaska, May–August 2016, NPAFC Doc. 1771. Washington, DC: National Oceanic and Atmospheric Administration.
Fergusson, E., Miller, T., McPhee, M. V., Fugate, C., and Schultz, H. (2020). Trophic responses of juvenile Pacific salmon to warm and cool periods within inside marine waters of Southeast Alaska. Prog. Oceanogr. 186:102378.
Fung, I. Y., Meyn, S. K., Tegen, I., Doney, S. C., John, J. G., and Bishop, J. K. B. (2000). Iron supply and demand in the upper ocean. Glob. Biogeochem. Cycles 14, 281–295. doi: 10.1029/1999gb900059
Gagnon, K., Rothäusler, E., Syrjänen, A., Yli-Renko, M., and Jormalainen, V. (2013). Seabird guano fertilizes Baltic Sea littoral food webs. PLoS One 8:e61284. doi: 10.1371/journal.pone.0061284
Gaston, K. J., Cox, D. T. C., Canavelli, S. B., García, D., Hughes, B., Maas, B., et al. (2018). Population abundance and ecosystem service provision: the case of birds. BioScience 68, 264–272. doi: 10.1093/biosci/biy005
Gende, S., Miller, A., and Hood, E. (2007). The effects of salmon carcasses on soil nitrogen pools in a riparian forest of southeastern Alaska. Can. J. For. Res. 37, 1194–1202. doi: 10.1139/x06-318
Hanselman, D., Pyper, B. J., and Peterson, M. (2018). Sperm whale depredation on longline surveys and implications for the assessment of Alaska sablefish. Fish. Res. 200, 75–83.
Hanselman, D., Rodgveller, C., Fenske, K. H., Shotwell, K., Echave, K. B., Malecha, P. W., et al. (2019). “Assessment of the sablefish stock of Alaska,” in Stock Assessment and Fishery Evaluation Report for the Groundfish Fisheries of the Gulf of Alaska and Bering Sea/Aleutian Islands Area, eds B. E. Fissel, M. Dalton, and R. G. Felthoven (Anchorage, AK: North Pacific Fishery Management Council) doi: 10.1016/j.fishres.2017.12.017
Harding, A. M., Piatt, J. F., Schmutz, J. A., Shultz, M. T., Pelt, T. I., Kettle, A. B., et al. (2007). Prey density and the behavioral flexibility of a marine predator: the common murre (Uria aalge). Ecology 88, 2024–2033. doi: 10.1890/06-1695.1
Harvey, C. J., Reum, J. C. P., Poe, M. R., Williams, G. D., and Kim, S. J. (2016). Using conceptual models and qualitative network models to advance integrative assessments of marine ecosystems. Coast. Manag. 44, 486–503.
Harvey, C., Garfield, N., Williams, G. D., Tolimieri, N., Andrews, K. S., Barnas, K. A., et al. (2020). Ecosystem Status Report of the California Current for 2019-20: A Summary of Ecosystem Indicators Compiled by the California Current Integrated Ecosystem Assessment Team (CCIEA). NOAA Technical Memorandum NMFS-NWFSC 160. Washington, DC: National Oceanic and Atmospheric Administration.
Helfield, J. M., and Naiman, R. J. (2006). Keystone interactions: salmon and bear in riparian forests of Alaska. Ecosystems 9, 167–180.
Hentati-Sundberg, J., Raymond, C., Sköld, M., Svensson, O., Gustafsson, B., and Bonaglia, S. (2020). Fueling of a marine-terrestrial ecosystem by a major seabird colony. Sci. Rep. 10:15455. doi: 10.1038/s41598-020-72238-6
Hill, P. S., Laake, J. L., and Mitchell, E. D. (1999). Results of a Pilot Program to Document Interactions Between Sperm Whales and Longline Vessels in Alaskan Waters, U.S. Dep. Commer., NOAA Tech. Memo.NMFS-AFSC-108. Washington, DC: National Oceanic and Atmospheric Administration.
Honig, S. E., and Mahoney, B. (2016). Evidence of seabird guano enrichment on a coral reef in Oahu, Hawaii. Mar. Biol. 163:22.
Hood, E., and Berner, L. (2009). Effects of changing glacial coverage on the physical and biogeochemical properties of coastal streams in southeastern Alaska. J. Geophys. Res. 114:G03001.
Hood, E., and Scott, D. (2008). Riverine organic matter and nutrients in southeast Alaska affected by glacial coverage. Nat. Geosci. 1, 583–587. doi: 10.1038/ngeo280
Hop, H., Pearson, T., Hegseth, E. N., Kovacs, K. M., Wiencke, C., Kwaśniewski, S., et al. (2002). The marine ecosystem of Kongsfjorden, Svalbard. Polar Res. 21, 167–208.
Ikeda, T., and Motoda, S. (1978). Estimated zooplankton production and their ammonia excretion in the Kuroshio and adjacent seas. Fish. Bull. 76, 357–367.
IPHC (2014). The Pacific Halibut: Biology, Fishery, and Management, Technical Report No. 59. Seattle, WA: IPHC.
Ivashchenko, Y. V., and Clapham, P. J. (2014). Too much is never enough: the cautionary tale of Soviet illegal whaling. Mar. Fish. Rev. 76, 1–21.
Ivashchenko, Y. V., Clapham, P. J., and Brownell, R. L. Jr. (2011). Soviet illegal whaling: the devil and the details. Mar. Fish. Rev. 73, 1–19. doi: 10.7755/mfr.76.1_2.1
Jefferson, T. A., Webber, M. A., and Pitman, R. L. (2015). Marine Mammals of the World: A Comprehensive Guide to Their Identification, second Edn. San Diego, CA: Academic Press.
Jurasz, C. M., and Jurasz, V. P. (1979). Feeding modes of the humpback whales, Megaptera novaeangliae, in Southeast Alaska. Sci. Rep. Whales Res. Inst. 31, 69–83.
Kolb, G. S., Ekholm, J., and Hambäck, P. A. (2010). Effects of seabird nesting colonies on algae and aquatic invertebrates in coastal waters. Mar. Ecol. Prog. Ser. 417, 287–300. doi: 10.3354/meps08791
Kooyman, G. L., Castellini, M. A., and Davis, R. W. (1981). Physiology of diving in marine mammals. Annu. Rev. Physiol. 43, 343–356.
Krieger, K. G., and Wing, B. L. (1986). Hydroacoustic Monitoring of Prey to Determine Humpback Whale Movements. NOAA Technical Memorandum NMFS F/NWC-98. Juneau, AK: National Marine Fisheries Service.
Lang, G., Livingston, P., and Dodd, K. (2003). Groundfish Food Habits and Predation on Commercially Important Prey Species in the Eastern Bering Sea From 1997 Through 2001. AFSC Processed Report 2003–04. Washington, DC: NOAA.
Lavery, T. J., Roudnew, B., Gill, P., Seymour, J., Seuront, L., Johnson, G., et al. (2010). Iron defecation by sperm whales stimulates carbon export in the Southern Ocean. Proc. R. Soc. B Biol. Sci. 277, 3527–3531. doi: 10.1098/rspb.2010.0863
Lavery, T. J., Roudnew, B., Seymour, J., Mitchell, J. G., Smetacek, V., and Nicol, S. (2014). Whales sustain fisheries: blue whales stimulate primary production in the Southern Ocean. Mar. Mamm. Sci. 30, 888–904. doi: 10.1111/mms.12108
Levin, P. S., Fogarty, M. J., Murawski, S. A., and Fluharty, D. (2009). Integrated ecosystem assessments: developing the scientific basis for ecosystem-based management of the ocean. PLoS Biol. 7:e1000014. doi: 10.1371/journal.pbio.1000014
Lorrain, A., Houlbrèque, F., Benzoni, F., Barjon, L., Tremblay-Boyer, L., Menkes, C. E., et al. (2017). Seabirds supply nitrogen to reef-building corals on remote Pacific islets. Sci. Rep. 7:3721. doi: 10.1038/s41598-017-03781-y
Marasco, R. J., Goodman, D., Grimes, C. B., Lawson, P. W., Punt, A. E., and Quinn, T. J. II (2007). Ecosystem-based fisheries management: some practical suggestions. Can. J. Fish. Aquat. Sci. 64, 928–939. doi: 10.1139/f07-062
Marston, B. H., Willson, M. F., and Gende, S. (2002). Predator aggregations during eulachon Thaleichthys pacificus spawning runs. Mar. Ecol. Prog. Ser. 231, 229–236. doi: 10.3354/meps231229
McNaughton, S. J., Banyikwa, F. F., and McNaughton, M. M. (1997). Promotion of the cycling of diet-enhancing nutrients by African grazers. Science 278, 1798–1800. doi: 10.1126/science.278.5344.1798
Melbourne-Thomas, J., Wotherspoon, S., Raymond, B., and Constable, A. (2012). Comprehensive evaluation of model uncertainty in qualitative network analyses. Ecol. Monogr. 82, 505–519. doi: 10.1890/12-0207.1
Moran, J. R., Heintz, R. A., Straley, J. M., and Vollenweider, J. J. (2018). Regional variation in the intensity of humpback whale predation on Pacific herring in the Gulf of Alaska. Deep Sea Res. Part II Top. Stud. Oceanogr. 147, 187–195. doi: 10.1016/j.dsr2.2017.07.010
Morissette, L., Christensen, V., and Pauly, D. (2012). Marine mammal impacts in exploited ecosystems: would large scale culling benefit fisheries? PLoS One 7:e43966. doi: 10.1371/journal.pone.0043966
Muto, M. M., Helker, V. T., Delean, B. J., Angliss, R. P., Boveng, P. L., Breiwick, J. M., et al. (2020). Alaska Marine Mammal Stock Assessments, 2019, U.S. Dep. Commer., NOAA Tech. Memo. NMFS-AFSC-404. Washington, DC: NOAA.
National Oceanic and Atmospheric Administration (NOAA) (2016). Endangered and Threatened Species; Identification of 14 Distinct Population Segments of the Humpback Whale (Megaptera novaeangliae) and Revision of Species-Wide Listing. Washington, DC: U.S. Department of Commerce.
Nicol, S., Bowie, A., Jarman, S., Lannuzel, D., Meiners, K. M., and Van Der Merwe, P. (2010). Southern Ocean iron fertilization by baleen whales and Antarctic krill. Fish Fish. 11, 203–209. doi: 10.1111/j.1467-2979.2010.00356.x
O’Connor, S., Campbell, R., Cortez, H., and Knowles, T. (2009). Whale Watching Worldwide: Tourism Numbers, Expenditures and Expanding Economic Benefits, A Special Report From the International Fund for Animal Welfare. Yarmouth, MA: International Fund for Animal Welfare (IFAW).
Öztürk, M., and Bizsel, N. (2003). Iron speciation and biogeochemistry in different nearshore water. Mar. Chem. 83, 145–156. doi: 10.1016/s0304-4203(03)00108-7
Paul, A. J., Coyle, K. O., and Ziemann, D. A. (1990). Timing of spawning of Thysanoessa raschii (Euphausiacea) and occurrence of their feeding-stage larvae in an Alaskan bay. J. Crustacean Biol. 10, 69–78. doi: 10.1163/193724090x00258
Peterson, M., and Carothers, C. (2013). Whale interactions with Alaskan sablefish and Pacific halibut fisheries: surveying fishermen perception, changing fishing practices and mitigation. Mar. Policy 42, 315–324. doi: 10.1016/j.marpol.2013.04.001
Peterson, M., Mueter, F., Criddle, K., and Haynie, A. C. (2014). Killer whale depredation and associated costs to Alaskan sablefish, Pacific halibut and Greenland turbot longliners. PLoS One 9:e88906. doi: 10.1371/journal.pone.0088906
Pirtle, J. L., Eckert, G. L., and Stoner, A. W. (2012). Habitat structure influences the survival and predator-prey interactions of early juvenile red king crab Paralithodes camtschaticus. Mar. Ecol. Prog. Ser. 465, 169–184. doi: 10.3354/meps09883
Poulsen, J., Rosin, C., Meier, A., Mills, E., Nuñez, C. L., Koerner, S. E., et al. (2017). The ecological consequences of forest elephant declines for Afrotropical forests. Conserv. Biol. 32, 559–567. doi: 10.1111/cobi.13035
Puccia, C. J., and Levins, R. (1985). Qualitative Modelling of Complex Systems: An Introduction to Loop Analysis and Time Averaging. Cambridge, MA: Harvard University Press.
Quinn, T., Carlson, S., Gende, S., and Rich, H. (2009). Quinn TP, Carlson SM, Gende SM, Rich HB. Transportation of Pacific salmon carcasses from streams to riparian forests by bears. Can. J. Zool. 87, 195–203. doi: 10.1139/z09-004
R Core Team (2019). R: A Language and Environment for Statistical Computing. Vienna: R Foundation for Statistical Computing.
Ratnarajah, L., Bowie, A. R., Lannuzel, D., Meiners, K. M., and Nicol, S. (2014). The biogeochemical role of baleen whales and krill in Southern Ocean nutrient cycling. PLoS One 9:e114067. doi: 10.1371/journal.pone.0114067
Ratnarajah, L., Melbourne-Thomas, J., Marzloff, M. P., Lannuzel, D., Meiners, K. M., Chever, F., et al. (2016). A preliminary model of iron fertilisation by baleen whales and Antarctic krill in the Southern Ocean: sensitivity of primary productivity estimates to parameter uncertainty. Ecol. Modell. 320, 203–212. doi: 10.1016/j.ecolmodel.2015.10.007
Raven, J. A. (1988). The iron and molybdenum use efficiencies of plant growth with different energy, carbon and nitrogen sources. New Phytol. 109, 279–287. doi: 10.1111/j.1469-8137.1988.tb04196.x
Raymond, B., McInnes, J., Dambacher, J. M., Way, S., and Bergstrom, D. M. (2011). Qualitative modelling of invasive species eradication on subantarctic Macquarie Island. J. Appl. Ecol. 48, 181–191. doi: 10.1111/j.1365-2664.2010.01916.x
Reum, J. C., McDonald, P. S., Ferriss, B. E., Farrell, D. M., Harvey, C. J., and Levin, P. S. (2015). Qualitative network models in support of ecosystem approaches to bivalve aquaculture. ICES J. Mar. Sci. 72, 2278–2288. doi: 10.1093/icesjms/fsv119
Roman, J., and McCarthy, J. J. (2010). The whale pump: marine mammals enhance primary productivity in a coastal basin. PLoS One 5:e13255. doi: 10.1371/journal.pone.0013255
Roman, J., Estes, J. A., Morissette, L., Smith, C., Costa, D., McCarthy, J., et al. (2014). Whales as marine ecosystem engineers. Front. Ecol. Environ. 12:377–385. doi: 10.1890/130220
Roman, J., Nevins, J., Altabet, M., Koopman, H., and McCarthy, J. (2016). Endangered right whales enhance primary productivity in the Bay of Fundy. PLoS One 11:e0156553. doi: 10.1371/journal.pone.0156553
Rosellon-Druker, J., Szymkowiak, M., Aydin, K. Y., Cunningham, C. J., Fergusson, E. A., Kasperski, S., et al. (2020). Participatory place-based integrated ecosystem assessment in Sitka, Alaska: constructing and operationalizing a socio-ecological conceptual model for sablefish (Anoplopoma fimbria). Deep Sea Res. Part II Top. Stud. Oceanogr. 18:104912.
Ruzicka, J. J., Steele, J. H., Ballerini, T., Gaichas, S. K., and Ainley, D. G. (2013). Dividing up the pie: whales, fish, and humans as competitors. Prog. Oceanogr. 116, 207–219. doi: 10.1016/j.pocean.2013.07.009
Scherer, R. D., Doll, A. C., Rea, L. D., Christ, A. M., Stricker, C. A., Witteveen, B., et al. (2015). Stable isotope values in pup vibrissae reveal geographic variation in diets of gestating Steller sea lions Eumetopias jubatus. Mar. Ecol. Prog. Ser. 527, 261–274. doi: 10.3354/meps11255
Schreiber, E. A., and Burger, J. (2001). Biology of Marine Birds, 1st Edn. Boca Raton, FL: CRC Press.
Shatova, O., Wing, S., Gault-Ringold, M., Wing, L., and Hoffmann, L. (2016). Seabird guano enhances phytoplankton production in the Southern Ocean. J. Exp. Mar. Biol. Ecol. 483, 74–87.
Sidle, R. C., and Shaw, C. G. III (1983). Evaluation of planting sites common to a southeast Alaska clear-cut. I. Nutrient status. Can. J. For. Res. 13, 1–8.
Sigler, M. F., Lunsford, C. R., Straley, J. M., and Liddle, J. B. (2008). Sperm whale depredation of sablefish longline gear in the northeast Pacific Ocean. Mar. Mamm. Sci. 24, 16–27. doi: 10.1111/j.1748-7692.2007.00149.x
Sigler, M., Womble, J., and Vollenweider, J. (2004). Availability to Steller sea lions (Eumetopias jubatus) of a seasonal prey resource: a prespawning aggregation of eulachon (Thaleichthys pacificus). Can. J. Fish. Aquat. Sci. 61, 1475–1484.
Sinclair, E. H., and Zeppelin, T. K. (2002). Seasonal and spatial differences in diet in the western stock of steller sea lions (Eumetopias Jubatus). J. Mammal. 83, 973–990. doi: 10.1644/1545-1542(2002)083<0973:sasdid>2.0.co;2
Smith, L., McMinn, A., Martin, A., Nicol, S., Bowie, A., Lannuzel, D., et al. (2013). Preliminary investigation into the stimulation of phytoplankton photophysiology and growth by whale faeces. J. Exp. Mar. Biol. Ecol. 446, 1–9. doi: 10.1016/j.jembe.2013.04.010
Springer, A. M., Estes, J. A., van Vliet, G. B., Williams, T. M., Doak, D. F., Danner, E. M., et al. (2003). Sequential megafaunal collapse in the North Pacific Ocean: an ongoing legacy of industrial whaling? Proc. Natl. Acad. Sci. U.S.A. 100:12223. doi: 10.1073/pnas.1635156100
Stabeno, P. J., Bond, N. A., Hermann, A. J., Kachel, N. B., Mordy, C. W., and Overland, J. E. (2004). Meteorology and oceanography of the Northern Gulf of Alaska. Cont. Shelf Res. 24, 859–897. doi: 10.1016/j.csr.2004.02.007
Stoner, A. (2009). Habitat-mediated survival of newly settled red king crab in the presence of a predatory fish: role of habitat complexity and heterogeneity. J. Exp. Mar. Biol. Ecol. 382, 54–60. doi: 10.1016/j.jembe.2009.10.003
Straley, J. M., Moran, J. R., Boswell, K. M., Vollenweider, J. J., Heintz, R. A., and Quinn, T. J. II, et al. (2018). Seasonal presence and potential influence of humpback whales on wintering Pacific herring populations in the Gulf of Alaska. Deep Sea Res. Part II Top. Stud. Oceanogr. 147, 173–186. doi: 10.1016/j.dsr2.2017.08.008
Sturdevant, M. V., Orsi, J. A., and Fergusson, E. A. (2012). Diets and trophic linkages of epipelagic fish predators in coastal Southeast Alaska during a period of warm and cold climate years. Mar. Coast. Fish. 4, 526–545.
Sugai, S. F., and Burrell, D. C. (1984). Transport of dissolved organic carbon, nutrients, and trace metals from the Wilson and Blossom Rivers to Smeaton Bay, Southeast Alaska. Can. J. Fish. Aquat. Sci. 41, 180–190.
Surma, S., and Pitcher, T. (2015). Predicting the effects of whale population recovery on Northeast Pacific food webs and fisheries: an ecosystem modelling approach. Fish. Oceanogr. 24, 291–305. doi: 10.1111/fog.12109
Szabo, A. R., and Batchelder, H. P. (2014). Late spring and summer patterns of euphausiid reproduction in Southeast Alaska fjord waters. Mar. Ecol. Prog. Ser. 516, 153–161. doi: 10.3354/meps11003
Szymkowiak, M., and Rhodes-Reese, M. (2020). Adaptive behaviors to marine ecosystem shifts: examining fishermen’s strategies in response to abundant juvenile sablefish (Anoplopoma fimbria) in Alaska. Front. Mar. Sci. 7:602281. doi: 10.3389/fmars.2020.602281
Theobald, M., Crittenden, P., Hunt, A., Tang, S., Dragosits, U., and Sutton, M. A. (2006). Ammonia emissions from a Cape fur seal colony, Cape Cross, Namibia. Geophys. Res. Lett. 33:L03812.
Thomas, A. C., Nelson, B. W., Lance, M. M., Deagle, B. E., and Trites, A. W. (2016). Harbour seals target juvenile salmon of conservation concern. Can. J. Fish. Aquat. Sci. 74, 907–921.
Tinker, M. T., Gill, V. A., Esslinger, G. G., Bodkin, J., Monk, M., Mangel, M., et al. (2019). Trends and carrying capacity of sea otters in Southeast Alaska. J. Wildl. Manag. 83, 1073–1089.
Wainright, S. C., Haney, J. C., Kerr, C., Golovkin, A. N., and Flint, M. V. (1998). Utilization of nitrogen derived from seabird guano by terrestrial and marine plants at St. Paul, Pribilof Islands, Bering Sea, Alaska. Mar. Biol. 131, 63–71. doi: 10.1007/s002270050297
Weingartner, T., Eisner, L., Eckert, G. L., and Danielson, S. (2009). Southeast Alaska: oceanographic habitats and linkages. J. Biogeogr. 36, 387–400. doi: 10.1111/j.1365-2699.2008.01994.x
Weitkamp, L. A., and Sturdevant, M. V. (2008). Food habits and marine survival of juvenile Chinook and coho salmon from marine waters of Southeast Alaska. Fish. Oceanogr. 17, 380–395. doi: 10.1111/j.1365-2419.2008.00485.x
Whelan, C. J., Wenny, D. G., and Marquis, R. J. (2008). Ecosystem services provided by birds. Ann. N. Y. Acad. Sci. 1, 25–60.
Willson, M. F., Gende, S. M., and Marston, B. H. (1988). Fishes and the forest. BioScience 48, 455–462.
Willson, M., and Womble, J. (2006). Vertebrate exploitation of pulsed marine prey: a review and the example of spawning herring. Rev. Fish Biol. Fish. 16, 183–200. doi: 10.1007/s11160-006-9009-7
Wing, S., Jack, L., Shatova, O., Leichter, J., Barr, D., Frew, R., et al. (2014). Seabirds and marine mammals redistribute bioavailable iron in the Southern Ocean. Mar. Ecol. Prog. Ser. 510, 1–13. doi: 10.3354/meps10923
Womble, J., and Sigler, M. (2006). Seasonal availability of abundant, energy-rich prey influences the abundance and diet of a marine predator, the Steller sea lion Eumetopias jubatus. Mar. Ecol. Prog. Ser. 325, 281–293.
Woodby, D., Carlile, D., Siddeek, S., Funk, F., Clark, J. H., and Hulbert, L. (2005). Commercial Fisheries of Alaska. Alaska Department of Fish and Game, Special Publication No. 05-09. Juneau, AK: Alaska Department of Fish and Game.
Worm, B., Barbier, E. B., Beaumont, N., Duffy, J. E., Folke, C., Halpern, B. S., et al. (2006). Impacts of biodiversity loss on ocean ecosystem services. Science 314:787.
Yasumiishi, E. M., Shotwell, K., Hanselman, D., Orsi, J., and Fergusson, E. (2014). “Southeast coastal monitoring survey indices and the recruitment of Gulf of Alaska sablefish,” in Ecosystem Considerations for 2014. Appendix C of the BSAI/GOA Stock Assessment and Fishery Evaluation Report. Technical Report, ed. S. Zador (Anchorage, AK: North Pacific Fishery Management Council).
Zador, S. G., Gaichas, S. K., Kasperski, S., Ward, C. L., Blake, R. E., Ban, N. C., et al. (2017). Linking ecosystem processes to communities of practice through commercially fished species in the Gulf of Alaska. ICES J. Mar. Sci. 74, 2024–2033. doi: 10.1093/icesjms/fsx054
Zador, S. G., Yasumiishi, E., and Whitehouse, G. A. (2019). Ecosystem Status Report 2019: Gulf of Alaska. Anchorage, AK: North Pacific Fishery Management Council, 99501.
Keywords: nutrient cycling, marine mammal, seabird, ecosystem-based management, qualitative network model, Alaska
Citation: Rhodes-Reese M, Clay D, Cunningham C, Moriles-Miller J, Reese C, Roman J, Warren JD and Pearson HC (2021) Examining the Role of Marine Mammals and Seabirds in Southeast Alaska’s Marine Ecosystem Dynamics. Front. Mar. Sci. 8:720277. doi: 10.3389/fmars.2021.720277
Received: 04 June 2021; Accepted: 03 December 2021;
Published: 22 December 2021.
Edited by:
Tomaso Fortibuoni, Istituto Superiore per la Protezione e la Ricerca Ambientale (ISPRA), ItalyReviewed by:
Jamie N. Womble, National Park Service, United StatesNor Azman Kasan, University of Malaysia Terengganu, Malaysia
Robert Suryan, Alaska Fisheries Science Center, National Oceanic and Atmospheric Administration (NOAA), United States
Copyright © 2021 Rhodes-Reese, Clay, Cunningham, Moriles-Miller, Reese, Roman, Warren and Pearson. This is an open-access article distributed under the terms of the Creative Commons Attribution License (CC BY). The use, distribution or reproduction in other forums is permitted, provided the original author(s) and the copyright owner(s) are credited and that the original publication in this journal is cited, in accordance with accepted academic practice. No use, distribution or reproduction is permitted which does not comply with these terms.
*Correspondence: Melissa Rhodes-Reese, bXJyaG9kZXNyZWVzZUBnbWFpbC5jb20=