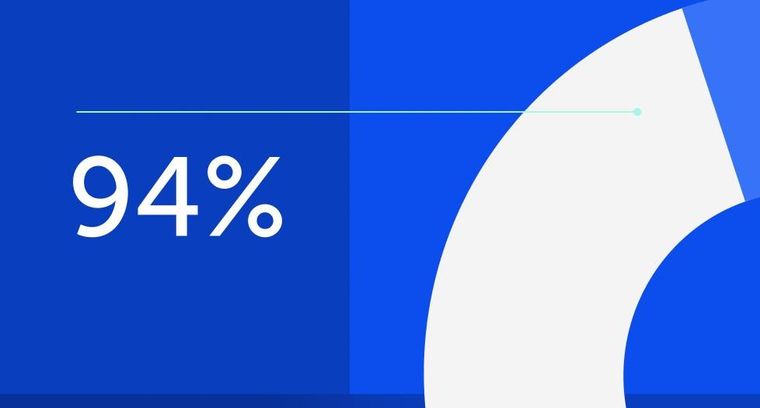
94% of researchers rate our articles as excellent or good
Learn more about the work of our research integrity team to safeguard the quality of each article we publish.
Find out more
SYSTEMATIC REVIEW article
Front. Mar. Sci., 30 August 2021
Sec. Global Change and the Future Ocean
Volume 8 - 2021 | https://doi.org/10.3389/fmars.2021.714462
Anthropogenic climate change is a significant driver of change in marine ecosystems globally. To improve mechanistic understanding of the impact of climate-related stressors, experimental work on marine organisms has intensified in recent decades. A previous synthesis paper published nearly a decade ago established that Marine Climate Change Experiments (MCCEs) published from 2000–2009 were primarily laboratory-based and focused on single stressors and individual focal temperate species. Using consistent methodology, we compared the 2000–2009 analysis to experiments published in the following decade (i.e. 2010–2019) to assess recent trends in MCCEs and to determine to what extent knowledge gaps and research priorities have been addressed. The search returned 854 papers, vs. 110 from the 2000s, indicating considerable intensification of research effort and output. We found again that single species studies were most common, particularly with benthic invertebrates as model organisms, and that laboratory-based research comprised over 90% of all studies. However, multiple stressor experiments increased substantially, where tests for interaction effects between ocean acidification (i.e., increased pCO2) and warming were particularly common. Furthermore, a wider range of model species were studied and more community-level experiments were conducted in the 2010s compared with the 2000s. In addition, studies on behavioral responses, transgenerational effects, genetic adaptation and extreme climatic events increased markedly. These recent advances in MCCEs have undoubtedly improved understanding of how climate change will affect marine organisms and the communities and ecosystems they underpin. Going forward, biases in the type and distribution of model organisms should be addressed to enhance general understanding of responses to environmental change. Similarly, experiments should manipulate a greater number and range of climate and non-climate factors and increase the number of target organisms to increase realism. Finally, where possible, further research should be combined and contextualized with field-based experiments and observations to better reflect the complexity of marine ecosystems and yield more representative responses to ocean climate change.
Anthropogenic climate change is recognized as a serious threat to natural systems and human societies across the world (IPCC, 2014; Lee et al., 2015; Pecl et al., 2017). Changes to the Earth's climate over time have modified properties of the ocean. For example, the global ocean has absorbed over 90% of the excess heat generated by atmospheric warming and ~30% of carbon dioxide emissions (IPCC, 2013, 2019). To date, burning of fossil fuels has led to a 0.89°C increase in the global average sea surface temperature from 1901–2012, a decrease of 0.1 pH units since preindustrial era and reduced dissolved oxygen concentrations (Andrews et al., 2013; IPCC, 2013, 2019). These stressors are predicted to intensify over this century (IPCC, 2014, 2019) with major implications for marine ecosystems, including species range shifts (Perry et al., 2005; Cheung et al., 2009; Last et al., 2011; Wernberg et al., 2011; Poloczanska et al., 2013), local extinctions (Parmesan, 2006; Cheung et al., 2009; Smale and Wernberg, 2013; Wernberg et al., 2016; Thomsen et al., 2019), biodiversity losses (Sala and Knowlton, 2006; Wernberg et al., 2013) and disruption to ecosystem structure and functioning (Sala and Knowlton, 2006; Worm et al., 2006; Doney et al., 2012).
Lowered pH, high temperature, reduced oxygen concentration and food availability are the four principal climate change stressors that have affected marine ecosystem structure and functioning and the adaptive capacity of marine biota (Duarte, 2014; IPCC, 2014). However, for ecosystems to be conserved and managed more effectively, a deeper mechanistic understanding of the impacts of co-occurring stressors have on organisms and communities is required (Parmesan, 2006; Benton et al., 2007; Poloczanska et al., 2013). Current evidence for climate change impacts should be underpinned by scientific experiments because only experimental approaches can reveal a cause-effect relationship, of crucial importance to predicting future changes (Tilman, 1989; Underwood, 1996; Sutherland, 2006).
A literature review by Wernberg et al. (2012) identified 110 Marine Climate Change Experiments (MCCEs) published in the decade spanning 2000 to 2009. The review revealed that studies published in this decade demonstrated that manipulated and controlled climate change test-factors can impact marine organisms in numerous ways, for example causing increased mortality (Anestis et al., 2007), increased coral bleaching (Anthony et al., 2008), decreased calcification rates (Gazeau et al., 2007), impaired growth and development (Berge et al., 2006, Arnold et al., 2009) and altered community structure (Dashfield et al., 2008) and ecosystem functioning (Sommer and Lengfellner, 2008). From 2000 to 2009, there was a steady year-on-year increase in the number of MCCEs published. The review also highlighted a marked publication bias toward laboratory-based experiments, particularly those that examined impacts of a single climate change variable and a single species at a time, and that focused primarily on model organisms from temperate climatic regions. In addition, over 40% of papers had an element of pseudoreplication or experimental caveat, such as using multiple aquaria within a single (unreplicated) large tank with elevated pCO2 (e.g., Dupont et al., 2008) or temperature (e.g., Peck et al., 2008). The study concluded that progress in five areas were required to achieve a more holistic, accurate and representative assessment of how climate change affects individuals, populations and communities. The five areas proposed were: (i) conduct experiments on multiple stressors; (ii) examine a wider range of species to represent more climatic regions and different types of study organism, (iii) conduct multi-species/community-level experiments to incorporate ecological interactions; (iv) increase replication and representativeness of experimental treatments; and (v) conduct more in situ experiments.
We followed the procedure and analysis of Wernberg et al. (2012) to examine the most recent decade of MCCEs (2010–2019) to determine the extent to which the knowledge gaps identified have been addressed.
The methods used in this study were largely consistent with the initial study (Wernberg et al., 2012) to allow for direct comparisons between the two time periods. MCCEs were collated by searching Web of Science, Google Scholar and reference lists from peer-reviewed papers, limited to studies published from 2010 to 2019. Search terms included combinations of “marine,” “climate change,” “temperature,” “warming,” “heatwave,” “ocean acidification,” “CO2,” “carbon dioxide,” “deoxygenation” and “stressors.” Only papers clearly stating climate change as the motivation for the study were included. Papers were also included only if the climate variables were experimentally manipulated in the field or laboratory. For example, time-series analyses, studies purely using modeling or natural gradients in the environment (e.g., volcanic vents) were not examined. The climatic variables of interest were temperature, pCO2/pH and other climate change related stressors reasoned by the authors, such as UV, oxygen concentration and food availability. Papers were also limited to include only those examining biological responses at the individual, population or community level, rather than sub-organismal level or below, in order to focus on response variables that can be more reliably used to infer ecological relevance.
The details from each study were extracted and papers were categorized based on key characteristics as in Wernberg et al. (2012). These were: (i) the type of climate variables that were manipulated; (ii) the climatic region in which the targeted biota belonged; (iii) the type of organisms (zooplankton included only holoplanktonic species, whilst larval stages of benthic invertebrates and fishes were placed under these categories, respectively); and (iv) the number of species studied. The year the paper was published, the main methods, results and whether the study was laboratory, or field-based was also recorded. In addition to the study characteristics evaluated in Wernberg et al. (2012) we conducted a further analysis by categorizing studies that examined the effects of extreme climatic events (ECEs, like marine heatwaves), or assessed transgenerational responses (TG, such as acclimation to future conditions experienced by parental generation), hereditable genetic adaptation (Adaptation) or behavioral responses (BR, such as escape responses and food preferences). These categories were added to our analysis and attributes were also extracted retrospectively from the 2000–2009 papers, because ECEs have emerged as one of the key manifestations of recent climate change in the past decade (e.g., Hobday et al., 2016) and TGR, adaptation and BR have been identified as important aspects of climate change impacts (Munday et al., 2013; Sunday et al., 2014; Donelson et al., 2018; Wang and Wang, 2020). For each category, a χ2 -test was performed to examine if frequencies differed significantly between decades or particular characteristic.
During the decade 2010 to 2019, 854 papers describing MCCEs met the criteria for inclusion this study (Supplementary Table 1). This was significantly more papers than the previous decade (χ2 = 574.2, P < 0.0001), with over a seven-fold increase. A maximum of 130 papers were published in a single year (2017) compared with 39 published in 2010. The first decade of the millennia saw a consistent increase in publications each year from just two papers in 2000 to 37 in 2009 (Wernberg et al., 2012, Figure 1). For the 2010s, although we found a significant difference in the frequency of studies per year (Figure 1A, χ2 = 115.7, P < 0.0001), we did not observe a constant year-on-year increase in publications. Overall, however, there has been a steady increase in the cumulative number of MCCE publications through the 2010s, whereas in the 2000s a sharp increase in publication rate occurred in the latter few years of the decade (Figure 1).
Figure 1. (A) Frequency of Marine Climate Change Experiments (MCCEs) published each year of two decades (2000–2009 vs. 2010–2019) and (B) the cumulative frequency of publications during each past decade.
MCCEs in the 2000s were predominantly single factor experiments, particularly the experiments that tested for effects of ocean acidification (Wernberg et al., 2012, Figure 2). During the 2010s, single-factor ocean acidification experiments continued to be the most prominent studied published with 284 papers (33.2%). However, studies on the combined effect of elevated temperature and ocean acidification dramatically increased, becoming the second-most abundant type of MCCEs (Figure 2A, χ2 = 93.0, P < 0.0001), with 275 papers; i.e., more than the total amount of MCCE papers published during the previous decade. This represented more than a 30% increase in the proportion of studies addressing the interaction between these two climatic factors (χ2 = 11.7, P < 0.001) (Figure 3). Despite this increase in number of multifactorial experiments, single factor experiments that manipulated only temperature or ocean acidification, still accounted for 44% of studies published between 2010 and 2019. Although MCCEs that manipulated three or more factors accounted for just ~8% of experiments in the 2010s (Figure 3), this approach still represented a 14-fold increase from the previous decade (Figure 2).
Figure 2. Characteristics of Marine Climate Change Experiments (MCCEs) published in two decades (2000–2009 vs. 2010–2019), shown as actual number of papers in each decade. Studies were classified by (A) the climate variables that was experimentally manipulated, (B) the climatic region of which the study organism(s) belonged, (C) the type of organism (experiments with multiple types were recorded more than once) and (D) the number of species studied. In panel (A) “Temp” = temperature, “OA” = ocean acidification, “Oxygen” = deoxygenation experiments. In panel (D) numerals donate number of species/taxa studied, “comm” = community level experiment (as reported by authors).
Figure 3. Characteristics of Marine Climate Change Experiments (MCCEs) published in two decades (2000–2009 vs. 2010–2019), shown as a percentage of total research effort in each decade. Studies were classified by (A) the climate variables that was experimentally manipulated, (B) the climatic region of which the study organism(s) belonged, (C) the type of organism (experiments with multiple types were recorded more than once) and (D) the number of species studied. In panel (A) “Temp” = temperature, “OA” = ocean acidification, “Oxygen” = deoxygenation experiments. In panel (D) numerals donate number of species/taxa studied, “comm” = community level experiment (as reported by authors).
Studies using organisms from temperate regions continued to be the most prevalent during 2010–2019, with 62% of papers coming from this region (χ2 = 88.7, P < 0.0001), almost 10% higher than the proportion from 2000–2009 (Figure 3). Numerically however, studies on polar and tropical species increased with 59 and 237 papers, respectively, compared to 10 and 22 from the 2000s (Figure 2). Furthermore, benthic invertebrates were, again, the most commonly studied organisms, with 50% of studies published between 2010–2019 focusing on this group (Figure 3; χ2 = 80.0, P < 0.0001). The absolute number of studies on benthic invertebrates, however, increased from 80 to 427 between the two decades (Figure 2). Research on macrophytes and fish also intensified in the 2010s, with a 13 and 30-fold increase in publications, respectively (Figure 2). The latter comprised 18% of studies, significantly higher than 4.5% in the previous decade (Figure 3; χ2 = 8.0, P < 0.005). During the 2010s, single species experiments were again by far the most prevalent (χ2 = 73.2, P < 0.0001), although the absolute number of multi-species studies increased more than seven-fold, compared with the 2010s (Figure 2D). We tallied 47 studies with a field component, four-times as many compared to the 2000s, but laboratory-based experiments still accounted for over 90% of all publications (Supplementary Table 1) a similar proportion to that of the 2000s.
Most patterns in experimental efforts to understand the effects of climate change on marine organisms were consistent across the two decades, however, three types of MCCE not considered by Wernberg et al. (2012) have become markedly more prevalent during the 2010s (Figure 4). Specifically, experiments examining biological responses to extreme climatic events increased from <1% of papers in the 2000s (1 publication) to 2.7% in the 2010s (23 publications). Similarly, the proportion of studies that examined transgenerational effects of climate change or behavioral responses, doubled, to 4% and 12%, respectively, while the numerical number of papers increased by a factor of 11 and 17 (Figure 4). Furthermore, experiments on the heritability and genetic adaptation increased from zero papers in the 2010s to 30; 3.5% of the overall studies.
Figure 4. The frequency of papers with new Marine Climate Change Experiments (MCCEs) characteristics not considered in the original study by Wernberg et al. (2012) in two decades (2000–2009 vs. 2010–2019). Reviewed papers were grouped into studies that tested for impacts of extreme climatic events (ECE), assessed transgenerational responses (TG), examined genetic adaptation (Adapt) or measured behavioral responses (BR).
Over the last two decades, experimental research on biological responses to marine climate change has intensified dramatically, with a near eight-fold increase in publications in the 2010s compared to the 2000s. In the most recent decade, multiple stressor experiments proliferated (e.g., Russell et al., 2013; Falkenberg et al., 2015; Araújo et al., 2018) and more papers focused on underrepresented regions and taxa, such as polar organisms (e.g., Ericson et al., 2012; Kapsenberg and Hofmann, 2014; Spicer and Morley, 2019) and macrophytes (e.g., Andrews et al., 2014; Burnell et al., 2014; Repolho et al., 2017). Moreover, studies examining responses across generations, behavioral responses, and impacts of extreme climatic events have emerged rapidly since the initial study (e.g., Pistevos et al., 2015; Thor and Dupont, 2015; Leggat et al., 2019). This progression in the field of marine climate change ecology reflects the general increase in awareness of the importance of marine ecosystems (Duarte et al., 2013; Bennett et al., 2016) and the immediate and significant threats posed by climate change factors in many regions (e.g., Smale et al., 2013; Filbee-Dexter et al., 2019; Wernberg et al., 2019). Moreover, this study shows that significant advances have been made to address several of the key knowledge gaps and limitations identified by Wernberg et al. (2012).
Wernberg et al. (2012) highlighted the need to move toward MCCEs that manipulate multiple factors. Studies from the first decade of the millennium focused predominantly on manipulating one factor, particularly pCO2/pH levels, probably because this was considered the “novel” and understudied stressor at the time that was less understood than responses to elevated temperature (Wernberg et al., 2012). Whilst single-factor experiments were crucial to develop a mechanistic understanding of causal relationships, natural systems are continually exposed to multiple co-occurring human and non-human induced stressors (Breitburg et al., 1998; Gruber, 2011). Furthermore, the cumulative effects of two or more factors can be additive, synergistic or antagonistic, often with varying responses across different levels of biological organization (Crain et al., 2008). For example, some evidence suggests that organisms experience increased sensitivity to ocean acidification when concurrently exposed to elevated temperatures (Kroeker et al., 2013). From our literature review, it is clear that research effort over the last decade has focused more on experiments that assessed the combined effects of multiple stressors, including both climate and non-climate related factors (e.g., Burnell et al., 2013; Al-Janabi et al., 2019; McMahon et al., 2020). Despite this, single factor ocean acidification experiments, the “novel stressor” from the 2000s (Wernberg et al., 2012) continued to be prevalent in the 2010s.
Across the multiple stressor studies, ocean acidification and warming was the most common combination of test factors, which is unsurprising given the pervasive and global co-occurrence of these stressors (Halpern et al., 2015). Other “additional” stressors included reduced food availability (Cheng et al., 2018), increased metal contamination (Campbell et al., 2014), altered grazer presence (Alsterberg et al., 2013) and deoxygenation (Al-Janabi et al., 2019). Twelve papers focused on the effects of change in oxygen concentration independently and a further 19 papers on changes to oxygen levels in combination with temperature and/or ocean acidification. In total, 70 papers considered three or more concurrent variables, for example, temperature, salinity, light and parasite infection (Brakel et al., 2019), temperature, nutrient level and density (Brooks and Crowe, 2018) and temperature, acidification, salinity and food availability (Cole et al., 2016). These studies provided further evidence that interactions between organisms and their environment are complex and context-dependent, ranging from synergistic effects of multiple stressors on mortality (Araújo et al., 2018) to the amelioration of negative impacts when exposed to multiple stressors (Sheppard-Brennand et al., 2010, García et al., 2015).
Consistent with studies undertaken during the 2000s, the majority of studies published in the most recent decade focused on organisms from temperate regions, probably because more marine biological laboratories are located in North America, Europe and Australasia (Wernberg et al., 2012). Given that tropical coral reef systems and polar ecosystems may be particularly susceptible to climate change impacts (Gattusso et al., 2018), geographical bias toward temperate organisms may limit the evidence base for global threats to marine ecosystems. For example, Arctic biota may respond strongly to warming, as polar species tend to have narrow thermal ranges and have less opportunities to colonize higher latitudes (Poloczanska et al., 2016). Indeed, it has been shown that climate change factors strongly influence the development (Hildebrandt et al., 2014), growth (Koenker et al., 2018) and survival (Kapsenberg and Hofmann, 2014) of polar organisms. However, with only 7% of studies published in the 2010s focusing on polar species, significant knowledge gaps remain. Similarly, reef-building corals are increasingly exposed to thermal stress, which can lead to mass bleaching and mortality (Hughes et al., 2018; Skirving et al., 2019), and ocean acidification, which can stunt coral growth and reduce calcification rates (Albright et al., 2018). Consequently, 75% of coral reefs globally may be threatened by climate change (Burke et al., 2011). Despite the notable increase in experiments conducted on polar and tropical organisms since 2009, their vulnerability to climate changes makes it particularly pertinent to better understand how these species might respond to climate change, and more research effort is warranted.
Publications from the 2010s were, like the previous decade, strongly skewed toward experiments conducted on benthic invertebrates, which comprised 50% of all studies. This is not surprising because invertebrates represent the most abundant and diverse animal group and includes reef-building and habitat-forming corals and their associated biota (Glynn, 1993; Cesar et al., 2003; Bellwood et al., 2004; Burke et al., 2011). Moreover, invertebrates are found across all marine habitats and trophic levels, underpin core ecological processes, can be highly responsive to climate change, and many are small, have short life-spans, are accessible to researchers and are therefore easily amendable to experimentation (Prather et al., 2012). This focus may not reflect taxa most affected by climate change, however, as phytoplankton and bony fish are responding most rapidly to warming (Poloczanska et al., 2013; Montie et al., 2020), whilst macroalgae are particularly sensitive to climatic changes (Straub et al., 2019; Smale, 2020). Even so, the 2010s saw a noticeable increase in the number of experiments performed on fish, comprising 18% of all studies, while approximately 32% of papers focused on primary producers.
In recent decades, researchers, conservationists and policy makers have shifted their attentions in recent decades from protecting single species to conserving the structure and functioning of entire ecosystems (McLeod et al., 2005; Borja, 2014; Link and Browman, 2017). An ecosystem-based management approach represents a move away from examining individual mechanistic responses of a single species to a single stressor, toward understanding the holistic effects of multiple stressors on many organisms and their functioning within entire ecosystems (Christensen et al., 1996; McLeod et al., 2005; Curtin and Prellezo, 2010). Given that climate change stressors strongly influence intraspecific and interspecific ecological interactions (Kordas et al., 2011; Nagelkerken and Munday, 2016), more experiments should address effects on multiple species or entire communities to better understand how climate change impacts manifest in natural systems. Encouragingly, over six times as many studies from 2010–2019 involved experiments conducted on three or more organisms compared with 2000–2009. The dramatic increase in the number of studies involving multiple stressors and multiple species over the past couple of decades represents significant progress.
Despite the progress acknowledged thus far toward filling the knowledge gaps addressed by Wernberg et al. (2012), the proportion of studies involving field-based manipulative experiments has remained low and relatively constant, even if the number of studies more than doubled between the two decades. The advantage of laboratory experiments (including mesocosms) is that they provide a controlled environment, allowing one or more variables to be manipulated at a time, and thereby clearly demonstrating causal relationships. On the other hand, the simplicity and artificiality of some laboratory-based experiments limits their relevance to natural systems that operate at vastly different spatiotemporal scales (Carpenter, 1996; Stewart et al., 2013). Additionally, laboratory-based manipulations of CO2 have a history of inadequate replication (Cornwall and Hurd, 2016). Marine ecosystems are spatiotemporally dynamic, heterogeneous and complex, and only in situ experiments have the ability to fully capture this variability and test how climate change factors may affect a range of interactions between organisms and their environment, thereby obtaining more representative results and, ultimately, developing more realistic predictions. During the 2010s, heated settlement panels have been deployed to study in situ community responses to warming (Smale and Wernberg, 2012; Ashton et al., 2017; Smale et al., 2017; Clark et al., 2019), and Free Ocean Carbon Enrichment (FOCE) have tested for impacts of carbon dioxide on entire benthic communities (Barry et al., 2014; Cox et al., 2016; Albright et al., 2018; Kline et al., 2019). Even so, these approaches have major limitations, mostly pertaining to the spatiotemporal scales over which treatments can be applied, and issues with achieving adequate and true levels of replication. Clearly, manipulating climate change factors such as temperature and pCO2 in the marine environment is both logistically and conceptually challenging, more so than for terrestrial ecosystems (Arft et al., 1999; Hobbie et al., 2003), but progress in this area will undoubtedly broaden the inference space of MCCEs.
In addition to the temporal changes in research effort related to the categories of MCCEs examined by Wernberg et al. (2012), recent trends in other types of MCCEs are noteworthy. First, there has been a marked increase in the number of MCCEs framed within the context of extreme climatic events as drivers of ecological change. Extreme climatic events, such as marine heatwaves (Hobday et al., 2018), have intensified in recent decades (Coumou and Rahmstorf, 2012; Oliver et al., 2018), and are emerging as forceful agents of disturbance in marine ecosystems (Babcock et al., 2019; Smale et al., 2019). Appropriately, the number of experimental studies addressing marine heatwaves and their impacts has increased over the years with 23 papers examining the effect of acute, extreme warming on marine biota from 2010–2019, compared to only one from the previous decade (Ehlers et al., 2008). Second, the number of transgenerational experiments, which primarily tested if offspring can adapt and acclimate to climate changes if their parents are exposed to stress, increased more than 11-fold between the two decades. Transgenerational experiments from the most recent decade have demonstrated that exposure over generations can reduce the negative effects to ocean warming and acidification on offspring, for example by restoring aerobic scope (Donelson et al., 2016), body size (McMahon et al., 2020) and alter sex ratios (Donelson and Munday, 2015). However, transgenerational responses to climate change have also shown to be negligible (Uthicke et al., 2013) or negative (Welch et al., 2014; Griffith and Gobler, 2017; de Bettignies et al., 2018), demonstrating that these effects can be very complex and difficult to predict. Third, which partially overlaps with transgenerational effects, is the potential role of genetic adaptation in responses to marine climate change. Experiments from this decade revealed that there may be some potential for adaptation (Welch and Munday, 2017; Jury et al., 2019; Munday et al., 2019), although trade-offs are likely (Kelly et al., 2016). Experimental testing of evolutionary processes on marine organisms has been historically limited, due to methodological limitations and a lack of model organisms (Munday et al., 2013). However, we returned 30 publications examining genetic adaptation in response to marine climate change factors, indicating significant process in this area. Moreover, as our study did not include experiments conducted at the sub-organismal level, the total number of studies published in this period will be substantially greater. Fourth, the number of studies on behavioral changes of marine biota to climate change factors has also risen. The first decade of the millennium predominantly examined ecophysiological responses, with just six out of the 110 papers examining behavioral changes. The following decade, however, 17 times as many papers tested effects of climate change on behavioral traits, including auditory (Simpson et al., 2011), learning (Vila Pouca et al., 2019) and foraging behavior (Wu et al., 2017). These types of studies are critical because they have documented that behavioral responses to climatic changes can have cascading effects and lead to further detrimental impact on survivorship, ecological interactions and, ultimately, community structure.
It is now well-established that marine organisms are increasingly exposed to warming, extreme events, ocean acidification, and decreased oxygen levels related to anthropogenic greenhouse gas emissions (IPCC, 2014). Two decades of experimental studies have dramatically increased our mechanistic understanding of processes and causal relationships between climate change factors and the responses of marine organisms. This mechanistic understanding is necessary to improve predictions of responses and how to best manage local biota into the future. During the 2010s, significant progress was made in the sub-discipline of marine climate change ecology, particularly by expanding multiple stressor and multiple species experiments, and by testing for impacts on a much wider range or study organisms, which have increased realism and broadened the generality of the findings.
However, our synthesis has highlighted knowledge gaps and challenges for the coming decade, which can be summarized through the following recommendations: (1) strong biases in publication effort remain, such as a prevalence of studies conducted on temperate benthic invertebrates, which should be addressed to widen the inference space and generality of findings. (2) Despite significant progress, the majority of experiments manipulated one or two variables and examined the response of a single species in isolation. Given that contemporary global change encapsulates a number of concurrent stressors and that communities and ecosystems are strongly influenced by species interactions, further experimental work should seek to increase realism through greater numbers of co-occurring stressors and species. (3) Although logistically challenging, field-based manipulative studies performed in marine environments lag way behind those conducted in the terrestrial realm; innovative experimental approaches performed under realistic conditions will reduce artificiality and caveats relating to experimental venue and choice of model organism. (4) Where possible, results of MCCEs should be combined with supplementary approaches, such as time-series data analysis, control-impact studies, and field observations or experiments conducted along natural environmental gradients (e.g., space-for-time substitutions across latitude). Such a multi-pronged approach will improve understanding of how multiple concurrent climate change stressors impact upon organisms, populations and communities, so that more effective conservation, management and adaptation measures can be developed and implemented.
The original contributions presented in the study are included in the article/Supplementary Material, further inquiries can be directed to the corresponding author.
The ideas in this paper were conceived and developed by all authors. AB collected and analyzed the data. AB led the writing and manuscript with significant input from DS. All authors contributed to drafts and gave final approval for publication.
DS was funded by a UKRI Future Leaders Fellowship (MR/S032827/1). TW was funded by the Australian Research Council (DP170100023). MT was funded by the Brian Mason Trust.
The authors declare that the research was conducted in the absence of any commercial or financial relationships that could be construed as a potential conflict of interest.
All claims expressed in this article are solely those of the authors and do not necessarily represent those of their affiliated organizations, or those of the publisher, the editors and the reviewers. Any product that may be evaluated in this article, or claim that may be made by its manufacturer, is not guaranteed or endorsed by the publisher.
The Supplementary Material for this article can be found online at: https://www.frontiersin.org/articles/10.3389/fmars.2021.714462/full#supplementary-material
Albright, R., Takeshita, Y., Koweek, D., Ninokawa, A., Wolfe, A., Rivlin, T., et al. (2018). Carbon dioxide addition to coral reef waters suppresses net community calcification. Nature 555, 516–519. doi: 10.1038/nature25968
Al-Janabi, B., Wahl, M., Karsten, U., Graiff, A., and Kruse, I. (2019). Sensitivities to global change drivers may correlate positively or negatively in a foundational marine macroalga. Sci. Rep. 9, 1–10. doi: 10.1038/s41598-019-51099-8.
Alsterberg, C., Eklöf, J., Gamfeldt, L., Havenhand, J., and Sundbäck, K. (2013). Consumers mediate the effects of experimental ocean acidification and warming on primary producers. Proc. Natl. Acad. Sci. 110, 8603–8608. doi: 10.1073/pnas.1303797110
Andrews, O., Bindoff, N., Halloran, P., Ilyina, T., and Le Quér,é, C. (2013). Detecting an external influence on recent changes in oceanic oxygen using an optimal fingerprinting method. Biogeosciences 10, 1799–1813. doi: 10.5194/bg-10-1799-2013
Andrews, S., Bennett, S., and Wernberg, T. (2014). Reproductive seasonality and early life temperature sensitivity reflect vulnerability of a seaweed undergoing range reduction. Mar. Ecol. Prog. Ser. 495, 119–129. doi: 10.3354/meps10567
Anestis, A., Lazou, A., Pörtner, H. O., and Michaelidis, B. (2007). Behavioral, metabolic, and molecular stress responses of marine bivalve Mytilus galloprovincialis during long-term acclimation at increasing ambient temperature. Am. J. Physiol. Reg. Int. Comp. Phys. 293, 911–921. doi: 10.1152/ajpregu.00124.2007
Anthony, K. R. N., Kline, D. I., Diaz-Pulido, G., Dove, S., and Hoegh-Guldberg, O. (2008). Ocean acidification causes bleaching and productivity loss in coral reef builders. Proc. Nat. Ac. Sci. USA 105, 17442–17446. doi: 10.1073/pnas.0804478105
Araújo, J., Madeira, D., Vitorino, R., Repolho, T., Rosa, R., and Diniz, M. (2018). Negative synergistic impacts of ocean warming and acidification on the survival and proteome of the commercial sea bream, Sparus aurata. J. Sea Res. 139, 50–61. doi: 10.1016/j.seares.2018.06.011
Arft, A. M., Walker, M. D., Gurevitch, J., Alatalo, J. M., Bret-Harte, M. S., Dale, M., et al. (1999). Responses of tundra plants to experimental warming: meta-analysis of the International Tundra experiment. Ecol. Monogr. 69, 491–511. doi: 10.1890/0012-9615(1999)069[0491:ROTPTE]2.0.CO;2
Arnold, K. E., Findlay, H. S., Spicer, J. I., Daniels, C. L., and Boothroyd, D. (2009). Effect of CO2-related acidification on aspects of the larval development of the European lobster, Homarus gammarus (L.). Biogeosci. Discuss. 6, 1747–1754. doi: 10.5194/bg-6-1747-2009
Ashton, G., Morley, S., Barnes, D., Clark, M., and Peck, L. (2017). Warming by 1°C drives species and assemblage level responses in Antarctica's marine shallows. Curr. Biol. 27, 2698–2705. doi: 10.1016/j.cub.2017.07.048
Babcock, R. C., Bustamante, R. H., Fulton, E. A., Fulton, D. J., Haywood, M. D., Hobday, A. J., et al. (2019). Severe continental-scale impacts of climate change are happening now: extreme climate events impact marine habitat forming communities along 45% of Australia's coast. Front. Mar. Sci. 6:411. doi: 10.3389/fmars.2019.00411
Barry, J., Lovera, C., Buck, K., Peltzer, E., Taylor, J., Walz, P., et al. (2014). Use of a Free Ocean CO2 Enrichment (FOCE) system to evaluate the effects of ocean acidification on the foraging behavior of a deep-sea urchin. Environ Sci. Technol. 48, 9890–9897. doi: 10.1021/es501603r
Bellwood, D., Hughes, T., Folke, C., and Nyström, M. (2004). Confronting the coral reef crisis. Nature 429, 827–833. doi: 10.1038/nature02691
Bennett, S., Wernberg, T., Connell, S. D., Hobday, A. J., Johnson, C. R., and Poloczanska, E. S. (2016). The ‘Great Southern Reef': social, ecological and economic value of Australia's neglected kelp forests. Mar. Freshw. Res. 67, 47–56. doi: 10.1071/MF15232
Benton, T., Solan, M., Travis, J., and Sait, S. (2007). Microcosm experiments can inform global ecological problems. Trends Ecol. Evol. 22, 516–521. doi: 10.1016/j.tree.2007.08.003
Berge, J. A., Bjerkeng, B., Pettersen, O., Schaanning, M. T., and Øxnevad, S. (2006). Effects of increased sea water concentrations of CO2 on growth of the bivalve Mytilus edulis L. Chemosphere 62, 681–687. doi: 10.1016/j.chemosphere.2005.04.111
Borja, A. (2014). Grand challenges in marine ecosystems ecology. Front. Mar Sci. 11:1. doi: 10.3389/fmars.2014.00001
Brakel, J., Jakobsson-Thor, S., Bockelmann, A., and Reusch, T. (2019). Modulation of the Eelgrass - Labyrinthula zosterae Interaction under predicted ocean warming, salinity change and light limitation. Front. Mar. Sci. 6:268. doi: 10.3389/fmars.2019.00268
Breitburg, D. L., Baxter, J. W., Hatfield, C. A., Howarth, R. W., Jones, C. G., Lovett, G. M., et al. (1998). “Understanding effects of multiple stressors: ideas and challenges,” in Successes, Limitations, and Frontiers in Ecosystem Science, eds M. L. Pace, and P. M. Groffman (New York, NY: Springer), 416–431. doi: 10.1007/978-1-4612-1724-4_17
Brooks, P., and Crowe, T. (2018). Density and biotic interactions modify the combined effects of global and local stressors. Oikos 127, 1746–1758. doi: 10.1111/oik.04459
Burke, L., Reytar, K., Spalding, M., and Perry, A. (2011). Reefs at Risk Revisited. Washington, DC: World Resources Institute.
Burnell, O. W., Russell, B. D., Irving, A. D., and Connell, S. D. (2013). Eutrophication offsets increased sea urchin grazing on seagrass caused by ocean warming and acidification. Mar. Ecol. Prog. Ser. 485, 37–46. doi: 10.3354/meps10323
Burnell, O. W., Russell, B. D., Irving, A. D., and Connell, S. D. (2014). Seagrass response to CO2 contingent on epiphytic algae: indirect effects can overwhelm direct effects. Oecologia 176, 871–882. doi: 10.1007/s00442-014-3054-z
Campbell, A., Mangan, S., Ellis, R., and Lewis, C. (2014). Ocean acidification increases copper toxicity to the early life history stages of the polychaete Arenicola marina in artificial seawater. Environ. Sci Tech. 48, 9745–9753. doi: 10.1021/es502739m
Carpenter, S. (1996). Microcosm experiments have limited relevance for community and ecosystem ecology. Ecology 77, 677–680.
Cesar, H., Burke, L., and Pet-Soede, L. (2003). The Economics of Worldwide Coral Reef Degradation. Arnhem: Cesar Environmental Economics Consulting (CEEC).
Cheng, M., Sar,à, G., and Williams, G. (2018). Combined effects of thermal conditions and food availability on thermal tolerance of the marine bivalve, Perna viridis. J. Therm. Biol. 78, 270–276. doi: 10.1016/j.jtherbio.2018.10.014
Cheung, W., Lam, V., Sarmiento, J., Kearney, K., Watson, R., and Pauly, D. (2009). Projecting global marine biodiversity impacts under climate change scenarios. Fish Fish. 10, 235–251. doi: 10.1111/j.1467-2979.2008.00315.x
Christensen, N., Bartuska, A., Brown, J., Carpenter, S., D'Antonio, C., Francis, R., et al. (1996). The report of the ecological society of america committee on the scientific basis for ecosystem management. Ecol. Appl. 6, 665–691. doi: 10.2307/2269460
Clark, M., Villota Nieva, L., Hoffman, J., Davies, A., Trivedi, U., Turner, F., et al. (2019). Lack of long-term acclimation in Antarctic encrusting species suggests vulnerability to warming. Nat. Comm. 10:3383. doi: 10.1038/s41467-019-11348-w
Cole, V., Parker, L., O'Connor, S., O'Connor, W., Scanes, E., Byrne, M., et al. (2016). Effects of multiple climate change stressors: ocean acidification interacts with warming, hyposalinity, and low food supply on the larvae of the brooding flat oyster Ostrea angasi. Mar. Biol. 163:125. doi: 10.1007/s00227-016-2880-4
Cornwall, C. E., and Hurd, C. L. (2016). Experimental design in ocean acidification research: problems and solutions. ICES J. Mar Sci. 73, 572–581. doi: 10.1093/icesjms/fsv118
Coumou, D., and Rahmstorf, S. (2012). A decade of weather extremes. Nat. Clim. Change 2, 491–496. doi: 10.1038/nclimate1452
Cox, T., Gazeau, F., Alliouane, S., Hendriks, I., Mahacek, P., Le Fur, A., et al. (2016). Effects of in situ CO2 enrichment on structural characteristics, photosynthesis, and growth of the Mediterranean seagrass Posidonia oceanica. Biogeosciences 13, 2179–2194. doi: 10.5194/bg-13-2179-2016
Crain, C., Kroeker, K., and Halpern, B. (2008). Interactive and cumulative effects of multiple human stressors in marine systems. Ecol. Lett. 11, 1304–1315. doi: 10.1111/j.1461-0248.2008.01253.x
Curtin, R., and Prellezo, R. (2010). Understanding marine ecosystem based management: a literature review. Mar. Policy 34, 821–830. doi: 10.1016/j.marpol.2010.01.003
Dashfield, S., Somerfield, P., Widdicombe, S., Austen, M., and Nimmo, M. (2008). Impacts of ocean acidification and burrowing urchins on within-sediment pH profiles and subtidal nematode communities. J. Exp. Mar. Biol. Ecol. 365, 46–52. doi: 10.1016/j.jembe.2008.07.039
de Bettignies, T., Wernberg, T., and Gurgel, C. F. D. (2018). Exploring the influence of temperature on aspects of the reproductive phenology of temperate seaweeds. Front. Mar. Sci. 5:218. doi: 10.3389/fmars.2018.00218
Donelson, J., and Munday, P. (2015). Transgenerational plasticity mitigates the impact of global warming to offspring sex ratios. Glob. Change Biol. 21, 2954–2962. doi: 10.1111/gcb.12912
Donelson, J., Wong, M., Booth, D., and Munday, P. (2016). Transgenerational plasticity of reproduction depends on rate of warming across generations. Evol. Appl. 9, 1072–1081. doi: 10.1111/eva.12386
Donelson, J. M., Salinas, S., Munday, P. L., and Shama, L. N. (2018). Transgenerational plasticity and climate change experiments: Where do we go from here? Glob. Change Biol. 24, 13–34. doi: 10.1111/gcb.13903
Doney, S. C., Ruckelshaus, M., Duffy, E. J., Barry, J. P., Chan, F., English, C. A., et al. (2012). Climate change impacts on marine ecosystems. Annu. Rev.Mar. Sci. 4, 11–37. doi: 10.1146/annurev-marine-041911-111611
Duarte, C. M. (2014). Global change and the future ocean: a grand challenge for marine sciences. Front. Mar. Sci. 1:63. doi: 10.3389/fmars.2014.00063
Duarte, C. M., Losada, I. J., Hendriks, I. E., Mazarrasa, I., and Marb,à, N. (2013). The role of coastal plant communities for climate change mitigation and adaptation. Nat. Clim. Change 3, 961–968. doi: 10.1038/nclimate1970
Dupont, S., Havenhand, J., Thorndyke, W., Peck, L., and Thorndyke, M. (2008). Near-future level of CO2-driven ocean acidification radically affects larval survival and development in the brittlestar Ophiothrix fragilis. Mar. Ecol. Prog. Ser. 373, 285–294. doi: 10.3354/meps07800
Ehlers, A., Worm, B., and Reusch, T. (2008). Importance of genetic diversity in eelgrass Zostera marina for its resilience to global warming. Mar. Ecol. Prog. Ser. 355, 1–7. doi: 10.3354/meps07369
Ericson, J., Ho, M., Miskelly, A., King, C., Virtue, P., Tilbrook, B., et al. (2012). Combined effects of two ocean change stressors, warming and acidification, on fertilization and early development of the Antarctic echinoid Sterechinus neumayeri. Polar Biol. 35, 1027–1034. doi: 10.1007/s00300-011-1150-7
Falkenberg, L. J., Connell, S. D., Coffee, O. I., Ghedini, G., and Russell, B. D. (2015). Species interactions can maintain resistance of subtidal algal habitats to an increasingly modified world. Glob. Ecol. Conserv. 4, 549–558. doi: 10.1016/j.gecco.2015.10.003
Filbee-Dexter, K., Wernberg, T., Fredriksen, S., Norderhaug, K. M., and Pedersen, M. F. (2019). Arctic kelp forests: diversity, resilience and future. Glob. Planet. Change 172, 1–14. doi: 10.1016/j.gloplacha.2018.09.005
García, E., Clemente, S., and Hernández, J. (2015). Ocean warming ameliorates the negative effects of ocean acidification on Paracentrotus lividus larval development and settlement. Mar. Environ. Res., 110, 61–68. doi: 10.1016/j.marenvres.2015.07.010
Gattusso, J.-P., Magnan, A. K., Bopp, L., Cheung, W. W., Duarte, C. M., Hinkel, J., et al. (2018). Ocean solutions to address climate change and its effects on marine ecosystems. Front. Mar. Sci. 5:337. doi: 10.3389/fmars.2018.00337
Gazeau, F., Quiblier, C., Jansen, J. M., Gattuso, J. P., Middelburg, J. J., and Heip, C. H. R. (2007). Impact of elevated CO2 on shellfish calcification. Geophys. Res. Lett. 34, 1–5. doi: 10.1029/2006GL028554
Griffith, A. W., and Gobler, C. J. (2017). Transgenerational exposure of North Atlantic bivalves to ocean acidification renders offspring more vulnerable to low pH and additional stressors. Sci. Rep. 7:11394. doi: 10.1038/s41598-017-11442-3
Gruber, N. (2011). Warming up, turning sour, losing breath: ocean biogeochemistry under global change. Philos. Trans. R. Soc. A 369, 1980–1996. doi: 10.1098/rsta.2011.0003
Halpern, B. S., Frazier, M., Potapenko, J., Casey, K. S., Koenig, K., Longo, C., et al. (2015). Spatial and temporal changes in cumulative human impacts on the world's ocean. Nat. Commun. 6, 1–7. doi: 10.1038/ncomms8615.
Hildebrandt, N., Niehoff, B., and Sartoris, F. (2014). Long-term effects of elevated CO2 and temperature on the Arctic calanoid copepods Calanus glacialis and C. hyperboreus. Mar. Pollut. Bull. 80, 59–70. doi: 10.1016/j.marpolbul.2014.01.050
Hobbie, J. E., Carpenter, S. R., Grimm, N. B., Gosz, J. R., and Seastedt, T. R. (2003). The US long term ecological research program. BioScience 53, 21–32. doi: 10.1641/0006-3568(2003)053[0021:TULTER]2.0.CO;2
Hobday, A. J., Alexander, L. V., Perkins, S. E., Smale, D. A., Straub, S. C., Oliver, E. C. J., et al. (2016). A hierarchical approach to defining marine heatwaves. Prog. Oceanogr. 141, 227–238. doi: 10.1016/j.pocean.2015.12.014
Hobday, A. J., Oliver, E. C., Gupta, A. S., Benthuysen, J. A., Burrows, M. T., Donat, M. G., et al. (2018). Categorizing and naming marine heatwaves. J. Oceanogr. 31, 162–173. doi: 10.5670/oceanog.2018.205
Hughes, T., Anderson, K., Connolly, S., Heron, S., Kerry, J., Lough, J., et al. (2018). Spatial and temporal patterns of mass bleaching of corals in the Anthropocene. Science 359, 80–83. doi: 10.1126/science.aan8048
IPCC (2013). “Climate change 2013: the physical science basis,” in Contribution of Working Group I to the Fifth Assessment Report of the Intergovernmental Panel on Climate Change, eds T. F. Stocker, D. Qin, G. K. Plattner, M. Tignor,S. K. Allen, J. Boschung, A. Nauels, Y. Xia, V. Bex, and P. M, Midgley (New York, NY; Cambridge: Cambridge University Press), 1535.
IPCC (2014). “Climate change 2014: synthesis report” in Contribution of Working groups I, II and III to the Fifth Assessment Report of the Intergovernmental Panel of Climate Change, eds Core Writing Team, R. K. Pachauri, and L. A. Meyer (Geneva: IPCC), 151.
IPCC (2019). “Summary for Policymakers,” in IPCC Special Report on the Ocean and Cryosphere in a Changing Climate, eds H. O. Pörtner, D. C. Roberts, V. Masson-Delmotte, P. Zhai, M. Tignor, E. Poloczanska, K. Mintenbeck, M. Nicolai, A. Okem, J. Petzold, B. Rama, and N. Weyer. Geneva, Switzerland.
Jury, C. P., Delano, M. N., and Toonen, R. J. (2019). High heritability of coral calcification rates and evolutionary potential under ocean acidification. Sci. Rep., 9:20419. doi: 10.1038/s41598-019-56313-1
Kapsenberg, L., and Hofmann, G. (2014). Signals of resilience to ocean change: high thermal tolerance of early stage Antarctic sea urchins (Sterechinus neumayeri) reared under present-day and future pCO2 and temperature. Polar Biol. 37, 967–980. doi: 10.1007/s00300-014-1494-x
Kelly, M. W., Debiasse, M. B., Villela, V. A., Roberts, H. L., and Cecola, C. F. (2016). Adaptation to climate change: Trade-offs among responses to multiple stressors in an intertidal crustacean. Evol. Appl. 9, 1147–1155. doi: 10.1111/eva.12394
Kline, D., Teneva, L., Okamoto, D., Schneider, K., Caldeira, K., Miard, T., et al. (2019). Living coral tissue slows skeletal dissolution related to ocean acidification. Nat. Ecol. Evol. 3, 1438–1444. doi: 10.1038/s41559-019-0988-x
Koenker, B., Laurel, B., Copeman, L., and Ciannelli, L. (2018). Effects of temperature and food availability on the survival and growth of larval Arctic cod (Boreogadus saida) and walleye pollock (Gadus chalcogrammus). ICES J. Mar. Sci. 75, 2386–2402. doi: 10.1093/icesjms/fsy062
Kordas, R. L., Harley, C. D., and O'Connor, M. I. (2011). Community ecology in a warming world: the influence of temperature on interspecific interactions in marine systems. J. Exp. Mar. Biol. Ecol. 400:218–226. doi: 10.1016/j.jembe.2011.02.029
Kroeker, K., Kordas, R., Crim, R., Hendriks, I., Ramajo, L., Singh, G., et al. (2013). Impacts of ocean acidification on marine organisms: quantifying sensitivities and interaction with warming. Glob. Change Biol. 19, 1884–1896. doi: 10.1111/gcb.12179
Last, P. R., White, W. T., Gledhill, D. C., Hobday, A. J., Brown, R., Edgar, G. J., et al. (2011). Long-term shifts in abundance and distribution of a temperate fish fauna: a response to climate change and fishing practices. Glob. Ecol. Biogeogr. 20, 58–72. doi: 10.1111/j.1466-8238.2010.00575.x
Lee, T., Markowitz, E., Howe, P., Ko, C., and Leiserowitz, A. A. (2015). Predictors of public climate change awareness and risk perception around the world. Nat. Clim. Change 5, 1014–1020. doi: 10.1038/nclimate2728
Leggat, W. P., Camp, E. F., Suggett, D. J., Heron, S. F., Fordyce, A. J., Gardner, S., et al. (2019). Rapid coral decay is associated with marine heatwave mortality events on reefs. Curr. Biol. 29, 2723–2730. doi: 10.1016/j.cub.2019.06.077
Link, J. S., and Browman, H. I. (2017). Operationalizing and implementing ecosystem-based management. ICES J. Mar. Sci. 74, 379–381. doi: 10.1093/icesjms/fsw247
McLeod, K. L., Lubchenco, J., Palumbi, S., and Rosenberg, A. A. (2005). Scientific Consensus Statement on Marine Ecosystem-Based Management. USA-National: Communication Partnership for Science and the Sea (COMPASS). Available online at: http://compassonline.org/?q=EBM (accessed March 03, 2021)
McMahon, S., Parsons, D., Donelson, J., Pether, S., and Munday, P. (2020). Elevated CO2 and heatwave conditions affect the aerobic and swimming performance of juvenile Australasian snapper. Mar. Biol. 167:6. doi: 10.1007/s00227-019-3614-1
Montie, S., Thomsen, M., Rack, W., and Broady, P. (2020). Extreme summer marine heatwaves increase chlorophyll a in the Southern Ocean. Antarct. Sci. 32, 508–509. doi: 10.1017/S0954102020000401
Munday, P. L., Schunter, C., Allan, B. J. M., Nichol, S., Parsons, D. M., Pether, S. M., et al. (2019). Testing the adaptive potential of yellowtail kingfish to ocean warming and acidification. Front. Ecol. Evol. 7:253. doi: 10.3389/fevo.2019.00253
Munday, P. L., Warner, R. R., Monro, K., Pandolfi, J. M., and Marshall, D. J. (2013). Predicting evolutionary responses to climate change in the sea. Ecol. lett. 16, 1488–1500. doi: 10.1111/ele.12185
Nagelkerken, I., and Munday, P. L. (2016). Animal behaviour shapes the ecological effects of ocean acidification and warming: moving from individual to community-level responses. Glob. Change Biol. 22, 974–989. doi: 10.1111/gcb.13167
Oliver, E., Donat, M., Burrows, M., Moore, P., Smale, D., Alexander, L., et al. (2018). Longer and more frequent marine heatwaves over the past century. Nat. Comm. 9:1324. doi: 10.1038/s41467-018-03732-9
Parmesan, C. (2006). Ecological and evolutionary responses to recent climate change. Annu. Rev. Ecol. Evol. Syst. 37, 637–669. doi: 10.1146/annurev.ecolsys.37.091305.110100.
Peck, L., Massey, A., Thorne, M., and Clark, M. (2008). Lack of acclimation in Ophionotus victoriae: brittle stars are not fish. Polar Biol. 32, 399–402. doi: 10.1007/s00300-008-0532-y
Pecl, G. T., Araújo, M. B., Bell, J. D., Blanchard, J., Bonebrake, T. C., Chen, I.-C., et al. (2017). Biodiversity redistribution under climate change: Impacts on ecosystems and human well-being. Science 355:6332. doi: 10.1126/science.aai9214
Perry, A., Low, P., Ellis, J., and Reynolds, J. (2005). Climate change and distribution shifts in marine fishes. Science 308, 1912–1915. doi: 10.1126/science.1111322
Pistevos, J. C. A., Nagelkerken, I., Rossi, T., Olmos, M., and Connell, S. D. (2015). Ocean acidification and global warming impair shark hunting behaviour and growth. Sci. Rep. 5:16293. doi: 10.1038/srep16293
Poloczanska, E., Brown, C., Sydeman, W., Kiessling, W., Schoeman, D., Moore, P., et al. (2013). Global imprint of climate change on marine life. Nat. Clim. Change 3, 919–925. doi: 10.1038/nclimate1958 Available online at: https://www.nature.com/articles/nclimate1958
Poloczanska, E., Burrows, M., Brown, C., Molinos, J., Halpern, B., Hoegh-Guldberg, O., et al. (2016). Responses of marine organisms to climate change across oceans. Front. Mar. Sci. 3:62. doi: 10.3389/fmars.2016.00062
Prather, C., Pelini, S., Laws, A., Rivest, E., Woltz, M., Bloch, C., et al. (2012). Invertebrates, ecosystem services and climate change. Biol. Rev. 88, 327–348. doi: 10.1111/brv.12002
Repolho, T., Duarte, B., Dionisio, G., Paula, J. R., Lopes, A. R., Rosa, I. C., et al. (2017). Seagrass ecophysiological performance under ocean warming and acidification. Sci. Rep. 7:41443. doi: 10.1038/srep41443
Russell, B. D., Connell, S. D., Findlay, H. S., Tait, K., Widdicombe, S., and Miezkowska, N. (2013). Ocean acidification and rising temperatures may increase biofilm primary productivity but decrease grazer consumption. Phil. Trans. R. Soc. B 368:20120438. doi: 10.1098/rstb.2012.0438
Sala, E., and Knowlton, N. (2006). Global marine biodiversity trends. Annu. Rev. Env. Resour. 31, 93–122. doi: 10.1146/annurev.energy.31.020105.100235
Sheppard-Brennand, H., Soars, N., Dworjanyn, S., Davis, A., and Byrne, M. (2010). Impact of ocean warming and ocean acidification on larval development and calcification in the sea urchin Tripneustes gratilla. PLoS ONE 5:e11372. doi: 10.1371/journal.pone.0011372
Simpson, S., Munday, P., Wittenrich, M., Manassa, R., Dixson, D., Gagliano, M., et al. (2011). Ocean acidification erodes crucial auditory behaviour in a marine fish. Biol. Lett. 7, 917–920. doi: 10.1098/rsbl.2011.0293
Skirving, W., Heron, S., Marsh, B., Liu, G., De La Cour, J., Geiger, E., et al. (2019). The relentless march of mass coral bleaching: a global perspective of changing heat stress. Coral Reefs 38, 547–557. doi: 10.1007/s00338-019-01799-4
Smale, D., Taylor, J., Coombs, S., Moore, G., and Cunliffe, M. (2017). Community responses to seawater warming are conserved across diverse biological groupings and taxonomic resolutions. Proc. R. Soc. B 284:20170534. doi: 10.1098/rspb.2017.0534
Smale, D., and Wernberg, T. (2012). Short-term in situ warming influences early development of sessile assemblages. Mar. Ecol. Prog. Ser. 453:129–136. doi: 10.3354/meps09680
Smale, D., and Wernberg, T. (2013). Extreme climatic event drives range contraction of a habitat-forming species. Proc. R. Soc. B 280:20122829. doi: 10.1098/rspb.2012.2829
Smale, D., Wernberg, T., Oliver, E., Thomsen, M., Harvey, B., Straub, S., et al. (2019). Marine heatwaves threaten global biodiversity and the provision of ecosystem services. Nat. Clim. Change 9, 306–312. doi: 10.1038/s41558-019-0412-1
Smale, D. A. (2020). Impacts of ocean warming on kelp forest ecosystems. New Phytol. 225, 1447–1454. doi: 10.1111/nph.16107
Smale, D. A., Burrows, M. T., Moore, P., O'Connor, N., and Hawkins, S. J. (2013). Threats and knowledge gaps for ecosystem services provided by kelp forests: a northeast Atlantic perspective. Ecol. Evol. 3, 4016–4038. doi: 10.1002/ece3.774
Sommer, U., and Lengfellner, K. (2008). Climate change and the timing, magnitude, and composition of the phytoplankton spring bloom. Glob. Change Biol. 14:1199–1208. doi: 10.1111/j.1365-2486.2008.01571.x
Spicer, J. I., and Morley, S. A. (2019). Will giant polar amphipods be first to fare badly in an oxygen-poor ocean? Testing hypotheses linking oxygen to body size. Phil. Trans. R. Soc. B 374:20190034. doi: 10.1098/rstb.2019.0034
Stewart, R. I., Dossena, M., Bohan, D. A., Jeppersen, E., Kordas, R. L., Ledger, M. E., et al. (2013). Chapter two - mesocosm experiments as a tool for ecological climate change research. Adv. Ecol. Res. 48, 71–181. doi: 10.1016/B978-0-12-417199-2.00002-1
Straub, S. C., Wernberg, T., Thomsen, M. S., Moore, P. J., Burrows, M., Harvey, B. P., et al. (2019). Resistance to obliteration; responses of seaweeds to marine heatwaves. Front. Mar. Sci. 6:763. doi: 10.3389/fmars.2019.00763
Sunday, J. M., Calosi, P., Dupont, S., Munday, P. L., Stillman, J. H., and Reusch, T. B. (2014). Evolution in an acidifying ocean. Trends Ecol. Evol. 29, 117–125. doi: 10.1016/j.tree.2013.11.001
Sutherland, W. (2006). Predicting the ecological consequences of environmental change: a review of the methods. J. Appl. Ecol. 43, 599–616. doi: 10.1111/j.1365-2664.2006.01182.x
Thomsen, M., Mondardini, L., Alestra, T., Gerrity, S., Tait, L., South, P., et al. (2019). Local extinction of bull kelp (Durvillaea spp.) due to a marine heatwave. Front. Mar. Sci. 6:84. doi: 10.3389/fmars.2019.00084
Thor, P., and Dupont, S. (2015). Transgenerational effects alleviate severe fecundity loss during ocean acidification in a ubiquitous planktonic copepod. Glob. Change Biol. 21, 2261–2271. doi: 10.1111/gcb.12815
Tilman, D. (1989). “Ecological experimentation: strengths and conceptual problems,” in Long-Term Studies in Ecology, ed G.E. Likens (New York, NY: Springer), 136–157. doi: 10.1007/978-1-4615-7358-6_6
Underwood, A. (1996). Detection, interpretation, prediction and management of environmental disturbances: some roles for experimental marine ecology. J. Exp. Mar. Biol. Ecol. 200, 1–27. doi: 10.1016/S0022-0981(96)02637-8
Uthicke, S., Soars, N., Foo, S., and Byrne, M. (2013). Effects of elevated pCO2 and the effect of parent acclimation on development in the tropical Pacific sea urchin Echinometra mathaei. Mar. Biol. 160, 1913–1926. doi: 10.1007/s00227-012-2023-5
Vila Pouca, C., Gervais, C., Reed, J., Michard, J., and Brown, C. (2019). Quantity discrimination in Port Jackson sharks incubated under elevated temperatures. Behav. Ecol. and Sociobiol. 73:93. doi: 10.1007/s00265-019-2706-8
Wang, T., and Wang, Y. (2020). Behavioural responses to ocean acidification in marine invertebrates: new insights and future directions. J. Oceanol. Limnol. 38, 759–772. doi: 10.1007/s00343-019-9118-5
Welch, M. J., and Munday, P. L. (2017). Heritability of behavioural tolerance to high CO2 in a coral reef fish is masked by nonadaptive phenotypic plasticity. Evol. Appl. 10, 682–693. doi: 10.1111/eva.12483
Welch, M. J., Watson, S.-A., Welsh, J. Q., McCormick, M. I., and Munday, P. L. (2014). Effects of elevated CO2 on fish behaviour is undiminished by transgenerational acclimation. Nat. Clim. Change 4, 1086–1089. doi: 10.1038/nclimate2400
Wernberg, T., Bennett, S., Babcock, R. C., de Bettignies, T., Cure, K., Depczynski, M., et al. (2016). Climate driven regime shift of a temperate marine ecosystem. Science 353:169–172. doi: 10.1126/science.aad8745
Wernberg, T., Coleman, M. A., Babcock, R. C., Bell, S. Y., Bolton, J. J., Connell, S. D., et al. (2019). Biology and ecology of the globally significant kelp Ecklonia radiata. Oceanogr. Mar. Biol. 57, 265–324. doi: 10.1201/9780429026379
Wernberg, T., Russell, B. D., Thomsen, M. S., Gurgel, C. F. D., Bradshaw, C. J. A., Poloczanska, E. S., et al. (2011). Seaweed communities in retreat from ocean warming. Curr. Biol. 21, 1828–1832. doi: 10.1016/j.cub.2011.09.028
Wernberg, T., Smale, D. A., and Thomsen, M. S. (2012). A decade of climate change experiments on marine organisms: procedures, patterns and problems. Glob. Change Biol. 18, 1491–1498. doi: 10.1111/j.1365-2486.2012.02656.x
Wernberg, T., Smale, D. A., Tuya, F., Thomsen, M. S., Langlois, T. J., de Bettignies, T., et al. (2013). An extreme climatic event alters marine ecosystem structure in a global marine hotspot. Nat. Clim. Change 3, 78–82. doi: 10.1038/nclimate1627
Worm, B., Barbier, E. B., Beaumont, N., Duffy, J. E., Folke, C., Halpern, B. S., et al. (2006). Impacts of biodiversity loss on ocean ecosystem services. Science 314, 787–790. doi: 10.1126/science.1132294
Keywords: climate change, experiments, multiple stressors, ocean warming and acidification, global change
Citation: Bass A, Wernberg T, Thomsen M and Smale D (2021) Another Decade of Marine Climate Change Experiments: Trends, Progress and Knowledge Gaps. Front. Mar. Sci. 8:714462. doi: 10.3389/fmars.2021.714462
Received: 25 May 2021; Accepted: 10 August 2021;
Published: 30 August 2021.
Edited by:
Martin Zimmer, Leibniz Centre for Tropical Marine Research (LG), GermanyReviewed by:
Philip Munday, ARC Centre of Excellence for Coral Reef Studies, AustraliaCopyright © 2021 Bass, Wernberg, Thomsen and Smale. This is an open-access article distributed under the terms of the Creative Commons Attribution License (CC BY). The use, distribution or reproduction in other forums is permitted, provided the original author(s) and the copyright owner(s) are credited and that the original publication in this journal is cited, in accordance with accepted academic practice. No use, distribution or reproduction is permitted which does not comply with these terms.
*Correspondence: Dan Smale, ZGFuc21hQG1iYS5hYy51aw==
Disclaimer: All claims expressed in this article are solely those of the authors and do not necessarily represent those of their affiliated organizations, or those of the publisher, the editors and the reviewers. Any product that may be evaluated in this article or claim that may be made by its manufacturer is not guaranteed or endorsed by the publisher.
Research integrity at Frontiers
Learn more about the work of our research integrity team to safeguard the quality of each article we publish.