- 1Red Sea Research Center (RSRC) and Computational Bioscience Research Center (CBRC), King Abdullah University of Science and Technology (KAUST), Thuwal, Saudi Arabia
- 2Biological and Environmental Science and Engineering Division (BESE), King Abdullah University of Science and Technology (KAUST), Thuwal, Saudi Arabia
- 3Red Sea Research Center (RSRC), King Abdullah University of Science and Technology (KAUST), Thuwal, Saudi Arabia
The characterization of the internal microenvironment of symbiotic marine invertebrates is essential for a better understanding of the symbiosis dynamics. Microalgal symbionts (of the family: Symbiodiniaceae) influence diel fluctuations of in host O2 and pH conditions through their metabolic activities (i.e., photosynthesis and respiration). These variations may play an important role in driving oxygen budgets and energy demands of the holobiont and its responses to climate change. In situ measurements using microsensors were used to resolve the O2 and pH diel fluctuations in the oral arms of non-calcifying cnidarian model species Cassiopea sp. (the “upside-down jellyfish”), which has an obligatory association with Symbiodiniaceae. Before sunrise, the internal O2 and pH levels were substantially lower than those in ambient seawater conditions (minimum average levels: 61.92 ± 5.06 1SE μmol O2 L–1 and 7.93 ± 0.02 1SE pH units, respectively), indicating that conditions within Cassiopea’s oral arms were acidified and hypoxic relative to the surrounding seawater. Measurements performed during the afternoon revealed hyperoxia (maximum average levels: 546.22 ± 16.45 1SE μmol O2 L–1) and internal pH similar to ambient levels (8.61 ± 0.02 1SE pH units). The calculated gross photosynthetic rates of Cassiopea sp. were 0.04 ± 0.013 1SE nmol cm–2 s–1 in individuals collected at night and 0.08 ± 0.02 1SE nmol cm–2 s–1 in individuals collected during the afternoon.
Introduction
The mutualistic endosymbiosis between marine invertebrates and photosynthetic dinoflagellates represents one of the most important associations in tropical marine environments, allowing the growth of diverse, healthy and productive marine ecosystems, including coral reefs (Done et al., 1996; Knowlton et al., 2010; Davy et al., 2012; de Groot et al., 2012; Fransolet et al., 2012; Kennedy et al., 2013). Dinoflagellates of the family Symbiodinaceae [formerly Symbiodinium; see the recent revision by LaJeunesse et al. (2018) are the most common group of symbionts found in tropical symbiotic invertebrates (LaJeunesse, 2001), such as corals, sea anemones, jellyfish, and clams (Lampert, 2016)]. Endosymbiotic relationships involving symbiotic dinoflagellates first evolved in the Triassic period (Muscatine et al., 2005) and are essential contributors to global primary production, accounting for 1–10% of the total benthic production (Muscatine, 1990).
In the cnidarian-Symbiodiniaceae relationship, the host provides the symbionts with inorganic nutrients (e.g., such as CO2, NH3, and PO43–) and shelter (Yellowlees et al., 2008). In return, the host receives organic nutrients and oxygen by the symbiont (Muscatine, 1967; Muscatine and Porter, 1977; Falkowski et al., 1984; Muscatine et al., 1984; Smith and Muscatine, 1999; Hoegh-Guldberg et al., 2007; Mortillaro et al., 2009). Briefly, in these relationships a nutrient exchange between host and symbionts occurs: inorganic nutrients, such as carbon, nitrogen and phosphorous, are released by the host, taken up by the symbionts and then used for their metabolic activities (e.g., carbon fixation, nitrogen assimilation and growth; Miller and Yellowlees, 1989). Up to 95% of the photosynthates are then translocated to the host (Muscatine, 1990), including oxygen, which helps the host to produce more ATP (Jokiel, 2011) and it is used to support both symbiont and host respiration (Kühl et al., 1995). The photosynthesis by symbionts provides a massive contributor to the holobiont’s (i.e., host and symbionts considered together) energy demands (Gattuso et al., 1999) and oxygen budget (Jokiel, 2011).
The metabolic processes of the holobiont generate, therefore, dynamic diel fluctuations in internal O2 and pH dynamics, as observed in symbiotic corals (Kühl et al., 1995; Al-Horani et al., 2003), algae (de Beer et al., 2000), sponges (Schönberg et al., 2005), and sea anemones (Dykens and Shick, 1982). These fluctuations are likely to be primarily driven by the symbionts’ metabolic activities (Fitt, 2000; Gordon and Leggat, 2010). Despite the potential role of internal O2 and pH dynamics in driving the breakdown of the cnidarian-dinoflagellates symbioses, few studies have characterized these dynamics.
Here we characterize internal O2 and pH dynamics of a non-calcifying model symbiotic cnidarian species (the upside-down jellyfish Cassiopea sp.; Hofmann et al., 1978). We performed microsensor measurements within the oral arms, where the abundance of the symbionts is highest, before sunrise and during the afternoon, when O2 and pH were expected to be at their extremes. Additionally, we performed photosynthesis measurements using the light-shift method described by Revsbech et al. (1981) and Revsbech and Jorgensen (1983).
Materials and Methods
Species Studied
Cassiopea sp. was chosen as a model symbiotic cnidarian species for our study as it is a benthic scyphozoan (Rhizostomae) inhabiting subtropical and tropical coastal shallow waters, especially mangroves and seagrass meadows (Lampert, 2016; Klein et al., 2017; Ohdera et al., 2018). In these environments they are exposed to extreme conditions, including fluctuations of O2 and pH in the environment (Gray et al., 2012). Additionally, Cassiopea sp. harbors populations of Symbiodiniaceae, which are mostly concentrated in its oral arms (Hofmann et al., 1996; Lampert, 2016). The Cassiopea-Symbiodiniacea association is thus an example of successful cooperation under potentially stressful conditions, that is becoming increasingly popular as a model species.
Experimental Approach
Eighteen individuals of Cassiopea sp. (bell diameter average: 5.82 cm ± 1.3 1SD; day/night bell pulsations average: 76.88 ± 5.72 1SE pulsation min–1; see Supplementary Material for additional data about the specimens) were collected in the King Abdullah Economic City lagoon (KAEC) in the Central Red Sea (Latitude: 22.38979 N, Longitude: 39.135547E), in November 2020 at 0.20–1 m depth. Seawater temperature, salinity and light conditions (at noon) were measured in situ and were 28.00°C ± 0.00 1SE, 42.00 ppt, and ∼ 1246.18 μmol photons m–2 s–1 (photosynthetically active radiation [PAR], measured at the depth of collection), respectively. After sampling, the jellyfish were immediately transported to the Coastal and Marine Resources Core Labs (CMR) aquaria facilities at the King Abdullah University of Science and Technology (KAUST). All the specimens were kept in an outdoor tank (200 L) under natural light conditions (approximately 12h:12h night:light cycle, 0.0 μmol photons m–2 s–1 ± 0.0 1SE and 2130.0 μmol photons m–2 s–1 ± 101.7 1SE during night and day, respectively, and measured within 15 cm from the jellyfish) and acclimated to outdoor aquaria conditions for 5 days. The tank was supplied by a continuous flow of unfiltered seawater at a rate of ∼ 300 L h–1 and mimicked conditions of the field collection site (at 28.8°C ± 0.23 1SE and salinity of 42 ppt). The aquarium was equipped with a thermometer, temperature controller, and PAR sensor (Odyssey®, Odipar). Medusae were fed daily with newly hatched Artemia naupilii. Each day, one jellyfish was randomly selected at ∼ 5:30 AM local time for microsensor measurements, which was the moment of darkness immediately before sunrise (after about ∼ 12 h of natural exposure to darkness). The specimen was placed in a container filled with seawater and covered with dark black plastic to protect against artificial light, before being transferred to the Red Sea Research Center (RSRC) laboratories. The specimen was then transferred for the microsensor measurements in the same water used for transport. Likewise, one jellyfish was randomly selected at ∼ 1:00 AM local time, which coincided with the maximum intensity of natural day light in the region. This process was repeated every day until microsensor measurements were performed on 18 jellyfish (i.e., three jellyfish for each parameter and each timepoint (day and night): in total six jellyfish were used each for dO2, pH, and photosynthesis measurements). The chosen number of replicates (n = 6) represents a compromise between (a) the time-consuming nature of microsensor measurements, (b) being limited to analyze only two jellyfish each day (one at sunrise and one in the afternoon), and (c) minimizing the time the organisms spent in artificial conditions. Additionally, previously published studies were used as a guide to decide the number of medusae considered adequate replication (e.g., n = 3 in Wangpraseurt et al., 2012, 2014).
Microenvironment Characterization
For each jellyfish, the dO2 and pH of three oral arms was characterized. After collection and transport to the laboratory, one oral arm was removed from the body using a sterile scalpel and transferred into a transparent container filled with ∼ 50 ml of 2% agarose. The oral arm was placed onto the agarose layer and then covered with seawater taken from the aquarium (∼ 28°C, ∼ 42 ppt) to maintain its hydration and to avoid the possibility of thermal shock during the profiling. Hypodermic needles were used to keep the oral arm in position, to avoid any movements/contractions during the measurements. Subsequently, the container with Cassiopea’s oral arm was placed in a water bath kept at ∼ 28°C to mimic the environmental temperature (Figure 1). The set-up was also equipped with a custom-made arm with LED lights, allowing the LEDs to be placed directly above the oral arm (achieving ∼ 1091 μmol photons m–2 s–1 PAR, measured at the level of the oral arm). This procedure was repeated for each oral arm. dO2 and pH measurements were taken within ∼1 h from the removal of the first oral arm. After removal of the first oral arm, the jellyfish bodies collected at ∼ 5.30 am were kept in a dark incubator (Model I-22LLVL, Percival Scientific) set at 28°C until the removal of additional oral arms. For jellyfish collected at 1pm, the jellyfish bodies were kept in a transparent container and stored in an incubator set at 28°C with PAR irradiance (∼ 200 μmol photons m–2 s–1) while individual oral arms were being measured.
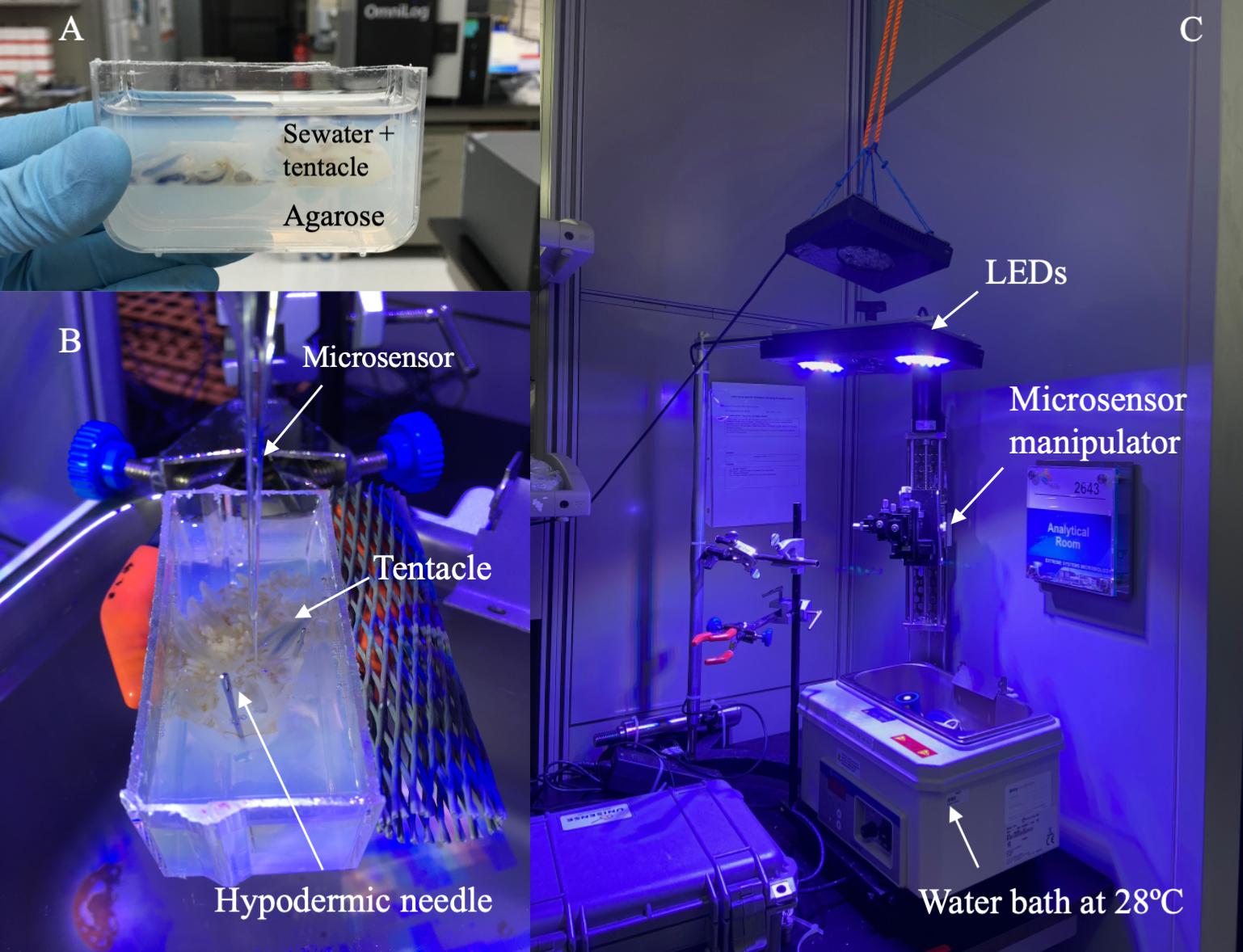
Figure 1. Experimental set up. (A) Oral arms were placed into a transparent container on a 2% agarose layer and covered with seawater from the aquarium. (B) Oral arms were kept in place using hypodermic needles. (C) The container with the oral arm was placed into a water bath set at 28oC (to mimic environmental conditions). The set-up included a custom-made arm equipped with LEDs and used to adjust the distance between the oral arm and lights. The microsensor manipulator was used to control the microsensor during our measurements.
Oxygen measurements were performed using 50 μm-diameter tip oxygen microsensors (OX-50, Unisense Denmark), following an overnight polarization and calibration at oxygen partial pressures of 0 and 21 kPa as previously described (Brune et al., 1995; Brune and Kühl, 1996; Brune, 1998; Brune and Friedrich, 2000). pH measurements were performed with pH microelectrodes with 50 μm-diameter tip, following a calibration using commercial pH standard solutions. Microsensors were connected to a motorized micromanipulator controlled by Unisense software and manually positioned above the oral arm as close as possible to the brownish areas, where symbionts concentrate (Fitt and Trench, 1983; Lampert, 2016), and ornamentation and colored vesicles are found. Once the microsensor’s tip perforated the tissue, it was remotely controlled to take measurements every 25 μm (n = 3). Control profiles were obtained by measuring seawater profiles of oxygen and pH, to account for eventual background sources of disturbance.
Since the data obtained from the microsensor profiles provides insight into the localization of symbionts within the oral arms (i.e., peaks or drops in oxygen/pH levels), the length of each microsensor profile was determined by the data collected. For instance, the initial profile showed similar levels to seawater, then a peak/drop and subsequently it stabilized. The measurements were stopped when no fluctuations were observed. Therefore, the relative depth of the profile was calculated depending on the position of the symbionts. The distance covered by our profiles was divided into 10 increments (i.e., number of steps from the entrance of the microsensor’s tip into the oral arm to the end of the profile), because each oral arm differed in shape and size. The distance covered by our profiles was divided into 10 increments (i.e., number of steps from the entrance of the microsensor’s tip into the oral arm to the end of the profile), because each oral arm differed in shape and size. Each increment measured on average ∼ 276.65 ± 15.54 1SE μm. The relative depth of the profile was calculated depending on the location of the symbiosomes.
Additional measurements were performed to characterize how dO2 levels changed during illumination and darkness within the oral arms. Briefly, the microsensor’s tip was manually placed inside the first millimeter of the oral arm and subjected to dark-light shifts of about 6 min each. Oxygen levels were recorded maintaining the microsensor at the same depth to characterize the rate of change between darkness and light.
When referring to measurements performed on jellyfish collected before sunrise we will use the terms “night,” while when referring to jellyfish collected in the afternoon, we will use the terms “afternoon.” Whereas, when referring to artificial dark-light shifts, we will use the terms “dark” and/or “light.”
Traits Measured
All the specimens were characterized by the following traits: bell diameter, jellyfish behavior, photochemical efficiency, wet weight, volume, chlorophyll and protein content, and symbiont density. Prior to the collection of the jellyfish, their bell diameter was measured at full extension using a ruler to the nearest mm. Jellyfish behavior was measured by counting the number of bell pulsations that occurred over 2 min (reported here as number of bell contractions for min–1; sensu Klein et al., 2016). Bell pulsation is a key behavior which allows the holobiont to capture food, expulse waste, exchange gases, release gametes and take up symbionts through the generation of water flows (Arai, 1997; Welsh et al., 2009).
Such measurements were performed through the transparent bottom of the aquarium to minimize disturbance. A Mini-pulse amplitude modulator (MiniPAM, Waltz GmbH, Germany) was used to measure photochemical efficiency (Fv/Fm) on four randomly selected oral arms. Finally, the wet weight of each individual was measured using a scale and their volume was estimated as the volume of displacement upon transferring the specimen into a graduated container, previously filled with a known volume of seawater taken from the aquarium. Seawater temperature, salinity, time of collection, sunrise and sunset time of the day were recorded throughout the experiment.
At the end of the experiment, the oral arms (in their entirety) were cut into smaller sections and stored at −20°C for chlorophyll a content, total protein, and Symbiodiniaceae density analyses (LaJeunesse et al., 2018) following a protocol adapted from Klein et al. (2016, 2019). Marginal appendages and vesicles were included in the analyses. A total of 18 oral arms (i.e., three from each jellyfish at each timepoint) were processed as follows: once defrosted, the oral arms were homogenized using a pestle and a glass tissue grinder (Wheaton Science Products, Millville, NJ, United States) until no tissue chunks were visible. Homogenized tissues were diluted to meet working range requirements of the analyses and dilutions were taken into account during subsequent calculations. Symbionts were counted using a hemocytometer in a 10 μL volume (n = 3) under a fluorescent microscope (Leica DM6000 B). A 200 μL aliquot was subsampled from the initial oral arm homogenate and processed to estimate chlorophyll a content. These samples were centrifuged at 3000 × g at 4°C for 10 min and the supernatant discarded. The pelleted symbionts were re-suspended in 1 ml of 100% ethanol and chl a was extracted overnight in darkness at 4°C. Samples were then centrifuged at 13000 × g for 5 min. The supernatant was transferred to a 96-wells plate and the absorption of the supernatant was determined at 629 and 665 nm (Ritchie, 2006) using a microplate reader spectrophotometer (SpectraMax Paradigm, Molecular Devices, CA, United States). Blanks (100% ethanol) were used as controls. Total chlorophyll concentrations were determined using coefficients from spectrophotometric equation for chlorophyll-a (μg/ml) (−2.6094 × A629 + 12.4380 × A665) and chlorophyll-c (29.8208 × A629–5.6461 × A665) for dinoflagellates in ethanol (Ritchie, 2006). Total protein content of the samples (expressed in μg/mL) was measured using the PierceTM BCA Protein Assay Kit (Thermo Fisher Scientific). Chlorophyll content was standardized to the total protein content of the oral arm and reported as μg mg–1 protein–1.
Gross Photosynthesis
Oxygen microsensors were used as described by Revsbech and Jorgensen (1983) and Kühl et al. (1995) to measure gross photosynthetic rates (nmol O2 cm–3 s–1) in the oral arms. The light-dark shift method (Revsbech and Jorgensen, 1983; Jensen and Revsbech, 1989) was used for this purpose. This method allows the measurements of photosynthetic rates by firstly subjecting the sample to a long period of illumination until a steady-state is reached. Then, the rate of oxygen drop observed within 1 to 2 s after darkness is used to calculate the gross photosynthetic rate P(I) (Equation 1; where the subscript 0 indicates the transition I→0 and where is the slope of the O2 profile at depth x; Revsbech and Jorgensen, 1983). This method requires long illumination periods to reach the steady state (∼ 10 min; Kühl et al., 1995; de Beer et al., 2000), therefore, we used illumination cycles of 300–500 s, falling within the range used by Al-Horani et al. (2003), to reduce the time employed for our measurements. As Polerecky et al. (2008) already mentioned, the estimation of the gross photosynthetic rate can be quantified using this method, even if the steady-state is not fully reached.
This method is based on some assumptions, such as (1) a steady-state of profile of oxygen before darkness, (2) an identical consumption of oxygen before and at the beginning of the darkness, and (3) identical diffusive fluxes during the observation period (Kühl et al., 1995). This estimation method may include carbon equivalents which are usually lost during respiration (Glud et al., 1995).
To avoid any effect due to time on our measurements, we limited the duration of the measurements to a maximum 1 h from the initial cut of the oral arm. To meet this requirement, depth profiles of photosynthetic rates were performed every 100 μm within the first 600 μm of the tissue.
Data Analysis
Normality of oxygen and pH data were tested by performing a Shapiro-Wilk’s test (p > 0.05) (Shapiro and Wilk, 1965; Razali and Wah, 2011), by analyzing Skewness and Kurtosis, and by visual inspection of histograms, normal Q-Q plots and box plots. A transformation ln(x+a+1) was used if normality was not met where a is the minimum (most negative) value in the ΔpH/ΔO2. This was done to ensure that × was above zero, as required for the logarithmic transformation. Linear Mixed Models (LMMs) were used to perform an analysis of the effects of Conditions (Night and Afternoon), Increments (i.e., the relative distance from the entrance of the microsensor tip into the oral arm), Day of analysis and Oral arm order. Conditions and Increments were considered as fixed factors, whereas Day of analysis and Tentacle order as random effects. Increments were considered as our repeated measure. Wald Z test of simultaneous coefficients and estimated covariance parameters were used to assess whether terms were redundant. They were retained to account for associated variance if a significant effect on the fit of the model was observed, otherwise they were removed and the analysis was performed again. To assess differences among means, post hoc analyses with pairwise multiple comparison were performed using IBM® SPSS® Statistics software (Version 27.0.1.0) as necessary. We used the Pearson correlation to test if there was a correlation between pH/O2 levels and chlorophyll concentration/symbiont counts.
Oxygen variations during experimental light-dark shifts were non-normally distributed, therefore a non-parametric Mann-Whitney test was used to test differences in distribution. Gross photosynthesis was analyzed using LMMs: Depth was considered as repeated measure and Conditions as fixed factor.
Data is reported as mean ± one standard error (1SE), unless otherwise indicated (e.g., standard deviation as 1SD).
Results
Oxygen Dynamics in Oral Arms
Our microelectrode measurements revealed extreme diel fluctuations of dO2 in Cassiopea sp. oral arms, from almost anoxia (i.e., lack of oxygen) to hyperoxia (i.e., oxygen levels ≥ 300 μmol L–1, Rees et al., 2012). ΔdO2 levels, calculated as the difference from ambient seawater, were equal to −91.32 ± 5.02 1SE μmol L–1 in jellyfish collected during the night and 252.02 ± 14.62 1SE μmol L–1 in jellyfish collected in the afternoon (LMMs, p < 0.001, Table 1), corresponding to average oxygen levels of 61.92 ± 5.06 1SE μmol L–1 during the night and 546.22 ± 16.45 1SE μmol L–1 during the afternoon (Figure 2A). Our results showed an dO2 increase of ∼ 480 μmol L–1 between night and afternoon, and a significant difference in dO2 with depth in the oral arms (Table 1). Values of ΔdO2 increased in the first part of the oral arms (0–600 μm) and remained fairly stable deeper into the oral arms (Figure 3A) in afternoon measurements. Similarly, but following an opposite trend, ΔdO2 decreased (0–600 μm) and then reached a plateau in night measurements.
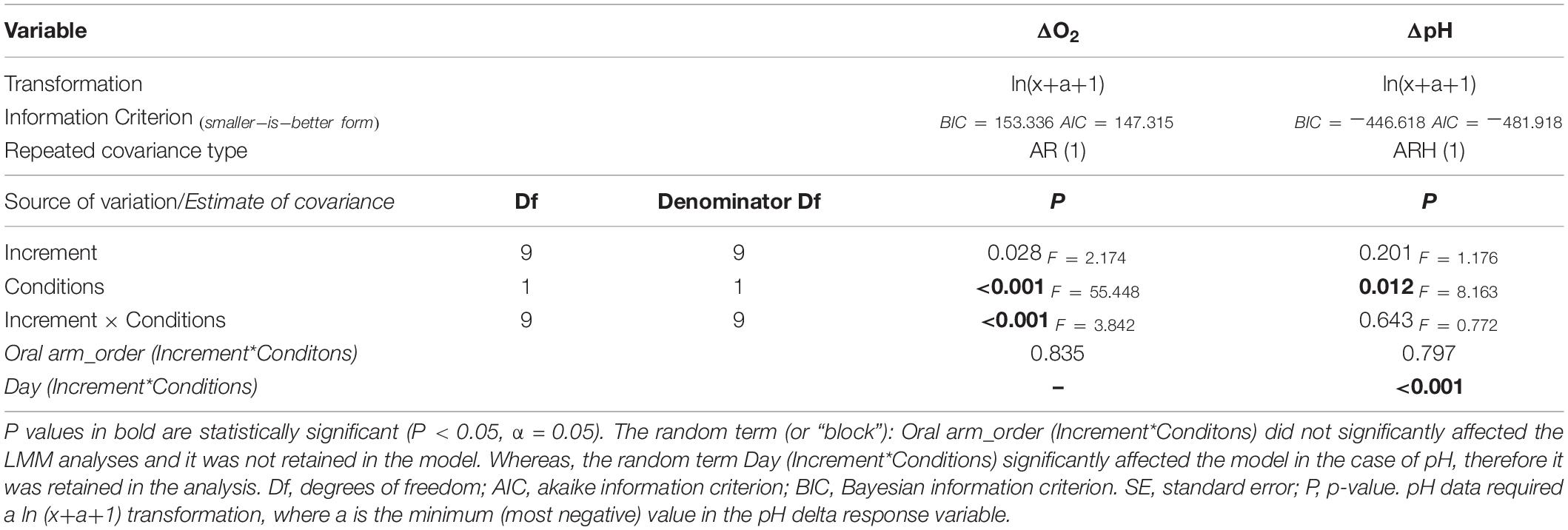
Table 1. Repeated Linear Mixed Models (LMMs) comparing pH and oxygen (to ambient levels) between the two considered conditions (night and afternoon).
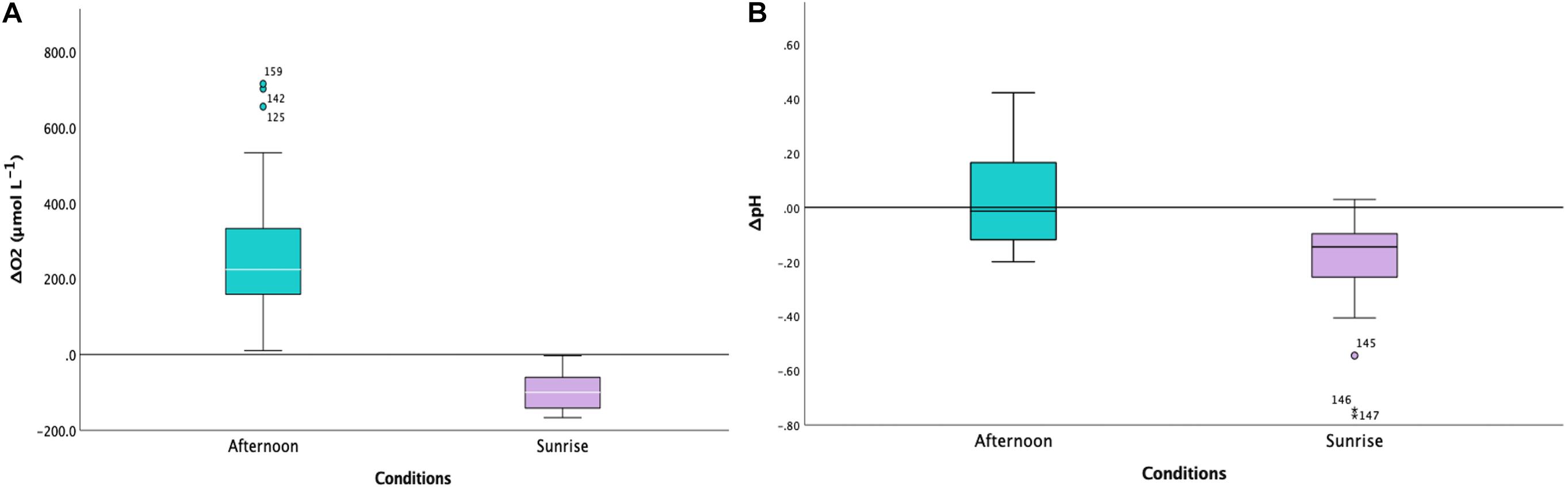
Figure 2. (A) Boxplot indicating the levels of ΔdO2 (to ambient levels in μmol L− 1) in Cassiopea sp. oral arms for the two conditions (afternoon, in turquoise, and sunrise, in lilac; n = 3). The difference between the two conditions was highly statistically significant (LMMs, p < 0.001, Table 1). The white horizontal line in the center of boxes represents the median value of data, the edges of the boxes are quartile and error bars are the extreme. (B) Boxplot indicating the levels of ΔpH (to ambient level) in Cassiopea sp. oral arms for the two conditions (afternoon, in turquoise, and sunrise, in lilac; n = 3). The difference between the two conditions was statistically significant (LMMs, p = 0.012, Table 1). The white horizontal line in the center of boxes represents the median value of data, the edges of the boxes are quartile and error bars are the extreme.
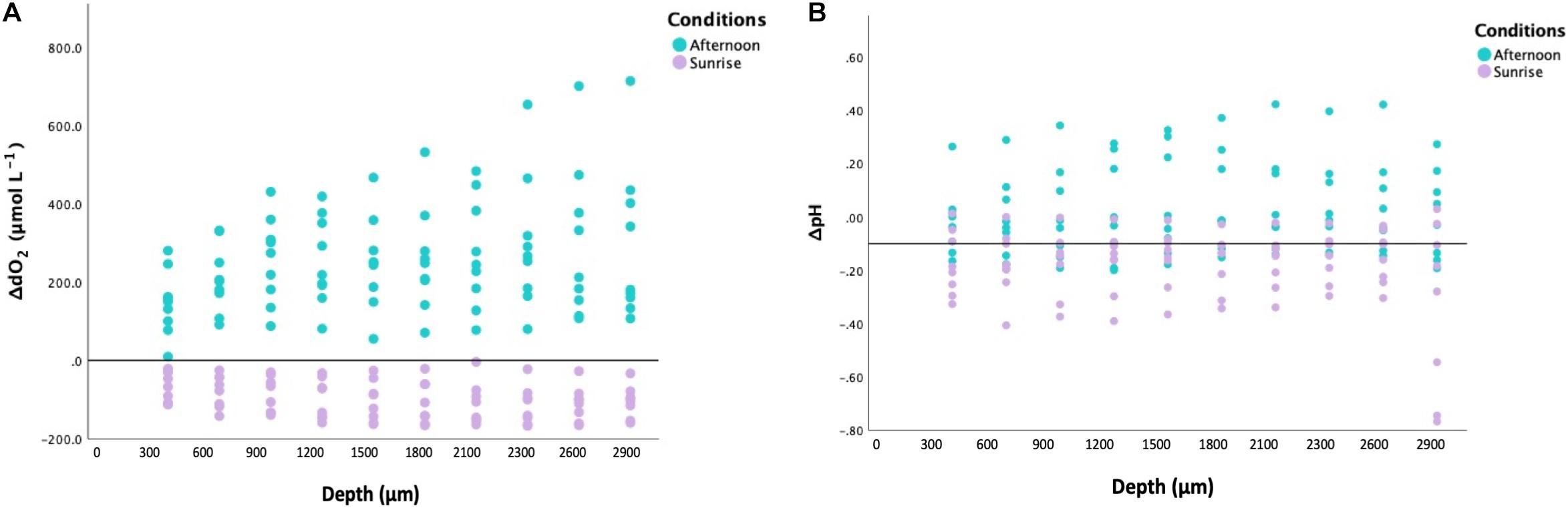
Figure 3. (A) Variability of ΔdO2 (relative to ambient levels in μmol L− 1; n = 3) within Cassiopea sp. oral arms (depth on X-axis in μm). In turquoise: the values obtained from specimens collected during the afternoon. In lilac: the values obtained from specimens collected at sunrise. (B) Variability of ΔpH (relative to ambient levels in μmol L− 1; n = 3) within Cassiopea sp. oral arms (depth on X-axis in μm). In turquoise: the values obtained from specimens collected during the afternoon. In lilac: the values obtained from specimens collected at sunrise.
Oxygen levels started to increase immediately after the animals were placed in the dark, and decreased when returned to light conditions, reaching a plateau in individuals collected during the night (Figure 4, Mann-Whitney, p < 0.001, N = 686).
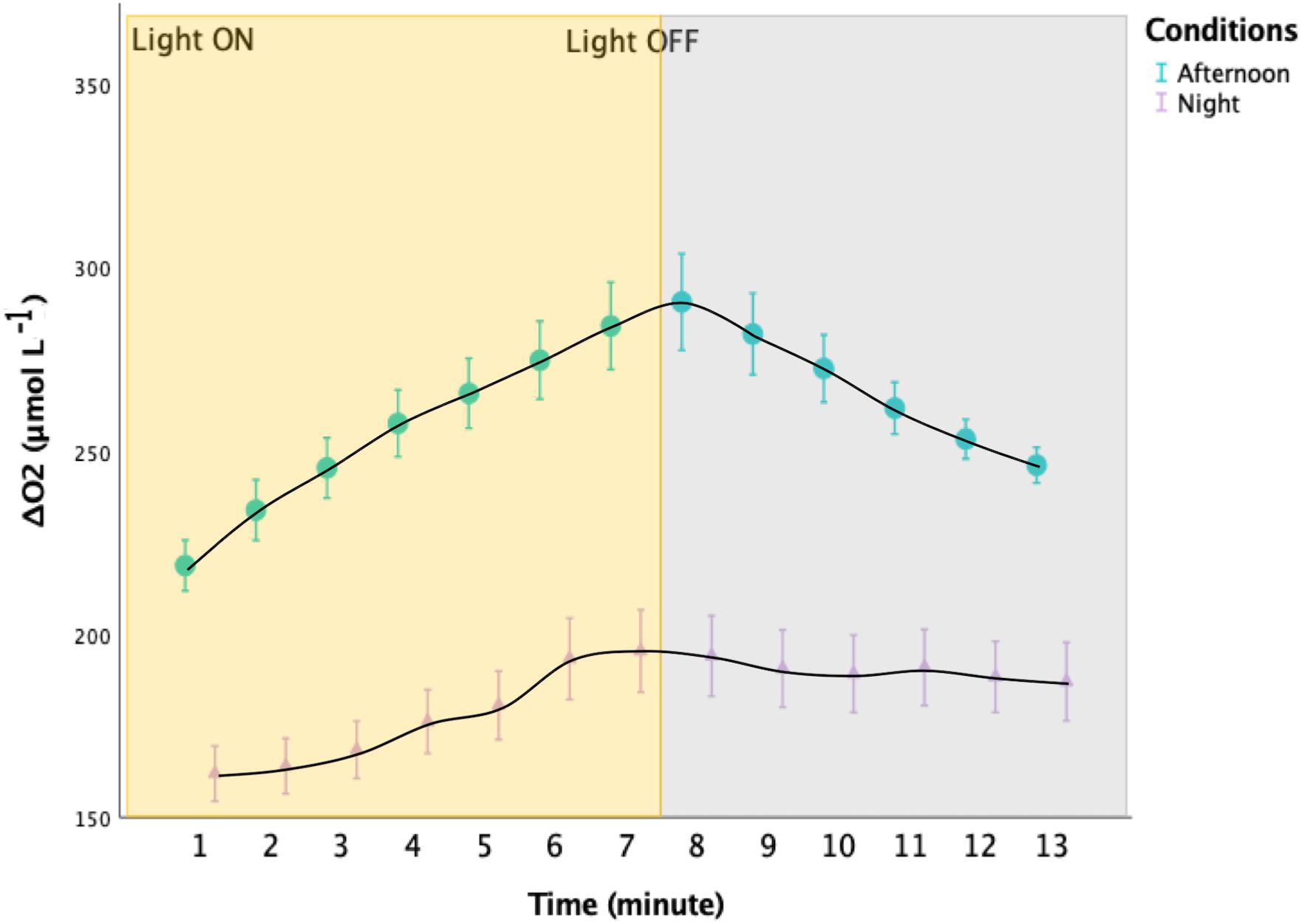
Figure 4. Oxygen variations in the upper ∼1 mm of the oral arms of Cassiopea sp. during experimental light-dark shifts. Incident light was ∼1091 μmol photons m− 2 s− 1. The yellow rectangle indicates when the light where turned ON, whereas the gray rectangle indicates when the LEDs were turned OFF. In turquoise: the values obtained from three specimens collected during the afternoon. In lilac: the values obtained from three specimens collected at sunrise. The two distributions are significantly different (Mann-Whitney, p < 0.001; n = 3). Error bars represent the extreme values.
pH Dynamics in Oral Arms
Diel fluctuations of pH in the oral arms were confirmed by our study. Average pH levels in Cassiopea sp. oral arms, calculated as the difference from ambient seawater, were equal to −0.18 ± 0.01 1SE pH units in individuals collected during the night and 0.06 ± 0.01 1SE pH units in individuals collected in the afternoon, with average pH levels ranging from 7.93 ± 0.02 1SE pH units during the night to 8.61 ± 0.02 1SE pH units during the afternoon (Figure 2B, LMMs, p = 0.012, Table 1). The change in pH between night and afternoon was equal to 0.68 pH units, and pH did not change significantly with depth into the oral arms (Table 1 and Figure 3B).
Chlorophyll Content and Symbiont Density
Average chlorophyll-a (chl-a) content, chlorophyll-c (chl-c) content and symbiont density were, respectively, equal to 0.19 ± 0.00 1SE μg ml–1 mg protein–1, 0.07 ± 0.00 1SE μg ml–1 mg protein –1, and 1165.26 ± 80.35 1SE cells protein–1 (0.24 ± 0.02 μg ml–1 mg protein–1, 0.08 ± 0.00 1SE μg ml–1 mg protein –1, and 1298.24 ± 132.39 1SE cells protein –1 at night and 0.20 ± 0.00 1SE μg ml–1 mg protein–1, 0.08 ± 0.01 1SE μg ml–1 mg protein –1, and 1009.17 ± 69.95 1SE cells protein –1 during the afternoon). ΔdO2 was significantly, but negatively, correlated with chl-a (Pearson’s correlation test, r = −0.82, p-value = 0.01, N = 8) for individuals collected at night. However, no correlation was found with any other parameter analyzed. ΔpH was negatively correlated with chl-c content (Pearson’s correlation test, r = −0.79, p-value = 0.02, N = 8) in individuals collected in the afternoon, but no other significant correlation was found.
Photosynthetic Rates and Photochemical Efficiency (Fv/Fm)
Photochemical efficiency measurements of individuals collected during the night averaged 0.67 ± 1SE 0.01, while that of individuals collected in the afternoon averaged 0.41 ± 1SE 0.02. Gross photosynthetic rates were estimated at 100 μm intervals using microsensors. These rates averaged 0.04 ± 0.013 1SE nmol cm–2 s–1 in individuals collected during the night and 0.08 ± 0.02 1SE nmol cm–2 s–1 in individuals collected during the afternoon. Photosynthetic rates did not follow a specific a consistent trend within depth into the oral arms (LMMs, Compound Symmetry, Numerator df = 1, Denominator df = 4, F = 30.897, p-value = 0.171; Figure 5). We calculated that depth-integrated (0–600 μm) photosynthesis was 88.53 ± 0.94 1SE nmol cm–2 s–1 and 156.51 ± 1.17 1SE nmol cm–2 s–1, respectively, in specimens collected during the night and specimens collected during the afternoon.
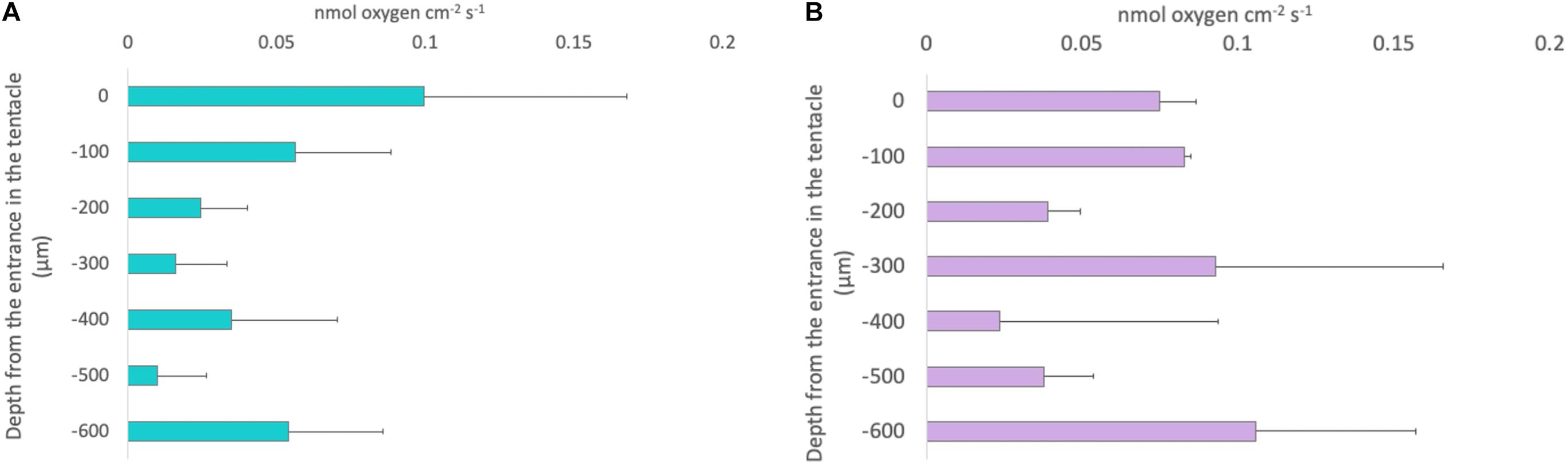
Figure 5. Depth profiles of gross photosynthesis in nmol cm− 2 s− 1 in Cassiopea sp. oral arms measured using the light-dark shift method. Each bar represents the gross photosynthesis measured at that specific depth within the oral arms. (A) Turquoise bars: the values of gross photosynthesis obtained form specimens collected during the afternoon. (B) Lilac bars: the values obtained from specimens collected at sunrise. Depth profiles are not significantly different between the two conditions (LMMs, Compound Symmetry, Numerator df = 1, Denominator df = 4, F = 30.897, p-value = 0.171; n = 3). Error bars represent the extreme values.
Discussion
Our results confirmed that Cassiopea sp. symbionts create a dynamic microenvironment, characterized by daily fluctuations of dO2 and pH levels, driven by symbiont photosynthesis during the day and holobiont respiration during the night. The light-dependent balance between respiration and photosynthesis leads to broad diel changes in environmental conditions inside the host’s oral arms (Shashar and Stambler, 1992; Kühl et al., 1995).
Extreme diel fluctuations of dO2 and pH have already observed in other cnidarian species such as symbiotic corals (Kühl et al., 1995; Al-Horani et al., 2003; Linsmayer et al., 2020) and sea anemones (Dykens and Shick, 1982). These studies observed extreme conditions within the holobionts and hypothesized that Symbiodiniaceae play a major role in both driving the host’s microenvironment and influencing their biology (Gardella and Edmunds, 1999). Variations of oxygen observed in Cassiopea sp. were comparable to those reported in the literature (e.g., from ∼ 710 μmol L–1 in the light to ∼ 5 μmol L–1 in the dark (Kühl et al., 1995); from ∼ 450 μmol L–1 in the light to ∼ 20 μmol L–1 in the dark (Shashar and Stambler, 1992); from ∼ 381 μmol L–1 in the day to ∼ 74 μmol L–1 at night (Linsmayer et al., 2020; Table 2). Kühl et al. (1995) showed that oxygen can change ∼ 745 μmol L–1 from darkness to light in the scleractinian coral Acropora sp., and Shashar and Stambler (1992) observed an O2 variation of ∼ 430 μmol L–1 in the coral Porites compressa. Dykens and Shick (1982) also showed that in the sea anemone Anthopleura elegantissima oxygen could reach ∼ 600 μmol L–1 in the light and ∼ 183 μmol L–1 in the dark, with a variation of ∼ 400 μmol L–1. Also, variations of pH reported in this study were comparable with those observed in previous experiments (e.g., from 7.3 in the dark to 8.5 in the artificial light (Kühl et al., 1995); from 7.7 in the dark to 8.5 in the daylight (Shashar and Stambler, 1992); from 7.4 in the dark to 8.9 in the artificial light (Furla et al., 1998; Table 2). Kühl et al. (1995) showed that pH change from darkness to artificial light is ∼ 1.2 pH units in the scleractinian coral Acropora sp., and Shashar and Stambler (1992) observed a pH variations of ∼ 0.8 pH units in the coral Porites compressa. Furla et al. (1998) found that in sea anemones the pH changes is ∼ 1.5 pH units. We conclude that symbiotic cnidarians and symbiotic dinoflagellates must be adapted to properly function under such extreme conditions (Shashar et al., 1993), which include potential exposure to oxidative stress. Indeed, several studies have observed a wide variety of protective enzymes, such as superoxide dismutase, catalase, and ascorbate peroxidase in symbiotic cnidarians (Shick and Dykens, 1985; Shashar and Stambler, 1992). In addition to such protective strategies, Cassiopea sp. might increase its own tolerance levels by contracting its bell. This behavior facilitates water and gas exchange within the jellyfish body (Kremien et al., 2013), thus likely reducing potential negative effects of extreme diel fluctuations of oxygen and pH.
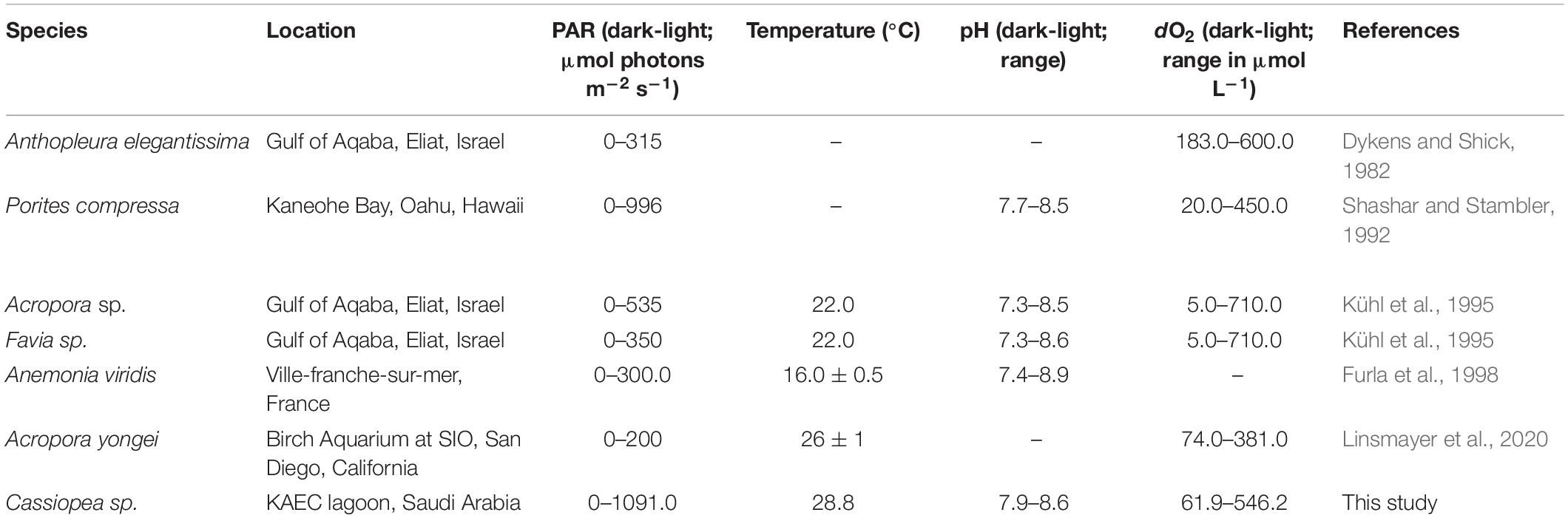
Table 2. Summary of oxygen and pH fluctuations in the body of different cnidarian and a comparison with findings deriving from this study.
In our study, measurements taken before sunrise showed that the internal dO2 and pH levels were substantially lower than those in ambient seawater. Average levels of oxygen and pH were 61.92 ± 5.06 1SE μmol dO2 L–1 and 7.93 ± 0.02 1SE, respectively, thus indicating that the conditions inside Cassiopea’s oral arms were hypoxic and acidified relative to the surrounding seawater, as a consequence of night-time respiration of the holobiont. Indeed, during the night, oxygen is quickly consumed and hypoxia is reached; these conditions are coupled with an increase of CO2 and therefore a decrease in pH (Shashar and Stambler, 1992). Exposure to extreme low pH levels may significantly affect the physiology of the holobiont, but cnidarians cells are able to buffer these changes and maintain their internal pH within a physiological range and recover quickly (Laurent et al., 2014).
Hypoxia during the night might be further facilitated by Cassiopea sp.’s activity patterns (Nath et al., 2017). Bell pulsation rates are usually lower during the night period than during the day, thus likely limiting the water flushing and oxygen supply, as well as removal of byproducts and ventilation of excess CO2 (Nath et al., 2017). In individuals collected before sunrise, oxygen changes happened rapidly after switching off the light (Figure 4), demonstrating the role of the holobiont photosynthesis-respiration metabolic balance in affecting the internal conditions in Cassiopea sp.’s oral arms (Shashar et al., 1993). The decrease in oxygen availability during the night makes oxygen supply to the symbionts diffusion-limited, therefore forcing them to hypoxic conditions, as suggested by Gardella and Edmunds (1999).
The characterization of the internal microenvironment in cnidarian species may inform the use of environmentally relevant conditions during experimental procedures and the development of optimized protocols for culturing symbionts. For instance, Symbiodiniaceae have been successfully extracted from hosts, and cultured in vitro, however, it remains unclear whether long-term culturing conditions provide the same environmental conditions as in hospite (Kühl et al., 1995).
Individuals collected during the afternoon showed internal oxygen levels dropping right after switching off the LEDs. Hence, hyperoxia in oral arms of Cassiopea sp. might happen right after sunset, as Shashar et al. (1993) already stated. In contrast, Cassiopea sp. experiences sustained hyperoxia during the daytime, but is able to cope with these conditions due to the presence of protective enzymes (Shick and Dykens, 1985; Shashar and Stambler, 1992). Day-time hyperoxic conditions can lead to an increase in host respiration (Shick, 1990) and to a decrease in symbionts photosynthesis as well (Shashar et al., 1993). However, hyperoxia may also have beneficial effects. Indeed, recent research showed that exposure to daytime hyperoxia exerts a protective role from elevated temperatures (Giomi et al., 2019).
Depth profiles of ΔdO2 showing that ΔdO2 increases in the first part of the oral arm and then reaches a plateau during the afternoon suggests that photosynthetic activity of the symbionts affects the external part of the oral arms while an equilibrium between oxygen diffusion and respiratory consumption is reached in the inner part of the oral arm. Whereas, depth profiles of ΔpH are subjected to overall changes according to light conditions, they are quite stable within the first millimeters of oral arms and similar to ambient levels. This suggests the presence of recovery strategies and the ability of cnidarian cells to maintain pH within specific physiological ranges, which are similar to those in ambient seawater (Laurent et al., 2013).
Difficulties encountered during our study were similar to the limitations described by Kühl et al. (1995). Firstly, jellyfish react to environmental stimuli, therefore physical disturbance, such as the microsensor tips, may result in contractions of the tissue. This may lead to both noisy signals and the breakage of the microelectrode. After breaking several sensors because of this problem, we decided to cut the oral arm and keep it still using hypodermic needles. Additionally, Cassiopea sp. produces enormous quantities of mucus, which often covered the tip of the microsensor and interfered with measurements, hence, making repeat experiments necessary. We also did our best to mimic environmental light and thermal conditions in the laboratory, to avoid any eventual effect on our measurements. Since we tried to take the measurements within maximum 1 h, we managed to investigate oxygen and pH within the first couple of millimeters of tissue (see Kühl et al., 1995), where most symbionts are harbored and which are likely to be the most affected by diel fluctuation.
Although jellyfish were chosen by the presence of morphological similarities, (e.g., presence appendages of similar size, similar colored vesicles, etc.), inconsistencies among them might contribute to differences in symbiont density, chlorophyll and protein content.
Although our study contribute to supporting the role of photosynthetic dinoflagellates in controlling the internal microenvironment of cnidarian species, further studies are needed to (1) better understand the metabolic dynamics, (2) investigate CO2 fluctuations within the oral arm during day and night, as well as their role in driving pH levels, (3) assess eventual effects at cell level and individuate biochemical processes that might protect cnidarian cells form such extreme diel variations, and (4) the role of the broader microbiome.
Conclusion
Our work has further provided results showing the large fluctuations occurring in the internal microenvironment of holobiont organisms containing photosymbionts (O2 and pH). These conditions range from hyperoxia, during daytime, to hypoxia, during the nighttime. As hyperoxia has been shown to enhance the thermal resistance of marine animals (Giomi et al., 2019), our results lay the basis for further studies investigating the potential role of internal hyperoxia in moderating the responses of cnidarians to thermal stress. The hypothesis that hyperoxia brought about by symbiont activity enhances thermal resistance is particularly important as thermal-induced bleaching becomes a source of loss to coral reefs, and need be tested, possibly by using model organisms that can be maintained in both symbiotic and aposymbiotic state.
Data Availability Statement
The raw data supporting the conclusions of this article will be made available by the authors, without undue reservation.
Author Contributions
CMD, MA, and SGK conceived the initial concept and designed the experiments. SA, AS, and AJP performed sampling of the specimens. SA, AB, MC, and S-HH performed the samples analyses. SGK and SA conducted the data analyses. SA wrote the first draft of the manuscript and prepared the tables, Supplementary Materials, and display figures. All authors contributed to improve the manuscript and approved the submission.
Funding
Financial support for this research was provided by the King Abdullah University of Science and Technology and the Tarek Ahmed Juffali Research Chair on Red Sea Ecology including the baseline research funds of CMD and DD.
Conflict of Interest
The authors declare that the research was conducted in the absence of any commercial or financial relationships that could be construed as a potential conflict of interest.
Publisher’s Note
All claims expressed in this article are solely those of the authors and do not necessarily represent those of their affiliated organizations, or those of the publisher, the editors and the reviewers. Any product that may be evaluated in this article, or claim that may be made by its manufacturer, is not guaranteed or endorsed by the publisher.
Acknowledgments
We thank the team of KAUST Coastal and Marine Resources Core Labs (CMOR) for their support and assistance.
Supplementary Material
The Supplementary Material for this article can be found online at: https://www.frontiersin.org/articles/10.3389/fmars.2021.705915/full#supplementary-material
References
Al-Horani, F. A., Al-Moghrabi, S. M., and de Beer, D. (2003). Microsensor study of photosynthesis and calcification in the scleractinian coral, Galaxea fascicularis: active internal carbon cycle. J. Exp. Mar. Biol. Ecol. 288, 1–15. doi: 10.1016/s0022-0981(02)00578-6
Brune, A., Emerson, D., and Breznak, J. A. (1995). The termite gut microflora as an oxygen sink: microelectrode determination of oxygen and pH gradients in guts of lower and higher termites. Appl. Environ. Microbiol. 61, 2681–2687. doi: 10.1128/aem.61.7.2681-2687.1995
Brune, A., and Kühl, M. (1996). pH profiles of the extremely alkaline hindguts of soil-feeding termites (Isoptera: Termitidae) determined with microelectrodes. J. Insect Physiol. 42, 1121–1127. doi: 10.1016/s0022-1910(96)00036-4
Brune, A. (1998). Termite guts: the world’s smallest bioreactors. Trends Biotechnol. 16, 16–21. doi: 10.1016/s0167-7799(97)01151-7
Brune, A., and Friedrich, M. (2000). Microecology of the termite gut: structure and function on a microscale. Curr. Opin. Microbiol. 3, 263–269. doi: 10.1016/s1369-5274(00)00087-4
Davy, S. K., Allemand, D., and Weis, V. M. (2012). Cell biology of cnidarian-dinoflagellate symbiosis. Microbiol. Mol. Biol. Rev. 76, 229–261. doi: 10.1128/mmbr.05014-11
de Beer, D., Kühl, M., Stambler, N., and Vaki, L. (2000). A microsensor study of light enhanced Ca2+ uptake and photosynthesis in the reef-building hermatypic coral Favia sp. Mar. Ecol. Progr. Series 194, 75–85. doi: 10.3354/meps194075
de Groot, R., Brander, L., Van Der Ploeg, S., Costanza, R., Bernard, F., Braat, L., et al. (2012). Global estimates of the value of ecosystems and their services in monetary units. Ecosyst. Serv. 1, 50–61. doi: 10.1016/j.ecoser.2012.07.005
Dykens, J. A., and Shick, J. M. (1982). Oxygen production by endosymbiotic algae controls superoxide dismutase activity in their animal host. Nature 297, 579–580. doi: 10.1038/297579a0
Done, T. J., Ogden, J. C., Weibe, W. J., and Rosen, B. R. (1996). “Biodiversity and ecosystem function of coral reefs,” in Functional Roles of Biodiversity: A Global Perspective, eds N. H. A. Mooney, J. H. Cushman, E. Medina, O. E. Sala, and E. D. Schulze (Hoboken, NJ: Wiley), 393–427.
Falkowski, P. G., Dubinsky, Z., Muscatine, L., and Porter, J. W. (1984). Light and the bioenergetics of a symbiotic coral. Bioscience 34, 705–709. doi: 10.2307/1309663
Fitt, W. K. (2000). Cellular growth of host and symbiont in a cnidarian-zooxanthellar symbiosis. Biol. Bull. 198, 110–120. doi: 10.2307/1542809
Fitt, W. K., and Trench, R. K. (1983). The relation of diel patterns of cell division to diel patterns of motility in the symbiotic dinoflagellate Symbiodinium microadria ticum Freudenthal in culture. New Phytol. 94, 421–432. doi: 10.1111/j.1469-8137.1983.tb03456.x
Fransolet, D., Roberty, S., and Plumier, J. C. (2012). Establishment of endosymbiosis: The case of cnidarians and Symbiodinium. J. Exp. Mar. Biol. Ecol. 420, 1–7. doi: 10.1016/j.jembe.2012.03.015
Furla, P., Bénazet-Tambutté, S., Jaubert, J., and Allemand, D. (1998). Functional polarity of the oral arm of the sea anemone Anemonia viridis: role in inorganic carbon acquisition. Am. J. Physiol. 274, R303–R310. doi: 10.1002/(sici)1097-010x(19970901)279:1<1::aid-jez1>3.0.co;2-t
Gardella, D. J., and Edmunds, P. J. (1999). The oxygen microenvironment adjacent to the tissue of the scleractinian Dichocoenia stokesii and its effects on symbiont metabolism. Mar. Biol. 135, 289–295. doi: 10.1007/s002270050626
Gattuso, J. P., Allemand, D., and Frankignoulle, M. (1999). Photosynthesis and calcification at cellular, organismal and community levels in coral reefs: a review on interactions and control by carbonate chemistry. Am. Zool. 39, 160–183. doi: 10.1093/icb/39.1.160
Giomi, F., Barausse, A., Duarte, C. M., Booth, J., Agusti, S., Saderne, V., et al. (2019). Oxygen supersaturation protects coastal marine fauna from ocean warming. Sci. Adv. 5:eaax1814. doi: 10.1126/sciadv.aax1814
Glud, R. N., Jensen, K., and Revsbech, N. P. (1995). Diffusivity in surficial sediments and benthic mats determined by use of a combined N2O-O2 microsensor. Geochim. Cosmochim. Acta 59, 231–237. doi: 10.1016/0016-7037(94)00321-c
Gordon, B. R., and Leggat, W. (2010). Symbiodinium—invertebrate symbioses and the role of metabolomics. Mar. Drugs 8, 2546–2568. doi: 10.3390/md8102546
Gray, S. E., Degrandpre, M. D., Langdon, C., and Corredor, J. E. (2012). Short-term, & seasonal pH, pCO2, & saturation state variability in a coral-reef ecosystem. Cycles Glob. Biogeochem. 26:GB3012.
Hoegh-Guldberg, O., Muller-Parker, G., Cook, C., Gates, R. D., Gladfelter, E., Trench, R., et al. (2007). Len Muscatine (1932–2007) and his contributions to the understanding of algal-invertebrate endosymbiosis. Coral Reef 26, 731–739. doi: 10.1007/s00338-007-0320-0
Hofmann, D., Neumann, R., and Henne, K. (1978). Strobilation, budding and initiation of scyphistoma morphogenesis in the rhizostome Cassiopea andromeda (Cnidaria: Scyphozoa). Mar. Biol. 47, 161–176. doi: 10.1007/bf00395637
Hofmann, D. K., Fitt, W. K., and Fleck, J. (1996). Checkpoints in the life-cycle of Cassiopea spp.: control of metagenesis and metamorphosis in a tropical jellyfish. Int. J. Dev. Biol. 40, 331–338.
Jensen, J., and Revsbech, N. P. (1989). Photosynthesis and respiration of a diatom biofilm cultured in a new gradient growth chamber. FEMS Microbiol. Ecol. 5, 29–38. doi: 10.1111/j.1574-6968.1989.tb03655.x
Jokiel, P. L. (2011). The reef coral two compartment proton flux model: a new approach relating tissue-level physiological processes to gross corallum morphology. J. Exp. Mar. Biol. Ecol. 409, 1–12. doi: 10.1016/j.jembe.2011.10.008
Kennedy, E. V., Perry, C. T., Halloran, P. R., Iglesias-Prieto, R., Schönberg, C. H., Wisshak, M., et al. (2013). Avoiding coral reef functional collapse requires local and global action. Curr. Biol. 23, 912–918. doi: 10.1016/j.cub.2013.04.020
Klein, S. G., Pitt, K. A., and Carroll, A. R. (2016). Reduced salinity increases susceptibility of zooxanthellate jellyfish to herbicide toxicity during a simulated rainfall event. Environ. Pollut. 209, 79–86. doi: 10.1016/j.envpol.2015.11.012
Klein, S. G., Pitt, K. A., Nitschke, M. R., Goyen, S., Welsh, D. T., Suggett, D. J., et al. (2017). Symbiodinium mitigate the combined effects of hypoxia and acidification on a noncalcifying cnidarian. Glob. Change Biol. 23, 3690–3703. doi: 10.1111/gcb.13718
Klein, S. G., Pitt, K. A., Lucas, C. H., Hung, S. H., Schmidt-Roach, S., Aranda, M., et al. (2019). Night-time temperature reprieves enhance the thermal tolerance of a symbiotic cnidarian. Front. Mar. Sci. 6:453. doi: 10.3389/fmars.2019.00453
Knowlton, N., Brainard, R. E., Fisher, R., Moews, M., Plaisance, L., and Caley, M. J. (2010). “Coral reef biodiversity,” in Life in the World’s Oceans: Diversity Distribution and Abundance, ed. A. D. McIntyre (Hoboken, NJ: Wiley-Blackwell), 65–74.
Kühl, M., Cohen, Y., Dalsgaard, T., Jørgensen, B. B., and Revsbech, N. P. (1995). Microenvironment and photosynthesis of zooxanthellae in scleractinian corals studied with microsensors for O2, pH and light. Mar. Ecol. Prog. Ser. 117, 159–172. doi: 10.3354/meps117159
Kremien, M., Shavit, U., Mass, T., and Genin, A. (2013). Benefit of pulsation in soft corals. Proc. Natl. Ac. Sci. U.S.A. 110, 8978–8983. doi: 10.1073/pnas.1301826110
LaJeunesse, T. C. (2001). Investigating the biodiversity, ecology, and phylogeny of endosymbiotic dinoflagellates in the genus Symbiodinium using the ITS region: in search of a “species” level marker. J. Phycol. 37, 866–880. doi: 10.1046/j.1529-8817.2001.01031.x
LaJeunesse, T. C., Parkinson, J. E., Gabrielson, P. W., Jeong, H. J., Reimer, J. D., Voolstra, C. R., et al. (2018). Systematic revision of Symbiodiniaceae highlights the antiquity and diversity of coral endosymbionts. Curr. Biol. 28, 2570–2580. doi: 10.1016/j.cub.2018.07.008
Lampert, K. P. (2016). “Cassiopea and its zooxanthellae,” in The Cnidaria, Past, Present and Future, eds S. Goffredo and Z. Dubinsky (Berlin: Springer International Publishing), 415–423. doi: 10.1007/978-3-319-31305-4_26
Laurent, J., Tambutté, S., Tambutté, É, Allemand, D., and Venn, A. (2013). The influence of photosynthesis on host intracellular pH in scleractinian corals. J. Exp. Biol. 216, 1398–1404.
Laurent, J., Venn, A., Tambutté, É, Ganot, P., Allemand, D., and Tambutté, S. (2014). Regulation of intracellular pH in cnidarians: response to acidosis in Anemonia viridis. FEBS J. 281, 683–695. doi: 10.1111/febs.12614
Linsmayer, L. B., Deheyn, D. D., Tomanek, L., and Tresguerres, M. (2020). Dynamic regulation of coral energy metabolism throughout the diel cycle. Sci. Rep. 10, 1–11.
Miller, D. J., and Yellowlees, D. (1989). Inorganic nitrogen uptake by symbiotic marine cnidarians: a critical review. Proc. R. Soc. Lond. B. Biol. Sci. 237, 109–125. doi: 10.1098/rspb.1989.0040
Mortillaro, J., Pitt, K., Lee, S., and Meziane, T. (2009). Light intensity influences the production and translocation of fatty acids by zooxanthellae in the jellyfish Cassiopea sp. J. Exp. Mar. Biol. Ecol. 378, 22–30. doi: 10.1016/j.jembe.2009.07.003
Muscatine, L. (1967). Glycerol excretion by symbiotic algae from corals and Tridacna and its control by the host. Science 156, 516–519. doi: 10.1126/science.156.3774.516
Muscatine, L., and Porter, J. (1977). Reef corals: mutualistic symbiosis adapted to nutrient-poor environments. Bioscience 27, 454–460. doi: 10.2307/1297526
Muscatine, L., Falkowski, P., Porter, J., and Dubinsky, Z. (1984). Fate of photosynthetically-fixed carbon in light and shade-adapted colonies of the symbiotic coral Stylophora pistillata. Proc. R. Soc. Lond. B. 222, 181–202. doi: 10.1098/rspb.1984.0058
Muscatine, L. (1990). The role of symbiotic algae in carbon and energy flux in reef corals. Coral Reefs 25, 75–87.
Muscatine, L., Goiran, C., Land, L., Jaubert, J., Cuif, J. P., and Allemand, D. (2005). Stable isotopes (δ13C and δ15N) of organic matrix from coral skeleton. Proc. Natl. Acad. Sci. U.S.A. 102, 1525–1530. doi: 10.1073/pnas.0408921102
Nath, R. D., Bedbrook, C. N., Abrams, M. J., Basinger, T., Bois, J. S., Prober, D. A., et al. (2017). The jellyfish Cassiopea exhibits a sleep-like state. Curr. Biol. 27, 2984–2990. doi: 10.1016/j.cub.2017.08.014
Ohdera, A. H., Abrams, M. J., Ames, C. L., Baker, D. M., Suescún-Bolívar, L. P., Collins, A. G., et al. (2018). Upside-down but headed in the right direction: review of the highly versatile Cassiopea xamachana system. Front. Ecol. Evol. 6:35. doi: 10.3389/fevo.2018.00035
Polerecky, L., Lott, C., and Weber, M. (2008). In situ measurement of gross photosynthesis using a microsensor-based light-shade shift method. Limnol. Ocean. Methods 6, 373–383. doi: 10.4319/lom.2008.6.373
Razali, N. M., and Wah, Y. B. (2011). Power comparisons of shapiro-wilk, interactio-smirnov, interactio and interact-darling tests. J. Stat. Modeling Anal. 2, 21–33.
Rees, B. B., Targett, T. E., Ciotti, B. J., Tolman, C. A., Akkina, S. S., and Gallaty, A. M. (2012). Temporal dynamics in growth and white skeletal muscle composition of the mummichog Fundulus heteroclitus during chronic hypoxia and hyperoxia. J. Fish Biol. 81, 148–164. doi: 10.1111/j.1095-8649.2012.03319.x
Revsbech, N. P., JØrgensen, B. B., and Brix, O. (1981). Primary production of microalgae in sediments measured by oxygen microprofile, H14CO3-fixation, and oxygen exchange methods 1. Limnol. Oceanogr. 26, 717–730. doi: 10.4319/lo.1981.26.4.0717
Revsbech, N. P., and Jorgensen, B. B. (1983). Photosynthesis of benthic microflora measured with high spatial resolution by the oxygen microprofile method: capabilities and limitations of the method 1. Limnol.Oceanogr. 28, 749–756. doi: 10.4319/lo.1983.28.4.0749
Ritchie, R. J. (2006). Consistent sets of spectrophotometric chlorophyll equations for acetone, methanol and ethanol solvents. Photosynth. Res. 89, 27–41. doi: 10.1007/s11120-006-9065-9
Schönberg, H. C. L., De Beer, D., and Lawton, A. (2005). Oxygen microsensor studies on zooxanthellate clionaid sponges from the Costa Brava, Mediterranean Sea 1. J. Phycol. 41, 774–779. doi: 10.1111/j.0022-3646.2005.04226.x
Shapiro, S. S., and Wilk, M. B. (1965). An analysis of variance test for normality (complete samples). Biometrika 52, 591–611. doi: 10.2307/2333709
Shashar, N., and Stambler, N. (1992). Endolithic algae within corals-life in an extreme environment. J. Exp. Mar. Biol. Ecol. 163, 277–286. doi: 10.1016/0022-0981(92)90055-f
Shashar, N., Cohen, Y., and Loya, Y. (1993). Extreme diel fluctuations of oxygen in diffusive boundary layers surrounding stony corals. Biol. Bull. 185, 455–461. doi: 10.2307/1542485
Shick, J. M. (1990). Diffusion limitation and hyperoxic enhancement of oxygen consumption in zooxanthellate sea anemones, zoanthids, and corals. Biol. Bull. 179, 148–158. doi: 10.2307/1541749
Shick, J. M., and Dykens, J. A. (1985). Oxygen detoxification in algal-invertebrate symbioses from the Great Barrier Reef. Oecologia 66, 33–41. doi: 10.1007/BF00378549
Smith, G. J., and Muscatine, L. (1999). Cell cycle of symbiotic dinoflagellates: variation in G1 phase-duration with anemone nutritional status and macronutrient supply in the Aiptasia pulchella–Symbiodinium pulchrorum symbiosis. Mar. Biol. 134, 405–418. doi: 10.1007/s002270050557
Wangpraseurt, D., Polerecky, L., Larkum, A. W., Ralph, P. J., Nielsen, D. A., Pernice, M., et al. (2014). The in situ light microenvironment of corals. Limnol. Oceanogr. 59, 917–926. doi: 10.4319/lo.2014.59.3.0917
Wangpraseurt, D., Weber, M., Røy, H., Polerecky, L., De Beer, D., and Nugues, M. M. (2012). In situ oxygen dynamics in coral-algal interactions. PLoS One 7:e31192. doi: 10.1371/journal.pone.0031192
Welsh, D. T., Dunn, R. J., and Meziane, T. (2009). Oxygen and nutrient dynamics of the upside down jellyfish (Cassiopea sp.) and its influence on benthic nutrient exchanges and primary production. Hydrobiologia 635, 351–362. doi: 10.1007/s10750-009-9928-0
Keywords: oxygen, pH, photosynthesis, microsensors, endosymbiosis, Symbiodiniaceae, Cassiopea sp.
Citation: Arossa S, Barozzi A, Callegari M, Klein SG, Parry AJ, Hung S-H, Steckbauer A, Aranda M, Daffonchio D and Duarte CM (2021) The Internal Microenvironment of the Symbiotic Jellyfish Cassiopea sp. From the Red Sea. Front. Mar. Sci. 8:705915. doi: 10.3389/fmars.2021.705915
Received: 06 May 2021; Accepted: 17 June 2021;
Published: 23 July 2021.
Edited by:
Satya Panigrahi, Indira Gandhi Centre for Atomic Research (IGCAR), IndiaReviewed by:
Ajit Kumar Mohanty, Indira Gandhi Centre for Atomic Research (IGCAR), IndiaAki Ohdera, California Institute of Technology, United States
Copyright © 2021 Arossa, Barozzi, Callegari, Klein, Parry, Hung, Steckbauer, Aranda, Daffonchio and Duarte. This is an open-access article distributed under the terms of the Creative Commons Attribution License (CC BY). The use, distribution or reproduction in other forums is permitted, provided the original author(s) and the copyright owner(s) are credited and that the original publication in this journal is cited, in accordance with accepted academic practice. No use, distribution or reproduction is permitted which does not comply with these terms.
*Correspondence: Silvia Arossa, c2lsdmlhLmFyb3NzYUBrYXVzdC5lZHUuc2E=