- 1Department of Arctic and Marine Biology, UiT The Arctic University of Norway, Tromsø, Norway
- 2Institute of Marine Research, Bergen, Norway
- 3Norwegian College of Fishery Science, UiT The Arctic University of Norway, Tromsø, Norway
- 4Faculty of Applied Ecology, Agricultural Sciences and Biotechnology, Inland Norway University of Applied Sciences, Elverum, Norway
- 5Department of Arctic Biology, The University Centre in Svalbard, Longyearbyen, Norway
- 6Centre for Autonomous Marine Operations and System, Department of Biology, Norwegian University of Science and Technology, Trondheim, Norway
Phyto- and zooplankton in Arctic and sub-Arctic seas show very strong seasonal changes in diversity and biomass. Here we document the seasonal variability in the mesozooplankton community structure in a sub-Arctic fjord in Northern Norway based on monthly sampling between November 2018 and February 2020. We combined traditional morphological zooplankton identification with DNA metabarcoding of a 313 base pair fragment of the COI gene. This approach allowed us to provide the most detailed mesozooplankton species list known for this region across an entire year, including both holo- and meroplankton. The zooplankton community was dominated by small copepods throughout the sampling period both in terms of abundance and relative sequence counts. However, meroplankton was the most diverse group, especially within the phylum polychaeta. We identified four distinct periods based on the seasonal analysis of the zooplankton community composition. The pre-spring bloom period (February–March) was characterized by low abundance and biomass of zooplankton. The spring bloom (April) was characterized by the presence of Calanus young stages, cirripedia and krill eggs. The spring-summer period (May–August) was characterized by a succession of meroplankton and a relatively high abundance of copepods of the genus Calanus spp. Finally, the autumn-winter period (September–December) was characterized by a high copepod diversity and a peak in abundance of small copepods (e.g., Oithona similis, Acartia longiremis, Pseudocalanus acuspes, Pseudocalanus elongatus, Pseudocalanus moultoni, Pseudocalanus minutus). During this period, we also observed an influx of boreal warm-water species which were notably absent during the rest of the year. Both the traditional community analysis and metabarcoding were highly complementary and with a few exceptions showed similar trends in the seasonal changes of the zooplankton community structure.
Introduction
Marine ecosystems in Arctic and sub-Arctic regions are governed by strong seasonality in incoming solar radiation, leading to distinct seasonal peaks in primary production. Additionally, nutrient availability, relevant to algal growth, is governed by stratification and mixing of water masses, which again are affected by the seasonality in snow and ice melt, river run-off, wind mixing and solar radiation, in addition to algal nutrient uptake dynamics. Herbivorous zooplankton, which plays an essential role in marine ecosystems at high latitudes, tunes their life cycle to the seasonality in primary production, often using lipid stores to survive the non-productive period. The relatively short and intense growing season necessitates a good synchronization between life history events in zooplankton grazers (such as reproduction and growth) and the productive periods of the primary producers. This synchronization allows the acquisition and accumulation of energy and an efficient energy transfer to higher trophic levels. The dark winter season is particularly poorly studied for activity of phyto- and zooplankton, although recent research demonstrated that this season is by no means a period of inactivity, and several trophic levels remain active and complete important parts of the life cycles in the dark season (Berge et al., 2020; Johnsen et al., 2020).
Mesozooplankton is a key link in the energy transfer between primary producers and higher trophic levels (Steele, 1974; Arnkvaern et al., 2005). They include animals that permanently live in the water column (holoplankton) and those who only spend their larval stage as plankton (meroplankton). Zooplankton abundance, diversity and distribution are considered good indicators of the state of marine ecosystems (Hughes, 2000; Taylor et al., 2002; Hays et al., 2005). They are strongly influenced by hydrography and currents, with advection being an important mode of transport and dispersal, and any changes in the hydrographic regime may affect their distribution and fitness dramatically. Most zooplankton species are not commercially harvested, so changes in distribution and abundance reflect changes in fitness due to environmental forcing (e.g., changes in food availability, predator pressure, and abiotic factors) rather than exploitation, although they can also be influenced by eutrophication and pollution. Furthermore, planktonic life cycles are often short and their population dynamics are not affected by the individual’s ability to persist over many years, so plankton populations rapidly respond to environmental changes (Hays et al., 2005).
Fjords are semi enclosed systems that are a characteristic feature of the Norwegian coastline (Stone, 1980; Cottier et al., 2010). Despite being coastal locations, they can have depths of 200–2000 m and provide habitats for deep-sea communities. Fjords that are separated from the open ocean by a shallow sill are more influenced by local processes, as advection from outside the fjord is reduced. Northern Norwegian fjords can be highly affected by freshwater inflow from either glacial or river discharges and snow melt, causing periods of partial coverage of sea ice. Many northern Norwegian fjords, however, are characterized by weak stratification and are also often influenced by Atlantic water masses (Eilertsen et al., 1981a; Reigstad and Wassmann, 1996) allowing them to remain mostly ice free. Due to their unique properties and often easy accessibility by small vessels, fjords provide excellent long-term sites to study seasonality in community composition and population structure of marine fauna. Most studies on fjord populations have, however, focused on either only single seasons or on specific groups or species, such as Calanus spp., or krill (e.g., Matthews et al., 1978; Bagoien et al., 2000; Niehoff and Hirche, 2005; Skreslet et al., 2015), and there are surprisingly few studies describing the seasonal variability in the zooplankton communities of Norwegian fjords or sub-Arctic fjords elsewhere.
The pelagic ecosystem of Balsfjord is one of the best studied among northern Norwegian fjords (Hopkins et al., 1989) mostly due to numerous studies conducted there in the 1980s (e.g., Eilertsen et al., 1981a; Falk-Petersen and Hopkins, 1981; Tande and Hopkins, 1981; Hopkins et al., 1984). Although located above the Arctic Circle, Balsfjord is not influenced by Arctic water masses and can be regarded as a sub-Arctic fjord. However Balsfjord is one of the coldest fjords in northern Norway due to the presence of a sill at the mouth of the fjord that limits the exchange of deep water (Oug and Høisœter, 2000). Since the 1990’s, seasonal studies of zooplankton in this region have been limited (Wexels Riser et al., 2010; Svensen et al., 2018; Barth-Jensen et al., 2020; Trudnowska et al., 2020; Ershova et al., In revision). The mesozooplankton community in Balsfjord has been defined as poor in diversity but high in biomass (Hopkins, 1981; Hopkins et al., 1989). It was found to be dominated by copepods in terms of species numbers and abundance (Hopkins, 1981), but euphausiids can also be abundant, forming large sound scattering layers (Hopkins et al., 1978) and play an important role in the vertical carbon flux (Wexels Riser et al., 2010). However, despite being a relatively well studied fjord system, seasonal zooplankton investigations in Balsfjord, as elsewhere, have been significantly biased toward a few organisms that are easily identified, i.e., large copepods and euphausiids. For example, the seasonal variability in the population structure, sex-ratio and gonad maturation, body weight, carbon and nitrogen content, and enzyme activities have been well studied for Calanus finmarchicus (Tande and Hopkins, 1981; Tande and Slagstad, 1982; Tande, 1982; Tande and Gronvik, 1983) and Metridia longa (Tande and Gronvik, 1983; Grønvik and Hopkins, 1984; Hopkins et al., 1984; Båmstedt et al., 1985), as have the seasonal changes in lipid composition (Falk-Petersen, 1981; Sargent and Falk-Petersen, 1981; Falk-Petersen et al., 1982; Falk-Petersen, 1985) and population dynamics (Falk-Petersen and Hopkins, 1981) in euphausiids in Balsfjord. However, only a few studies have focused on other parts of the zooplankton community in Balsfjord, such as population dynamics and overwintering strategies in small copepod species (Norrbin et al., 1990; Barthel et al., 1995; Svensen et al., 2018; Barth-Jensen et al., 2020), or the role of zooplankton in the vertical carbon flux (Reigstad and Wassmann, 1996; Pasternak et al., 2000). Even less is known about the meroplankton community (Falk-Petersen, 1982), as the benthic community, and especially their larval stages, are generally much less studied (Oug, 1977; Michelsen et al., 2017).
One challenge of working with mesozooplankton is the complexity of accurate identification to species level. Zooplankton species identification is time consuming and requires specialist taxonomic expertise (Pan et al., 2008). In addition, the presence of cryptic species, and difficulties to morphologically identify even the most common copepod species complexes, such as Calanus or Pseudocalanus (Gabrielsen et al., 2012; Choquet, 2017; Choquet et al., 2017, 2018), severely limit our ability to document zooplankton biodiversity or identify species-specific life history strategies. For example, recent advances using molecular tools have revealed that the Calanus communities in Northern Norwegian fjords are not, as previously assumed, largely to exclusively dominated by C. finmarchicus (Choquet et al., 2017) but by a mix of C. finmarchicus and Calanus glacialis, demonstrating our lack of understanding of the Calanus species complex in this region. This also raises the question in how far previous studies on population structure and reproductive strategies of C. finmarchicus in Balsfjord (and elsewhere) (Tande and Hopkins, 1981; Tande and Slagstad, 1982; Tande, 1982; Tande and Gronvik, 1983) are biased by the undetected presence of other Calanus species in the fjord. Morphological species identification, of meroplankton in particular is almost impossible due to the small size and lack of species-specific morphological differences between many larval and juvenile stages. Meroplanktonic organisms are therefore often only identified to phylum and little is known about species-, or even family-, specific seasonal variability within the meroplankton community (Michelsen et al., 2017).
Over the last decades, advances in high-throughput DNA sequencing technology have improved our ability to identify the “hidden” diversity in plankton communities (Lindeque et al., 2013). Metabarcoding allows for a large-scale taxonomic identification of community samples by analysis of one or more barcode regions (Lindeque et al., 2013; Bucklin et al., 2016). Barcoded sequences are grouped in molecular operational taxonomic units (MOTU) and can be identified to the species level when compared to sequences stored in genetic libraries. Metabarcoding has the advantage of being faster than sorting samples under the microscope and is rapidly becoming more cost-effective compared to traditional morphological approaches. It can provide more detailed assessment of species diversity (Lindeque et al., 2013; Gran-Stadniczeñko et al., 2019), including groups that do not retain their morphological features in preservatives or lack them altogether, like most larval forms. Although the quantitative value of metabarcoding is still disputed (Bucklin et al., 2016), protocols are emerging that allow to use the numbers of sequence reads as a semi-quantitative proxy of organism’s biomass (Ershova et al., In revision). This semi-quantitative approach is particularly useful for seasonal studies, allowing to document the succession and seasonal changes in the contribution of different species of both holo- and meroplankton. However, metabarcoding cannot provide details on the developmental stage composition of a population, or the size composition within a zooplankton community. Thus, it appears essential to combine traditional microscopic analysis with metabarcoding to understand the variability in species diversity and zooplankton community structure in relation to seasonal changes in hydrography.
Here we used the combination of both approaches to (1) provide a complete species zooplankton inventory, (2) describe the seasonal variability in zooplankton community structure in relation to seasonal changes in hydrography and the availability of microalgae, and (3) described the population dynamics of sibling species of common copepods species that are difficult to identify based on morphology alone (i.e., Calanus spp. and Pseudocalanus spp.) in Ramfjord, a side arm of Balsfjord (Figure 1). A sill at 30 m at Balsfjord mouth separates the Balsfjord system from the open sea limiting the deep-water exchange and enabling us to observe seasonal patterns in the zooplankton community relatively undisturbed by exchange of water and organisms between the Balsfjord system and the open sea.
Materials and Methods
Study Area
The study was conducted in Ramfjord (Figure 1), northern Norway, a 13 km-long and 1 km-wide fjord, which consists of two basins. At their deepest, the innermost and the outermost basins are 50 and 130 m deep, respectively. The fjord can be partly ice-covered as the inner part of the fjord is heavily affected by freshwater inflow between October and April. The sampling station (125 m water depth; location 69°31′49.9N, 19°02′11.9E) was located close to the deepest point of the outer basin, which was ice-free for the entire sampling period.
Field Sampling
Hydrography
Monthly sampling was conducted between November 2018 and February 2020 (Table 1) on board of R/V Hyas. Vertical temperature and salinity profiles were measured during each sampling event with a conductivity-temperature-depth (CTD) profiler (CTD SBE 19plus). In addition, temperature, salinity and in situ chlorophyll fluorescence (relative values not comparable with fluorescence value from other studies) were measured continuously at hourly intervals from 18th March 2019 to 11th June 2020 from a moored underwater observatory (69°32.005′N, 19°02.904′E,115 m water depth) which included a CTD (Seabird Electronics SBE 16) at 18 m depth and 10 temperature loggers (SBE 65) at 10–15 m intervals between 17 and 107 m water depth along the mooring cable.
Chlorophyll a Concentration and Phytoplankton Community
Chlorophyll a (Chl a) concentration was measured at 13 sampling events (Table 1, note missing data in August, September 2019 and February 2020) from water samples taken with a 5 L Niskin bottle at 5 and 30 m. About 250 mL triplicate samples were filtered onto GF/F filters (Whatman plc, Maidstone, United Kingdom) in the dark and frozen at –20°C until processing. Chl a was extracted in 96% Ethanol for about 24 h at 4°C. The extracts were measured on a Turner Trilogy AU-10 fluorometer (Turner Designs, 2019) before and after acidification with 5% HCl. Chl a and phaeophytin concentrations were calculated based on calibrations done with a Chl a standard (Sigma S6144).
Nine phytoplankton samples (Table 1) were taken with a phytoplankton net (KC Denmark) with 10 μm mesh size from 35 to 0 m depth. The samples were fixed in 2% (final concentration) neutral Lugol and stored in a dark borosilicate glass bottle until counting. Phytoplankton taxa were identified in a 2 mL well plate using an inverted microscope (Zeiss Primovert, Carl Zeiss AG, Germany) and taxa were identified using Throndsen et al. (2007).
Zooplankton Sampling
A WP2 net (Hydro-bios, Kiel, Germany) with a closing mechanism and an opening of 0.25 m2 was used to sample zooplankton. Between November 2018 and March 2019, a net with a mesh size of 64 μm was used and from April 2019 on the mesh size was changed to 180 μm. Three net hauls were taken at each of the 15 sampling events (Table 1). One sample was taken from 115 to 50 m and one from 50 to 0 m. Both were preserved in 4% formaldehyde-in-seawater solution. These samples were used to analyze the community composition based on morphology. The third tow was taken from 115 to 0 m and immediately preserved in 96% ethanol. Every net haul was taken with a towing speed ranging between 0.4 and 0.5 m s–1.
Zooplankton Sample Analyses
Morphological Mesozooplankton Community Analysis
For each formalin-preserved sample, the community composition was determined under a Zeiss Discovery V20 stereo microscope (Zeiss, Oberkochen, Germany). First, large (>5 mm) and conspicuous organisms were picked out from the entire sample using fine forceps, identified and counted. Then, the remaining sample was diluted to a known volume and 5 ml subsamples were taken with an automatic pipette with the pipette tip cut at 5 mm diameter to allow free collection of mesozooplankton. The number of subsamples were determined to count at least 100 Calanus spp. copepodites which usually corresponded to more than 100 counted individuals of the most common genera in the same sample (Oithona similis, Microcalanus pusillus, Pseudocalanus spp., Acartia longiremis). Copepods were identified to the lowest taxonomic level possible based on morphological traits. The developmental stages were determined for Calanus spp. individuals. Non-copepods were identified to phylum. Abundance (individuals m–2) was estimated by dividing the number of species per sample with the mouth opening area assuming 100% net filtration efficiency.
For further analyses, the abundance from the two depth layers was combined to one depth integrated abundance (115–0 m).
The copepods were classified into two groups, according to their adult prosome length, with copepods with an adult size < 1.5 mm being classified as “small copepods” while the rest were classified as “large copepods” (Table 2).
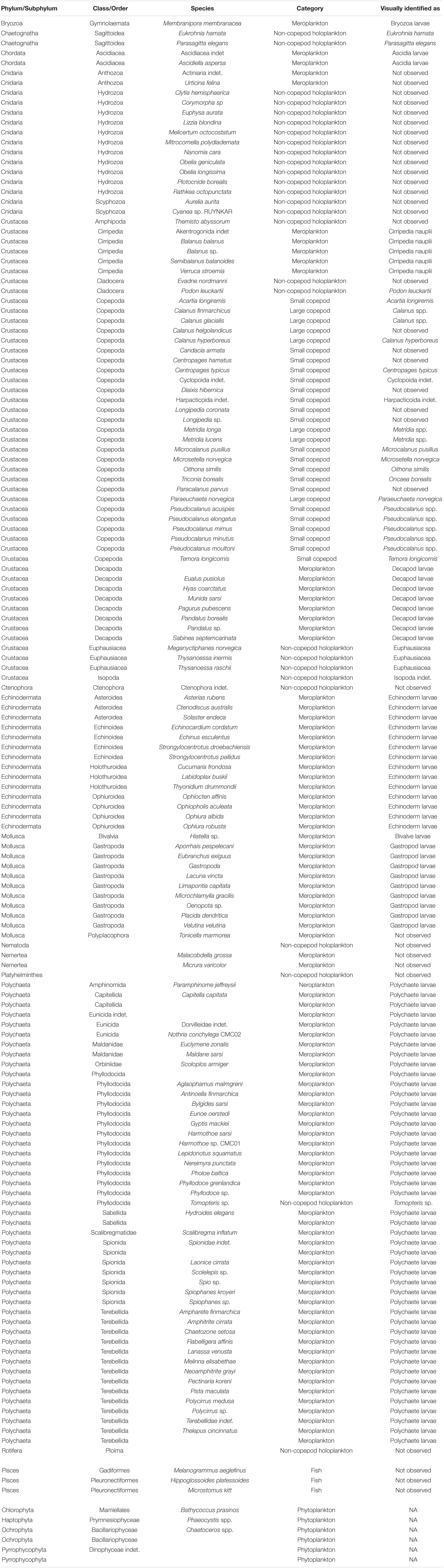
Table 2. List of all species identified using metabarcoding, how they were categorized, and if or to what level they were identified in the visual inspection.
Dry Weight and Biomass
After being analyzed, each sample was split in two parts using a Motoda box splitter. One half was archived. The other half was used to determine the biomass in terms of dry weight (DW) by removing excess water using a 180 μm sieve, washing the sample with fresh water, drying it for at least 24 h at 50°C and then weighing it with a microbalance (Sartorius BP 615; precision 0.1 mg).
Metabarcoding
The ethanol-preserved sample was split into two parts using Motoda box splitter. One split was homogenized for 30–60 s using a 1000 W blender and allowed to settle for 3–4 h. Excess ethanol was removed by centrifugation and three replicates (±0.3 g) of the homogenized sample were transferred to 2 ml microcentrifuge tubes. DNA was extracted from each replicate using the E.Z.N.A. Mollusc DNA Kit (Omega-Pro) (samples from November 2018 to September 2019) or the PowerSoil DNA Extraction Kit (Qiagen, October 2019–February 2020) (Table 1) following the manufacturer’s protocols. Leray-XT primers containing sample tags (Wangensteen et al., 2018), including the forward primer mlCOIintF-XT 5′-GGWACWRGWTGRACWITITAYCCYCC-3′ and reverse primer jgHCO2198 5′-TAIACYTCIGGRTGICCRAARAAYCA-3′ (Geller et al., 2013) were used to amplify a 313 base pair (b.p.) region of the mitochondrial cytochrome c oxidase (COI) gene. The PCR protocol was 10 min at 95°C, followed by 35 cycles of: 94°C for 1 min, 45°C for 1 min, and 72°C for 1 min, and a final extension of 5 min at 72°C. The tagged PCR products were pooled into a single library and cleaned using Minelute PCR purification columns1. The Illumina library was prepared from the DNA pool using the NextFlex PCR-free library preparation kit (Perkin-Elmer), quantified using the NEBNext Library Quant Kit for Illumina (New England BioLabs) and was sequenced on an Illumina MiSeq using a V3 2 × 250 bp kit.
Bioinformatics
Initial quality filtering of the sequencing data was conducted using OBITools v1.01.22 (Boyer et al., 2016). Illuminapairedend was used for aligning paired end sequences and filtering out those with an alignment score < 40. ngsfilter was used for demultiplexing and removal of primer sequences. Reads with a length of 299–300 b.p. were selected using obigrep and dereplicated using obiuniq. Chimeric sequences were then removed using the uchime_denovo algorithm (Edgar et al., 2011) in vsearch v1.10.1 (Rognes et al., 2016). Step-by-step clustering was performed in SWARM 2.1.13 (Mahé et al., 2015) using a distance value of d = 13 (Antich et al., 2021) to cluster individual sequences into Molecular Operational Taxonomic Units (MOTUs). After removing singletons (MOTUs with abundance of 1 read), taxonomic assignment of the representative sequence of remaining MOTUs was then performed using ecotag (Boyer et al., 2016) against DUFA-Leray v.2020-06-10, a custom reference database (publicly available from github.com/uit-metabarcoding/DUFA), which includes Leray fragment sequences extracted from BOLD and Genbank, completed with in-house generated sequences. Putative pseudogene sequences in the resulting dataset were then removed using LULU (Frøslev et al., 2017). MOTUs assigned to Prokaryotes and clearly non-planktonic organisms (e.g., insects, mammals) were removed, and a second taxonomy check of the remaining MOTUs was conducted using BOLD (Barcode of Life Database2). A species level identification was assigned with a minimum of 97% similarity. Finally, only MOTU’s observed in a minimum of two sample replicates and accounting for at least 0.01% of the total reads of any sample were kept in the final dataset.
Diversity Index
Specific richness was defined as the number of taxa identified by metabarcoding. The specific richness was calculated using the entire metabarcoding data set (total specific richness) as well as using only the data set excluding phytoplankton and fish species (zooplankton specific richness) and the data set including only the copepod species (copepod specific richness). The iNEXT R package (Hsieh et al., 2016) was used to ensure that the richness saturation plateau was reached for all samples (Supplementary Figure 3).
Data Analysis
We used the metabarcoding data as a semi-quantitative estimate of relative biomass of zooplankton taxa (Ershova et al., In revision) by multiplying the total zooplankton biomass with the proportion of the sequence reads for each species for each corresponding month to calculate biomass-weighted sequence reads (BWSR, mg DW m–2).
Multivariate analyses of the community composition were performed on morphological identification data (abundance). An estimation of the abundance of C. finmarchicus, C. glacialis, C. helgolandicus, P. acuspes, P. elongatus, P. minutus, and P. moultoni was calculated based on the relative composition obtained with metabarcoding, by multiplying the genus abundance by the proportion of the targeted species, and add to the data set used for the multivariate analysis. As metabarcoding data were missing in mid-April, the abundances estimation at this sampling point was estimated as the average of estimated abundance over the entire period. Abundance data were fourth-root transformed in order to reduce the impact of super abundant and rare species. Copepod nauplii, Microsetella norvegica and rare copepods species were excluded from the analyses since abundance estimates of these taxa were likely biased due to the change of the net mesh size over the study period. Chi-squared distances were calculated and used to perform hierarchical cluster analysis.
To elucidate the relationships between zooplankton community structure across seasons and environmental parameters, a Canonical Correspondence Analysis (CCA) was performed using the previously described data set. Explanatory variables included average water column temperature salinity and algal fluorescence obtained from the CTD profile as well as the Chl a concentrations from water samples. The significance of the overall model and individual terms was calculated using permutation tests [ANOVA function in the R package vegan; Oksanen et al., 2020)] at a significance level of p < 0.05 and only significant constraining factors were retained. Missing CTD data in mid-April were assumed to be similar to the ones obtained 14 days before in early April. Missing measurements of Chl a concentrations in August, early September and February 2020 were replaced by the average Chl a concentration over the study period. All analyses were preformed using R (version 4.0.1) (R Core Team, 2020) and the package vegan (Oksanen et al., 2020).
Results
Hydrography
The water column was cold, and well mixed from January to mid-May. The lowest temperatures were observed between March and May (0–2°C) (Figure 2A). The surface started to warm in mid-May, and from mid-May to August, the water column was stratified with warm water (5–10°C) in the uppermost 40 m and colder water (around 4°C) below 70 m. By September, the entire water column had warmed to >6°C and highest surface temperatures (12°C) were observed in early September. The water column started to cool down in November, and for the rest of the year the water was well mixed. From mid-December the water temperature was between 2 and 4°C (Figure 2A). Salinity varied between 32 and 33.5 throughout the year in most samples (Figure 2B). A relative fresh surface layer (salinity < 25) was observed starting in mid-May, coinciding with the onset of snowmelt on land resulting in increased freshwater runoff.
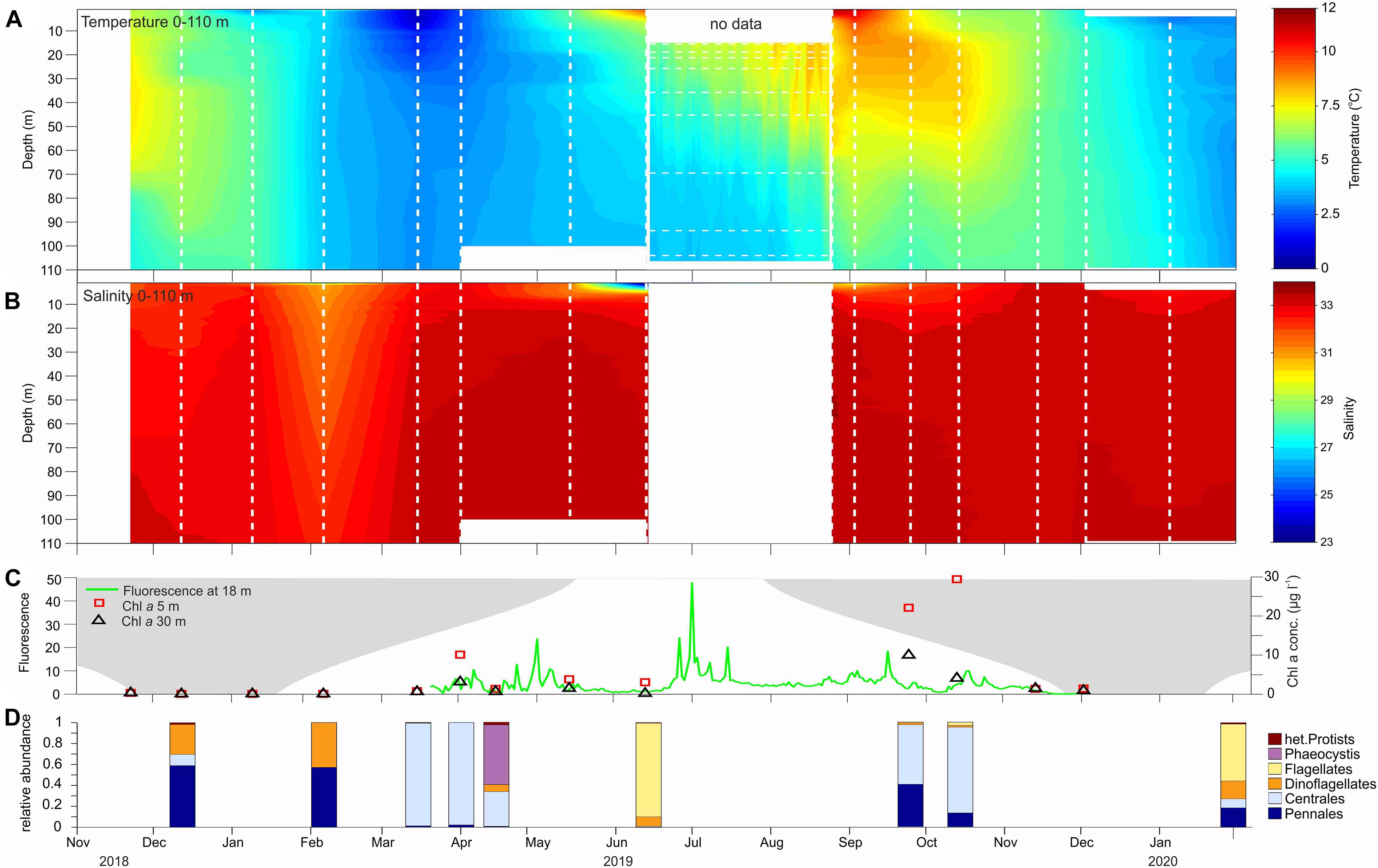
Figure 2. Seasonal variation (November 2018–January 2020) in temperature (A) and salinity (B) based on CTD profiles taken at each sampling event (dates indicated by white vertical lines), fluorescence at 18 m and Chl a concentration at 5 and 30 m (C), and taxonomic composition of phytoplankton community (D). No CTD data available in (A,B) from 15 June to 18 August. Gap in (A) is filled with temperature measurements from mooring located close to the sampling site (with horizontal lines indicating depth of sensors along the mooring line). Fluorescence data available starting 15 March 2019; no fluorescence data after mid November 2019 due to biofouling of sensor. Seasonal changes in light climate is indicated in (C) with dark shaded areas corresponding to number of hours of darkness each day.
Chlorophyll and Phytoplankton Community
In situ concentrations of Chl a were low from November to March (Figure 2C). The abundance of microalgae was low, and the community consisted mainly of pennate diatoms and dinoflagellates during the polar night and in February 2019, while flagellates dominated in February 2020 (Figure 2D). Chl a concentration and fluorescence increased strongly in early April (10.2 mg m–3 at 5 m) and the algae community was dominated by centric diatoms (mainly Chaetoceros socialis; Figure 2D). By mid-April, the prymnesiophyte Phaeocystis pouchetii dominated, but Chl a concentration and fluorescence were low. Fluorescence at 18 m depth peaked in early May (∼20 mg m–3), coinciding with the onset of stratification, and in July (∼50 mg m–3). However, no Chl a and phytoplankton data are available in those periods. In June, flagellates, mainly Chrysochromulina sp. dominated the phytoplankton community, and the fluorescence and Chl a concentrations (3 mg m–3) were relatively low (Figures 2C,D). Very high Chl a concentrations at the end of September (22 mg m–3) and in October (29 mg m–3) at both depth, indicate the occurrence of an intense autumn phytoplankton bloom dominated by centric diatoms (Chaetoceros sp.). Interestingly, this signal was not caught by the fluorescence sensors on the mooring in 18 m depth. From late November 2019 the fluorescence sensor did not provide reliable readings due to biofouling and in situ Chl a concentrations were low.
Seasonal Variability in the Zooplankton Community
Mesozooplankton Diversity
Thirty-five unique taxa were identified morphologically over the study period. Of these, 25 belonged to holoplankton, including 13 species of copepods: nine species or genera of small copepods and four species or genera of large copepods (Table 2). Ten taxonomic groups (class or phylum) of meroplankton were identified. The highest specific richness was observed in late April, and the lowest in February (2020). Only five copepod taxa were present throughout the entire study (i.e., Calanus spp., Pseudocalanus spp., Microcalanus pusillus, Oithona similis, Acartia longiremis), while all other taxa were absent during some months.
Metabarcoding revealed a total of 490 MOTUs, which corresponded to 154 unique taxa (Table 2). Hundred and fourteen of these were identified to species level, 17 to genus, and 22 to family or broader. Of the 154 unique identified taxa, 121 were present in more than four samples (Supplementary Table 1). Twenty-six species were present during every month, but only two of them (Pseudocalanus acuspes and M. pusillus) represented more than 1% of the sequence reads every month. Seven species represented at least 1% of the sequence reads during most of the months. These included A. longiremis, Calanus finmarchicus, C. glacialis, O. similis, Pseudocalanus moultoni, Nanomia cara, and Parasagitta elegans.
Of the 154 identified taxa, 36% (55) belonged to the holoplankton, while 58% (90) are known as benthic species and were therefore categorized as meroplankton. The remaining nine belong to fish and phytoplankton groups. Polychaeta presented the highest species richness with 46 taxa identified. Copepods were second in terms of number of taxa with 27 taxa identified over the study period (Table 2). Cnidaria and echinodermata were well represented as well, with respectively 15 and 14 taxa identified (Table 2). However, half of the identified cnidarians were present during only 1–4 months. Other groups such as bivalvia, amphipoda, ctenophora or chitonida were only detected as single taxa, generally identified at taxonomic levels above species (Table 2). Metabarcoding indicated that species richness was highest between December 2018 and February 2019 with a maximum number of species (110) in February (Table 3). It is noteworthy that during this period, sampling was conducted using a smaller mesh size. Between April and late September, the species richness varied between 80 and 104. Species richness was lowest between October 2019 and February 2020, when a different DNA extraction kit was used (Table 1 and Supplementary Figure 2B), with around 50 taxa identified, while between 80 and 110 taxa were identified in the other months (Table 3). The use of a different DNA extraction kit reduced the diversity that we were able to identified (Supplementary Figure 2), However, it did not impacted the diversity of Copepoda taxa that we were able to detect (Supplementary Figure 2B). A maximum Copepoda species richness of 26–28 was reached between November 2018 and February 2019 (Table 3) when we used the smallest net mesh size (Table 1). However, it reached the same number in September (Table 3) when the largest net mesh size was used (Table 1).
Mesozooplankton Biomass, Abundance, and Community Structure
Total mesozooplankton abundance and biomass in Ramfjord varied between 1.2 × 104 to 23 × 104 ind m–2 and 174 to 2609 mg DW m–2, respectively. Lowest abundance and biomass were measured between December and March during both winter seasons, while the highest values were recorded in summer/early autumn between August and October (Figures 3A,B). The mesozooplankton community was dominated in terms of abundance and biomass by small copepods (adult size < 1.5 mm) all year-round (Figures 3C,D). Small copepods represented up to 97% of the community in terms of abundance and up to 94% in terms of sequence read (Figures 3C,D). Non-copepod holoplankton (Figure 3 and Table 2), consisting mainly of chaetognaths and krill, dominated in terms of sequence reads in November and December 2018, although their abundance was negligible (<1%). In late April, a high number of krill eggs was observed (64920 ind m–2). The estimated BWSR of non-copepods varied between 0.44 mg DW m–2 in November 2019 and 690.96 mg DW m–2 in late September, which accounts for <0.1% to 55% of the total biomass (Figure 3D).
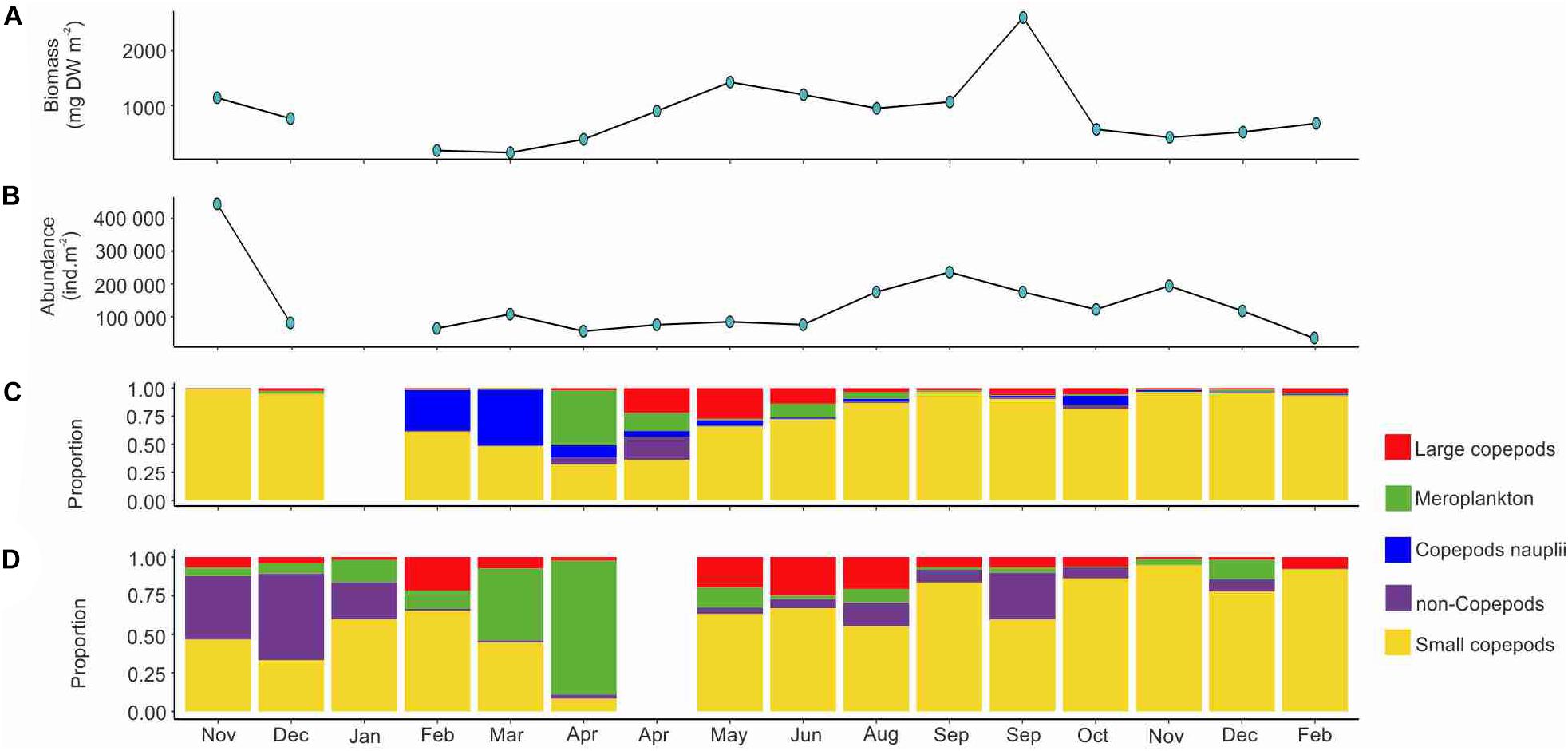
Figure 3. Seasonal changes in the total zooplankton biomass (A), total zooplankton abundance (B), proportions of main taxonomic groups based on morphological analysis (C), and metabarcoding (D) in Ramfjord during November 2018–February 2020.
Large copepods (>2 mm at adult stage) were present year around, with highest abundance observed between April and June (Figure 3C). In terms of abundance, they represented a maximum of 27% of the zooplankton community in May, but only 2% in early September (Figure 3C). High abundances of copepod nauplii were recorded in February and March, accounting for up to 49% of the total abundance (Figure 3C). The highest BWSR of large copepods was observed in June (253 mg DW m–2), and their contribution to the sequence reads varied between 1 and 25%, in November 2019 and June, respectively (Figure 3D).
Small copepods
The lowest proportion of small copepods, in terms of abundance, was observed in late April when only 20% of the community consisted of small copepods, while they represented between 50 and 90% of the community during the rest of the year (Figure 3C). The BWSR of small copepods varied between 0.35 mg DW m–2 in early April and 1359 mg DW m–2 in late September. The small copepods community had a relatively high diversity with nine species identified morphologically and 24 species detected using metabarcoding (Table 2). Acartia longiremis, M. pusillus, O. similis and four species of Pseudocalanus (P. acuspes, P. elongatus, P. minutus, P. moultoni) were present in Ramfjord year-round (Supplementary Table 1). These species represented more than 1% of all the sequence reads, together with Paracalanus parvus and Temora longicornis. We combined these species as the main representatives of the small copepod community (Figure 4). Paracalanus parvus was not observed visually, likely due to its morphological similarity to Pseudocalanus spp. at juvenile stages (Table 2 and Figure 4).
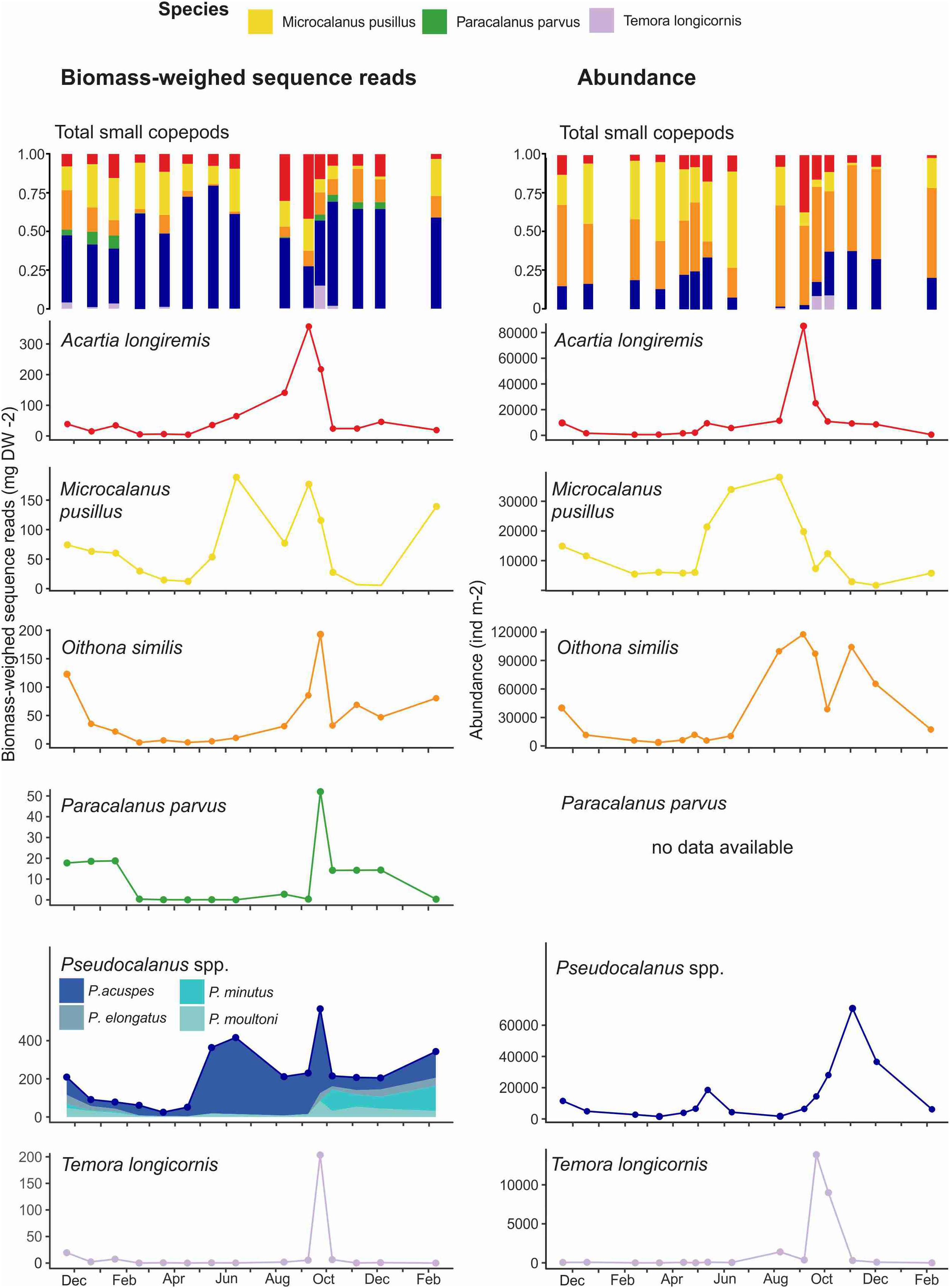
Figure 4. Seasonal changes in small copepod BWSR (mg DW m− 2, left column) and abundance (individuals m− 2, right column) in Ramfjord between November 2018 and February 2020. The first row represents the relative proportion of the main small copepod species to the small copepod community remaining rows represent the seasonal variation of the main small copepod species.
The abundance of small copepods was relatively low between November and May (<3.104 ind m2) (Figure 4). Only Microsetella norvegica had a peak in abundance during this time, dominating the community in terms of abundance in February and March 2019 with up to 39 × 103 ind m2. However, the number of sequence reads of M. norvegica was negligible (less than 1% of the total sequence reads) during the entire study period.
From May, the abundance of small copepods increased and reached its maximum in September (23 × 104 ind m–2). O. similis dominated the small copepod community throughout most of the study period in terms of abundance, contributing up to 65% and with a maximum abundance of 11 × 104 ind m–2 in September (Figure 4). In terms of sequence reads, O. similis represented a low portion of the small copepod community, with a maximum of 20% in November 2019 (Figure 4), and its BWSR varied from 1 mg DW m–2 to 192 mg DW m–2.
Between November 2018 and January 2019, the small copepod community as estimated by BWSR was represented by all the main species depicted in Figure 4. The contribution of O. similis and M. pusillus BWSR was slightly higher, contributing 24 and 27%, respectively, while the other species contributed 1–15% to the small copepod BWSR (Figure 4). Between February and August P. acuspes dominated the community in terms of BWSR (up to 73%), which varied between 33 mg DW m–2 and 441 mg DW m–2 (Figure 4). The rest of the BWSR was composed of A. longiremis (between 5 and 41%) and M. pusillus (between 1.7 and 28%) (Figure 4). From late September to the end of the study, copepods of the genus Pseudocalanus dominated the small copepod community in terms of the BWSR (Figure 4). P. minutus had the highest proportion of sequence reads, up to 35% in October, with a BWSR varying from 1.94 mg DW m–2 to 113 mg DW m–2. Pseudocalanus elongatus contributed least to the Pseudocalanus biomass, representing only between 2 and 12% of the small copepod BWSR between late September and December 2019 (Figure 4).
Meroplankton community
Eight groups of meroplankton, the six presented in Figure 3 plus Bryozoa and Decapoda, were identified during the entire study period.
Meroplankton accounted for only 0.5–12% of the total zooplankton abundance between November 2018 and August 2019, and the abundance was negligible during the study period except in April when Chl a concentrations were high (Figure 3C). However, meroplankton BWSR varied between 1.81 mg DW m–2 in late September and 312 mg DW m–2 in March, which represented respectively 3.1 and 46% of the BWSR. The highest proportion of meroplankton, in terms of BWSR, was observed in early April when it accounted for 86% of the total BWSR (Figure 3C).
The composition of the meroplankton community estimated by metabarcoding followed the overall trends provided by morphological analysis, but with a higher taxonomic resolution. Polychaeta larvae and juveniles were present year-round in Ramfjord (Figure 5) and made up a high proportion of the meroplankton community in terms of abundance, contributing between 9% in October and 82% in December 2019 (Figure 5). The highest abundance of polychaeta larvae was observed in mid-April (4360 ind m–2) (Figure 5). They dominated the meroplankton community in terms of BWSR between November 2018 and March 2019 and between October and December 2019 when they accounted for 14–80% of the meroplankton BWSR (Figure 5). Cirripedia larvae dominated the meroplankton community in terms of abundance and BWSR in April with a peak in abundance (19 × 103 ind m–2) in mid-April (Figure 5). In June, echinoderm larvae accounted for 89% of the meroplankton abundance and 70% of the meroplankton BWSR (Figure 5). Their maximum abundance, 8.2 × 103 ind m–2, was also observed in June (Figure 5).
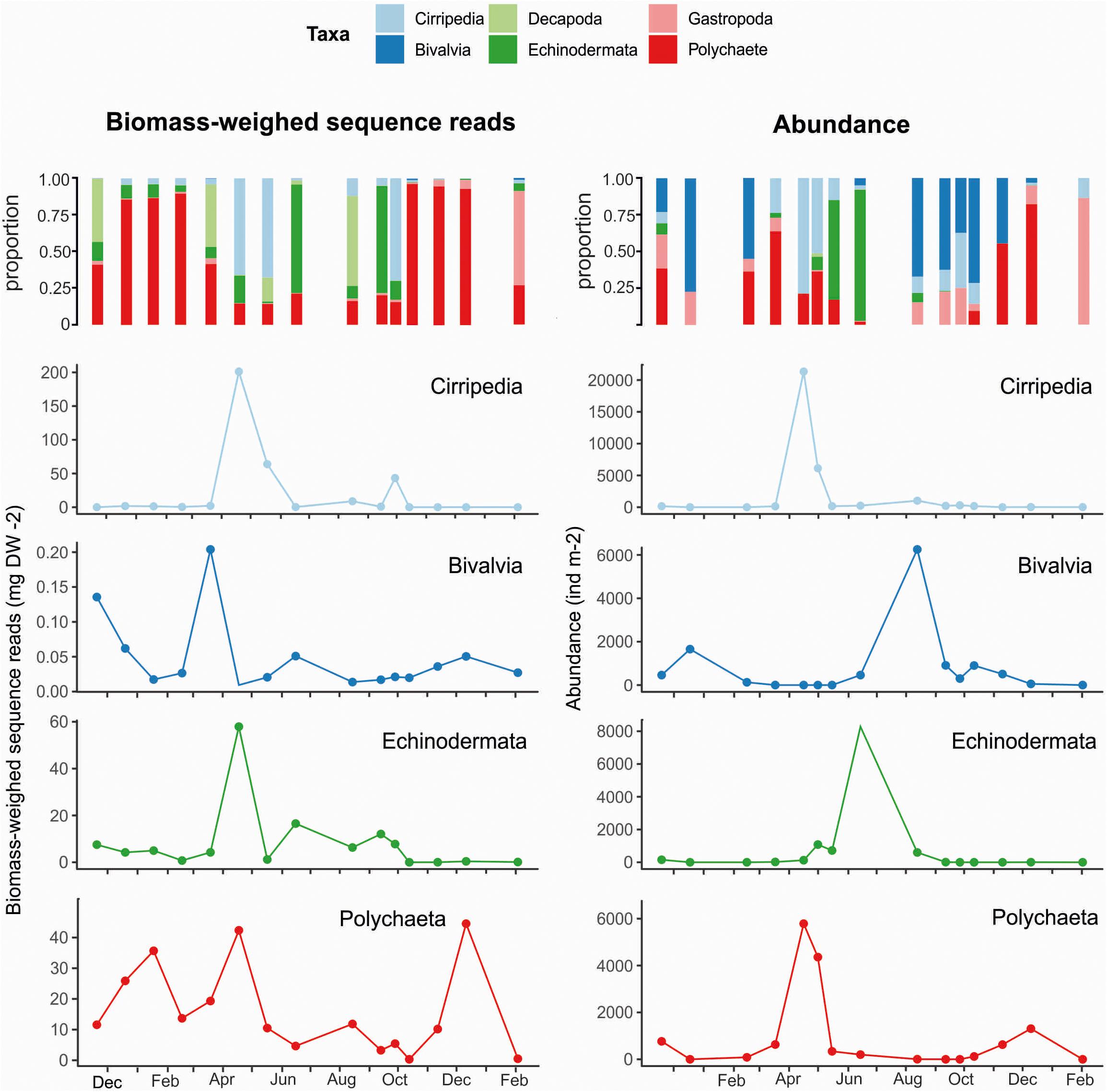
Figure 5. Seasonal changes in the meroplankton BWSR (mg DW m− 2, left column) and abundance (individuals m− 2, right column) in Ramfjord between November 2018 and February 2020. The first row represents the relative proportions of the main meroplankton groups to the meroplankton community; the remaining rows represent the seasonal variation of the main meroplankton groups.
Juveniles bivalves dominated the meroplankton community between November 2018 and February 2019 (23–68% of meroplankton) (Figure 5), and between August and November 2019 (up to 70%; Figure 5), with a peak in abundance in August (62 × 102 ind m–2). However, their BWSR was low or negligible over the entire study period (Figure 5). Likewise, the BWSR of gastropod larvae was low, never contributing more than 7% to the meroplankton community (Figure 5). However, in terms of abundance they represented up to 43% of the community in February 2019 (Figure 5).
Calanus spp. species and stage composition
Based on morphological characteristics, only Calanus hyperboreus copepodite stage III (CIII)-adults could be identified with confidence and their abundance was overall very low (maximum 80 ind. m–2 in May). We did not identify other Calanus individuals to species morphologically and defined them as Calanus spp. Abundance of Calanus spp. was relatively low between November and early April (around 1000 ind. m–2) and reached a maximum of 20 820 ind. m–2 in May (Figure 6A). In March and April, adult stages dominated the Calanus spp. population, with females contributing 77% in March and 66% in early April (Figure 6B). In mid- April, males were the dominating stage, representing 50% of the population (Figure 6B). Young copepodite stages (CI, CII, and CIII) started to appear in March, and were dominating the population in May (Figure 6B). Older copepodite stages (CIV and CV) were present in later winter (February–March), reappeared in higher proportions in May and were dominating the population from June on for the rest of the year (Figure 6B). CVs contributed up to 93% of the population between June and October (Figure 6B). A second peak in Calanus spp. abundance (10428 ind. m–2) was observed in late September (Figure 6A). In October and November, CIIIs were detected again, representing 20 and 40% of the population, respectively (Figure 6B).
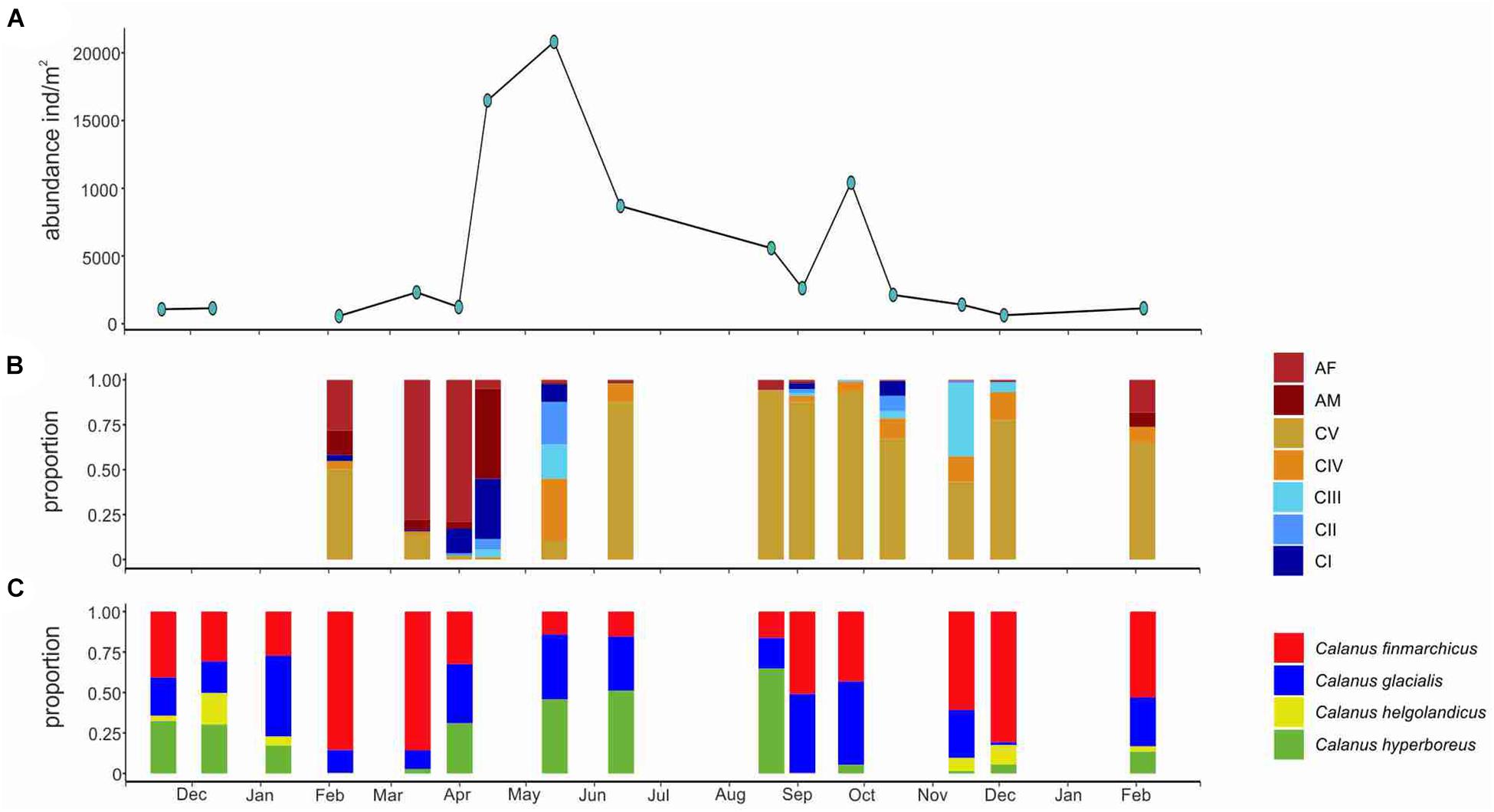
Figure 6. Seasonal changes in Calanus spp. Abundance (A), stage composition (B), and species composition (based on metabarcoding) (C) in Ramfjord November 2018–February 2020.
Metabarcoding detected the presence of four species of the genus Calanus: C. hyperboreus, C. finmarchicus, C. glacialis, and C. helgolandicus (Figure 6C). C. finmarchicus and C. glacialis dominated the Calanus community in Ramfjord for most part of the year, contributing equally in September, while C. finmarchicus dominated in February and March, and from October to December, and C. glacialis in January, and from April to June. In contrast to the low abundance observed, C. hyperboreus BWSR was relatively high between November 2018 and January 2019 and from April to August, when they represented between 0.3 and 64% of the Calanus sequence reads (Figure 6C). The contribution of C. helgolandicus to the Calanus community was low year-round (from 0 to 19%), with higher proportion observed during autumn-winter months (between 0.1 and 19%) (Figure 6C).
Seasonality of zooplankton community in Ramfjord
Hierarchical cluster analysis of abundance data of the entire zooplankton community, identified four main assemblages: spring bloom (SB), spring/summer (SS), Autumn/winter (AW), and pre-spring bloom (PS) (Supplementary Figure 1).
The CCA models was significant (p < 0.05) with temperature and fluorescence as significant constraining factors (p < 0.05), while salinity and Chl a were insignificant. The resulting CCA model for abundance explained 29% of the total inertia (variance) in the abundance data, with 21% accounted for by the first axis (Figure 7). The results of the CCA were consistent with the cluster analysis, showing a clear separation of the samples based on season. The two April samples formed their own group (SB), being distinguished by the presence of cirripedia nauplii, euphausiid larvae and decapod larvae (Figure 7), and a high abundance of krill eggs (1333 ind m–2) The SB and PSB assemblages were most distinct on the ordination, while SS and AW grouped closer together (Figure 7). The SS group was distinguished by relatively high fluorescence and water temperatures (Figure 7), and a higher abundance of Calanus spp. (Figure 7), particularly C. hyperboreus and C. glacialis (Figure 7), as well as echinoderm larvae and A. longiremis. The AW group was characterized by a wide range of water temperatures and low fluorescence. Furthermore, high abundance of small copepods (e.g., O. similis, Pseudocalanus spp., M. pusillus), Metridia longa, Paraeuchaeta norvegica, gastropoda larvae, chaetognatha and a relatively low abundance of Calanus spp. were characteristic for AW. The PS group, which included February and March samples, was characterized by low temperatures and fluorescence. Zooplankton abundance was overall low within this group, with a higher relative contribution of nudibranch larvae and Triconia sp. Although they were not included in the multivariate analyses, during this season we also registered a high abundance of copepod nauplii (23348 ind m–2 in February and 53908 ind m–2 in March).
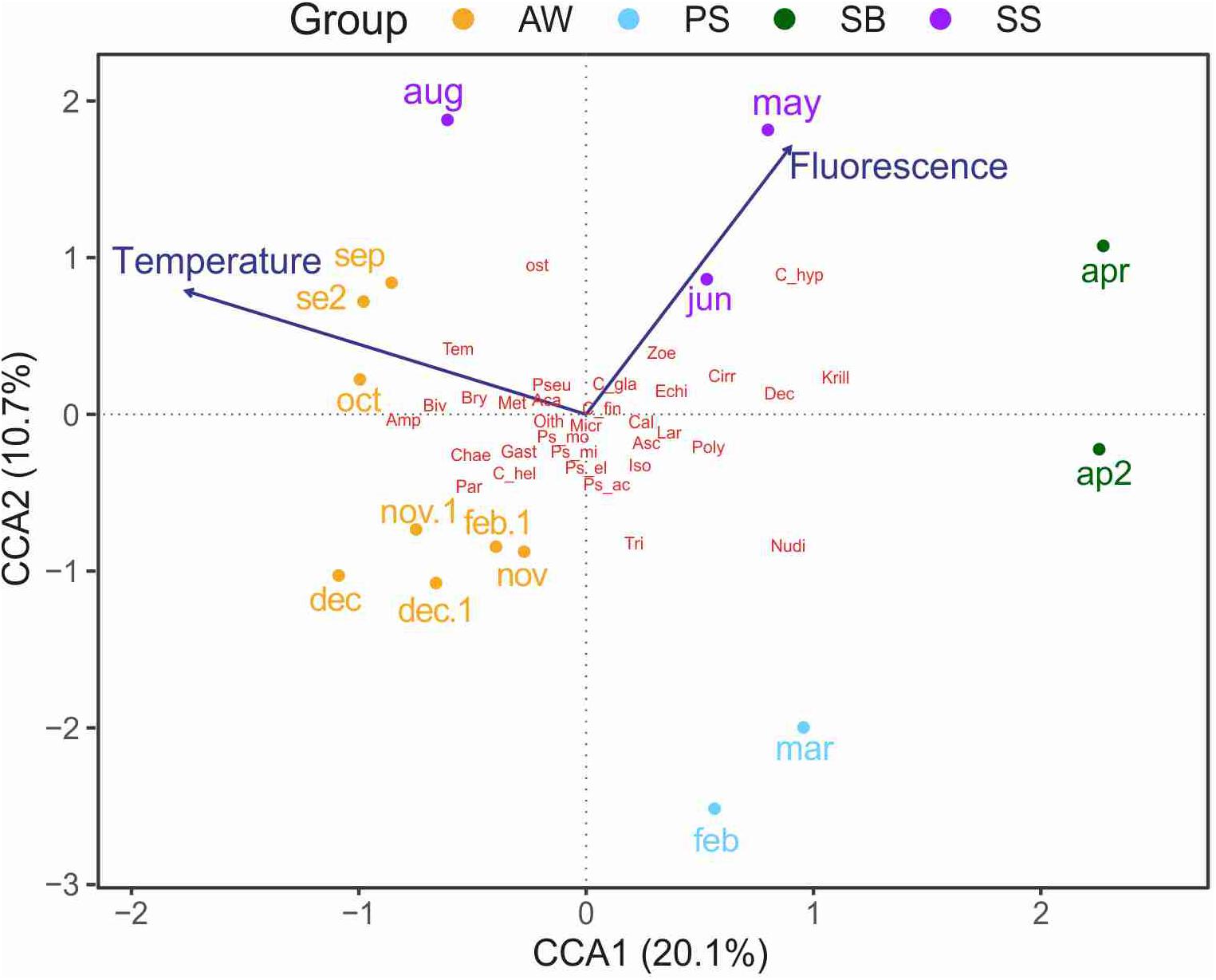
Figure 7. Canonical correspondence analysis (CCA) of fourth-root transformed abundance data. The eigenvalues as percentages are provided for dimensions I and II with a total of 30.8% and variance explained. Colors correspond to the assemblages obtained by the hierarchical cluster analysis (Supplementary Figure 1). The abbreviations used in this analysis are explained in Table 4.
Discussion
The present study is one of only a few studies combining metabarcoding and morphological identification to study the seasonal variability of a zooplankton community with relatively high temporal resolution (Gran-Stadniczeñko et al., 2019; Schroeder et al., 2020). This approach enabled us to describe the seasonal variability of the zooplankton community in Ramfjord with high quantitative and taxonomical accuracy, while at the same time also linking the detected changes to environmental variables.
The Zooplankton Community Diversity
Using metabarcoding, we identified 154 taxa in Ramfjord over the study period, which was four times as many taxa as using the morphological identification alone. The mesozooplankton diversity in this fjord system has previously been described as low (Hopkins, 1981) although the authors did not specify compared to what this assessment was made. Mesozooplankton diversity is rarely fully described, but compared to other well-studied high latitude fjords, such as Kongsfjorden (97 taxa over 20-year-long time series, Hop et al., 2019b), Rijpfjorden (42 taxa over 8 months) (Weydmann et al., 2013) and Dolgaya Bay (33 taxa in July, Dvoretsky and Dvoretsky, 2010), the zooplankton community in Ramfjord does not appear to be particularly low in diversity. However, the majority of taxa identified in our study can be categorized as meroplankton (i.e., larvae and juveniles of 90 benthic adult taxa), which are usually not identified to lower taxonomic level. Of the 55 holoplanktonic taxa, copepods accounted for 27, which is low compared to Kongsfjorden (52 species), but diverse compared to Rijpfjorden and Dolgoya Bay as well as Hudson Bay (Estrada et al., 2012) (13, 15, and 13 species, respectively). The lower number of copepod species from studies relying on visual identification are, however, only comparable to the number of copepod species we identified morphologically (13), strongly supporting the concept of combining metabarcoding with morphological identification.
Meroplankton diversity in Ramfjord exceeded estimates from other northern Norwegian locations (including Balsfjord) that were based on morphology. Here, meroplankton species richness varied between 37 (Porsangerfjord) and 65 taxa (Vesterålen) (Falk-Petersen, 1982; Andersen, 1984; Fetzer and Arntz, 2008; Silberberger et al., 2016; Michelsen et al., 2017). Meroplankton diversity was also higher than the diversity of benthic organisms previously assessed in Ramfjord (Oug, 1977), particularly with regard to polychaetes and echinoderms. However, the benthic communities of Ramfjord and Balsfjord are poorly studied (Oug and Høisœter, 2000), which makes it difficult to conclude whether the meroplankton species identified in Ramfjord were part of local populations or present due to advection. Among meroplankton, polychaetes were the most diverse group. This is not surprising, since polychaetes are the most diverse group of benthic organisms in other Arctic, sub-Arctic and Norwegian fjords (Holte, 1998; Ellingsen and Gray, 2002; Bluhm et al., 2011). Oug and Høisœter (2000) found that polychaetes represented 97% of all species of the soft-bottom macrofauna community in Balsfjord (of a total of 78 species). Little is known about the biodiversity of taxa usually associated with hard bottom communities in our study area. The presence of larval stages of cirripedia and ascidia has been reported previously in this fjord system (Falk-Petersen, 1982). Sandnes and Gulliksen (1980) identified the sea urchins Strongylocentrotus droebachiensis and S. pallidus as key species in the system, controlling the abundance of sessile organisms such as the barnacle Semibalanus balanoides and the limpet Testudinalia testudinalis. Larval stages of these species were present in our study (Table 2 and Supplementary Table 1) while the gastropods and bivalves mentioned by Sandnes and Gulliksen (1980) did not show up in our species inventory. Bivalvia were the only group whose diversity of larval stages was lower than the diversity of adult forms previously described in the study area (Oug, 1977; Sandnes and Gulliksen, 1980; Vahl, 1980; Drent, 2002) with only one MOTU of bivalve identified. For juvenile bivalves we also found the largest difference between the visual analysis and metabarcoding. While high abundance was observed in summer, bivalves hardly featured in the BWSR. This underestimation of bivalve diversity can be explained by a lack of relevant data in the reference database, PCR bias using our chosen primer set, or problems with DNA extraction. Molluscs are notoriously hard to extract DNA from Pereira et al. (2011) presumably due to the presence of polysaccharides that inhibit DNA polymerase, and although we used an extraction kit tailored for molluscs during most of our study period, this demonstrates the ongoing challenges for this taxonomic group.
Most of the holoplankton taxa identified using metabarcoding (Table 2 and Supplementary Table 1) are species common to boreal and Arctic zooplankton communities (e.g., Dvoretsky and Dvoretsky, 2010; Estrada et al., 2012; Weydmann et al., 2013; Hop et al., 2019b). Particularly for Calanus and Pseudocalanus, metabarcoding provided a more detailed insight into the species composition than previous studies. Since life cycles and life strategies may differ even in closely related species within the same genus (McLaren et al., 1989; Lischka and Hagen, 2005; Ershova et al., 2021), correct species identification is crucial for describing species specific life history strategies and for documenting changes in population dynamics (see below). Pseudocalanus lacks easily distinguishable morphological features that would aid species identification, particularly when it comes to the early life stages, and they are therefore often reported as Pseudocalanus spp. Only two species of Pseudocalanus, i.e., P. acuspes and P. minutus (Norrbin, 1993, 1994) and one species of Calanus, i.e., Calanus finmarchicus (Hopkins et al., 1989) have previously been reported from Ramfjord/Balsfjord, while we revealed the presence of four species of each genus coexisting in Ramfjord, (i.e., C. finmarchicus, C. glacialis, Calanus helgolandicus, Calanus hyperboreus, Pseudocalanus acuspes, Pseudocalanus elongatus, Pseudocalanus minutus, Pseudocalanus moultoni, Table 2 and Supplementary Table 1).
While morphological features such as size (e.g., Daase and Eiane, 2007) or coloration (Nielsen et al., 2014) may enable species identification of Calanus spp. with some degree of accuracy in the high Arctic, they are not reliable in populations along the Norwegian coast (Choquet et al., 2017). This can lead to an under representation of C. glacialis in particular and explains the lack of records of these species in Norwegian fjords (included Balsfjord) in most previous studies. The Arctic C. hyperboreus on the other hand, is easily distinguishable from the other Calanus species due to its large size and a clear morphological feature (a spine on the last prosome segment) in the older copepodite stages and in adults. Metabarcoding revealed a relatively high proportion of C. hyperboreus in Ramfjord, while only very few individuals were recorded by visual identification. This discrepancy between molecular and visuals tools could be explained by the fact that the number of sequence reads is a proxy of the biomass (Lindeque et al., 2013; Ershova et al., In revision). Because of its large size, C. hyperboreus (prosome length up to 5 mm) can represent a substantial part of the biomass, even if the abundance is low. However, we only identified very few C. hyperboreus individuals per sample visually (1 or 2 per sample) compared to Calanus in the size range of C. finmarchicus and C. glacialis (2–4 mm, 100- 5000 per sample), thus even in terms of BWSR C. hyperboreus should not contribute to the Calanus weighted sequence reads in such high proportion. If C. hyperboreus was present in high numbers as young copepodite stages (CI -II) they could have been misidentified, as size differences of young stages are less pronounced between the different Calanus species. However, the sequence reads indicate highest relative contribution of C. hyperboreus in June and August when the Calanus population was dominated by late copepodite stages and young stages were rare. Thus, further studies are needed, e.g., using mock samples, to investigate the relationship between C. hyperboreus biomass, abundance and relative sequence reads.
Seasonality in the Zooplankton Community in Ramfjord
Long term data on hydrographic conditions are not available for the Ramfjord/Balsfjord system. However, the hydrographic conditions we observed in Ramfjord during our study were similar to observations from Balsfjord in 1976–1977 (Eilertsen et al., 1981a) and 2013–2014 (Svensen et al., 2018), with temperature peaking between 8 and 12°C in the surface during summer, and otherwise varying between 2 and 4°C at depth during summer and throughout the water column during winter and spring. We also observed salinity in a similar range to observations from Balsfjord (32 -33), with lower salinity in surface waters during snow melt and heavy snow fall (Eilertsen et al., 1981a; Svensen et al., 2018). We concluded that no strong differences in hydrographic settings was evident between the current and historical data.
A tight coupling of seasonal changes in zooplankton community structure and the strong seasonality in phytoplankton biomass and production, driven by the light regime, is typical for polar and sub-polar areas (e.g., Pertsova and Kosobokova, 2003; Weydmann et al., 2013; McKinstry and Campbell, 2018). In Ramfjord, the seasonal variability in the zooplankton community structure was manifested in four distinct periods (Figure 8), characterized by differences in overall abundance, presence of meroplankton, and shifts in diversity.
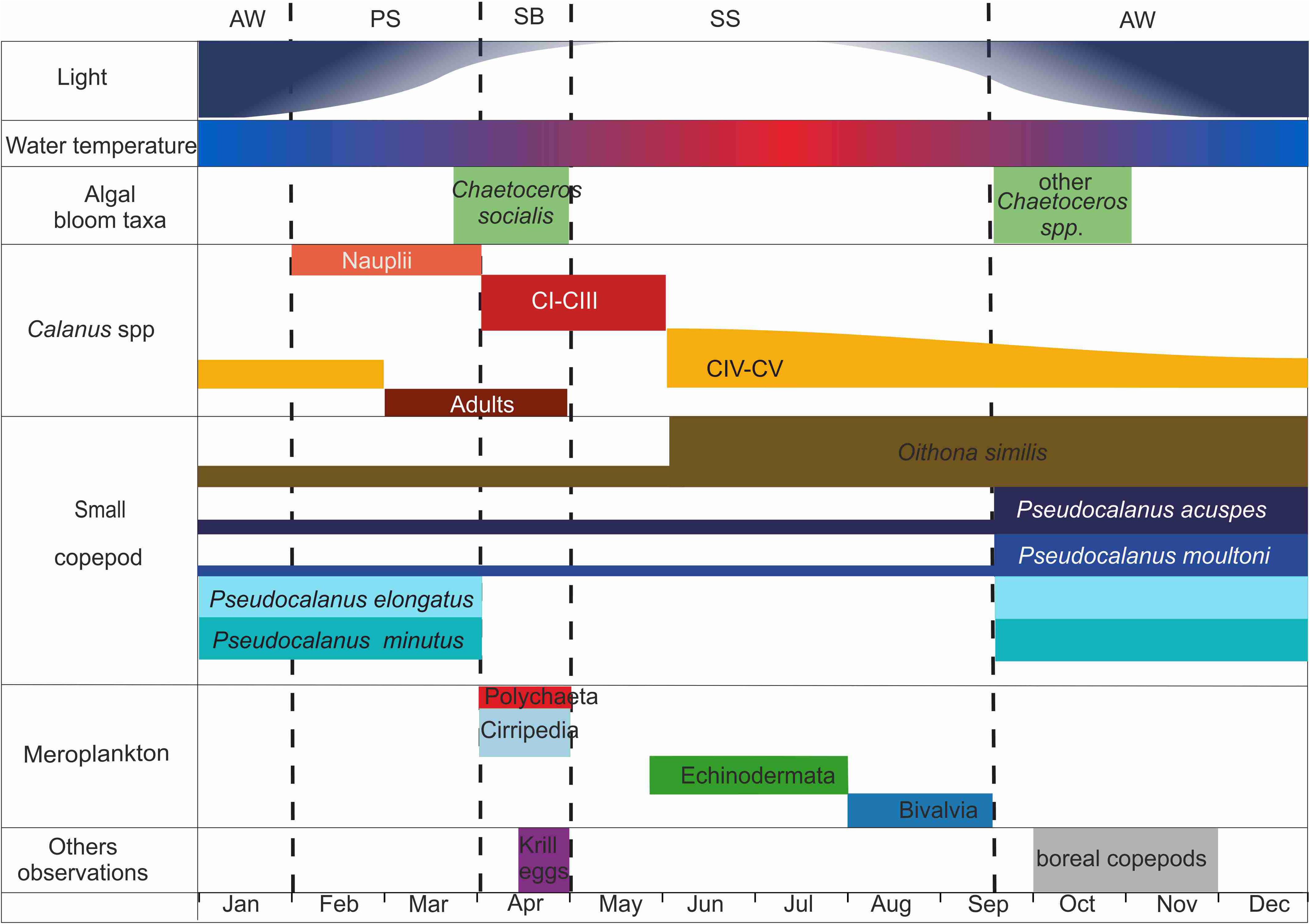
Figure 8. Conceptual understanding of the seasonality variability in the zooplankton community in Ramfjord. The size of the boxes is proportional to the abundance of the organisms, but size of boxes is not comparable between the different compartments.
The pre-spring bloom (PS) period (February–March) was characterized by increasing day length but low water temperatures and an overall low phytoplankton and zooplankton abundance. However, the high abundance of copepod nauplii indicates that reproduction had started and given the low Chl a concentration, most of this effort was likely fueled by internal energy reserves, indicating a dominance of capital breeders among the reproducing copepods (Varpe, 2009) (see below).
April was initiated with a peak in Chl a concentration, marking the start of the spring bloom (SB). A succession from a dominance of diatoms such as Chaetoceros socialis and Thalassiosira spp. to a dominance of Phaeocystis pouchetii has previously been observed in Ramfjord and other areas including, e.g., the Barents Sea and the marginal ice zone in the Greenland sea (Eilertsen et al., 1981b; Tande and Bamstedt, 1987; Gradinger and Baumann, 1991; Orkney et al., 2020). We did not observe P. pouchetii beyond late April and thus lack data to assess how long it dominated the spring bloom in Ramfjord and its importance during the rest of the year as we may have underestimated the presence of the single cell stage of P. pouchetii for most parts of the year using a 10 μm phytoplankton net, which may only collect larger colonies that form during periods of high abundance.
The spring bloom fueled reproduction and development in the zooplankton and benthos community, as indicated by an increasing dominance of nauplii and juvenile stages. Adults and early developmental stage of Calanus spp. were abundant as well, as were krill nauplii and eggs, and within the meroplankton community, cirripedia nauplii and polychaete larvae reached their peak abundance. The SS period, starting in May, was characterized by low Chl a concentration and fluorescence, indicating a post bloom situation, dominated by flagellates as previously observed by Gaarder (1938) and Eilertsen et al. (1981b). We unfortunately lack data on Chl a and microalgae taxonomy from July to September to properly describe seasonal changes in the phytoplankton community throughout summer. The fluorescence measurements at 18 m depth indicate the presence of microalgae throughout summer with a potential bloom event in July. Thus, algal food was available throughout summer, and a succession of different meroplankton taxa characterized the SS period. Cirripedia nauplii and polychaete larvae had disappeared from the water column by June, while the abundance of echinoderm larvae increased in June followed by an increase of juvenile bivalves in August. A similar succession in meroplankton has previously been described in Ramfjord (Falk-Petersen, 1982) and corresponds to observations from Arctic fjords in Svalbard (Kuklinski et al., 2013; Kwasniewski et al., 2013; Stübner et al., 2016) and Greenland (Nielsen et al., 2007).
Algal bloom dynamics in boreal and sub-Arctic regions typically include at least two seasonal peaks, one in spring and one in autumn (Eilertsen et al., 1981b; Eilertsen and Frantzen, 2007), and the AW period (AW, mid-September–January) in Ramfjord commenced with an autumn bloom dominated by diatoms (a mix of Chaetoceros species) similar to observations by Eilertsen et al. (1981b). Particularly high Chl a concentration measured in October far exceeded those during the spring bloom. This autumn bloom coincided with highest copepod diversity and peak in zooplankton abundance but also a steep decline in zooplankton biomass from September to October, largely driven by the decrease in abundance of the large Calanus species. Small copepods thus became the main contributors to the zooplankton community not only in terms of abundance but also in terms of BWSR, and biomass remained relatively constant throughout the AW period, even when temperature and light decreased, and Chl a concentration remained low. Hansen et al. (1999) hypothesized that the descent to overwintering depth of the large Calanus species creates a free niche in upper water layers that benefits small copepods, such as Oithona similis, Microcalanus pusillus, Acartia longiremis, and Pseudocalanus spp. These predominately omnivorous species (Saiz and Kiørboe, 1995; Castellani et al., 2005; Cleary et al., 2016) thus become the dominating functional group in the zooplankton community during AW.
The occurrence of species regarded as Atlantic/boreal, such as the copepods C. helgolandicus, P. elongatus, Temora longicornis, P. minutus, and P. elongatus and other species such as Themisto abyssorum and Evadne normanni, was restricted to AW when strong south-west winds often prevail in the Tromsø area (Eilertsen et al., 1981a), forcing water of Atlantic origin into the fjord system. Those species were likely advected into the fjord system and do not have established populations in the fjord.
Life History Strategies of Common Copepods
Except for Calanus spp., we did not identify copepodite stages of most common copepod species, thus we lack data to discuss the population dynamics of these species. However, changes in the total and relative abundance throughout the seasons nevertheless allow us to draw some conclusions about their life history strategies in Ramfjord. As observed in Disko Bay, Greenland (Madsen et al., 2008), the small copepods peak in Autumn in Ramfjord. This abundance peak of small copepod was prior or simultaneously to the second phytoplankton bloom, while it occurred after the algal bloom in Disko Bay (Madsen et al., 2008). The ubiquitous O. similis is known to actively feed and reproduce all year-round (Svensen et al., 2011; Zamora-Terol et al., 2014). Similar to observations from Kongsfjorden (Lischka and Hagen, 2005; Hop et al., 2019b), Greenland (Digby, 1954; Madsen et al., 2008), Malangen (Falkenhaug et al., 1997), and Kola Bay (Dvoretsky and Dvoretsky, 2009), we observed the highest abundance between August and December with peaks in September and November, thus coinciding with periods of relative warm water temperature that may affect recruitment and development rates positively (Svensen et al., 2011; Zamora-Terol et al., 2014). The life cycles and life history strategies of A. longiremis, T. longicornis, and M. pusillus are overall not well studied. A. longiremis is a neritic species commonly observed in Arctic and sub-arctic zooplankton communities (e.g., Hopcroft et al., 2010; Estrada et al., 2012; Ershova and Kosobokova, 2019; Hop et al., 2019a). In Ramfjord, A. longiremis was present year-round, indicating that it has established a population here, although its abundance sharply peaked during October. Falkenhaug et al. (1997) also observed a peak of Acartia spp. at the same period in Malangen, however the authors did not discuss this increase of abundance. We suggest a possible advective source as well. T. longicornis, a temperate, brackish water weakly selective herbivorous species whose reproduction is coupled to the phytoplankton spring bloom (Peters et al., 2013), appeared only during the autumn bloom, suggesting that the population was not established in Ramfjord but was the result of advection. M. pusillus represented a substantial proportion of the small copepod community particularly in spring and summer, with a peak of abundance in August. This is similar to Microcalanus pygmaeus in west Greenland (Digby, 1954) but in contrast to observations from Kongsfjorden (Hop et al., 2019b), east Greenland (Ussing, 1938) and the Amundsen Gulf (Darnis and Fortier, 2014), where Microcalanus spp. abundance peaked in later autumn and winter, indicating different strategies in the sub-Arctic Microcalanus populations compared to the high Arctic.
Pseudocalanus Species Complex
The peak in Pseudocalanus abundance in October coincided with a change in the Pseudocalanus species composition, although we cannot discount the possibility of a bias related to a change in DNA extraction kit coinciding with this change. While P. acuspes dominated in spring/summer and only P. moultoni was found in addition, P. minutus and P. elongatus appeared in autumn when all four species were found in more or less equal proportions. A similar succession has been recently observed in Svalbard (Ershova et al., 2021) indicating species specific differences in life history strategies. The pronounced annual cycle in abundance in P. acuspes, peaking during summer and declining in winter, indicates a dependence on the spring bloom for growth and development. This is in agreement with recent observations from Svalbard (Ershova et al., 2021) and previous studies in the Baltic sea (Peters et al., 2006; Renz and Hirche, 2006), Balsfjord (Norrbin, 1991) and Nova Scotia (McLaren et al., 1989). P. acuspes life cycle is described as mostly annual, but the P. acuspes population can also produce a second generation (McLaren et al., 1989; Norrbin, 1991; Peters et al., 2006) and such a second reproductive effort could explain the second peak in BWSR in autumn. It has been suggested that P. moultoni has a more boreal distribution where it reproduces year-round (McLaren et al., 1989), but little is known of P. moultoni life cycle in Arctic and sub-Arctic waters as it has been misidentified for a long time (Aarbakke et al., 2017; Hop et al., 2019b). Our study confirmed that this species is established in Ramfjord year-round, and thus likely can reproduce successfully in sub-Arctic fjords. P. minutus the largest of the observed species, has a strictly annual life cycle in other parts of the world, spending most of its life as C4–C5 copepodites and relying on lipid stores more than its sibling species (McLaren et al., 1989). The distribution of P. minutus is generally restricted to ice-covered Arctic shelf seas (Melnikov et al., 2005; Persson et al., 2012; Ershova and Kosobokova, 2019) or the deep Atlantic Ocean (Wiborg, 1955; Aarbakke et al., 2017). In Isfjorden, a Svalbard fjord heavily influenced by Atlantic advection and lacking a seasonal ice cover, P. minutus failed to complete its life cycle and was only advected into the fjord during the summer months (Ershova et al., 2021). Similarly, we observed P. minutus in Ramfjord only between September and March, together with other non-resident species, suggesting an Atlantic origin. Likewise, P. elongatus is a warm water boreal species (Unal et al., 2006) and was present in Ramfjord only during the fall and the winter, likely due to advection.
Calanus Life Cycle
The co-existence of several Calanus species is not unusual for Arctic and sub-Arctic locations where sympatric populations of C. glacialis, C. finmarchicus and C. hyperboreus are common (e.g., Madsen et al., 2001; Arnkvaern et al., 2005; Darnis and Fortier, 2014; Choquet et al., 2017). These species differ in life history strategies such as timing of reproduction, life cycle duration and overwintering stages (Tande et al., 1985; Arnkvaern et al., 2005; Falk-Petersen et al., 2009) even when living in the same habitat. Our data do not allow us to distinguish life history strategies of the different Calanus species in Ramfjord. Higher sampling resolution around the spawning event as well as genetic identification of nauplii and the young copepodite stages are needed to describe the life cycles of the different Calanus species in Ramfjord more precisely.
Development from egg to nauplii and CV takes around 60 days at temperatures observed in Ramfjord (McLaren, 1978; Campbell et al., 2001; Daase et al., 2011). Since we observed high abundance of copepod nauplii in February and March, and CI were recorded in high abundance in early April, spawning of Calanus spp. probably started in February and thus before the phytoplankton spring bloom. Spawning prior to the spring bloom is common in C. glacialis (Daase et al., 2013), while C. finmarchicus is often described to follow an income breeding strategy (i.e., being dependent of the spring bloom for gonad maturation and reproduction (Varpe et al., 2009). Previous studies from Balsfjord described a close synchronization of spawning with the phytoplankton bloom in C. finmarchicus (Tande and Hopkins, 1981; Tande, 1982; Grønvik and Hopkins, 1984; Hopkins et al., 1984). However, Hirche et al. (2001) observed CI and CII C. finmarchicus in the water column before the spring bloom in the Norwegian Sea, indicating that C. finmarchicus is able to start reproduction ahead of the bloom, and we suggest that nauplii and young copepodites likely consisted of both C. glacialis and C. finmarchicus in Ramfjord.
An increase in abundance of young copepodites prior to the autumn bloom in October suggested a second spawning event, most likely by C. finmarchicus who can produce a second generation as previously described in the Scotian shelf (McLaren et al., 2001) and the Norwegian Sea (Wiborg, 1954; Marshall and Orr, 1955; Matthews et al., 1978; Strand et al., 2020).
We suggest that the C. finmarchicus/glacialis population in Ramfjord has a 1-year life cycle as the population was dominated by CV for large parts of the year, a common overwintering stage of both C. finmarchicus and C. glacialis (Tande, 1982; Arnkvaern et al., 2005). For C. glacialis, a 1–2 years life cycle (e.g., Kosobokova, 1999; Arnkvaern et al., 2005; Daase et al., 2013) and for C. finmarchicus a 1-year life cycle is commonly described for Svalbard fjords (Kwasniewski et al., 2003; Arnkvaern et al., 2005), the Barents Sea (Tande et al., 1985), as well as in sub-Arctic locations (Wiborg, 1954; Marshall and Orr, 1955; McLaren, 1978; Gislason and Astthorsson, 1998; Astthorsson and Gislason, 2003) including Balsfjord (Tande, 1982). In Ramfjord, Calanus spp. had disappeared from the upper 50 m by August (data not shown) except for the young stages observed in October and November 2019. The generally low abundance of Calanus throughout the water column in autumn and winter suggests that the population either suffered high mortality already at the start of the overwintering period, or that they do not overwinter in Ramfjord but seek refuge elsewhere. Relatively low abundance and high mortality of C. finmarchicus have been observed in other Norwegian fjords during winter (Bagoien et al., 2001; Skreslet et al., 2015), although deep fjords can be suitable for overwintering (Hirche, 1983; Espinasse et al., 2016). Due to its shallowness, Ramfjord is likely not a good overwintering habitat for Calanus spp., whose overwintering population is generally found at greater depth, particular in oceanic populations of C. finmarchicus and C. hyperboreus (600–2000 m, Hirche, 1991; Heath et al., 2004). These deep habitats provide not only refuge from predation and physiological advantages (lower metabolic cost in cold water), but also affect the buoyancy of lipid rich copepods with implications on their energy budget during diapause. Changes in wax ester chemistry reduces the buoyancy when descending below 500 m (Pond and Tarling, 2011), thus affecting buoyancy control during overwintering. Calanus spp. in shallow waters such as Ramfjord cannot reach a depth where they are neutrally buoyant, thus may have to work actively to remain at depth, which can be energetically demanding and reduce fitness.
Methodical Considerations
While metabarcoding provides a much more detailed species inventory than visual inspection, how the proportion of frequency reads relates to the actual proportion of species in the community and how sequences reads can be used to quantify species biomass is still in debate. A meta-analyses of 22 studies looking at a wide variety of biological communities ranging from land plants to fish investigated to which degree metabarcoding is quantitative (Lindeque et al., 2013; Lamb et al., 2019) and showed a weak correlation between the number of reads and biomass with a large degree of uncertainties. However, only two of those examined studies looked at marine zooplankton, and neither of those used the primer set that we employed in this work. The quantitative value of our approach is described in detail in Ershova et al. (In revision) and has shown robust correlations between relative biomass and sequence read counts in high latitude marine zooplankton communities, although with biases toward certain taxonomic groups. The higher quantitative value of this method is obtained by the application of universal primers with a high level of degeneracy and the absence of a second PCR step at the library preparation stage. However, it is important to highlight that the BWSR measure that we use in this study remains semi-quantitative, meaning that it is useful in the context of seasonal comparisons of taxa-specific biomass in a single study, but likely not for comparing biomass estimates using other methods. It is noteworthy that in our study taxonomy and metabarcoding generally showed similar trends in the seasonal changes of the zooplankton community structure. BWSR captured the same seasonal peaks in O. similis, Microcalanus spp., A. longiremis, and T. longicornis as the abundance estimates. Only for Pseudocalanus and M. norvegica did the two methods show different patterns. The high BWSR of P. acuspes between May and August contradicted the low abundance of Pseudocalanus spp. in that period, although this could have been biased by the presence of nauplii which also peak during this time period (Vazyulya et al., 2001) and were not identified to genus. Low abundance but high BWSR would indicate a dominance of older stages and adults (few individuals but high individual biomass), but given P. acuspes life history (see above) the population should have been composed mainly of young stages with low individual biomass during that time period, and high abundance of those would have been recognized during the visual inspection. Microsetella norvegica is not sampled effectively by traditional zooplankton nets (Svensen et al., 2018) and was likely underestimated, especially after the change in mesh size when abundance decreased markedly. Despite its low individual biomass, we would have expected a higher proportion of sequence reads at the early part of the study when we used the smaller mesh size, but the number of sequence reads of M. norvegica were almost negligible year-round.
The increase in mesh size from April did not only result in an underestimation of M. norvegica but likely also other smaller organisms, such as small meroplankton and young copepodite stages, particularly those of small copepods (Vinogradov and Shushkina, 1987; Nielsen and Andersen, 2002; Tseng et al., 2011). Consequently, their abundances and the species richness between November 2018 and April are not directly comparable with the rest of the study. There is however little evidence that larger copepods and older life stage of smaller copepods (including copepodites stage CIII-adults of Calanus and Pseudocalanus) are caught less efficiently with a mesh size of 64 μm compared to 180 μm (e.g., Nichols and Thompson, 1991; Di Mauro et al., 2009; Altukhov et al., 2015; Chen et al., 2016). Thus, we are confident that the seasonal patterns we observed in the morphological data are not severely biased by the change in mesh size, especially when we take the change of mesh size into consideration in our data interpretation. Furthermore, despite the use of the 180 μm mesh, we did observe a strong increase of the abundance of most of the small copepod. Even if their abundance is underestimated, our data provide clear evidence of seasonal changes, such as a strong increase in abundance in autumn. Finally, the application of two different DNA extraction kits during the course of the study highlights the biases that can be introduced at this stage of the analysis. The EZNA Mollusc DNA Kit recovered, on average, 44% higher diversity than the Qiagen PowerSoil Kit, especially among the meroplankton and non-crustacean taxa (Supplementary Figure 2). Unfortunately, the lack of a temporal overlap in the application of the two kits precludes a more concrete analysis of the taxonomic biases of either and remains to be resolved in future studies.
One of the main concerns of the effect of climate warming on plankton communities is the potentially negative effect of changes in the algal bloom phenology related to zooplankton life history strategies. These changes may alter the energy transfer through the pelagic food web and potentially also impact benthic invertebrates through their pelagic early life stages. Furthermore, biogeographical distributional shifts may change community composition with repercussions on energy transfer and ecosystem structure (Beaugrand et al., 2009; Chust et al., 2013). In order to document changes and to be able to distinguish between natural seasonal variability and climate change impacts on ecosystems structure and functioning, we need to establish baselines, such as detailed species inventories and how community composition varies seasonally. Species specific changes in life histories can only be observed if species are correctly identified, as even closely related species may vary in their annual routines and their role in the ecosystem structure. Our study demonstrates that the combination of both morphological and metabarcoding approaches is providing the necessary quantitative and qualitative detail to document seasonal changes in community composition and population structure. While our study focused on mesozooplankton, future studies are needed to fully describe the community composition of the microplankton and macrozooplankton community.
Conclusion
There are few studies from sub-Arctic locations describing the seasonal variability in the zooplankton community structure. The combination of traditional methods of identification and state-of-the-art molecular tools allowed us to provide high-resolution data on seasonal variability in zooplankton abundance and diversity at a much higher taxonomic resolution. Both methods were highly complementary, with metabarcoding providing the most extensive species list of mesozooplankton from a Norwegian fjord to date, particular in terms of the meroplankton which are rarely identified to species in most zooplankton studies. 154 unique taxa were identified in Ramfjord over the study period, 58% were meroplankton organisms. Seasonality in the zooplankton community structure was driven by the seasonal changes in temperature and algae biomass and was manifested not only by changes in abundance and biomass but also by changes in diversity, although methodological shortcomings limits our ability to identify seasonal changes in diversity to some extent. The succession of meroplankton was an important factor driving the seasonal changes in the mesozooplankton community over summer. An assessment of the diversity of the benthic community is needed to determine the role of advection and local production of the meroplankton community in the Ramfjord/Balsfjord system, and how seasonal changes in meroplankton composition and abundance are linked to difference in the life history strategies of the various species.
Data Availability Statement
The datasets presented in this study can be found in online repositories. The names of the repository/repositories and accession number(s) can be found below: https://www.gbif.org/dataset/b4804f19-8a8a-49e7-8dc2-79b528635696. The raw sequencing datasets presented in this study are publicly 882 available in the Sequence Read Archive (SRA) repository of NCBI. Bioproject ID: PRJNA742507. 883 https://www.ncbi.nlm.nih.gov/bioproject/742507.
Author Contributions
EE, MD, RG, TV, and EC designed the study. EC, TV, and EE realized the sampling. EE and OW did the metabarcoding analysis and the bioinformatic. EC, MD, and EE did the zooplankton visual identification and wrote the first draft of the manuscript. TV did the phytoplankton cell count and the measure of the cholorophyll a concentration. MD, EC, and EE did the data analysis and data visualization. All authors contributed to the article and approved the submitted version.
Funding
This study was supported by funding from the “Arctic ABC” project funded by the Norwegian Research Council (NRC no. 244319), by the project “CalAct” funded by Sentinel Nord through the Université Laval/University of Tromsø research partnership, and the CHASE project, part of the Changing Arctic Ocean program, jointly funded by the UKRI Natural Environment Research Council (NERC, project no.: NE/R012733/1) and the German Federal Ministry of Education and Research (BMBF, project no.: 03F0803A) and by Arctic SIZE funded by the Norwegian Government and Tromsø Research Foundation (project #01vm/h15). The work of EE was partially supported by Research Council of Norway (Norges forskningsråd) through the CoastRisk initiative (NFR 299554/F40) and within the framework of the state assignment of IO RAS (theme no. 0128–2021–0007). JB and MD were financially supported by the Norwegian Research Council projects Deep Impact (project no 300333) and the Centre of Excellence AMOS (project no. 223254). This is a contribution to the ARCTOS research network (arctos.uit.no) and the Arctic Science Partnership (www.asp-net.org).
Conflict of Interest
The authors declare that the research was conducted in the absence of any commercial or financial relationships that could be construed as a potential conflict of interest.
Publisher’s Note
All claims expressed in this article are solely those of the authors and do not necessarily represent those of their affiliated organizations, or those of the publisher, the editors and the reviewers. Any product that may be evaluated in this article, or claim that may be made by its manufacturer, is not guaranteed or endorsed by the publisher.
Acknowledgments
We thank the captains and crew of R/V Hyas, for their help during the sampling. We thank Daniel Vogedes for his work with the mooring, its deployment and its recovery. We also thank C. Barth-Jensen and R. Descoteaux for helping with the molecular work. We thank the two reviewers for their constructive comments.
Supplementary Material
The Supplementary Material for this article can be found online at: https://www.frontiersin.org/articles/10.3389/fmars.2021.705042/full#supplementary-material
Footnotes
References
Aarbakke, O. N. S., Fevolden, S.-E., and Weydmann, A. (2017). Relative summer abundances and distribution of Pseudocalanus spp. (Copepoda: Calanoida) adults in relation to environmental variables in the Nordic Seas and Svalbard fjords. Polar Biol. 40, 51–59. doi: 10.1007/s00300-016-1923-0
Altukhov, D., Siokou, I., Pantazi, M., Stefanova, K., Timofte, F., Gubanova, A., et al. (2015). Intercomparison of five nets used for mesozooplankton sampling. Mediterr. Mar. Sci. 16, 550–561.
Andersen, O. G. N. (1984). Meroplankton in Jorgen Bronlund Fjord, North Greenland. Copenhagen: Museum Tusculanum Press.
Antich, A., Palacin, C., Wangensteen, O. S., and Turon, X. (2021). To denoise or to cluster, that is not the question: optimizing pipelines for COI metabarcoding and metaphylogeography. BMC Bioinformatics 22:177.
Arnkvaern, G., Daase, M., and Eiane, K. (2005). Dynamics of coexisting Calanus finmarchicus, Calanus glacialis and Calanus hyperboreus populations in a high-Arctic fjord. Polar Biol. 28, 528–538. doi: 10.1007/s00300-005-0715-8
Astthorsson, O. S., and Gislason, A. (2003). Seasonal variations in abundance, development and vertical distribution of Calanus finmarchicus, C. hyperboreus and C. glacialis in the East Icelandic current. J. Plankton Res. 25, 843–854. doi: 10.1093/plankt/25.7.843
Bagoien, E., Kaartvedt, S., Aksnes, D. L., and Eiane, K. (2001). Vertical distribution and mortality of overwintering Calanus. Limnol. Oceanogr. 46, 1494–1510. doi: 10.4319/lo.2001.46.6.1494
Bagoien, E., Kaartvedt, S., and Overas, S. (2000). Seasonal vertical migrations of Calanus spp. in Oslofjorden. Sarsia 85, 299–311. doi: 10.1080/00364827.2000.10414581
Båmstedt, U., Tande, K., and Nicolajsen, H. (1985). “Ecological investigations on the zooplankton community of Balsfjorden, northern Norway: physiological adaptations in Metridia longa (copepoda) to the overwintering periods,” in Marine Biology of Polar Regions and Effects of Stress on Marine Organisms, eds J. S. Gray and M. E. Christiansen (Chichester: John Wiley & Sons Ltd), 313–327.
Barthel, K.-G., Noji, T. T., and Noji, C. I.-M. (1995). Zooplankton dynamics during the onset of winter in a northern Norwegian fjord. Vertical distribution and metabolic activity in relation to phytoplankton and sedimentation. Sarsia 80, 23–32. doi: 10.1080/00364827.1995.10413575
Barth-Jensen, C., Koski, M., Varpe, Ø, Glad, P., Wangensteen, O. S., Præbel, K., et al. (2020). Temperature-dependent egg production and egg hatching rates of small egg-carrying and broadcast-spawning copepods Oithona similis, Microsetella norvegica and Microcalanus pusillus. J. Plankton Res. 42, 564–580. doi: 10.1093/plankt/fbaa039
Beaugrand, G., Luczak, C., and Edwards, M. (2009). Rapid biogeographical plankton shifts in the North Atlantic Ocean. Glob. Chang. Biol. 15, 1790–1803. doi: 10.1111/j.1365-2486.2009.01848.x
Berge, J., Daase, M., Hobbs, L., Falk-Petersen, S., Darnis, G., and Søreide, J. E. (2020). “Zooplankton in the Polar Night,” in Polar Night Marine Ecology–Life and Light in the Dead of the Night, 1st Edn, eds J. Berge, G. Johnsen, and J. H. Cohen (Cham: Springer International Publishing), 113–159.
Bluhm, B. A., Ambrose, W. G., Bergmann, M., Clough, L. M., Gebruk, A. V., Hasemann, C., et al. (2011). Diversity of the arctic deep-sea benthos. Mar. Biodivers. 41, 87–107. doi: 10.1007/s12526-010-0078-4
Boyer, F., Mercier, C., Bonin, A., Le Bras, Y., Taberlet, P., and Coissac, E. (2016). obitools: A unix−inspired software package for DNA metabarcoding. Mol. Ecol. Resour. 16, 176–182. doi: 10.1111/1755-0998.12428
Bucklin, A., Lindeque, P. K., Rodriguez-Ezpeleta, N., Albaina, A., and Lehtiniemi, M. (2016). Metabarcoding of marine zooplankton: prospects, progress and pitfalls. J. Plankton Res. 38, 393–400. doi: 10.1093/plankt/fbw023
Campbell, R. G., Wagner, M. M., Teegarden, G. J., Boudreau, C. A., and Durbin, E. G. (2001). Growth and development rates of the copepod Calanus finmarchicus reared in the laboratory. Mar. Ecol. Prog. Ser. 221, 161–183. doi: 10.3354/meps221161
Castellani, C., Irigoien, X., Harris, R. P., and Lampitt, R. S. (2005). Feeding and egg production of Oithona similis in the North Atlantic. Mar. Ecol. Prog. Ser. 288, 173–182. doi: 10.3354/meps288173
Chen, H., Yu, H., and Liu, G. (2016). Comparison of copepod collection efficiencies by three commonly used plankton nets: a case study in Bohai Sea, China. J. Ocean Univ. China 15, 1007–1013.
Choquet, M. (2017). Combining Ecological and Molecular Approaches to Redefine the Baseline Knowledge of the Genus Calanus in the North Atlantic and the Arctic Ocean. Ph. D. thesis. Bodø: Nord University.
Choquet, M., Hatlebakk, M., Dhanasiri, A. K. S., Kosobokova, K., Smolina, I., Søreide, J. E., et al. (2017). Genetics redraws pelagic biogeography of Calanus. Biol. Lett. 13:20170588. doi: 10.1098/rsbl.2017.0588
Choquet, M., Kosobokova, K., Kwaśniewski, S., Hatlebakk, M., Dhanasiri, A. K. S., Melle, W., et al. (2018). Can morphology reliably distinguish between the copepods Calanus finmarchicus and C. glacialis, or is DNA the only way? Limnol. Oceanogr. Methods 16, 237–252. doi: 10.1002/lom3.10240
Chust, G., Castellani, C., Licandro, P., Ibaibarriaga, L., Sagarminaga, Y., and Irigoien, X. (2013). Are Calanus spp. shifting poleward in the North Atlantic? A habitat modelling approach. ICES J. Mar. Sci. 71, 241–253. doi: 10.1093/icesjms/fst147
Cleary, A. C., Durbin, E. G., Rynearson, T. A., and Bailey, J. (2016). Feeding by Pseudocalanus copepods in the Bering Sea: trophic linkages and a potential mechanism of niche partitioning. Deep Sea Res. 2 Top. Stud. Oceanogr. 134, 181–189. doi: 10.1016/j.dsr2.2015.04.001
Cottier, F., Nilsen, F., Skogseth, R., Tverberg, V., Skarðhamar, J., and Svendsen, H. (2010). Arctic fjords: a review of the oceanographic environment and dominant physical processes. Geol. Soc. Lond. Spec. Publ. 344, 35–50. doi: 10.1144/SP344.4
Daase, M., and Eiane, K. (2007). Mesozooplankton distribution in northern Svalbard waters in relation to hydrography. Polar Biol. 30, 969–981. doi: 10.1007/s00300-007-0255-5
Daase, M., Falk-Petersen, S., Varpe, Ø, Darnis, G., Søreide, J. E., Wold, A., et al. (2013). Timing of reproductive events in the marine copepod Calanus glacialis: a pan-Arctic perspective. Can. J. Fish. Aquat. Sci. 70, 871–884. doi: 10.1139/cjfas-2012-0401
Daase, M., Søreide, J. E., and Martynova, D. (2011). Effects of food quality on naupliar development in Calanus glacialis at subzero temperatures. Mar. Ecol. Prog. Ser. 429, 111–124. doi: 10.3354/meps09075
Darnis, G., and Fortier, L. (2014). Temperature, food and the seasonal vertical migration of key arctic copepods in the thermally stratified Amundsen Gulf (Beaufort Sea, Arctic Ocean). J. Plankton Res. 36, 1092–1108. doi: 10.1093/plankt/fbu035
Di Mauro, R., Capitanio, F., and Viñas, M. D. (2009). Capture efficiency for small dominant mesozooplankters (Copepoda, Appendicularia) off Buenos Aires Province (34°S-41°S), Argentine Sea, using two plankton mesh sizes. Braz. J. Oceanogr. 57, 205–214.
Digby, P. S. B. (1954). The biology of the marine planktonic copepods of scoresby-sound, East Greenland. J. Anim. Ecol. 23, 298–338. doi: 10.2307/1984
Drent, J. (2002). Temperature responses in larvae of Macoma balthica from a northerly and southerly population of the European distribution range. J. Exp. Mar. Biol. Ecol. 275, 117–129. doi: 10.1016/s0022-0981(02)00141-7
Dvoretsky, V. G., and Dvoretsky, A. G. (2009). Life cycle of Oithona similis (Copepoda: Cyclopoida) in Kola Bay (Barents Sea). Mar. Biol. 156, 1433–1446. doi: 10.1007/s00227-009-1183-4
Dvoretsky, V. G., and Dvoretsky, A. G. (2010). Mesozooplankton structure in Dolgaya Bay (Barents Sea). Polar Biol. 33, 703–708. doi: 10.1007/s00300-009-0748-5
Edgar, R. C., Haas, B. J., Clemente, J. C., Quince, C., and Knight, R. (2011). UCHIME improves sensitivity and speed of chimera detection. Bioinformatics 27, 2194–2200. doi: 10.1093/bioinformatics/btr381
Eilertsen, H. C., Falk-Petersen, S., Hopkins, C., and Tande, K. (1981a). Ecological investigations on the plankton community of Balsfjorden, northern Norway: program for the project, study area, topography, and physical environment. Sarsia 66, 25–34. doi: 10.1080/00364827.1981.10414517
Eilertsen, H. C., and Frantzen, S. (2007). Phytoplankton from two sub-Arctic fjords in northern Norway 2002–2004: I. Seasonal variations in chlorophyll a and bloom dynamics. Mar. Biol. Res. 3, 319–332. doi: 10.1080/17451000701632877
Eilertsen, H. C., Schei, B., and Taasen, J. (1981b). Investigations on the plankton community of Bals-fjorden, Northern Norway: the phytoplankton 1976–1978. Abundance, species composition, and succession. Sarsia 66, 129–141. doi: 10.1080/00364827.1981.10414530
Ellingsen, K., and Gray, J.s. (2002). Spatial patterns of benthic diversity: is there a latitudinal gradient along the Norwegian continental shelf? J. Anim. Ecol. 71, 373–389. doi: 10.1046/j.1365-2656.2002.00606.x
Ershova, E. A., and Kosobokova, K. N. (2019). Cross-shelf structure and distribution of mesozooplankton communities in the East-Siberian Sea and the adjacent Arctic Ocean. Polar Biol. 42, 1353–1367. doi: 10.1007/s00300-019-02523-2
Ershova, E. A., Nyeggen, M. U., Yurikova, D. A., and SØreide, J. E. (2021). Seasonal dynamics and life histories of three sympatric species of Pseudocalanus in two Svalbard fjords. J. Plankton Res. 43, 209–223. doi: 10.1093/plankt/fbab007
Ershova, E. A., Wangensteen, O. S., Descoteaux, R., Barth-Jensen, C., and Præbel, K. (In revision). Metabarcoding as a quantitative tool for estimation biodiversity and relative biomass of marine zooplankton. ICES.
Espinasse, B., Basedow, S. L., Tverberg, V., Hattermann, T., and Eiane, K. (2016). A major Calanus finmarchicus overwintering population inside a deep fjord in northern Norway: implications for cod larvae recruitment success. J. Plankton Res. 38, 604–609. doi: 10.1093/plankt/fbw024
Estrada, R., Harvey, M., Gosselin, M., Starr, M., Galbraith, P. S., and Straneo, F. (2012). Late-summer zooplankton community structure, abundance, and distribution in the Hudson Bay system (Canada) and their relationships with environmental conditions, 2003-2006. Prog. Oceanogr. 101, 121–145. doi: 10.1016/j.pocean.2012.02.003
Falkenhaug, T., Tande, K., and Timonin, A. (1997). Spatio-temporal patterns in the copepod community in Malangen, northern Norway. J. Plankton Res. 19, 449–468.
Falk-Petersen, I.-B. (1982). Ecological investigations on the zooplankton community of Balsfjorden, northern Norway. Sarsia 67, 69–78. doi: 10.1080/00364827.1982.10421335
Falk-Petersen, S. (1981). Ecological investigation on the zooplankton community of Balsfjorden, Northern Norway: seasonal changes in body weight and the main biochemical composition of Thysanoessa inermis (Krøyer), T. raschii (M. Sars) and Meganyctiphanes norvegica (M. Sars) in relation to environmental factors. J. Exp. Mar. Biol. Ecol. 49, 103–120. doi: 10.1016/0022-0981(81)90065-4
Falk-Petersen, S. (1985). Growth of the euphausiids Thysanoessa inermis, Thysanoessa raschii, and Meganyctiphanes norvegica in a subarctic fjord, North Norway. Can. J. Fish. Aquat. Sci. 42, 14–22. doi: 10.1139/f85-002
Falk-Petersen, S., and Hopkins, C. C. E. (1981). Ecological investigations on the zooplankton community of Balsfjorden, northern Norway: population dynamic of the euphaciids Thysanoessa inermis (Kröyer), Thysanoessa raschii (M.Sars) and Meganyctiphanes norvegica (M.Sars) in 1976 and 1977. J. Plankton Res. 3, 177–191. doi: 10.1093/plankt/3.2.177
Falk-Petersen, S., Mayzaud, P., Kattner, G., and Sargent, J. (2009). Lipids and life strategy of Arctic Calanus. Mar. Biol. Res. 5, 18–39. doi: 10.1080/17451000802512267
Falk-Petersen, S., Sargent, J. R., Hopkins, C. C. E., and Vaja, B. (1982). Ecological investigations on the zooplankton community in Balsfjorden, Northern Norway: lipids in Euphasiids Thysanoessa raschi and T. inermis during spring. Mar. Biol. 68, 97–102. doi: 10.1007/BF00393147
Fetzer, I., and Arntz, W. E. (2008). Reproductive strategies of benthic invertebrates in the Kara Sea (Russian Arctic): adaptation of reproduction modes to cold water. Mar. Ecol. Prog. Ser. 356, 189–202. doi: 10.3354/meps07271
Frøslev, T. G., Kjøller, R., Bruun, H. H., Ejrnæs, R., Brunbjerg, A. K., Pietroni, C., et al. (2017). Algorithm for post-clustering curation of DNA amplicon data yields reliable biodiversity estimates. Nat. Commun. 8:1188. doi: 10.1038/s41467-017-01312-x
Gaarder, K. R. (1938). Phytoplankton Studies from the Tromsø District, 1930-31. Tromsø: Tromsø museum.
Gabrielsen, T., Merkel, B., Søreide, J., Johansson-Karlsson, E., Bailey, A., Vogedes, D., et al. (2012). Potential misidentifications of two climate indicator species of the marine arctic ecosystem: Calanus glacialis and C. finmarchicus. Polar Biol. 35, 1621–1628. doi: 10.1007/s00300-012-1202-7
Geller, J., Meyer, C., Parker, M., and Hawk, H. (2013). Redesign of PCR primers for mitochondrial cytochrome c oxidase subunit I for marine invertebrates and application in all−taxa biotic surveys. Mol. Ecol. Resour. 13, 851–861. doi: 10.1111/1755-0998.1238
Gislason, A., and Astthorsson, O. S. (1998). Seasonal variations in biomass, abundance and composition of zooplankton in the subarctic waters north of Iceland. Polar Biol. 20, 85–94. doi: 10.1007/s003000050280
Gradinger, R., and Baumann, M. (1991). Distribution of phytoplankton communities in relation to the large-scale hydrographical regime in the Fram Strait. Mar. Biol. 111, 311–321. doi: 10.1007/BF01319714
Gran-Stadniczeñko, S., Egge, E., Hostyeva, V., Logares, R., Eikrem, W., and Edvardsen, B. (2019). Protist diversity and seasonal dynamics in Skagerrak plankton communities as revealed by metabarcoding and microscopy. J. Eukaryot. Microbiol. 66, 494–513. doi: 10.1111/jeu.12700
Grønvik, S., and Hopkins, C. C. E. (1984). Ecological investigations of the zooplankton community of Balsfjorden, Northern Norway: generation cycle, seasonal vertical distribution and seasonal variations in body weight and carbon and nitrogen content of the copepod Metridia longa (Lubbock). J. Exp. Mar. Biol. Ecol. 80, 93–107. doi: 10.1016/0022-0981(84)90096-0
Hansen, B. W., Nielsen, T. G., and Levinsen, H. (1999). Plankton community structure and carbon cycling on the western coast of Greenland during the stratified summer situation. III. Mesozooplankton. Aquat. Microb. Ecol. 16, 233–249. doi: 10.3354/ame016233
Hays, G. C., Richardson, A. J., and Robinson, C. (2005). Climate change and marine plankton. Trends Ecol. Evol. 20, 337–344. doi: 10.1016/j.tre.2005.03.004
Heath, M. R., Boyle, P. R., Gislason, A., Gurney, W. S. C., Hay, S. J., Head, E. J. H., et al. (2004). Comparative ecology of over-wintering Calanus finmarchicus in the northern North Atlantic, and implications for life-cycle patterns. ICES J. Mar. Sci. 61, 698–708. doi: 10.1016/j.icesjms.2004.03.013
Hirche, H. J. (1983). Overwintering of Calanus finmarchicus and Calanus helgolandicus. Mar. Ecol. Prog. Ser. 11, 281–290.
Hirche, H. J. (1991). Distribution of dominant calanoid copepod species in the Greenland Sea during late fall. Polar Biol. 11, 351–362. doi: 10.1007/BF00239687
Hirche, H. J., Brey, T., and Niehoff, B. (2001). A high-frequency time series at ocean weather ship station M (Norwegian Sea): population dynamics of Calanus finmarchicus. Mar. Ecol. Prog. Ser. 219, 205–219. doi: 10.3354/meps219205
Holte, B. (1998). The macrofauna and main functional interactions in the sill basin sediments of the pristine holandsfjord, Northern Norway, with autecological reviews for some key-species. Sarsia 83, 55–68. doi: 10.1080/00364827.1998.10413669
Hop, H., Assmy, P., Wold, A., Sundfjord, A., Daase, M., Duarte, P., et al. (2019a). Pelagic ecosystem characteristics across the Atlantic water boundary current from Rijpfjorden, Svalbard, to the Arctic ocean during summer (2010–2014). Front. Mar. Sci. 6:181. doi: 10.3389/fmars.2019.00181
Hop, H., Wold, A., Vihtakari, M., Daase, M., Kwasniewski, S., Gluchowska, M., et al. (2019b). “Zooplankton in Kongsfjorden (1996–2016) in relation to climate change,” in The Ecosystem of Kongsfjorden, Svalbard, eds H. Hop and C. Wiencke (Cham: Springer International Publishing), 229–300.
Hopcroft, R. R., Kosobokova, K. N., and Pinchuk, A. I. (2010). Zooplankton community patterns in the Chukchi Sea during summer 2004. Deep Sea Res. 2 Top. Stud. Oceanogr. 57, 27–39. doi: 10.1016/j.dsr2.2009.08.003
Hopkins, C. (1981). Ecological investigations on the zooplankton community of Balsfjorden, northern Norway: changes in zooplankton abundance and biomass in relation to phytoplankton and hydrography, March 1976–February 1977. Kiel. Meeresforsch. 5, 124–139.
Hopkins, C. C. E., Falk-Petersen, S., Tande, K., and Eilertsen, H. C. (1978). A preliminary study of zooplankton sound scattering layers in Balsfjorden: structure, energetics and migrations. Sarsia 63, 255–264.
Hopkins, C. C. E., Grotnes, P. E., and Eliassen, J.-E. (1989). Organization of a fjord community at 70 North: the pelagic food web in Balsfjord, northern Norway. Rapports et proces-verbaux des reunions conseil permanent International pour l’. Explor. de la Mer 188, 146–153.
Hopkins, C. C. E., Tande, K. S., and Grønvik, S. (1984). Ecological investigations of the zooplankton community of Balsfjorden, Northern Norway. An analysis of growth and overwintering tactics in relation to niche and environment in Metridia longa (Lubbock), Calanus finmarchicus (Gunnerus), Thysanoessa inmeris (Krøyer) and T. raschi (M. Sars). J. Exp. Mar. Biol. Ecol. 82, 77–99.
Hsieh, T., Ma, K., and Chao, A. (2016). iNEXT: an R package for rarefaction and extrapolation of species diversity (H ill numbers). Methods Ecol. Evol. 7, 1451–1456.
Hughes, L. (2000). Biological consequences of global warming: is the signal already apparent? Trends Ecol. Evol. 15, 56–61. doi: 10.1016/S0169-5347(99)01764-4
Johnsen, G., Leu, E., and Gradinger, R. (2020). “Marine micro-and macroalgae in the polar night,” in POLAR NIGHT Marine Ecology, eds J. Berge, G. Johnsen, and J. Cohen (Cham: Springer), 67–112.
Kosobokova, K. N. (1999). The reproductive cycle and life history of the Arctic copepod Calanus glacialis in the White Sea. Polar Biol. 22, 254–263. doi: 10.1007/s003000050418
Kuklinski, P., Berge, J., McFadden, L., Dmoch, K., Zajaczkowski, M., Nygård, H., et al. (2013). Seasonality of occurrence and recruitment of Arctic marine benthic invertebrate larvae in relation to environmental variables. Polar Biol. 36, 549–560. doi: 10.1007/s00300-012-1283-3
Kwasniewski, S., Hop, H., Falk-Petersen, S., and Pedersen, G. (2003). Distribution of Calanus species in Kongsfjorden, a glacial fjord in Svalbard. J. Plankton Res. 25, 1–20. doi: 10.1093/plankt/25.1.1
Kwasniewski, S., Walkusz, W., Cottier, F. R., and Leu, E. (2013). Mesozooplankton dynamics in relation to food availability during spring and early summer in a high latitude glaciated fjord (Kongsfjorden), with focus on Calanus. J. Mar. Syst. 11, 83–96. doi: 10.1016/j.jmarsys.2012.09.012
Lamb, P. D., Hunter, E., Pinnegar, J. K., Creer, S., Davies, R. G., and Taylor, M. I. (2019). How quantitative is metabarcoding: a meta-analytical approach. Mol. Ecol. 28, 420–430. doi: 10.1111/mec.14920
Lindeque, P. K., Parry, H. E., Harmer, R. A., Somerfield, P. J., and Atkinson, A. (2013). Next generation sequencing reveals the hidden diversity of zooplankton assemblages. PLoS One 8:e81327. doi: 10.1371/journal.pone.0081327
Lischka, S., and Hagen, W. (2005). Life histories of the copepods Pseudocalanus minutus, P. acuspes (Calanoida) and Oithona similis (Cyclopoida) in the Arctic Kongsfjorden (Svalbard). Polar Biol. 28, 910–921. doi: 10.1007/s00300-005-0017-1
Madsen, S. D., Nielsen, T. G., and Hansen, B. W. (2001). Annual population development and production by Calanus finmarchicus, C. glacialis and C. hyperboreus in Disko Bay, western Greenland. Mar. Biol. 139, 75–93. doi: 10.1007/s002270100552
Madsen, S. D., Nielsen, T. G., and Hansen, B. W. (2008). Annual population development and production by small copepods in Disko Bay, western Greenland. Mar. Biol. 155, 63–77. doi: 10.1007/s00227-008-1007-y
Mahé, F., Rognes, T., Quince, C., de Vargas, C., and Dunthorn, M. (2015). Swarm v2: highly-scalable and high-resolution amplicon clustering. PeerJ 3:e1420. doi: 10.7717/peerj.1420
Marshall, S. M., and Orr, A. P. (1955). The Biology of a Marine Copepod (Calanus finmarchicus Gunnerus). Edinburgh: Oliver & Boyd.
Matthews, J. B. L., Hestad, L., and Bakke, J. L. W. (1978). Ecological studies in Korsfjorden, Western Norway–generations and stocks of Calanus hyperboreus and Calanus finmarchicus in 1971-1974. Oceanol. Acta 1, 277–284.
McKinstry, C. A. E., and Campbell, R. W. (2018). Seasonal variation of zooplankton abundance and community structure in Prince William Sound, Alaska, 2009–2016. Deep Sea Res. 2 Top. Stud. Oceanogr. 147, 69–78. doi: 10.1016/j.dsr2.2017.08.016
McLaren, I. A. (1978). Generation lengths of some temperate marine copepods–estimation, prediction and implications. J. Fish. Res. Board Can. 35, 1330–1342. doi: 10.1139/f78-208
McLaren, I. A., Head, E., and Sameoto, D. D. (2001). Life cycles and seasonal distributions of Calanus finmarchicus on the central Scotian Shelf. Can. J. Fish. Aquat. Sci. 58, 659–670. doi: 10.1139/f01-007
McLaren, I. A., Laberge, E., Corkett, C. J., and Sevigny, J. M. (1989). Life-cycles of 4 species of Pseudocalanus in Nova-Scotia. Can. J. Zool. 67, 552–558. doi: 10.1139/z89-078
Melnikov, I., Dikarev, S., Egorov, V., Kolosova, E., and Zhitina, L. (2005). Structure of the coastal ice ecosystem in the zone of sea-river interactions. Oceanology 45, 511–519.
Michelsen, H. K., Svensen, C., Reigstad, M., Nilssen, E. M., and Pedersen, T. (2017). Seasonal dynamics of meroplankton in a high-latitude fjord. J. Mar. Syst. 168, 17–30. doi: 10.1016/j-marsys.2016.12.001
Nichols, J. H., and Thompson, A. B. (1991). Mesh selection of copepodite and nauplius stages of four calanoid copepod species. J. Plankt. Res. 13, 661–671.
Niehoff, B., and Hirche, H. J. (2005). Reproduction of Calanus glacialis in the Lurefjord (western Norway): indication for temperature-induced female dormancy. Mar. Ecol. Prog. Ser. 285, 107–115. doi: 10.3354/meps285107
Nielsen, T., and Andersen, C. (2002). Plankton community structure and production along a freshwater-influenced Norwegian fjord system. Mar. Biol. 141, 707–724.
Nielsen, T. G., Kjellerup, S., Smolina, I., Hoarau, G., and Lindeque, P. (2014). Live discrimination of Calanus glacialis and C. finmarchicus females: can we trust phenological differences? Mar. Biol. 161, 1299–1306. doi: 10.1007/s00227-014-2419-5
Nielsen, T. G., Ottosen, L. D., and Hansen, B. W. (2007). “Structure and function of the pelagic ecosystem in Young Sound, NE Greenland,” in Carbon Cycling in Arctic Marine Ecosystems: Case Study Young Sound, eds S. Rysgaard and R. N. Glud (Copenhagen: Danish Polar Center), 88–107.
Norrbin, F. (1994). Overwintering Strategies of Small Copepods in High-Latitude Marine Environments. Ph. D. thesis. Gothenburg: Goteborgs universitet.
Norrbin, F. M. (1993). Skewed sex ratios in overwintering copepodites of Pseudocalanus in Norwegian Fjords—a result of sex-specific selection? Bull. Mar. Sci. 53, 204–215.
Norrbin, M. F. (1991). Gonad maturation as an indication of seasonal cycles for several species of small copepods in the Barents Sea. Polar Res. 10, 421–432. doi: 10.1111/j.1751-8369.1991.tb00663.x
Norrbin, M. F., Olsen, R.-E., and Tande, K. S. (1990). Seasonal variation in lipid class and fatty acid composition of two small copepods in Balsfjorden, northern Norway. Mar. Biol. 105, 205–211. doi: 10.1007/BF01344288
Oksanen, J., Guillaume Blanchet, F., Kindt, R., Legendre, P., Minchin, P. R., O’Hara, R. B., et al. (2020). “vegan: Community Ecology Package”. R package version 2.5-6 ed.
Orkney, A., Platt, T., Narayanaswamy, B. E., Kostakis, I., and Bouman, H. A. (2020). Bio-optical evidence for increasing Phaeocystis dominance in the Barents Sea. Philos. Trans. R. Soc. A 378:20190357. doi: 10.1098/rsta.2019.0357
Oug, E. (1977). Faunal distribution close to sediment of a shallow marine-environment. Sarsia 63, 115–121. doi: 10.1080/00364827.1977.10411329
Oug, E., and Høisœter, T. (2000). Soft-bottom macrofauna in the high-latitude ecosystem of Balsfjord, northern Norway: species composition, community structure and temporal variability. Sarsia 85, 1–13. doi: 10.1080/00364827.2000.10414551
Pan, M., McBeath, A. J., Hay, S. J., Pierce, G. J., and Cunningham, C. O. (2008). Real-time PCR assay for detection and relative quantification of Liocarcinus depurator larvae from plankton samples. Mar. Biol. 153, 859–870. doi: 10.1007/s00227-007-0858-y
Pasternak, A., Arashkevich, E., Riser, C. W., Ratkova, T., and Wassmann, P. (2000). Seasonal variation in zooplankton and suspended faecal pellets in the subarctic Norwegian Balsfjorden, in 1996. Sarsia 85, 439–452. doi: 10.1080/00364827.2000.10414593
Pereira, J. C., Chaves, R., Bastos, E., Leitão, A., and Guedes-Pinto, H. (2011). An efficient method for genomic DNA extraction from different molluscs species. Int. J. Mol. Sci. 12, 8086–8095. doi: 10.3390/ijms12118086
Persson, J., Stige, L. C., Stenseth, N. C., Usov, N., and Martynova, D. (2012). Scale-dependent effects of climate on two copepod species, Calanus glacialis and Pseudocalanus minutus, in an Arctic-boreal sea. Mar. Ecol. Prog. Ser. 468, 71–83. doi: 10.3354/meps09944
Pertsova, N. M., and Kosobokova, K. N. (2003). Zooplankton of the White Sea: features of the composition and structure, seasonal dynamics, and the contribution to the formation of matter fluxes. Oceanology 43, S108–S122.
Peters, J., Dutz, J., and Hagen, W. (2013). Trophodynamics and life-cycle strategies of the copepods Temora longicornis and Acartia longiremis in the Central Baltic Sea. J. Plankton Res. 35, 595–609. doi: 10.1093/plankt/fbt004
Peters, J., Renz, J., van Beusekom, J., Boersma, M., and Hagen, W. (2006). Trophodynamics and seasonal cycle of the copepod Pseudocalanus acuspes in the Central Baltic Sea (Bornholm Basin): evidence from lipid composition. Mar. Biol. 149, 1417–1429. doi: 10.1007/s00227-006-0290-8
Pond, D. W., and Tarling, G. A. (2011). Phase transitions of wax esters adjust buoyancy in diapausing Calanoides acutus. Limnol. Oceanogr. 56, 1310–1318. doi: 10.4319/lo.2011.56.4.1310
R Core Team (2020). R: A Language and Environment for Statistical Computing. Vienna: R Foundation for Statistical Computing.
Reigstad, M., and Wassmann, P. (1996). Importance of advection for pelagic-benthic coupling in north Norwegian fjords. Sarsia 80, 245–257. doi: 10.1080/00364827.1996.10413599
Renz, J., and Hirche, H.-J. (2006). Life cycle of Pseudocalanus acuspes Giesbrecht (Copepoda, Calanoida) in the Central Baltic Sea: I. Seasonal and spatial distribution. Mar. Biol. 148, 567–580. doi: 10.1007/s00227-005-0103-5
Rognes, T., Flouri, T., Nichols, B., Quince, C., and Mahé, F. (2016). VSEARCH: a versatile open source tool for metagenomics. PeerJ 4, e2584. doi: 10.7717/peerj.2584
Saiz, E., and Kiørboe, T. (1995). Predatory and suspension feeding of the copepod Acartia tonsa in turbulent environments. Mar. Ecol. Prog. Ser. 122, 147–158. doi: 10.3354/meps122147
Sandnes, O., and Gulliksen, B. (1980). Monitoring and manipulation of a sublittoral hard bottom biocoenosis in Balsfjord, northern Norway. Helgoländer Meeresuntersuchungen 33, 467–472. doi: 10.1007/BF02414771
Sargent, J. R., and Falk-Petersen, S. (1981). Ecological investigations on the zooplankton community in Balsfjorden, Northern Norway–lipids and fatty acids in Meganyctiphanes norvegica, Thysanoessa raschi and Thysanoessa inermis during mid-winter. Mar. Biol. 62, 131–137. doi: 10.1007/BF00388175
Schroeder, A., Stanković, D., Pallavicini, A., Gionechetti, F., Pansera, M., and Camatti, E. (2020). DNA metabarcoding and morphological analysis-assessment of zooplankton biodiversity in transitional waters. Mar. Environ. Res. 160:104946. doi: 10.1016/j.marenvres.2020.104946
Silberberger, M. J., Renaud, P. E., Espinasse, B., and Reiss, H. (2016). Spatial and temporal structure of the meroplankton community in a sub-Arctic shelf system. Mar. Ecol. Prog. Ser. 555, 79–93. doi: 10.3354/meps11818
Skreslet, S., Olsen, K., Chelak, M., and Eiane, K. (2015). NE Atlantic zooplankton wintering in fjord habitats responds to hemispheric climate. J. Plankton Res. 37, 773–789.
Stone, D. P. (1980). “The distribution of zooplankton communities in a glacial run-off fjord and exchanges with the open sea,” in Fjord Oceanography, eds H. J. Freeland, D. M. Farmer, and C. D. Levings (Boston, MA: Springer), 291–297.
Strand, E., Bagøien, E., Edwards, M., Broms, C., and Klevjer, T. (2020). Spatial distributions and seasonality of four Calanus species in the Northeast Atlantic. Prog. Oceanogr. 185:102344. doi: 10.1016/j.pocean.2020.102344
Stübner, E. I., Søreide, J. E., Reigstad, M., Marquardt, M., and Blachowiak-Samolyk, K. (2016). Year-round meroplankton dynamics in high-Arctic Svalbard. J. Plankton Res. 38, 522–536. doi: 10.1093/plankt/fbv124
Svensen, C., Antonsen, M. T., and Reigstad, M. (2018). Small copepods matter: population dynamics of Microsetella norvegica in a high-latitude coastal ecosystem. J. Plankton Res. 40, 446–457. doi: 10.1093/plankt/fby019
Svensen, C., Seuthe, L., Vasilyeva, Y., Pasternak, A., and Hansen, E. (2011). Zooplankton distribution across Fram Strait in autumn: are small copepods and protozooplankton important? Prog. Oceanogr. 91, 534–544. doi: 10.1016/j.pocean.2011.08.001
Tande, K., Hassel, A., and Slagstad, D. (1985). “Gonad maturation and possible life cycle strategies in Calanus finmarchicus and Calanus glacialis in the northwestern part of the Barents Sea,” in Marine Biology of Polar Regions and Effects of Stress on Marine Organisms, eds J. S. Gray and M. E. Christiansen (Chichester: Wiley), 141–155.
Tande, K., and Slagstad, D. (1982). Ecological investigation on the zooplankton community of Balsfjorden, Northern Norway- seasonal and short time variations in enzyme activity in copepodite stage V and VI males and females of Calanus finmarchicus (Gunnerus). Sarsia 67, 63–68. doi: 10.1080/00364827.1982.10421334
Tande, K. S. (1982). Ecological investigations on the zooplankton community of Balsfjorden, Northern Norway–generation cycles, and variations in body-weight and body content of carbon and nitrogen related to overwintering and reproduction in the copepod Calanus finmarchicus (Gunnerus). J. Exp. Mar. Biol. Ecol. 62, 129–142. doi: 10.1016/0022-0981(82)90087-9
Tande, K. S., and Bamstedt, U. (1987). On the trophic fate of Phaeocystis pouchetii 1. Copepod feeding rates on solitary cells and colonies of Phaeocystis pouchetii. Sarsia 72, 313–320. doi: 10.1080/00364827.1987.10419728
Tande, K. S., and Gronvik, S. (1983). Ecological investigations on the zooplankton community of Balsfjorden, Northern Norway–sex-ratio and gonad maturation cycle in the copepod metridia-longa (Lubbock). J. Exp. Mar. Biol. Ecol. 71, 43–54. doi: 10.1016/0022-0981(83)90103-X
Tande, K. S., and Hopkins, C. C. E. (1981). Ecological investigations of the zooplankton community of Balsfjorden, Northern Norway–the genital system in Calanus finmarchicus and the role of gonad development in overwintering strategy. Mar. Biol. 63, 159–164. doi: 10.1007/BF00406824
Taylor, A. H., Allen, J. I., and Clark, P. A. (2002). Extraction of a weak climatic signal by an ecosystem. Nature 416, 629–632. doi: 10.1038/416629a
Throndsen, J., Hasle, G. R., and Tangen, K. (2007). Phytoplankton of Norwegian Coastal Waters. Amsterdam: Elsevier.
Trudnowska, E., Stemmann, L., Błachowiak-Samołyk, K., and Kwasniewski, S. (2020). Taxonomic and size structures of zooplankton communities in the fjords along the Atlantic water passage to the Arctic. J. Mar. Syst. 204:103306. doi: 10.1016/j.jmarsys.2020.103306
Tseng, L.-C., Dahms, H.-U., Hung, J.-J., Chen, Q.-C., and Hwang, J.-S. (2011). Can different mesh sizes affect the results of copepod community studies? J. Exp. Mar. Biol. Ecol. 398, 47–55. doi: 10.1016/j.jembe.2010.12.007
Unal, E., Frost, B. W., Armbrust, V., and Kideys, A. E. (2006). Phylogeography of Calanus helgolandicus and the Black Sea copepod Calanus euxinus, with notes on Pseudocalanus elongatus (Copepoda, Calanoida). Deep Sea Res. 2 Top. Stud. Oceanogr. 53, 1961–1975. doi: 10.1016/j.dsr2.2006.03.017
Ussing, H. H. (1938). The biology of some important plankton animals in the fjords of east Greenland. Medd. Grønland 100, 1–108.
Vahl, O. (1980). Seasonal variatins in seston and in the growth rate of the iceland scallop, Chlamys islandica (O.F. Müller) from balsfjord, 70°N. J. Exp. Mar. Biol. Ecol. 48, 195–204. doi: 10.1016/0022-0981(80)90017-9
Varpe, Ø (2009). Stealing bivalves from common eiders: kleptoparasitism by glaucous gulls in spring. Polar Biol. 33, 359–365. doi: 10.1007/s00300-009-0712-4
Varpe, Ø, Jørgensen, C., Tarling, G. A., and Fiksen, Ø (2009). The adaptive value of energy storage and capital breeding in seasonal environments. Oikos 118, 363–370. doi: 10.1111/j.1600-0706.2008.17036.x
Vazyulya, S., Kopelevich, O. V., Sheberstov, S. V., Burenkov, V. I., and Khrapko, A. N. (2001). A method for estimating the penetration of solar visual and ultraviolet radiation into the waters of the Arctic seas using satellite data: the case of a cloudless atmosphere. Oceanology 41, 317–325.
Vinogradov, M., and Shushkina, E. (1987). Function of Plankton Communities in Epipelagic Zone of the Ocean. Moscow: Nauka Press.
Wangensteen, O. S., Palacín, C., Guardiola, M., and Turon, X. (2018). DNA metabarcoding of littoral hard-bottom communities: high diversity and database gaps revealed by two molecular markers. PeerJ 6:e4705. doi: 10.7717/peerj.4705
Wexels Riser, C., Reigstad, M., and Wassmann, P. (2010). Zooplankton-mediated carbon export: a seasonal study in a northern Norwegian fjord. Mar. Biol. Res. 6, 461–471. doi: 10.1080/17451000903437067
Weydmann, A., Søreide, J. E., Kwasniewski, S., Leu, E., Falk-Petersen, S., and Berge, J. (2013). Ice-related seasonality in zooplankton community composition in a high Arctic fjord. J. Plankton Res. 35, 831–842. doi: 10.1093/plankt/fbt031
Wiborg, K. F. (1954). Investigations on zooplankton in coastal and offshore waters of western and northwestern Norway. Fiskeridirektoratets Skrifter Serie Havundersøkelser 11, 1–246.
Wiborg, K. F. (1955). Zooplankton in relation to hydrography in the Norwegian Sea. Fiskeridirektoratets Skrifter Serie Havundersøkelser 11, 1–66.
Keywords: metabarcoding, sub-arctic, seasonal community dynamics, zooplankton, meroplankton, copepods
Citation: Coguiec E, Ershova EA, Daase M, Vonnahme TR, Wangensteen OS, Gradinger R, Præbel K and Berge J (2021) Seasonal Variability in the Zooplankton Community Structure in a Sub-Arctic Fjord as Revealed by Morphological and Molecular Approaches. Front. Mar. Sci. 8:705042. doi: 10.3389/fmars.2021.705042
Received: 04 May 2021; Accepted: 12 July 2021;
Published: 04 August 2021.
Edited by:
Ulisses Miranda Azeiteiro, University of Aveiro, PortugalReviewed by:
Vladimir G. Dvoretsky, Murmansk Marine Biological Institute, RussiaLeocadio Blanco-Bercial, Bermuda Institute of Ocean Sciences, Bermuda
Copyright © 2021 Coguiec, Ershova, Daase, Vonnahme, Wangensteen, Gradinger, Præbel and Berge. This is an open-access article distributed under the terms of the Creative Commons Attribution License (CC BY). The use, distribution or reproduction in other forums is permitted, provided the original author(s) and the copyright owner(s) are credited and that the original publication in this journal is cited, in accordance with accepted academic practice. No use, distribution or reproduction is permitted which does not comply with these terms.
*Correspondence: Estelle Coguiec, estelle.a.coguiec@uit.no
†These authors have contributed equally to this work