- 1Central Caribbean Marine Institute, Little Cayman, Cayman Islands
- 2Bermuda Institute of Ocean Sciences, St. George’s, Bermuda
- 3The Interuniversity Institute for Marine Sciences, Eilat, Israel
- 4Department of Marine Biology, Leon H. Charney School of Marine Sciences, University of Haifa, Haifa, Israel
- 5Department of Ecology and Evolutionary Biology, Yale University, New Haven, CT, United States
- 6Morris Kahn Marine Research Station, Leon H. Charney School of Marine Sciences, University of Haifa, Haifa, Israel
- 7Coral Ecophysiology Team, Centre Scientifique de Monaco, Monaco, Monaco
As the devastating impacts of global climate change and local anthropogenic stressors on shallow-water coral reefs are expected to rise, mesophotic coral ecosystems have increasingly been regarded as potential lifeboats for coral survival, providing a source of propagules to replenish shallower reefs. Yet, there is still limited knowledge of the capacity for coral larvae to adjust to light intensities that change with depth. This study elucidates the mechanisms underlying plasticity during early life stages of the coral Porites astreoides that enable survival across broad depth gradients. We examined physiological and morphological variations in larvae from shallow (8–10 m) and mesophotic (45 m) reefs in Bermuda, and evaluated differences in survival, settlement patterns and size among recruits depending on light conditions using a reciprocal ex situ transplantation experiment. Larvae released from mesophotic adults were found to have significantly lower respiration rates and were significantly larger than those derived from shallow adults, indicating higher content of energetic resources and suggesting a greater dispersal potential for mesophotic larvae compared to their shallow counterparts. Additionally, larvae released from mesophotic adults experienced higher settlement success and larger initial spat size compared to larvae from shallow adults, demonstrating a potential connection between parental origin, offspring quality, and recruitment success. Although both shallow and mesophotic larvae exhibited the capacity to survive and settle under reciprocal light conditions, all larvae had higher survival under mesophotic light conditions regardless of parental origin, suggesting that conditions experienced under low light may enable longer larval life, further extending the dispersal period. These results indicate that larvae from mesophotic Porites astreoides colonies are likely capable of reseeding shallow reefs in Bermuda, thereby supporting the Deep Reef Refugia Hypothesis.
Introduction
By creating complex 3-dimensional calcium carbonate frameworks, reef building corals support the most productive and biologically diverse marine ecosystems on Earth. Coral reefs are not only a critical habitat for a wide variety of species, but also provide indispensable ecosystem services for millions of people (Burke et al., 2011). Despite their great importance, coral reefs are facing significant challenges due to human activities (e.g., pollution, overfishing, and the physical destruction of reefs) (Burke et al., 2011). In addition, changes in environmental features determined by ocean warming and seawater acidification are seriously threatening the survival of entire coral reef ecosystems (Hoegh-Guldberg et al., 2017). Coral losses are particularly pronounced on shallow water reef systems, where corals and their dinoflagellate symbionts live close to their physiological thermal maximum, and as a result, warming of 1°C or more can significantly reduce their fitness and cause whole-colony mortality (Hoegh-Guldberg, 1999; Jones, 2008). However, coral reefs extend far beyond the well-studied shallow reef zones into Mesophotic Coral Ecosystems (MCEs) ranging from 30 m depth down to depths potentially greater than 170 m (Hinderstein et al., 2010; Rouzé et al., 2021). In the last few years, MCEs have gained significant interest as they appear not to have experienced the same trend in coral decline as their shallow-water counterparts, displaying relatively stable coral populations over time (Bak et al., 2005). Thus, it is hypothesized that MCEs may act as refugia for corals, serving as potential sources to reseed shallower reefs through larval exchange and thereby increasing overall reef resilience [termed the Deep Reef Refugia Hypothesis “DRRH”; (Bongaerts et al., 2010; Lesser et al., 2018)].
Resilience of ecosystems under rapid environmental change relies, in part, on the capacity of organisms to adapt and/or acclimatize to new conditions. From shallow to mesophotic depths, wide variations in light intensity shape different physiological, morphological and gene expression patterns in both the coral and the algal symbiont (Mass et al., 2007; Einbinder et al., 2016; Goodbody-Gringley and Waletich, 2018; Martinez et al., 2020; Malik et al., 2021). For instance, differences in photosynthetic efficiency have been observed in the algal endosymbionts between shallow and mesophotic corals (Bongaerts et al., 2011). Moreover, coral larvae and recruits from different light environments significantly differ in size (Turner et al., 2018; Scucchia et al., 2020) and possess differential recruitment patterns (Turner et al., 2018). These wide variations among conspecifics are recurrently suggested to result from plasticity in response to differing environmental conditions at depth, such as light intensity changes (Goodbody-Gringley and Waletich, 2018). Nevertheless, there is still limited knowledge of the physiological and morphological contributors that underlie such plasticity, particularly in early life stages. This reduces our capacity to adequately assess the ability of mesophotic larvae to survive under higher light intensities and replenish shallower reefs.
If MCEs are in fact an important lifeboat for coral survival, it is imperative to evaluate the degree of physiological plasticity of coral larvae that would enable them to successfully migrate and settle across wide depth gradients, as successful settlement is fundamental to coral population dynamics as well as reef community structure (Piniak et al., 2005). We assessed this plasticity in the reef-building coral Porites astreoides, a hermaphroditic, brooding species (Brazeau et al., 1998) that inhabits large environmental clines (Elizalde-Rendón et al., 2010) and exhibits high phenotypic plasticity at various life stages (Green et al., 2008). This Atlantic coral is common throughout the Caribbean and Western Atlantic with a broad depth distribution, ranging from 0 to 50 m (Fricke and Meischner, 1985; Chornesky and Peters, 1987). In Bermuda, P. astreoides is a main contributor to reef community structure and is ubiquitously distributed across the lagoon and rim reef zones, with relative frequency decreasing with increasing depth beyond 20 m (Fricke and Meischner, 1985; de Putron and Smith, 2011; Goodbody-Gringley et al., 2019). Due to the high levels of fecundity and settlement success, P. astreoides is considered a resilient species (Bak and Engel, 1979; Chornesky and Peters, 1987). Additionally, variable bleaching responses and different host gene expression patterns between inshore and offshore populations exposed to heat stress suggest that P. astreoides may be able to acclimatize or adapt to thermal stress (Kenkel et al., 2013a,b; Wong et al., 2021). Across a depth gradient, this species was also found to have similar thermal tolerance ranges, despite variable thermal histories, suggesting high levels of plasticity to temperature (Gould et al., 2021). Consequently, P. astreoides is expected to increase in relative abundance compared to other Atlantic scleractinian species (Green et al., 2008), and is therefore an ideal study species as it will likely be an important contributor to the future community composition of Caribbean and Western Atlantic reefs. Importantly, this species was also found to maintain high degrees of genetic connectivity in Bermuda across inshore and offshore sites that ranged in depth from 4 to 26 m (Serrano et al., 2016), and multiple studies have described Symbiodinium spp. [ITS2 type A4; (LaJeunesse et al., 2018)] as the dominant symbiont in P. astreoides in Bermuda (Venn et al., 2006; Serrano et al., 2016; Zhang et al., 2016; Reich et al., 2017). Thus, there is strong support for host and symbiont homogeneity in Bermuda across depths enabling comparisons of phenotypic response to environmental conditions.
In this study, we examined physiological and morphological characteristics of P. astreoides larvae released from shallow (8–10 m) and mesophotic (45 m) adults and, through a reciprocal ex situ transplantation experiment, we assessed differences in survival, settlement patterns and size among recruits depending on settlement light conditions. With this approach, we elucidated the mechanisms underlying the plasticity of P. astreoides early life stages that enables them to survive across broad depth gradients. The existence of such plasticity increases the probability of effective connectivity between shallow and mesophotic depths, ultimately advancing our understanding of these ecosystems and the potential for MCEs to serve as refugia.
Materials and Methods
Site Selection
Two study sites on the north Atlantic Bermuda platform were selected for this study: a rim reef (Hog Shallow; 8–10 m depth; 32°27′26″N, 64°50′05″W), and a mesophotic reef (Hog Deep; 45 m depth; 32°29′18″N, 64°51′18″W) (Figure 1). Mesophotic (∼45 m) Bermudian coral reefs have been characterized as a light and nutrient-limited environment with colder temperatures compared to shallow reefs (Goodbody-Gringley et al., 2015). Temperature (°C) was measured from early June 2019 through mid August 2019 using HOBO water temperature ProV2 loggers deployed on the benthos at 10, 30, 45, and 60 m. Temperatures were found to be consistently lower at deeper depths throughout the summer, but converged toward the peak of the summer (Figure 1). Maximum temperature at the shallow site (10 m) peaked at 28.9°C, compared to 26.8°C at the mesophotic site (45 m).
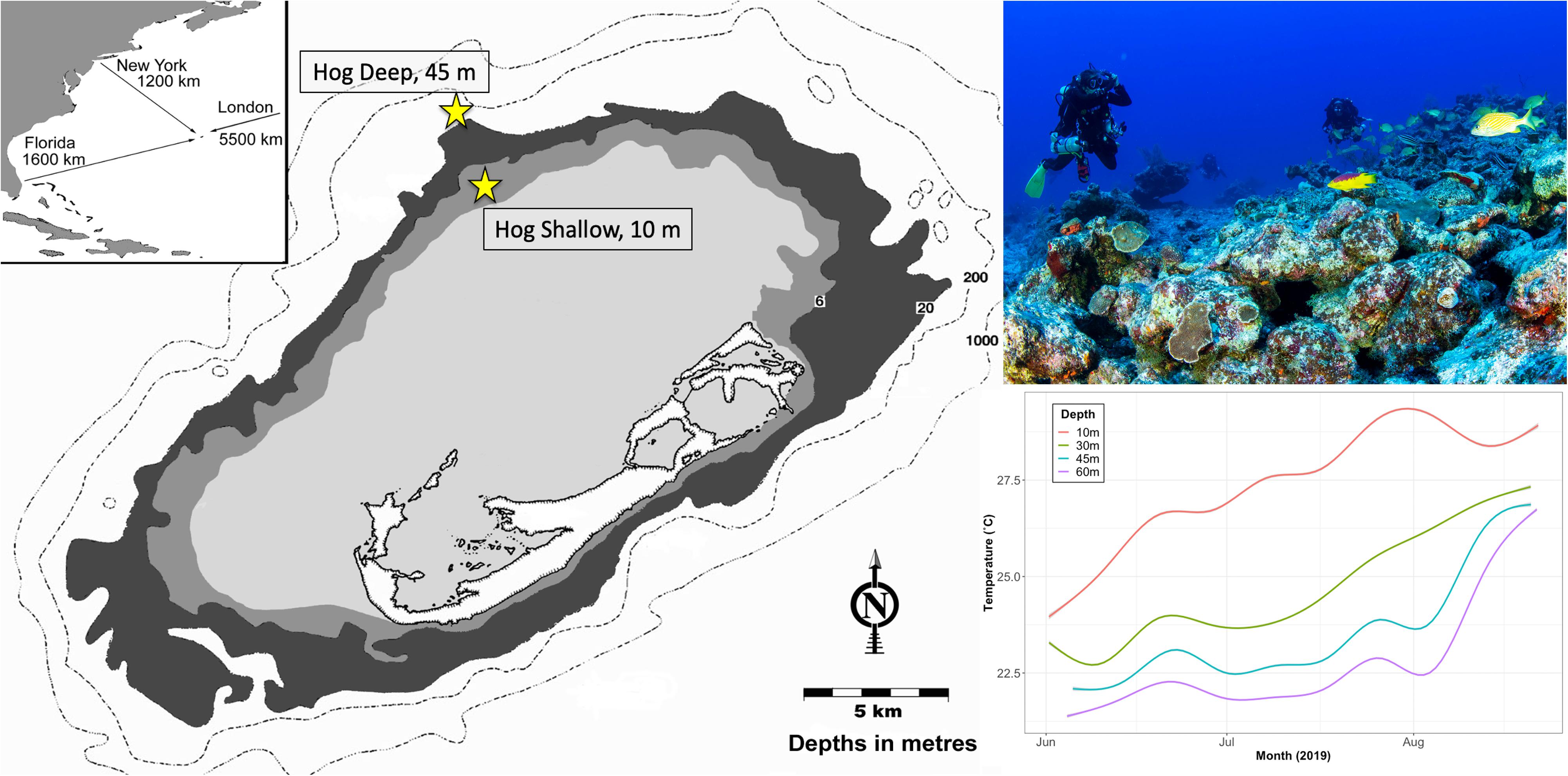
Figure 1. Map of Bermuda indicating its’ location within the Western Atlantic (inset) as well as the sample sites on the northern edge of the platform (image left, yellow symbol). Image of team collecting adult coral colonies from the reef site at 45 m depth using closed-circuit rebreathers (top right; Image by HN). In situ mean seawater temperature (°C) measured with a HOBO water temperature ProV2 logger deployed on the benthos at 10, 30, 45, and 60 m depth from June to August 2019. Shaded area represents 95% confidence intervals (bottom right). Asterisk indicates significant difference.
Larval Collection
The reproductive timing of P. astreoides has been relatively well studied in Bermuda. This brooding species is capable of both self-fertilization and outcrossing (Brazeau et al., 1998) and releases symbiotic larvae in accordance with the lunar cycle (de Putron and Smith, 2011). In Bermuda, the planula release period of P. astreoides occurs over several days beginning up to 10 days before the new moons of July, August, and September (de Putron and Smith, 2011), enabling predictable larval collections.
A total of 20 adult colonies with a minimum surface area of 170 cm2 were randomly chosen and collected for this experiment using a hammer and chisel (PSLicenses #2019061407): 10 from the shallow reef (10 m depth) and 10 from the mesophotic reef (45 m depth; Figure 1), 9 days prior to the July new moon (22 July 2019). Any minimal differences in surface area are not expected to affect reproductive patterns, as it has been shown that there is no significant relationship between numbers of planulae released and the surface areas of reproductive colonies of P. astreoides in Bermuda (de Putron and Smith, 2011).
Adult corals were transferred to the outdoor mesocosm at the Bermuda Institute of Ocean Sciences (BIOS) where they were maintained in 400-L flow tanks under ambient temperature conditions. Temperature was recorded in the mesocosm tanks daily using a HOBO onset data logger on 1-min intervals (27.6°C ± 0.004, shallow; 27.5°C ± 0.005, mesophotic). To reduce light stress and maintain temperature controls, water level heights of ∼45 cm above the colony surface were maintained during the day. All corals experienced a natural light period. Mesophotic colonies were covered with a single layer of light filter (Lagoon Blue, ©LEE Filters) to mimic mesophotic light levels, while shallow colonies were left uncovered. Daily light readings using a PME miniPAR logger were recorded for each condition between 12:00 and 13:00 over the period that corals were in the mesocosm (10 days; Table 1). In situ light measurements from the collection sites were taken every 30 s for a period of 3–4 min between 12:00 and 13:00 at depths of 10 m and 45 m on the day of coral collections using a Li-COR sensor on a stable frame with a 100 m fiber optic cable (Table 1).

Table 1. Mean PAR (μmol/s m2) readings taken on in situ drops using a Li-COR PAR sensor at 10 m and 45 m, and ex situ in the mesocosm using a PME miniPAR logger in unshaded and light-filtered conditions.
Larval collections occurred from July 24 to July 26, 2019. During the larval collection period, tank water levels were dropped at night and individual colonies were placed into 2-L plastic separated jugs with individual lines of flowing seawater. An 800 mL polypropylene beaker with a 153 μm mesh bottom was placed under the spout of each container to collect the positively buoyant larvae following the methods of Goodbody-Gringley et al. (2018). Every morning, all larvae released from each colony were collected with a clear transfer pipette and pooled by reef depth. On the final day of larval collections (July 26, 2019), subsamples of the pooled larvae were used to quantify larval volume, respiration, and settlement success. By pooling larvae across the 3 collection days, variations in parameters were only assessed at the population level and thus the effects of individual colony or lunar day of release were not evaluated in this study.
Larval Sizing
Larvae from samples pooled by depth were randomly sub-sampled (n = 41, shallow; n = 19 mesophotic) and digitally photographed from a side view (elliptical in shape) using a MicroViewer camera (Mighty Scope 5M, Aven Tools). Larval volume was determined by measuring the length and width of the longitudinal and transverse axes in ImageJ and applying the equation for volume of an elliptical sphere, V = 4/3 πab2, where a is 1/2 the width and b is 1/2 the length (Isomura and Nishihira, 2001). Larval volumes met the assumptions of normality (Shapiro–Wilk’s test) and homogeneity (Levene’s test) and were compared by parental depth using an Analysis of Variance (ANOVA) followed by a Tukey’s HSD post hoc test performed in RStudio v1.2.5001 (RStudio Team, 2019) using the “aov” function in the package “car” (Fox and Weisberg, 2019).
Larval Respiration
Larval respiration was quantified using a sensor dish reader (SDR) and 24 80 μL-well microplate system (Loligo Systems) and recorded using PreSens-SDR software. Ten wells were filled with planulae from mesophotic corals (n = 30 larvae, 3 per well), ten wells were filled with planulae from rim corals (n = 40 larvae, 4 per well), and four wells were filled with filtered seawater to be used as blanks. One blank well was also used to monitor temperature with a Thermocoupler probe (Fluke 80PJ-1 Bead Probe). Due to observed differences in larval size, fewer mesophotic larvae were used per well to allow for adequate swimming space, prevent rapid oxygen depletion and standardize total larval volume within each well. The wells were sealed with Parafilm and the entire SDR system was placed in an incubator set to 27°C. Oxygen concentration and internal plate temperature were recorded every 15 s over a 20-min trial. Following the respiration trials, larvae were fixed in 10% formalin and volume (mm3) was determined using the methods described above. The sum larval volume was then calculated for each microplate well.
Raw data from respirometry trials were filtered to remove oxygen concentration measurements below 70% air saturation. Wells with less than 4 min of data were discarded. Respiration rate for each plate well was determined using a least squares linear regression of oxygen concentration against time in R v.4.0.2 (R Core Team, 2020) with the package “LoLinR” (v0.0.0.9000; Olito et al., 2017). Sample respiration rates were normalized using the mean blank rate. Rates were calculated per unit of larval volume (nmol O2 min–1 mm–3) rather than per larva as individual larval size differed significantly between the two depths. Several previous studies have normalized respiration to number of larvae (Edmunds et al., 2011; Cumbo et al., 2013; Serrano et al., 2018) rather than larval volume, however, these studies were not comparing larvae from different adult cohorts that differed significantly in size. Alternatively, Putnam and Gates (2015) compared size-normalized respiration rates of larvae from parental colonies with differing thermal histories due to differences in larval size between treatments. Metabolic rates are documented to correlate directly with larval size in other marine invertebrates (Manahan, 1990; Pan et al., 2016), and thus, while it is possible that our approach to normalize respiration to larval volume may have biased our results, significant differences in larval volume between depths suggests that normalization to organism size provides a more accurate comparison of physiological traits for these data.
Larval respiration data were assessed for normality and variance homogeneity using the Shapiro–Wilk test and Levene’s F-test to ensure t-test assumptions had been met. Group means were compared using a two-sample t-test.
Settlement Success
A total of 60 mesophotic larvae and 222 shallow larvae were randomly sub-sampled and used for ex situ settlement experiments. The sub-sampled larvae from each reef site were transferred into individual 0.5-L plastic settlement chambers containing two plastic settlement plugs (n = 37 shallow; n = 10 mesophotic, larvae per chamber). Plugs were preconditioned on an inshore patch reef for approximately 1 month prior to use to allow them to acquire a mixed cover of calcareous algae, bacterial communities and other organisms. Three replicate chambers from each origin depth were placed under each ex situ light condition (unshaded shallow vs. light filtered mesophotic; Table 1). Settlement chamber design followed Goodbody-Gringley (2010), where containers were covered with 150 μm mesh to facilitate water flow but prevent larvae from escaping. Settlement containers were randomly placed in flow-through filtered seawater tanks on an outdoor wet-bench and kept fully submerged for the duration of the experiment. After 72 h, the settlement containers were removed and percent settlement was determined using a dissecting microscope. Settlement was defined as the metamorphic transformation from planula to primary polyp (appearance of primary septa) as in Goodbody-Gringley (2010). Larval settlement and survival met the assumption of normality and variance homogeneity (Shapiro–Wilk test and Levene’s F-test, α = 0.05), and were compared using a two-way ANOVA for the interactive effects of parental origin and settlement light conditions followed by a Tukey’s HSD post hoc test. Statistical analyses were performed in RStudio v1.1.423 (R Development Team) using the “aov” function in the package “car” (Fox and Weisberg, 2019).
Results
Larval Physiology
Mean larval volume differed significantly by site of parental origin (p < 0.001; ANOVA), where larvae released by colonies originating from the mesophotic reef site were larger than those released from colonies originating from the shallow reef site (Figure 2). Rate of respiration also differed significantly between experimental treatments (p < 0.001; ANOVA), where respiration rates based on total larval volume were higher for wells containing larvae released by shallow colonies compared to those released by mesophotic colonies (Figure 3).
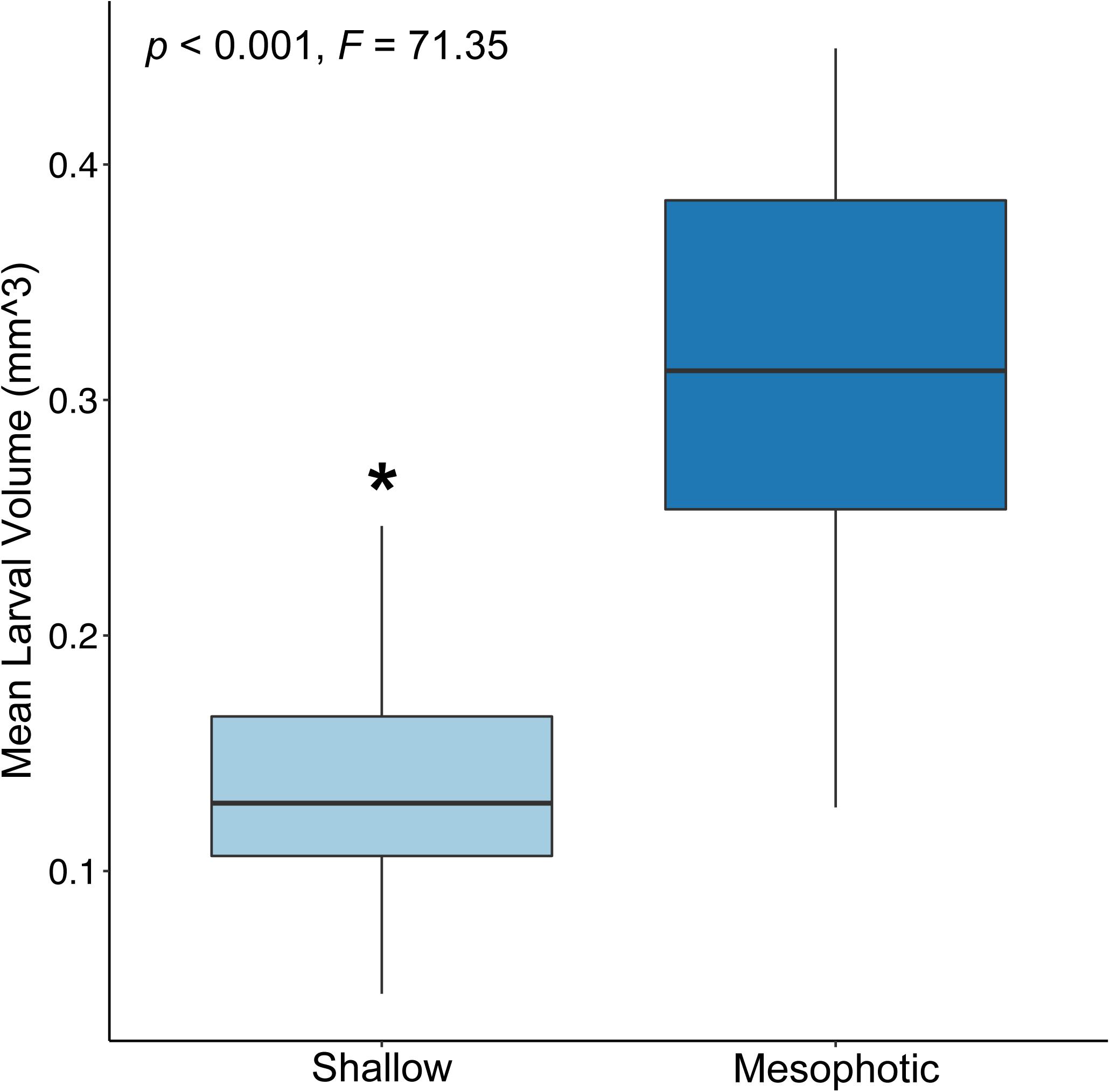
Figure 2. Box plot of the volume (mm3) of larvae released by shallow (light blue) and mesophotic (dark blue) adult Porites astreoides colonies (p < 0.001; ANOVA). The solid horizontal bars correspond to median values, the upper and lower sections (box outlines) correspond to the first and third quartiles, the whiskers extend to the highest and lowest values within 1.5 times the inter-quartile range. Asterisk indicates significant difference (α = 0.05).
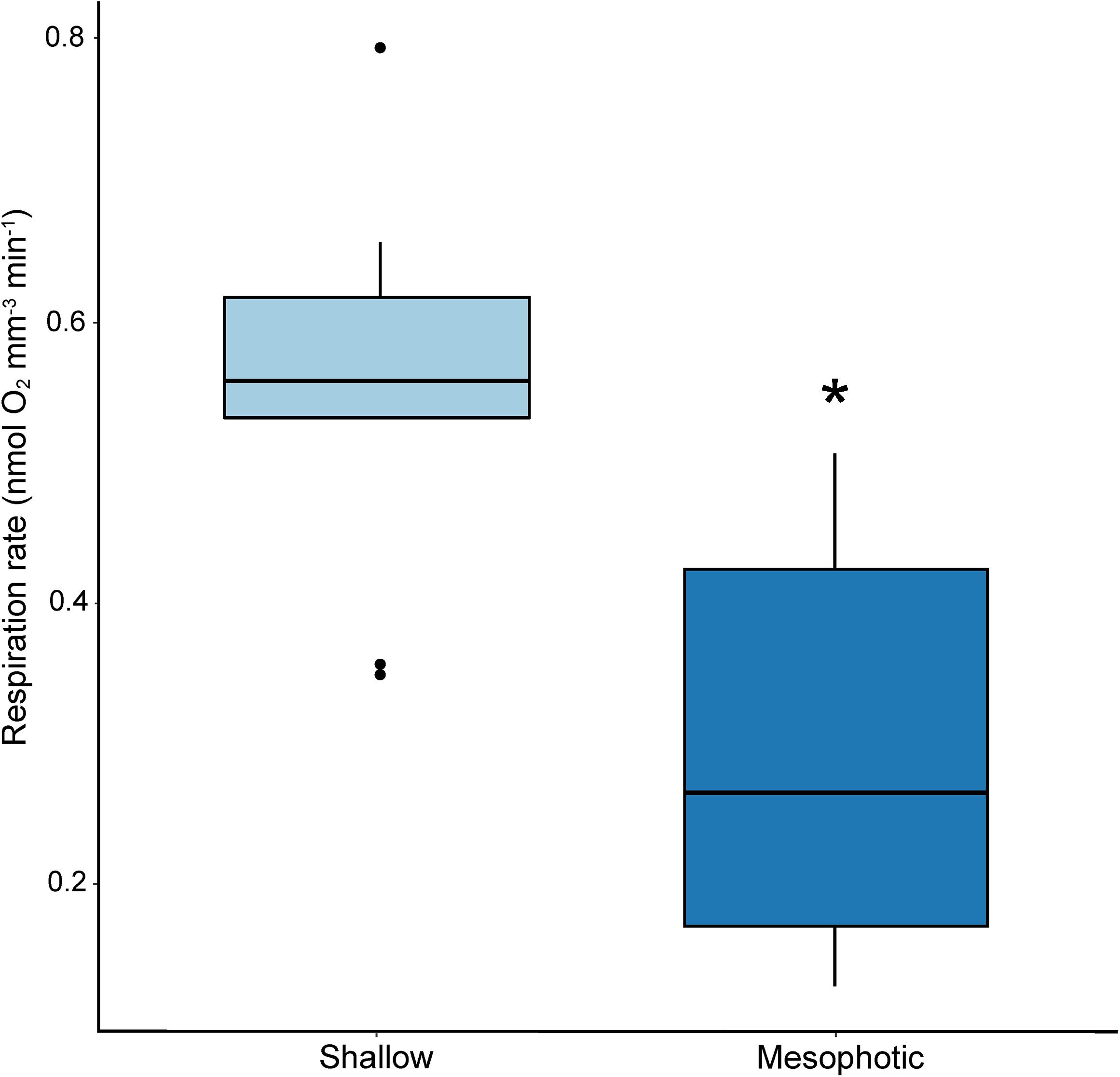
Figure 3. Box plot of respiration rates for larvae released by shallow (light blue) and mesophotic (dark blue) adult Porites astreoides colonies (p < 0.001; ANOVA). The solid horizontal bars correspond to median values, the upper and lower sections (box outlines) correspond to the first and third quartiles, the whiskers extend to the highest and lowest values within 1.5 times the inter-quartile range. Asterisk indicates significant difference (α = 0.05).
Larval Settlement and Survival
Settlement success differed significantly by depth of parental origin (p = 0.007; ANOVA), where percent of settlement success relative to total number of larvae per chamber was higher in chambers containing larvae released by mesophotic corals than those released by shallow corals. Settlement success did not differ between settlement light conditions (Figure 4A; p = 0.117; ANOVA), and there was no significant effect of the interaction of parental depth and settlement light conditions on settlement success (p = 0.123; ANOVA).
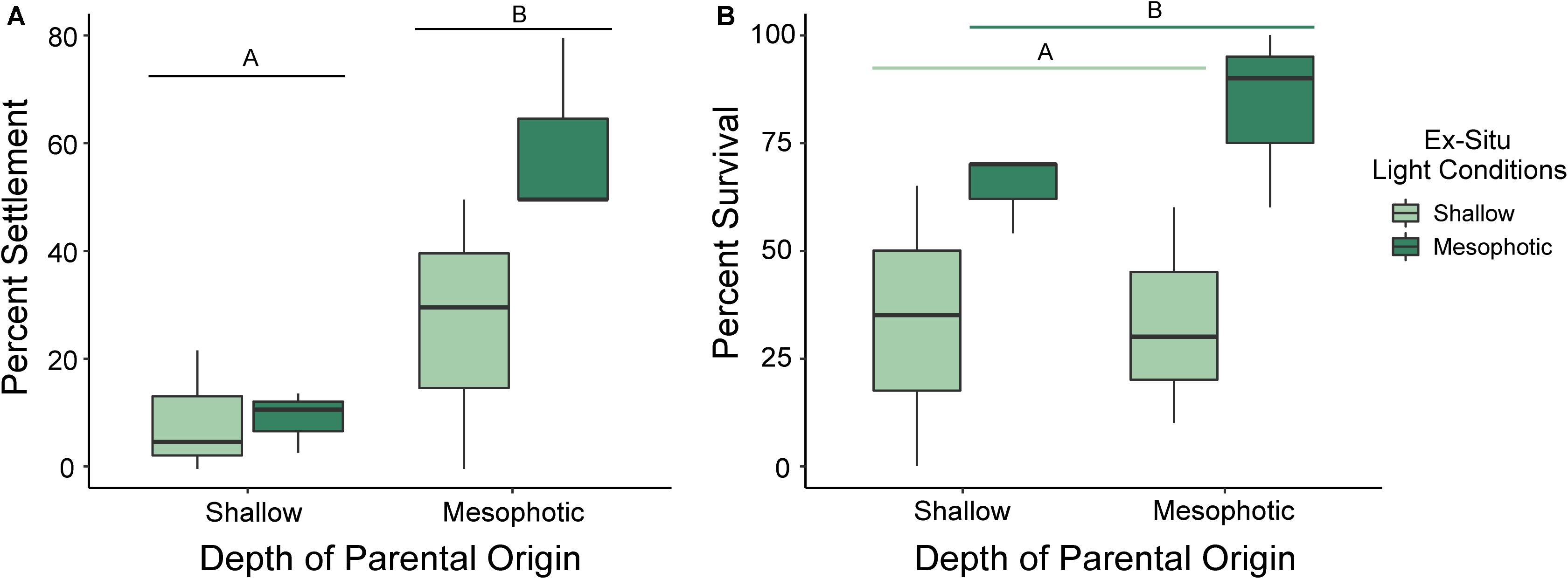
Figure 4. Box plots of (A) settlement success and (B) survival under shallow (no shade) and mesophotic (Lagoon Blue Filter, ©Lee Filters) light conditions for larvae released by adult Porites astreoides colonies originating from shallow (10 m) and mesophotic (45 m) reefs. The solid horizontal bars correspond to median values, the upper and lower sections (box outlines) correspond to the first and third quartiles, the whiskers extend to the highest and lowest values within 1.5 times the inter-quartile range. Significant differences are designated by letters (α = 0.05).
Larval survival, which includes settled spat and swimming larvae, did not differ significantly by depth of parental origin (p = 0.511; ANOVA) but did differ significantly by settlement light conditions (p = 0.017; ANOVA). However, there was no effect of the interaction of parental depth and settlement light conditions on larval survival (Figure 4B; p = 0.408; ANOVA). Larvae settled under mesophotic light conditions had higher survival than those settled under shallow light conditions, regardless of parental origin.
Juvenile Size
Mean surface area of newly settled spat differed significantly based on depth of parental origin (p < 0.001; ANOVA), where juvenile spat originating from mesophotic parental colonies were larger than those from shallow colonies (Figure 5). Mean surface area of newly settled spat also differed significantly by settled light conditions (p < 0.001; ANOVA), where juvenile spat settled under mesophotic reef light conditions were larger than those settled under shallow reef light conditions. The interaction of parental depth and settlement light conditions did not significantly affect juvenile size (p = 0.684).
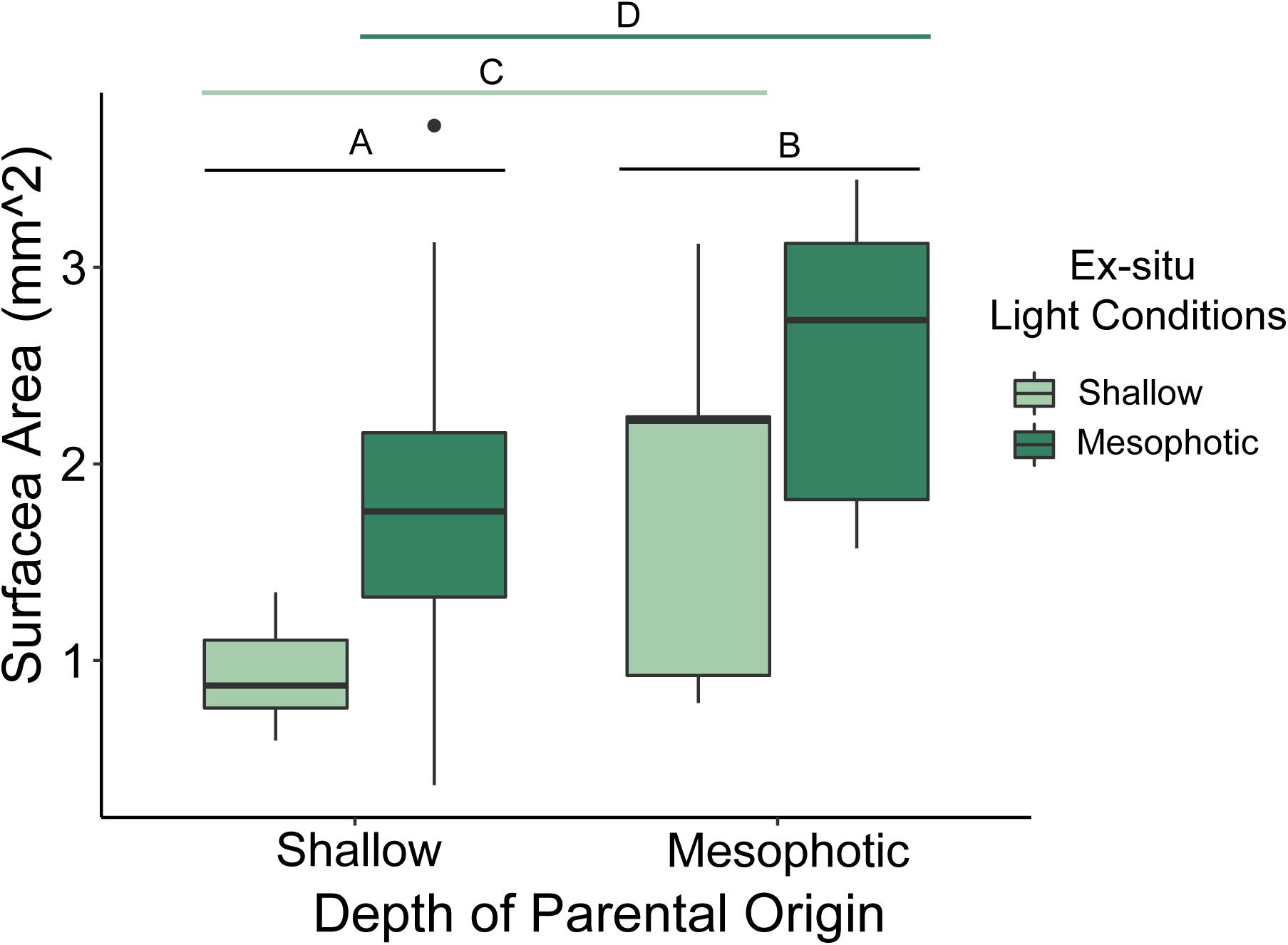
Figure 5. Box plot of juvenile spat surface area (mm2) originating from larvae released by shallow and mesophotic adult Porites astreoides colonies and settled under ex situ shallow and mesophotic light conditions. The solid horizontal bars correspond to median values, the upper and lower sections (box outlines) correspond to the first and third quartiles, the whiskers extend to the highest and lowest values within 1.5 times the inter-quartile range. Significant differences are designated by letters (α = 0.05).
Discussion
The persistence of coral reefs under global environmental change is built upon larval survival and settlement success. Evaluating the phenotypic diversity and plasticity of coral larvae is fundamental to understand their range of environmental tolerance. P. astreoides larvae originating from shallow and mesophotic corals show clear differences in physiological and morphological traits, likely stemming from adaptation to different light environments experienced during the brooding period. Larvae released from mesophotic colonies have significantly lower respiration rates per unit biomass than those derived from shallow colonies (Figure 3). Decreased coral respiration rate appears to be linked to lower availability of photosynthetically fixed carbon at light-limited mesophotic depths (McCloskey et al., 1984). Low metabolic rates of coral larvae are proposed to significantly extend dispersal potential, enhancing population connectivity (Graham et al., 2013). Likewise, a larger larval size allows for longer planktonic periods, enabling coral larvae to disperse farther (Isomura and Nishihira, 2001; Hartmann et al., 2013; Chamberland et al., 2017). In this study, larvae released from mesophotic colonies were found to be significantly larger than those released from shallow colonies (Figure 2), which suggests higher content of energetic resources in larvae of mesophotic origin, as larval size often correlates with energetic content in the form of maternally derived lipids (Wendt, 2000; Hartmann et al., 2013; de Putron et al., 2017). Active swimming represents the major energetic cost during marine invertebrates’ larval stages (Marshall and Keough, 2003), and in coral larvae this energy may be acquired from lipid catabolism (Harii et al., 2007). In addition, lipids within coral larvae are used for buoyancy and as an energy source to sustain longer periods in the plankton (Harii et al., 2007; Figueiredo et al., 2012). Considering that phytoplankton availability and light-dependent productivity markedly decrease with depth (Lesser et al., 2010), a high content of maternally derived lipid stores could significantly support metabolic requirements of mesophotic larvae. Collectively, these observations suggest that P. astreoides larvae of mesophotic origin possess a greater dispersal potential compared to their shallow counterparts. In fact, as previously hypothesized for P. astreoides larvae in Bermuda, the smaller size of shallow larvae may infer a shortage of energetic reserves, which could lead to lower rates of competency and survival (de Putron et al., 2017; Goodbody-Gringley et al., 2018). However, P. astreoides larvae acquire Symbiodinium through vertical transmission, enabling direct translocation of photosynthate as an energy source that can likely sustain the higher metabolic rates of shallow larvae (Putnam and Gates, 2015). Further examinations of larval lipid content and stable isotopes to determine rates of reliance on heterotrophy versus autotrophy in larvae from differing parental depths may help elucidate variations in larval duration and dispersal potential.
Larvae in the water column can change buoyancy in response to light intensity, in order to maximize the chance of finding the substratum at the optimal depth for recruitment (Mundy and Babcock, 1998). This indicates that the light environment varying with depth is a crucial element controlling the survival and successful settlement of coral larvae. In this study, P. astreoides larvae of both shallow and mesophotic origin exhibit the potential to adjust to wide differences in light intensity, by displaying the capacity to survive and successfully settle under reciprocal light settlement conditions (Figure 4). Furthermore, their phenotypic plasticity allowed larvae of both shallow and mesophotic parental origin to develop differential juvenile size based on the light environment encountered upon settlement (Figure 5).
Coral depth distribution largely correlates with light gradients and is highly influenced by the differing light tolerance and photosynthetic properties of their endosymbiotic algae (Iglesias-Prieto et al., 2004; LaJeunesse et al., 2004). Previous studies on the same species in Bermuda found that while densities of zooxanthellae cells within larvae of upper-mesophotic origin (30 m) were higher than those of shallow inshore patch reef origin (5 m), zooxanthellae densities did not differ between larvae from upper-mesophotic and rim reef origin (10 m) (Goodbody-Gringley et al., 2018). The ability of P. astreoides larvae to successfully adjust to differing light environments observed in this study, therefore, might stem from the endosymbionts’ photo-acclimatory potential. In fact, corals hosting symbionts in the genus Symbiodinium (formerly clade A; LaJeunesse et al., 2018), which is the dominant type in P. astreoides in Bermuda across depths (Venn et al., 2006; Serrano et al., 2016; Zhang et al., 2016; Reich et al., 2017), have been shown to possess high resistance to light variations (Reynolds et al., 2008) and to be less susceptible to bleaching (Warner et al., 1999). In addition, Symbiodinium spp. symbionts abundantly produce UV-protective amino acids that prevent damage to the photosynthetic apparatus due to changes in light intensity (Banaszak et al., 2006). The colonization of a wide range of light environments may not only be determined by the endosymbionts’ photosynthetic plasticity, but also from the ability of corals to modulate their tissue light field by either filtering out excess light that can be harmful to the algae (i.e., in high-light conditions), or by increasing the photon flux reaching the algae (i.e., in low-light conditions) (Lichtenberg et al., 2016). As such, a combination of host-symbiont phenotypic plasticity might be at the root of the capacity of P. astreoides larvae to colonize highly different light environments.
Overall, low light conditions appear to promote both higher survival and settlement of P. astreoides larvae (Figure 4). Under shallow light conditions, larvae from shallow adults experienced lower survival and settlement success compared to those from mesophotic adults, in accordance with previous observations by Goodbody-Gringley et al. (2018). Coral recruitment is known to be influenced by several physical characteristics varying with depth, with light representing one of the most critical factors (Babcock and Mundy, 1996; Mundy and Babcock, 1998). Sufficient light quality and intensity are required for photosynthesis, however, at shallow depths, light levels may be too high for young coral settlers (Gleason and Hofmann, 2011) leading to settlement in more cryptic sites (Babcock and Mundy, 1996). In addition, larval settlement is significantly influenced by recruit density, with fewer larvae settling in preferred locations as density increases (Cameron and Harrison, 2020). Thus, if larval densities are too high, cryptic and shaded space availability might be limited, leading to fewer larvae settling. As such, the higher density of larvae in the shallow settlement chambers may have negatively affected settlement success. However, this is unlikely as previous experiments using similarly sized chambers and available substrate with equal larval densities found analagous results, with higher settlement by upper-mesophotic larvae compared to shallow larvae (Goodbody-Gringley et al., 2018). Larval settlement is also documented to be driven in part by the benthic community, specifically the presence of crustose coralline algae (Morse et al., 1988; Ritson-Williams et al., 2009) and a bacterial film (Sneed et al., 2014). Shlesinger and Loya (2021) found that substrate type significantly influenced settlement choice by shallow and mesophotic larvae in the Red Sea, where larvae preferentially settled onto tiles conditioned at the corresponding depth of parental origin. Settlement tiles used in the present study, however, were all conditioned in a common garden environment on a nearshore shallow patch reef where conditions did not directly correspond to either site of parental origin, thereby reducing potential effects associated with tile conditioning.
Recruitment success of lecithotrophic coral larvae is largely influenced by larval size, with smaller larvae (i.e., less energetic reserves) translating to lower competency and survival (de Putron et al., 2017), and lower recruit sizes leading to decreased post-settlement survivorship (Raymundo and Maypa, 2004). Collectively, our results indicate that offspring quality and settlement success are deeply interconnected, and show an increase in larval fitness with increasing reef depth and decreasing light. These results suggest that differential quality of larvae released from shallow and mesophotic adults may be related to latent effects of parental history. For many animals, the environment experienced by parents influences the phenotype and fitness of their offspring, in a process known as “parental effects” (Marshall, 2008; Badyaev and Uller, 2009; Torda et al., 2017). Such parental effects influence the development of coral larvae and the recruits’ fitness (Dixon et al., 2015; Kenkel et al., 2015; Quigley et al., 2016; Putnam et al., 2020; Wong et al., 2021). Therefore, the increased performance of mesophotic offspring observed in this study may be due to the higher stability of environmental conditions experienced by mesophotic parental colonies during the brooding period, compared to consistently higher temperatures experienced by the adults on shallow reefs (Figure 1; Goodbody-Gringley et al., 2018). Furthermore, shallow reefs in Bermuda are more likely impacted by anthropogenic activities (Smith et al., 2013), which can also reduce the fitness of shallow adult colonies. Parental effects have been indicated as major factors limiting coral larval dispersal across depths as larvae may be adapted to the conditions experienced by their parent (Shlesinger and Loya, 2021), however, our findings show that offspring plasticity also plays a crucial role in shaping coral capacity to colonize differing environments. Yet, the density of adult P. astreoides colonies on shallow and mesophotic reefs in Bermuda does not reflect a pattern of increased recruitment to mesophotic depth, as adult colony frequency is documented to decrease with increasing depth (Fricke and Meischner, 1985). This apparent disconnect may be related to post-recruitment mortality and the adaptive potential of individual settlers, which were not assessed in this study. Future studies that follow post-recruitment survival in situ and examine variations in genomic responses through early life history stages at differing depths may better elucidate the mechanisms underlying these discrepancies and potential influences of parental effects.
A greater dispersal potential combined with the ability to survive and settle under a range of light conditions indicates that P. astreoides larvae from mesophotic reefs are likely capable of reseeding shallow reefs in Bermuda, thereby supporting the Deep Reef Refugia Hypothesis. Importantly, the higher settlement success of mesophotic larvae under shallow light conditions compared to shallow larvae under mesophotic light conditions suggests that recruitment may preferentially occur up the slope (mesophotic to shallow) rather than down the slope (shallow to mesophotic). These results oppose previous observations of asymmetric gene flow (Prada and Hellberg, 2013; Serrano et al., 2014; Bongaerts et al., 2017) and larval settlement patterns (Shlesinger and Loya, 2021) occurring from shallow to deeper reefs. Nevertheless, the higher reproductive success observed for mesophotic corals in the Western Atlantic (Holstein et al., 2015; Goodbody-Gringley et al., 2018) and subsequent larval effective recruitment found in the present study suggest that mesophotic reefs likely represent a significant source of propagules across the depth gradient and may aid in the recovery of degraded shallow reefs in Bermuda. Thus, mesophotic corals appear to be important contributors to the resilience of Bermuda’s reef ecosystems, which highlights the importance of establishing management strategies aimed at protecting mesophotic reefs. Future research assessing larval dispersal potential and recruitment success in situ is warranted to provide accurate data on population dynamics for mesophotic and shallow corals. Such studies will inform effective management of the ecological functions and ecosystem services provided by connected coral reef communities.
Data Availability Statement
The raw data supporting the conclusions of this article will be made available by the authors, without undue reservation.
Author Contributions
GG-G and TM designed and conducted the research. HN, GG-G, SM, SE, AC, and TM performed the underwater collection and ex situ experiments. RJ carried out the respiration experiments. GG-G, FS, and TM wrote the manuscript. All authors contributed to improving, revision, and approval of the manuscript.
Funding
This project received funding from the United States National Science Foundation and United States – Israel Binational Science Foundation (NSF #1937770 to GG-G and BSF #2019653 to TM), a Grant-in-Aid award from BIOS, and the BIOS REU program from the National Science Foundation’s Diversity of Ocean Sciences (NSF #1757475).
Conflict of Interest
The authors declare that the research was conducted in the absence of any commercial or financial relationships that could be construed as a potential conflict of interest.
Publisher’s Note
All claims expressed in this article are solely those of the authors and do not necessarily represent those of their affiliated organizations, or those of the publisher, the editors and the reviewers. Any product that may be evaluated in this article, or claim that may be made by its manufacturer, is not guaranteed or endorsed by the publisher.
Acknowledgments
We thank Alexander Hunter and the Bermuda Institute of Ocean Sciences, for facilitating technical SCUBA diving in Bermuda. We also thank Kevin Wong and Danielle Becker for their assistance with maintaining corals in the mesocosm and Hollie Putnam for use of the larval respiration set up and guidance with respiration data analysis. This work was conducted under permission from the Bermuda Government Department of Environment and Natural Resources permit #2019061407.
References
Babcock, R., and Mundy, C. (1996). Coral recruitment: consequences of settlement choice for early growth and survivorship in two scleractinians. J. Exp. Mar. Bio. Ecol. 206, 179–201. doi: 10.1016/S0022-0981(96)02622-6
Badyaev, A. V., and Uller, T. (2009). Parental effects in ecology and evolution: mechanisms, processes and implications. Philos. Trans. R. Soc. Lond. B Biol. Sci. 364, 1169–1177. doi: 10.1098/rstb.2008.0302
Bak, R. P. M., and Engel, M. S. (1979). Distribution, abundance and survival of juvenile hermatypic corals (Scleractinia) and the importance of life history strategies in the parent coral community. Mar. Biol. 54, 341–352. doi: 10.1007/BF00395440
Bak, R. P. M., Nieuwland, G., and Meesters, E. H. (2005). Coral reef crisis in deep and shallow reefs: 30 years of constancy and change in reefs of Curacao and Bonaire. Coral Reefs 24, 475–479. doi: 10.1007/s00338-005-0009-1
Banaszak, A. T., Barba Santos, M. G., LaJeunesse, T. C., and Lesser, M. P. (2006). The distribution of mycosporine-like amino acids (MAAs) and the phylogenetic identity of symbiotic dinoflagellates in cnidarian hosts from the Mexican Caribbean. J. Exp. Mar. Bio. Ecol. 337, 131–146. doi: 10.1016/j.jembe.2006.06.014
Bongaerts, P., Ridgway, T., Sampayo, E. M., and Hoegh-Guldberg, O. (2010). Assessing the “deep reef refugia” hypothesis: focus on Caribbean reefs. Coral Reefs 29, 309–327. doi: 10.1007/s00338-009-0581-x
Bongaerts, P., Riginos, C., Brunner, R., Englebert, N., Smith, S. R., and Hoegh-Guldberg, O. (2017). Deep reefs are not universal refuges: reseeding potential varies among coral species. Sci. Adv. 3:e1602373. doi: 10.1126/sciadv.1602373
Bongaerts, P., Riginos, C., Hay, K. B., van Oppen, M. J. H., Hoegh-Guldberg, O., and Dove, S. (2011). Adaptive divergence in a scleractinian coral: physiological adaptation of Seriatopora hystrix to shallow and deep reef habitats. BMC Evol. Biol. 11:303. doi: 10.1186/1471-2148-11-303
Brazeau, D. A., Gleason, D. F., and Morgan, M. E. (1998). Self-fertilization in brooding hermaphroditic Caribbean corals: evidence from molecular markers. J. Exp. Mar. Bio. Ecol. 231, 225–238. doi: 10.1016/S0022-0981(98)00097-5
Burke, L., Reytar, K., Spalding, M., and Perry, A. (2011). Reefs at Risk Revisited. World Resources Institute, 114: 1–130.
Cameron, K. A., and Harrison, P. L. (2020). Density of coral larvae can influence settlement, post-settlement colony abundance and coral cover in larval restoration. Sci. Rep. 10:5488. doi: 10.1038/s41598-020-62366-4
Chamberland, V. F., Latijnhouwers, K. R. W., Huisman, J., Hartmann, A. C., and Vermeij, M. J. A. (2017). Costs and benefits of maternally inherited algal symbionts in coral larvae. Proc. Biol. Sci. 284:20170852. doi: 10.1098/rspb.2017.0852
Chornesky, E. A., and Peters, E. C. (1987). Sexual reproduction and colony growth in the scleractinian coral Porites astreoides. Biol. Bull. 172, 161–177. doi: 10.2307/1541790
Cumbo, V. R., Edmunds, P. J., Wall, C. B., and Fan, T. Y. (2013). Brooded coral larvae differ in their response to high temperature and elevated pCO2 depending on the day of release. Mar. Bio. 160, 2903–2917. doi: 10.1007/s00227-013-2280-y
de Putron, S. J., Lawson, J. M., White, K. Q. L., Costa, M. T., Geronimus, M. V. B., and MacCarthy, A. (2017). Variation in larval properties of the Atlantic brooding coral Porites astreoides between different reef sites in Bermuda. Coral Reefs 36, 383–393. doi: 10.1007/s00338-016-1527-8
de Putron, S. J., and Smith, S. R. (2011). Planula release and reproductive seasonality of the scleractinian coral Porites astreoides in Bermuda, a high-latitude reef. Bull. Mar. Sci. 87, 75–90. doi: 10.5343/bms.2009.1027
Dixon, G. B., Davies, S. W., Aglyamova, G. V., Meyer, E., Bay, L. K., and Matz, M. V. (2015). Genomic determinants of coral heat tolerance across latitudes. Science 348, 1460–1462. doi: 10.1126/science.1261224
Edmunds, P. J., Cumbo, V. R., and Fan, T. Y. (2011). Effects of temperature on the respiration of brooded larvae from tropical reef corals. J. Exp. Biol. 214, 2783–2790. doi: 10.1242/jeb.055343
Einbinder, S., Gruber, D. F., Salomon, E., Liran, O., Keren, N., and Tchernov, D. (2016). Novel adaptive photosynthetic characteristics of mesophotic symbiotic microalgae within the reef-building coral, Stylophora pistillata. Front. Mar. Sci. 3:195. doi: 10.3389/fmars.2016.00195
Elizalde-Rendón, E. M., Horta-Puga, G., González-Diaz, P., and Carricart-Ganivet, J. P. (2010). Growth characteristics of the reef-building coral Porites astreoides under different environmental conditions in the Western Atlantic. Coral Reefs 29, 607–614. doi: 10.1007/s00338-010-0604-7
Figueiredo, J., Baird, A. H., Cohen, M. F., Flot, J.-F., Kamiki, T., Meziane, T., et al. (2012). Ontogenetic change in the lipid and fatty acid composition of scleractinian coral larvae. Coral Reefs 31, 613–619. doi: 10.1007/s00338-012-0874-3
Fox, J., and Weisberg, S. (2019). An {R} companion to Applied Regression, 3rd Edn. Thousand Oaks, CA: Sage Publication.
Fricke, H., and Meischner, D. (1985). Depth limits of Bermudan scleractinian corals: a submersible survey. Mar. Biol. 88, 175–187. doi: 10.1007/BF00397165
Gleason, D. F., and Hofmann, D. K. (2011). Coral larvae: from gametes to recruits. J. Exp. Mar. Bio. Ecol. 408, 42–57. doi: 10.1016/j.jembe.2011.07.025
Goodbody-Gringley, G. (2010). Diel planulation by the brooding coral Favia fragum (Esper, 1797). J. Exp. Mar. Biol. Ecol. 389, 70–74. doi: 10.1016/j.jembe.2010.03.016
Goodbody-Gringley, G., Marchini, C., Chequer, A. D., and Goffredo, S. (2015). Population structure of Montastraea cavernosa on shallow versus mesophotic reefs in Bermuda. PLoS One 10:e0142427. doi: 10.1371/journal.pone.0142427
Goodbody-Gringley, G., Noyes, T., and Smith, S. R. (2019). “Bermuda,” in Mesophotic Coral Ecosystems Coral Reefs of the World, eds Y. Loya, K. Puglise, and T. Bridge (Berlin: Springer).
Goodbody-Gringley, G., and Waletich, J. (2018). Morphological plasticity of the depth generalist coral, Montastraea cavernosa, on mesophotic reefs in Bermuda. Ecology 99, 1688–1690. doi: 10.1002/ecy.2232
Goodbody-Gringley, G., Wong, K. H., Becker, D. M., Glennon, K., and de Putron, S. J. (2018). Reproductive ecology and early life history traits of the brooding coral, Porites astreoides, from shallow to mesophotic zones. Coral Reefs 37, 483–494. doi: 10.1007/s00338-018-1673-2
Gould, K., Bruno, J. B., Ju, R., and Goodbody-Gringley, G. (2021). Upper-mesophotic and shallow reef corals exhibit similar thermal tolerance, sensitivity and optima. Coral Reefs 40, 907–920. doi: 10.1007/s00338-021-02095-w
Graham, E. M., Baird, A. H., Willis, B. L., and Connolly, S. R. (2013). Effects of delayed settlement on post-settlement growth and survival of scleractinian coral larvae. Oecologia 173, 431–438. doi: 10.1007/s00442-013-2635-6
Green, D. H., Edmunds, P. J., and Carpenter, R. C. (2008). Increasing relative abundance of Porites astreoides on Caribbean reefs mediated by an overall decline in coral cover. Mar. Ecol. Prog. Ser. 359, 1–10. doi: 10.3354/meps07454
Harii, S., Nadaoka, K., Yamamoto, M., and Iwao, K. (2007). Temporal changes in settlement, lipid content and lipid composition of larvae of the spawning hermatypic coral Acropora tenuis. Mar. Ecol. Prog. Ser. 346, 89–96. doi: 10.3354/meps07114
Hartmann, A. C., Marhaver, K. L., Chamberland, V. F., Sandin, S. A., and Vermeij, M. J. A. (2013). Large birth size does not reduce negative latent effects of harsh environments across life stages in two coral species. Ecology 94, 1966–1976. doi: 10.1890/13-0161.1
Hinderstein, L. M., Marr, J. C. A., Martinez, F. A., Dowgiallo, M. J., Puglise, K. A., Pyle, R. L., et al. (2010). Theme section on “Mesophotic Coral Ecosystems: characterization, Ecology, and Management.”. Coral Reefs 29, 247–251. doi: 10.1007/s00338-010-0614-5
Hoegh-Guldberg, O. (1999). Climate change, coral bleaching and the future of the world’s coral reefs. Mar. Freshw. Res. 50, 839–866. doi: 10.1071/mf99078
Hoegh-Guldberg, O., Poloczanska, E. S., Skirving, W., and Dove, S. (2017). Coral reef ecosystems under climate change and ocean acidification. Front. Mar. Sci. 4:158. doi: 10.3389/fmars.2017.00158
Holstein, D. M., Smith, T. B., Gyory, J., and Paris, C. B. (2015). Fertile fathoms: deep reproductive refugia for threatened shallow corals. Sci. Rep. 5:12407. doi: 10.1038/srep12407
Iglesias-Prieto, R., Beltrán, V. H., LaJeunesse, T. C., Reyes-Bonilla, H., and Thomé, P. E. (2004). Different algal symbionts explain the vertical distribution of dominant reef corals in the eastern Pacific. Proc. Biol. Sci. 271, 1757–1763. doi: 10.1098/rspb.2004.2757
Isomura, N., and Nishihira, M. (2001). Size variation of planulae and its effect on the lifetime of planulae in three pocilloporid corals. Coral Reefs 20, 309–315. doi: 10.1007/s003380100180
Jones, R. J. (2008). Coral bleaching, bleaching-induced mortality, and the adaptive significance of the bleaching response. Mar. Biol. 154, 65–80. doi: 10.1007/s00227-007-0900-0
Kenkel, C. D., Goodbody-Gringley, G., Caillaud, D., Davies, S. W., Bartels, E., and Matz, M. V. (2013a). Evidence for a host role in thermotolerance divergence between populations of the mustard hill coral (Porites astreoides) from different reef environments. Mol. Ecol. 22, 4335–4348. doi: 10.1111/mec.12391
Kenkel, C. D., Meyer, E., and Matz, M. V. (2013b). Gene expression under chronic heat stress in populations of the mustard hill coral (Porites astreoides) from different thermal environments. Mol. Ecol. 22, 4322–4334. doi: 10.1111/mec.12390
Kenkel, C. D., Setta, S. P., and Matz, M. V. (2015). Heritable differences in fitness-related traits among populations of the mustard hill coral. Porites Astreoides Heredity 115, 509–516. doi: 10.1038/hdy.2015.52
LaJeunesse, T. C., Bhagooli, R., Hidaka, M., deVantier, L., Done, T., Schmidt, G. W., et al. (2004). Closely related Symbiodinium spp. differ in relative dominance in coral reef host communities across environmental, latitudinal and biogeographic gradients. Mar. Ecol. Prog. Ser. 284, 147–161. doi: 10.3354/meps284147
LaJeunesse, T. C., Parkinson, J. E., Gabrielson, P. W., Jeong, H. J., Reimer, J. D., Voolstra, C. R., et al. (2018). Systematic revision of Symbiodiniaceae highlights the antiquity and diversity of coral endosymbionts. Curr. Biol. 28, 2570.e6–2580.e6. doi: 10.1016/j.cub.2018.07.008
Lesser, M. P., Slattery, M., and Mobley, C. D. (2018). Biodiversity and functional ecology of mesophotic coral reefs. Annu. Rev. Ecol. Evol. Syst. 49, 49–71. doi: 10.1146/annurev-ecolsys-110617-062423
Lesser, M. P., Slattery, M., Stat, M., Ojimi, M., Gates, R. D., and Grottoli, A. (2010). Photoacclimatization by the coral Montastraea cavernosa in the mesophotic zone: light, food, and genetics. Ecology 91, 990–1003. doi: 10.1890/09-0313.1
Lichtenberg, M., Larkum, A. W. D., and Kühl, M. (2016). Photosynthetic acclimation of Symbiodinium in hospite depends on vertical position in the tissue of the Scleractinian coral Montastrea curta. Front. Microbiol. 7:230. doi: 10.3389/fmicb.2016.00230
Malik, A., Einbinder, S., Martinez, S., Tchernov, D., Haviv, S., Almuly, R., et al. (2021). Molecular and skeletal fingerprints of scleractinian coral biomineralization: from the sea surface to mesophotic depths. Acta Biomater. 120, 263–276. doi: 10.1016/j.actbio.2020.01.010
Manahan, D. T. (1990). Adaptations by invertebrate larvae for nutrient acquisition from seawater. Am. Zool. 30, 147–160. doi: 10.1093/icb/30.1.147
Marshall, D. J. (2008). Transgenerational plasticity in the sea: context-dependent maternal effects across the life history. Ecology 89, 418–427. doi: 10.1890/07-0449.1
Marshall, D. J., and Keough, M. J. (2003). Variation in the dispersal potential of non-feeding invertebrate larvae: the desperate larva hypothesis and larval size. Mar. Ecol. Prog. Ser. 255, 145–153. doi: 10.3354/meps255145
Martinez, S., Kolodny, Y., Shemesh, E., Scucchia, F., Nevo, R., Levin-Zaidman, S., et al. (2020). Energy sources of the depth-generalist mixotrophic coral Stylophora pistillata. Front. Mar. Sci. 7:988. doi: 10.3389/fmars.2020.566663
Mass, T., Einbinder, S., Brokovich, E., Shashar, N., Vago, R., Erez, J., et al. (2007). Photoacclimation of Stylophora pistillata to light extremes: metabolism and calcification. Mar. Ecol. Prog. Ser. 334, 93–102. doi: 10.3354/meps334093
McCloskey, L. R., Muscatine, L., and Smith, D. C. (1984). Production and respiration in the Red Sea coral Stylophora pistillata as a function of depth. Proc. R. Soc. Lond. Series B. Biol. Sci. 222, 215–230. doi: 10.1098/rspb.1984.0060
Morse, D. E., Hooker, N., Morse, A. N. C., and Jensen, R. A. (1988). Control of larval metamorphosis and recruitment in sympatric agariciid corals. J. Exp. Mar. Bio. Ecol. 116, 193–217. doi: 10.1016/0022-0981(88)90027-5
Mundy, C. N., and Babcock, R. C. (1998). Role of light intensity and spectral quality in coral settlement: implications for depth-dependent settlement? J. Exp. Mar. Bio. Ecol. 223, 235–255. doi: 10.1016/s0022-0981(97)00167-6
Olito, C., White, C. R., Marshall, D. J., and Barneche, D. R. (2017). Estimating monotonic rates from biological data using local linear regression. J. Exp. Biol. 220, 759–764. doi: 10.1242/jeb.148775
Pan, T. C. F., Applebaum, S. L., Lentz, B. A., and Manahan, D. T. (2016). Predicting phenotypic variation in growth and metabolism of marine invertebrate larvae. J. Exp. Mar. Bio. Ecol. 483, 64–73. doi: 10.1016/j.jembe.2016.06.006
Piniak, G. A., Fogarty, N. D., Addison, C. M., and Kenworthy, W. J. (2005). Fluorescence census techniques for coral recruits. Coral Reefs 24, 496–500. doi: 10.1007/s00338-005-0495-1
Prada, C., and Hellberg, M. E. (2013). Long pre-reproductive selection and divergence by depth in a Caribbean candelabrum coral. Proc. Natl. Acad. Sci. U.S.A. 110, 3961–3966. doi: 10.1073/pnas.1208931110
Putnam, H. M., and Gates, R. D. (2015). Preconditioning in the reef-building coral Pocillopora damicornis and the potential for trans-generational acclimatization in coral larvae under future climate change conditions. J. Exp. Biol. 218, 2365–2372. doi: 10.1242/jeb.123018
Putnam, H. M., Ritson-Williams, R., Cruz, J. A., Davidson, J. M., and Gates, R. D. (2020). Environmentally-induced parental or developmental conditioning influences coral offspring ecological performance. Sci. Rep. 10:13664. doi: 10.1038/s41598-020-70605-x
Quigley, K. M., Willis, B. L., and Bay, L. K. (2016). Maternal effects and Symbiodinium community composition drive differential patterns in juvenile survival in the coral Acropora tenuis. R. Soc. Open Sci. 3:160471. doi: 10.1098/rsos.160471
R Core Team. (2020). R: A Language and Environment for Statistical Computing. Vienna: R Foundation for Statistical Computing.
Raymundo, L. J., and Maypa, A. P. (2004). Getting bigger faster: mediation of size-specific mortality via fusion in juvenile coral transplants. Ecol. Appl. 14, 281–295. doi: 10.1890/02-5373
Reich, H. G., Robertson, D. L., and Goodbody-Gringley, G. (2017). Do the shuffle: changes in Symbiodinium consortia throughout juvenile coral development. PLoS One 12:e0171768. doi: 10.1371/journal.pone.0171768
Reynolds, J. M., Bruns, B. U., Fitt, W. K., and Schmidt, G. W. (2008). Enhanced photoprotection pathways in symbiotic dinoflagellates of shallow-water corals and other cnidarians. Proc. Natl. Acad. Sci. U.S.A. 105, 13674–13678. doi: 10.1073/pnas.0805187105
Ritson-Williams, R., Arnold, S. N., Fogarty, N. D., Steneck, R. S., Vermeij, M. J. A., and Paul, V. J. (2009). New perspectives on ecological mechanisms affecting coral recruitment on reefs. Smithson. Contrib. Mar. Sci. 38:437. doi: 10.5479/si.01960768.38.437
Rouzé, H., Galand, P. E., Medina, M., Bongaerts, P., Pichon, M., Pérez-Rosales, G., et al. (2021). Symbiotic associations of the deepest recorded photosynthetic scleractinian coral (172 m depth). ISME J. 15, 1564–1568. doi: 10.1038/s41396-020-00857-y
Scucchia, F., Nativ, H., Neder, M., Goodbody-Gringley, G., and Mass, T. (2020). Physiological characteristics of Stylophora pistillata larvae across a depth gradient. Front. Mar. Sci. 7:13. doi: 10.3389/fmars.2020.00013
Serrano, X., Baums, I. B., O’Reilly, K., Smith, T. B., Jones, R. J., Shearer, T. L., et al. (2014). Geographic differences in vertical connectivity in the Caribbean coral Montastraea cavernosa despite high levels of horizontal connectivity at shallow depths. Mol. Ecol. 23, 4226–4240. doi: 10.1111/mec.12861
Serrano, X. M., Baums, I. B., Smith, T. B., Jones, R. J., Shearer, T. L., and Baker, A. C. (2016). Long distance dispersal and vertical gene flow in the Caribbean brooding coral Porites astreoides. Sci. Rep. 6:21619. doi: 10.1038/srep21619
Serrano, X. M., Miller, M. W., Hendee, J. C., Jensen, B. A., Gapayao, J. Z., Pasparakis, C., et al. (2018). Effects of thermal stress and nitrate enrichment on the larval performance of two Caribbean reef corals. Coral Reefs 37, 173–182. doi: 10.1007/s00338-017-1645-y
Shlesinger, T., and Loya, Y. (2021). Depth-dependent parental effects create invisible barriers to coral dispersal. Commun Biol. 4:202. doi: 10.1038/s42003-021-01727-9
Smith, S. R., Sarkis, S., Murdoch, T. J. T., Weil, E., Croquer, A., Bates, N. R., et al. (2013). “Threats to coral reefs of Bermuda,” in Coral Reefs of the United Kingdom Overseas Territories, Coral Reefs of the World 4, ed. C. R. C. Sheppard (Dordrecht: Springer Science+Business Media), 173–188. doi: 10.1007/978-94-007-5965-7_13
Sneed, J. M., Sharp, K. H., Ritchie, K. B., and Paul, V. J. (2014). The chemical cue tetrabromopyrrole from a biofilm bacterium induces settlement of multiple Caribbean corals. Proc. Biol. Sci. 281:20133086. doi: 10.1098/rspb.2013.3086
Torda, G., Donelson, J. M., Aranda, M., Barshis, D. J., Bay, L., Berumen, M. L., et al. (2017). Rapid adaptive responses to climate change in corals. Nat. Clim. Chang. 7, 627–636. doi: 10.1038/nclimate3374
Turner, J. A., Thomson, D. P., Cresswell, A. K., Trapon, M., and Babcock, R. C. (2018). Depth-related patterns in coral recruitment across a shallow to mesophotic gradient. Coral Reefs 37, 711–722. doi: 10.1007/s00338-018-1696-8
Venn, A. A., Wilson, M. A., Trapido-Rosenthal, H. G., Keely, B. J., and Douglas, A. E. (2006). The impact of coral bleaching on the pigment profile of the symbiotic alga, Symbiodinium. Plant Cell Environ. 29, 2133–2142. doi: 10.1111/j.1365-3040.2006.001587.x
Warner, M. E., Fitt, W. K., and Schmidt, G. W. (1999). Damage to photosystem II in symbiotic dinoflagellates: a determinant of coral bleaching. Proc. Natl. Acad. Sci. U.S.A. 96, 8007–8012. doi: 10.1073/pnas.96.14.8007
Wendt, D. E. (2000). Energetics of larval swimming and metamorphosis in four species of Bugula (Bryozoa). Biol. Bull. 198, 346–356. doi: 10.2307/1542690
Wong, K., Goodbody-Gringley, G., de Putron, S. J., Chequer, A. D., and Putnam, H. (2021). Brooded coral offspring physiology depends on the combined effects of parental press and pulse thermal history. Glob. Chang. Biol. 27, 3179–3195. doi: 10.1111/gcb.15629
Keywords: mesophotic, plasticity, refugia, respiration, coral larvae, Bermuda
Citation: Goodbody-Gringley G, Scucchia F, Ju R, Chequer A, Einbinder S, Martinez S, Nativ H and Mass T (2021) Plasticity of Porites astreoides Early Life History Stages Suggests Mesophotic Coral Ecosystems Act as Refugia in Bermuda. Front. Mar. Sci. 8:702672. doi: 10.3389/fmars.2021.702672
Received: 29 April 2021; Accepted: 05 August 2021;
Published: 03 September 2021.
Edited by:
Hajime Kayanne, The University of Tokyo, JapanReviewed by:
Frederic Sinniger, University of the Ryukyus, JapanAlma Paola Rodríguez-Troncoso, University of Guadalajara, Mexico
Copyright © 2021 Goodbody-Gringley, Scucchia, Ju, Chequer, Einbinder, Martinez, Nativ and Mass. This is an open-access article distributed under the terms of the Creative Commons Attribution License (CC BY). The use, distribution or reproduction in other forums is permitted, provided the original author(s) and the copyright owner(s) are credited and that the original publication in this journal is cited, in accordance with accepted academic practice. No use, distribution or reproduction is permitted which does not comply with these terms.
*Correspondence: Gretchen Goodbody-Gringley, Z2dvb2Rib2R5QHJlZWZyZXNlYXJjaC5vcmc=; Tali Mass, dG1hc3NAdW5pdi5oYWlmYS5hYy5pbA==