- 1School of Environmental Sciences, University of Liverpool, Liverpool, United Kingdom
- 2Freshwater Institute, Fisheries and Oceans Canada, Winnipeg, MB, Canada
- 3Sea Mammal Research Unit, Gatty Marine Laboratory, St. Andrews, United Kingdom
Warming of the Arctic has resulted in environmental and ecological changes, termed borealization, leading to the northward shift of temperate species. Borealization has occurred across all trophic levels, altering the structure of the food web. The onset and rate of borealization likely varies with latitude, depending on local warming and advection of warmer water into the Arctic. In order to assess latitudinal trends in food web structure in the Arctic, we analyzed stable nitrogen isotopes of specific amino acids alongside bulk stable carbon isotopes in ringed seal muscle tissue from the Canadian Arctic Archipelago (high-Arctic) and Southern Baffin Bay (mid-Arctic) from 1990 to 2016. Our results indicate a shift in food web structure in the high-Arctic that has occurred more recently when compared with the mid-Arctic. Specifically, over the past 25 years, the trophic position of ringed seals from the mid-Arctic was largely constant, whereas the trophic position of ringed seals decreased in the high-Arctic, reaching similar values observed in the mid-Arctic in 2015–2016. This suggests a potential shortening of the food chain length in the high-Arctic, possibly driven by changes in zooplankton communities feeding complexity in association with sea ice decline. This study identifies a temporal offset in the timing of borealization in the Canadian Arctic, resulting in different response of food webs to ecological changes, depending on latitude.
Introduction
The Arctic is changing rapidly, warming twice as fast as the global average causing sea ice extent and thickness to decline (Meredith et al., 2019). Increased sea surface temperature has led to northward shifts of subarctic and temperate phytoplankton, zooplankton and fish species, a process described as “borealization” (Fossheim et al., 2015; Møller and Nielsen, 2020). These changes in community structure have resulted in changes to predator-prey interactions, with implications for food web stability and resilience (Kortsch et al., 2015; Yurkowski et al., 2018; Pecuchet et al., 2020). Monitoring changes in the marine environment, especially in polar regions, is logistically challenging due to strong seasonality and remoteness. Archives of marine predator tissues offer a unique resource in overcoming the limited environmental sampling opportunities in the Arctic.
Trophic position is a fundamental property of ecological communities that can reflect integrated changes in ecosystems. Food web structure and the functional roles of predators can be assessed using trophic position as an expression of food chain length using top and near-top trophic level species (Post, 2002a). Here, we used the trophic position of ringed seals (Pusa hispida), a generalist ice-dependent Arctic predator, to assess latitudinal differences in multi-decadal trends in food chain length in the Canadian Arctic. Ringed seals are circumpolar, and have an extensive distribution in northern Canada, occurring continuously across latitudes from Newfoundland and Labrador to the Arctic basin (Ferguson et al., 2020). They are predators and display high plasticity in their diet with dietary items consisting of a wide variety of pelagic invertebrates, e.g., mysids and amphipods species, and forage fish, e.g., Arctic cod (Boreogadus saida) and capelin (Mallotus villosus; Ogloff et al., 2019; Bengtsson et al., 2020). Despite being generalists, ringed seals display a preference for Arctic prey, especially the lipid-rich Arctic cod (Hamilton et al., 2019; Bengtsson et al., 2020), which makes them excellent indicators of biotic changes in Arctic food webs.
The ratios between heavy (15N and 13C) and light (14N and 12C) stable nitrogen and carbon isotopes, referred to as δ15Nbulk and δ13Cbulk hereafter, are a common tool for studying food webs. δ13Cbulk typically undergoes minimal fractionation (0.4 ± 1.3‰; Post, 2002b) between trophic steps and has been used to trace the origin of resources, e.g., phytoplankton versus sea ice algae (Hobson et al., 1995). δ15Nbulk is a commonly used tracer for estimating trophic position, as it generally undergoes greater fractionation between each trophic level (3.4 ± 1.0‰; Post, 2002b), but is heavily dependent on knowledge of δ15N at the base of the food web, or “baseline.” δ15N of nitrate, taken up by phytoplankton, represents the δ15N at the baseline (Mariotti et al., 1981). The δ15N-nitrate differs between Atlantic and Pacific waters (Somes et al., 2010), thus supplying nitrate with distinct water mass properties alongside δ15N-nitrate values. Spatial trends in water mass influence alongside temporal changes in circulation leads to a heterogenous and evolving δ15N baseline across the Arctic, that needs to be accounted for in order to reliably detect changes in trophic position of predators (de la Vega et al., 2020).
Compound-specific stable nitrogen isotopes of amino acids (δ15NAA) can overcome this issue. δ15NAA is a powerful biomarker approach that disentangles baseline and fractionating trophic effects when using δ15N values to estimate trophic position. The δ15N of the “source” amino acid (AA) phenylalanine (δ15NPhe), can conservatively trace the δ15N at the baseline as it experiences minimal fractionation (<1‰) during trophic transfer (McMahon and McCarthy, 2016). The δ15N of “trophic” AAs (δ15Ntrophic), e.g., glutamic acid, undergoes significant fractionation (>3‰) resulting in 15N enrichment between each trophic step (McMahon and McCarthy, 2016). This approach simultaneously fingerprints both the δ15N baseline and trophic information in a predator from the analysis of predator tissue alone. This allows accurate estimation of changes in relative trophic position while accounting for variation in δ15N baseline, using baseline-corrected δ15Ntrophic (Cor-δ15Ntrophic; de la Vega et al., 2020). This is particularly powerful when studying multi-decadal trends in predators that integrated temporally evolving baselines across latitudes.
Mechanisms involved in the northward expansion of boreal and temperate species are directly associated with the local rate of warming as well as the advection of warmer water into the Arctic (Wassmann, 2015). Hence, the rate and timing of borealization likely varies with latitude (Kortsch et al., 2015). Here, we used temporal trends in mean sea ice concentration and length of the open water period (OWP) as an indication of the rate of warming across latitudes. The Canadian Arctic Archipelago (CAA) is one of the two Arctic outflow shelves, and receives water exiting the Arctic, mainly consisting of Pacific waters flowing southward into the Baffin Bay (Figure 1; Carmack and Wassmann, 2006). In addition, Southern Baffin Bay receives warmer Atlantic water advected via the Irminger current and flowing northward along the West coast of Greenland (Figure 1; Tang et al., 2004), potentially enhancing the northward spread of boreal and temperate species (Wassmann, 2015), and providing new potential prey species for opportunistic predators, such as ringed seals.
The main objective of this study was to assess the latitudinal difference in the response of food webs to environmental changes in the Arctic. We used δ15NAA and δ13Cbulk in ringed seal muscle from 1990 to 2016, from the CAA and Baffin Island to monitor the trophic structure of both systems, respectively at high- and mid-Arctic latitudes, influenced by different water masses (Figure 1). We predicted that changes in the mid-Arctic will be more prominent than in the high-Arctic in relation to the changing environment, which in turn influences the rate of borealization in these systems. We hypothesized that: (1) δ15NPhe and δ13Cbulk, representing isotopic baselines, will vary temporally in association with environmental changes; (2) Cor-δ15Ntrophic, representing the relative trophic position of ringed seals will vary temporally and reflect changes in trophic structure; and (3) the temporal trends in δ13Cbulk, δ15NPhe, and Cor-δ15Ntrophic will differ between the high- and mid-Arctic, reflecting differences in the onset and rate of environmental change, and in turn borealization between latitudes.
Materials and Methods
Environmental Data
Sea ice concentrations for the CAA and the Baffin Island region were obtained using Canadian Ice Service’s IceGraph 2.0 tool1 which disseminates sea ice data on regional and sub-regional spatial scales across the Canadian Arctic. We queried databases for each of the pre-defined sub-regions of “Eastern Parry” and “Davis Strait,” which encompassed Resolute and Pangnirtung where ringed seal muscle where collected, for total accumulated ice concentrations from early February to end of September, representing the period integrated in ringed seal muscles, per year from 1978 to 2021. OWP was estimated as the number of days on which the weekly sea ice concentration reached and remained below 50% in “Eastern Parry” and “Davis Strait” sub-regions.
Seal Sampling Design
Muscle tissue from adult ringed seals were opportunistically sampled in the CAA and Baffin Island as part of Inuit subsistence harvests. Samples from the CAA were collected in Resolute, Nunavut (latitude 75°N) from 1992 to 2016 (n = 66) and samples from Baffin Island were collected in Pangnirtung, Nunavut (latitude 66°N) from 1990 to 2016 (n = 39, Table 1). All samples were stored at −20°C prior to analysis. δ15N and δ13C values of marine mammals do not change through long-term storage in freezers (Yurkowski et al., 2017).

Table 1. Sampling years, age class and number of muscle samples (n) of ringed seals from the Canadian Arctic Archipelago (CAA) and the Baffin Island (BI).
The majority of the samples were collected between early summer (June) and early fall (September). The turnover time of muscle tissues is poorly known for marine mammals. Vander Zanden et al. (2015) estimated the stable isotope half-life of muscle tissue for a mammal to be 29.4 days for 50 g of body mass. Assuming an average weight for ringed seals of 60 kg, ringed seal’s muscle tissue integrates δ15N and δ13C over ∼5 months, therefore mainly reflecting the seal foraging from spring (March) to summer (July). In the Canadian Arctic, ringed seals tend to be resident during the ice-season and can undertake extensive movements during the ice-free season (Yurkowski et al., 2016b). Here, muscle tissue from ringed seals from both the CAA and Baffin Island mainly represented the diet during the ice-season and break-up, likely reflecting the stable isotope signal from the CAA and Southern Baffin Bay, respectively.
Stable Isotope Analyses of Seal Samples
Stable isotope (δ13Cbulk, δ15Nbulk, and δ15NAA) analysis of seal muscle tissue was carried out at the Liverpool Isotopes for Environmental Research Laboratory, University of Liverpool and results are reported in standard δ-notation (‰).
Stable Isotope Analyses of Bulk Tissue
Each muscle sample was freeze-dried and homogenized. Approximately 0.5 mg of sample was precisely weighed (±1 μg) and sealed in a tin capsule. Samples were run for δ15Nbulk and δ13Cbulk separately. Lipids were removed from the samples using five repeated rinses with 2:1 dichloromethane:methanol to avoid the bias due to the depletion in 13C in lipids relative to the diet, before δ13Cbulk analyses.
Samples were analyzed using an elemental analyzer (Costech) coupled to Delta V isotope ratio mass spectrometer (IRMS; Thermo Fisher Scientific). Isotope values are reported in standard δ-notation (‰) relative to Vienna PeeDee Belemnite and atmospheric N2 for δ13Cbulk and δ15Nbulk, respectively. We used an internal standard of ground prawn Penaeus vannamei with well characterized δ13C and δ15N values (−22.6 and 6.8‰, respectively) to monitor precision. We analyzed this internal standard every 10 samples, and precision was < 0.2‰ for both δ13Cbulk and δ15Nbulk. To determine accuracy, we used international reference standards with known δ13C and δ15N values, USGS40 (δ13C = −26.24‰ and δ15N = −4.52‰) and USGS41a (δ13C = 36.55‰ and δ15N = 47.55‰). Both standards were analyzed at the beginning, middle and end of each run and accuracies for all the EA-IRMS runs combined here. For USGS40, mean accuracy was 0.13 ± 0.09‰ for δ13C and 0.03 ± 0.11‰ for δ15N. For USGS41a, mean accuracy was 0.01 ± 0.13‰ for δ13C and 0.01 ± 0.15‰ for δ15N (1σ, n = 19).
δ15N Analyses on Amino Acids
Approximately 7 mg of sample was hydrolyzed and propylated. Amino acid methyl esters were then acetylated, dissolved in DCM and stored at −20°C until analysis. For a full description of the laboratory protocol see the Supplementary Material.
δ15NAA values were determined using a Trace Ultra gas chromatograph (GC) coupled to a Delta V Advantage IRMS with a ConFlo IV interface (Thermo Fisher Scientific). A liquid nitrogen trap was added after the reduction oven to remove CO2 from the sample stream. The separation of AA was achieved using an HP INNOWax capillary column (Agilent). Each sample was analyzed in triplicate (measurement error <1.0‰). Precision and accuracy were determined using a mixed AA standard prepared from eight AA with known δ15N values (University of Indiana, United States and SI Science, Japan), analyzed every four injections. The mean precision of all standards was ± 0.9‰ and ranged from ± 0.7‰ for phenylalanine and ± 1.2‰ for valine (n = 35; Supplementary Table 1). The mean accuracy of all standards was ± 0.7‰ and ranged from ± 0.1‰ for leucine and ± 1.4‰ for glutamic acid (n = 35; Supplementary Table 1). Details of the method, typical precisions and accuracies, and δ15N of all identified AA are shown in Supplementary Table 1.
Seal Trophic Position Estimation
We used δ15NPhe, to track the δ15N baseline in ringed seals. We used the mean δ15N value of three main trophic amino acids (glutamic acid, aspartic acid, and leucine; de la Vega et al., 2020), to estimate the relative trophic position of ringed seals. The uncertainty regarding trophic fractionation factors between “source” and “trophic” amino acids across taxa prevents accurate estimation of an organism’s absolute trophic position (Nielsen et al., 2015). To assess the decadal trends in relative trophic position, we averaged the δ15N values of the three trophic amino acids that we baseline-corrected using δ15NPhe (Eq. 1), providing baseline-corrected δ15Ntrophic values (Cor-δ15Ntrophic).
Statistical Analyses
Statistical analyses were performed in R version 3.5.1 (R Core Team, 2018). We assessed the relationship between δ15Nbulk, δ15NPhe, and Cor-δ15Ntrophic using linear models. To analyze temporal variation in sea ice concentration and OWP, and δ13Cbulk, δ15NPhe, and Cor-δ15Ntrophic in ringed seals, linear models were fitted with sea ice concentration, OWP, δ13Cbulk, δ15NPhe, and Cor-δ15Ntrophic as a function of year for each site separately. We used the Gaussian family with no transformation of the data, assuming that measurement errors were normally distributed. Model fit was checked by residual analyses with visual inspection of quantile–quantile plots, and residuals and standardized residuals versus fitted values plots (Supplementary Material). To check for potential influential data points on the temporal trends in stable isotopes, we used leverage (i.e., hat values), studentized residuals and the Cook’s distance (Zuur et al., 2009), using the “Car package” in R (Fox et al., 2007; Supplementary Material). p-Values, R2, F-statistics, and degrees of freedom are reported for each model (Table 2). We compared the Cor-δ15Ntrophic values between Southern Baffin Bay and the CAA for years when data was available from both regions, i.e., 1993–1994, 2006 and 2015–2016, using Student t-tests. Significance was set at α = 0.01.
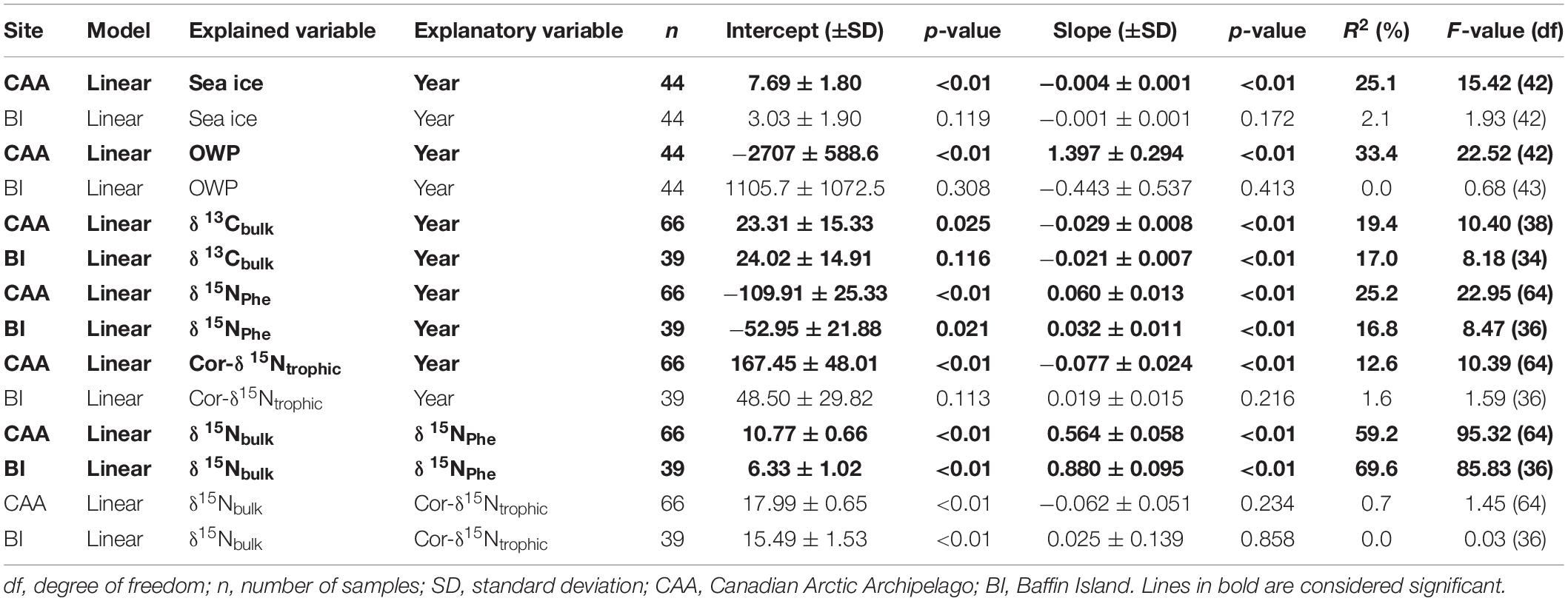
Table 2. Statistical parameters of the linear models for stable isotopes of ringed seal muscle, sea ice concentration, and open water period from each Arctic regions.
Results
Temporal Trends in Sea Ice Concentration
In the CAA, mean sea ice concentration decreased by 0.7 ± 0.2% year–1 (linear model: p < 0.01, R2 = 33.7%) and did not vary in Southern Baffin Bay from 1990 to 2016 (Figure 2A and Table 2). The OWP became longer in the CAA by 2.0 ± 0.6 days year–1 (linear model: p < 0.01, R2 = 33.7%) and did not vary in Southern Baffin Bay from 1990 to 2016 (Figure 2B and Table 2). Generally, the CAA had higher sea ice concentration (58.0 ± 9.2%) and a much shorter OWP (96 ± 27 days) than Southern Baffin Bay (38.5 ± 7.3% and 224 ± 32 days, respectively; Figures 2A,B).
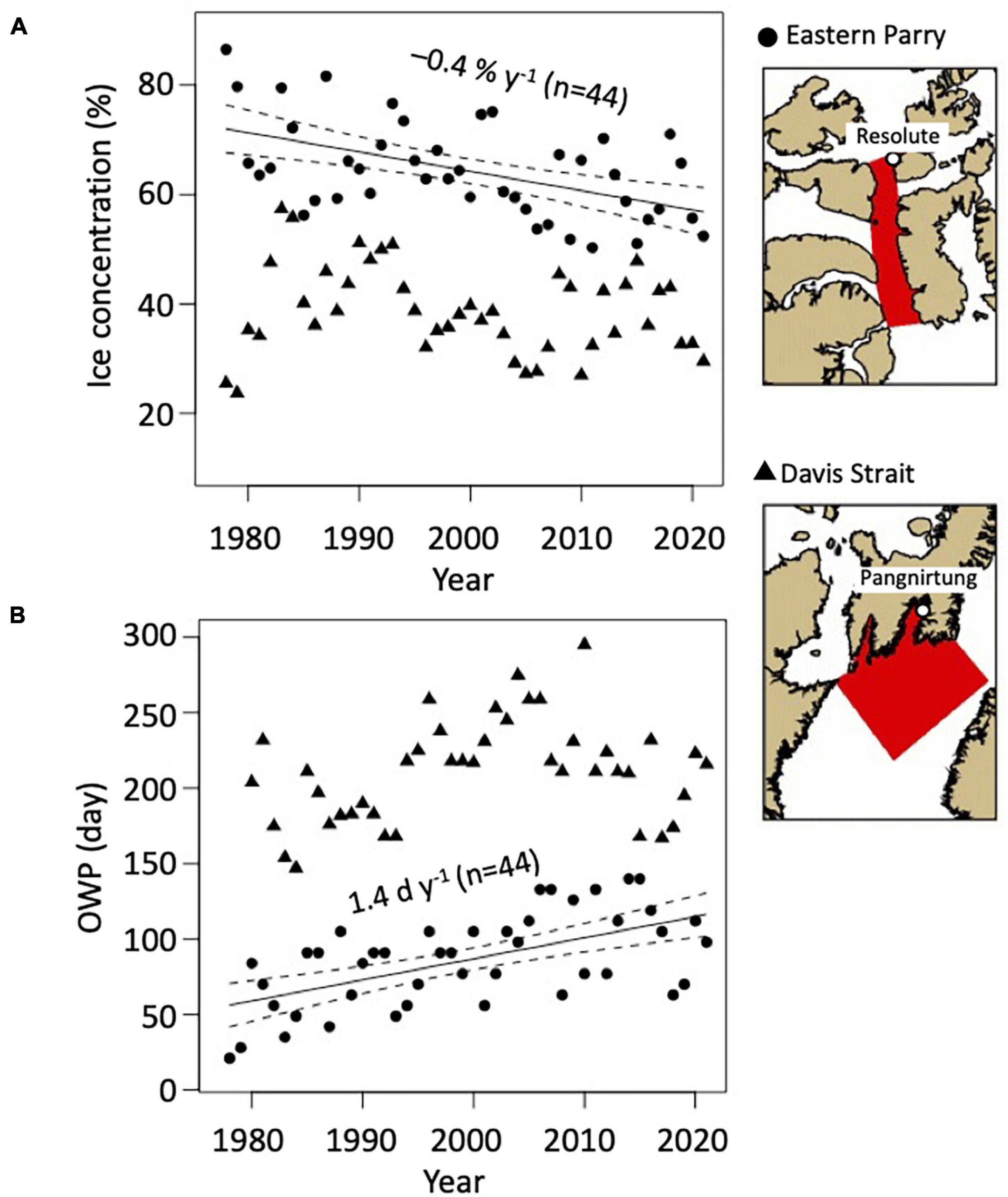
Figure 2. (A) Decadal trends in mean sea ice coverage (%), averaged over the time period integrated in ringed seal muscle (February–September) and (B) open water period (OWP) in ordinal day; the slope of the linear models (solid lines; p < 0.01) and 95% confidence interval (dashed lines) are shown on the graphs; n, number of data points; the circles show the data extracted in Eastern Parry (Canadian Arctic Archipelago) and the triangles show the data extracted in Davis Strait (Baffin Bay).
Temporal Trends in Stable Isotopes Values in Ringed Seals
δ13Cbulk became depleted in 13C (hereafter decreased) by −0.029 ± 0.008‰ y–1 in ringed seals from the CAA from 1992 (−17.7 ± 0.7‰) to 2016 (−18.6 ± 0.8‰; linear model: p < 0.01, R2 = 9.4%) and by −0.021 ± 0.007‰ y–1 in ringed seals from Baffin Island from 1990 (−18.1 ± 0.5‰) to 2015–2016 (−18.9 ± 0.2‰; linear model: p < 0.01, R2 = 17.0%; Figures 3A,B and Table 2).
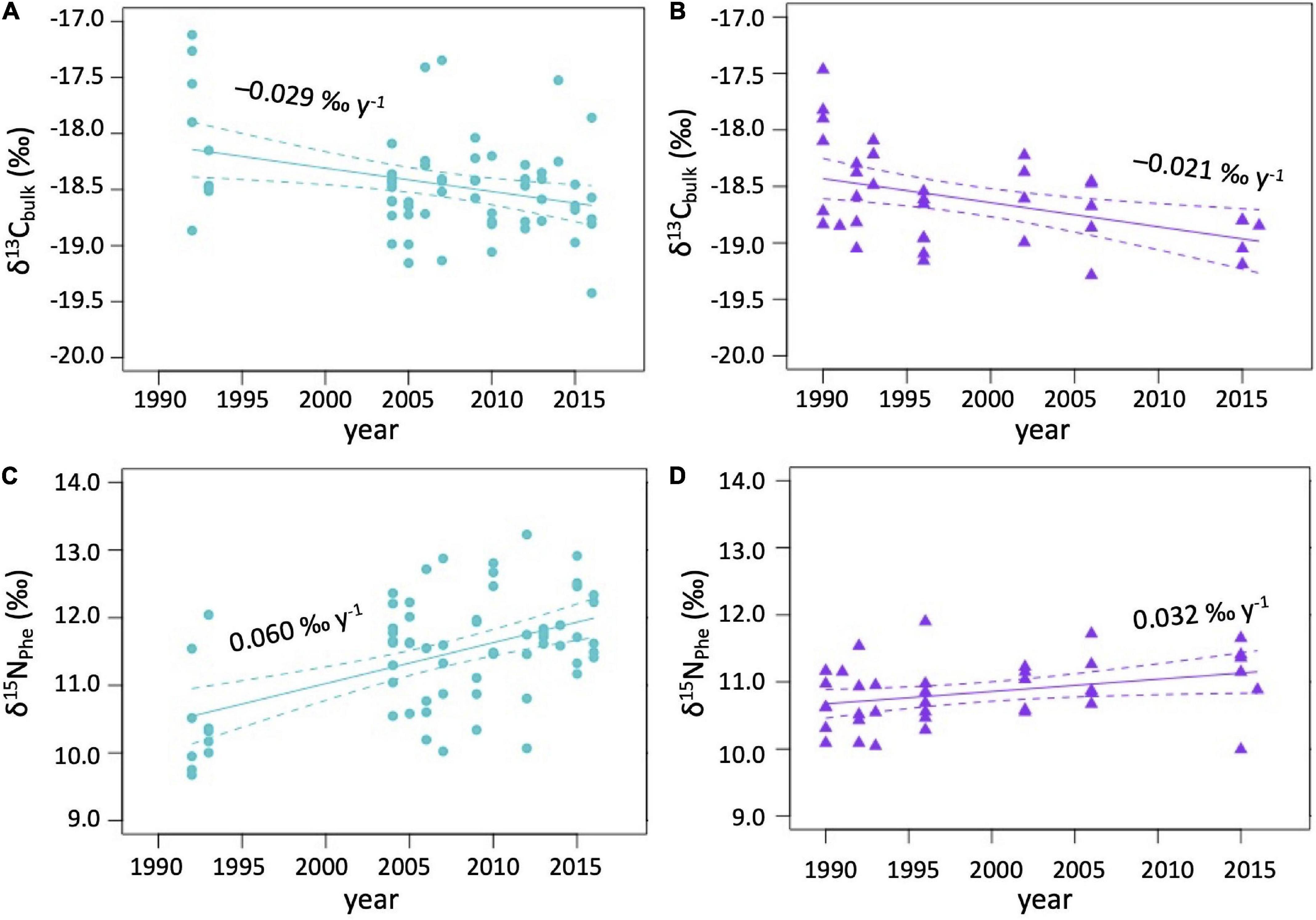
Figure 3. δ13Cbulk values in per mil (‰) per year in ringed seals muscle from (A) the Canadian Arctic Archipelago (CAA) and (B) Baffin Island; δ15NPhe values in per mil (‰) per year in ringed seals muscle from (C) the CAA and (D) Baffin Island.
δ15NPhe became enriched in 15N (hereafter increased) by 0.060 ± 0.013‰ year–1 in ringed seals from the CAA (linear model: p < 0.01, R2 = 25.2%) and by 0.032 ± 0.011‰ year–1 in ringed seals from Baffin Island (linear model: p < 0.01, R2 = 16.8%; Figures 3C,D and Table 2). δ15NPhe increased from the early 1990s to the mid-2010s from 10.4 ± 0.7‰ (1992–1993) to 11.9 ± 0.6‰ (2015–2016) in ringed seals from the CAA and from 10.7 ± 0.5‰ (1990–1992) to 11.1 ± 0.6‰ (2015–2016) in ringed seals from Baffin Island.
Cor-δ15Ntrophic of ringed seals from the CAA decreased by −0.077 ± 0.024‰ year–1 from 1992 to 2016 (linear model: p < 0.01, R2 = 12.6%; Figure 4A and Table 2). Cor-δ15Ntrophic of ringed seals from Baffin Island did not vary with time (Figure 4A and Table 2). Cor-δ15Ntrophic values were enriched in 15N in ringed seals from the CAA compared to Baffin Island in 1992–1993 (12.6 ± 1.6 and 10.9 ± 0.9‰, respectively; Student test: p < 0.01, n = 18) and in 2006 (12.5 ± 0.7 and 10.7 ± 0.9‰, respectively; Student test: p < 0.01, n = 10; Figure 4A). Cor-δ15Ntrophic values were similar in ringed seals from the CAA (11.4 ± 1.4‰) and Baffin Island (10.8 ± 0.5‰) in 2015–2016 (Student test: p = 0.159, n = 17; Figure 4A).
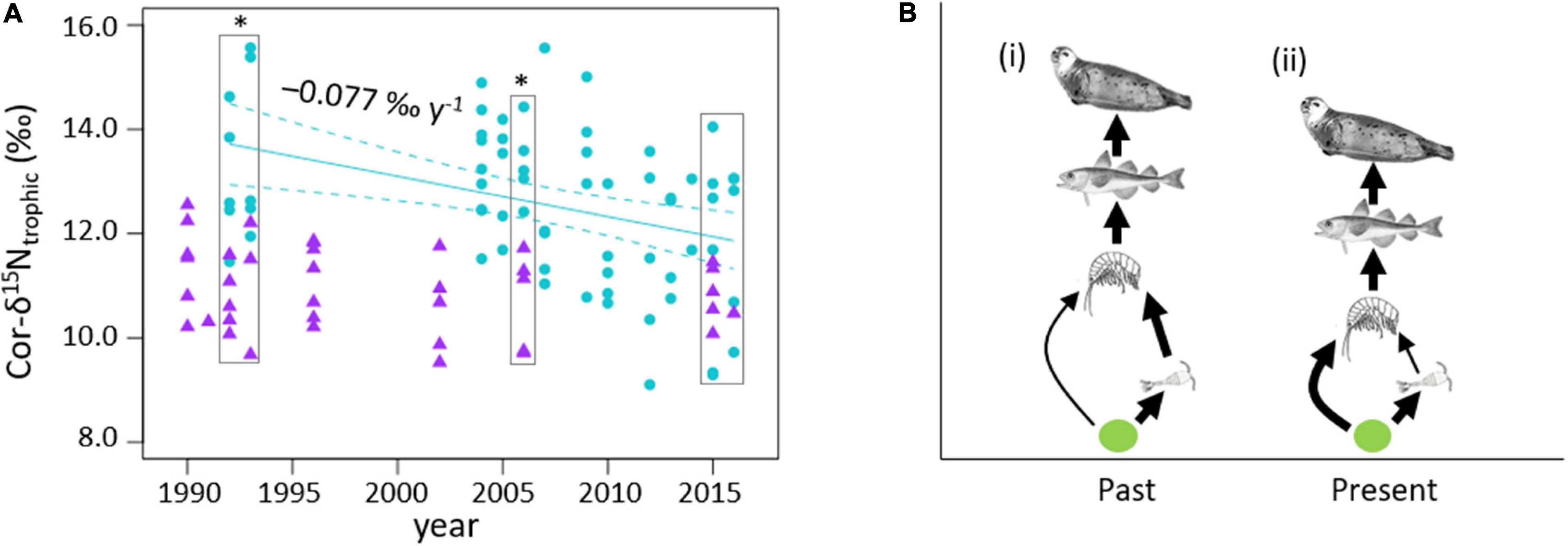
Figure 4. (A) Baseline-corrected δ15Ntrophic values (Cor-δ15Ntrophic) in per mil (‰) per year in ringed seals muscle from the CAA (turquoise circles) and Baffin Island (purple triangles); The slope of significant linear models (solid lines; p < 0.01, Table 2) and 95% confidence interval (dashed lines) are shown on the graphs; the boxes indicate the years when the Cor-δ15Ntrophic values were compared between the Baffin Island and the CAA, * indicates significant differences between the Southern Baffin Bay and the CAA; (B) proposed food web structure in time explaining the shortening of the food chain in the CAA (i) high omnivory in the zooplankton community and (ii) high herbivory in the zooplankton community, modified after McMeans et al. (2015).
Relationship Between δ15Nbulk and δ15NAA
There was a strong positive correlation between δ15Nbulk and δ15NPhe in ringed seals muscle from the CAA (linear model: p < 0.01, R2 = 59.2%; Figure 5A) and Baffin Island (linear model: p < 0.01, R2 = 69.6%; Figure 5B and Table 2). δ15Nbulk was not correlated with Cor-δ15Ntrophic at either location (Figures 5C,D and Table 2).
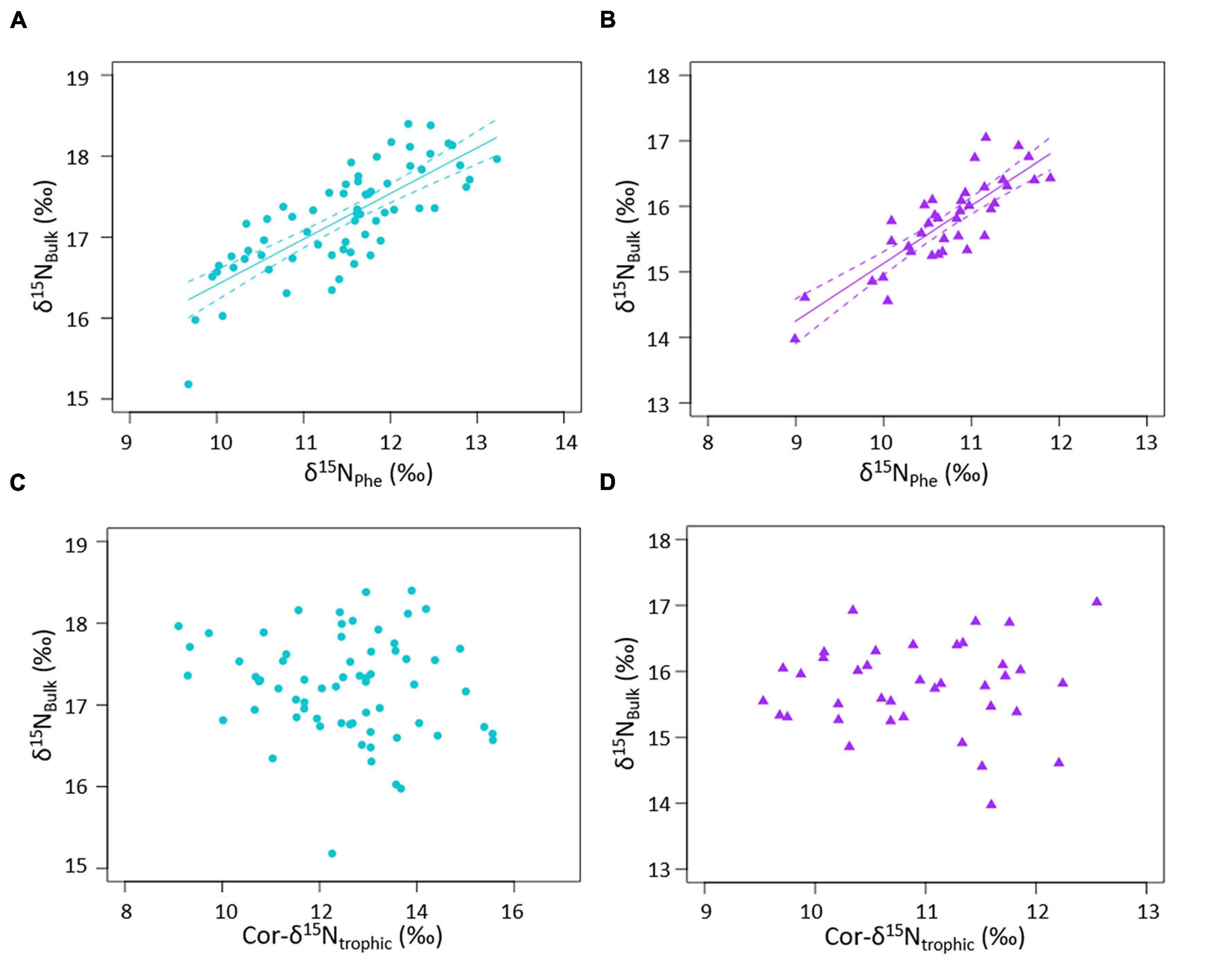
Figure 5. Relationship between δ15Nbulk and δ15NAA. δ15Nbulk and δ15NPhe in per mil (‰) in ringed seals from (A) the Canadian Arctic Archipelago (CAA; turquoise circles) and (B) Baffin Island (purple triangles), and relationship between δ15Nbulk and Cor-δ15Ntrophic in ringed seals from (C) the CAA and (D) Baffin Island; solid lines show significant linear models (p < 0.01, Table 2), dashed lines show 95% confidence intervals of the linear models.
Discussion
Temporal Trends in δ13Cbulk
The annual decline in δ13Cbulk in ringed seals of −0.029‰ year–1 in the CAA and −0.021‰ year–1 in the Baffin Island region (Figures 3A,B) is in accordance with the temporal decline in δ13Cbulk of −0.020 ± 0.003‰ year–1 in Northern fur seals from the Bering Sea (Newsome et al., 2007) and of −0.021 ± 0.006‰ year–1 in beluga whales from Baffin Bay (Matthews and Ferguson, 2014). However, these trends need to be interpreted with caution, as a few points were driving the trends in both the CAA (two points in 1992 and two points in 2015) and the Baffin Island region (two points in 1990; Supplementary Material). This decline is likely due to a combination of several factors. First, enhanced emission of anthropogenic carbon dioxide (CO2), which is 13C-deplete relative to natural CO2 (Quay et al., 2003), has led to the increase in oceanic CO2 (Sabine et al., 2004) and a decline in δ13C of dissolved inorganic carbon (δ13CDIC). This is known as the Suess effect. δ13CDIC is fractionated by phytoplankton during photosynthesis and represents the δ13C baseline in marine food webs (Young et al., 2013). In the Arctic, the decline in δ13CDIC is estimated to be in average −0.011‰ year–1 and is transferred through the food web to predators (de la Vega et al., 2019). Second, sea ice derived carbon is generally 13C-enriched compared to phytoplankton derived carbon (Hobson et al., 1995; de la Vega et al., 2019). The strong sea ice decline alongside increased OWP in the CAA (Figures 2A,B) could result in a decreased contribution of 13C-enriched sea-ice sympatric production versus 13C-depleted pelagic phytoplankton, leading to an overall 13C-depleted carbon pool entering the food web in the high-Arctic (Yurkowski et al., 2020). Third, climate change has led to increased coastal erosion, river flow and melting of permafrost in the Canadian Arctic (MacDonald and Birchall, 2020; Terhaar et al., 2021). This could result in an increased influence of 13C-depleted terrestrial organic matter (Boutton, 1991) into the surrounding marine environment, contributing to the decline in δ13Cbulk in ringed seals from both the CAA and the Baffin Island region.
Temporal Trends in the δ15N Baseline
The strong positive correlation between δ15Nbulk and δ15NPhe, and the lack of correlation between δ15Nbulk and Cor-δ15Ntrophic (Figure 5), demonstrates that temporal changes in δ15Nbulk of ringed seals from both the CAA and Baffin Island was mainly driven by change in the δ15N at the baseline. The δ15N baseline in the marine environment is represented by the δ15N of nitrate, an essential nutrient taken up by phytoplankton (Mariotti et al., 1981). Phytoplankton take on the isotopic signature of nitrate, to create the particulate organic matter that forms the base of marine food webs. Nitrate is supplied to the Arctic mainly by Pacific water, entering via the Bering Strait, and Atlantic water, entering via the Barents Sea and Fram Strait (Torres-Valdés et al., 2013), and to a lesser extent via rivers (McClelland et al., 2007; Fouest et al., 2013). Water column denitrification in the Pacific Ocean leaves an enriched imprint on δ15N of nitrate, resulting in a 2–3‰ 15N-enrichment end-member in the Pacific Arctic inflow compared to the Atlantic Arctic inflow (Somes et al., 2010). The δ15N of particulate organic matter depends on the δ15N of nitrate, and is also influenced by fractionation during primary production (Sigman et al., 2009) and bacterial activity (Morata et al., 2008).
The increasing trend in δ15NPhe in ringed seals from both the CAA and Baffin Island (Figures 3C,D) could be explained by several processes. First, the Pacific inflow through the Bering Strait has increased by more than 50% as a result of warming and increased winds (Woodgate, 2018). In conjunction, the Beaufort gyre has expanded in areal extent and sea surface height (Regan et al., 2019), increasing the surface slope between the Canadian Basin and Baffin Bay (Michel et al., 2006). Although the downstream effects of these changes are not well known, model predictions suggest that the export of water from the Beaufort Gyre through the CAA is increasing and will continue to increase into the coming decades, influencing the surface waters as far South as the Labrador Sea (McGeehan and Maslowski, 2012). The increasing influence of 15N-enriched Pacific derived water in the CAA and Baffin Bay might explain the increasing trend in δ15NPhe in ringed seals from the CAA and the similar but less pronounced increasing trend in ringed seals from Baffin Island, which could be a result of a dilution effect of the Pacific water influence when reaching Southern Baffin Bay.
Second, benthic denitrification in the western Arctic and Bering Sea has also been shown to impart a 15N-enriched signature in the overlying water column, known as the “sedimentary isotope effect” (Granger et al., 2011; Fripiat et al., 2018). Previous work has suggested that increasing net primary production on Arctic shelves would increase organic matter supply to sediments, leading to increased benthic denitrification rates (Arrigo and van Dijken, 2015), resulting in an enrichment in 15N of water column nitrate. The increasing trend in δ15NPhe in ringed seals from the CAA and Baffin Island could reflect the effect of increasing primary production and in turn benthic denitrification, either occurring locally (i.e., within the CAA and the Baffin Island shelf), or occurring in the Beaufort Sea and the Canadian Basin (Lewis et al., 2020), the resulting 15N-enriched water column nitrate being subsequently transported via the Arctic outflow through the CAA. Finally, increasing bacterial degradation activity with increasing temperature in the Arctic (Vaqué et al., 2019) may also increase the δ15N values of organic matter entering the food web (Morata et al., 2008).
The difference in the δ15N at the baseline between the early 1990s and the mid-2010 was ∼1.5‰ in ringed seals from the CAA. This is close to representing an increase of half a trophic position, as trophic discrimination factors for trophic amino acids in marine tertiary and higher consumers range from 2.5 to 4.3‰ (McMahon and McCarthy, 2016). Not accounting for this change in the δ15N at the baseline would lead to misestimation of ringed seal trophic position using δ15Nbulk alone (de la Vega et al., 2020). This highlights the power of using Cor-δ15Ntrophic values to assess temporal trend in trophic position of ringed seals from the Canadian Arctic, especially when the δ15N at the baseline is increasing at varying rates by latitude.
Temporal Trend in Food Web Structure
In the CAA, the decrease in Cor-δ15Ntrophic in ringed seals of ∼1.5‰ (Figure 4A) represents a change of approximately half a trophic level (McMahon and McCarthy, 2016). This could be explained by a change in diet, for example from a more piscivorous to an omnivorous diet consisting of more invertebrates. Conversely, the decrease in the difference between δ15NGlu–δ15NPhe (Cor-δ15Ntrophic) could also suggest a change in the ringed seals diet from a “low quality diet” (e.g., omnivorous diet consisting of more invertebrates) to a “high quality diet” (e.g., more piscivorous diet), as fractionation of individual amino acids is influenced by the degree of similarity in amino acid composition between the prey and consumers (McMahon and McCarthy, 2016). Currently there is no direct documented evidence to support this diet shift as ringed seals collected from the CAA primarily consume Arctic cod (Yurkowski et al., 2016a). A change in the diet of their prey items, resulting in change in amino acid composition of these prey, could also influence the Cor-δ15Ntrophic values of ringed seals. For example, the decrease in Arctic sea ice might affect the feeding patterns and prey composition of Arctic cod (Matley et al., 2013) potentially modifying their amino acid composition, but such change is not currently documented.
Alternatively, the decreasing Cor-δ15Ntrophic could reflect a change near the base of the food chain which indirectly influences the trophic position of the ringed seals. The copepods Calanus glacialis and Calanus finmarchicus, and the amphipod, Themisto libellula, are key components of the Arctic and sub-Arctic food webs, constituting the main trophic links to Arctic cod, and predators such as seabirds and marine mammals (Dalpadado et al., 2016; Møller and Nielsen, 2020). In the Barents Sea and West Greenland, increasing sea water temperature and decreased sea ice extent, has led to an increase in the relative abundance of the smaller herbivorous Atlantic crustacean species, C. finmarchicus compared to the large omnivorous and carnivorous Arctic crustacean species, e.g., C. glacialis and T. libellula (Dalpadado et al., 2016; Møller and Nielsen, 2020). Warming in the CAA, reflected in the strong decrease in sea ice concentration and increase of OWP (Figure 2), might have led to a decrease in omnivory versus herbivory in the zooplankton communities, resulting in a shortened food chain length (Figure 4B; McMeans et al., 2015) in the high-Arctic, reflected in the gradual decrease in Cor-δ15Ntrophic values of ringed seals from the CAA since 1992.
It is likely that such changes in zooplankton dynamics also occurred at lower latitudes. The CAA is typically more ice-covered throughout the year and has exhibited stronger environmental change compared to Southern Baffin Bay (Figure 2), and Hudson Bay since 1990 (Ferguson et al., 2020). The consistency in Cor-δ15Ntrophic values of ringed seals from Baffin Island in the last 25 years suggests that the food web structure in Southern Baffin Bay has remained stable since the 1990s. The similar Cor-δ15Ntrophic values in ringed seals from the two regions in 2015–2016, in contrast with the 15N-enriched Cor-δ15Ntrophic values in ringed seals from the CAA compared to those from the Baffin Island in 1992–1993 (Figure 4A) suggests that the food chain length in the CAA became similar to the food chain length in the Baffin Bay in recent years in response to environmental change. This observation supports latitudinal differences in the onset and rate of environmental changes, and in turn borealization between mid- and high-Arctic, and suggests that the high-Arctic responded more recently than the mid-Arctic. We have not been able to determine the onset and rate of borealization of the food chain in the mid-Arctic in this study, because access to sample archives or data from earlier than the 1990s would be required.
As the Arctic continues to warm, borealization of the Arctic will likely continue, with consequences for ringed seal population dynamics. Firstly, dietary analyses of Arctic predators such as sea birds, beluga whales, and harp seals, have suggested a recent increase of capelin in Southern Baffin Bay, coinciding with a decreased availability of Arctic cod (Gaston et al., 2012; Yurkowski et al., 2018). A shift in prey fish composition from Arctic cod to capelin is unlikely to reduce the food quality of the prey available to marine predators (Pedro et al., 2019). However, ringed seals from the Canadian Arctic have continued to feed primarily on Arctic cod despite high availability of capelin (Ogloff et al., 2019). In the Northern Barents Sea, ringed seals have also maintained their dietary preferences for arctic prey species in a restricted foraging habitat at an increased energetic expense (Hamilton et al., 2019; Bengtsson et al., 2020). Considering that Arctic prey might become less abundant, the selective pressure on ringed seals to modify or adapt their foraging strategies to consume more boreal species might increase, leading to higher energetical stress. Second, species shift in Calanus communities, which have recently become dominated by less lipid-rich smaller individuals, decreases the availability of energy rich lipids for higher trophic level species leading to negative impacts on their body condition (Møller and Nielsen, 2020), with consequences for reproduction (Ferguson et al., 2017). This is in accordance with the decreasing blubber thickness of ringed seals from the CAA since 1990 (Harwood et al., 2020), and coincides with our finding of a decreasing trophic position over time. Ferguson et al. (2020) forecasted reproductive difficulties and higher mortality for ringed seals in the low Arctic, which showed greater seasonal fluctuation in body condition compared to ringed seals from the high-Arctic. This could be amplified by the longer exposure of ringed seals to the less lipid-rich food web in the mid-Arctic compared to ringed seals in the CAA. Management and implementation of protection measures in the Arctic should take into account the stage and state of adaptation of each ecosystem, as we observe and suggest here, that the high-Arctic has become increasingly “borealized” during the last two decades, whilst the food chain in the mid-Arctic remained stable for the last 25 years of monitoring.
Data Availability Statement
The datasets presented in this study can be found in online repositories. The names of the repository/repositories and accession number(s) can be found below: De la Vega, C., Kershaw, J., Yurkowski, D. J., Ferguson, S. H., Stenson, G. B., Haug, T., Biuw, M., Frie, A. K., Smout, S. C., Jeffreys, R. M., and Mahaffey, C. (2021). ARISE project–Work package 3: stable nitrogen isotopes of bulk tissue and amino-acids of ringed seals muscle and teeth’s growth layer groups of harp seals from the Arctic and sub-Arctic (Version 1.0) (Data set). NERC EDS UK Polar Data Centre. https://doi.org/10.5285/6AAA53E8-3D0A-48FE-838E-31C5B5F24CE7.
Ethics Statement
Ethical review and approval was not required for the animal study because all tissue samples were acquired through Fisheries and Oceans Canada Licences to Fish for Scientific Purposes.
Author Contributions
CV, CM, and RJ designed the study. DY and SF collected the samples. CV, LN, and ES analyzed the samples. CV performed the data and statistical analyses and wrote the first draft of the manuscript. CM, DY, SS, SF, and RJ contributed substantially to the revisions. All authors contributed to the article and approved the submitted version.
Funding
This work resulted from the ARISE project (NE/P006035/1), part of the Changing Arctic Ocean program, funded by the UKRI Natural Environment Research Council (NERC).
Conflict of Interest
The authors declare that the research was conducted in the absence of any commercial or financial relationships that could be construed as a potential conflict of interest.
Publisher’s Note
All claims expressed in this article are solely those of the authors and do not necessarily represent those of their affiliated organizations, or those of the publisher, the editors and the reviewers. Any product that may be evaluated in this article, or claim that may be made by its manufacturer, is not guaranteed or endorsed by the publisher.
Acknowledgments
We thank Jim Ball for his help in the isotopic lab in University of Liverpool. We also thank Wesley Ogloff, and the Resolute Bay and Pangnirtung Hunters, and Trappers Associations and their hunters for sample collection.
Supplementary Material
The Supplementary Material for this article can be found online at: https://www.frontiersin.org/articles/10.3389/fmars.2021.700687/full#supplementary-material
Footnotes
- ^ http://iceweb1.cis.ec.gc.ca/IceGraph, accessed July 2021
References
Arrigo, K. R., and van Dijken, G. L. (2015). Continued increases in Arctic Ocean primary production. Prog. Oceanogr. 136, 60–70. doi: 10.1016/j.pocean.2015.05.002
Bengtsson, O., Lydersen, C., Kovacs, K. M., and Lindstrøm, U. (2020). Ringed seal (Pusa hispida) diet on the west coast of spitsbergen, svalbard, norway: during a time of ecosystem change. Polar Biol. 43, 773–788. doi: 10.1007/s00300-020-02684-5
Boutton, T. W. (1991). “Stable carbon isotope ratios of natural materials: II. Atmospheric, terrestrial, marine and freshwater environments,” in Carbon Isotope Techniques, ed. D. C. C. B. Fry (San Diego, CA: Academic Press), 173–186. doi: 10.1016/B978-0-12-179730-0.50016-3
Carmack, E., and Wassmann, P. (2006). Food webs and physical–biological coupling on pan-Arctic shelves: unifying concepts and comprehensive perspectives. Prog. Oceanogr. 71, 446–477. doi: 10.1016/j.pocean.2006.10.004
Dalpadado, P., Hop, H., Rønning, J., Pavlov, V., Sperfeld, E., Buchholz, F., et al. (2016). Distribution and abundance of euphausiids and pelagic amphipods in kongsfjorden, isfjorden and rijpfjorden (Svalbard) and changes in their relative importance as key prey in a warming marine ecosystem. Polar Biol. 39, 1765–1784. doi: 10.1007/s00300-015-1874-x
de la Vega, C., Jeffreys, R. M., Tuerena, R., Ganeshram, R., and Mahaffey, C. (2019). Temporal and spatial trends in marine carbon isotopes in the Arctic Ocean and implications for food web studies. Glob. Change Biol. 25, 4116–4130. doi: 10.1111/gcb.14832
de la Vega, C., Mahaffey, C., Tuerena, R. E., Yurkowski, D. J., Ferguson, S. H., Stenson, G. B., et al. (2020). Arctic seals as tracers of environmental and ecological change. Limnol. Oceanogr. Lett. 6, 24–32. doi: 10.1002/lol2.10176
Ferguson, S. H., Young, B. G., Yurkowski, D. J., Anderson, R., Willing, C., and Nielsen, O. (2017). Demographic, ecological, and physiological responses of ringed seals to an abrupt decline in sea ice availability. PeerJ 5:e2957. doi: 10.7717/peerj.2957
Ferguson, S. H., Yurkowski, D. J., Young, B. G., Fisk, A. T., Muir, D. C., Zhu, X., et al. (2020). Comparing temporal patterns in body condition of ringed seals living within their core geographic range with those living at the edge. Ecography 43, 1521–1535. doi: 10.1111/ecog.04988
Fossheim, M., Primicerio, R., Johannesen, E., Ingvaldsen, R. B., Aschan, M. M., and Dolgov, A. V. (2015). Recent warming leads to a rapid borealization of fish communities in the Arctic. Nat. Clim. Chang. 5:673. doi: 10.1038/nclimate2647
Fouest, V. L., Babin, M., and Tremblay, J. -É (2013). The fate of riverine nutrients on Arctic shelves. Biogeosciences 10, 3661–3677. doi: 10.5194/bg-10-3661-2013
Fox, J., Friendly, G. G., Graves, S., Heiberger, R., Monette, G., Nilsson, H., et al. (2007). The Car Package. R Foundation for Statistical Computing.
Fripiat, F., Declercq, M., Sapart, C., Anderson, L., Brüchert, V., Deman, F., et al. (2018). Influence of the bordering shelves on nutrient distribution in the Arctic halocline inferred from water column nitrate isotopes. Limnol. Oceanogr. 63, 2154–2170. doi: 10.1002/lno.10930
Gaston, A. J., Smith, P. A., and Provencher, J. F. (2012). Discontinuous change in ice cover in Hudson Bay in the 1990s and some consequences for marine birds and their prey. ICES J. Mar. Sci. 69, 1218–1225. doi: 10.1093/icesjms/fss040
Granger, J., Prokopenko, M., Sigman, D. M., Mordy, C., Morse, Z., Morales, L., et al. (2011). Coupled nitrification-denitrification in sediment of the eastern Bering Sea shelf leads to 15N enrichment of fixed N in shelf waters. J. Geophys. Res. 116:C11006. doi: 10.1029/2010JC006751
Hamilton, C. D., Vacquié-Garcia, J., Kovacs, K. M., Ims, R. A., Kohler, J., and Lydersen, C. (2019). Contrasting changes in space use induced by climate change in two Arctic marine mammal species. Biol. Lett. 15:20180834. doi: 10.1098/rsbl.2018.0834
Harwood, L. A., Smith, T. G., Alikamik, J., Alikamik, E., Lea, E. V., Stirling, I., et al. (2020). Long-term, harvest-based monitoring of ringed seal body condition and reproduction in Canada’s western arctic: an update through 2019. Arctic 73, 206–220. doi: 10.14430/arctic70428
Hobson, K. A., Ambrose, W. G. Jr., and Renaud, P. E. (1995). Sources of primary production, benthic-pelagic coupling, and trophic relationships within the Northeast Water Polynya: insights from δ13C and δ15N analysis. Mar. Ecol. Prog. Ser. 128, 1–10. doi: 10.3354/meps128001
Kortsch, S., Primicerio, R., Fossheim, M., Dolgov, A. V., and Aschan, M. (2015). Climate change alters the structure of arctic marine food webs due to poleward shifts of boreal generalists. Proc. R. Soc. B Biol. Sci. 282:20151546. doi: 10.1098/rspb.2015.1546
Lewis, K., Van Dijken, G., and Arrigo, K. R. (2020). Changes in phytoplankton concentration now drive increased Arctic Ocean primary production. Science 369, 198–202. doi: 10.1126/science.aay8380
MacDonald, S., and Birchall, S. J. (2020). Climate change resilience in the Canadian Arctic: the need for collaboration in the face of a changing landscape. Can. Geogr. 64, 530–534. doi: 10.1111/cag.12591
Mariotti, A., Germon, J., Hubert, P., Kaiser, P., Letolle, R., Tardieux, A., et al. (1981). Experimental determination of nitrogen kinetic isotope fractionation: some principles; illustration for the denitrification and nitrification processes. Plant Soil 62, 413–430. doi: 10.1007/BF02374138
Matley, J. K., Fisk, A. T., and Dick, T. A. (2013). The foraging ecology of Arctic cod (Boreogadus saida) during open water (July–August) in Allen Bay. Arctic Canada. Mar. Biol. 160, 2993–3004. doi: 10.1007/s00227-013-2289-2
Matthews, C. J., and Ferguson, S. H. (2014). Validation of dentine deposition rates in beluga whales by interspecies cross dating of temporal δ13C trends in teeth. NAMMCO Sci. Publications 10:3196. doi: 10.7557/3.3196
McClelland, J., Stieglitz, M., Pan, F., Holmes, R., and Peterson, B. (2007). Recent changes in nitrate and dissolved organic carbon export from the upper Kuparuk River, North Slope, Alaska. J. Geophy. Res. Biogeosci. 112:G04S60. doi: 10.1029/2006JG000371
McGeehan, T., and Maslowski, W. (2012). Evaluation and control mechanisms of volume and freshwater export through the Canadian Arctic Archipelago in a high-resolution pan-Arctic ice-ocean model. J. Geophys. Res. 117:C00D14. doi: 10.1029/2011JC007261
McMahon, K. W., and McCarthy, M. D. (2016). Embracing variability in amino acid δ15N fractionation: mechanisms, implications, and applications for trophic ecology. Ecosphere 7:e01511. doi: 10.1002/ecs2.1511
McMeans, B. C., McCann, K. S., Humphries, M., Rooney, N., and Fisk, A. T. (2015). Food web structure in temporally-forced ecosystems. Trends Ecol. Evol. 30, 662–672. doi: 10.1016/j.tree.2015.09.001
Meredith, M., Sommerkorn, M., Cassotta, S., Derksen, C., Ekaykin, A., and Hollowed, A., et al. (eds) (2019). “Polar regions,” in IPCC Special Report on the Ocean and Cryosphere in a Changing Climate, eds H. O. Pörtner, D. C. Roberts, V. Masson-Delmotte, P. Zhai, M. Tignor, E. Poloczanska, et al. (Geneva: IPCC).
Michel, C., Ingram, R., and Harris, L. (2006). Variability in oceanographic and ecological processes in the Canadian Arctic Archipelago. Prog. Oceanogr. 71, 379–401. doi: 10.1016/j.pocean.2006.09.006
Møller, E. F., and Nielsen, T. G. (2020). Borealization of Arctic zooplankton—smaller and less fat zooplankton species in Disko Bay, Western Greenland. Limnol. Oceanogr. 65, 1175–1188. doi: 10.1002/lno.11380
Morata, N., Renaud, P. E., Brugel, S., Hobson, K. A., and Johnson, B. J. (2008). Spatial and seasonal variations in the pelagic–benthic coupling of the southeastern Beaufort Sea revealed by sedimentary biomarkers. Mar. Ecol. Prog. Ser. 371, 47–63. doi: 10.3354/meps07677
Newsome, S., Etnier, M., Kurle, C., Waldbauer, J., Chamberlain, C., and Koch, P. (2007). Historic decline in primary productivity in western Gulf of Alaska and eastern Bering Sea: isotopic analysis of northern fur seal teeth. Mar. Ecol. Prog. Ser. 332, 211–224. doi: 10.3354/meps332211
Nielsen, J. M., Popp, B. N., and Winder, M. (2015). Meta-analysis of amino acid stable nitrogen isotope ratios for estimating trophic position in marine organisms. Oecologia 178, 631–642. doi: 10.1007/s00442-015-3305-7
Ogloff, W. R., Yurkowski, D. J., Davoren, G. K., and Ferguson, S. H. (2019). Diet and isotopic niche overlap elucidate competition potential between seasonally sympatric phocids in the Canadian Arctic. Mar. Biol. 166:103. doi: 10.1007/s00227-019-3549-6
Pecuchet, L., Blanchet, M. A., Frainer, A., Husson, B., Jørgensen, L. L., Kortsch, S., et al. (2020). Novel feeding interactions amplify the impact of species redistribution on an Arctic food web. Glob. Change Biol. 26, 4894–4906. doi: 10.1111/gcb.15196
Pedro, S., Fisk, A. T., Ferguson, S. H., Hussey, N. E., Kessel, S. T., and McKinney, M. A. (2019). Limited effects of changing prey fish communities on food quality for aquatic predators in the eastern Canadian Arctic in terms of essential fatty acids, methylmercury and selenium. Chemosphere 214, 855–865. doi: 10.1016/j.chemosphere.2018.09.167
Post, D. M. (2002a). The long and short of food-chain length. Trends Ecol. Evol. 17, 269–277. doi: 10.1016/S0169-5347(02)02455-2
Post, D. M. (2002b). Using stable isotopes to estimate trophic position: models, methods, and assumptions. Ecology 83, 703–718. doi: 10.1890/0012-9658(2002)083[0703:USITET]2.0.CO;2
Quay, P., Sonnerup, R., Westby, T., Stutsman, J., and McNichol, A. (2003). Changes in the 13C/12C of dissolved inorganic carbon in the ocean as a tracer of anthropogenic CO2 uptake. Glob. Biogeochem. Cycles 17:1004. doi: 10.1029/2001GB001817
R Core Team (2018). R: A Language and Environment for Statistical Computing. R Foundation for Statistical Computing. Vienna: R Core Team.
Regan, H. C., Lique, C., and Armitage, T. W. (2019). The beaufort gyre extent, shape, and location between 2003 and 2014 from satellite observations. J. Geophys. Res. 124, 844–862. doi: 10.1029/2018JC014379
Sabine, C. L., Feely, R. A., Gruber, N., Key, R. M., Lee, K., Bullister, J. L., et al. (2004). The oceanic sink for anthropogenic CO2. Science 305, 367–371. doi: 10.1126/science.1097403
Sigman, D. M., Karsh, K. L., and Casciotti, K. L. (2009). “Nitrogen isotopes in the ocean,” in Encyclopedia of Ocean sciences 2nd Edn, eds J. H. Steele, S. A. Thorpe, and K. K. Turekian (London: Academic), 40–54. doi: 10.1016/B978-012374473-9.00632-9
Somes, C. J., Schmittner, A., Galbraith, E. D., Lehmann, M. F., Altabet, M. A., Montoya, J. P., et al. (2010). Simulating the global distribution of nitrogen isotopes in the ocean. Glob. Biogeochem. Cycles 24:GB4019. doi: 10.1029/2009GB003767
Tang, C. C. L., Ross, C. K., Yao, T., Petrie, B., DeTracey, B. M., and Dunlap, E. (2004). The circulation, water masses and sea-ice of Baffin Bay. Prog. Oceanogr. 63, 183–228. doi: 10.1016/j.pocean.2004.09.005
Terhaar, J., Lauerwald, R., Regnier, P., Gruber, N., and Bopp, L. (2021). Around one third of current Arctic Ocean primary production sustained by rivers and coastal erosion. Nat. Commun. 12:169. doi: 10.1038/s41467-020-20470-z
Torres-Valdés, S., Tsubouchi, T., Bacon, S., Naveira-Garabato, A. C., Sanders, R., McLaughlin, F. A., et al. (2013). Export of nutrients from the Arctic Ocean. J. Geophys. Res. 118, 1625–1644. doi: 10.1002/jgrc.20063
Vander Zanden, M. J., Clayton, M. K., Moody, E. K., Solomon, C. T., and Weidel, B. C. (2015). Stable isotope turnover and half-life in animal tissues: a literature synthesis. PLoS One 10:e0116182. doi: 10.1371/journal.pone.0116182
Vaqué, D., Lara, E., Arrieta, J. M., Holding, J., Sà, E. L., Hendriks, I. E., et al. (2019). Warming and CO2 enhance arctic heterotrophic microbial activity. Front. Microbiol. 10:494. doi: 10.3389/fmicb.2019.00494
Wassmann, P. (2015). Overarching perspectives of contemporary and future ecosystems in the Arctic Ocean. Prog. Oceanogr. 139, 1–12. doi: 10.1016/j.pocean.2015.08.004
Woodgate, R. A. (2018). Increases in the Pacific inflow to the Arctic from 1990 to 2015, and insights into seasonal trends and driving mechanisms from year-round Bering Strait mooring data. Prog. Oceanogr. 160, 124–154. doi: 10.1016/j.pocean.2017.12.007
Young, J., Bruggeman, J., Rickaby, R., Erez, J., and Conte, M. (2013). Evidence for changes in carbon isotopic fractionation by phytoplankton between 1960 and 2010. Glob. Biogeochem. Cycles 27, 505–515. doi: 10.1002/gbc.20045
Yurkowski, D. J., Brown, T. A., Blanchfield, P. J., and Ferguson, S. H. (2020). Atlantic walrus signal latitudinal differences in the long-term decline of sea ice-derived carbon to benthic fauna in the Canadian Arctic. Proc. R. Soc. B Biol. Sci. 287:20202126. doi: 10.1098/rspb.2020.2126
Yurkowski, D. J., Ferguson, S. H., Semeniuk, C. A., Brown, T. M., Muir, D. C., and Fisk, A. T. (2016a). Spatial and temporal variation of an ice-adapted predator’s feeding ecology in a changing Arctic marine ecosystem. Oecologia 180, 631–644. doi: 10.1007/s00442-015-3384-5
Yurkowski, D. J., Hussey, A. J., Hussey, N. E., and Fisk, A. T. (2017). Effects of decomposition on carbon and nitrogen stable isotope values of muscle tissue of varying lipid content from three aquatic vertebrate species. Rapid Commun. Mass Spectrom. 31, 389–395. doi: 10.1002/rcm.7802
Yurkowski, D. J., Hussey, N. E., Ferguson, S. H., and Fisk, A. T. (2018). A temporal shift in trophic diversity among a predator assemblage in a warming Arctic. R. Soc. Open Sci. 5:180259. doi: 10.1098/rsos.180259
Yurkowski, D. J., Semeniuk, C. A., Harwood, L. A., Rosing-Asvid, A., Dietz, R., Brown, T. M., et al. (2016b). Influence of sea ice phenology on the movement ecology of ringed seals across their latitudinal range. Mar. Ecol. Prog. Ser. 562, 237–250. doi: 10.3354/meps11950
Keywords: borealization, Canadian Arctic, ringed seals, stable isotopes, amino acids, latitudes, trophic position, food web structure
Citation: de la Vega C, Mahaffey C, Yurkowski DJ, Norman L, Simpson E, Smout S, Ferguson SH and Jeffreys RM (2021) Biomarkers in Ringed Seals Reveal Recent Onset of Borealization in the High- Compared to the Mid-Latitude Canadian Arctic. Front. Mar. Sci. 8:700687. doi: 10.3389/fmars.2021.700687
Received: 26 April 2021; Accepted: 13 August 2021;
Published: 07 September 2021.
Edited by:
Dongyan Liu, East China Normal University, ChinaReviewed by:
Xiaotong Xiao, Ocean University of China, ChinaKirsteen M. MacKenzie, Institut Français de Recherche pour l’Exploitation de la Mer (IFREMER), France
Copyright © 2021 de la Vega, Mahaffey, Yurkowski, Norman, Simpson, Smout, Ferguson and Jeffreys. This is an open-access article distributed under the terms of the Creative Commons Attribution License (CC BY). The use, distribution or reproduction in other forums is permitted, provided the original author(s) and the copyright owner(s) are credited and that the original publication in this journal is cited, in accordance with accepted academic practice. No use, distribution or reproduction is permitted which does not comply with these terms.
*Correspondence: Camille de la Vega, Y2FtaWxsZS5kZS1sYS12ZWdhQGxpdmVycG9vbC5hYy51aw==
†Present address: Camille de la Vega, Leibniz Institute for Baltic Sea Research, Warnem nde, Germany