- 1Marine Botany, Faculty of Biology and Chemistry and MARUM, University of Bremen, Bremen, Germany
- 2Alfred Wegener Institute, Helmholtz Centre for Polar and Marine Research, Bremerhaven, Germany
- 3Department of Algae Production, Division of Biotechnology and Plant Health, Norwegian Institute of Bioeconomy Research (NIBIO), Ås, Norway
- 4Division of Food Production and Society, Norwegian Institute of Bioeconomy Research (NIBIO), Bodø, Norway
- 5The Marine Science Institute, College of Science, University of the Philippines Diliman, Quezon City, Philippines
- 6Applied Ecology and Phycology, Institute of Biological Sciences, University of Rostock, Rostock, Germany
Kelps are important foundation species in coastal ecosystems currently experiencing pronounced shifts in their distribution patterns caused by ocean warming. While some populations found at species’ warm distribution edges have been recently observed to decline, expansions of some species have been recorded at their cold distribution edges. Reduced population resilience can contribute to kelp habitat loss, hence, understanding intraspecific variations in physiological responses across a species’ latitudinal distribution is crucial for its conservation. To investigate potential local responses of the broadly distributed kelp Saccharina latissima to marine heatwaves in summer, we collected sporophytes from five locations in Europe (Spitsbergen, Bodø, Bergen, Helgoland, Locmariaquer), including populations exposed to the coldest and warmest local temperature regimes. Meristematic tissue from sporophytes was subjected to increasing temperatures of Δ+2, Δ+4 and Δ+6°C above the respective mean summer temperatures (control, Δ±0°C) characteristic for each site. Survival and corresponding physiological and biochemical traits were analyzed. Vitality (optimum quantum yield, Fv/Fm) and growth were monitored over time and biochemical responses were measured at the end of the experiment. Growth was highest in northern and lowest in southern populations. Overall, northern populations from Spitsbergen, Bodø and Bergen were largely unaffected by increasing summer temperatures up to Δ+6°C. Conversely, sporophytes from Helgoland and Locmariaquer were markedly stressed at Δ+6°C: occurrence of tissue necrosis, reduced Fv/Fm, and a significantly elevated de-epoxidation state of the xanthophyll cycle (DPS). The variations in phlorotannins, mannitol and tissue C and N contents were independent of temperature treatments and latitudinal distribution pattern. Pronounced site-specific variability in response to increasing temperatures implies that exceeding a threshold above the mean summer temperature exclusively affect rear-edge (southernmost) populations.
Introduction
Temperature is a major factor controlling global biogeographic patterns of many marine benthic organisms, such as macroalgae (Lüning, 1990; Adey and Steneck, 2001; Wiencke and Bischof, 2012). Hence, global warming will likely provoke shifts in the latitudinal distribution of algal species (King et al., 2018; Smale, 2020). Changes in temperature regimes, such as an increase in frequency and amplitude of marine heatwaves (MHWs), often impair primary production or even survival of macroalgae, with consequences for standing stock biomass or even loss of important foundation communities as well as the shift into a novel ecosystem status (Frölicher et al., 2018; Harris et al., 2018; Smale et al., 2019; and references therein), such as shifts to turf algae communities (Wernberg et al., 2013, 2016a). Sea surface temperature (SST) has increased by 0.63°C globally in the period 1986–2005 compared to 1850–1900 (IPCC, 2019a). However, on a regional scale SSTs rose even higher, for example, on Helgoland (North Sea) > 1.6°C between 1962 and 2010 (Wiltshire et al., 2010). Under different climate change scenarios, the global mean SST is likely to increase by 1.6°C up to more than 4°C until the end of this century and additionally MHWs are expected to become more severe (Frölicher et al., 2018; IPCC, 2019a). MHWs are defined as periods of SST increases of 3–5°C above the long-term mean, which can last from a few days to months (Meehl and Tebaldi, 2004; Hobday et al., 2016). Worldwide, the annual duration of MHWs has already increased by 54% between 1925 and 2016 (Oliver et al., 2018).
Considering temperature tolerance limits, species with a wide latitudinal distribution range are generally more tolerant than narrow-ranging species (Wiencke et al., 1994; Kelly et al., 2012; Sunday et al., 2015). Among trees, species with a temperate to Arctic distribution will respond neutrally or even positively to future warming near their cold-range edge (Reich et al., 2015). This response might also apply to broadly distributed macroalgal species, such as kelps. In previous studies, different macroalgal populations were often treated as a single homogenous physiological unit, irrespective of their distribution (Reed et al., 2011). However, local adaptation and phenotypic plasticity can result in intraspecific differences in thermal tolerance, and population loss might occur not only among thermal rear edges but also in other regions (King et al., 2018). Recently, studies on the kelp Laminaria digitata, revealed that despite considerable plasticity at intermediate temperatures, plasticity at the upper survival limit was low (Liesner et al., 2020a, b; Franke et al., 2021). Thus, models assuming a uniform climatic envelope for wide-ranging species, particularly the local temperature threshold for survival, may underestimate local adaptation and extinction (Kelly et al., 2012; Filbee-Dexter et al., 2020).
Kelps are large canopy-forming brown algae of the order Laminariales (Bartsch et al., 2008). They are important primary producers and foundation species in coastal ecosystems providing habitat, nurseries and food for many associated organisms (Dayton, 1985; Bartsch et al., 2008). Alterations in distribution patterns of kelp forests in response to global climate change, including expansions and declines, were observed worldwide (Krumhansl et al., 2016). In Europe, species distribution models project a northward expansion of different kelp species (Müller et al., 2009; Assis et al., 2018). Newly exposed hard substrates in Arctic regions are already colonized by kelps (Krause-Jensen et al., 2020), whereas drastic loss of kelps is occurring at the southern distribution boundary (e.g., Voerman et al., 2013; Filbee-Dexter et al., 2020).
One widely distributed kelp along the European Atlantic is the boreal-temperate species Saccharina latissima, which is found from Arctic regions to northern Portugal (Araújo et al., 2016). The species exhibits a high degree of polymorphism and physiological plasticity, and is regarded to exhibit an opportunistic growth strategy (reviewed by Bartsch et al., 2008). Optimum growth of sporophytes is reported between 10 and 15°C (Bolton and Lüning, 1982). However, the sporophytes can briefly survive high temperatures of 23°C up to 1 week, but increasing mortality rates were already observed above 20°C (Fortes and Lüning, 1980; Bolton and Lüning, 1982; Lüning, 1984). In fact, the published information on upper survival temperatures derived from experiments without a pre-acclimation phase and may not reveal the actual resilience among wild populations where kelps are normally subjected to slowly increasing temperature. Nevertheless, Casado-Amezúa et al. (2019) report a decline in the S. latissima population along the north-western Iberian Coast during the last three decades, and presently, individuals are only rarely found in the North of Portugal (F. Arenas, pers. comm.). Massive alterations in population density and distribution pattern of European S. latissima populations were not only reported at their southern distribution range, but also in their intermediate northern locations, e.g., in the North Sea areas of Helgoland and Skagerrak, and along the southern and southwestern coasts of Norway (Pehlke and Bartsch, 2008; Bekkby and Moy, 2011; Moy and Christie, 2012).
Photosynthesis and growth of S. latissima is suboptimal at low temperatures (Bolton and Lüning, 1982; Karsten, 2007) while exposure to high and sublethal temperatures can result to biomass loss when, e.g., photosynthetic apparatus and other cellular structures are damaged (Andersen et al., 2013; Simonson et al., 2015b) leading to reduced growth. Aside from physiological acclimation, kelps and other seaweeds also use different biochemical protective mechanisms to adjust to temperature variations, such as adjustments in the xanthophyll cycle (de-epoxidation, DPS; Goss and Jakob, 2010).
The general impacts of MHWs on kelps and other foundation seaweeds have been intensively studied (e.g., Bennett et al., 2015; Burdett et al., 2019; Nepper-Davidsen et al., 2019; Straub et al., 2019; Saha et al., 2020), while there are only few studies on thermal plasticity of species across their latitudinal distribution (Winters et al., 2011; Jueterbock et al., 2014; Pereira et al., 2015; Wernberg et al., 2016b; Liesner et al., 2020a). Besides local variations in temperature, variable local thermal susceptibility may contribute to the mortality of European S. latissima, as suggested for other kelps (Wernberg et al., 2010; King et al., 2018). For instance, Wernberg et al. (2016b) showed in a common garden experiment that independent of their latitudinal distribution, the optimum photosynthetic temperature remained the same in different seaweeds in Australia, while Q10-values for photosynthesis and respiration and also chlorophyll a concentrations decreased from cooler to warmer locations. Bennett et al. (2019) and Filbee-Dexter et al. (2020) emphasized the relevance of understanding intraspecific variations in ecology, and until now, most experimental studies on temperature stress across latitudes did not consider the different respective local temperatures.
Contrary to the usual common garden experiments, our experimental design aims to reveal the responses of S. latissima to local MHWs. Therefore, we conducted a short-term experiment using wild sporophytes collected from five locations along the European Atlantic coast (i.e., Spitsbergen, Bodø, Bergen, Helgoland, Locmariaquer). We investigated whether different amplitudes of summer MHWs across latitudes will have differential effects on distinct S. latissima populations or not. In our mechanistic experimental set-up we increased the respective local mean summer temperature (control, Δ±0°C) by Δ+2, Δ+4 and Δ+6°C to investigate the relative responses of different kelp populations to MHWs over a large geographical scale.
We hypothesized that the tolerance of European S. latissima to summer MHWs, ranging from Δ+2 to Δ+6°C, is related to the mean summer temperature experienced in situ. Since the maximum temperature during summer in northern populations is lower than their physiological limit (Bolton and Lüning, 1982; Lüning, 1984), we expect these populations at their expanding edge to benefit or be unaffected (neutral response) by the temperature-amplitude treatments. In contrast, among the rear-edge populations at the southern limit, we expect them to suffer physiological stress from the temperature increase, i.e., when experiencing temperatures higher than the lethal limit determined for S. latissima (Bolton and Lüning, 1982; Lüning, 1984).
Materials and Methods
Sampling and Experimental Design
Sporophytes (> 1 m) of Saccharina latissima were collected in Spitsbergen (Ny-Ålesund, Norway), Bodø (North Norway), Bergen (South Norway), Helgoland (German Bight, Germany) and Locmariaquer (Brittany, France) in June 2018, 2019 and early July 2020 (Figure 1A and Table 1). The map (data copyrights: EuroGeographics for the administrative boundaries) was generated using QGIS 3.8.2-Zanzibar software (QGIS Development Team, 2019).
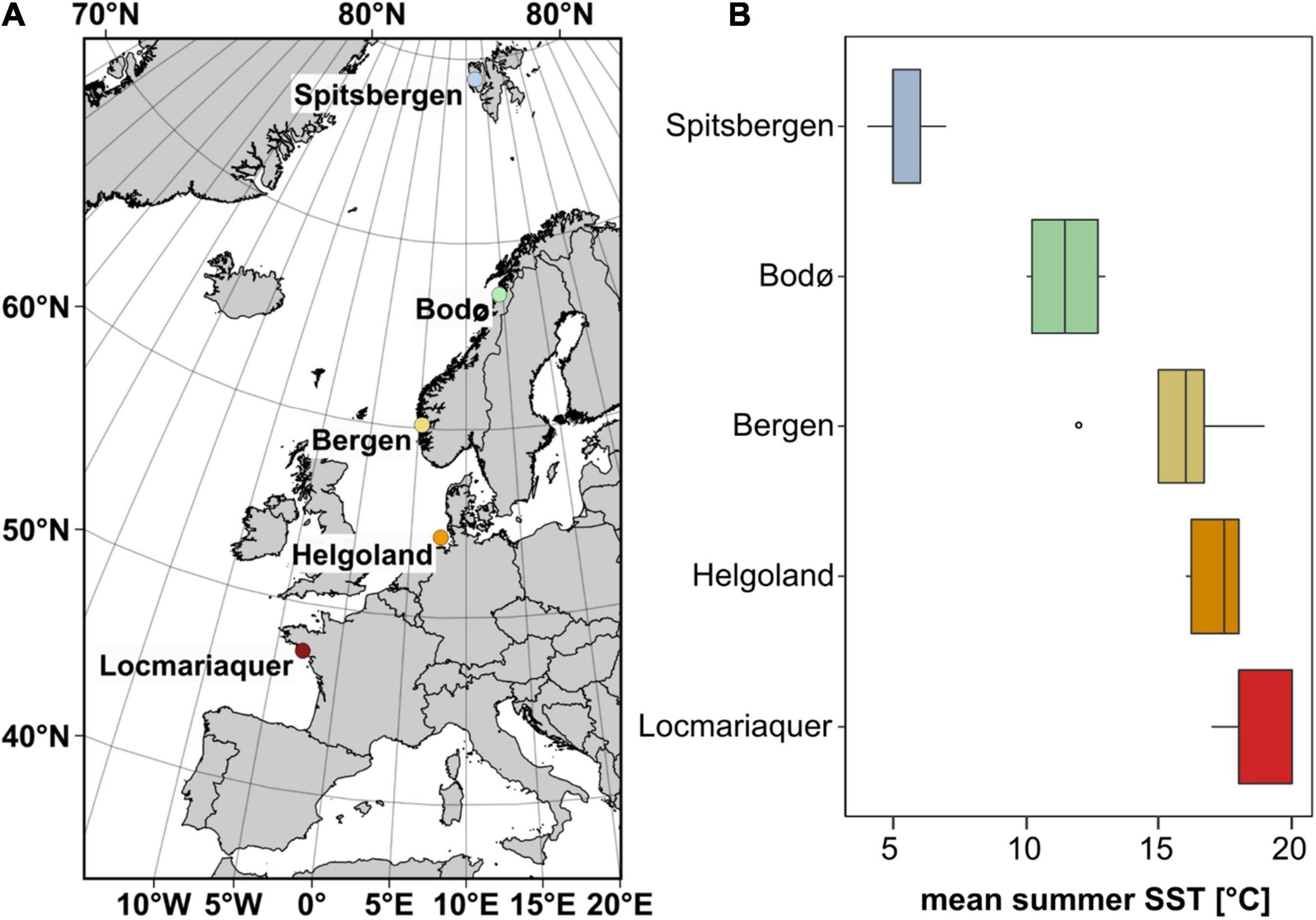
Figure 1. (A) Sampling locations of Saccharina latissima (map data: EuroGeographics for the administrative boundaries) and (B) mean summer (June–August) sea surface temperature (SST) from 2016 to 2020 at each sampling location based on satellite-obtained mean monthly SST datasets (Giovanni Satellite: Acker and Leptoukh, 2007).
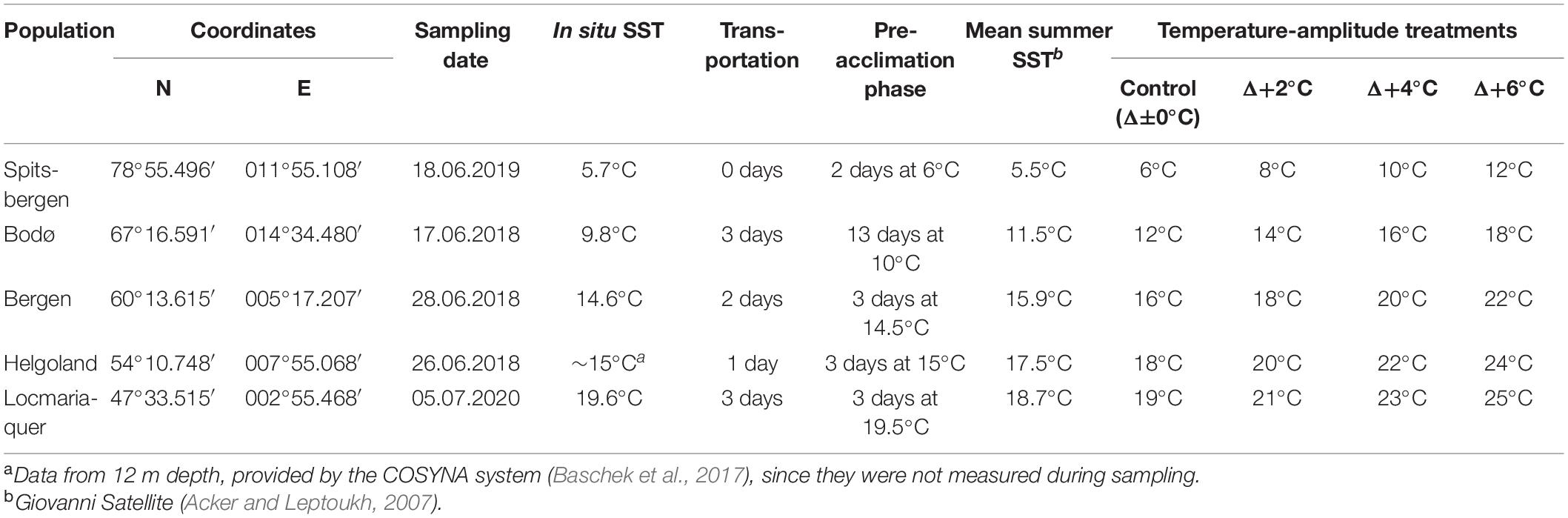
Table 1. Summary of sampling of Saccharina latissima: population, North-East coordinates, in situ sea surface temperature (SST) measured while collecting on sampling day, duration of sample transportation, duration and temperature of pre-acclimation phase, satellite-obtained mean summer SST (2016–2020) and respective absolute temperatures of the temperature-amplitude treatments.
Meristematic discs (diameter 22–24 mm) from 20 to 25 non-fertile sporophytes were excised within the 2–10 cm length of the basal lamina. Samples from Bodø, Bergen, Helgoland and Locmariaquer were transported moist, cool (< 15°C) and dark (Table 1). All the experiments, except for the Spitsbergen material, were conducted at the Alfred Wegener Institute for Polar and Marine Research in Bremerhaven, Germany. The Spitsbergen samples were processed locally at Ny-Ålesund and no transportation of the samples was necessary. For recovery and wound healing (pre-acclimation phase, Figure 2), the samples were maintained in the control seawater temperature (Table 1). Due to logistic issues, the pre-acclimation phase varied between the sampling sites (Spitsbergen: 2 days, Bodø: 13 days, Bergen: 3 days, Helgoland: 3 days, Locmariaquer: 3 days).
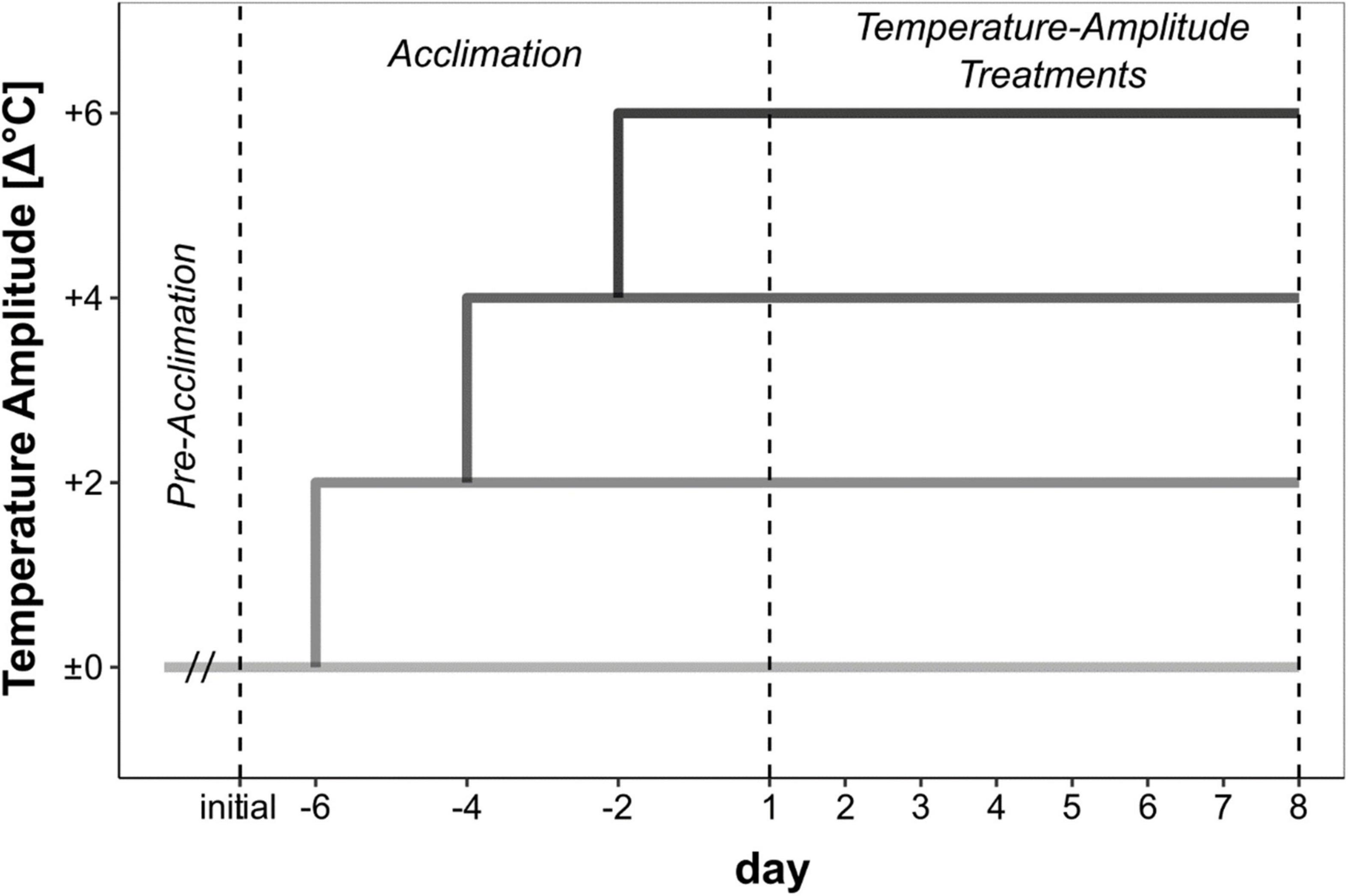
Figure 2. Set-up of the temperature-amplitude experiment with Saccharina latissima. Pre-acclimation phase varied between the sampling sites due to logistic constraints. During the acclimation phase, the control temperature (Δ±0°C) was increased by 2°C every second day to reach the temperature-amplitude treatments (Δ+2–Δ+6°C). Sampling for biochemical analyses was conducted on day 8 of the temperature-amplitude treatments.
During the pre-acclimation phase, four pools of discs were prepared in separate flasks to represent the four replicates. Each pool consisted of 10 meristematic discs excised from five distinct sporophytes, this means: twenty distinct sporophytes were used to ensure the independence of each replicate when representative discs from each pool were harvested during subsequent sampling to measure respective response variables.
In the acclimation phase (Figure 2), the discs were successively acclimated to different temperature amplitudes (Δ+2, Δ+4, Δ+6°C) with an increase of 2°C every second day. Afterwards, the final temperature-amplitude treatments were applied for another week. Respectively, the highest temperature-amplitude treatment (Δ+6°C) run for 9 days, while Δ+4°C run for 11 days and Δ+2°C for 13 days, according to the treatment scheme (Figure 2). We based the different absolute temperatures on the averaged mean summer sea surface temperatures (SST) at each sampling site (Table 1), using the respective mean summer SST as control (Δ±0°C). Figure 1B displays the satellite-obtained mean summer (June–August) SST between 2016 and 2020 with a resolution of 4 × 4 km at each sampling site (Giovanni Satellite: Acker and Leptoukh, 2007).
During the entire cultivation, acclimation and temperature exposure, samples were kept in aerated 2-L clear plastic bottles at 30–35 μmol photons m–2 s–1 (16:8 LD, ProfiLux 3 with LED Mitras daylight 150, GHL Advanced Technology, Kaiserslautern, Germany and Econlux, SolarStringer LED SunStrip “daylight,” Cologne, Germany) and in ½ Provasoli-enriched seawater (½ PES, Provasoli, 1968, modifications: HEPES-buffer instead of Tris, double concentration of Na2glycerophosphate, iodine enrichment after Tatewaki, 1966). Water was exchanged twice a week.
The integrity of all replicate discs was monitored to measure survival, vitality (Fv/Fm) and growth during the temperature exposure treatments. After the acclimation phase, first sampling was conducted (day 1, data not shown). Five discs were kept for the temperature-amplitude treatments which then run for 1 week (day 1–8, Figure 2). For biochemical analyses samples were shock frozen in liquid N2, stored at −80°C, and freeze-dried (Alpha 1–4 LO plus, Martin Christ Gefriertrocknungsanlagen GmbH, Osterode am Harz, Germany). Statistical evaluation (data not shown) did not reveal any relevant significant differences in the biochemical response parameters between day 1 and day 8. Therefore, this study exclusively presents biochemical results after the temperature-amplitude treatments (day 8).
Physiological Response Variables
At the end of the experiment, all discs were qualified according to their physical state as healthy or dead. A disc was categorized as “dead” when tissue necrosis was observed (Supplementary Figure 1). Disc survival (%) at day 8 was computed as:
For growth analysis, the discs were photographed (Figure 2: initial, day 1, 2, 4, 6, 8) together with a known 9 cm2 size object a reference. The algal disc size was then analyzed using the ImageJ (Version 1.52a, Java 1.8.0_112, Wayne Rasband, National Institute of Health, United States) software. As the samples grew with different rates prior to the temperature-amplitude treatments, the initial size of the replicates was adjusted to 100% and the increase in size calculated as % of initial for a better comparison of algal growth. Decomposing discs were excluded from the growth analyses.
As proxy for photosynthetic performance and, hence, of algal vitality, the in vivo chlorophyll-fluorescence of photosystem II (optimum quantum yield, Fv/Fm) was measured every day (day 1–8) after 5 min of dark acclimation using a pulse-amplitude-modulated fluorometer (Imaging-PAM, Walz GmbH Mess- und Regeltechnik, Effeltrich, Germany), which was set up to determine the amplitude of the initial fluorescence signal (Ft) between 0.15 and 0.2 (SP intensity = 8, SP duration = 3 s), as recommended in the manual (Imaging-PAM M-Series Chlorophyll Fluorometer, Heinz Walz GmbH, Effeltrich, Germany). Fv/Fm of each disc was monitored in all stages of visible stress, until the tissue was completely decomposed (see Supplementary Figure 1).
Biochemical Response Variables
Pigments, total carbon (C), total nitrogen (N), C:N ratio, mannitol and phlorotannins were analyzed following the protocol in Diehl and Bischof (2021).
To determine differentiations in the photosynthetic apparatus, the ratios of chlorophyll a to accessory pigment pool (Chla:Acc) and of chlorophyll a to xanthophyll pigments pool (Chla:VAZ) were calculated. The de-epoxidation state of the xanthophyll cycle (DPS) was calculated after Colombo-Pallotta et al. (2006):
Statistics
Outliers (Bonferroni, p < 0.05) in the biochemical parameters were excluded from the statistical analyses. All datasets were tested for normal distribution (Shapiro-Wilk test, p > 0.05) and homogeneity of variance (Levene’s test, p > 0.05). F-statistic was reported to be robust against a moderate violation of normal distributions at small sample sizes in terms of Type I errors (Blanca et al., 2017), thus parametric tests were performed. The increase of size (% of initial) monitored over several time points (normally distributed after log10-transformation) and Fv/Fm, which was monitored every day (non-normally distributed) was analyzed using a repeated measures two-way ANOVA [population (P) × temperature-amplitude (TA)] followed by a post hoc Tukey’s test. All response variables measured on a single time point at the end of the experiment were examined using two-way ANOVAs (P × TA) followed by post hoc Tukey’s tests. The level of significance was set to p < 0.05 for normally distributed data. A more conservative level of significance was applied for non-normally distributed data (p < 0.01). All statistical analyzes were conducted with RStudio (Version 1.3.1073, 2020, Boston, MA, United States).
Results
Significant effects of the temperature-amplitude treatments (TA) within each population are marked in Figures 3–5 and Tables 2,3 using different letters. The statistical results of overall population (P) and TA effects, and their interaction are presented in Table 4.
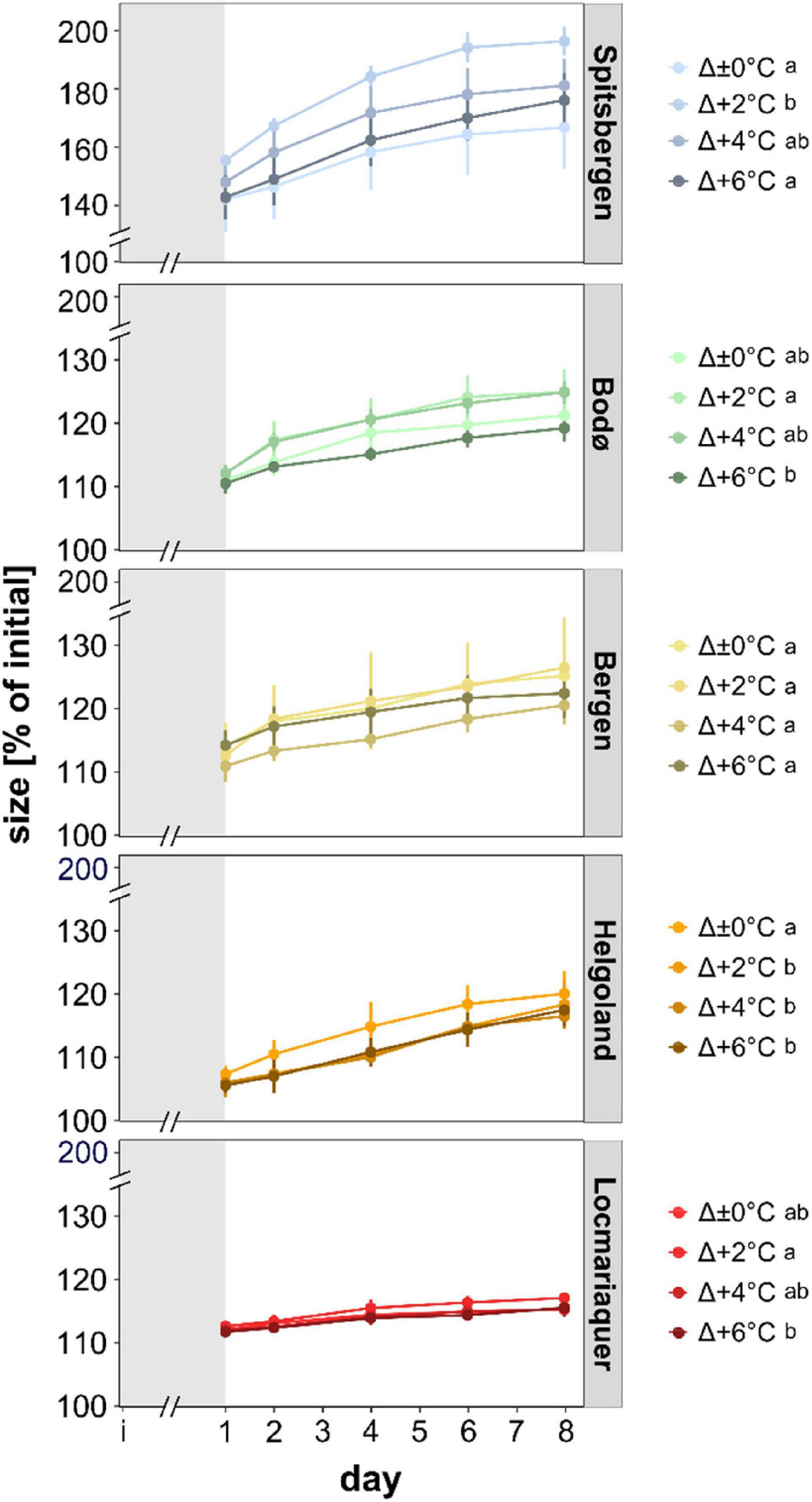
Figure 3. Size (% of initial) of meristematic discs of Saccharina latissima across latitudes in Europe during the temperature-amplitude experiment. Experimental set-up: mean summer SST (control, Δ±0°C) and temperature stress treatments: Δ+2, Δ+4, Δ+6°C. The gray shading represents the temperature acclimation phase (initial day [i] – day 1). Values are means ± SD (n = 4). Significant differences are marked by different letters.
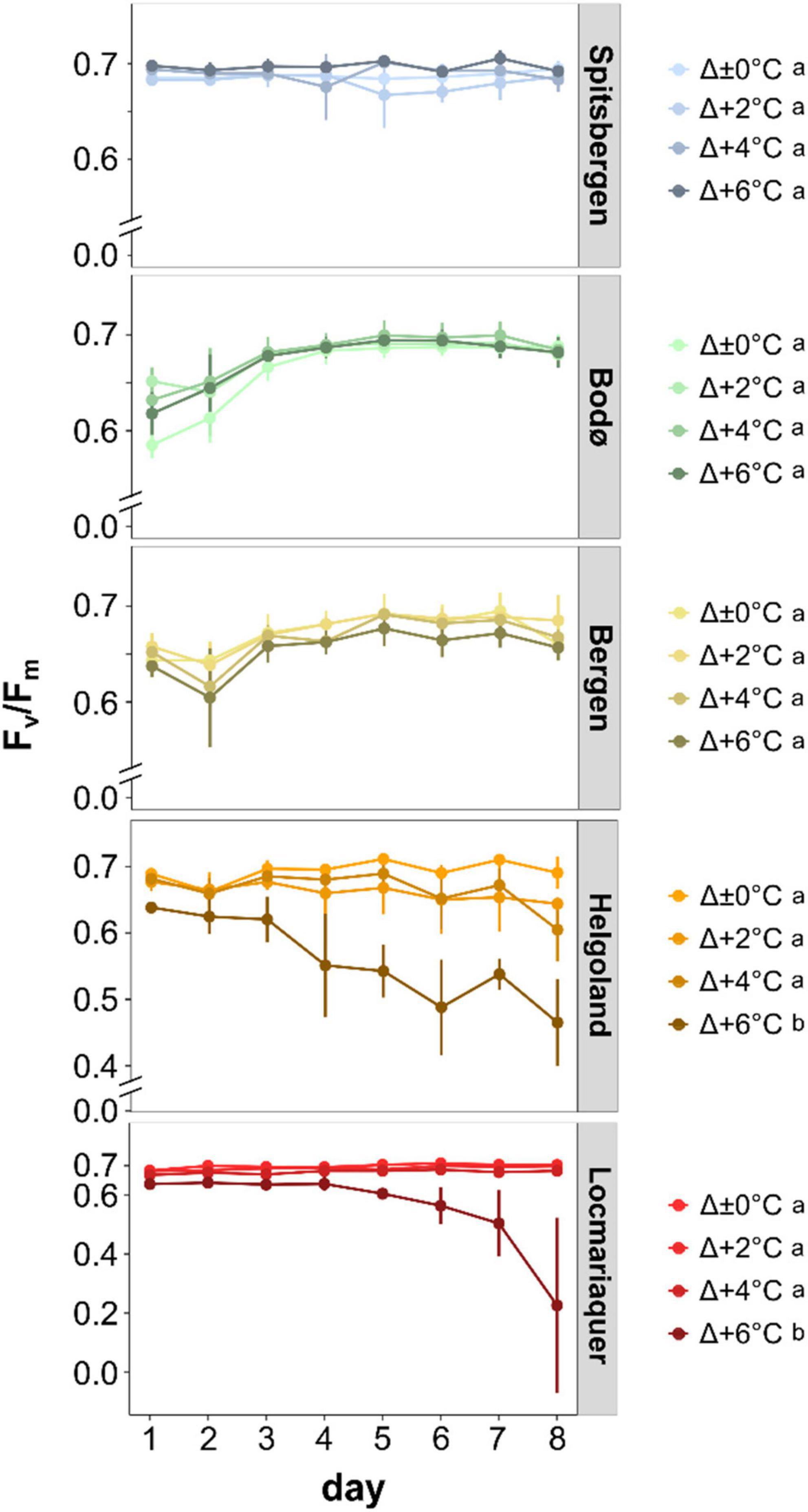
Figure 4. Optimum quantum yield of photosystem II (Fv/Fm) of Saccharina latissima across latitudes in Europe during the temperature-amplitude experiment. Experimental set-up: mean summer SST (control, Δ±0°C) and temperature stress treatments: Δ+2, Δ+4, Δ+6°C. Values are means ± SD (n = 4). The dotted line indicates the threshold for a good physiological status. Significant differences are marked by different letters.
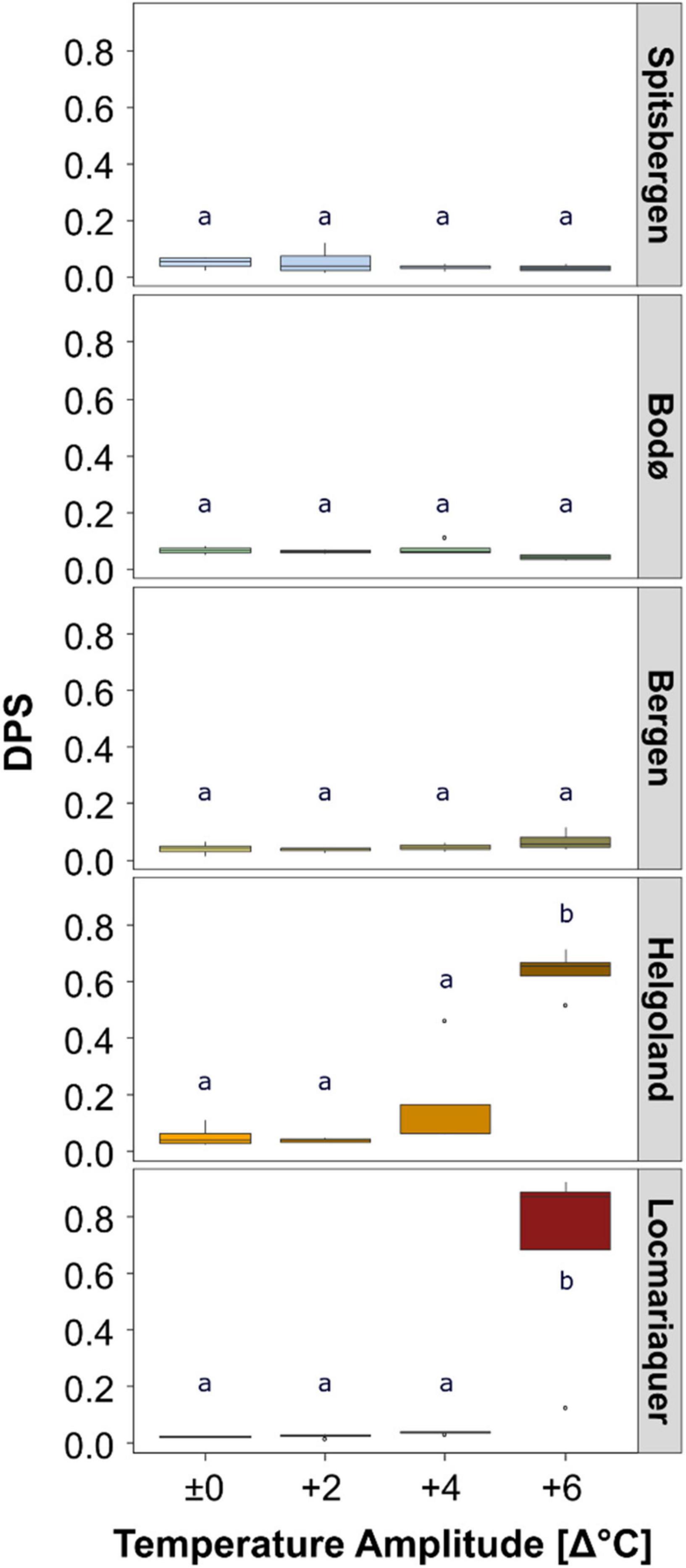
Figure 5. De-epoxidation state of the xanthophyll cyle (DPS) of Saccharina latissima across latitudes in Europe after the temperature-amplitude experiment. Experimental set-up: mean summer SST (control, Δ±0°C) and temperature stress treatments: Δ+2, Δ+4, Δ+6°C (n = 4). The bottom line of the box represents the 25th percentile, the center line of the box the median and the top line of the box the 75th percentile. The lower and upper whiskers represent the lowest and the highest values in the data. Significant differences are marked by different letters.
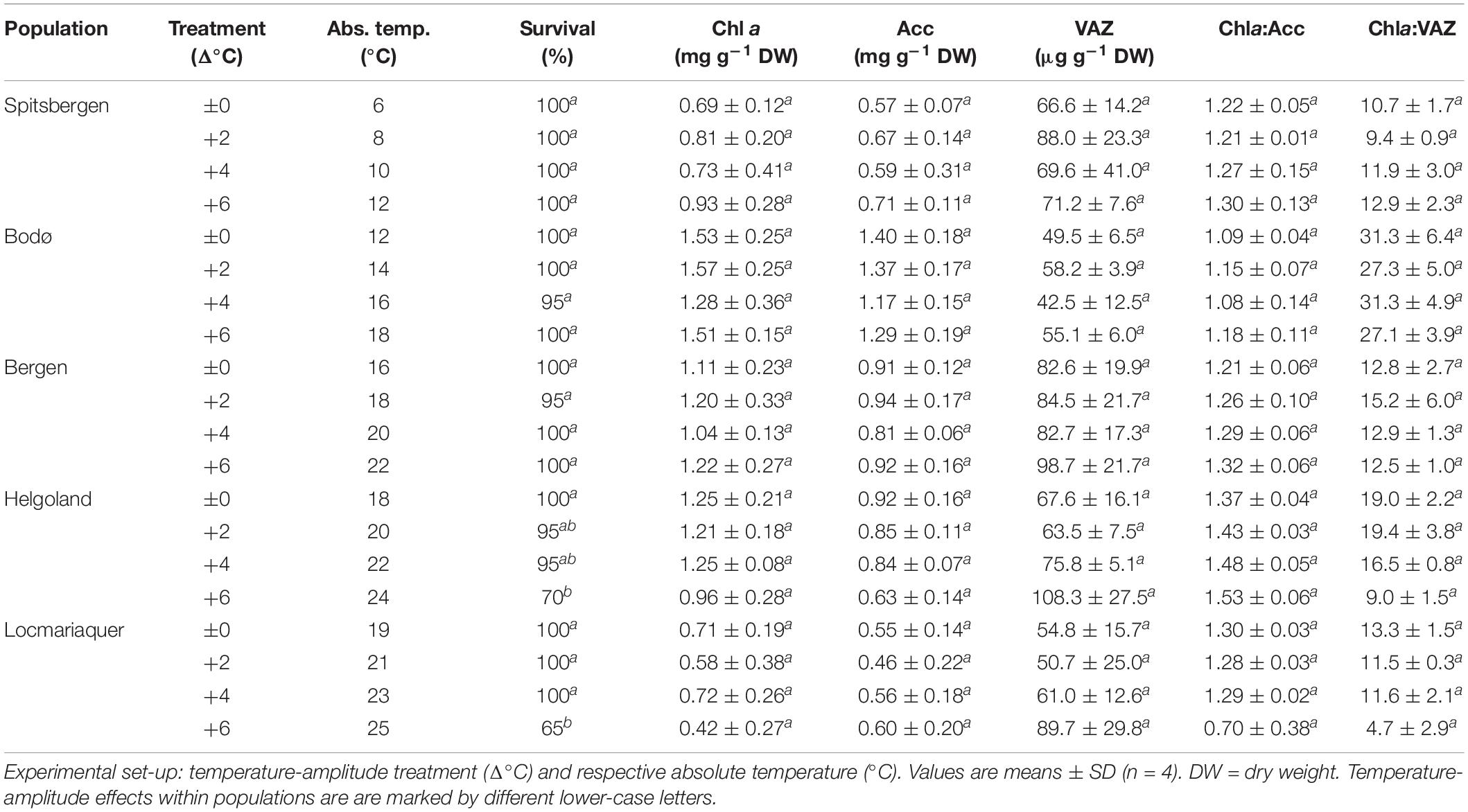
Table 2. Survival, chlorophyll a (Chl a), pool-size of accessory pigments (Acc) and pool-size of xanthophylls (VAZ), Chla:Acc ratio and Chla:VAZ ratio of S. latissima across latitudes in Europe after the temperature-amplitude experiment.
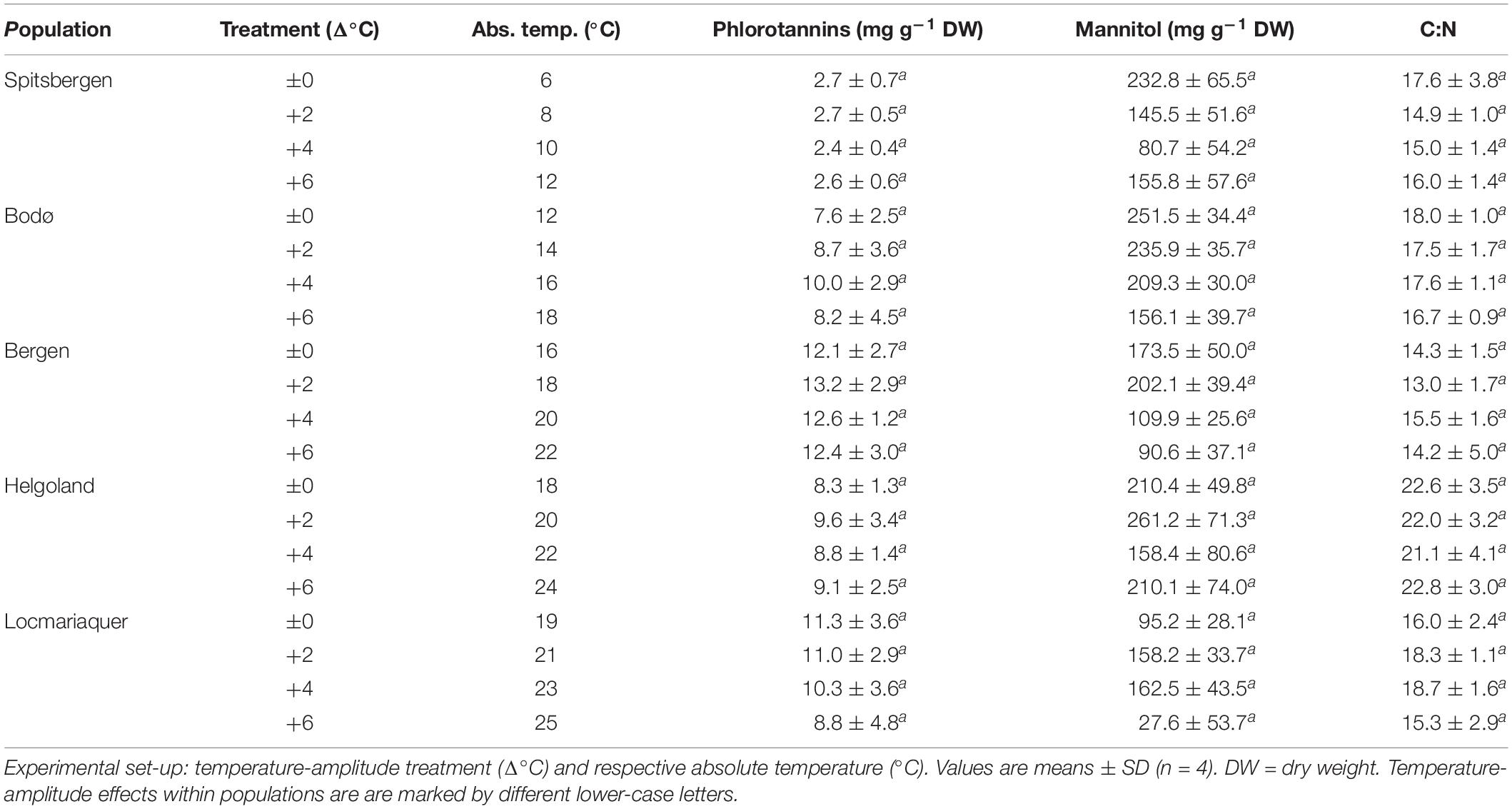
Table 3. Phlorotannins, mannitol and C:N ratio of S. latissima across latitudes in Europe after the temperature-amplitude experiment.
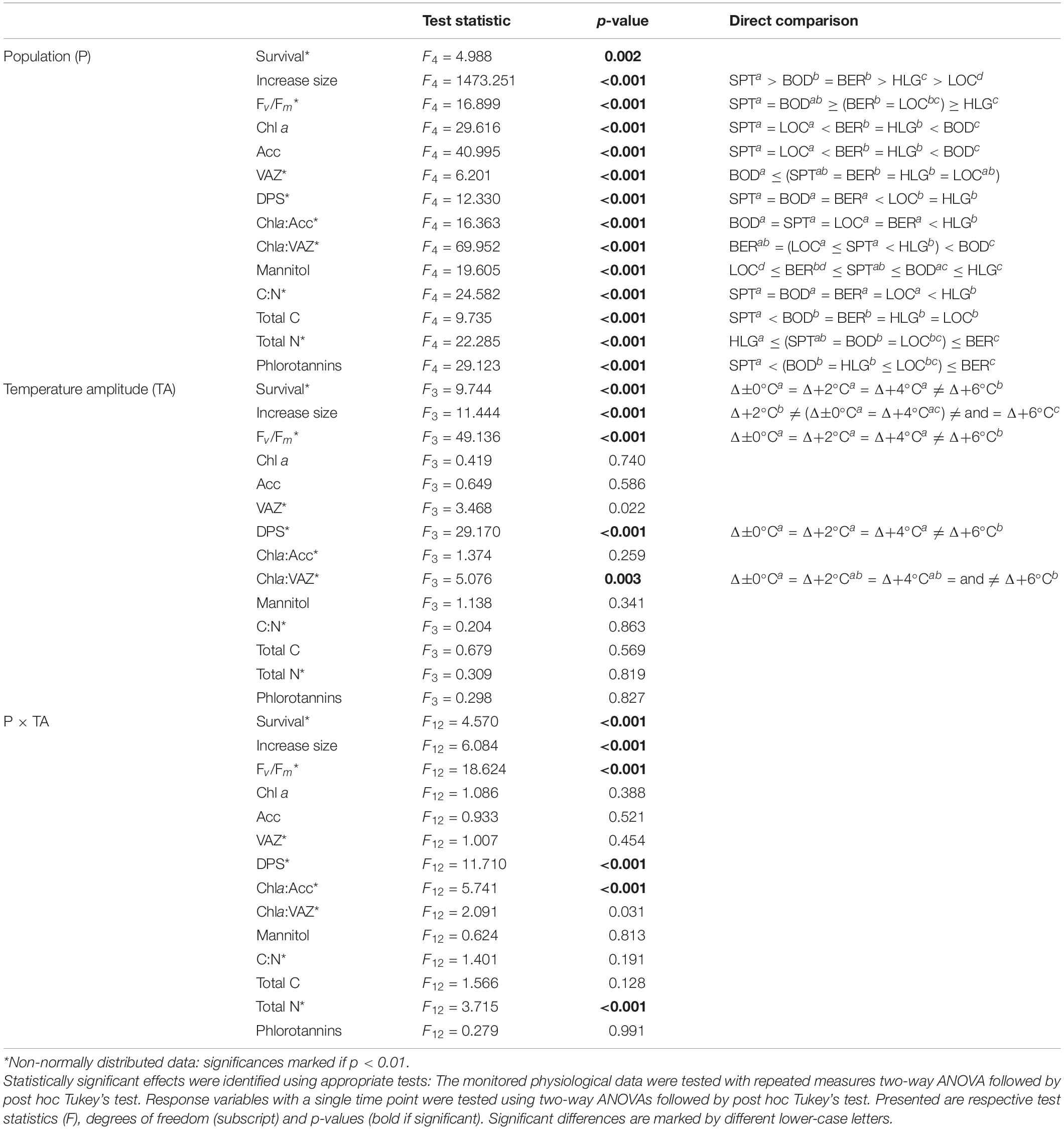
Table 4. Summary of results of statistical analyses determined between the populations [P: Spitsbergen (SPT), Bodø (BOD), Bergen (BER), Helgoland (HLG), Locmariaquer (LOC)] and temperature-amplitude treatments (TA: Δ±0°C, Δ+2°C, Δ+4°C, Δ+6°C), and their interaction (P × TA) for the measured physiological and biochemical parameters.
Survival among different populations from the species northern to southern distribution range (Table 2) varied significantly in response to increasing TA (p < 0.001) and significant interactive effects of P and TA were found (p < 0.001). The mortality of the samples strongly increased at absolute temperatures (AT) > 23°C (Table 2 and Supplementary Figure 2). Samples from Spitsbergen showed survival of 100% (AT 6–12°C) and samples from Bodø (AT 12–18°C) and Bergen (AT 16–22°C) of 95–100%, while samples collected at the locations with highest mean summer temperatures—Helgoland and Locmariaquer—died and decomposed at Δ+6°C (Helgoland: AT 24°C, 70% survival; Locmariaquer: AT 25°C, 65% survival).
For all five populations, size of the discs increased over time (Figure 3; p < 0.001). There were significant differences in growth (as reflected in rising disc diameter in % of initial) between the populations (p < 0.001), independently of the temperature treatments: While Spitsbergen samples grew considerably reaching 160–200% of their initial size after the pre-acclimation phase, samples from all other populations exhibited reduced growth activity (<130%), being lowest in Helgoland and Locmariaquer. Differences between samples from Bodø and Bergen were non-significant. Even though statistical significances were also detected for the temperature-amplitude treatments (TA) (p < 0.001) and P × TA interaction (p < 0.001), these could not be assigned to temperature increase per se (Supplementary Figure 3). For instance, growth in Spitsbergen at Δ+2°C (AT 8°C) was significantly higher than at Δ+6°C (AT 12°C), whereas at Δ±0°C (AT 10°C) and Δ+6°C (AT 12°C) growth did not differ significantly and at Δ+6°C samples from Spitsbergen were considerably larger than samples from Bodø at Δ±0°C, even though both treatments were subjected to the same absolute temperature of 12°C.
Survival and growth responses are supported by the optimum quantum yield (Fv/Fm) measurements (Figure 4). We determined significant differences between the five populations (p < 0.001) and significant impact of TA (p < 0.001) and P × TA interaction (p < 0.001) on Fv/Fm. All samples from Spitsbergen, Bodø and Bergen revealed Fv/Fm values > 0.6 throughout the experiment, whereas Fv/Fm values of samples from Helgoland and Locmariaquer markedly decreased with increasing temperatures of Δ+6°C (AT > 23°C). Clear trends to decreasing Fv/Fm values from north to south and from lower to higher temperatures were determined. Plotting the Fv/Fm values of day 8 against the absolute temperatures of the treatments (Supplementary Figure 4) reveals a strong impact of absolute temperatures on quantum yield of S. latissima. A strong diminution was solely detected at absolute temperatures above 23°C.
The absolute pigment contents of chlorophyll a (Chl a), the pool of the accessory pigments (Acc), the pool of the xanthophyll cycle pigments (VAZ) and the ratios of Chla:VAZ and Chla:Acc sampled on day 8 did not reveal any trends assigning to the latitudinal distribution of S. latissima, even though significant differences were found between the populations (Tables 2, 4). There was also no significant impact of TA and no P × TA interaction on Chl a, Acc and VAZ (Table 4). For the ratio between Chla:VAZ, significant impact of TA (p < 0.01) but not of P × TA were determined (Table 4). Still, trends to decreasing Chl a, increasing VAZ and decreasing Chla:VAZ were detected in the Δ+6°C treatments from Helgoland and Locmariaquer (Table 2). Even though a significant effect of P × TA on Chla:Acc was found (p < 0.001), no pattern regarding temperature were detected. The de-epoxidation state of the xanthophyll cycle (DPS; Figure 5) was significantly affected by P, TA and P × TA (p < 0.001; Table 4), revealing increasing DPS at the high temperature treatments in the southern populations (AT ≥ 24°C). DPS of the samples from Helgoland and Locmariaquer both increased significantly at Δ+6°C (p < 0.001).
None of the other biochemical response variables (Table 3)—mannitol, C:N ratio, phlorotannins—were significantly affected by the TA or P × TA, but significant differences were present between samples from the five populations in all three parameters (p < 0.001; Table 4), however, without correlation to the sampling latitude. Regarding phlorotannins, the lowest concentrations were found in the samples from Spitsbergen and highest in the samples from Bergen and Locmariaquer. Overall, mannitol concentrations were similar in all populations, though the samples from Locmariaquer exhibited the lowest concentrations. Saccharina latissima from Helgoland exceeded C:N ratios above 20, while samples from all other populations had values of about 13–19, independent of the TA. These differences were mainly based on significantly lower nitrogen (N) concentrations in the Helgoland samples and not on variations in total carbon (C) (Table 4 and Supplementary Table 1).
Discussion
Impacts of Short-Term Temperature Increase on Saccharina latissima Across Latitudes
Results of our study showed that S. latissima sampled across the species’ latitudinal distribution reveals differences regarding their physiology and biochemical status in response to temperature increases of Δ+2, Δ+4 or Δ+6°C above the respective local mean summer temperature. We detected adverse effects of rising temperatures exclusively in the samples of southernmost S. latissima, for which the temperature-amplitude treatments exceeded absolute temperatures (AT) of 20°C. Northern isolates overall were not impaired. In addition, we found strong site-specific and temperature-independent differences, which were especially prominent in growth response but also determined in C:N and phlorotannins.
Prior studies reported an increased mortality of S. latissima sporophytes for temperatures above 20°C (Fortes and Lüning, 1980; Bolton and Lüning, 1982; Lüning, 1984) and blade tissue of S. latissima from Nova Scotia was severely damaged and decomposed after 1-week exposure to high temperatures of 18 and 21°C (Simonson et al., 2015b). Contrary to these studies, samples from Helgoland and Locmariaquer in our experiment survived a temperature increase by Δ+2 and Δ+4°C (AT up to 23°C) and revealed high mortality only in the Δ+6°C treatment (AT ≥ 24°C) after 8 days of treatment. Thus, S. latissima from these two locations showed extraordinary resilience to MHWs in summer. The application of a stepwise increase in temperature, despite done within a short timescale, very likely allowed the specimens from Helgoland and Locmariaquer to acclimate and survive up to Δ+4°C (Terblanche et al., 2007). Thus, we can conclude that S. latissima at their warm-edge distribution can withstand short periods of summer MHWs up to AT 25°C, although mortality already occurred and increases at AT > 23°C after 1 week. As it has been shown that increased nitrogen supply supports heat tolerance in kelp (Gerard, 1997; Fernández et al., 2020), the application of ½ PES (274 μmol NO3– l–1; Sarker et al., 2013) in our study could have ameliorated the negative impacts of enhanced temperatures. Moreover, we monitored survival of excised meristematic discs, although kelp thalli under heat stress usually start degenerating from the tip (Franke et al., 2021). Furthermore, it has to be considered that adult meristematic tissue collected from the field might be more resilient than laboratory cultures or young sporophytes (Hanelt et al., 1997; Heinrich et al., 2016).
Along the entire latitudinal range, growth of the surviving discs did not differ based on the temperature-amplitude treatments but differed between the populations. In contrast to previous studies on Arctic S. latissima working with comparable absolute temperatures [Olischläger et al., 2017 (AT 4 and 10°C), Li et al., 2020 (AT 0, 8, and 15°C), Diehl and Bischof, 2021 (AT 4, 6, 8 and 10°C)], we did not detect enhanced growth with increasing temperatures in the samples from Spitsbergen. Strong site-specific differences in growth became evident in our study. An overall decreased growth from north to south was determined but was independent of absolute temperatures in general. For instance, at AT 12°C samples from Spitsbergen (Δ+6°C) revealed markedly higher growth activity than samples from Bodø (Δ±0°C). Growth in samples from Spitsbergen was highest by far in all treatments, even though the control temperature (Δ±0°C, AT 6°C) and the Δ+2°C treatment (AT 8°C) were below the reported optimum growth temperature range of 10–15°C (Bolton and Lüning, 1982). Growth was significantly lower in samples from Helgoland (AT 18–24°C) and Locmariaquer (AT 19–25°C), which were both exposed to temperatures exceeding the optimum growth temperature of the species. Even at ambient temperature (Δ±0°C) and lower amplitude of temperature increase, samples from Locmariaquer recorded the lowest growth. This suggests that S. latissima in this region is already exposed to the proximate upper temperature tolerance limit and occurrence of MHW can be detrimental to the population. As we collected all meristems in June to early July, according to Lüning (1979) meristems should still have been in some kind of growth mode. As growth activity differs with day length (Fortes and Lüning, 1980), and our latitudinal collection gradient is characterized by a steep day length difference, it might have been that the physiological growth activity state was different besides locations despite sampling at a uniform time period. However, our treatment conditions always applied the same day length condition and thereby it can be excluded that the experimental light set-up had an influence on growth. Spurkland and Iken (2011, 2012) suggest that S. latissima exhibits growth plasticity within a genetically fixed seasonal growth window that might be an advantage in locations with high environmental variability, such as the Arctic. The particular irradiance conditions in the Arctic (Polar Day) prior the experiment may have promoted the extraordinary growth activity of the Spitsbergen population, whereas shorter day lengths may have decelerated growth of S. latissima from lower latitudes (Fortes and Lüning, 1980). Consequently, observed growth activity might have been more dependent on internal growth patterns than on their exposure temperatures during the experiment, resulting in S. latissima from the Arctic growing most and from Locmariaquer least in summer. The causes for the observed differences in growth patterns of European S. latissima requires further investigation.
Temperature treatment corresponding to the absolute temperature rather than the respective temperature amplitude treatments affected the optimum quantum yield of S. latissima. For example, samples from Spitsbergen were slightly stimulated at the highest temperature amplitude treatment of Δ+6°C (AT 12°C), but samples from warmer locations decreased under Δ+6°C treatments (AT > 20°C). Several studies already reported an increase in optimum quantum yield of wild Arctic S. latissima sporophytes exposed to increasing absolute temperatures up to 10°C (Iñiguez et al., 2016; Diehl and Bischof, 2021). On the other hand, a decrease in Fv/Fm was reported among populations from southern Norway and Denmark (Andersen et al., 2013; Nepper-Davidsen et al., 2019). Our study showed that regardless of origin, all S. latissima exhibited relatively high Fv/Fm values (> 0.6) suggesting a healthy physiological status under MHW conditions of absolute temperatures of up to 20°C. This suggests that seaweeds can withstand short-term increase in SST during summer across latitudes. It is striking that S. latissima from Locmariaquer recorded higher photosynthetic quantum yields compared to Helgoland, even though both populations were exposed to comparable high temperatures. Locmariaquer samples maintained a very good physiological status at AT 23°C (> 0.65), while Fv/Fm values for Helgoland samples already declined at AT 22°C (0.605). This response is similar to that observed for disc survival. If these responses are based on local adaptation or different phenotypic plasticity between the populations, requires further examination.
Saccharina latissima and other kelps exhibit different biochemical mechanisms to acclimate to temperature variations. In general, the effects of temperature, e.g., in the environment or during laboratory experiments, on pigment content and composition were reported to be very complex (e.g., Machalek et al., 1996; Fernandes et al., 2016). However, we did not detect a clear link between variation in pigment concentrations and the species latitudinal distribution range or exposure to absolute temperature treatments. The tested temperature amplitudes only revealed significant impact of the Δ+6°C treatments. In particular, when surpassing the sublethal thresholds (Bolton and Lüning, 1982; Lüning, 1984), Δ+6°C imposed considerable stress in S. latissima from Helgoland and Locmariaquer (AT: > 24°C). During temperature stress, the interconversion of violaxanthin to zeaxanthin (de-epoxidation state of the xanthophyll cycle, DPS) acts as precursory protective mechanism for the dissipation of excessive energy, thereby preventing the generation of reactive oxygen species (ROS) (Goss and Jakob, 2010). Comparable to Nepper-Davidsen et al. (2019) we measured a strong increase of DPS at high temperatures in samples from Helgoland and Locmariaquer, indicating the exertion to counteract negative impacts of ROS. The increasing trend in the pool size of the xanthophyll cycle pigments (VAZ) in the same treatments reflects the importance of DPS as a protective mechanism at high temperatures. Changes in the pigment composition within the photosynthetic apparatus, for instance a decrease in chlorophyll a (Chl a) in response to suboptimal temperatures, is associated to a consequential reduced photosynthetic activity (Wernberg et al., 2016b; Nepper-Davidsen et al., 2019). Interestingly, we did not observe a significant decrease in Chl a under different temperatures in our study. Still, the combination of slightly increasing VAZ and decreasing Chl a resulted in decreases of the Chla:VAZ ratio at Δ+6°C with potential negative implications for the functioning of the photosynthetic apparatus of Helgoland and Locmariaquer populations.
Phlorotannins, which act as antioxidants and protection against ROS (Amsler, 2008), were not affected by the temperature-amplitude treatments. In accordance with the study by Simonson et al. (2015a), they did not increase significantly at high temperatures as was observed in other brown algae, e.g., Agarum clathratum, but strong differentiations across populations were found. Significantly fewer phlorotannins were measured in the samples from Spitsbergen and the concentrations were comparable to those of Arctic S. latissima in a previous study (Diehl and Bischof, 2021).
The storage carbohydrate mannitol is the main photoassimilatory product in brown algae (Reed et al., 1985). We did not find any temperature effects or differences in mannitol content across latitudes. Diehl and Bischof (2021) proposed that Arctic S. latissima may constantly store high mannitol concentrations as a mechanism to acclimate to frequent environmental variation, which we cannot verify in the present study. In their experiment, Diehl and Bischof (2021) mimicked Arctic summer conditions and maintained the samples under constant 24 h of light exposure. For our current study, we kept all samples at a 16:8-h-light:dark rhythm to eliminate potential light effects at the different latitudes, which might have influenced the synthesis of mannitol.
Nutrient uptake in seaweeds is usually affected by temperature (Roleda and Hurd, 2019). In our experiment, the C:N ratio did not vary in the temperature-amplitude treatments and no pattern of intracellular nitrogen (N) was detected across latitudes. Generally, local variations in C:N were very small and mainly based on changes in total N rather than total carbon (C). N is frequently limiting in coastal systems over summer. Still, only samples from Helgoland revealed C:N ratios of above 20, indicating N limitation (Atkinson and Smith, 1983) despite high supply with N (½ PES) during the experiment.
Implications for the Population Biology of Saccharina latissima in Europe
Even though S. latissima tolerates wide amplitudes of different physico-chemical drivers (reviewed by Bartsch et al., 2008), strong declines and changes in biomass were observed from several regions in Europe (Pehlke and Bartsch, 2008; Moy and Christie, 2012; Casado-Amezúa et al., 2019; Filbee-Dexter et al., 2020). Heat tolerance and the capacity for acclimation may differ between genetic strains, thus, populations (Clark et al., 2013). Our study shows that local MHWs in summer only pose stress to S. latissima in regions with higher mean summer sea surface temperatures. Overall, the thermal responses of all five populations sampled along the latitudinal gradient were dependent on the experienced absolute temperatures during the experiments. However, population genetics revealed low connectivity among S. latissima populations in Europe within short distances (Guzinski et al., 2020). Thus, analyses of only one population per site may not be fully representative of the latitudinal range.
Our study is short-term and worked on excised discs of sporophytes incubated in small-volume vessels in the laboratory; therefore, has some limitations. For instance, transportation and different pre-acclimation periods could have affected the sensitivity toward temperature of the samples. Long-term studies on whole sporophytes in outdoor mesocosms may measure different responses and provide a more realistic prognosis for the future. Moreover, our experiments were run in different years (2018, 2019, and 2020) but consistently during summer months (June and July); hence, inter-annual variation in the quality of sporophytes may be imminent but cannot be addressed. Rearing temperature of sporophytes is known to affect the photosynthetic performance of S. latissima (Davison, 1987; Davison and Davison, 1987; Davison et al., 1991), and the importance of temperature history on thermal plasticity of kelps was recently shown by Liesner et al. (2020b). They provided evidence for non-genetic carry-over and cross-generational effects and reported a stimulating effect of cold temperatures during early life stages on several physiological and biochemical responses to high temperatures. Rapid and intense ocean warming does not only occur in summer but has also been detected in winter and is predicted to further increase in the near future (Maturilli et al., 2013; IPCC, 2019b). This might additionally affect the thermal responses and stress resilience of S. latissima to MHWs in summer and should be addressed in future studies. Furthermore, it has to be considered that also at suboptimal temperatures, specimens might be less resilient to other stressors or less competitive against other algae (Wernberg et al., 2010; Moy and Christie, 2012; Andersen et al., 2013; Filbee-Dexter and Wernberg, 2018).
Summarizing, European S. latissima exposed to realistic local summer MHW events reveals that stress responses are rather independent from temperature amplitudes, but mostly dependent on the absolute temperatures experienced across latitudes, especially when surpassing sub-lethal and lethal limits for a period of 8 days. Thus, the increase in frequency and amplitude of MHWs will considerably stress southern S. latissima, causing further decline of rear-edge populations while northern populations stay safe for the time being.
Data Availability Statement
The datasets analyzed for this study can be found in the PANGAEA Database (https://doi.org/10.1594/PANGAEA.931637).
Author Contributions
ND and KB planned the experiments. MR helped collecting in Bodø and helped designing the experimental set-up. ND conducted the experiments and analyzed the data. UK and IB provided the facilities for experiments and biochemical analyses and helped with interpretation. IB supported the experimental procedure. KB supervised the project. ND wrote the manuscript, which was reviewed, revised, and approved by all co-authors. All authors contributed to the data interpretation and discussion.
Funding
This study was further supported by the 2015-2016 BiodivERsA COFUND call for research proposals (program MARFOR), with the national funder German Research Foundation (DFG; Grant No. VA 105/25-1), and has received funding from the European Union’s Horizon 2020 Research and Innovation Programme under grant agreement No. 869154.
Conflict of Interest
The authors declare that the research was conducted in the absence of any commercial or financial relationships that could be construed as a potential conflict of interest.
Publisher’s Note
All claims expressed in this article are solely those of the authors and do not necessarily represent those of their affiliated organizations, or those of the publisher, the editors and the reviewers. Any product that may be evaluated in this article, or claim that may be made by its manufacturer, is not guaranteed or endorsed by the publisher.
Acknowledgments
Abiotic temperature data used in this manuscript were produced with the Giovanni online data system, developed and maintained by the NASA GES DISC. Sampling in France was conducted in accordance with the French legislation on the Access to Genetic Resources and Benefit-Sharing. We also acknowledge the MODIS mission scientists and associated NASA personnel for the production of the data used in this research effort. This study has been conducted at the Alfred Wegener Institute for Polar and Marine Research (AWI), Bremerhaven and at the AWIPEV Research Station in Ny-Ålesund, Svalbard. We are grateful to the station staff of AWIPEV for support and logistics and to the scientific diving teams on Spitsbergen and Helgoland for sampling. We thank A. Wagner (AWI) for sampling support on Helgoland and his support in the setting up of the experiments, and also J. Müller (University of Rostock) for running the C:N analyzer. B. Meyer-Schlosser (University of Bremen) supported sampling and pigment analyses.
Supplementary Material
The Supplementary Material for this article can be found online at: https://www.frontiersin.org/articles/10.3389/fmars.2021.695821/full#supplementary-material
References
Acker, J. G., and Leptoukh, G. (2007). Online analysis enhances use of NASA Earth Science Data. Eos Trans. Am. Geophys. Union 88, 14–17.
Adey, W. H., and Steneck, R. S. (2001). Thermogeography over time creates biogeographic regions: a temperature/space/time-integrated model and an abundance-weighted test for benthic marine algae. J. Phycol. 37, 677–698. doi: 10.1046/j.1529-8817.2001.00176.x
Amsler, C. D. (2008). Algal Chemical Ecology, ed. C. D. Amsler. Berlin: Springer-Verlag Berlin Heidelberg.
Andersen, G., Pedersen, M., and Nielsen, S. (2013). Temperature acclimation and heat tolerance of photosynthesis in Norwegian Saccharina latissima (Laminariales, Phaeophyceae). J. Phycol. 49, 689–700. doi: 10.1111/j.1529-8817.2013.12077
Araújo, R. M., Assis, J., Aguillar, R., Airoldi, L., Bárbara, I., Bartsch, I., et al. (2016). Status, trends and drivers of kelp forests in Europe: an expert assessment. Biodivers. Conserv. 25, 1319–1348. doi: 10.1007/s10531-016-1141-7
Assis, J., Araújo, M. B., and Serrão, E. A. (2018). Projected climate changes threaten ancient refugia of kelp forests in the North Atlantic. Glob. Chang. Biol. 24, e55–e66. doi: 10.1111/gcb.13818
Atkinson, M. J., and Smith, S. V. (1983). C:N:P ratios of benthic marine plants. Limnol. Oceanogr. 28, 568–574.
Bartsch, I., Wiencke, C., Bischof, K., Buchholz, C. M., Buck, B. H., Eggert, A., et al. (2008). The genus Laminaria sensu lato: recent insights and developments. Eur. J. Phycol. 43, 1–86. doi: 10.1080/09670260701711376
Baschek, B., Schroeder, F., Brix, H., Riethmüller, R., Badewien, T. H., Breitbach, G., et al. (2017). The coastal observing system for northern and arctic seas (COSYNA). Ocean Sci. 13, 379–410.
Bekkby, T., and Moy, F. E. (2011). Developing spatial models of sugar kelp (Saccharina latissima) potential distribution under natural conditions and areas of its disappearance in Skagerrak. Estuar. Coast. Shelf Sci. 95, 477–483. doi: 10.1016/j.ecss.2011.10.029
Bennett, S., Duarte, C. M., Marbà, N., and Wernberg, T. (2019). Integrating within-species variation in thermal physiology into climate change ecology. Philos. Trans. R. Soc. B 374:20180550. doi: 10.1098/rstb.2018.0550
Bennett, S., Wernberg, T., Joy, B. A., De Bettignies, T., and Campbell, A. H. (2015). Central and rear-edge populations can be equally vulnerable to warming. Nat. Commun. 6:10280. doi: 10.1038/ncomms10280
Blanca, M. J., Alarcón, R., Arnau, J., Bono, R., and Bendayan, R. (2017). Non-normal data: is ANOVA still a valid option? Psicothema 29, 552–557. doi: 10.7334/psicothema2016.383
Bolton, J. J., and Lüning, K. (1982). Optimal growth and maximal survival temperatures of Atlantic Laminaria species (Phaeophyta) in culture. Mar. Biol. 66, 89–94. doi: 10.1007/BF00397259
Burdett, H. L., Wright, H., and Smale, D. A. (2019). Photophysiological responses of canopy-forming kelp species to short-term acute warming. Front. Mar. Sci. 6:516. doi: 10.3389/fmars.2019.00516
Casado-Amezúa, P., Araújo, R., Bárbara, I., Bermejo, R., Borja, Á, Díez, I., et al. (2019). Distributional shifts of canopy-forming seaweeds from the Atlantic coast of Southern Europe. Biodivers. Conserv. 28, 1151–1172. doi: 10.1007/s10531-019-01716-9
Clark, J. S., Poore, A. G. B., Ralph, P. J., and Doblin, M. A. (2013). Potential for adaptation in response to thermal stress in an intertidal macroalga. J. Phycol. 49, 630–639. doi: 10.1111/jpy.12067
Colombo-Pallotta, M. F., García-Mendoza, E., and Ladah, L. B. (2006). Photosynthetic performance, light absorption, and pigment composition of Macrocystis pyrifera (Laminariales, Phaeophyceae) blade from different depths. J. Phycol. 42, 1225–1234. doi: 10.1111/j.1529-8817.2006.00287.x
Davison, I. R. (1987). Adaptation of photosynthesis in Laminaria saccharina (Phaeophyta) to changes in growth temperature. J. Phycol. 23, 273–283.
Davison, I. R., and Davison, J. O. (1987). The effect of growth temperature on enzyme activities in the brown alga Laminaria saccharina. Br. Phycol. J. 22, 77–87. doi: 10.1080/00071618700650101
Davison, I. R., Greene, R. M., and Podolak, E. J. (1991). Temperature acclimation of respiration and photosynthesis in the brown alga Laminaria saccharina. Mar. Biol. 110, 449–454. doi: 10.1007/BF01344363
Dayton, P. K. (1985). Ecology of kelp communities. Annu. Rev. Ecol. Syst. 16, 215–245. doi: 10.1146/annurev.ecolsys.16.1.215
Diehl, N., and Bischof, K. (2021). Coping with a changing Arctic: mechanisms of acclimation in the brown seaweed Saccharina latissima from Spitsbergen. Mar. Ecol. Prog. Ser. 657, 43–57. doi: 10.3354/meps13532
Fernandes, F., Barbosa, M., Oliveira, A. P., Azevedo, I. C., Sousa-Pinto, I., Valentão, P., et al. (2016). The pigments of kelps (Ochrophyta) as part of the flexible response to highly variable marine environments. J. Appl. Phycol. 28, 3689–3696. doi: 10.1007/s10811-016-0883-7
Fernández, P. A., Gaitán-Espitia, J. D., Leal, P. P., Schmid, M., Revill, A. T., and Hurd, C. L. (2020). Nitrogen sufficiency enhances thermal tolerance in habitat-forming kelp: implications for acclimation under thermal stress. Sci. Rep. 10:3186. doi: 10.1038/s41598-020-60104-4
Filbee-Dexter, K., and Wernberg, T. (2018). Rise of turfs: a new battlefront for globally declining kelp forests. Bioscience 68, 64–76. doi: 10.1093/biosci/bix147
Filbee-Dexter, K., Wernberg, T., Grace, S. P., Thormar, J., Fredriksen, S., Narvaez, C. N., et al. (2020). Marine heatwaves and the collapse of marginal North Atlantic kelp forests. Sci. Rep. 10:13388. doi: 10.1038/s41598-020-70273-x
Fortes, M. D., and Lüning, K. (1980). Growth rates of North Sea macroalgae in relation to temperature, irradiance and photoperiod. Helgoländer Meeresuntersuchungen 34, 15–29. doi: 10.1007/BF01983538
Franke, K., Liesner, D., Heesch, S., and Bartsch, I. (2021). Looks can be deceiving: contrasting temperature characteristics of two morphologically similar kelp species co-occurring in the Arctic. Bot. Mar. 64, 163–175. doi: 10.1515/bot-2021-0014
Frölicher, T. L., Fischer, E. M., and Gruber, N. (2018). Marine heatwaves under global warming. Nature 560, 360–364. doi: 10.1038/s41586-018-0383-9
Gerard, V. A. (1997). The role of nitrogen nutrition in high-temperature tolerance of the kelp, Laminaria saccharina (Chromophyta). J. Phycol. 33, 800–810. doi: 10.1111/j.0022-3646.1997.00800.x
Goss, R., and Jakob, T. (2010). Regulation and function of xanthophyll cycle-dependent photoprotection in algae. Photosynth. Res. 106, 103–122. doi: 10.1007/s11120-010-9536-x
Guzinski, J., Ruggeri, P., Ballenghien, M., Mauger, S., Jacquemin, B., Jollivet, C., et al. (2020). Seascape genomics of the sugar kelp Saccharina latissima along the north eastern Atlantic latitudinal gradient. Genes (Basel). 11:1503. doi: 10.3390/genes11121503
Hanelt, D., Wiencke, C., Karsten, U., and Nultsch, W. (1997). Photoinhibition and recovery after high light stress in different developmental and life-history stages of Laminaria saccharina (Phaeophyta). J. Phycol. 33, 387–395. doi: 10.1111/j.0022-3646.1997.00387.x
Harris, R. M. B., Beaumont, L. J., Vance, T. R., Tozer, C. R., Remenyi, T. A., Perkins-Kirkpatrick, S. E., et al. (2018). Biological responses to the press and pulse of climate trends and extreme events. Nat. Clim. Chang. 8, 579–587. doi: 10.1038/s41558-018-0187-9
Heinrich, S., Valentin, K., Frickenhaus, S., and Wiencke, C. (2016). Origin matters–comparative transcriptomics in Saccharina latissima (Phaeophyceae). J. Exp. Mar. Bio. Ecol. 476, 22–30. doi: 10.1016/j.jembe.2015.12.006
Hobday, A. J., Alexander, L. V., Perkins, S. E., Smale, D. A., Straub, S. C., Oliver, E. C. J. J., et al. (2016). A hierarchical approach to defining marine heatwaves. Prog. Oceanogr. 141, 227–238. doi: 10.1016/j.pocean.2015.12.014
Iñiguez, C., Carmona, R., Lorenzo, M. R., Niell, F. X., Wiencke, C., and Gordillo, F. J. L. (2016). Increased temperature, rather than elevated CO2, modulates the carbon assimilation of the Arctic kelps Saccharina latissima and Laminaria solidungula. Mar. Biol. 163:248. doi: 10.1007/s00227-016-3024-6
IPCC (2019a). “Summary for policymakers,” in IPCC Special Report on the Ocean and Cryosphere in a Changing Climate, eds P. R. Shukla, J. Skea, E. Calvo Buendia, V. Masson-Delmotte, H.-O. Pörtner, D. C. Roberts, et al. (Geneva: IPCC).
IPCC (2019b). “Summary for Policymakers,” in Climate Change and Land: an IPCC Special Report on Climate Change, Desertification, Land Degradation, Sustainable Land Management, Food Security, and Greenhouse Gas Fluxes in Terrestrial Ecosystems, eds P. R. Shukla, J. Skea, E. Calvo Buendia, V. Masson-Delmotte, H.-O. Pörtner, D. C. Roberts, et al. (Geneva: IPCC), doi: 10.4337/9781784710644
Jueterbock, A., Kollias, S., Smolina, I., Fernandes, J. M. O., Coyer, J. A., Olsen, J. L., et al. (2014). Thermal stress resistance of the brown alga Fucus serratus along the North-Atlantic coast: acclimatization potential to climate change. Mar. Genomics 13, 27–36. doi: 10.1016/j.margen.2013.12.008
Karsten, U. (2007). Salinity tolerance of Arctic kelps from Spitsbergen. Phycol. Res. 55, 257–262. doi: 10.1111/j.1440-1835.2007.00468.x
Kelly, M. W., Sanford, E., and Grosberg, R. K. (2012). Limited potential for adaptation to climate change in a broadly distributed marine crustacean. Proc. R. Soc. B Biol. Sci. 279, 349–356. doi: 10.1098/rspb.2011.0542
King, N. G., McKeown, N. J., Smale, D. A., and Moore, P. J. (2018). The importance of phenotypic plasticity and local adaptation in driving intraspecific variability in thermal niches of marine macrophytes. Ecography (Cop.) 41, 1469–1484. doi: 10.1111/ecog.03186
Krause-Jensen, D., Archambault, P., Assis, J., Bartsch, I., Bischof, K., Filbee-dexter, K., et al. (2020). Imprint of climate change on Pan-Arctic marine vegetation. Front. Mar. Sci. 7:617324. doi: 10.3389/fmars.2020.617324
Krumhansl, K. A., Okamoto, D. K., Rassweiler, A., Novak, M., Bolton, J. J., Cavanaugh, K. C., et al. (2016). Global patterns of kelp forest change over the past half-century. Proc. Natl. Acad. Sci. U.S.A. 113, 13785–13790. doi: 10.1073/pnas.1606102113
Li, H., Monteiro, C., Heinrich, S., Bartsch, I., Valentin, K., and Harms, L. et al. (2020). Responses of the kelp Saccharina latissima (Phaeophyceae) to the warming Arctic: from physiology to transcriptomics. Physiol. Plant. 168, 5–26. doi: 10.1111/ppl.13009
Liesner, D., Fouqueau, L., Valero, M., Roleda, M. Y., Pearson, G. A., Bischof, K., et al. (2020a). Heat stress responses and population genetics of the kelp Laminaria digitata (Phaeophyceae) across latitudes reveal differentiation among North Atlantic populations. Ecol. Evol. 10, 9144–9177. doi: 10.1002/ece3.6569
Liesner, D., Shama, L. N. S., Diehl, N., Valentin, K., and Bartsch, I. (2020b). Thermal plasticity of the kelp Laminaria digitata (Phaeophyceae) across life cycle stages reveals the importance of cold seasons for marine forests. Front. Mar. Sci. 7:456. doi: 10.3389/fmars.2020.00456
Lüning, K. (1979). Growth strategies of three Laminaria species (Phaeophyceae) inhabiting different depth zones in the sublittoral region of Helgoland (North Sea). Mar. Ecol. Prog. Ser. 1, 195–207. doi: 10.3354/meps001195
Lüning, K. (1984). Temperature tolerance and biogeography of seaweeds: the marine algal flora of Helgoland (North Sea) as an example. Helgoländer Meeresuntersuchungen 28, 305–317.
Lüning, K. (1990). Seaweeds–Their Environment, Biogeography, and Ecophysiology, eds C. Yarish and H. Kirkman. Stuttgart: John Wiley & Sons, Inc.
Machalek, K., Davison, R., and Falkwoski, P. (1996). Thermal acclimation and photoacclimation of photosynthesis in the brown alga Laminaria saccharina. Plant Cell Environ. 19, 1005–1016.
Maturilli, M., Herber, A., and König-Langlo, G. (2013). Climatology and time series of surface meteorology in Ny-Ålesund, Svalbard. Earth Syst. Sci. Data 5, 155–163. doi: 10.5194/essd-5-155-2013
Meehl, G. A., and Tebaldi, C. (2004). More intense, more frequent, and longer lasting heat waves in the 21st century. Science 305, 994–997. doi: 10.1126/science.1098704
Moy, F. E., and Christie, H. (2012). Large-scale shift from sugar kelp (Saccharina latissima) to ephemeral algae along the south and west coast of Norway. Mar. Biol. Res. 8, 309–321. doi: 10.1080/17451000.2011.637561
Müller, R., Laepple, T., Bartsch, I., and Wiencke, C. (2009). Impact of oceanic warming on the distribution of seaweeds in polar and cold-temperate waters. Bot. Mar. 52, 617–638. doi: 10.1515/BOT.2009.080
Nepper-Davidsen, J., Andersen, D., and Pedersen, M. (2019). Exposure to simulated heatwave scenarios causes long-term reductions in performance in Saccharina latissima. Mar. Ecol. Prog. Ser. 630, 25–39. doi: 10.3354/meps13133
Olischläger, M., Iñiguez, C., Koch, K., Wiencke, C., and Gordillo, F. J. L. (2017). Increased pCO2 and temperature reveal ecotypic differences in growth and photosynthetic performance of temperate and Arctic populations of Saccharina latissima. Planta 245, 119–136. doi: 10.1007/s00425-016-2594-3
Oliver, E. C. J. J., Donat, M. G., Burrows, M. T., Moore, P. J., Smale, D. A., Alexander, L. V., et al. (2018). Longer and more frequent marine heatwaves over the past century. Nat. Commun. 9:1324. doi: 10.1038/s41467-018-03732-9
Pehlke, C., and Bartsch, I. (2008). Changes in depth distribution and biomass of sublittoral seaweeds at Helgoland (North Sea) between 1970 and 2005. Clim. Res. 37, 135–147. doi: 10.3354/cr00767
Pereira, T. R., Engelen, A. H., Pearson, G. A., Valero, M., and Serrão, E. A. (2015). Response of kelps from different latitudes to consecutive heat shock. J. Exp. Mar. Bio. Ecol. 463, 57–62. doi: 10.1016/j.jembe.2014.10.022
Provasoli, L. (1968). “Media and prospects for the cultivation of marine algae,” in Cultures and collections of algae,” in Proceedings of the Japanese Conference Hakone, eds A. Watanabe and A. Hattori (Tokyo: Japanese Society of Plant Physiology), 63–75.
Reed, R. H., Davison, I. R., Chudek, J. A., and Foster, R. (1985). The osmotic role of mannitol in the Phaeophyta: an appraisal. Phycologia 24, 35–47. doi: 10.2216/i0031-8884-24-1-35.1
Reed, T. E., Schindler, D. E., and Waples, R. S. (2011). Interacting effects of phenotypic plasticity and evolution on population persistence in a changing climate. Conserv. Biol. 25, 56–63. doi: 10.1111/j.1523-1739.2010.01552.x
Reich, P. B., Sendall, K. M., Rice, K., Rich, R. L., Stefanski, A., Hobbie, S. E., et al. (2015). Geographic range predicts photosynthetic and growth response to warming in co-occurring tree species. Nat. Clim. Chang. 5, 148–152. doi: 10.1038/nclimate2497
Roleda, M. Y., and Hurd, C. L. (2019). Seaweed nutrient physiology: application of concepts to aquaculture and bioremediation. Phycologia 58, 552–562. doi: 10.1080/00318884.2019.1622920
Saha, M., Barboza, F. R., Somerfield, P. J., Al-Janabi, B., Beck, M., Brakel, J., et al. (2020). Response of foundation macrophytes to near-natural simulated marine heatwaves. Glob. Chang. Biol. 26, 417–430. doi: 10.1111/gcb.14801
Sarker, M. Y., Bartsch, I., Olischläger, M., Gutow, L., and Wiencke, C. (2013). Combined effects of CO2, temperature, irradiance and time on the physiological performance of Chondrus crispus (Rhodophyta). Bot. Mar. 56, 63–74. doi: 10.1515/bot-2012-0143
Simonson, E. J., Scheibling, R. E., and Metaxas, A. (2015b). Kelp in hot water: I. Warming seawater temperature induces weakening and loss of kelp tissue. Mar. Ecol. Prog. Ser. 537, 89–104. doi: 10.3354/meps11438
Simonson, E. J., Metaxas, A., and Scheibling, R. E. (2015a). Kelp in hot water: II. Effects of warming seawater temperature on kelp quality as a food source and settlement substrate. Mar. Ecol. Prog. Ser. 537, 105–119. doi: 10.3354/meps11421
Smale, D. A. (2020). Impacts of ocean warming on kelp forest ecosystems. New Phytol. 225, 1447–1454. doi: 10.1111/nph.16107
Smale, D. A., Wernberg, T., Oliver, E. C. J., Thomsen, M., Harvey, B. P., Straub, S. C., et al. (2019). Marine heatwaves threaten global biodiversity and the provision of ecosystem services. Nat. Clim. Chang. 9, 306–312. doi: 10.1038/s41558-019-0412-1
Spurkland, T., and Iken, K. (2011). Kelp bed dynamics in estuarine environments in subarctic Alaska. J. Coast. Res. 27, 133–143. doi: 10.2112/JCOASTRES-D-10-00194.1
Spurkland, T., and Iken, K. (2012). Seasonal growth patterns of Saccharina latissima (Phaeophyceae, Ochrophyta) in a glacially-influenced subarctic estuary. Phycol. Res. 60, 261–275. doi: 10.1111/j.1440-1835.2012.00657.x
Straub, S. C., Wernberg, T., Thomsen, M. S., Moore, P. J., Burrows, M. T., Harvey, B. P., et al. (2019). Resistance, extinction, and everything in between–the diverse responses of seaweeds to marine heatwaves. Front. Mar. Sci. 6:763. doi: 10.3389/fmars.2019.00763
Sunday, J. M., Pecl, G. T., Frusher, S., Hobday, A. J., Hill, N., Holbrook, N. J., et al. (2015). Species traits and climate velocity explain geographic range shifts in an ocean-warming hotspot. Ecol. Lett. 18, 944–953. doi: 10.1111/ele.12474
Tatewaki, M. (1966). Formation of a crustaceous sporophyte with unilocular sporangia in Scytosiphon lomentaria. Phycologia 6, 62–66.
Terblanche, J. S., Deere, J. A., Clusella-trullas, S., Janion, C., and Chown, S. L. (2007). Critical thermal limits depend on methodological context. Proc. R. Soc. B 274, 2935–2942. doi: 10.1098/rspb.2007.0985
Voerman, S. E., Llera, E., and Rico, J. M. (2013). Climate driven changes in subtidal kelp forest communities in NW Spain. Mar. Environ. Res. 90, 119–127. doi: 10.1016/j.marenvres.2013.06.006
Wernberg, T., Bennett, S., Babcock, R. C., de Bettignies, T., Cure, K., Depczynski, M., et al. (2016a). Climate-driven regime shift of a temperate marine ecosystem. Science 353, 169–172. doi: 10.1126/science.aad8745
Wernberg, T., de Bettignies, T., Joy, B. A., and Finnegan, P. M. (2016b). Physiological responses of habitat-forming seaweeds to increasing temperatures. Limnol. Oceanogr. 61, 2180–2190. doi: 10.1002/lno.10362
Wernberg, T., Smale, D. A., Tuya, F., Thomsen, M. S., Langlois, T. J., De Bettignies, T., et al. (2013). An extreme climatic event alters marine ecosystem structure in a global biodiversity hotspot. Nat. Clim. Chang. 3, 78–82. doi: 10.1038/nclimate1627
Wernberg, T., Thomsen, M. S., Tuya, F., Kendrick, G. A., Staehr, P. A., and Toohey, B. D. (2010). Decreasing resilience of kelp beds along a latitudinal temperature gradient: potential implications for a warmer future. Ecol. Lett. 13, 685–694. doi: 10.1111/j.1461-0248.2010.01466.x
Wiencke, C., and Bischof, K. (2012). Seaweed Biology–Novel Insights into Ecophysiology, Ecology and Utilization. Heidelberg: Springer.
Wiencke, C., Bischoff, B., Bartsch, I., Peters, A. F., and Breeman, A. M. (1994). Temperature requirements and biogeography of Antarctic, Arctic and amphiequatorial seaweeds. Bot. Mar. 37, 247–260. doi: 10.1515/botm.1994.37.3.247
Wiltshire, K. H., Kraberg, A., Bartsch, I., Boersma, M., Franke, H.-D. H. D., Freund, J., et al. (2010). Helgoland roads, North Sea: 45 years of change. Estuaries Coast. 33, 295–310. doi: 10.1007/s12237-009-9228-y
Keywords: Fv/Fm, growth, kelp, latitudes, marine heatwave, Saccharina latissima, seaweed, DPS
Citation: Diehl N, Roleda MY, Bartsch I, Karsten U and Bischof K (2021) Summer Heatwave Impacts on the European Kelp Saccharina latissima Across Its Latitudinal Distribution Gradient. Front. Mar. Sci. 8:695821. doi: 10.3389/fmars.2021.695821
Received: 15 April 2021; Accepted: 13 September 2021;
Published: 11 October 2021.
Edited by:
Gretchen E. Hofmann, University of California, Santa Barbara, United StatesReviewed by:
Dan Smale, Marine Biological Association of the United Kingdom, United KingdomSamuel Starko, University of Victoria, Canada
Copyright © 2021 Diehl, Roleda, Bartsch, Karsten and Bischof. This is an open-access article distributed under the terms of the Creative Commons Attribution License (CC BY). The use, distribution or reproduction in other forums is permitted, provided the original author(s) and the copyright owner(s) are credited and that the original publication in this journal is cited, in accordance with accepted academic practice. No use, distribution or reproduction is permitted which does not comply with these terms.
*Correspondence: Nora Diehl, bmRpZWhsQHVuaS1icmVtZW4uZGU=
†ORCID: Nora Diehl, orcid.org/0000-0002-7245-340X; Michael Y. Roleda, orcid.org/0000-0003-0568-9081; Inka Bartsch, orcid.org/0000-0001-7609-2149; Ulf Karsten, orcid.org/0000-0002-2955-0757; Kai Bischof, orcid.org/0000-0002-4497-1920