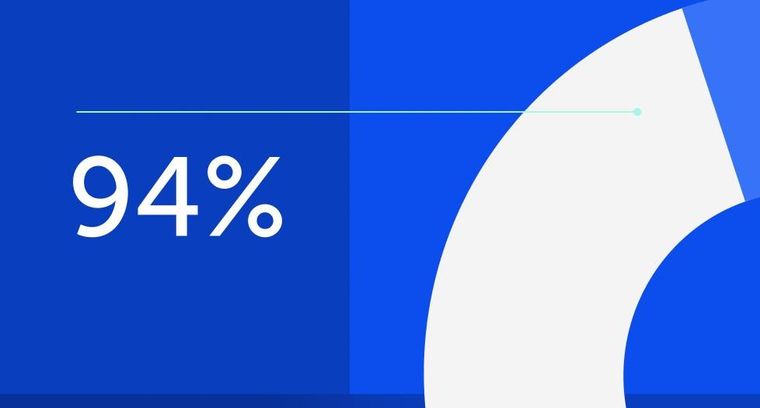
94% of researchers rate our articles as excellent or good
Learn more about the work of our research integrity team to safeguard the quality of each article we publish.
Find out more
ORIGINAL RESEARCH article
Front. Mar. Sci., 29 October 2021
Sec. Marine Pollution
Volume 8 - 2021 | https://doi.org/10.3389/fmars.2021.688224
This article is part of the Research TopicMarine Litter WindrowsView all 5 articles
The abundance and distribution of plastic debris at the sea surface shows considerable variability over different spatial scales. Some of the oceanographic processes at small (<1 km) and submeso (1–10 km) scales manifest themselves as slicks at the sea surface, which might have the potential to concentrate organisms and particles (such as positively buoyant plastics), putting species that feed in these areas at risk of ingesting plastics. Slicks can be filaments, lines, meanders, or patches, which are lighter in color and smoother in surface roughness compared to the surrounding area. Here we tested the hypothesis that passive particles (including plastics) and organisms are aggregated in the surface waters within these slicks. According to their main features (orientation to coast and/or wind), the studied slicks were most likely generated by oceanographic processes such as topographically controlled fronts, other types of fronts and internal waves. Neuston samples were collected from the sea surface inside and outside of slicks (n = 11 sites with slicks) in the coastal waters of Rapa Nui (Easter Island) during two campaigns in austral summer (January 2018) and autumn (April 2019). In general, passive particles, including plastics, exuviae, eggs and foraminiferans, were found more frequently inside than outside the slicks. In some cases, motile zooplankton organisms such as chaetognaths, vertically migrating crustaceans and early developmental stages (EDS) of fish were also more common within the slicks. In addition, a positive relationship was found between plastics and planktonic organisms such as foraminiferans, snails and jellyfish (e.g., Velella velella), although a strong correlation was also found with fish EDS and chaetognaths. These results suggest that surface slicks are areas of aggregation for both passive particles and active organisms, thus playing an important ecological role in food retention and particle concentration where the risk of plastic ingestion by fish and seabirds is enhanced.
Plastic particles are accumulating in many different marine environments (Thompson et al., 2004; Cózar et al., 2014; Eriksen et al., 2014; van Sebille et al., 2020). Many types of plastics are positively buoyant, and as passive particles they are transported by currents and concentrated in the large oceanic gyres (Maximenko et al., 2012; van Sebille et al., 2020). Dense accumulations of floating plastics, mainly from the mainland and industrial fisheries, are also found in the center of the South Pacific Subtropical Gyre (SPSG), which is close to Rapa Nui (Easter Island) (Eriksen et al., 2013, 2018; Miranda-Urbina et al., 2015; van Gennip et al., 2019).
Different oceanographic processes acting over various scales determine the spatio-temporal dynamics of organisms and particles. For example, the oceanic distribution patterns of floating plastics are driven by large-scale oceanographic processes (>200 km; Eriksen et al., 2014; van Sebille et al., 2020). Extensive convergence zones that concentrate floating particles are controlled by Ekman currents, a global process driving the large-scale oceanic circulation (Maximenko et al., 2012; van Sebille et al., 2020). These dynamics are generating (among others of smaller scale) the accumulations of floating plastics in the subtropical gyres (Martínez et al., 2009; Maximenko et al., 2012; Eriksen et al., 2014). On the other hand, the mesoscale (10–200 km) circulation in form of eddies and fronts influences the horizontal movements of particles in the open ocean (Pichel et al., 2007; Howell et al., 2012; Frère et al., 2017; Brach et al., 2018). Spatial variability of particle abundance and distribution at smaller scales (<10 km) may be due to water movements resulting from processes such as internal waves, Langmuir cells, submesoscale fronts and other related roll-like instabilities with surface convergence (Hamner and Schneider, 1986; van Sebille et al., 2020; Cózar et al., 2021; Shanks, 2021).
In particular, processes that create fronts, internal waves, and/or Langmuir cells can also generate zones of aggregation and desegregation of particles at small and submesoscales (Thomas et al., 2008; Caruso et al., 2013; Acha et al., 2015; van Sebille et al., 2020, Cózar et al., 2021; Shanks, 2021). In these frontal zones, different bodies of water are found that create horizontal gradients of physical-chemical parameters (Belkin and Cornillon, 2003). These processes of aggregation of particles often have superficial manifestations at the sea surface that are called slicks or windrows (Kingsford and Choat, 1986; Cózar et al., 2021).
Slicks have been observed in freshwater and marine environments around the world (Pingree et al., 1974; Kingsford, 1990; Marmorino et al., 2002; Belkin and Cornillon, 2003; Ryan et al., 2010). Thomson (1862) was the first to emphasize slicks as features accumulating different objects and organisms. The formation of slicks is associated with convergence zones (Kingsford, 1990; Moum et al., 1990; Caruso et al., 2013; Cózar et al., 2021), which promote surface accumulations of natural oils, lipid derivatives or phenolic materials. These surface oil films have a dampening effect on small waves (generating smooth surface areas), making the convergence zones visible at low to moderate wind speeds (Ewing, 1950; Carlson, 1982; Kingsford, 1990). In the literature, slicks are described as dynamic and short-lived features that directly depend on wind and waves for their visualization (Shanks, 2021; Whitney et al., 2021). Therefore, the slicks appear when the wind is calm, and they break up when wind speeds are equal to or greater than 8–10 m s–1, disrupting the thin oil films (Caruso et al., 2013; Gade et al., 2013).
There are many forcing processes capable of generating convergent structures, although the physics behind these small-scale structures are only beginning to be understood (van Sebille et al., 2020; Cózar et al., 2021). The main documented processes that generate slicks are different types of fronts (Marmorino et al., 2002; Ryan et al., 2010; Cózar et al., 2021), Langmuir circulation and internal waves (Kingsford, 1990; Cózar et al., 2021; Shanks, 2021). These processes play an important role in concentrating organic matter and plankton (Shanks, 1983; Kingsford and Choat, 1986; Mattos and Mujica, 2012). They are also recognized as zones of high activity for planktivorous organisms such as fish and seabirds (Bourne and Clark, 1984; Kingsford and Choat, 1986; Young and Adams, 2010; Gove et al., 2019).
The spatial accumulation and distribution of zooplankton is modulated by biotic and abiotic factors, such as wind-induced movements, eddies and tidal advection (local scale and short duration; hours to weeks; Flagg et al., 1994; Mann and Lazier, 1996; Wiafe and Frid, 1996; Pineda, 1999; Genin, 2004; Acha et al., 2015). Some of the biotic factors are food variability, physiology and behavior, which influence the temporal dynamics of planktonic assemblages. Many zooplankton organisms migrate vertically during the day (Lampert, 1989; Gibson et al., 2009) and seasonal variability is typically associated with phytoplankton growth cycles, but tropical and subtropical marine environments are traditionally considered oligotrophic, and so they have low seasonal variability (Burford et al., 1995).
The structure of the zooplankton assemblage also depends on the interactions between ecological processes and environmental factors (Locke, 1992). Neuston refers to zooplankton that are closely associated with the thin film of the immediate surface of the ocean and include organisms that are exposed to wind drift and surface currents (Zaitsev, 1970; Hempel and Weikert, 1972). While neuston organisms are closely linked to the sea surface, other zooplankton organisms adjust their position in the water column (vertical migration) in function of their sensorial capacities, which vary depending on the taxa and ontogenetic state of the individuals (Pineda, 1999; Kingsford et al., 2002; Whitney et al., 2021). The accumulation of planktonic organisms within the slicks is also affected by the organisms’ ability to move (Weidberg et al., 2014). Some organisms aggregate passively because of convergence or a poor ability to swim. However, other organisms (e.g., fish larvae) may also aggregate actively in slicks, taking advantage of a large supply of prey within slicks (e.g., Gove et al., 2019; Whitney et al., 2021). In contrast to zooplanktonic organisms, the spatial and temporal distribution of passive and positively buoyant particles, such as wood, volcanic rocks, floating algae, remains of organic matter, exuviae, eggs and plastics (often found in neuston samples), responds mainly to physical processes, and they drift along the sea surface, often directly being pushed by the wind and surface currents (Son et al., 2015; van Sebille et al., 2020). It is because of this that in zones of particle aggregation, all these different types of passive particles (including plastics) are expected to accumulate, and consequently their densities at the sea surface should be positively correlated with each other. In contrast, some of the more agile zooplankton organisms have the capacity for active swimming and vertical migration, and consequently their densities may not be directly correlated with that of passive particles and plastic.
These considerations suggest that there is a potential ecological risk caused by overlapping plastic particles and zooplankton. This risk should be particularly high in the subtropical gyres where large amounts of microplastics are concentrated (Eriksen et al., 2014; van Sebille et al., 2020) in areas of low overall productivity (Morel et al., 2010). Here meso- and submesoscale convergence events have the potential to locally concentrate many small plastic particles and organic matter in zones of elevated primary production (Denman and Gargett, 1983; Williams, 2011). Slicks may therefore be attractive for fish and seabirds (Rothschild and Osborn, 1988; Sundby and Fossum, 1990; Mackenzie, 2000; Young and Adams, 2010; Dell’Ariccia et al., 2014) that would be drawn to these productivity hotspots (contaminated by plastic pollution) within the otherwise oligotrophic waters of the open ocean. Slicks can accumulate large amounts of organic matter and organisms, which can also be accompanied by plastic debris. For example, Bourne and Clark (1984) mentioned plastic litter off the coast of Chile in what they called a “line of scum,” which would be equivalent to slicks. Similarly, Young and Adams (2010), Law et al. (2014), and Gove et al. (2019) found large concentrations of plastics within these slicks, which could accumulate plastic along with other passive particles and low-mobility organisms. The slicks are therefore sporadic and ephemeral oases for many organisms, from plankton to large predators such as fish and seabirds (Godø et al., 2012). Thus, the overlap between plastic particles and zooplankton enhances the risk for various species, including visual predators such as seabirds and fish (larval fish and pelagic fish; Gove et al., 2019), as they will be exposed to high concentrations of plastic when foraging for their prey (Young and Adams, 2010; Fossi et al., 2017; Ory et al., 2017, 2018).
Rapa Nui is a good model system to study this potential overlap of plastics and zooplankton/neuston organisms as the abundance of microplastics in the waters surrounding Rapa Nui is greater (>50,000 microplastics km–2) than what is expected from a remote, non-industrialized island (Eriksen et al., 2013, 2018; Ory et al., 2017). This is probably due to Rapa Nui’s proximity to the SPSG, where plastics are concentrated (Martínez et al., 2009; Eriksen et al., 2013, 2018; van Gennip et al., 2019). Despite the high densities of microplastics in the surface waters around Rapa Nui, there is substantial variation on a mesoscale and submesoscale between sampling stations (Ory et al., 2017; Eriksen et al., 2018). In addition, Rapa Nui has hyper-oligotrophic conditions (Morel et al., 2010) and it has been reported that chlorophyll α and meroplankton concentrations are associated with geostrophic flow, vertical migration, larval behavior, and the generation and release of submesoscale eddies and other structures (Andrade et al., 2014a; Meerhoff et al., 2017).
The aim of this study was to compare the abundance of plastics and organisms inside and outside of visually identified slicks from coastal waters of Rapa Nui. In particular, we tested whether there is a positive correlation between the abundance of inert particles and zooplankton with that of plastic particles. In addition, the possible processes that cause the variability in the abundance of plastics at small (<1 km) and submeso (1–10 km) scales were explored.
All sampling was done along the southwest coast of Rapa Nui (Figure 1). Rapa Nui is located in close vicinity to the center of the South Pacific Subtropical Gyre (Luna-Jorquera et al., 2019). The biological importance of this region (Rapa Nui Ecoregion) is supported by the observed increase in coastal phytoplankton biomass with respect to the subtropical oligotrophic gyre, which has been described as the most transparent waters in the world (Pizarro et al., 2006). The coastal circulation around Rapa Nui is affected by the island’s topography and by the wind system, with the possibility of hourly and daily variations (Argandoña and Moraga, 2000). The geostrophic currents observed around Rapa Nui flow predominantly in northeast direction (Moraga et al., 2010; Meerhoff et al., 2017) and are influenced by mesoscale eddies (Andrade et al., 2014a). Slicks are commonly observed, especially close to three small islets (called “motu” by the local people). At smaller spatial scales close to Hanga Roa harbor and to these motu, we sampled inside and outside of natural slick lines that were visually identified from a small boat.
Figure 1. Map and position of transects in the slicks from Rapa Nui. All slicks identified with a letter in the order they were sampled in (red letter) 2018, and (black letter) 2019.
Paired samples (inside and outside of slicks) were collected during summer, on the 6th, 12th, and 13th of January 2018 (eight samples in total, four inside and four outside of slick), and autumn, on 5th and 6th of April 2019 (14 samples in total, seven inside and seven outside of slick). Thus, throughout this study we collected a total of 11 samples inside slicks and 11 outside the slicks (Table 1). The weather conditions differed between the two sampling campaigns. In 2018, during the days before the sampling the average wind speeds were 4.1 ± 1.1 m s–1. During the days before the sampling in autumn 2019, there were strong wind and heavy rainfall, with average wind speeds of 5.6 ± 2.0 m s–1. The average wind speeds in 2019 were greater than those in 2018 (Supplementary Table 1). All daily wind, temperature and precipitation data were obtained from the Dirección General de Aeronáutica Civil (DGAC), specifically from the meteorological station located at the MATAVERI airport (Figure 1), belonging to the Dirección Meteorológica de Chile1. The wind speed and temperature reported in Table 1 correspond to the average conditions, as reported by the DGAC, during the duration of each sampling inside and outside the slicks.
The presence of a slick was visually detected by two observers on board of a small fishing boat. Slicks were characterized by a long streak at the sea surface that appeared smoother than its surroundings, sometimes with foam, bubbles, and/or floating debris and a marked change in the color of the water (Figure 2). Only clearly visible slicks stretching at least over a couple of hundreds of meters were examined in this study. A decision tree was created with the main oceanographic processes that generate the surface features, based on the literature, with the main characteristics of each process (Figure 3). The number of slicks observed may be useful to identify the process of origin of the slicks (Kingsford, 1990), as several lines unrelated to the wind may indicate internal waves (Kingsford, 1990; Woodson, 2018). The internal wave packets can be separated from each other by meters up to several kilometers, so from land or from a boat a single visible wave can be observed, although lonely internal waves that generate slicks have also been documented (Ermakov et al., 1992; Woodson, 2018). Several slick lines parallel to the wind can be indicative of Langmuir cells (Leibovich, 1983), although Langmuir cells may also occasionally converge (see Chubarenko et al., 2010). On the other hand, there are the slicks originated by the fronts controlled by local topography (Kingsford, 1990). We distinguish the island mass effect (which is usually at larger scales) in which the slick is associated with the direction of the main currents (Hernández-León, 1991). Headland fronts and islet wakes, on the other hand, generate slicks that are typically aligned to the wind and originate from an island mass, coastal point or shallow subtidal reef (Pingree et al., 1978; Wolanski and Hamner, 1988; Kingsford, 1990; Pattiaratchi, 1994). Based on this decision tree, the most probable processes that generated the slicks sampled in Rapa Nui during the two campaigns were qualitatively deduced.
Figure 2. Examples of sea slicks (red arrows) visible at the sea surface along the coast of Rapa Nui. Sea slicks are characterized by (a,b) a smoother sea surface than the surrounding water, (c) changes in water color, and (d) the presence of foam, bubbles and/or floating debris. Sampling dates: (a) 12 January 2018; (b–d) 5 May 2019.
Figure 3. Decision tree with the possible processes and main characteristics that generate slicks. Diagram shows images that were modified based on examples by Katherine Smith 2019: (https://www.integratedecosystemassessment.noaa.gov/regions/hawaii/hi-surface-slicks). Note for single slicks: In the case of finding a slick that is positioned parallel to the coast and unrelated to the wind, it appears that it is a solitary internal wave, e.g., when the wave train has a wavelength of up to 16 km. In the case of finding a slick that is parallel to the wind, unrelated to the coast and has arms or Y-shaped protections, it is possible that they are amalgamation/destruction of Langmuir circulation cells by the background topography. Black arrows indicate direction of wind or currents, as applicable.
A total of 22 trawls were conducted, of which 11 trawls were collected inside the slicks and 11 trawls were collected outside the slicks (approximately 200 m away). At each sampling site, the DiSalvo neuston net was towed with a fishing boat at a speed of approximately 1.5–2.0 knots (mean: 1.7 knots). Once a slick was detected, the boat moved to one end of the slick. The vessel followed the longitudinal edges of the slicks, so the sampling time depended on the length of each slick. At no time did we pass through an area of the slick that had been sampled previously. The slicks sampled were wide and well-formed, sufficient for the vessel’s movement to not disturb the slick. It is also important to mention that at the time of the CTD station inside the slick, the opening of the DiSalvo net was raised to stop the epineustonic sampling. The length of the different slicks was variable, but the net was towed in each slick on average for 40 min in 2018 and for 12 min in 2019. The sample outside the slick was taken parallel and at about 200 m distance to the slick, over the same distance and time as the sample taken inside the slick.
The vertical temperature and salinity profile of the water were measured with a CTD (48M Sea & Sun Technology GmBH) that was manually lowered with a rope from the surface to 20–40 m depth. The CTD measurements were made once in the center of the slick and halfway through the sampling outside the slick, in both years. In addition, in 2019 before the start of a trawl inside a slick, a transect was made crossing the slick in perpendicular direction and the horizontal sea surface temperature was measured with a HOBO data logger attached to the underside of the frame of the DiSalvo net (without codend).
As an indicator for the seasonal variability of currents in the motu zone, current measurements taken between 30 March 2015 and 21 February 2016 were used. These measurements were conducted with a RDI Workhorse 300 kHz Acoustic Doppler Current Profiler (ADCP), which collected data every 30 min with a vertical bin resolution of 1 m. The instrument was located at 100 m depth (27.214°S; 109.4412°W). The surface data (12 m) were included to compare the average current direction with the main direction of the observed slicks, which allowed us to infer the possible influence of these islets on slick generation. The mean surface currents were obtained for autumn (May 2015) and summer (January 2016) as an indicator of monthly and seasonal current variability for the study of slicks (summer 2018 and autumn 2019).
Neuston samples were collected with a DiSalvo neuston trawl (rectangular aperture: 80 cm wide × 40 cm high; 0.32 m2 opening area) mounted with a 2.2 m long epineustonic net with a 300 μm mesh opening closed by a PVC collector cod end (11 cm diameter × 29 cm length; Eriksen et al., 2018). The volume of the water filtered through the net was estimated with a HydroBios flowmeter attached across the middle of the trawl opening. The volume (V) of the water filtered through the net was determined using the following equation provided by the supplier2 : V (m3) = (net opening m height x net opening m width/2) × total number of flowmeter revolutions × 0.25 m. The area is divided by 2, because only the lower half of the net is submerged. The constant 0.25 corresponds to the pitch of the impeller (i.e., 0.25 m per revolution).
After each sampling, the net was briefly towed without the cod end to remove potential neuston debris remaining from a previous sample from the interior of the net (see Ory et al., 2017). At the end of each sampling, the content of the collector was transferred into 500 ml vials, sealed, and placed into a cooler. In the laboratory on Easter Island, the water samples were filtered through a 300 μm sieve and fixed in 95% ethanol for further analysis in the laboratory at the Universidad Católica del Norte of Coquimbo, Chile.
Water samples were visually examined under a stereoscopic microscope to sort out all potential plastic particles. Every potential plastic particle was retrieved, its shape (fragment, line, film, foam, pellet, other) and color was determined following the description by Ory et al. (2017), and it was photographed with a Canon Powershot SX210 IS mounted to the dissecting microscope. The size of each plastic particle was measured to the nearest 0.1 mm from the pictures using ImageJ3. Fibers (diameter ≤ 0.05 mm) were not recorded in this study due to the high risk of contamination with airborne fibers during the collection and processing of the samples in Rapa Nui and Coquimbo, where no chamber with clean air flow was available (see Foekema et al., 2013 and Kühn et al., 2020).
The chemical signature of a subset of 204 (9.4%) of the 2167 visually examined potential microplastics was analyzed using Fourier-transform infrared spectroscopy (FTIR) with an Agilent Cary 630 FTIR spectrometer. The obtained spectra were compared using siMPle version 1.0.1 (Primpke et al., 2020) with the siMPle ATR single spectra IR library version 1.0.2 (Primpke et al., 2018). Only spectra with a correspondence of ≥70% (Lusher et al., 2013) were considered to describe the particles’ polymer types. The diamond tip and sampling plate were cleaned between each measurement with a cellulose cloth.
After sorting out all plastics, large-sized particles and organisms were separated from the sample, to avoid overestimating the abundance of these large particles or organisms (i.e., feathers, piece of wood, etc.). Then the remaining neuston samples were split into equal parts (usually in two or four portions) using the Folsom methodology and device (McEwen et al., 1954; Alden et al., 1982). Zooplankton organisms were identified and grouped according to the most distinctive morphological characteristics of each taxonomic group. All zooplankton organisms and passive particles (crustacean exuviae, organic and inorganic material such as wood, volcanic stones, algae, etc.) were classified into four ranges of mobility indices. The criteria for the mobility index were based on the structures and ability to move in the water column. Category 0 is a passive particle with no movement capacity and no appendages to move (such as foraminiferans and eggs), category 1 represents organisms with reduced movement capacity and no appendages to move (e.g., Velella velella and polychaete larvae with spines), category 2 represents organisms with movement capacity with appendages to move (e.g., zoea larvae of brachyuran and anomuran crabs), and category 3 is for organisms with high movement capacity, and appendages modified for swimming (e.g., copepods and euphausiids; see Supplementary Table 2 with the indices of each group). The abundance of each group was first calculated by multiplying the number of individuals found in a section of Folsom by the number of times divided. Then, the total number of individuals was divided by the filtered volume (per cubic meter). After sorting and counting, all organisms in a sample were stored by taxa in 70% alcohol vials.
We used single-factor non-parametric statistical analyses and in particular the paired Wilcoxon test to compare plastic abundances between inside and outside the slicks as the normality and homogeneity of variance of the data was not met (Q-Q plots and Levene’s test). Also, a Mann–Whitney U-test was used to compare the microplastic abundance between the two sampling years. Spearman’s correlation coefficients were used to compare the overall abundance (inside and outside the slicks pooled together) of inert particles and plastics. All analyses and plot were performed in R Studio v.3.4.3 (Package Performance Analytics version 1.5.3; Peterson and Carl, 2019, Package corrplot version 0.84; Taiyun and Viliam, 2017 and ggplot2 version 3.3.2, Wickham, 2016). In addition, an association index was calculated for all particles and organisms, where the value of abundance per m3 inside the slick was divided by the value outside the slick; in the cases where the inside value is divided by zero, for conservative reasons we kept the original inside-slick value and there was no case where a number smaller than 1 was divided by 1. This association index was used to determine whether the highest concentrations of individuals/particles were inside or outside the slicks.
The average wind speed recorded by DGAC during the time of slick sampling was 1.5 ± 0.4 m s–1 in 2018, ranging from 0.9 to 1.8 m s–1. In 2019, average wind speed was 4.1 ± 0.7 m s–1 and the ranges were 2.8–4.8 m s–1 (Table 1). Five of the 11 slicks were parallel to the coast, but the position was more related to the wind direction and close to the islet (motu) at the same time, and five of all 11 slicks were close to the motu (Table 1). Based on the visual characteristics of slicks on Rapa Nui, such as the visible number of slicks, shape, position relative to the wind, coast or islet, the processes most likely to have generated the slicks are considered to be topographically controlled fronts (such as headland fronts or island wakes; Table 1), other types of fronts and internal waves. The length and width of the slicks are approximately 100–1,000 m and 3–20 m, respectively. In many slicks we observed foam or bubbles on the smooth surface inside the slicks. Furthermore, at two slicks we observed the presence of masked boobies Sula dactylatra.
The vertical temperature profiles from 2018 (Supplementary Figure 1) show that there was a trend of profiles with a weak stratification (thermocline), while in 2019 (Supplementary Figure 1) there was no stratification, and no shallow thermoclines were observed. The horizontal temperature profiles taken across the visible slicks showed a strong temperature gradient between a cold and a warmer side (Supplementary Figure 2 and Supplementary Table 3). On the other hand, mean currents obtained in 2015–2016 from the ADCP mooring (used as indicator for monthly and seasonal variability in the zone) for autumn (May 2015) and summer (January 2016) show that the general pattern of the surface currents (12 m) near the motu matches the direction of several slicks observed in both 2018 and 2019, for example, “a” and “h” (Figure 1 and Supplementary Figure 3).
A total of 2,167 plastic particles were found in 22 water samples, with a median concentration of 0.27 ± 0.57 particles m–3 (median ± interquartile range; range = 16). We found significant differences between the abundance of plastics inside (0.29 ± 0.93 particles m–3; median ± interquartile range) and outside (0.097 ± 0.35 particles m–3; median ± interquartile range) of the slicks, with more plastics inside than outside the slicks (p < 0.005; Z = −2.8; Figure 4). Significantly more plastics were found in 2018 than in 2019 (p < 0.001). The median and interquartile range of plastic concentrations in 2018 (summer) was 1.26 ± 3.83 particles m–3, while the median in 2019 (autumn) was 0.13 ± 0.18 particles m–3.
Figure 4. Density of microplastics (plastics/m3) inside and outside the slicks. The lines represent pairs of slicks, (2018) n = 4 pairs of slicks, and (2019) n = 7 pairs. Note that the scale is different between 2018 and 2019.
The plastic particles found in the samples had median length of 1.00 ± 1.00 mm (median ± interquartile range). In 2018, the majority (75%) of the plastics were <2 mm, while in 2019 this size fraction made up only 42% of all particles; mesoplastics (>5 mm) comprised 9% of all plastic particles in 2018 whereas it was 29% in 2019 (Table 2). Fragments, lines and films predominated in all samples, and most of these plastics were white/transparent and blue/green (Table 2). The FTIR analysis confirmed that >87% of the analyzed particles were plastics. Of these, 65.2% (n = 133 particles) were polyethylene (PE) and 10.8% (n = 22) were polypropylene (PP) (see Supplementary Table 4).
Table 2. The characteristics of the mesoplastics (>5 mm) and microplastics (<5 mm) found inside and outside the slicks.
Ten taxonomic groups were found: crustaceans (58.6%), foraminiferans (26%), mollusks (7.2%), fishes (2.4%), cnidarians (1.9%), polychaetes (1.7%), chaetognaths (1.2%), echinoderms (0.7%), insects and sponges (both found <0.0%). For the analyses, only those groups with >1% occurrence were used. On the other hand, many passive particles such as eggs, pieces of wood, algae, rocks, crustacean exuviae, dead insects, among other inert particles, were found. In general, eggs and foraminiferans were most abundant. The abundance of organisms inside and outside of the slicks varied between years for some organisms, with particles and organisms with low movement ability being more abundant in 2018 (austral summer) than in 2019 (austral autumn; Figure 5); in the case of Velella velella no individuals were found in 2019. The abundance of eggs (median = 3.99 particles m–3 inside vs. 2.66 particles m–3 outside) and foraminiferans (median = 0.59 particles m–3 inside vs. 0.35 particles m–3 outside) was significantly higher inside the slicks than outside (p < 0.02; Supplementary Table 5), and they were found in lower abundance in autumn 2019 than in spring 2018 (Figure 5). For the more mobile organisms, motile crustaceans were four times more abundant in 2019 than in 2018 (U = 27; p < 0.05; Figure 5 and Supplementary Table 5), mostly because of the high abundance of copepods and brachyuran larvae in 2019 (see Supplementary Figure 4 for details of the crustaceans). However, other mobile organisms such as fish and chaetognaths were more abundant in 2018 than in 2019 (Figure 6).
Figure 5. Main passive particles and organisms. Density of organisms and particles per m3. Note that the scale is different between (A) and (B). Plastic abundance are shown for reference purposes.
Figure 6. Main motile organisms. Density of organisms found inside and outside the slicks in 2018 (A) and 2019 (B). The groups are ordered by their mobility in the water column. Plastic density is shown for reference purposes. Insert shows less abundant groups with a different scale for better reference.
Of the passive particles, exuviae (median = 3.40 particles m–3 inside vs. 0.31 particles m–3 outside) were more abundant inside than outside the slicks both years (p < 0.03; Figure 5 and Supplementary Table 5). Moreover, for highly motile organisms, such as chaetognaths and early developmental stages (EDS) of fish (2–5 mm total length), there were significantly more incidences where there were more individuals inside than outside the slicks (p = 0.006; Supplementary Table 5). A positive relationship was found between plastics and passive particles such as foraminiferans, eggs and jellyfish (Spearman’s coefficient: 0.70, 0.74 and 0.72, respectively; Figure 7A and Supplementary Figure 5), although a strong correlation was also found with fish and chaetognaths (Spearman’s coefficient: 0.79 and 0.64, respectively; Figure 7B and Supplementary Figure 6).
Figure 7. The correlation matrix between (A) plastics and groups with no or moderate motility, and (B) plastics and groups with high motility. Positive correlations are blue and negative correlations are red, the color intensity is proportional to the significance of the Spearman correlations coefficients and the number inside the box corresponds to Spearman’s coefficient (i.e., the closer to –1 or 1). Crustacean M, crustaceans with moderate mobility; Crustacean H, crustaceans with high mobility; Polychaete L, polychaete larvae; Fish J, early developmental stages of fish. The order of the groups is given by the mobility index: crustaceans were grouped into moderately mobile species (M: index 0–1) and highly mobile species (H: index 2–3; see Table 1).
The slicks sampled during both sampling campaigns in Rapa Nui were most likely generated by topographically controlled fronts and internal waves. It is possible that some of the slicks sampled during the summer of 2018 in Rapa Nui were related to internal waves, since the water column was more stratified and there was less wind, conditions that are favorable for the propagation of internal waves (Holloway et al., 1997; Walter et al., 2014). Internal waves have frequently been reported to contribute to the generation of slicks (Ermakov et al., 1992; Law et al., 2014; Gove et al., 2019; Whitney et al., 2021), which may be common in stratified water columns with a shallow thermocline (Kingsford, 1990). In general, internal waves are considered to generate slicks that are unrelated to wind (Dietz and Lafond, 1950; Ewing, 1950; Woodson, 2018; Shanks, 2021).
Slicks near small islets could be generated by the topographic interaction of the islets with the current parallel to the coast as inferred for some of the slicks near the motu of Rapa Nui. These slicks, likely generated by topographically controlled fronts, are similar to those observed by Miller (1974) in Hawaii, where Whitney et al. (2021) also reported some slicks generated by topographic fronts. These headland fronts generate complex flows and particle retention (Pingree et al., 1978; Wolanski and Hamner, 1988). The wind-induced wakes that form on the leeward side of the islands are protected from the wind, so surface mixing and/or heat loss is minimal, which is why the wakes are characterized by higher temperatures (Fosberg, 1957; Wolanski and Hamner, 1988; Kingsford, 1990; Caldeira et al., 2005). Therefore, the wakes on the leeward side of the islets could generate a temperature gradient, possibly similar to the one found in the slicks sampled in Rapa Nui, but on a smaller scale, also related to the size of the islets (motu). Even in the case of slicks that are not related to islets, it has been reported that fronts or convergence zones are almost always accompanied by stronger horizontal temperature gradients (Fedorov, 1986; Belkin and Cornillon, 2003). This is also observed for the gradients of the other slicks sampled without direct topographical influence. However, no shallow thermoclines were observed in 2019, probably because on Rapa Nui the thermocline is described to be usually at great depths, greater than 80 m (Moraga et al., 1999; Cornejo et al., 2016), which could explain the lower concentrations of microplastics in the slick zones during this year.
Another possible process is reported by Jones (1962) who showed evidence for the mass effects of the Marquesas Islands in the equatorial Pacific. This has also been observed in coastal waters of Juan Fernandez Island (Andrade et al., 2014b) and the Island of Gran Canaria (Hernández-León, 1991). The slicks for which we could not infer the generating mechanism are possibly the expression of small submesoscale eddies, which are the main process behind the island mass effect, as mentioned by Andrade et al. (2014b) or Meerhoff et al. (2017), describing submesoscale (<10 km) structures in chlorophyll distribution around Rapa Nui.
The determining factor in the generation and duration of some slicks could be the wind speed (Romano, 1996). Langmuir circulation may be the engine creating the parallel windrows sampled during autumn (2019) in Rapa Nui, similar as had been reported from Catalina Island in California (Alldredge, 1982). We also realized that it was more difficult to identify and find slicks at higher wind speeds. Slicks are dynamic and have a short lifetime (Caruso et al., 2013; Gade et al., 2013). Indeed, three of the eleven slicks sampled in this study could not be matched with a possible mechanism of origin. Future studies are needed to better understand the formation of oceanic slicks: long-term observations and frequent measurements of temperature profiles and local currents together with remote sensing data (e.g., Whitney et al., 2021) will help to determine the spatio-temporal occurrence of slicks and the underlying processes. It is important to highlight that our current results have certain limitations (sampled in different seasons, few slicks sampled and with basic equipment), and need to be confirmed in future studies with higher spatio-temporal resolution.
In this study, the overall densities of microplastics were higher in summer 2018 than in autumn 2019, which could be due to contrasting weather conditions (storm, rain, and strong winds) during these different sampling seasons. The 2019 sampling followed a period of stormy days with strong wind and rainfall, whereas the 2018 sampling was conducted during a period of relatively calm weather. In the open ocean, the amounts of microplastics collected by surface trawls are reduced during stormy weather due to wave-induced mixing (Eriksen et al., 2013; Reisser et al., 2015), as the buoyant plastic particles at the sea surface are mixed both vertically and horizontally by wind and waves (Kukulka et al., 2012; Reisser et al., 2015). Thus, the observed differences in plastic abundances and sizes at the sea surface may be mostly due to differences in wind-mixing and pushing of smaller plastics into subsurface layers during the 2019 sampling, when the densities of microplastics were lower. Due to their high buoyancy larger plastic particles are less likely to be pushed into deeper water layers by wind or wave surges, which might have led to the higher proportions of mesoplastics (>5 mm) in 2019. Similarly, horizontal movements produced by internal waves can be slowed by the strong actions of wind and waves (Jeans and Sherwin, 2001; Shanks, 2021), which could break or decrease the surface concentration of zooplankton in the slicks generated by internal waves (Owen, 1981; Kingsford and Choat, 1986).
Vertical water movements occur in mixing zones (convergence zones) and upwelling regions (divergence zones), which directly influence the production and distribution of plankton organisms in the water column (Zeitzschel, 1978). Therefore weather conditions (mainly wind) between the two seasons could have influenced the formation or duration of slicks and the abundance of particles and zooplankton organisms in the slicks. For example, Jamodiong et al. (2018) reported that 1 year there were few slicks due to extended wind turbulence and the following year the surface water conditions were calmer, which allowed the formation of slicks that were wide and extended up to at least 4 km.
The differences we found in zooplankton abundance between summer 2018 and autumn 2019 could be due to physical factors (waves or wind) and/or seasonal variability of plankton abundances. The differences between summer (2018) and autumn (2019) abundances in foraminiferans and eggs (less in autumn 2019; Figure 5) might be due to mixing induced by waves and wind, which might act in a similar way on eggs and foraminiferans as discussed above for plastics. In addition, the annual cycles of zooplankton quantity and composition are related to key environmental variables, e.g., surface temperature, nutrient availability, chlorophyll concentration (Mackas et al., 2012). In Rapa Nui, due to the contrasting nutrient availability, the highest concentrations of Chl-a occur during the winter (the minimum concentrations were reported for autumn and spring), when the mixed layer is deep (McClain et al., 2004; Andrade et al., 2014a; von Dassow and Collado-Fabbri, 2014). For organisms with meroplanktonic larvae it has been observed that the timing of spawning may have evolved to maximize larval survival. For example, spawning typically coincides with the highest primary productivity (Starr et al., 1990; Highfield et al., 2010). Thus, the difference in abundances between the two seasons may also be due to spawning times or presence of early larval stages. Fish eggs were found in greater abundance in summer 2018, coinciding with the spawning period reported for some fish species, which occurs during the months of November and January in Rapa Nui or the subtropical zone (Sazima and Sazima, 2001; Schaefer, 2001; Vega et al., 2009).
Our results indicate that high densities of plastics accumulate within the slicks in Rapa Nui coastal waters, which is consistent with reports from elsewhere in the world (Young and Adams, 2010; Gove et al., 2019; Cózar et al., 2021; Shanks, 2021; Whitney et al., 2021). Thus, the frequent (albeit ephemeral) formation of slicks further exacerbate the high densities of microplastics accumulating within the subtropical gyres (e.g., van Sebille et al., 2020), which are then further concentrated in the slicks. It is likely that the high variability in plastic concentrations found by Ory et al. (2017) and Thiel et al. (2018) around the island is due to samples that have passed through these slicks on small scales.
On the other hand, in both sampling periods, zooplankton and passive particles were more frequent within the slicks, suggesting that there may be active and passive accumulation, respectively, by the effect of physical processes. The slicks are found in convergence zones where organic matter and organisms with low swimming capacity and high buoyancy accumulate (Kingsford, 1990; Cózar et al., 2021). Thus, swimming capacity and buoyancy are considered important in determining whether organisms resist subduction and are aggregated in the slicks (Olson and Backus, 1985; Kingsford, 1990; Pineda, 1999). In some organisms, swimming ability and positive phototaxis (Zeldis and Jillett, 1982) or the amount of lipids and the ability to alter buoyancy with swim bladders (Kingsford and Choat, 1985, 1986) allow them to escape the convergent zones of internal waves. The accumulation of passive particles and organisms with limited mobility (e.g., eggs and larvae of polychaetes) inside the slicks observed here is generally consistent with studies in coastal waters (Mattos and Mujica, 2012; Whitney et al., 2021). Similarly, densities of plastic and floating organic matter or algae (passive particles) were generally higher in the slicks than in adjacent waters (Kingsford and Choat, 1986; Young and Adams, 2010; Gove et al., 2019). Therefore, the abundances of these passive particles, plastics and organisms may depend on the timing of slick generation, the intensity of vertical displacement in the convergence zone, the seasonal variation in plankton abundance and the physiology and ability to stay afloat (Kingsford, 1990; Shanks, 2021).
On the other hand, zooplankton and some of the more mobile organisms, such as fish EDS, were also found in greater abundances inside than outside the slicks, which is consistent with findings of Gove et al. (2019) and Whitney et al. (2021). These organisms with greater mobility may be concentrated in the slicks both by oceanographic processes and by their behavior (Zeldis and Jillett, 1982; Kingsford and Choat, 1986; Shanks and Wright, 1987; Weidberg et al., 2014). The positive correlation between inert particles and swimming organisms may be due to the ecological role played by slicks in accumulating and concentrating food (Kingsford and Choat, 1986), effectively functioning as fish nurseries (Gove et al., 2019; Whitney et al., 2021), and transporting meroplankton organisms toward the coast (Shanks, 1983, 1986, 2021; Kingsford and Choat, 1986; Weidberg et al., 2014). Zooplankton organisms, in addition to being affected by oceanographic processes at the ocean surface, also have behaviors that maintain them at the surface, such as sustained swimming, which would be directly related to ontogeny-dependent sensory capabilities and morphology (Kingsford et al., 2002). Many fish EDS migrate vertically and some species are near the sea surface during the day (Olivar et al., 2016). These organisms could be distributed vertically and horizontally according to their ontogenetic stage (Shanks, 1986; Morgan and Fisher, 2010), which can influence their abundances both inside and outside the slicks. Thus, the concentrations of organic matter and zooplankton prey in the slicks may attract active swimmers such as fish larvae, which seek out these areas for better feeding conditions (Gove et al., 2019; Whitney et al., 2021).
Our results indicate that slicks are surface convergence zones that accumulate high abundances of plastics, inert particles, zooplankton organisms, and juvenile fishes. Other visual predators, including ocean skaters Halobates and larger fishes that are also attracted to slick zones, might have escaped capture due to the type of sampling (slow with DiSalvo neuston trawl). In order to adequately sample these larger, more mobile predators, other types of nets, with wider mouths or nets that can be towed at a higher speed (such as AVANI – see Eriksen et al., 2018) should be used.
Within the slicks all kinds of particles and organisms are accumulated, including both active and passive swimmers (Kingsford and Choat, 1986; Bakun, 2006; Mattos and Mujica, 2012; Gove et al., 2019; Whitney et al., 2021). This is due to the different mechanisms that affect the retention and movement of particles/organisms in slicks and convergence zones (Shanks, 1983; Shanks et al., 2000; Weidberg et al., 2014). In this way, meroplankton is carried to, or in some cases away from, coastal environments (Shanks, 1983, 1986; Kingsford and Choat, 1986; Kingsford, 1990; Pineda, 1999; Woodson et al., 2012; Weidberg et al., 2014). The association of slicks with reefs should facilitate the retention of meroplankton over varying periods of time (Kingsford, 1990). It is known that fronts can change position depending on the time of year (e.g., Mulhearn, 1987). Therefore, the position of the slick could potentially influence the settlement rates of meroplanktonic organisms on small islands or reefs (Kingsford, 1990). In addition, the favorable conditions of food retention within the slicks makes these a nursery place for many species, including EDS of fish (Gove et al., 2019; Whitney et al., 2021). Thus, slicks are temporary oases in the highly oligotrophic waters surrounding these oceanic islands (Godø et al., 2012). Also, slicks are hotspots in the interaction of plastics and marine organisms (Cózar et al., 2021).
Plastics were found to be more abundant inside the slicks than outside, which is consistent with other observations where slicks were found to retain and accumulate plastics (Young and Adams, 2010; Gove et al., 2019; Cózar et al., 2021; Shanks, 2021). Slicks concentrate prey and plastics and thereby increase the likelihood of encounter and ingestion by visual predators such as fish (Ory et al., 2017; Gove et al., 2019). EDS of fish found inside slicks consumed 2.3 times more plastic than those outside the slicks (Gove et al., 2019). Ingestion of plastics could reduce the survival of these EDS and/or convert them into a vector transferring plastics to other species that feed on these small fish, such as tunas, seabirds, or even large whales (Young and Adams, 2010; Fossi et al., 2017; Chagnon et al., 2018).
This study confirms higher concentrations of plastics and organisms inside than outside the slicks, with internal waves and topographically controlled fronts being the most likely processes contributing to slick formation in the coastal waters of Rapa Nui. The different densities of organisms and plastics between the two sampling years (summer 2018 and autumn 2019) could be due to seasonal cycles (biology) and meteorological conditions affecting the dynamics of slick formation and passive particle concentration. Future work should provide measurements of vertical and horizontal currents, as well as estimates of the abundance of organisms and plastics at different depths inside and outside the slick.
The positive relationship between the densities of plastics and passive organisms with lower mobility supports the suggestion that slicks concentrate particles. However, a positive relationship was also found for some organisms with higher mobility such as juvenile fish, suggesting that these groups actively approach these slicks for feeding or transport to shore (Gove et al., 2019; Shanks, 2021; Whitney et al., 2021). These slicks retained significant amounts of plastics, lower trophic level taxonomic groups, and juvenile fish. This suggests that organisms within the slicks are susceptible to ingestion of plastics (Gove et al., 2019), and transfer to higher trophic level organisms could be a result. Slicks play an important ecological role in food retention and aggregation, where the risk of ingestion of plastics by mistake (confusion with their natural prey) or indirectly (with their prey organisms) appears to be high in visual predators such as seabirds, fish, and megafauna species that feed in these areas (Ory et al., 2017, 2018; Gove et al., 2019; Hidalgo-Ruz et al., 2021).
While evidence is growing that slicks accumulate plastics and organisms, the spatio-temporal dynamics of slick formation, persistence and break-up are still poorly known. Since these dynamics govern the organism-particle interactions within these ephemeral hotspots of productivity and plastic pollution (Cózar et al., 2021), future studies should focus on the ecology in the slick zones. This is of particular relevance in the oligotrophic subtropical gyres, which are characterized by high concentrations of microplastics and where slick zones are thought to be temporary oases attracting a wide range of organisms, including fish larvae and long-distance foragers.
The original contributions presented in the study are included in the article/Supplementary Material, further inquiries can be directed to the corresponding author.
MT, NO, and CG contributed to the conception and design of the study. LB and MR contributed with the oceanographic data and analysis. CG and MG organized the database and performed the statistical analyses. CG, MG, and MT wrote the first draft of the manuscript. All authors contributed to manuscript revision, read, and approved the submitted version.
Funding was provided by ANID – Millennium Science Initiative Program – Millennium Nucleus for Ecology and Sustainable Management of Oceanic Islands ESMOI (NC120030) and post-doctoral grant (No. D21/18) from “The Future Ocean” Clusters of Excellence funded within the framework of the Excellence Initiative by the Deutsche Forschungsgemeinschaft on behalf of the German Federal and State Governments and UFOTriNet project funded by the Federal Ministry of Food and Agriculture (BMEL) based on a decision of the Parliament of the Federal Republic of Germany via the Federal Office for Agriculture and Food (BLE) under the innovation support program (funding number 2819111918). LB acknowledges support from FONDECYT Iniciación No. 11190918. MG acknowledges support from Beca de Postdoctorado UCN No. 003 and FONDECYT 1181153.
The authors declare that the research was conducted in the absence of any commercial or financial relationships that could be construed as a potential conflict of interest.
All claims expressed in this article are solely those of the authors and do not necessarily represent those of their affiliated organizations, or those of the publisher, the editors and the reviewers. Any product that may be evaluated in this article, or claim that may be made by its manufacturer, is not guaranteed or endorsed by the publisher.
We thank Abril Sanchez and Benjamin Aguila for their support in the 2018 samplings in Rapa Nui. We are especially grateful to Friederike Wölke from DAAD RISE Research Internship Program and Sami Jai Wagner Beaulieu from Université Laval for their help in the analysis of zooplankton samples. We thank Katrin Knickmeier and the Kieler Forschungswerkstatt to have lent us their infrared spectrometer for the chemical identification of the microplastics and Grace Walls to have conducted the analysis. Three reviewers provided many constructive suggestions to the initial version of this manuscript.
The Supplementary Material for this article can be found online at: https://www.frontiersin.org/articles/10.3389/fmars.2021.688224/full#supplementary-material
Acha, E. M., Piola, A., Iribarne, O., and Mianzan, H. (2015). Ecological processes at marine fronts: oases in the ocean. Spring. Briefs Environ. Sci. 73, 14–19.
Alden, R. W., Dahiya, R. C., and Young, R. J. (1982). A method for the enumeration of zooplankton subsamples. J. Exp. Mar. Biol. Ecol. 59, 185–206. doi: 10.1016/0022-0981(82)90115-0
Alldredge, A. L. (1982). Aggregation of spawning appendicularians in surface windrows. Bull. Mar. Sci. 32, 250–254.
Al-Yamani, F. Y., Skryabin, V., Gubanova, A., Khvorov, S., and Prusova, I. (2011). Marine Zooplankton Practical Guide, 2nd Edn. Kuwait: Kuwait Institute for Scientific Research.
Andrade, I., Hormazábal, S., and Correa-Ramírez, M. (2014a). Time-space variability of satellite chlorophyll-a in the Easter Island Province, southeastern Pacific Ocean. Lat. Am. J. Aquat. Res. 42, 871–887. doi: 10.3856/vol42-issue4-fulltext-13
Andrade, I., Sangrà, P., Hormazabal, S., and Correa-Ramirez, M. (2014b). Island mass effect in the Juan Fernández archipelago (33°S), southeastern Pacific. Deep Sea Res. I. 84, 86–99. doi: 10.1016/j.dsr.2013.10.009
Argandoña, W., and Moraga, J. (2000). Dinámica de las aguas costeras de isla de Pascua, una aproximación. En Result. Crucero Cimar 5, 50–55.
Bakun, A. (2006). Fronts and eddies as key structures in the habitat of marine fish larvae: opportunity, adaptive response and competitive advantage. Sci. Mar. 70, 105–122. doi: 10.3989/scimar.2006.70s2105
Belkin, I. M., and Cornillon, P. C. (2003). SST fronts of the Pacific coastal and marginal seas. Pac. Oceanogr. 1, 90–113.
Bourne, W. R. P., and Clark, G. C. (1984). The occurrence of birds and garbage at the Humboldt front of Valparaiso, Chile. Mar. Pollut. Bull. 17, 343–344. doi: 10.1016/0025-326x(84)90493-4
Brach, L., Deixonne, P., Bernard, M. F., Durand, E., Desjean, M. C., Perez, E., et al. (2018). Anticyclonic eddies increase accumulation of microplastic in the North Atlantic subtropical gyre. Mar. Pollut. Bull. 126, 191–196. doi: 10.1016/j.marpolbul.2017.10.077
Burford, M. A., Rothlisberg, P. C., and Wang, Y. G. (1995). Spatial and temporal distribution of tropical phytoplankton species and biomass in the Gulf of Carpentaria, Australia. Mar. Ecol. Prog. Ser. 118, 255–266. doi: 10.3354/meps118255
Caldeira, R. M., Marchesiello, P., Nezlin, N. P., DiGiacomo, P. M., and McWilliams, J. C. (2005). Island wakes in the southern California Bight. J. Geophys. Res. Oceans 110:C11012.
Carlson, D. J. (1982). Surface microlayer phenolic enrichments indicate sea surface slicks. Nature 296, 426–429. doi: 10.1038/296426a0
Caruso, M. J., Migliaccio, M., Hargrove, J. T., Garcia-Pineda, O., and Graber, H. C. (2013). Oil spills and slicks imaged by synthetic aperture radar. Oceanography 26, 112–123.
Chagnon, C., Thiel, M., Antunes, J., Ferreira, J. L., Sobral, P., and Ory, N. C. (2018). Plastic ingestion and trophic transfer between Easter Island flying fish (Cheilopogon rapanouiensis) and yellowfin tuna (Thunnus albacares) from Rapa Nui (Easter Island). Environm. Pollut. 243, 127–133. doi: 10.1016/j.envpol.2018.08.042
Chubarenko, I., Chubarenko, B., Esiukova, E., and Baudler, H. (2010). Mixing by Langmuir circulation in shallow lagoons. Baltica 23, 13–24.
Cornejo, D., Ottone, M., Bravo, L., Ramos, M., Pizarro, O., Karstensen, J., et al. (2016). Biogeochemical characteristics of a long-lived anticyclonic eddy in the eastern South Pacific Ocean. Biogeosciences 13, 2971–2979. doi: 10.5194/bg-13-2971-2016
Cózar, A., Aliani, S., Basurko, O. C., Arias, M., Isobe, A., Topouzelis, K., et al. (2021). Marine litter windrows: a strategic target to understand and manage the ocean plastic pollution. Front. Mar. Sci. 8:571796. doi: 10.3389/fmars.2021.571796
Cózar, A., Echevarría, F., González-Gordillo, J. I., Irigoien, X., Úbeda, B., Hernández-León, S., et al. (2014). Plastic debris in the open ocean. Proc. Natl. Acad. Sci. U.S.A. 111, 10239–10244.
Dell’Ariccia, G., Célérier, A., Gabirot, M., Palmas, P., Massa, B., and Bonadonna, F. (2014). Olfactory foraging in temperate waters: sensitivity to dimethylsulphide of shearwaters in the Atlantic Ocean and Mediterranean Sea. J. Exp. Biol. 217, 1701–1709.
Denman, K. L., and Gargett, A. E. (1983). Time and space scales of vertical mixing and advection of phytoplankton in the upper ocean. Limnol. Oceanogr. 28, 801–815. doi: 10.4319/lo.1983.28.5.0801
Eriksen, M., Lebreton, L. C. M., Carson, H. S., Thiel, M., Moore, C. J., Borerro, J. C., et al. (2014). Plastic pollution in the world’s oceans: more than 5 trillion plastic pieces weighing over 250 000 tons afloat at sea. PLoS One 9:e111913. doi: 10.1371/journal.pone.0111913
Eriksen, M., Liboiron, M., Kiessling, T., Charron, L., Alling, A., Lebreton, L., et al. (2018). Microplastic sampling with the AVANI trawl compared to two neuston trawls in the Bay of Bengal and South Pacific. Environ. Pollut. 232, 430–439. doi: 10.1016/j.envpol.2017.09.058
Eriksen, M., Maximenko, N., Thiel, M., Cummins, A., Lattin, G., Wilson, S., et al. (2013). Plastic pollution in the south Pacific subtropical gyre. Mar. Pollut. Bull. 68, 71–76. doi: 10.1016/j.marpolbul.2012.12.021
Ermakov, S. A., Salashin, S. G., and Panchenko, A. R. (1992). Film slicks on the sea surface and some mechanisms of their formation. Dynam. Atmos. Oceans 16, 279–304. doi: 10.1016/0377-0265(92)90010-q
Ewing, G. C. (1950). Relation between band slicks and internal waves in the sea. Science 111, 91–94. doi: 10.1126/science.111.2874.91-a
Fedorov, K. N. (1986). The Physical Nature and Structure of Oceanic Fronts. Berlin: Springer-Verlag, 1–333.
Flagg, C. N., Wirick, C. D., and Smith, S. L. (1994). The interaction of phytoplankton, zooplankton and currents from 15 months of continuous data in the mid-Atlantic bight. Deep Sea. Res. II Top. Stud. Oceanogr. 41, 411–435. doi: 10.1016/0967-0645(94)90030-2
Foekema, E. M., De Gruijter, C., Mergia, M. T., Van Franeker, J. A., Murk, A. J., and Koelmans, A. A. (2013). Plastic in North Sea fish. ıEnviron. Sci. Technol. 47, 8818–8824. doi: 10.1021/es400931b
Fosberg, F. R. (1957). Slicks on ocean surface downwind from coral reefs. Atoll Res. Bull. 53, 1–4. doi: 10.5479/si.00775630.53.1
Fossi, M. C., Romeo, T., Baini, M., Panti, C., Marsili, L., Campani, T., et al. (2017). Plastic debris occurrence, convergence areas and fin whales feeding ground in the Mediterranean marine protected area pelagos sanctuary: a modeling approach. Front. Mar. Sci. 4:167. doi: 10.3389/fmars.2017.00167
Frère, L., Paul-Pont, I., Rinnert, E., Petton, S., and Jaffré, J. (2017). Influence of environmental and anthropogenic factors on the composition, concentration and spatial distribution of microplastics: a case study of the Bay of Brest (Brittany, France). Environ. Pollut. 25, 211–222. doi: 10.1016/j.envpol.2017.03.023
Gade, M., Byfield, V., Ermakov, S., Lavrova, O., and Mitnik, L. (2013). Slicks as indicator for marine processes. Oceanography 26, 138–149.
Genin, A. (2004). Bio-physical coupling in the formation of zooplankton and fish aggregations over abrupt topographies. J. Mar. Syst. 50, 3–20. doi: 10.1016/j.jmarsys.2003.10.008
Gibson, R. N., Atkinson, R. J., and Gordon, J. D. (2009). Zooplankton diel vertical migration—a review of proximate control. Oceanogr. Mar. Biol. Annu. Rev. 47, 77–110. doi: 10.1201/9781420094220.ch2
Godø, O. R., Samuelsen, A., Macaulay, G. J., Patel, R., Hjøllo, S. S., Horne, J., et al. (2012). Mesoscale eddies are oases for higher trophic marine life. PLoS One 7:30161. doi: 10.1371/journal.pone.0030161
Gove, J. M., Whitney, J. L., McManus, M. A., Lecky, J., Carvalho, F. C., Lynch, J. M., et al. (2019). Prey-size plastics are invading larval fish nurseries. Proc. Natl. Acad. Sci. U.S.A. 11648, 24143–24149. doi: 10.1073/pnas.1907496116
Hamner, W. M., and Schneider, D. (1986). Regularly spaced rows of medusae in the bering sea: role of Langmuir circulation. Limnol. Oceanogr. 31, 171–177. doi: 10.4319/lo.1986.31.1.0171
Hempel, G., and Weikert, H. (1972). The neuston of the subtropical and boreal North-eastern Atlantic Ocean. A review. Mar. Biol. 13, 70–88.
Hernández-León, S. (1991). Accumulation of mesozooplankton in a wake area as a causative mechanism of the “island-mass effect”. Mar. Biol. 109, 141–147. doi: 10.1007/bf01320241
Hidalgo-Ruz, V., Luna-Jorquera, G., Eriksen, M., Frick, H., Miranda-Urbina, D., Portflitt-Toro, M., et al. (2021). Factors (type, colour, density, and shape) determining the removal of marine plastic debris by seabirds from the South Pacific Ocean: is there a pattern? Aquat. Conserv. Mar. Freshw. Ecosyst. 31, 389–407. doi: 10.1002/aqc.3453
Highfield, J., Eloire, D., Conway, D. V., Lindeque, P. K., Attrill, M. J., and Somerfield, P. J. (2010). Seasonal dynamics in meroplankton assemblages at station L4. J. Plankton. Res. 32, 681–691. doi: 10.1093/plankt/fbp139
Holloway, P. E., Pelinovsky, E., Talipova, T., and Barnes, B. (1997). A nonlinear model of internal tide transformation on the Australian North West Shelf. J. Phys. Oceanogr. 27, 871–896. doi: 10.1175/1520-0485(1997)027<0871:anmoit>2.0.co;2
Howell, E. A., Bograd, S. J., Morishige, C., Seki, M. P., and Polovina, J. J. (2012). On North Pacific circulation and associated marine debris concentration. Mar. Pollut. Bull. 65, 16–22. doi: 10.1016/j.marpolbul.2011.04.034
Jamodiong, E. A., Maboloc, E. A., Leriorato, J. C., Tanedo, Mcs, Diaz, L., Tabalanza, T. D., et al. (2018). Coral spawning and spawn-slick observation in the Philippines. Mar. Biodiv. 48, 2187–2192. doi: 10.1007/s12526-017-0680-9
Jeans, D. R. G., and Sherwin, T. J. (2001). The variability of strongly non-linear solitary internal waves observed during an upwelling season on the Portuguese shelf. Cont. Shelf Res. 21, 1855–1878. doi: 10.1016/s0278-4343(01)00026-7
Jones, E. C. (1962). Evidence of an island effect upon the standing crop of zooplankton near the Marquesas Islands, Central Pacific. ICES J. Mar. Sci. 27, 223–231. doi: 10.1093/icesjms/27.3.223
Kingsford, M. J. (1990). Linear oceanographic features: a focus for research on recruitment processes. Austral Ecol. 15, 391–401. doi: 10.1111/j.1442-9993.1990.tb01465.x
Kingsford, M. J., and Choat, J. H. (1985). The fauna associated with drift algae captured with a plankton-mesh purse seine net. Limnol. Oceanogr. 30, 618–630. doi: 10.4319/lo.1985.30.3.0618
Kingsford, M. J., and Choat, J. H. (1986). Influence of surface slicks on the distribution and onshore movements of small fish. Mar. Biol. 91, 161–171. doi: 10.1007/bf00569432
Kingsford, M. J., Leis, A., Shanks, K., Lindeman, S., Morgan, S., and Pineda, J. (2002). Sensory environments, larval abilities and local self-recruitment. Bull. Mar. Sci. 70, 309–340.
Kühn, S., van Franeker, J. A., O’Donoghue, A. M., Swiers, A., Starkenburg, M., van Werven, B., et al. (2020). Details of plastic ingestion and fibre contamination in North Sea fishes. Environ. Pollut. 257:113569. doi: 10.1016/j.envpol.2019.113569
Kukulka, T., Proskurowski, G., Morét-Ferguson, S., Meyer, D. W., and Law, K. L. (2012). The effect of wind mixing on the vertical distribution of buoyant plastic debris. Geophys. Res. Lett. 39, 1–6.
Lampert, W. (1989). The adaptive significance of diel vertical migration of zooplankton. Funct. Ecol. 3, 21–27. doi: 10.2307/2389671
Law, K. L., Morét-Ferguson, S. E., Goodwin, D. S., Zettler, E. R., DeForce, E., Kukulka, T., et al. (2014). Supporting information for: distribution of surface plastic debris in the eastern Pacific Ocean from an 11-year dataset. Environ. Sci. Technol. 48, 1–10.
Leibovich, S. (1983). The form and dynamics of Langmuir circulations. Annu. Rev. Fluid. Mech. 15, 391–427. doi: 10.1146/annurev.fl.15.010183.002135
Locke, A. (1992). Factors influencing community structure along stress gradients: zooplankton responses to acidification. Ecology 73, 903–990. doi: 10.2307/1940167
Luna-Jorquera, G., Thiel, M., Portflitt-Toro, M., and Dewitte, B. (2019). Marine protected areas invaded by floating anthropogenic litter: an example from the South Pacific. Aquat. Conserv. Mar. Freshw. Ecosyst. 29, 245–259. doi: 10.1002/aqc.3095
Lusher, A., McHugh, M., and Thompson, R. (2013). Occurrence of microplastics in the gastrointestinal tract of pelagic and demersal fish from the English Channel. Mar. Pollut. Bull. 67, 94–99. doi: 10.1016/j.marpolbul.2012.11.028
Mackas, D. L., Greve, W., Edwards, M., Chiba, S., Tadokoro, K., Eloire, D., et al. (2012). Changing zooplankton seasonality in a changing ocean: comparing time series of zooplankton phenology. Prog. Oceanogr. 97, 31–62. doi: 10.1016/j.pocean.2011.11.005
Mackenzie, B. (2000). Turbulence, larval fish ecology and fisheries recruitment: a review of field studies. Oceanol. Acta 23, 357–375. doi: 10.1016/s0399-1784(00)00142-0
Mann, K., and Lazier, J. (1996). “Dynamics of marine ecosystems,” in Biological-Physical Interactions in the Oceans, Second Edn, ed. K. H. Mann (Hoboken, NJ: Blackwell Science Publications).
Marmorino, G. F., Askari, F., and Mied, R. (2002). Observations of the creation and evolution of small-scale oceanic frontal cusps and slicks. J. Mar. Syst. 37, 17–29. doi: 10.1016/s0924-7963(02)00193-8
Martínez, E., Maamaatuaiahutapu, K., and Taillandier, V. (2009). Floating marine debris surface drift: convergence and accumulation toward the South Pacific subtropical gyre. Mar. Pollut. Bull. 58, 1347–1355. doi: 10.1016/j.marpolbul.2009.04.022
Mattos, H., and Mujica, A. (2012). Composición de zooplancton superficial en zonas lisas y rugosas en la bahía de Coquimbo (noviembre 2001). Lat. Am. J. Aquat. Res. 40, 453–461. doi: 10.3856/vol40-issue2-fulltext-21
Maximenko, N., Hafner, J., and Niiler, P. (2012). Pathways of marine debris from trajectories of Lagrangian drifters. Mar. Pollut. Bull. 65, 51–62. doi: 10.1016/j.marpolbul.2011.04.016
McClain, C. R., Signorini, S. R., and Christian, J. R. (2004). Subtropical gyre variability observed by ocean-color satellites. Deep Sea Res. II 51, 281–301. doi: 10.1016/j.dsr2.2003.08.002
McEdward, L. (ed.) (1995). Ecology of Marine Invertebrate Larvae (1st ed.). Boca Raton, FL: CRC Press.
McEwen, G. F., Johnson, M. W., and Folsom, T. R. (1954). A statistical analysis of the performance of the Folsom plankton sample splitter, based upon test observations. Arch. Met. Geoph. Biokl. A. 7, 502–527. doi: 10.1007/bf02277939
Meerhoff, E., Ramos, M., Yannicelli, B., Bravo, L., Zambra, D., and Varela, C. (2017). Meroplankton distribution in South Pacific islands, implications for larval connectivity. J. Plankton Res. 40, 77–90. doi: 10.1093/plankt/fbx053
Miller, J. M. (1974). “Nearshore distribution of Hawaiian marine fish larvae: effects of water quality, turbidity and currents,” in The Early Life History of Fish, ed. E. Kamler (Berlin: Springer), 217–231. doi: 10.1007/978-3-642-65852-5_18
Miranda-Urbina, D., Thiel, M., and Luna-Jorquera, G. (2015). Litter and seabirds found across a longitudinal gradient in the South Pacific Ocean. Mar. Pollut. Bull. 96, 235–244. doi: 10.1016/j.marpolbul.2015.05.021
Moraga, J., Lagos, P., and Argandoña, W. (2010). Dinámica de las aguas costeras de Isla de Pascua. Cienc. Tecnol. Mar. 33, 5–16.
Moraga, J., Valle-Levinson, A., and Olivares, J. (1999). Hydrography and geostrophy around Easter Island. Deep Sea Res. PT I 46, 715–731. doi: 10.1016/s0967-0637(98)00083-1
Morel, A., Claustre, H., and Gentili, B. (2010). The most oligotrophic subtropical zones of the global ocean: similarities and differences in terms of chlorophyll and yellow substance. Biogeosciences 7, 3139–3151. doi: 10.5194/bg-7-3139-2010
Morgan, S. G., and Fisher, J. L. (2010). Larval behavior regulates nearshore retention and offshore migration in an upwelling shadow and along the open coast. Mar. Ecol. Prog. Ser. 404, 109–126. doi: 10.3354/meps08476
Moum, J. N., Carlson, D. J., and Cowles, T. J. (1990). Sea slicks and surface strain. Deep Sea Res. I 37, 767–775. doi: 10.1016/0198-0149(90)90005-g
Mulhearn, P. J. (1987). The Tasman Front: a study using satellite infrared imagery. J. Fhys. Oceanogr. 17, 1148–1155. doi: 10.1175/1520-0485(1987)017<1148:ttfasu>2.0.co;2
Olivar, M. P., González-Gordillo, J. I., Salat, J., Chust, G., Cózar, A., Hernández-León, S., et al. (2016). The contribution of migratory mesopelagic fishes to neuston fish assemblages across the Atlantic, Indian and Pacific Oceans. Mar. Freshw. Res. 67, 1114–1127. doi: 10.1071/mf14391
Olson, D. B., and Backus, R. H. (1985). The concentrating of organisms at fronts: a cold-water fish and a warm core Gulf Stream ring. Mar. Res. 43, 13–37.
Ory, N. C., Gallardo, C., Lenz, M., and Thiel, M. (2018). Capture, swallowing, and egestion of microplastics by a planktivorous juvenile fish. Environ. Pollut. 240, 566–573. doi: 10.1016/j.envpol.2018.04.093
Ory, N. C., Sobral, P., Ferreira, J. L., and Thiel, M. (2017). Amberstripe scad Decapterus muroadsi (Carangidae) fish ingest blue microplastics resembling their copepod prey along the coast of Rapa Nui (Easter Island) in the South Pacific subtropical gyre. Sci. Total Environ. 586, 430–437. doi: 10.1016/j.scitotenv.2017.01.175
Owen, R. (1981). “Fronts and eddies in the sea: mechanisms, interactions, and biological effects,” in Analysis and Marine Ecosystems, ed. A. R. Longhurst (London: Academic Press), 197–233.
Pattiaratchi, C. (1994). “Physical oceanographic aspects of the dispersal of coral spawn slicks: a review,” in The Bio-Physics of Marine Larval Dispersal, eds P. W. Sammarco and M. L. Heron (Washington, D.C: American Geophysical Union), 89–105. doi: 10.1029/ce045p0089
Peterson, B., and Carl, P. (2019). R package “Performance Analytics”: Econometric Tools for Performance and Risk Analysis. R package version 1.5.3.
Pichel, W. G., Churnside, J. H., Veenstra, T. S., Foley, D. G., Friedman, K. S., and Clemente-Colon, P. (2007). Marine debris collects within the North Pacific subtropical convergence zone. Mar. Pollut. Bull. 54, 1207–1211. doi: 10.1016/j.marpolbul.2007.04.010
Pineda, J. (1999). Circulation and larval distribution in internal tidal bore warm fronts. Limnol. Oceanogr. 44, 1400–1414. doi: 10.4319/lo.1999.44.6.1400
Pingree, R. D., Bowman, M. J., and Esaias, W. E. (1978). “Headland fronts,” in Oceanic Fronts in Coastal Processes, eds M. J. Bowman and W. E. Esaias (Berlin: Springer-Verlag), 78–86. doi: 10.1007/978-3-642-66987-3_9
Pingree, R. D., Forster, G., and Morrison, G. K. (1974). Turbulent convergent tidal fronts. J. Mar. Biol. Assoc. U.K. 54, 469–479. doi: 10.1017/s0025315400058653
Pizarro, G., Montecino, V., Astoreca, R., Alarcón, G., Yuras, G., and Guzmán, L. (2006). Variabilidad espacial de condiciones bio-ópticas de la columna de agua entre las costas de Chile insular y continental, primavera 1999 y 2000. Cienc. Tecnol. Mar. 29, 45–58.
Primpke, S., Christiansen, S. H., Cowger, W., De Frond, H., Deshpande, A., Fischer, M., et al. (2020). Critical assessment of analytical methods for the harmonized and cost-efficient analysis of microplastics. Appl. Spectrosc. 74, 1012–1047. doi: 10.1177/0003702820921465
Primpke, S., Wirth, M., Lorenz, C., and Gerdts, G. (2018). Reference database design for the automated analysis of microplastic samples based on Fourier transform infrared (FTIR) spectroscopy. Anal. Bioanal. Chem. 410, 5131–5141. doi: 10.1007/s00216-018-1156-x
Reisser, J., Slat, B., Noble, K., du Plessis, K., Epp, M., Proietti, M., et al. (2015). The vertical distribution of buoyant plastics at sea: an observational study in the North Atlantic Gyre. Biogeosciences 12, 1249–1256. doi: 10.5194/bg-12-1249-2015
Romano, J. C. (1996). Sea-surface slick occurrence in the open sea (Mediterranean, Red Sea, Indian Ocean) in relation to wind speed. Deep Sea Res. I 43, 411–423. doi: 10.1016/0967-0637(96)00024-6
Rothschild, B. J., and Osborn, T. R. (1988). Small-scale turbulence and plankton contact rates. J. Plankton Res. 10, 465–474. doi: 10.1093/plankt/10.3.465
Ryan, J. P., Fischer, A. M., Kudela, R. M., McManus, M. A., Myers, J. S., Paduan, J. D., et al. (2010). Recurrent frontal slicks of a coastal ocean upwelling shadow. J. Geophys. Res. Oceans 115, 1–15.
Sazima, C., and Sazima, I. (2001). Plankton-feeding aggregation and occasional cleaning by adult butterflyfish, Chaetodon striatus (Chaetodontidae), in southwestern Atlantic. Cybium 25, 145–151.
Schaefer, K. M. (2001). “Reproductive biology of tunas,” in Fish Physiology, eds B. Barbara and E. Stevens (Cambridge, MA: Academic Press), 225–270. doi: 10.1016/s1546-5098(01)19007-2
Shanks, A. (1983). Surface slicks associated with tidally forced internal waves may transport pelagic larvae of benthic invertebrates and fishes shoreward. Mar. Ecol. Prog. Ser. 13, 311–315. doi: 10.3354/meps013311
Shanks, A. (1986). Vertical migration and eross shelf dispersal of larval Cancer spp. and Ranclallia ornala (Crustacea: Brachyura) off the coast of southern California. Mar. Biol. 92, 189–199. doi: 10.1007/bf00392836
Shanks, A., and Wright, W. G. (1987). Internal wave mediated shoreward transport of cyprids, megalopae, and gammarids and correlated longshore differences in the settling rate of intertidal barnacles. J. Exp. Mar. Biol. Ecol. 114, 1–13. doi: 10.1016/0022-0981(87)90135-3
Shanks, A. L. (1995). “Mechanisms of cross-shelf dispersal of larval invertebrates and fish,” in Ecology of Marine Invertebrate Larvae, ed. L. McEdward (Boca Raton, FL: CRC Press), 323–367. doi: 10.1201/9780138758950-10
Shanks, A. L. (2021). Observational evidence and open questions on the role of internal tidal waves on the concentration and transport of floating plastic debris. Front. Mar. Sci. 8:621062. doi: 10.3389/fmars.2021.621062
Shanks, A. L., Largier, J., Brink, L., Brubaker, J., and Hooff, R. (2000). Demonstration of the onshore transport of larval invertebrates by the shoreward movement of an upwelling front. Limnol. Oceanogr. 45, 230–236. doi: 10.4319/lo.2000.45.1.0230
Son, Y. B., Choi, B. J., Kim, Y. H., and Park, Y. G. (2015). Tracing floating green algae blooms in the yellow Sea and the East China sea using GOCI satellite data and Lagrangian transport simulations. Remote. Sens. Environ. 156, 21–33. doi: 10.1016/j.rse.2014.09.024
Starr, M., Himmelman, J. H., and Therriault, J. C. (1990). Direct coupling of marine invertebrate spawning with phytoplankton blooms. Science 247, 1071–1074. doi: 10.1126/science.247.4946.1071
Sundby, S., and Fossum, P. (1990). Feeding conditions of Arcto-norwegian cod larvae compared with the Rothschild-Osborn theory on small-scale turbulence and plankton contact rates. J. Plankton. Res. 12, 1153–1162. doi: 10.1093/plankt/12.6.1153
Taiyun, W., and Viliam, S. (2017). R Package “corrplot”: Visualization of a Correlation Matrix. R Package Version 0.84.
Thiel, M., Luna-Jorquera, G., Álvarez-Varas, R., Gallardo, C., Hinojosa, I. A., Luna, N., et al. (2018). Impacts of marine plastic pollution from continental coasts to subtropical gyres - fish, seabirds, and other vertebrates in the SE Pacific. Front. Mar. Sci. 5:238. doi: 10.3389/fmars.2018.00238
Thomas, L. N., Tandon, A., and Mahadevan, A. (2008). Submesoscale processes and dynamics. J. Geophys. Res. 177, 17–38. doi: 10.1029/177gm04
Thompson, R. C., Olsen, Y., Mitchell, R. P., Davis, A., Rowland, S. J., John, A. W. G., et al. (2004). Lost at sea, where is all the plastic? Science 304, 838–838. doi: 10.1126/science.1094559
Thomson, J. (1862). On the calm lines often seen on a rippled sea. Philos. Mag. J. Sci. 24, 247–248. doi: 10.1080/14786446208643350
van Gennip, S. J., Dewitte, B., Garçon, V., Thiel, M., Popova, E., Drillet, Y., et al. (2019). In search for the sources of plastic marine litter that contaminates the Easter Island Ecoregion. Sci. Rep. 9, 1–13.
van Sebille, E., Aliani, S., Law, K. L., Maximenko, N., Alsina, J., Bagaev, A., et al. (2020). The physical oceanography of the transport of floating marine debris. Environ. Res. Lett. 15:23003.
Vega, R., Licandeo, R., Rosson, G., and Yáñez, E. (2009). Species catch composition, length structure and reproductive indices of swordfish (Xiphias gladius) at Easter Island zone. Lat. Am. J. Aquat. Res. 37, 83–95. doi: 10.3856/vol37-issue1-fulltext-7
von Dassow, P., and Collado-Fabbri, S. (2014). Biological oceanography, biogeochemical cycles, and pelagic ecosystem functioning of the east-central South Pacific Gyre: focus on Easter Island and Salas-y-Gómez Island. Lat. Am. J. Aquat. Res. 42, 703–742. doi: 10.3856/vol42-issue4-fulltext-4
Walter, R. K., Woodson, C. B., Leary, P. R., and Monismith, S. G. (2014). Connecting wind-driven upwelling and offshore stratification to nearshore internal bores and oxygen variability. J. Geophys. Res. Oceans. 119, 3517–3534. doi: 10.1002/2014jc009998
Weidberg, N., Lobón, C., López, E., García Flórez, L., Fernández Rueda Md, P., Largier, J., et al. (2014). Effect of nearshore surface slicks on meroplankton distribution: role of larval behaviour. Mar. Ecol. Prog. Ser. 506, 15–30. doi: 10.3354/meps10777
Whitney, J. L., Gove, J. M., McManus, M. A., Smith, K. A., Lecky, J., Neubauer, P., et al. (2021). Surface slicks are pelagic nurseries for diverse ocean fauna. Sci. Rep. 11, 1–18.
Wiafe, G., and Frid, C. L. (1996). Short-term temporal variation in coastal zooplankton communities: the relative importance of physical and biological mechanisms. J. Plankton. Res. 18, 1485–1501. doi: 10.1093/plankt/18.8.1485
Wickham, H. (2016). R package “ggplot2”: Elegant Graphics for Data Analysis. Available online at: https://ggplot2.tidyverse.org (accessed October 2, 2021).
Williams, R. (2011). Ocean eddies and plankton blooms. Nat. Geosci. 4, 739–740. doi: 10.1038/ngeo1307
Wolanski, E., and Hamner, W. M. (1988). Topographically controlled fronts in the ocean and their biological influence. Science 241, 177–181. doi: 10.1126/science.241.4862.177
Woodson, C. B. (2018). The fate and impact of internal waves in nearshore ecosystems. Annu. Rev. Mar. Sci. 10, 421–441. doi: 10.1146/annurev-marine-121916-063619
Woodson, C. B., McManus, M. A., Tyburczy, J. A., Barth, J. A., Washburn, L., Caselle, J. E., et al. (2012). Coastal fronts set recruitment and connectivity patterns across multiple taxa. Limnol. Oceanogr. 57, 582–596. doi: 10.4319/lo.2012.57.2.0582
Young, M., and Adams, N. J. (2010). Plastic debris and seabird presence in the Hauraki Gulf, New Zealand. N. Z. J. Mar. Freshw. Res. 44, 167–175. doi: 10.1080/00288330.2010.498089
Zaitsev, Y. P. (1970). Marine Neustonology. Jerusalem: Israel Program for the Scientific translations.
Zeitzschel, B. (1978). Oceanographic factors influencing the distribution of plankton in space and time. Micropaleontology 24, 139–159. doi: 10.2307/1485247
Keywords: plastics, zooplankton, neuston, particle retention, convergence zones, surface slicks
Citation: Gallardo C, Ory NC, Gallardo MÁ, Ramos M, Bravo L and Thiel M (2021) Sea-Surface Slicks and Their Effect on the Concentration of Plastics and Zooplankton in the Coastal Waters of Rapa Nui (Easter Island). Front. Mar. Sci. 8:688224. doi: 10.3389/fmars.2021.688224
Received: 30 March 2021; Accepted: 21 September 2021;
Published: 29 October 2021.
Edited by:
Andrés Cózar, University of Cadiz, SpainReviewed by:
Giuseppe Suaria, National Research Council, Consiglio Nazionale delle Ricerche (CNR), ItalyCopyright © 2021 Gallardo, Ory, Gallardo, Ramos, Bravo and Thiel. This is an open-access article distributed under the terms of the Creative Commons Attribution License (CC BY). The use, distribution or reproduction in other forums is permitted, provided the original author(s) and the copyright owner(s) are credited and that the original publication in this journal is cited, in accordance with accepted academic practice. No use, distribution or reproduction is permitted which does not comply with these terms.
*Correspondence: Martin Thiel, dGhpZWxAdWNuLmNs
Disclaimer: All claims expressed in this article are solely those of the authors and do not necessarily represent those of their affiliated organizations, or those of the publisher, the editors and the reviewers. Any product that may be evaluated in this article or claim that may be made by its manufacturer is not guaranteed or endorsed by the publisher.
Research integrity at Frontiers
Learn more about the work of our research integrity team to safeguard the quality of each article we publish.