- 1National Museum of Marine Biology and Aquarium, Checheng, Taiwan
- 2Graduate Institute of Marine Biology, National Dong Hwa University, Checheng, Taiwan
- 3National Oceanic and Atmospheric Administration, Miami, FL, United States
- 4Cooperative Institute for Marine and Atmospheric Studies, University of Miami, Miami, FL, United States
The effects of seagrass on microalgal assemblages under experimentally elevated temperatures (28°C) and CO2 partial pressures (pCO2; 800 μatm) were examined using coral reef mesocosms. Concentrations of nitrate, ammonium, and benthic microalgal chlorophyll a (chl-a) were significantly higher in seagrass mesocosms, whereas phytoplankton chl-a concentrations were similar between seagrass and seagrass-free control mesocosms. In the seagrass group, fewer parasitic dinoflagellate OTUs (e.g., Syndiniales) were found in the benthic microalgal community though more symbiotic dinoflagellates (e.g., Cladocopium spp.) were quantified in the phytoplankton community. Our results suggest that, under ocean acidification conditions, the presence of seagrass nearby coral reefs may (1) enhance benthic primary productivity, (2) decrease parasitic dinoflagellate abundance, and (3) possibly increase the presence of symbiotic dinoflagellates.
Introduction
Seagrass meadows are common in coastal waters (Unsworth et al., 2012, 2019b) and support diverse fish and invertebrate communities (de los Santos et al., 2019; Unsworth et al., 2019a; Liu et al., 2020). They also offer a number of ecosystem services to humans, including coastline protection, serving as habitats for commercially important food sources, and mitigation of climate change impacts. However, there is much to be learned with respect to their interactions with other species, particularly microbial communities (Unsworth et al., 2019a).
For example, Lamb et al. (2017) reported that seagrass ecosystems can sequester bacterial pathogens that are harmful to fish, invertebrates, and even humans, and Inaba et al. (2019) noted that seagrass provide habitats for bacteria that kill or limit the growth of the harmful alga Chattonella antiqua. Although seagrass and algae compete for the same nutrients and trace elements (Unsworth et al., 2012), seagrass can provide substrates for microbenthic algal settlement (Tew et al., 2012, 2017a); microalgae can sometimes overgrow their seagrass hosts (Lin et al., 2018). Algal blooms can also reduce light intensity, which consequently inhibits seagrass growth (Tiling and Proffitt, 2017). These studies highlight the complex dynamic between seagrass and other microbes (especially microalgae) and point to a better need to understand the underlying ecosystem processes.
Since increases in CO2 lower seawater pH, studies in the Philippines (Marbà et al., 2007), the Great Barrier Reef (Unsworth et al., 2012), and the Mediterranean (Invers et al., 1997) have recorded diel changes in pH between 0.5 and 0.7 in seagrass habitats. Seagrass can increase pH by up to 0.4 (Unsworth et al., 2012) by reducing CO2 through photosynthetic uptake (dependent on water residence time and water depth). Thus, seagrass could potentially buffer future ocean acidification (OA), thereby potentially modulating OA impacts on other members of the seagrass bed community, such as phytoplankton, benthic algae, or even corals in areas where seagrass and corals are located in proximity (Huan et al., 2015; Lin et al., 2018; Lee et al., 2019).
Coral reef mesocosms have previously been used to investigate the effects of simulated climate change on coral reef-associated benthic microalgal communities, and some diatom species were shown to grow faster and smaller at high temperatures (Tew et al., 2014); however, OA benefits benthic microalgal primary productivity pending sufficient nutrient levels (Tew et al., 2017b). Given the aforementioned potential for direct and indirect effects of seagrass on the seagrass meadow’s microalgal communities, we investigated the effects of OA (800 μatm) on phytoplankton and benthic microalgal communities in mesocosms with and without the presence of seagrass at 25 and 28°C. We specifically hypothesized that potentially detrimental OA effects on these microbial communities would be mitigated by the co-cultured seagrass.
Materials and Methods
Mesocosm Establishment
The six mesocosms (5.14 m2 × 1 m depth, ∼5,000 L each) have been maintained continuously since 2001 at Taiwan’s National Museum of Marine Biology and Aquarium, with a variety of reef-building corals present at all times. These flow-through systems utilize sand-filtered seawater from the adjacent (∼50 m) coastal areas and have been developed to mimic tropical coral reef ecosystems of Southern Taiwan (Liu et al., 2009, 2015, 2020; Tew et al., 2017b). The mesocosms were set up in a completely randomized design (i.e., A-1 of Cornwall and Hurd, 2016), and temperatures were maintained between 25 and 26°C to simulate field conditions in the Taiwanese spring prior to the experiment. The photosynthetically active radiation (PAR) at 0.5 m depth was maintained at 258 ± 16.7 μmol photons m–2 s–1 from 0700 to 1,700 h with a 10:14 h light:dark cycle using LED lamps (XLamp XT-E LEDs, Cree, Taiwan). Seawater was continuously pumped into the tanks at 3 L min–1. The organisms in the mesocosms, such as corals, fish, sea urchins, and sea anemones (see Liu et al., 2020 for details.), were collected from nearby coral reefs under permits issued by the Kenting National Park Headquarters (Liu et al., 2009, 2015).
Individual seagrass (Thalassia hemprichii) shoots with intact rhizomes were randomly collected from a depth of ∼1 m in submerged seagrass meadows at Nanwan Bay, Taiwan and transported to the laboratory within 2 h of collection. Seagrass were acclimated for 5 days in aerated seawater, and individuals with similar lengths and numbers of leaves and roots were selected and cleaned of visible epiphytes before experiments. Each seagrass mesocosm (n = 3) received approximately 681 ± 7 shoots m–2 during the experiments.
Experimental Design
Two separate experiments were conducted with or without (“control”) seagrass (n = 3 mesocosms/seagrass treatment) under 800 ppm pCO2 (Representative Concentration Pathway, RCP 6.0 in 2100, IPCC, 2014) (hereafter “OA”): one at 25°C and another at 28°C. We did not conduct a 2-temperature × 2-pCO2 × 2 seagrass treatment (with or without) factorial design due to the limited number of mesocosms (n = 6). Each experiment lasted for 4 weeks. After the completion of the first experiment (OA + 25°C, with or without seagrass), we raised the temperature by 1°C per day, rearranged the flora and fauna within each mesocosm, and initiated the second experiment (OA + 28°C) 3 days later.
Abiotic Parameters
During the experiment, seawater temperature and salinity were measured daily with a handheld meter (YSI Model-30, YSI, United States), with dissolved oxygen (DO) and pH measured daily by a multiparameter meter (YSI Model-556). All measurements were taken between 0900 and 1,000 h local time). A total alkalinity (TA) test kit (Thermo-Scientific’s “Orion,” United States) was used to measure TA, and the pCO2 and aragonite saturation state (ΩAr) were calculated by inputting pH, TA, salinity, and temperature into CO2SYS (Lewis et al., 1998). To monitor mesocosm nutrient levels, we collected water samples weekly and used a flow injection analyzer and spectrophotometer (Hitachi model U-5100, Japan) to estimate nitrate, nitrite, ammonia, and phosphate concentrations (Pai et al., 1990, 2001; Pai and Riley, 1994). Details of the nutrient measurement methods can be found in Tew et al. (2013).
Biotic Parameters
We used glass slides as artificial substrata for benthic microalgal attachment. They were placed upright in an acrylic cage (all slides were put in one cage in each mesocosm) to exclude grazing by snails and sea urchins (sensu Tew et al., 2017b), with one collection from each of the six mesocosms after each week of the experiment. The benthic microalgae were removed from the glass slides weekly with a razor blade, re-suspended in 100 mL of 0.22 μm-filtered seawater (FSW), sonicated for 10 min, filtered through 0.45 μm GF/F filter paper (Whatman, United Kingdom), and chlorophyll a (chl-a) was extracted as described below. Benthic microalgal chl-a was expressed per unit surface area of the slides. Phytoplankton samples were collected weekly by filtering 2 L of water through a 0.45 μm GF/F filter paper, and chl-a was extracted from the benthic and seawater sample filters after 24 h immersion in 90% acetone at 4°C in the dark. Chl-a concentrations were estimated with a spectrophotometer (Hitachi U-5100) using the equations of Parsons (2013). After 4 and 8 weeks (hereafter “Wk4” and “Wk8,” respectively), additional phytoplankton and benthic microalgal samples were collected from each mesocosm for DNA analysis. Phytoplankton samples from each treatment were pooled into a single sample at each sampling time since genomic DNA (gDNA) yield from samples derived from a single mesocosm were too low for successful amplicon sequencing (resulting in a total of four DNA extracts for the phytoplankton communities). Of the 12 benthic samples (six mesocosms at each of two times), one extraction failed (mesocosm 3 of Wk8), resulting in a final sample size of 11 (5 seagrass and 6 controls).
gDNA Extraction, 18S rRNA Tag Sequencing, and Bioinformatics
Total gDNA was extracted from the 18 samples and purified using the method of Kuo et al. (2014). Amplicon PCR, purification, and tag sequencing were performed at Welgene Biotech (Taipei, Taiwan) according to their standard protocols. The eukaryotic universal primers 528F (′5-GCGGTAATTCCAGCTCCAA-3′) and 706R (′5-AATCCRAGAATTTCACCTCT-3′) were used to amplify the V4 region of the 18S SSU rDNA, and amplicons were sequenced on an Illumina Hiseq 2500 sequencing system (United States).
The 250-bp paired-end raw reads were merged using FLASH version 1.2.7 (Magoc and Salzberg, 2011), and merged reads were trimmed. Low-quality reads (average quality score < 25) and chimeras were detected using USEARCH v6.1 (Edgar et al., 2011) and removed Clean reads were aligned against the eukaryotic SILVA database release 132 (Quast et al., 2013), and reads with >97% similarity were clustered into operational taxonomic units (OTUs) using the UCLUST algorithm (Edgar, 2010) and open-reference OTU picking strategy of QIIME (version 13.8; Caporaso et al., 2010). Representative OTU sequences were compared with the eukaryotic SILVA database, and taxonomy was assigned using BLAST. Non-algal OTUs were removed, and rarefaction curves of algal sequences for each sample were generated with custom perl scripts. To ensure an equal sampling depth for all samples, an OTU abundance table was rarefied to identical read counts equal to the lowest. All subsequent analyses were then conducted based on the rarefied OTU table. Alpha diversity indices (Good’s coverage index, Chao1 indexes for richness, and Shannon-Weaver, and Simpson diversity), beta diversity indices (Bray-Curtis, Euclidean, and Jaccard dissimilarity), and principal coordinate analysis (PCoA) of beta diversity indexes for each library were calculated/carried out in QIIME. The raw sequence data were deposited in the NCBI Short Read Archive (SRA; BioSample accessions SAMN12855862-65).
Statistical Analysis
The values are presented as mean ± standard deviation (n = 3). One-way repeated-measures (RM) ANOVA was used to analyze treatment effects on the biotic and abiotic parameters by considering sampling date (co-varying with temperature) as a repeated factor. Where significant differences occurred, Tukey’s post hoc tests were used to test for individual mean differences (p < 0.05). One-way RM ANOVA, rather than two-way (temperature × pCO2), was used because we did not intend to compare the results between different temperatures (25 vs. 28oC) since they were compared neither simultaneously nor independently. Data were natural log-transformed when necessary to meet the assumptions of normality and homogeneity of variance. SigmaPlot 12.5 was used for all univariate analyses. For the benthic microalgal OTU data, the assemblages were compared between seagrass and control groups at Wk 4 and Wk 8, respectively, with a non-metric multidimensional scaling ordination analysis (MDS, Kruskal and Wish, 1978; Field et al., 1982). Biotic data similarity matrices were constructed using the Bray-Curtis similarity measure on non-standardized, arcsine-transformed percentage data. Analysis of similarity (ANOSIM) was conducted with PRIMER 6 to determine whether the microbenthic algal assemblages separated by MDS ordination differed significantly (Clarke and Warwick, 1994).
Results
Seawater Quality
At both OA + 25°C and OA + 28°C, the mean seawater temperature, salinity, pH, and DO were similar between seagrass and seagrass-free control mesocosms (Figure 1), although some parameters differed significantly (Table 1). DO was lower in seagrass mesocosms when the temperature was raised from 25 to 28°C (Figure 1). TA was 2,073 ± 80 and 2,087 ± 42 μmol kg–1 for the seagrass treatment and 2,047 ± 64 and 2,124 ± 69 μmol kg–1 in the controls at 25 and 28°C, respectively (Figure 2). pCO2 was consistently maintained around 800 μatm (Table 1). ΩAr values dropped to around 1.6–1.7 when CO2 increased (Figure 2), and no significant difference was detected between seagrass and control treatments (Table 1). The HCO3-, CO2, and CO32– concentrations were similar between treatments (Figure 2) at both temperatures (Table 1).
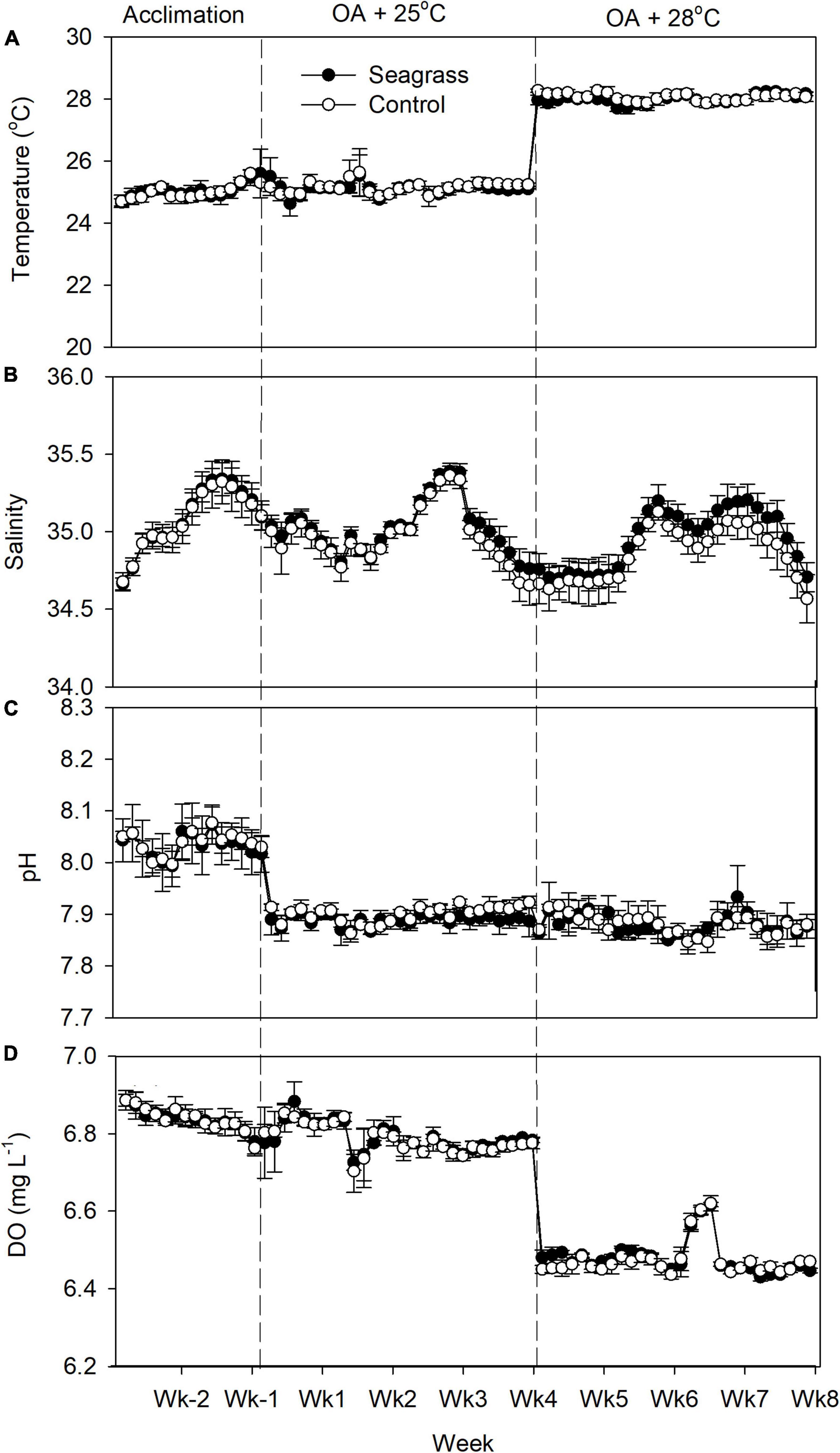
Figure 1. (A) Temperature, (B) salinity, (C) pH, and (D) dissolved oxygen (DO) concentration in the experimental mesocosms. Error bars represent standard deviation (n = 3).
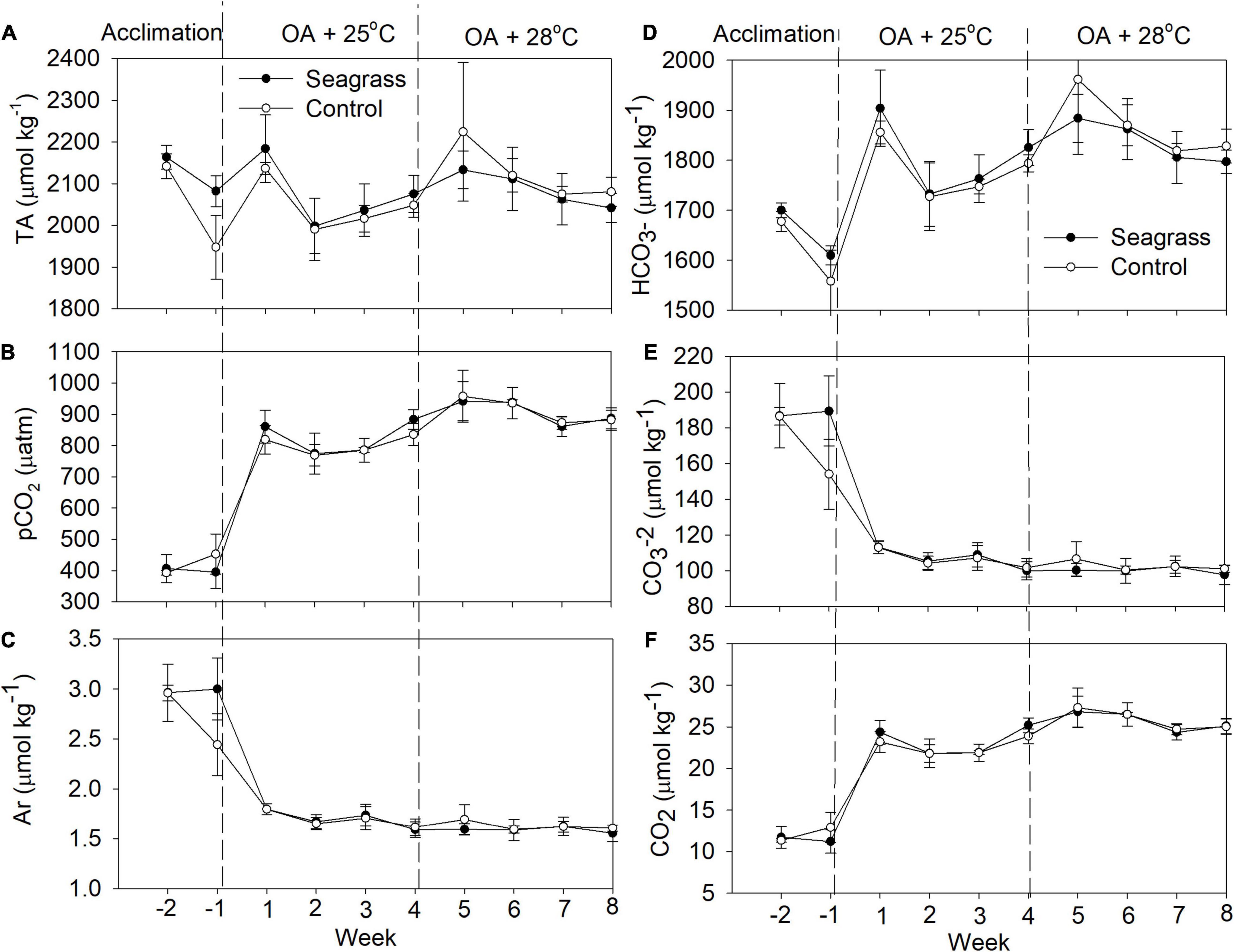
Figure 2. (A) Total alkalinity (TA), (B) pCO2, (C) ΩAr, and (D–F) other carbonate system parameters in the experimental mesocosms. Error bars represent standard deviation (n = 3).
The seawater nutrient concentrations during the acclimation period were low, with average concentrations of NO3–, NO2–, NH3–, and PO43– of 0.028, 0.004, 0.002, and 0.010 mg L–1, respectively (Figure 3). At 25°C, when CO2 was bubbled into the six mesocosms, all nutrient concentrations except NH3– were significantly higher in the seagrass group (Figure 3 and Table 1). When the temperature was raised to 28oC, only NO3– and NH3– concentrations were significantly higher in the seagrass group (Figure 3 and Table 1). For details on the mesocosms, including environmental data, please also see Liu et al. (2020).
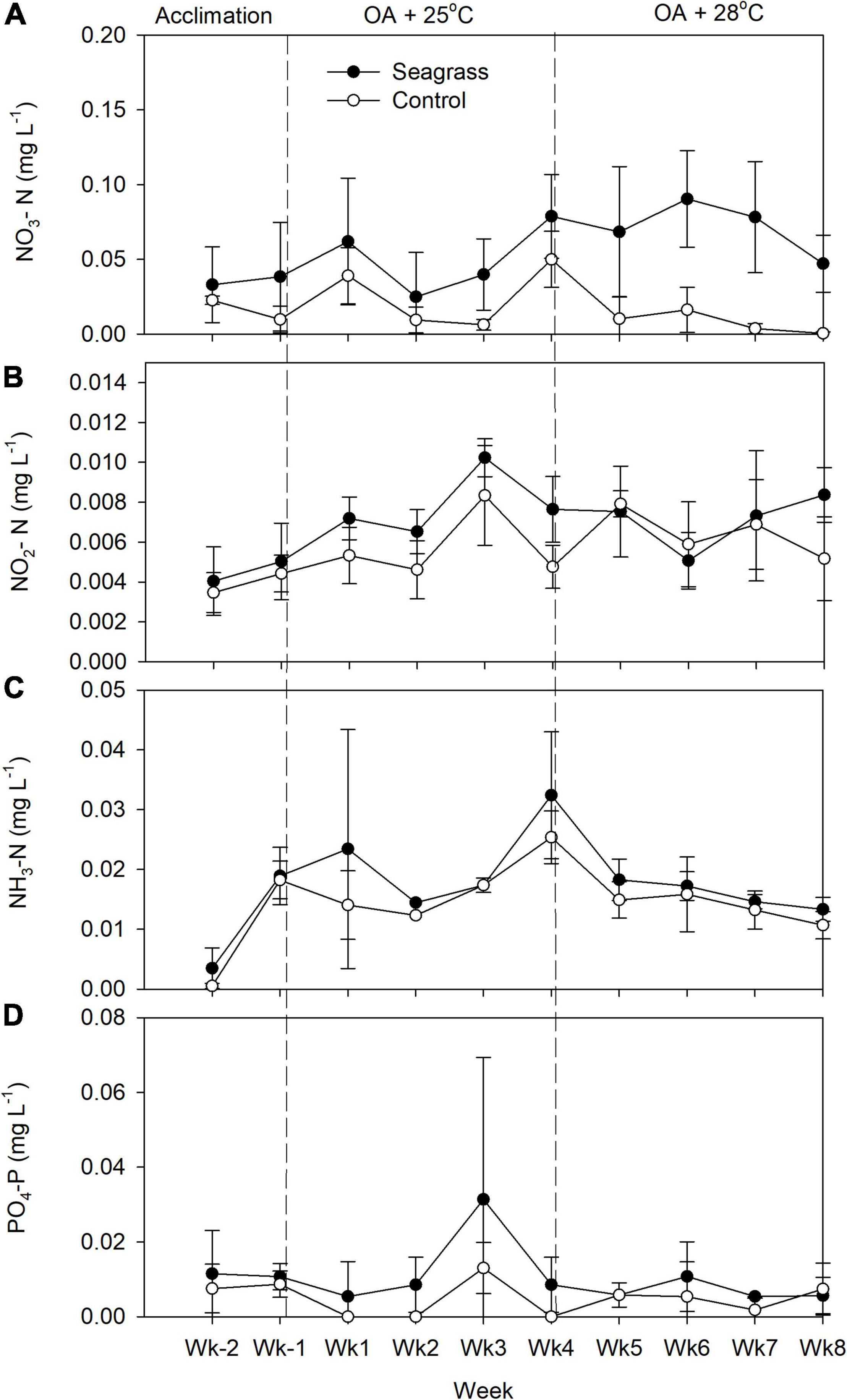
Figure 3. Weekly measurements of (A) nitrate, (B) nitrite, (C) ammonia, and (D) phosphate concentrations in the experimental mesocosms. Error bars represent standard deviation (n = 3).
18S Tag Sequencing Analysis
After removing low-quality reads and chimeras, a total of 764,049 clean sequences (average length = 304 bp) containing the V4 variable region of the 18S rRNA gene were obtained from 17 samples. These clean sequences were assigned to 1,814 unique OTUs based on 97% sequence identity (Table 2), and 474,688 sequences were of hypothetical algal origin. The rarefaction curves for algal reads did not approach a plateau (Supplementary Figure 1), indicating further sampling efforts are required to reveal the total diversity within the mesocosm microalgal community. The percentage of algae reads across samples was highly variable, ranging from 8 to 99% (average = 62%; Table 2). After normalizing, 4,698 randomly subsampled sequences were left for each sample. Table 2 presents a summary of the subsampled OTUs, coverage, Chao1 indexes for richness, as well as Shannon-Weaver and Simpson diversity indexes. All indexes were not significantly different between seagrass and control groups at both temperatures (t-test, P > 0.05).
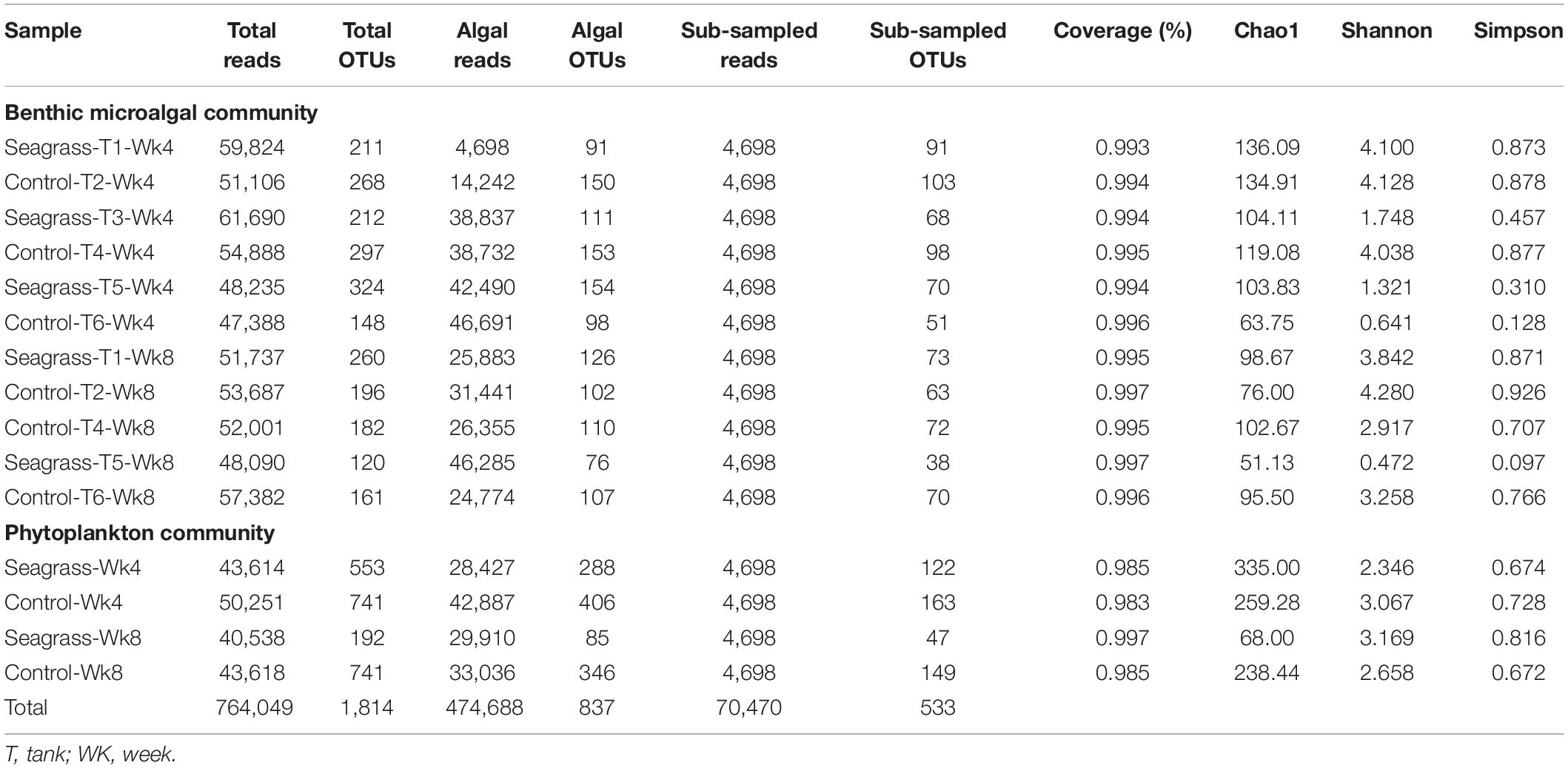
Table 2. Numbers of reads and OTUs, in addition to diversity indices, of the 18S rRNA gene sequences of all algal communities.
Phytoplankton chl-a concentrations were similar between seagrass and control mesocosms: 0.189 ± 0.050 and 0.180 ± 0.018 μg L–1 under OA + 25°C and 0.172 ± 0.047 and 0.181 ± 0.063 μg L–1 under OA + 28°C, respectively (Figure 4A and Supplementary Table 1). Phytoplankton communities (pooled genomic DNA from triplicate samples in each treatment) were dominated by Chlorophyta and Dinophyta at Wk4 between seagrass and control groups (Figure 4B). At Wk 8, the phytoplankton communities were also similar between treatments, with Dinophyta comprising >80% of the total algal OTUs (Figure 4C). However, analysis of the phytoplankton OTUs at the genus level revealed that, at 28oC, percent OTUs from the genus Paragymnodinium were 3 and 82% of the total Dinophyta OTUs in the seagrass mesocosms vs. in the controls, respectively. The dinoflagellate genus Cladocopium (formally Symbiodinium sp. clade C; LaJeunesse et al., 2018), on the other hand, were 20% and <1% of the total Dinophyta OTUs in the seagrass mesocosms vs. in the controls, respectively. Note that these community results were from one sample (pooled triplicate-samples) in each treatment.
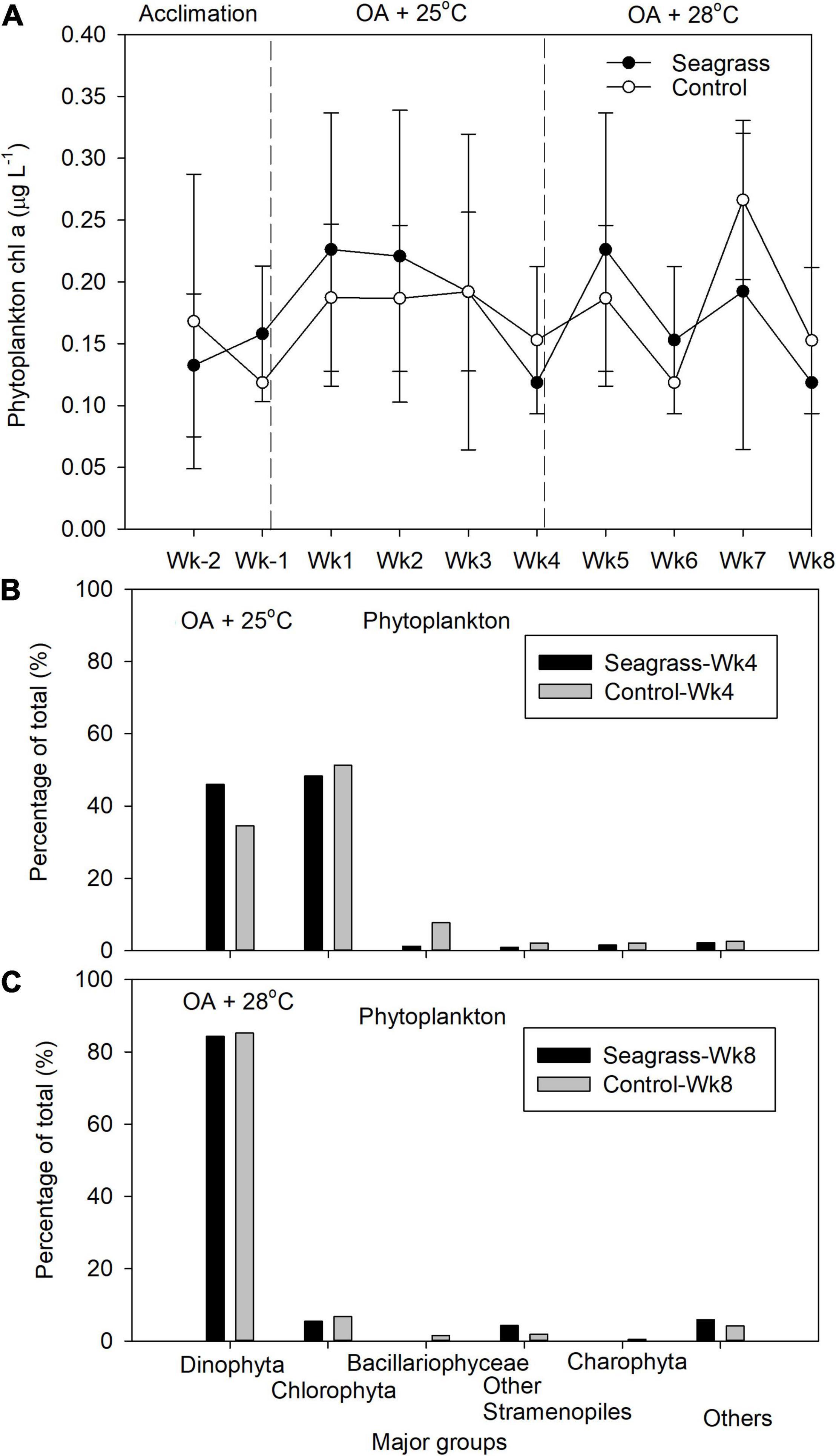
Figure 4. (A) The phytoplankton chlorophyll a concentration and relative abundance of the five most abundant phytoplankton groups after (B) 4 (Wk4) and (C) 8 (Wk8) weeks of treatment exposure. Error bars represent standard deviation (n = 3).
Benthic microalgal chl-a concentrations were significantly higher (p < 0.05) in seagrass mesocosms under both OA + 25°C and OA + 28°C (0.088 ± 0.038 and 0.434 ± 0.199 mg cm–2, respectively) compared to controls (0.041 ± 0.020 and 0.112 ± 0.036 mg cm–2, respectively, Figure 5A and Supplementary Table 1). Chlorophyta was the main benthic microalgal group in both seagrass and control groups at Wk4 (Figure 5B). After raising temperature to 28°C, the benthic microalgal communities in the seagrass group were still dominated by Chlorophyta at Wk8, whereas the controls comprised more families Bacillariophyceae and Pelogophyceae (Figure 5C). OTUs of Syndiniales were 0.04 and 0.15% of the total OTUs in the seagrass and control groups, respectively, at 25°C. However, their relative abundances increased to 2.9 and 6.5%, respectively, when temperature rose to 28oC (Figure 5C). MDS results revealed that the community structures of the benthic microalgae were similar between seagrass and control groups at Wk 4 (Figure 6A; ANOSIM test, global R = −0.111, P > 0.05) and at Wk 8 (Figure 6B; ANOSIM test, global R = 0.250, P > 0.05).
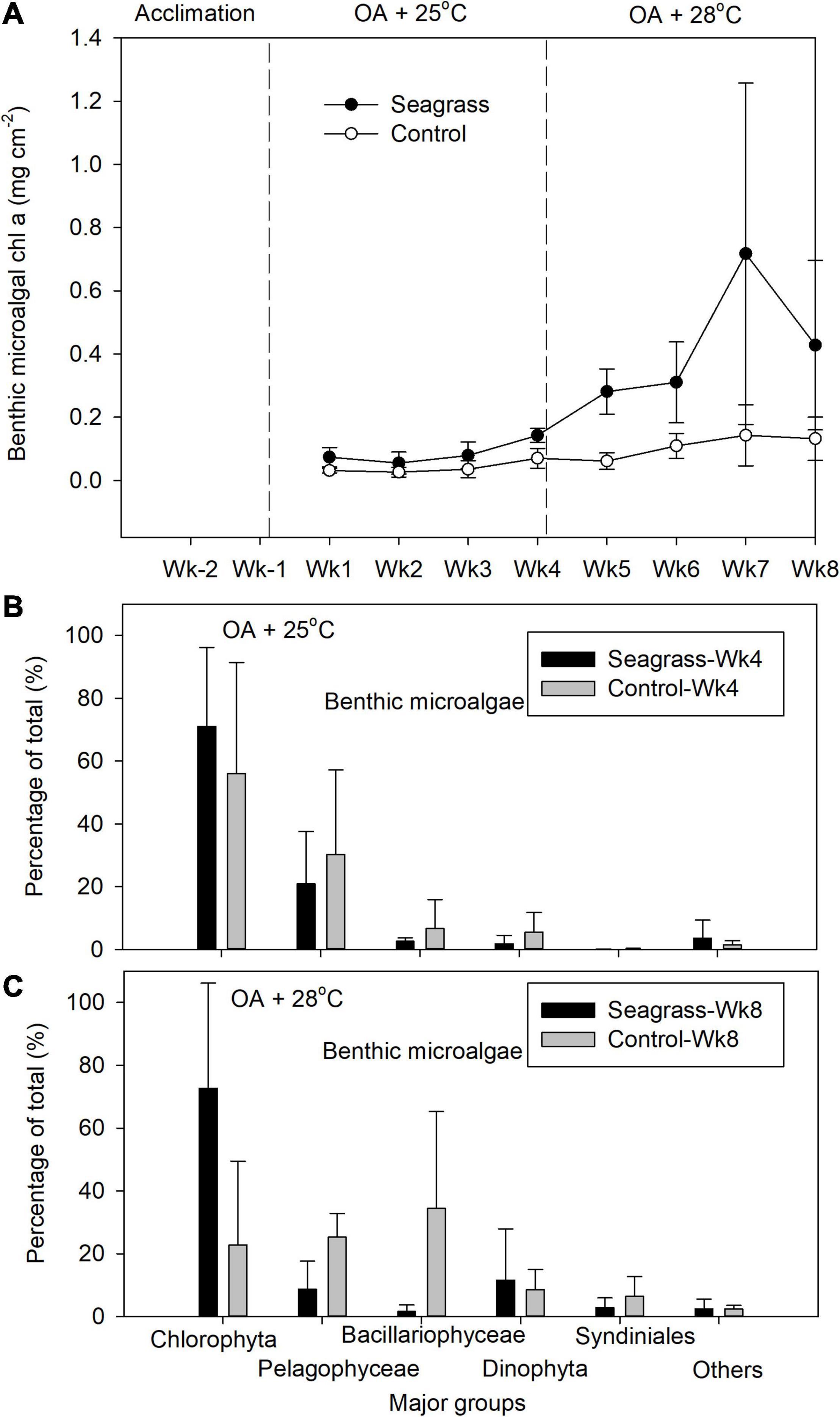
Figure 5. (A) The benthic microalgae chlorophyll a concentration and relative abundance of the five most abundant benthic microalgal groups after (B) 4 (Wk4) and (C) 8 (Wk8) weeks of treatment exposure. Error bars represent standard deviation (n = 3).
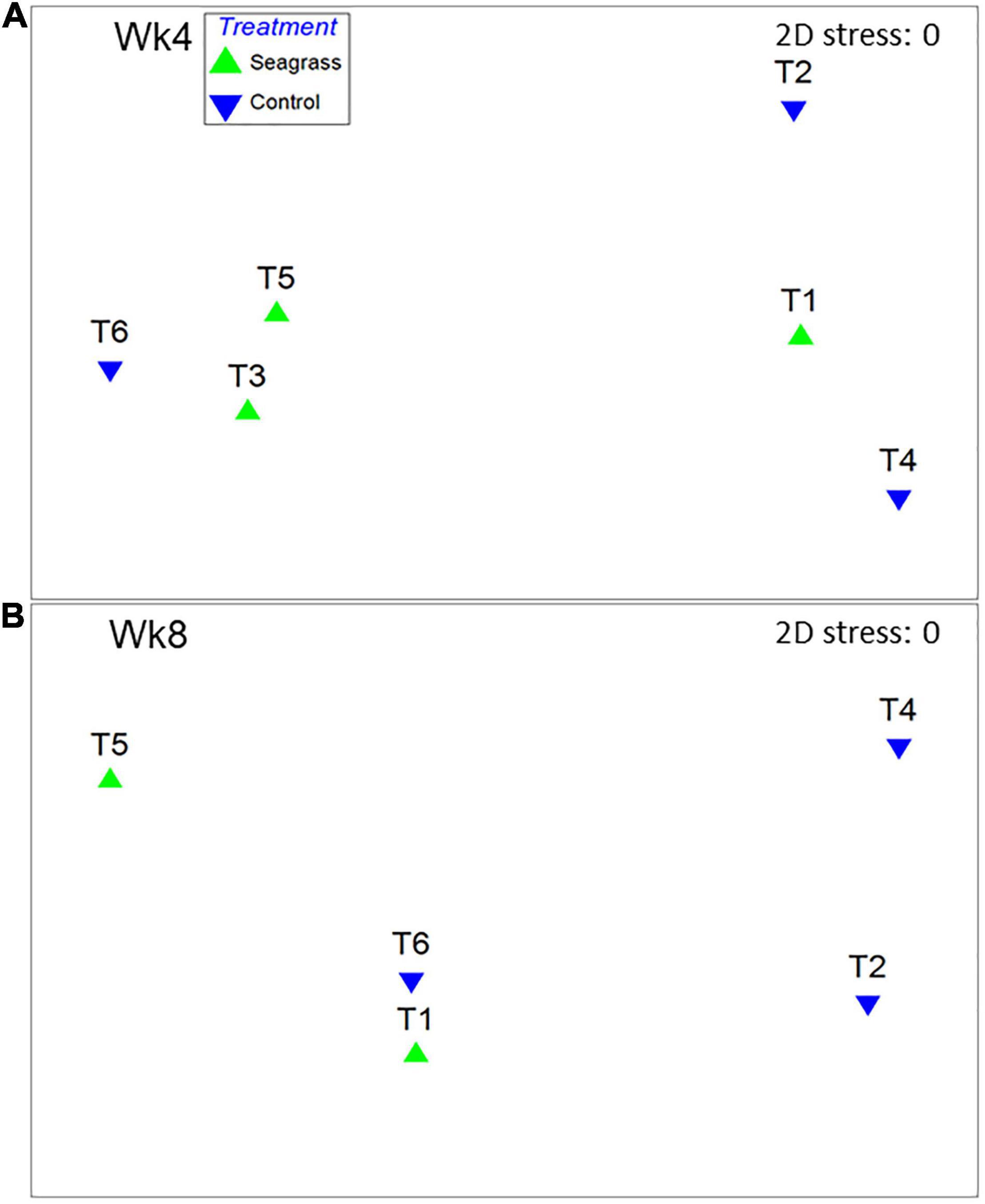
Figure 6. Multi-dimensional scaling (MDS) ordination of non-standardized, arcsine-transformed major benthic microalgal groups percentage data in (A) week-4 and (B) week-8. T1∼T6 represent the experimental mesocosms.
Discussion
A previous study has shown that increasing CO2 may boost benthic microalgal primary productivity in coral reef ecosystem (Tew et al., 2017b). In the present study, we further found that the nutrients (NO3–, NO2–, NH3–, PO43–) and benthic microalgal chl a increased even more when seagrass meadow is present in the coral reef ecosystem under OA conditions.
Contrary to our expectation of lower chl-a concentrations when seagrass blades were present, the benthic microalgal abundance was actually higher in the seagrass mesocosms at both temperatures. Since seagrass blades can change the flow regime and therefore fine sediment accumulation (Licci et al., 2019), the enhanced sedimentation (which was not measured) could have driven these elevated nutrient levels. These higher nutrient levels, then, could explain the higher benthic microalgal densities. However, a more thorough sediment analysis in the future must be undertaken to ascertain whether there is a relationship between seagrass bed hydrodynamics, sedimentation, and nutrient levels.
At OA + 28°C we saw an increase in the parasitic algae Syndiniales, a diverse yet understudied group found in all marine environments (Clarke et al., 2019). Although they have recently been reported from Taiwanese coral reefs (Cleary, 2019), their ecological niche is unknown. Whether their increase herein is due to temperature or simply the prolonged culture duration cannot be known given the experimental design utilized, though the observation that Syndiniales OTUs were in lower relative abundance in the seagrass group, may suggest an active role of the seagrass in modulating the density of this parasite.
Despite our phytoplankton OTU result represented only one sample from each treatment, it is worthy to note that the pooled phytoplankton sample collected from the seagrass mesocosms at 28°C, Wk 8, contained a high proportion of the symbiotic dinoflagellate Cladocopium sp. Given that temperatures well below the local coral bleaching threshold of ∼31°C were utilized, there was no apparent coral bleaching during the experiment; nevertheless, it cannot be ruled out that the corals themselves (rather than the sand-filtered seawater), were the source of these dinoflagellates. Since nitrate and ammonium concentrations were higher in the seagrass mesocosms at 28°C, perhaps the accelerate algal growth in hospite stimulated by partial eutrophication and an elevated N:P ratio (sensu Ezzat et al., 2016) led to the release of excess Cladocopium spp. cells from the corals, all of which naturally harbor Cladocopium (Mayfield et al., 2013). Although the physiological performance of the corals in the seagrass mesocosms was not assessed herein, coral + seagrass co-culture in OA-stimulated mesocosms actually resulted in corals that were more resilient than those of seagrass-free mesocosms in a prior work (Liu et al., 2020); this leads us to suspect that the higher Cladocopium levels in the seagrass mesocosms at high temperatures is not a testament to physiologically compromised corals. Future investigation in this area might be needed because of the growing frequencies of massive coral bleaching worldwide.
Seagrass-associated microbial communities can alter the nutrient cycles in the water column (Agawin et al., 2016) and in the sediment (Ugarelli et al., 2017). For example, Caffrey and Kemp (1990) documented higher rates of nitrification, denitrification and ammonification in the rhizosphere of Zostera marina than in bare sediments, and Agawin et al. (2016) documented significant nitrogen fixation activity in the phyllosphere of Posidonia oceanica. While most ecological functions of the seagrass microbiome and the interactions with their seagrass host are still unknown (Ugarelli et al., 2017 for more detail), the higher nutrient concentrations we observed in the seagrass mesocosms could also attribute to the seagrass-associated microbiome activities (Hurtado-McCormick et al., 2019), which in turn enhance the growth of benthic microalgae.
Elevated CO2 can increase the growth rate of diatoms (Li and Campbell, 2013) such as Skeletonema costatum (Kim et al., 2006), Nitzschia palea, Chaetoceros muelleri (Hu and Gao, 2008), Amphora coffeaeformis (Tew et al., 2014), as well as the chlorophyte Dunaliella tertiolecta (Beardall and Raven, 2004) and picocyanobacteria Synechococcus (Fu et al., 2007). However, no effects of OA were reported for diatom Nitzschia ovalis (Tew et al., 2014), the picocyanobacteria Prochlorococcus (Fu et al., 2007), and several others, suggesting that the response of some phytoplankton species to OA is negligible (Tortell et al., 2000; Fu et al., 2007). The species-specific nature of the algal OA response reflected by such heterogeneous responses may be due to differences in the efficiency of carbon acquisition (Johnson et al., 2015). Since the benthic microalgal and phytoplankton communities consist of hundreds to thousands of species (Cahoon, 1999; Tew et al., 2017b), it may be more pragmatic to instead monitor cumulative effects of OA on the system, rather than at a species by species level. At the community level, OA can enhance phytoplankton growth (McCarthy et al., 2012; Pierangelini et al., 2016), alter assemblages (Tortell et al., 2002; Ziveri et al., 2014), and negatively affect certain diatom species (Gao et al., 2012; Torstensson et al., 2012), and we found higher abundances of benthic microalgae in the seagrass mesocosms, particularly upon raising the temperature to 28°C. Although it is tempting to speculate that this could be due to seagrass sequestering of CO2, the carbonate system was actually similar between seagrass and control mesocosms. Instead, only nitrate levels differed significantly. Therefore, it may be that the elevated nitrate levels present in the seagrass mesocosms promoted the growth of benthic microalgae. However, the exact mechanism by which seagrass blades enhance benthic primary productivity remains to be determined and should be the focus of future works.
Data Availability Statement
The datasets presented in this study can be found in online repositories. The names of the repository/repositories and accession number(s) can be found in the article/ Supplementary Material.
Author Contributions
KT and P-JL conceived the presented idea, designed the experiments, verified the analytical methods, and wrote the manuscript. JK and J-OC performed DNA extractions and sequence analyses. F-CK and P-JM performed abiotic parameters analyses. AM assisted in statistical analyses and English proof-reading. All authors discussed the results and contributed to the final manuscript.
Funding
This work was supported by the Ministry of Science and Technology of Taiwan (MOST 106-2611-M-291-003 and MOST 107-2611-M-291-006) to KT.
Conflict of Interest
The authors declare that the research was conducted in the absence of any commercial or financial relationships that could be construed as a potential conflict of interest.
Supplementary Material
The Supplementary Material for this article can be found online at: https://www.frontiersin.org/articles/10.3389/fmars.2021.679683/full#supplementary-material
References
Agawin, N. S. R., Ferriol, P., Cryer, C., Alcon, E., Busquets, A., Sintes, E., et al. (2016). Significant nitrogen fixation activity associated with the phyllosphere of Mediterranean seagrass Posidonia oceanica: first report. Mar. Ecol. Prog. Ser. 551, 53–62. doi: 10.3354/meps11755
Beardall, J., and Raven, J. A. (2004). The potential effects of global climate change on microalgal photosynthesis, growth and ecology. Phycologia 43, 26–40. doi: 10.2216/i0031-8884-43-1-26.1
Caffrey, J., and Kemp, W. (1990). Nitrogen cycling in sediments with estuarine populations of Potamogeton perfoliatus and Zostera marina. Mar. Ecol. Prog. Ser. 66, 147–160. doi: 10.3354/meps066147
Cahoon, L. (1999). “The role of benthic microalgae in neritic ecosystems,” in Oceanography and Marine Biology, an Annual Review, Vol. 37, eds A. Ansell, R. Gibson, and M. Barnes (Milton Park, MI: Taylor & Francis Group), 47–86.
Caporaso, J. G., Kuczynski, J., Stombaugh, J., Bittinger, K., Bushman, F. D., Costello, E. K., et al. (2010). QIIME allows analysis of high-throughput community sequencing data. Nat. Methods 7, 335–336.
Clarke, K. R., and Warwick, R. M. (1994). Changes in Marine Communities: An Approach to Statistical Analysis and Interpretation. London: Plymouth Marine Laboratory.
Clarke, L. J., Bestley, S., Bissett, A., and Deagle, B. E. (2019). A globally distributed Syndiniales parasite dominates the Southern Ocean micro-eukaryote community near the sea-ice edge. ISME J. 13, 734–737. doi: 10.1038/s41396-018-0306-7
Cleary, D. F. R. (2019). A comparison of microeukaryote communities inhabiting sponges and seawater in a Taiwanese coral reef system. Ann. Microbiol. 69, 861–866. doi: 10.1007/s13213-019-01476-5
Cornwall, C. E., and Hurd, C. L. (2016). Experimental design in ocean acidification research: problems and solutions. ICES J. Mar. Sci. 73, 572–581. doi: 10.1093/icesjms/fsv118
de los Santos, C. B., Krause-Jensen, D., Alcoverro, T., Marba, N., Duarte, C. M., van Katwijk, M. M., et al. (2019). Recent trend reversal for declining European seagrass meadows. Nat. Commun. 10:8.
Edgar, R. C. (2010). Search and clustering orders of magnitude faster than BLAST. Bioinformatics 26, 2460–2461. doi: 10.1093/bioinformatics/btq461
Edgar, R. C., Haas, B. J., Clemente, J. C., Quince, C., and Knight, R. (2011). UCHIME improves sensitivity and speed of chimera detection. Bioinformatics 27, 2194–2200. doi: 10.1093/bioinformatics/btr381
Ezzat, L., Towle, E., Irisson, J. O., Langdon, C., and Ferrier-Pages, C. (2016). The relationship between heterotrophic feeding and inorganic nutrient availability in the scleractinian coral T. reniformis under a short-term temperature increase. Limnol. Oceanogr. 61, 89–102. doi: 10.1002/lno.10200
Field, J. G., Clarke, K. R., and Warwick, R. M. (1982). A practical strategy for analyzing multispecies distribution patterns. Mar. Ecol. Prog. Ser. 8, 37–52. doi: 10.3354/meps008037
Fu, F. X., Warner, M. E., Zhang, Y. H., Feng, Y. Y., and Hutchins, D. A. (2007). Effects of increased temperature and CO2 on photosynthesis, growth, and elemental ratios in marine Synechococcus and Prochlorococcus (Cyanobacteria). J. Phycol. 43, 485–496. doi: 10.1111/j.1529-8817.2007.00355.x
Gao, K., Xu, J., Gao, G., Li, Y., Hutchins, D. A., Huang, B., et al. (2012). Rising CO2 and increased light exposure synergistically reduce marine primary productivity. Nat. Clim. Chang. 2, 519–523. doi: 10.1038/nclimate1507
Hu, H. H., and Gao, K. S. (2008). Impacts of CO2 enrichment on growth and photosynthesis in freshwater and marine diatoms. Chin. J. Oceanol. Limnol. 26, 407–414. doi: 10.1007/s00343-008-0407-7
Huan, Y. H., Lee, C. L., Chung, C. Y., Hsiao, S. C., and Lin, H. J. (2015). Carbon budgets of multispecies seagrass beds at Dongsha Island in the South China Sea. Mar. Environ. Res. 106, 92–102. doi: 10.1016/j.marenvres.2015.03.004
Hurtado-McCormick, V., Kahlke, T., Petrou, K., Jeffries, T., Ralph, P. J., and Seymour, J. R. (2019). Regional and microenvironmental scale characterization of the Zostera muelleri seagrass microbiome. Front. Microbiol. 10:1011. doi: 10.3389/fmicb.2019.01011
Inaba, N., Trainer, V. L., Nagai, S., Kojima, S., Sakami, T., Takagi, S., et al. (2019). Dynamics of seagrass bed microbial communities in artificial Chattonella blooms: a laboratory microcosm study. Harmful Algae 84, 139–150. doi: 10.1016/j.hal.2018.12.004
Invers, O., Romero, J., and Perez, M. (1997). Effects of pH on seagrass photosynthesis: a laboratory and field assessment. Aquat. Bot. 59, 185–194. doi: 10.1016/s0304-3770(97)00072-7
IPCC (2014). “Climate change 2014: Synthesis report,” in Contribution of Working Groups I, II and III to the Fifth Assessment Report of the Intergovernmental Panel on Climate Change, eds Core Writing Team, R. K. Pachauri and L. A. Meyer (Geneva: IPCC), 151.
Johnson, V. R., Brownlee, C., Milazzo, M., and Hall-Spencer, J. M. (2015). Marine microphytobenthic assemblage shift along a natural shallow-water CO2 gradient subjected to multiple environmental stressors. J. Mar. Sci. Eng. 3, 1425–1447. doi: 10.3390/jmse3041425
Kim, J. M., Lee, K., Shin, K., Kang, J. H., Lee, H. W., Kim, M., et al. (2006). The effect of seawater CO2 concentration on growth of a natural phytoplankton assemblage in a controlled mesocosm experiment. Limnol. Oceanogr. 51, 1629–1636. doi: 10.4319/lo.2006.51.4.1629
Kuo, J., Tew, K. S., Ye, Y. X., Cheng, J. O., Meng, P. J., and Glover, D. C. (2014). Picoplankton dynamics and picoeukaryote diversity in a hyper-eutrophic subtropical lagoon. J. Environ. Sci. Health A Tox. Hazard Subst. Environ. Eng. 49, 116–124. doi: 10.1080/10934529.2013.824784
LaJeunesse, T. C., Parkinson, J. V., Gabrielson, P. W., Jeong, H. J., Reimer, J. D., Voolstra, C. R., et al. (2018). Systematic revision of Symbiodiniaceae highlights the antiquity and diversity of coral endosymbionts. Curr. Biol. 28, 2570–2580. doi: 10.1016/j.cub.2018.07.008
Lamb, J. B., van de Water, J., Bourne, D. G., Altier, C., Hein, M. Y., Fiorenza, E. A., et al. (2017). Seagrass ecosystems reduce exposure to bacterial pathogens of humans, fishes, and invertebrates. Science 355, 731–733. doi: 10.1126/science.aal1956
Lee, C. L., Wen, C. K. C., Huang, Y. H., Chung, C. Y., and Lin, H. J. (2019). Ontogenetic habitat usage of juvenile carnivorous fish among seagrass-coral mosaic habitats. Diversity 11:17.
Lewis, E., Wallace, D., and Allison, L. J. (1998). Program Developed for CO2 System Calculations. Bethesda: Carbon Dioxide Information Analysis Center.
Li, G., and Campbell, D. A. (2013). Rising CO2 interacts with growth light and growth rate to alter photosystem II photoinactivation of the coastal diatom Thalassiosira pseudonana. PLoS One 8:e55562. doi: 10.1371/journal.pone.0055562
Licci, S., Nepf, H., Delolme, C., Marmonier, P., Bouma, T. J., and Puijalon, S. (2019). The role of patch size in ecosystem engineering capacity: a case study of aquatic vegetation. Aquat. Sci. 81:11.
Lin, H. J., Lee, C. L., Peng, S. E., Hung, M. C., Liu, P. J., and Mayfield, A. B. (2018). The effects of El Nino-southern oscillation events on intertidal seagrass beds over a long-term timescale. Glob. Chang. Biol. 24, 4566–4580. doi: 10.1111/gcb.14404
Liu, P. J., Ang, S. J., Mayfield, A. B., and Lin, H. J. (2020). Influence of the seagrass Thalassia hemprichii on coral reef mesocosms exposed to ocean acidification and experimentally elevated temperatures. Sci. Total Environ. 700, 134464. doi: 10.1016/j.scitotenv.2019.134464
Liu, P. J., Hsin, M. C., Huang, Y. H., Fan, T. Y., Meng, P. J., Lu, C. C., et al. (2015). Nutrient enrichment coupled with sedimentation favors sea anemones over corals. PLoS One 10:e0125175. doi: 10.1371/journal.pone.0125175
Liu, P. J., Lin, S. M., Fan, T. Y., Meng, P. J., Shao, K. T., and Lin, H. J. (2009). Rates of overgrowth by macroalgae and attack by sea anemones are greater for live coral than dead coral under conditions of nutrient enrichment. Limnol. Oceanogr. 54, 1167–1175. doi: 10.4319/lo.2009.54.4.1167
Magoc, T., and Salzberg, S. L. (2011). FLASH: fast length adjustment of short reads to improve genome assemblies. Bioinformatics 27, 2957–2963. doi: 10.1093/bioinformatics/btr507
Marbà, N., Holmer, M., Gacia, E., and Barron, C. (2007). Seagrasses: Biology, Ecology and Conservation. Berlin: Springer, 135–157.
Mayfield, A. B., Fan, T. Y., and Chen, C. S. (2013). Physiological acclimation to elevated temperature in a reef-building coral from an upwelling environment. Coral Reefs 32, 909–921. doi: 10.1007/s00338-013-1067-4
McCarthy, A., Rogers, S. P., Duffy, S. J., and Campbell, D. A. (2012). Elevated carbon dioxide differentially alters the photophysiology of Thalassiosira pseudonana (Bacillariophyceae) and Emiliania huxleyi (Haptophyta). J. Phycol. 48, 635–646. doi: 10.1111/j.1529-8817.2012.01171.x
Pai, S. C., and Riley, J. P. (1994). Determination of nitrate in the presence of nitrite in natural waters by flow injection analysis with a non-quantitative on-line cadmium redactor. Int. J. Environ. Anal. Chem. 57, 263–277. doi: 10.1080/03067319408027460
Pai, S. C., Tsau, Y. J., and Yang, T. I. (2001). pH and buffering capacity problems involved in the determination of ammonia in saline water using the indophenol blue spectrophotometric method. Anal. Chim. Acta 434, 209–216. doi: 10.1016/s0003-2670(01)00851-0
Pai, S. C., Yang, C. C., and Riley, J. P. (1990). Formation kinetics of the pink azo dye in the determination of nitrite in natural waters. Anal. Chim. Acta 232, 345–349. doi: 10.1016/s0003-2670(00)81252-0
Parsons, T. R. (2013). A Manual of Chemical & Biological Methods for Seawater Analysis. Amsterdam: Elsevier.
Pierangelini, M., Raven, J. A., and Giordano, M. (2016). The relative availability of inorganic carbon and inorganic nitrogen influences the response of the dinoflagellate Protoceratium reticulatum to elevated CO2. J. Phycol. 53, 298–307. doi: 10.1111/jpy.12463
Quast, C., Pruesse, E., Yilmaz, P., Gerken, J., Schweer, T., Yarza, P., et al. (2013). The SILVA ribosomal RNA gene database project: improved data processing and web-based tools. Nucleic Acids Res. 41, D590–D596.
Tew, K. S., Jhange, Y. S., Meng, P. J., and Leu, M. Y. (2017a). Environmental factors influencing the proliferation of microscopic epiphytic algae on giant kelp under aquarium conditions. J. Appl. Phycol. 29, 2877–2886. doi: 10.1007/s10811-017-1148-9
Tew, K. S., Siao, Y. J., Liu, P. J., Lo, W. T., and Meng, P. J. (2017b). Taiwanese marine microbenthic algal communities remain similar yet chlorophyll a concentrations rise in mesocosms with elevated CO2 and temperature. Mar. Pollut. Bull. 124, 929–937. doi: 10.1016/j.marpolbul.2017.06.050
Tew, K. S., Kao, Y.-C., Ko, F.-C., Kuo, J., Meng, P.-J., Liu, P.-J., et al. (2014). Effects of elevated CO2 and temperature on the growth, elemental composition, and cell size of two marine diatoms: potential implications of global climate change. Hydrobiologia 741, 79–87. doi: 10.1007/s10750-014-1856-y
Tew, K. S., Meng, P. J., and Leu, M. Y. (2012). Factors correlating with deterioration of giant kelp Macrocystis pyrifera (Laminariales, Heterokontophyta) in an aquarium setting. J. Appl. Phycol. 24, 1269–1277. doi: 10.1007/s10811-011-9775-z
Tew, K. S., Meng, P. J., Lin, H. S., Chen, J. H., and Leu, M. Y. (2013). Experimental evaluation of inorganic fertilization in larval giant grouper (Epinephelus lanceolatus Bloch) production. Aquac. Res. 44, 439–450. doi: 10.1111/j.1365-2109.2011.03051.x
Tiling, K., and Proffitt, C. E. (2017). Effects of Lyngbya majuscula blooms on the seagrass Halodule wrightii and resident invertebrates. Harmful Algae 62, 104–112. doi: 10.1016/j.hal.2016.11.015
Torstensson, A., Chierici, M., and Wulff, A. (2012). The influence of increased temperature and carbon dioxide levels on the benthic/sea ice diatom Navicula directa. Pol. Biol. 35, 205–214. doi: 10.1007/s00300-011-1056-4
Tortell, P. D., DiTullio, G. R., Sigman, D. M., and Morel, F. M. (2002). CO2 effects on taxonomic composition and nutrient utilization in an Equatorial Pacific phytoplankton assemblage. Mar. Ecol. Prog. Ser. 236, 37–43. doi: 10.3354/meps236037
Tortell, P. D., Rau, G. H., and Morel, F. M. (2000). Inorganic carbon acquisition in coastal Pacific phytoplankton communities. Limnol. Oceanogr. 45, 1485–1500. doi: 10.4319/lo.2000.45.7.1485
Ugarelli, K., Chakrabarti, S., Laas, P., and Stingl, U. (2017). The seagrass holobiont and its microbiome. Microorganisms 5:81. doi: 10.3390/microorganisms5040081
Unsworth, R. K. F., Collier, C. J., Henderson, G. M., and McKenzie, L. J. (2012). Tropical seagrass meadows modify seawater carbon chemistry: implications for coral reefs impacted by ocean acidification. Environ. Res. Lett. 7:024026. doi: 10.1088/1748-9326/7/2/024026
Unsworth, R. K. F., McKenzie, L. J., Collier, C. J., Cullen-Unsworth, L. C., Duarte, C. M., Eklof, J. S., et al. (2019a). Global challenges for seagrass conservation. Ambio 48, 801–815.
Unsworth, R. K. F., Nordlund, L. M., and Cullen-Unsworth, L. C. (2019b). Seagrass meadows support global fisheries production. Conserv. Lett. 12:e12566. doi: 10.1111/conl.12566
Keywords: Cladocopium, climate change, dinoflagellates, nutrients, seagrass, sedimentation, syndiniales
Citation: Tew KS, Kuo J, Cheng J-O, Ko F-C, Meng P-J, Mayfield AB and Liu P-J (2021) Impacts of Seagrass on Benthic Microalgae and Phytoplankton Communities in an Experimentally Warmed Coral Reef Mesocosm. Front. Mar. Sci. 8:679683. doi: 10.3389/fmars.2021.679683
Received: 12 March 2021; Accepted: 14 May 2021;
Published: 10 June 2021.
Edited by:
Maren Ziegler, University of Giessen, GermanyReviewed by:
Caroline Eve Dubé, Laval University, CanadaElena Bollati, National University of Singapore, Singapore
Copyright © 2021 Tew, Kuo, Cheng, Ko, Meng, Mayfield and Liu. This is an open-access article distributed under the terms of the Creative Commons Attribution License (CC BY). The use, distribution or reproduction in other forums is permitted, provided the original author(s) and the copyright owner(s) are credited and that the original publication in this journal is cited, in accordance with accepted academic practice. No use, distribution or reproduction is permitted which does not comply with these terms.
*Correspondence: Kwee Siong Tew, dGV3a3NAbm1tYmEuZ292LnR3; Pi-Jen Liu, cGlqZW5saXVAZ21zLm5kaHUuZWR1LnR3