- 1Centre for Marine Ecosystems Research, School of Science, Edith Cowan University, Joondalup, WA, Australia
- 2School of Environmental and Life Sciences, The University of Newcastle, Callaghan, NSW, Australia
Microbes are ubiquitous but our knowledge of their effects on consumers is limited in benthic marine systems. Shorelines often form hotspots of microbial and detritivore activity due to the large amounts of detrital macrophytes that are exported from other coastal ecosystems, such as kelp forests, and accumulate in these systems. Shoreline ecosystems therefore provide a useful model system to examine microbial-detritivore interactions. We experimentally test whether bacteria in the biofilm of kelp provide a bottom-up influence on growth and reproductive output of detritivores in shorelines where detrital kelp accumulates, by manipulating the bacterial abundances on kelp (Ecklonia radiata). The growth rates for both male and female amphipods (Allorchestes compressa) were greater in treatments containing bacteria than those in which bacteria were reduced through antibiotic treatment, and this effect was greater for males offered aged kelp. The proportions of ovigerous females were greater when reared on kelp with intact bacteria, indicating a more rapid reproductive development in the presence of more bacteria. Bacterial abundance had little to no influence on nutrient content and palatability of kelp, based on tissue toughness, nitrogen and carbon content and C:N ratio. Thus, the most likely pathway for a microbial effect on detritivores was through feeding on kelp-associated bacteria. Regardless of the pathway, kelp-associated microbes have a strong influence on the fitness of a highly abundant detritivore that feeds preferentially on E. radiata in shoreline systems, and therefore form a hidden trophic step in this “brown” food web and a hotspot of secondary production.
Introduction
Microbes are ubiquitous and can produce strong bottom-up and top-down controls on ecosystem functions (Crowther and Grossart, 2015). Much of our understanding of how microbes provide a bottom-up influence on secondary production is limited to detrital-based (“brown”) food webs in terrestrial systems, where microbes can improve nutritional quality of detritus (Zimmer and Topp, 1997; Filipiak and Weiner, 2014), provide a direct nutrient-rich food source (Thompson et al., 1999), and ultimately improve fitness of consumers through increased reproductive success and growth (Zimmer and Topp, 1997; Horváthová et al., 2016). In marine ecosystems, our understanding of the bottom-up controls of microbes on consumers is limited (except pelagic systems, see Pomeroy et al., 2007), although they are known to form highly abundant and diverse communities on the surfaces of benthic marine macrophytes (Egan et al., 2013; Weigel and Pfister, 2019). Bacteria form the main component of microbial communities in benthic systems (Wahl et al., 2012), providing a range of functions that can have both positive (e.g., provision of nutrients) and negative (e.g., disease) effects on their host (Egan et al., 2013). However, two decades of microbe-host research has invariably focused on the interactions between microbial communities and their hosts (Douglas, 2018). Surprisingly, there has been very limited research on the influence of macrophyte-associated microbes on consumers, despite the importance of grazing and detritivory in coastal marine systems (Poore et al., 2012).
Shorelines often form hotspots of decomposition via microbes and detritivores due to the large amounts of detrital macrophyte material (known as wrack) that accumulates in these systems (Rodil et al., 2019; van Erk et al., 2020). Detrital material exported from highly productive kelp forests and seagrass meadows (Heck et al., 2008; Krumhansl and Scheibling, 2012) form the bulk of wrack in shoreline ecosystems (Hyndes et al., 2014). Such material that fluxes across ecosystem boundaries are termed “spatial subsidies” when they flow from a donor ecosystem (more productive) to a recipient ecosystem (less productive) where it enhances production and biodiversity (Polis et al., 1997), and microbes have been proposed to play an important role in this process (Säwström et al., 2016). In shoreline ecosystems, kelp appears to play a disproportionately important role in their food webs compared with other forms of detrital inputs (Lastra et al., 2008; Crawley et al., 2009; Suárez-Jiménez et al., 2017). Thus, kelp detritus in sandy shoreline ecosystems provides a suitable model system to examine the bottom-up influence of microbes on the detrital-based coastal food webs.
Here, we examine the role of microbes in enhancing secondary production in the detrital-based food web of sandy shorelines, using the kelp Ecklonia radiata as a model of a highly productive autotrophic system that exports material into sandy-beach ecosystems. We experimentally tested whether kelp-associated microbes enhanced growth and reproduction of detritivores by manipulating bacterial abundances in kelp biofilm. To establish whether food palatability and nutritional quality explain any effect of microbes on detritivores, we also examined traits of kelp with intact and reduced microbial biofilms during the course of the experiment. Finally, we determined kelp biomass and bacterial abundances in the study region to place the laboratory experiment in the context of the natural system.
Materials and Methods
Study Design and Rationale
The amphipod Allorchestes compressa is abundant in moist wrack in the lower zone of the sandy beach and the nearshore waters adjacent to the beach in south-western Australia (Crawley and Hyndes, 2007; Ince et al., 2007). Aquaria-reared amphipods were fed different treatments of the kelp E. radiata to determine whether growth rate and reproduction were influenced by bacteria. This kelp is a highly productive and abundant canopy-forming kelp on reefs in the temperate regions of Australasia (Kirkman and Kendrick, 1997), which exports large biomass of kelp (de Bettignies et al., 2013) to other coastal ecosystems, particularly along shorelines (Figure 1A, Kirkman and Kendrick, 1997). A. compressa (Figure 1B inset) is an ideal model species to examine the effect of microbes on detritivores as it: (1) has successfully been used in aquaria experiments in the past (Robertson and Lucas, 1983; Crawley and Hyndes, 2007); (2) is a highly abundant detritivore in wrack accumulations along the shoreline of south-western Australia, where it grazes on fresh and aged E. radiata (Robertson and Lucas, 1983; Crawley and Hyndes, 2007); (3) has been shown to have higher growth, survival and reproductive rates on aged vs fresh kelp (Robertson and Lucas, 1983); and (4) is a major food source to higher order consumers (Crawley et al., 2009).
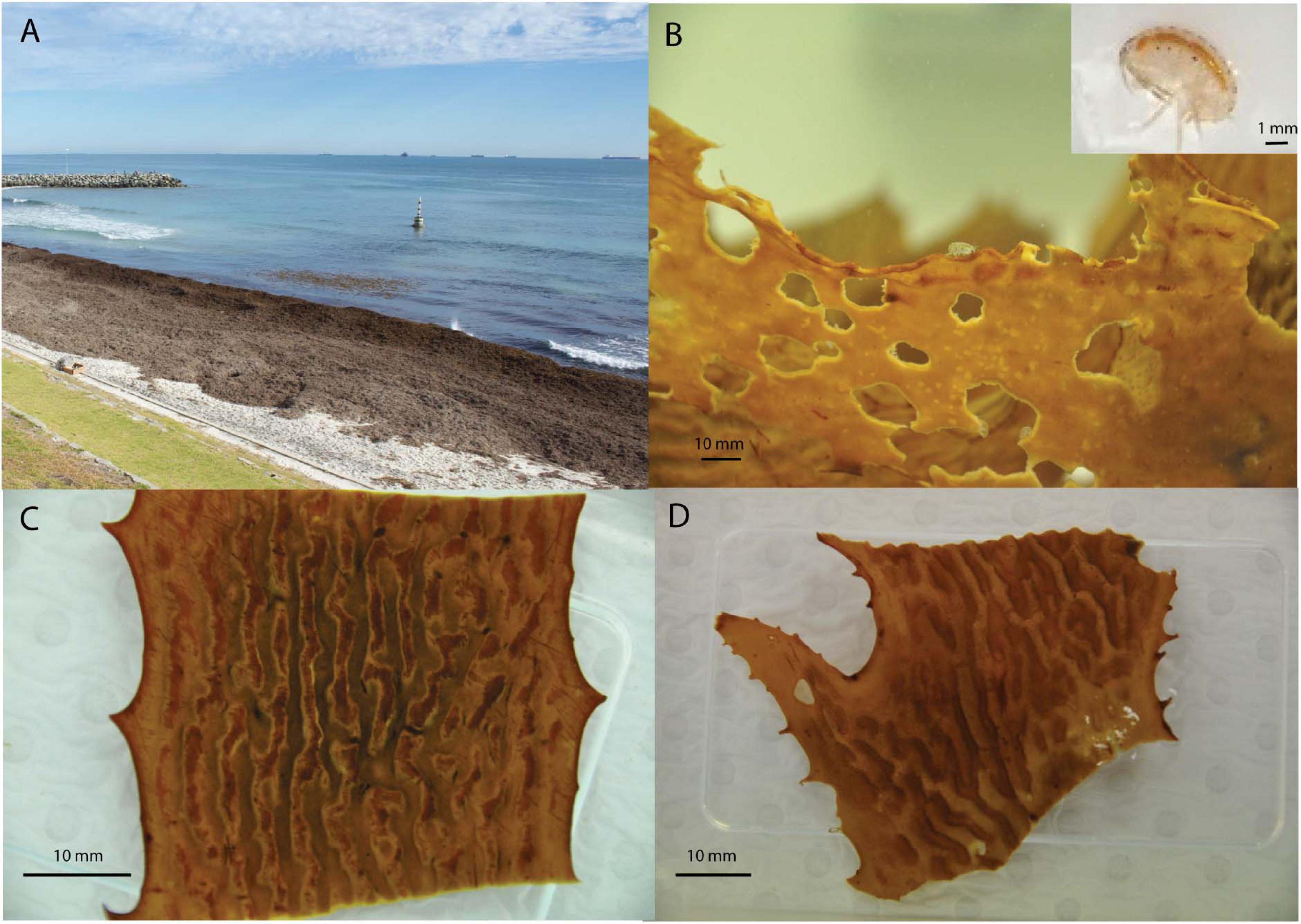
Figure 1. (A) Wrack in the surf zone and on the beach in south-western Australia; (B) the amphipod Allorchestes compressa (inset) feeding on the kelp Ecklonia radiata and its biofilm; and examples of the levels of biofilm on experimental pieces of kelp which (C) had not been subjected antiobiotic treatment and (D) had been treated with antibiotics.
Juvenile amphipods were experimentally raised on fresh and aged E. radiata (“Type”), either treated with antibiotics to reduce bacterial abundances or left untreated to represent kelp with natural abundances of bacteria (“Treatment”: control and antibiotic treated). Because bacterial abundances on kelp increase with decomposition (Koop and Griffiths, 1982), fresh vs aged kelp represented a range of decomposition levels of kelp in shoreline wrack. We confirmed that the use of aged and fresh kelp mimicked bacterial abundances found on shoreline wrack by comparing bacterial abundances on kelp in the experiments and in the field (see section “Results”). The antibiotic treatment procedure used here (see below) has been shown to have no effect on the chemistry of macroalgae but affects the diversity and abundances of bacteria (Huggett et al., 2006). Assessment of the broad chemical and physical properties of treated and control kelp in the current study indicated no effect on the chemical properties or tissue toughness of kelp (see section “Results”). The aquarium experiment comprised five replicates for each treatment/type combination, with the experiment run over 6 weeks to examine growth and 8 weeks to examine reproduction of A. compressa. Bacterial abundances, along with variables representing food palatability and quality, were measured at intervals throughout the experiment.
Collection and Maintenance of Kelp and Amphipods
Kelp with the holdfast was harvested from reefs near Perth (−31°49′13.60″S, 115°44′13.46″E) during September-December 2014, and transported to aquaria facilities, where they were rinsed with fresh seawater to remove any adhering fauna and sand. The kelp (one whole sporophyte per tank) was then placed in 10 L aquaria containing 8 L of fresh seawater. The water was kept aerated and maintained at 22∘C with a 12/12 h light/dark cycle for 2 weeks, to represent “aged” kelp with higher bacterial loads present in the surf zone. Fresh kelp was collected from the same location just prior to the initiation of the experiment, to represent “fresh” kelp. This procedure was repeated two times each week over 10 weeks, allowing fresh and aged kelp pieces to be replaced in the experiment frequently and accounting for temporal variability in microbial composition and abundances in the biofilm of kelp in the experiment.
The amphipod A. compressa was harvested in October 2014 from the surf zone near the location for kelp collection. Fresh seawater was filtered through a 4 mm and then a 125 μm mesh and the retained amphipods were placed in a 20 L container with fresh seawater, then transported and transferred to 30 L aquaria containing aerated fresh seawater, and maintained in similar conditions as the kelp. They were fed on a mixture of brown algae at various levels of decomposition and allowed to acclimatize to the new environment. Juveniles resulting from mating of the captured amphipods were separated from the parent population a day prior to the experiment and housed in separate aquaria under the same conditions as the parents.
Treatment of Kelp
Freshly harvested and aged kelp were cut into pieces of 150–200 g wet weight (n = 5) using a sterile blade and placed immediately into sterile bottles containing sterile, 0.22 μm-filtered seawater. Capped bottles were shaken vigorously for a few seconds to remove loosely attached macrobionts (Kientz et al., 2011), repeating the procedure twice. For antibiotic treatment, both sides of the kelp piece were wiped on a sterile agar plate to facilitate the physical removal of the biofilm from the surface of the macroalga (Huggett et al., 2006), and then immersed for 10 min in 10% BetadineTM (povidone-iodine) solution, prepared using sterile 0.22 μm-filtered seawater. Pieces were again wiped on sterile agar plates and then immersed in a sterile 0.22 μm-filtered antibiotic solution containing 20 mg/l Streptomycin sulfate, 10 mg/l Kanamycin sulfate and 10 mg/l Penicillin-G (sodium salt) for 24 h (Huggett et al., 2006). Kelp pieces were then wiped on sterile agar plates again and rinsed in sterile 0.22 μm-filtered seawater, followed by two 1-min immersions in 10% BetadineTM solution and two rinses in 0.22 μm sterile filtered seawater before deploying in the experiment. This procedure was adopted to remove any residual antibiotic and BetadineTM and minimize their potential influence on grazers. Evidence from control experiments in Huggett et al. (2006) indicated that, while this procedure involves handling of algae across a number of days, the main effect is from the antibiotic treatment, rather than any impacts of handling.
Experimental Procedures
The experimental aquaria consisted of 0.75 L containers under the same conditions as the breeding aquarium, but using 0.22 μm-filtered, autoclaved seawater. Treated and untreated fresh and aged kelp were randomly allocated to containers housing amphipods and replaced every 24 h to maintain consistent bacterial and kelp condition across the experiment. Just prior to the start of the experiment, the lengths of five of the 105 randomly-selected juvenile amphipods for each replicate were measured to the nearest 0.001 mm along the curved dorsal surface from the anterior of the head to the tip of the telson (Poore et al., 2013) using images captured by a NIKON D5000 camera mounted on a dissecting microscope at 40X magnification and Image J software (version 1.46r). The remaining 100 amphipods were added to each replicate container and maintained for a 6-week period to determine growth, and a further 2 weeks to determine the reproductive output. For growth, the termination point was based on the time at which amphipods showed the first signs of pairing, which represented a sign of maturity and maximum length. For reproduction, the termination point was based on time when eggs or broods were first visible in the most mature amphipods. Continuous monitoring prior to this termination point confirmed that the pairings and associated broods represented the first generation of broods.
At the termination of the growth component of the experiment, 5 male and 5 female amphipods were randomly selected from each replicate container and measured for their final lengths using the above procedure. The remaining amphipods continued to be raised for a further 2 weeks, after which the number of females with eggs or broods and the remaining female and male amphipods were counted. For females with eggs or broods, the eggs and juveniles were dissected from the brood pouch under a dissecting microscope using fine needle and forceps. Images of dissected female amphipods and eggs were captured using a NIKON D5000 camera mounted on a dissecting microscope and used to determine the number of eggs per female.
Bacterial Abundance on Kelp
To enumerate bacteria that were present on kelp tissue from each of the four treatment/type combinations, a sub-sample of the kelp tissue designated to each replicate aquarium at the start (Week 1), middle (Week 4), and end of the experiment (Week 8) was retained. Bacteria were detached from the kelp substrate by weighing 1 g (wet weight) of kelp from each replicate piece separately and immediately placing the material in 9 ml 0.22 μm sterile filtered seawater and shaken vigorously by hand. The samples were then sonicated in an ultrasonic bath for 3 min and then shaken for 10 min on an orbital shaker at highest speed (Buesing and Gessner, 2002). An aliquot of the supernatant was fixed in glutaraldehyde (final concentration 2%) and stored in −20∘C. Later, the samples were thawed in the dark and bacterial abundances were enumerated using a Beckman Coulter GalliosTM flow cytometer (FC), equipped with an air-cooled laser providing 22 mW at 488 nm band-pass filter. 20 μl of each sample was added to 380 μl of 0.22 μm filtered TE (Tris–EDTA, pH = 8.0) buffer + 10 μl of SYBR Gold (nucleic acid DNA stain, at a 1/20,000 final dilution of the stock solution supplied by the manufacturer) and incubated in the dark for 15 min in an 80∘C water bath (Marie et al., 1999). After incubation, 5 μl of vortexed 0.95 μm beads (Polysciences Inc.), with final concentration of 105 beads ml–1, was added. This mixture was then transferred to U-bottom polypropylene tubes and read on the flow cytometer, which was calibrated before and after each sample series. The cytograms were analyzed using Kaluza Analysis version 2.1 software.
Food Quality and Palatability Characteristics of Kelp
Toughness and nutrient content of kelp were determined for a subsample of kelp material placed in each replicate in each treatment/type at the start (Week 1), middle (Week 4), and end of the experiment (Week 8). The toughness of kelp tissue was measured immediately using a penetrometer (Duffy and Hay, 1991), consisting of a pointed needle (1 mm diameter) vertically glued to a cup, pointing down and mounted over the piece of kelp tissue placed on the bottom panel. Dried sand was added to the cup until the needle pierced the kelp tissue. The weight of cup + needle + sand was recorded as the weight required to penetrate the kelp tissue, expressed in grams (g). For total carbon (TC) and nitrogen (TN) content of kelp, samples were defrosted after being frozen at −20∘C, oven dried at 70∘C for 48 h, and then ground to a fine powder using a ball mill grinder. Samples were analyzed for N and C content (% by weight) using an Automated Nitrogen Carbon Analyzer system consisting of a Sercon 20-22 mass spectrometer and an EA (SERCON, United Kingdom).
In situ Kelp and Bacterial Abundances
Wrack was collected in July, August and September/October 2013 from the same locations described above in the surf zone and on the beach (−31°49′13.60″S, 115°44′13.46″E), which regularly receive large inputs of wrack during the late autumn-early spring. On the beach in each month, five replicate wrack samples were collected from a 0.25 m2 quadrat, while five replicate samples from the surf zone were collected using a hand-held scoop net with a 23 × 20 cm opening and 1 mm mesh pulled through the water column from bottom to top. Wrack samples from both zones were rinsed with seawater to remove sand, other debris and associated fauna, and then spun in a salad spinner for 3–4 cycles to remove excess water and loose epibionts. Kelp (E. radiata) was removed and weighed to provide fresh weight (g) per m2. Additional kelp (n = 5) was collected randomly along the two zones, and 1.0 g (wet weight) of kelp from each sample was placed in a sterile 15 ml centrifuge tube containing 9 ml of 0.22 μm filtered seawater. Bacterial abundance was then determined using the same procedures described for the experiment (see above).
Data Analysis
Since amphipods within each replicate container were not independent, data were pooled for each replicate for each type/treatment combination. Amphipod growth rate was calculated as the difference in length over the time period of the experiment (average final length minus the average length of the sub-sample of amphipods at the beginning of the experiment). Since two-way Analysis of Variance (ANOVA) showed no difference between the initial lengths in the different wrack “type” and “treatment” combinations, an average across all replicates for all wrack age and treatments was used to determine the initial lengths of amphipods for growth data at the end of the experiment. Due to the high degree of variability in the survival of amphipods among aquaria across treatments and kelp age, the percentage of ovigerous females to total females was calculated. The number of eggs per female was based on the ovigerous females that were present at the end of the experiment. A two-way ANOVA was conducted to test for effects of Type (fresh vs aged) and Treatment (antibiotic treated vs untreated) on the growth rate of male and female amphipods, the number of eggs per female, and the proportion of brooding females to all females. Since an initial three-way ANOVA on bacterial abundance, kelp tissue toughness, and kelp tissue C and N content and C:N ratios showed no time effect, a series of two-way ANOVAs was conducted to determine the effects of Type (fixed; fresh vs aged) and Treatment (fixed; antibiotic treated vs control) on these variables. While not presented in the results, a two-way ANOVA on survival of amphipods indicated no treatment (p = 0.96), type (p = 0.12), or interactive effect between these factors (p = 0.30), indicating that survival does not affect the results of the experiment. Data were tested using Shapiro–Wilk’s test (p > 0.05) and a visual inspection of the normal Q-Q plots for normality, and Levene’s test for homogeneity of variance. Data not meeting the assumptions were log10 or square-root transformed, and if they were still not met, alpha was set at 0.01 (Underwood, 1997). All analyses were carried out using SPSS version 22 software (SPSS Inc., Chicago, IL, United States).
Results
Experimental Bacterial Abundances and Kelp Characteristics
Bacterial abundances were higher on control vs treated kelp, regardless of age of kelp, but the abundances in both treatments were far higher on aged than on fresh kelp (Table 1 and Figure 2). For aged kelp, the average bacterial abundance was 5.4 × 106 on treated kelp compared to 39 × 106 cells g FW kelp–1 on untreated (control) kelp. For fresh kelp, bacterial abundance was 0.4 × 106 cells g FW kelp–1 on treated kelp compared to 13 × 106 cells g FW kelp–1 on untreated kelp (Figure 2). There was visibly more biofilm on control vs treated kelp for both fresh and aged kelp in the experiment (see Figures 1C,D for examples of control aged and treated kelp, respectively).
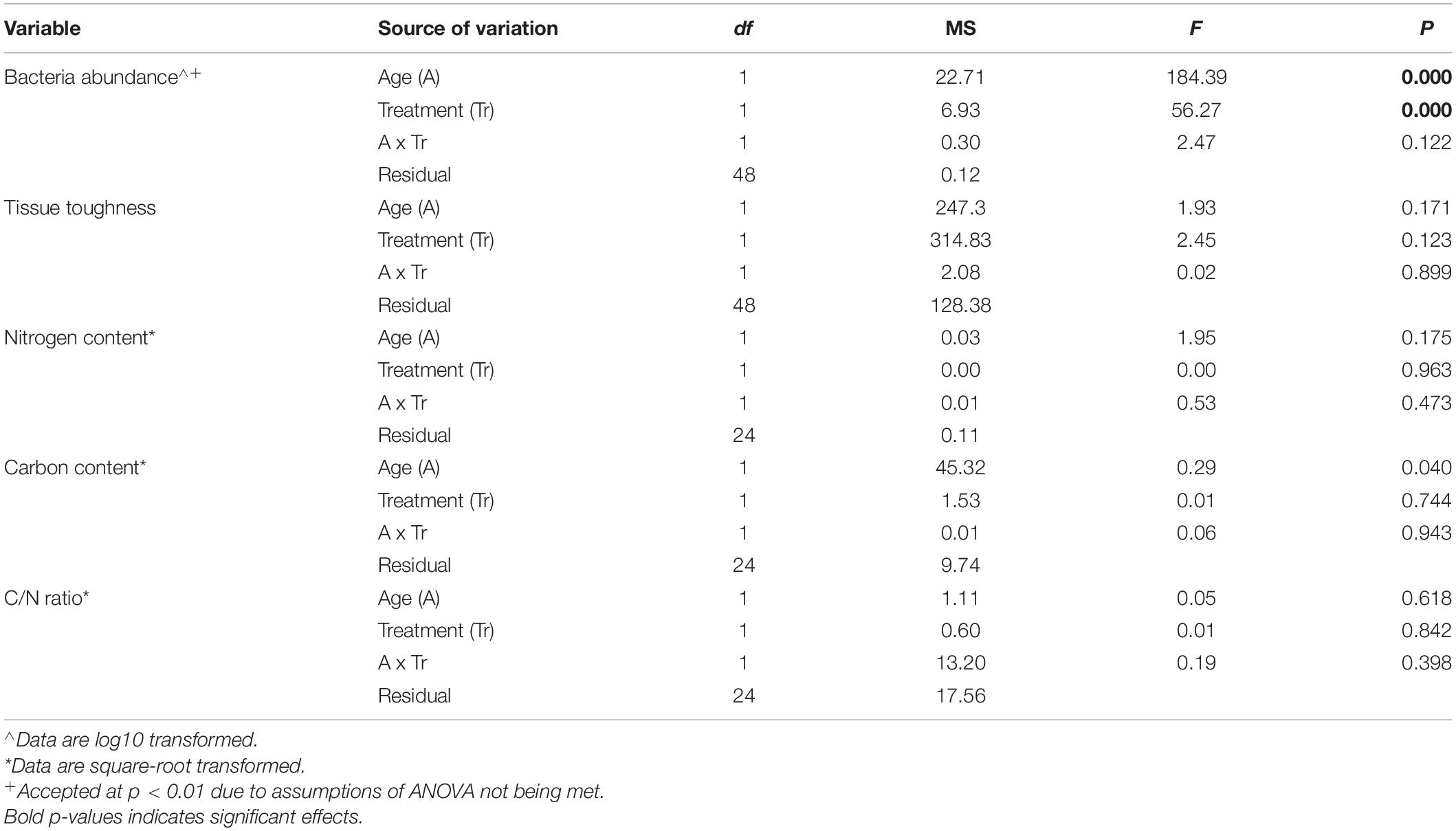
Table 1. Results of two-way ANOVA examining the effects of wrack type (Fresh kelp and 2 weeks Aged kelp) and treatment (Control and Antibiotic treatment) on heterotrophic bacterial abundances, and tissue toughness, nitrogen content (%N), carbon content (%C), and C/N ratio of kelp tissue.
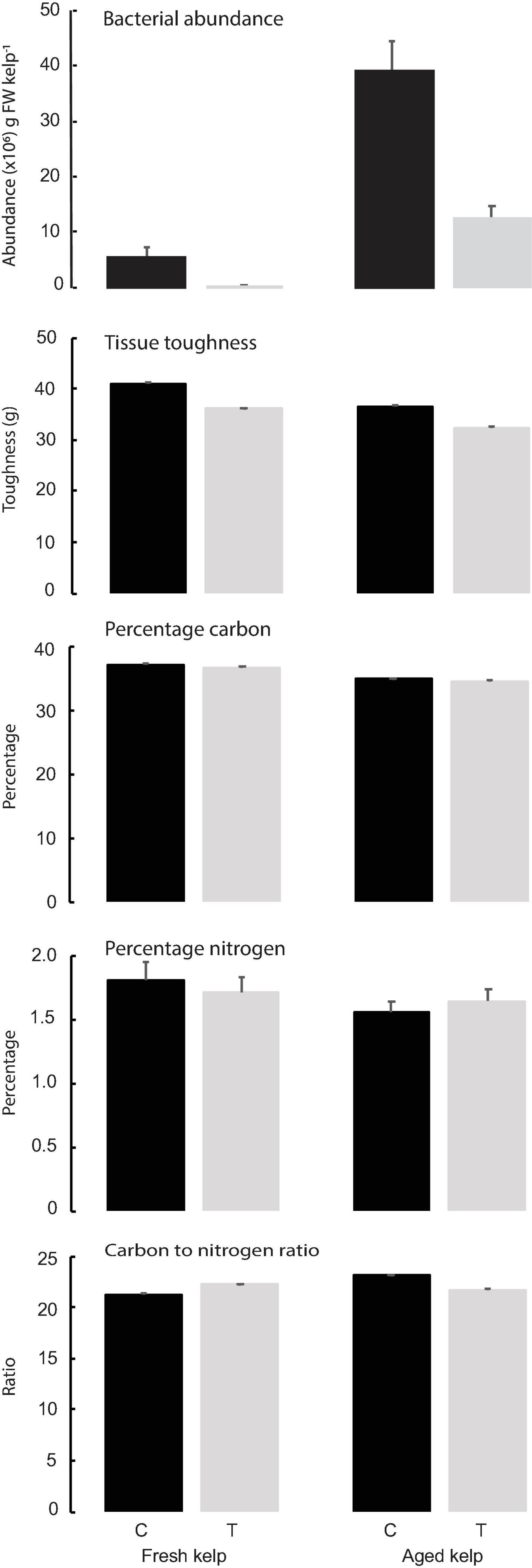
Figure 2. Mean (±SE) bacterial abundance (×106 cells gFW–1) on kelp, kelp tissue toughness (g, n = 15), nitrogen content, carbon content, and C:N ratio (n = 9) of kelp tissue in different kelp type (Fresh and 2 weeks Aged kelp) and treatment [Control (C) and Antibiotic treatment (T)]. Data for different times (Weeks 1, 4, and 8) were pooled since ANOVA showed no time effects.
Tissue toughness did not differ between treatments (control vs antibiotic) or age (fresh vs aged kelp (Table 1 and Figure 2). Similarly, there was no significant effect of kelp age or treatment on nitrogen (N) and carbon (C) content or C:N ratio of kelp tissue, and these kelp characteristics did not change over the course of the experiment (Table 1 and Figure 2).
Growth Rates and Reproduction of Amphipods
For female amphipods, growth rates were greater in treatments containing bacteria than those where bacteria had been reduced, regardless of kelp age (Table 2 and Figure 3). For males, there was a significant interactive effect of age x treatment on growth rate. While growth rates were higher in the control than in the treatment, the effect was greater in aged than fresh kelp. Growth rates for females were ∼0.08 mm⋅d–1 to ∼0.05–0.06 mm⋅d–1 in control and treatments, respectively, while those for males were 0.11–0.13 mm⋅d–1 in kelp with bacteria compared to ∼0.08 mm⋅d–1 in kelp with reduced bacteria (Figure 3).
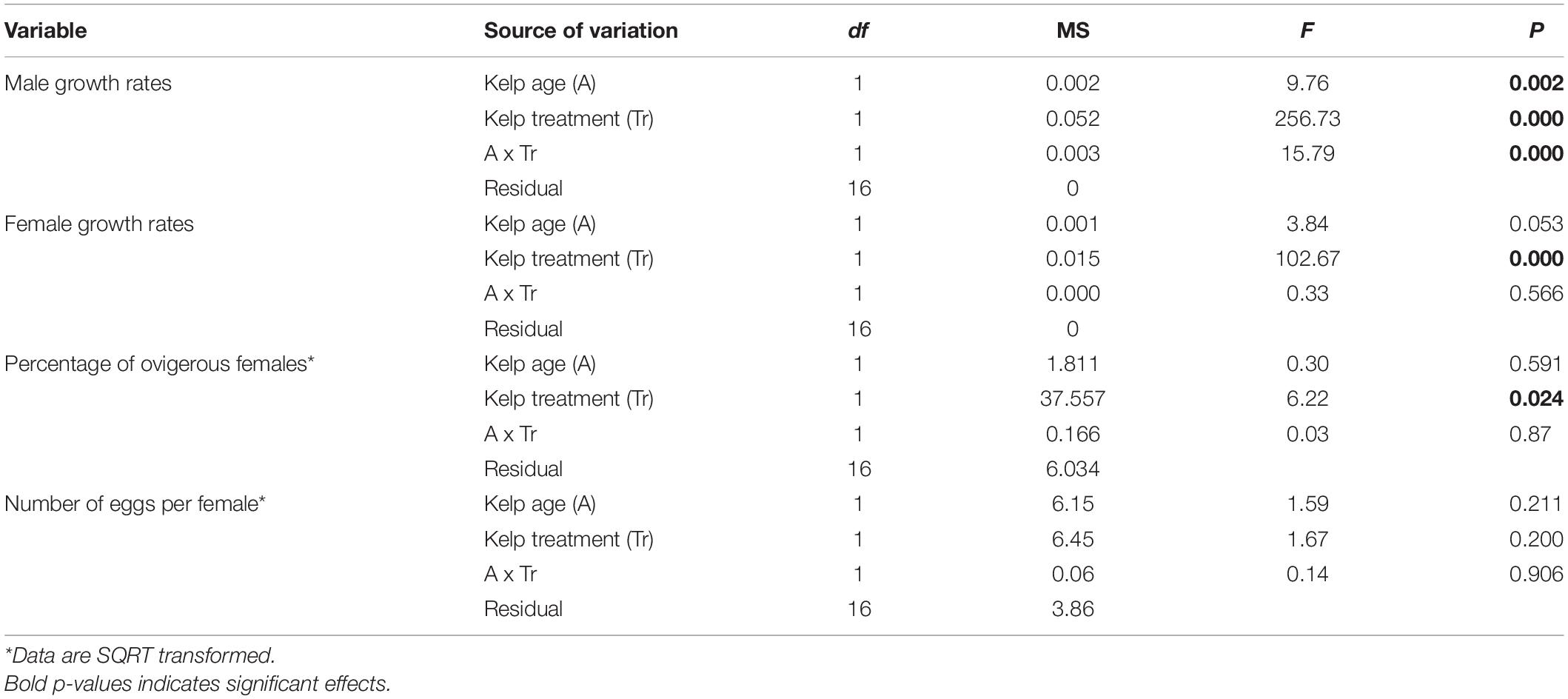
Table 2. Result of two-way ANOVA testing the effect of Treatment (Control and Antibiotic treatment) and Age of kelp (Fresh and Aged kelp) on growth rate of male and female amphipods, percentage of ovigerous female amphipods, and number of eggs per total female amphipods.
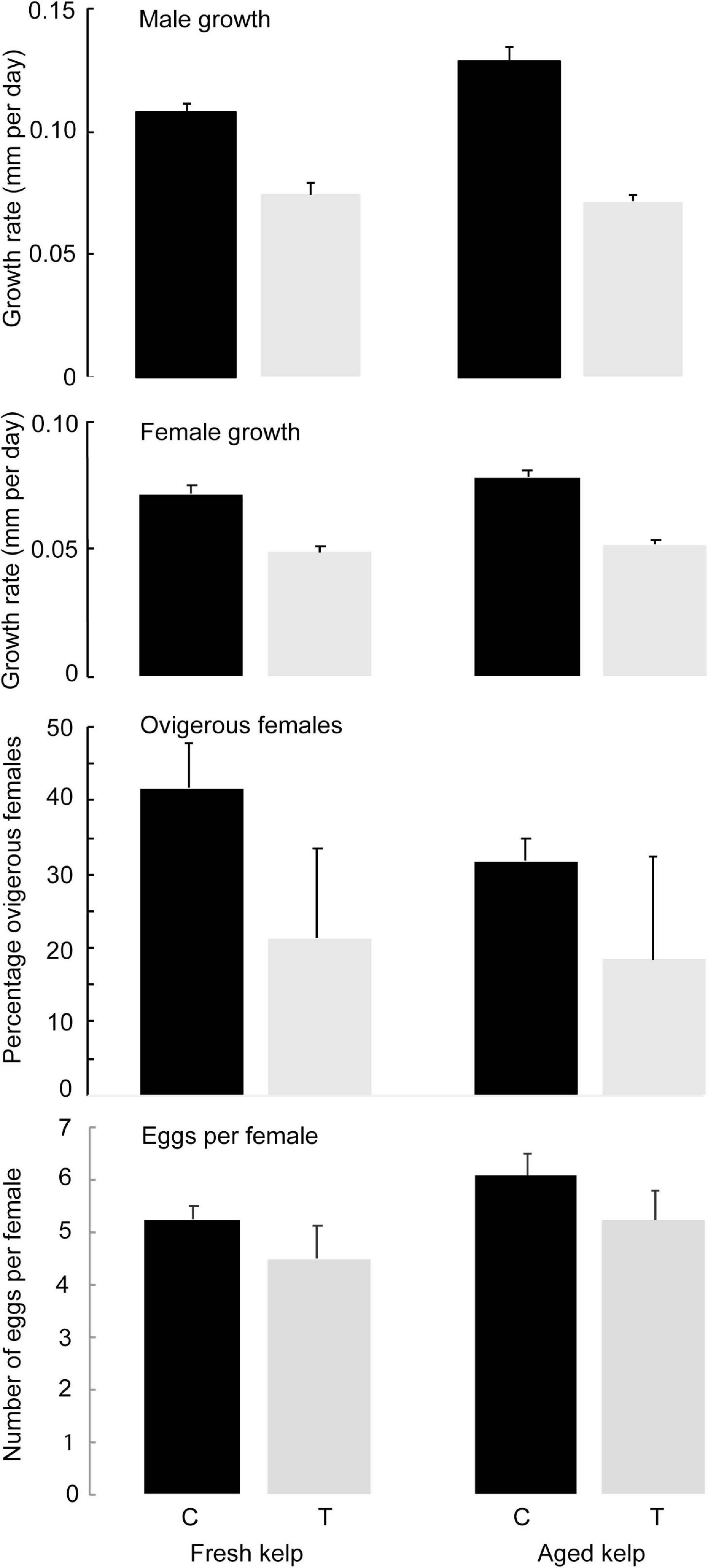
Figure 3. Mean (±SE, n = 5) growth rates of male and female amphipods (mm/day), and percentage of ovigerous female and number of eggs per female amphipods in different wrack types (Fresh and 2 weeks Aged kelp) and treatment [Control (C) and Antibiotic treatment (T)].
The percentage of ovigerous females was about twice as high for treatments containing kelp with bacteria (31 and 41% in fresh and aged kelp, respectively) compared with antibiotic treated kelp where the bacterial abundance had been reduced (18 and 21%, respectively; Table 1 and Figure 3), indicating that females became ovigerous more rapidly on control kelp that contained more bacteria. Indeed, females in two of the five replicate aquaria for both fresh and aged kelp that had been treated with antibiotics did not become ovigerous. For those females that had become ovigerous, the number of eggs per female did not differ between control and treatment and age of kelp (Table 1), with mean values ranging from 4.5 to 6.1 eggs per female (Figure 3).
Kelp Biomass and Bacterial Abundance in Shoreline Wrack
The biomass of kelp in the wrack on beaches and in the surf zone ranged between 111 and 978 g FW kelp m–2 and 52 and 393 g FW kelp m–2, respectively (Table 3). The abundances of heterotrophic bacteria on kelp ranged from 0.7 × 106 to 16.0 × 106 cells⋅g FW kelp–1 and from 0.9 × 106 to 39.1 × 106 cells⋅g FW kelp–1 in the respective habitats (Table 3).

Table 3. Average (±SE) bacterial abundance on kelp (Ecklonia radiata) and kelp biomass on the beach and in the surf zone over three times when wrack is abundant in sandy beach ecosystems in south-western Australia.
Discussion
We show that bacteria in the biofilm of the kelp E. radiata enhance both the growth rate and the reproductive output of the amphipod A. compressa fed on fresh and aged kelp in a lab experiment mimicking a detrital-based, shoreline food web. Shoreline ecosystems containing wrack are recognized as hotspots for decomposition, which is clearly linked to wrack-associated bacteria (Koop et al., 1982; Inglis, 1989). However, with the exception of the likely reliance of the seaweed flies (Coelopa spp.) on wrack-associated microbes (e.g., Cullen et al., 1987), we know surprisingly little about the role of these microbes in enhancing secondary production in shoreline systems, and marine systems as a whole. Indeed, while microbes have been proposed as the “hidden” trophic step in detrital food webs in terrestrial and freshwater ecosystems, their bottom-up effects on consumers are poorly understood even in those ecosystems (Mancinelli and Mulder, 2015). Here, we argue that bacteria also form a “hidden” trophic step in wrack along the shoreline in coastal, marine environments, and such a role could also be important in other coastal, marine ecosystems.
Our results clearly show that bacteria are abundant on kelp (39 × 106 cells g FW kelp–1 on untreated kelp) and these bacteria appear to enhance the fitness of amphipods in this detrital pathway, as evidenced by increasing their growth rates by ∼33% for females and 50% for males and doubling the proportion of ovigorous females over the course of the experiment. Amphipods are typically the dominant detritivore that consume wrack along sandy beaches and surf zones around the world (Colombini and Chelazzi, 2003). In our study region, the amphipod A. compressa is highly abundant and its strong preference for kelp, particularly aged kelp, over other macrophytes leads to high feeding rates on this detrital material (Figure 1B, Robertson and Lucas, 1983; Crawley and Hyndes, 2007). While Robertson and Lucas (1983) showed that A. compressa had higher grazing rates and higher numbers of ovigerous females when fed on decomposed kelp, they suggested that bacterial abundances on the kelp were relatively low. In contrast, we show that bacteria are likely to play an important role in enhancing the fitness of this amphipod. Our results are unlikely to be influenced by an indirect effect of the antibiotic treatment since factors that affect grazing such as carbon and nitrogen content and ratios as well as tissue toughness (Duffy and Hay, 1991; Pennings et al., 2000; Taylor and Steinberg, 2005) did not differ between treatments. Similarly, the antibiotic treatment procedure we used here was previously shown to have no influence on a key chemically-mediated interaction (larval settlement) on macroalga (Huggett et al., 2006). Kelp treated with antibiotics had less than one-third the abundance of bacteria than untreated kelp. We therefore argue that the increased growth rates of both male and female amphipods, and the more rapid development of ovigerous females, in controls reflect the higher abundances of bacteria. This is further supported by a ∼20% and ∼10% increase in growth rates of male and female amphipods, respectively, when fed on aged kelp characterized by high abundances of bacteria vs fresh kelp with lower abundances of bacteria. The more rapid reproductive activity of females, as shown through the percentage of ovigerous females in the presence of higher bacterial abundances, almost certainly related to the increased growth rates of amphipods on kelp with increased bacteria load, since the timing of sexual maturity is influenced by size (Longo and Mancinelli, 2014). While the fecundity of amphipods did not differ between treatments, the more rapid development of reproductively active (ovigerous) females would lead to increased reproductive output of A. compressa. The increased growth rates and fecundity of amphipods would result in a faster population growth rate that could rapidly take advantage of the large inputs of detrital kelp into the shoreline ecosystem. Furthermore, since A. compressa forms the major food source for juvenile fish that use shoreline wrack as a nursery habitat (Crawley et al., 2006), the kelp-associated bacteria would facilitate the transfer of kelp-derived nutrients through the food web (Crawley et al., 2009). In addition, the enhanced population growth of amphipods would increase food availability for those juvenile fish, allowing more fish to occupy the wrack and/or enhance their growth rates, and ultimately lead to an increase in biomass of fish migrating into their sub-adult and spawning habitats in more offshore, coastal ecosystems.
The ability of amphipods to process macrophytes is influenced by food quality (see Duffy and Hay, 1991; Taylor and Steinberg, 2005). In both subtidal and shoreline systems, these mesograzers often prefer brown algae over other macrophytes and prefer some species of brown algae over others (Pennings et al., 2000; Taylor and Steinberg, 2005; Crawley and Hyndes, 2007; Lastra et al., 2008; Duarte et al., 2010), although those preferences may not relate to food quality (Pennings et al., 2000; Taylor and Steinberg, 2005). For example, Pennings et al. (2000) demonstrated that feeding patterns of mesograzers were not related to palatability and quality of algal species, but mesograzers displayed preferences for those same algal species when cast on the beach, despite tissue toughness being higher and nitrogen content being lower in beach-cast material. In terms of living macrophytes, palatability and food quality can influence mesograzer fitness (Duffy and Hay, 1991), and higher nitrogen and protein content has been shown to support faster growth rates in grazers (Barile et al., 2004). However, palatability and food quality did not appear to account for the differences in growth and reproductive output of A. compressa fed on kelp with natural or reduced bacterial abundances since tissue toughness and nutrient content did not differ across these treatments. Compensatory feeding may overcome the consequences of poor food quality on fitness traits such as survival, reproductive output and growth (Cruz-Rivera and Hay, 2001). While we observed grazed areas of kelp in our experiments (Figure 1) that supports direct grazing on kelp and possible compensatory feeding by amphipods, as also shown in previous feeding experiments for this food source and grazer (Crawley and Hyndes, 2007), we argue that A. compressa also feeds directly on kelp-associated bacteria based on the observed grazing marks in the biofilm of kelp (Figure 1).
In terrestrial and freshwater ecosystems, microbes can play a dual role in detrital food webs by enhancing the quality and palatability of detritus for consumers, or competing with consumers for nutrients (Mancinelli and Mulder, 2015). In saltmarsh systems, grazers preferentially feed on areas of leaves with high microbial densities or areas that have been conditioned by microbes (Zimmer et al., 2002; Silliman and Newell, 2003). Microbial activity can improve the food attractiveness and quality of detritus (Zimmer and Topp, 1997; Ihnen and Zimmer, 2008; Filipiak and Weiner, 2014), or supply nutrients through direct consumption by foragers (Thompson et al., 1999). Indeed, microbial activity beyond the 2-week “aging” period for kelp in our experiment would likely alter the integrity of the kelp and increase its direct consumption by amphipods as the kelp decomposes. Sosik and Simenstad (2013) hypothesized that biofilm absorbs organic compounds from the decomposing host that would otherwise be lost to the external environment, which could explain the lack of, or limited shift, in C:N ratios and C and N content in aged vs fresh kelp seen in our study. The low C:N ratios of 6:1 for bacteria (Fukuda et al., 1998), compared to approximately 22:1 for fresh and aged kelp in this study, would provide a more nitrogen rich source than the alga itself. Similarly, the high levels of lipids and poly-unsaturated fatty acids in bacteria (De Carvalho and Caramujo, 2012) would enhance nutrient supply to those grazers. Biofilm has high levels of proteins and lipids (Fernandes Da Silva et al., 2008), and increased biofilm load on leaves increases reproductive success and growth of isopods in forest systems (Zimmer and Topp, 1997; Horváthová et al., 2016). Thus, kelp-associated bacteria are likely to provide a more nutrient rich food source for A. compressa in the shoreline system that would enhance this amphipod’s fitness.
In our study region, kelp is released from the canopy at 6.6 kg⋅m–2⋅year–1 (de Bettignies et al., 2013), compared to the global average 1.16 kg m–1⋅year–1 (Krumhansl and Scheibling, 2012), with large quantities transported to the shore in our region and across the globe (Kirkman and Kendrick, 1997; Hyndes et al., 2014). Here, we show that bacteria in the biofilm of E. radiata tripled to 39 × 106 cells⋅g FW kelp–1 within 2 weeks of harvesting, which represents the detachment of thalli from the reef and movement to the shoreline, and reflected the upper range of bacterial abundances we recorded in the surf zone (0.9 × 106 to 39.1 × 106 cells⋅g FW kelp–1). Similarly, Koop and Griffiths (1982) showed that bacterial abundances on freshly collected Ecklonia maxima in South Africa increased rapidly over 8 days. Given that we placed kelp sections in filtered and sterilized seawater, the increase in bacterial abundance likely reflects the growth of existing colonies. The decomposition of kelp occurs through the concomitant lysis of epidermal cells and bacterial colonization, with an estimated 90% of the leachates being utilized by bacteria and 23–27% of the carbon being converted to bacterial biomass from E. maxima on South African shores (Koop et al., 1982). Bacteria are key decomposers of macrophytes in sandy beach ecosystems, where they are strongly linked to the high production of nutrients and metabolism (Rodil et al., 2019; van Erk et al., 2020). This activity forms biochemical hotspots (Coupland et al., 2007; Dugan et al., 2011; Rodil et al., 2019) that provide an important coastal example of biochemical hotspots at the interface between aquatic and terrestrial systems (McClain et al., 2003). But our study suggests that bacteria in these hotspots also directly influence the food web via the consumption of bacteria by amphipods, forming hotspots of secondary production.
Similar to shoreline systems, detrital kelp has been shown to accumulate and contribute to secondary production in other coastal systems, such as the kelp forests themselves (Vanderklift and Kendrick, 2005), adjacent seagrass meadows (Hyndes et al., 2012; Cartraud et al., 2021), and deep habitats (Dethier et al., 2014). Thus, kelp-associated bacteria could play a similar role in facilitating the transfer of nutrients into other coastal food webs. Indeed, Tarquinio et al. (2018) showed that heterotrophic microbes facilitate the uptake of kelp-derived nitrogen into seagrass ecosystems, supporting the mechanistic role that microbes play in trophic subsidies that enhance biodiversity and productivity in ecosystems (Säwström et al., 2016). However, this role is likely to vary across kelp species due to variability in microbial abundances and decomposition across kelp species (Sosik and Simenstad, 2013; Dethier et al., 2014). The need for further research on microbe-detritivore/grazer interactions is highlighted by the link between disease on marine macrophytes and ocean warming (e.g., Campbell et al., 2011), and disease-mediated grazing on macroalgae (Campbell et al., 2014). This is particularly pertinent for the major habitat-forming kelp, which is being lost at an alarming rate (Krumhansl et al., 2016), and whose epibiont communities and condition are affected by ocean warming and acidification (Qiu et al., 2019).
Data Availability Statement
The data have now been provided in the ECU data repository. The link to the data is: http://dx.doi.org/10.25958/59z2-mk18.
Author Contributions
CSi, GH, PL, CSä, and MH conceived the ideas and designed the methodology. CSi collected and analyzed the data. CSi and GH led the writing of the manuscript, while PL, CSä, and MH reviewed and edited the manuscript. All authors contributed to the article and approved the submitted version.
Funding
CSi was supported by an ECU Postgraduate Research Scholarship, a scholarship from the Western Australian Marine Sciences Institution, and field support grants from the School of Science, ECU.
Conflict of Interest
The authors declare that the research was conducted in the absence of any commercial or financial relationships that could be construed as a potential conflict of interest.
Acknowledgments
We thank Rob Czarnik, James Bongiovanni, Roisin McCallum, Mark Bannister, Pierre Bouvais, Simon Collins, Jody Harris-Walker, Clay Miller, Charlie Phelps, Caitlin Rae, Federico Vitelli, and Simone Strydom for advice and help with the aquaria and laboratory work.
References
Barile, P. J., Lapointe, B. E., and Capo, T. R. (2004). Dietary nitrogen availability in macroalgae enhances growth of the sea hare Aplysia californica (Opisthobranchia: Anaspidea). J. Exp. Mar. Biol. Ecol. 303, 65–78. doi: 10.1016/j.jembe.2003.11.004
Buesing, N., and Gessner, M. O. (2002). Comparison of detachment procedures for direct counts of bacteria associated with sediment particles plant litter and epiphytic biofilms. Aqua. Microbial. Ecol. 27, 29–36. doi: 10.3354/ame027029
Campbell, A. H., Harder, T., Nielsen, S., Kjelleberg, S., and Steinberg, P. D. (2011). Climate change and disease: Bleaching of a chemically defended seaweed. Glob. Change Biol. 17, 2958–2970. doi: 10.1111/j.1365-2486.2011.02456.x
Campbell, A. H., Vergés, A., and Steinberg, P. D. (2014). Demographic consequences of disease in a habitat-forming seaweed and impacts on interactions between natural enemies. Ecology 95, 142–152. doi: 10.1890/13-0213.1
Cartraud, A. E., Lavery, P. S., Rae, C. M., and Hyndes, G. A. (2021). The role of allochthonous kelp in providing spatial subsidies in seagrass meadows. Estuar. Coasts 44, 468–480. doi: 10.1007/s12237-020-00860-8
Colombini, I., and Chelazzi, L. (2003). Influence of marine allochthonous input on sandy beach communities. Oceanogr. Mar. Biol. 41, 115–159.
Coupland, G. T., Duarte, C. M., and Walker, D. I. (2007). High metabolic rates in beach cast communities. Ecosystems 10, 1341–1350. doi: 10.1007/s10021-007-9102-3
Crawley, K. R., and Hyndes, G. A. (2007). The role of different types of detached macrophytes in the food and habitat choice of a surf-zone inhabiting amphipod. Mar. Biol. 151, 1433–1443. doi: 10.1007/s00227-006-0581-0
Crawley, K. R., Hyndes, G. A., and Ayvazian, S. G. (2006). Influence of different volumes and types of detached macrophytes on fish community structure in surf zones of sandy beaches. Mar. Ecol. Prog. Ser. 307, 233–246. doi: 10.3354/meps307233
Crawley, K. R., Hyndes, G. A., Vanderklift, M. A., Revill, A. T., and Nichols, P. D. (2009). Allochthonous brown algae are the primary food source for consumers in a temperate, coastal environment. Mar. Ecol. Prog. Ser. 376, 33–44. doi: 10.3354/meps07810
Crowther, T. W., and Grossart, H.-P. (2015). “The role of bottom-up and top-down interactions in determining microbial and fungal diversity and function,” in Trophic Ecology: Bottom-Up and Top-Down Interactions Across Aquatic and Terrestrial Systems, eds T. C. Hanley and K. J. La Pierre (Cambridge: Cambridge University Press), 260–287. doi: 10.1017/cbo9781139924856.011
Cruz-Rivera, E., and Hay, M. E. (2001). Macroalgal traits and the feeding and fitness of an herbivorous amphipod: The roles of selectivity, mixing, and compensation. Mar. Ecol. Prog. Ser. 218, 249–266. doi: 10.3354/meps218249
Cullen, S. J., Young, A. M., and Day, T. H. (1987). Dietary requirements of seaweed flies (Coelopa frigida). Estuar. Coast. Shelf Sci. 24, 701–710. doi: 10.1016/0272-7714(87)90108-9
de Bettignies, T., Wernberg, T., Lavery, P. S., Vanderklift, M. A., and Mohring, M. B. (2013). Contrasting mechanisms of dislodgement and erosion contribute to production of kelp detritus. Limnol. Oceanogr. 58, 1680–1688. doi: 10.4319/lo.2013.58.5.1680
De Carvalho, C. C. C. R., and Caramujo, M. J. (2012). Lipids of prokaryotic origin at the base of marine food webs. Mar. Drug. 10, 2698–2714. doi: 10.3390/md10122698
Dethier, M. N., Brown, A. S., Burgess, S., Eisenlord, M. E., Galloway, A. W. E., Kimber, J., et al. (2014). Degrading detritus: changes in food quality of aging kelp tissue varies with species. J. Exp. Mar. Bio. Ecol. 460, 72–79. doi: 10.1016/j.jembe.2014.06.010
Douglas, A. E. (2018). What will it take to understand the ecology of symbiotic microorganisms? Environ. Microbiol. 20, 1920–1924. doi: 10.1111/1462-2920.14123
Duarte, C., Navarro, J. M., Acuña, K., and Gómez, I. (2010). Feeding preferences of the sandhopper Orchestoidea tuberculata: the importance of algal traits. Hydrobiologia 651, 291–303. doi: 10.1007/s10750-010-0309-5
Duffy, J. E., and Hay, M. E. (1991). Food and shelter as determinants of food choice by an herbivorous marine amphipod. Ecology 72, 1286–1298. doi: 10.2307/1941102
Dugan, J. E., Hubbard, D. M., Page, H. M., and Schimel, J. P. (2011). Marine macrophyte wrack inputs and dissolved nutrients in beach sands. Estuar. Coast. 34, 839–850. doi: 10.1007/s12237-011-9375-9
Egan, S., Harder, T., Burke, C., Steinberg, P., Kjelleberg, S., and Thomas, T. (2013). The seaweed holobiont: Understanding seaweed-bacteria interactions. FEMS Microbiol. Rev. 37, 462–476. doi: 10.1111/1574-6976.12011
Fernandes Da Silva, C., Ballester, E., Monserrat, J., Geracitano, L., Wasielesky, W. Jr., and Abreu, P. C. (2008). Contribution of microorganisms to the biofilm nutritional quality: Protein and lipid contents. Aquacult. Nut. 14, 507–514. doi: 10.1111/j.1365-2095.2007.00556.x
Filipiak, M., and Weiner, J. (2014). How to make a beetle out of wood: Multi-elemental stoichiometry of wood decay, xylophagy and fungivory. PLoS One 9:e115104. doi: 10.1371/journal.pone.0115104
Fukuda, R., Ogawa, H., Nagata, T., and Koike, I. (1998). Direct determination of carbon and nitrogen contents of natural bacterial assemblages in marine environments. Appl. Environ. Microbiol. 64, 3352–3358. doi: 10.1128/aem.64.9.3352-3358.1998
Heck, K. L. Jr., Carruthers, T. J. B., Duarte, C. M., Hughes, A. R., Kendrick, G., Orth, R. J., et al. (2008). Trophic transfers from seagrass meadows subsidize diverse marine and terrestrial consumers. Ecosystems 11, 1198–1210. doi: 10.1007/s10021-008-9155-y
Horváthová, T., Babik, W., and Bauchinger, U. (2016). Biofilm feeding: Microbial colonization of food promotes the growth of a detritivorous arthropod. ZooKeys 577, 25–41. doi: 10.3897/zookeys.577.6149
Huggett, M. J., Williamson, J. E., De Nys, R., Kjelleberg, S., and Steinberg, P. D. (2006). Larval settlement of the common Australian sea urchin Heliocidaris erythrogramma in response to bacteria from the surface of coralline algae. Oecologia 149, 604–619. doi: 10.1007/s00442-006-0470-8
Hyndes, G. A., Lavery, P. S., and Doropoulos, C. (2012). Dual processes for cross-boundary subsidies: Incorporation of nutrients from reef-derived kelp into a seagrass ecosystem. Mar. Ecol. Prog. Ser. 445, 97–107. doi: 10.3354/meps09367
Hyndes, G. A., Nagelkerken, I., Mcleod, R. J., Connolly, R. M., Lavery, P. S., and Vanderklift, M. A. (2014). Mechanisms and ecological role of carbon transfer within coastal seascapes. Biol. Rev. 89, 232–254. doi: 10.1111/brv.12055
Ihnen, K., and Zimmer, M. (2008). Selective consumption and digestion of litter microbes by Porcellio scaber (Isopoda: Oniscidea). Pedobiologia 51, 335–342. doi: 10.1016/j.pedobi.2007.06.001
Ince, R., Hyndes, G. A., Lavery, P. S., and Vanderklift, M. A. (2007). Marine macrophytes directly enhance abundances of sandy beach fauna through provision of food and habitat. Estuar. Coast. Shelf Sci. 74, 77–86. doi: 10.1016/j.ecss.2007.03.029
Inglis, G. (1989). The colonisation and degradation of stranded Macrocystis pyrifera (L.) C. Ag. by the macrofauna of a New Zealand sandy beach. J. Exp. Mar. Biol. Ecol. 125, 203–217. doi: 10.1016/0022-0981(89)90097-x
Kientz, B., Thabard, M., Cragg, S. M., Pope, J., and Hellio, C. (2011). A new method for removing microflora from macroalgal surfaces: An important step for natural product discovery. Bot. Mar. 54, 457–469.
Kirkman, H., and Kendrick, G. A. (1997). Ecological significance and commercial harvesting of drifting and beach-cast macro-algae and seagrasses in Australia: A review. J. Appl. Phycol. 9, 311–326.
Koop, K., and Griffiths, C. L. (1982). The relative significance of bacteria, meio- and macrofauna on an exposed sandy beach. Mar. Biol. 66, 295–300. doi: 10.1007/bf00397035
Koop, K., Newell, R., and Lucas, M. (1982). Biodegradation and carbon flow based on kelp (Ecklonia maxima) debris in a sandy beach microcosm. Mar. Ecol. Prog. Ser. 7, 315–326. doi: 10.3354/meps007315
Krumhansl, K. A., Okamoto, D. K., Rassweiler, A., Novak, M., Bolton, J. J., Cavanaugh, K. C., et al. (2016). Global patterns of kelp forest change over the past half-century. Proc. Nation. Acad. Sci. USA. 113, 13785–13790.
Krumhansl, K. A., and Scheibling, R. E. (2012). Production and fate of kelp detritus. Mar. Ecol. Prog. Ser. 467, 281–302. doi: 10.3354/meps09940
Lastra, M., Page, H. M., Dugan, J. E., Hubbard, D. M., and Rodil, I. F. (2008). Processing of allochthonous macrophyte subsidies by sandy beach consumers: Estimates of feeding rates and impacts on food resources. Mar. Biol. 154, 163–174. doi: 10.1007/s00227-008-0913-3
Longo, E., and Mancinelli, G. (2014). Size at the onset of maturity (SOM) revealed in length-weight relationships of brackish amphipods and isopods: An information theory approach. Estuar. Coast. Shelf Sci. 136, 119–128. doi: 10.1016/j.ecss.2013.11.013
Mancinelli, G., and Mulder, C. (2015). Detrital dynamics and cascading effects on supporting ecosystem services. Adv. Ecol. Res. 53, 97–160. doi: 10.1016/bs.aecr.2015.10.001
Marie, D., Partensky, F., Vaulot, D., and Brussaard, C. (1999). Enumeration of phytoplankton, bacteria, and viruses in marine samples. Cur. Prot. Cytom. 10:11.11.
McClain, M. E., Boyer, E. W., Dent, C. L., Gergel, S. E., Grimm, N. B., Groffman, P. M., et al. (2003). Biogeochemical hotspots and hot moments at the interface of terrestrial and aquatic ecosystems. Ecosystems 6, 310–312.
Pennings, S. C., Carefoot, T. H., Zimmer, M., Danko, J. P., and Ziegler, A. (2000). Feeding preferences of supralittoral isopods and amphipods. Can. J. Zool. 78, 1918–1929. doi: 10.1139/z00-143
Polis, G. A., Anderson, W. B., and Holt, R. D. (1997). Toward an integration of landscape and food web ecology: The dynamics of spatially subsidized food webs. Ann. Rev. Ecol. Syst. 28, 289–316. doi: 10.1146/annurev.ecolsys.28.1.289
Pomeroy, L. R., le Williams, P. J. B., Azam, F., and Hobbie, J. E. (2007). The microbial loop. Oceanography 20, 28–33.
Poore, A. G. B., Campbell, A. H., Coleman, R. A., Edgar, G. J., Jormalainen, V., Reynolds, P. L., et al. (2012). Global patterns in the impact of marine herbivores on benthic primary producers. Ecol. Let. 15, 912–922. doi: 10.1111/j.1461-0248.2012.01804.x
Poore, A. G. B., Graba-Landry, A., Favret, M., Sheppard Brennand, H., Byrne, M., and Dworjanyn, S. A. (2013). Direct and indirect effects of ocean acidification and warming on a marine plant-herbivore interaction. Oecologia 173, 1113–1124. doi: 10.1007/s00442-013-2683-y
Qiu, Z., Coleman, M. A., Provost, E., Campbell, A. H., Kelaher, B. P., Dalton, S. J., et al. (2019). Future climate change is predicted to affect the microbiome and condition of habitat-forming kelp. Proc. R. Soc. B 286:20181887. doi: 10.1098/rspb.2018.1887
Robertson, A. I., and Lucas, J. S. (1983). Food choice, feeding rates, and the turnover of macrophyte biomass by a surf-zone inhabiting amphipod. J. Exp. Mar. Biol. Ecol. 72, 99–124. doi: 10.1016/0022-0981(83)90138-7
Rodil, I. F., Lastra, M., López, J., Mucha, A. P., Fernandes, J. P., Fernandes, S. V., et al. (2019). Sandy beaches as biogeochemical hotspots: the metabolic role of macroalgal wrack on low-productive shores. Ecosystems 22, 49–63. doi: 10.1007/s10021-018-0253-1
Säwström, C., Hyndes, G. A., Eyre, B. D., Huggett, M. J., Fraser, M. W., Lavery, P. S., et al. (2016). Coastal connectivity and spatial subsidy from a microbial perspective. Ecol. Evol. 6, 6662–6671. doi: 10.1002/ece3.2408
Silliman, B. R., and Newell, S. Y. (2003). Fungal farming in a snail. Proc. Nat. Acad. Sci. U.S.A. 100, 15643–15648. doi: 10.1073/pnas.2535227100
Sosik, E. A., and Simenstad, C. A. (2013). Isotopic evidence and consequences of the role of microbes in macroalgae detritus-based food webs. Mar. Ecol. Prog. Ser. 494, 107–119. doi: 10.3354/meps10544
Suárez-Jiménez, R., Hepburn, C., Hyndes, G. A., McLeod, R. J., Taylor, R. B., and Hurd, C. L. (2017). The invasive kelp Undaria pinnatifida hosts an epifaunal assemblage similar to native seaweeds with comparable morphologies. Mar. Ecol. Prog. Ser. 582, 45–55. doi: 10.3354/meps12321
Tarquinio, F., Bourgoure, J., Koenders, A., Laverock, B., Säwström, C., and Hyndes, G. A. (2018). Microorganisms facilitate uptake of dissolved organic nitrogen by seagrass leaves. ISME J. 12, 2796–2800. doi: 10.1038/s41396-018-0218-6
Taylor, R. B., and Steinberg, P. D. (2005). Host use by Australasian seaweed mesograzers in relation to feeding preferences of larger grazers. Ecology 86, 2955–2967. doi: 10.1890/04-1480
Thompson, F. L., Abreu, P. C., and Cavalli, R. (1999). The use of microorganisms as food source for Penaeus paulensis larvae. Aquaculture 174, 139–153. doi: 10.1016/s0044-8486(98)00511-0
Underwood, A. J. (1997). Experiments in Ecology. The Logical Design and Interpretation Using Analysis of Variance. Cambridge: Cambridge University Press.
van Erk, M. R., Meier, D. V., Ferdelman, T., Harder, J., Bussmann, I., and de Beer, D. (2020). Kelp deposition changes mineralization pathways and microbial communities in a sandy beach. Limnol. Oceanogr. 65, 3066–3084. doi: 10.1002/lno.11574
Vanderklift, M. A., and Kendrick, G. A. (2005). Contrasting influence of sea urchins on attached and drift macroalgae. Mar. Ecol. Prog. Ser. 299, 101–110. doi: 10.3354/meps299101
Wahl, M., Goecke, F., Labes, A., Dobretsov, S., and Weinberger, F. (2012). The second skin: Ecological role of epibiotic biofilms on marine organisms. Front. Microbiol. 3:292. doi: 10.3389/fmicb.2012.00292
Weigel, B. L., and Pfister, C. A. (2019). Successional dynamics and seascape-level patterns of microbial communities on the canopy-forming kelps Nereocystis luetkeana and Macrocystis pyrifera. Front. Microbiol. 10:346. doi: 10.3389/fmicb.2019.0034
Zimmer, M., Pennings, S. C., Buck, T. L., and Carefoot, T. H. (2002). Species-specific patterns of litter processing by terrestrial isopods (Isopoda: Oniscidea) in high intertidal salt marshes and coastal forests. Funct. Ecol. 16, 596–607. doi: 10.1046/j.1365-2435.2002.00669.x
Keywords: epibiont bacteria, beach, growth, reproduction, amphipod, detritus, surf zone, grazer
Citation: Singh CL, Huggett MJ, Lavery PS, Säwström C and Hyndes GA (2021) Kelp-Associated Microbes Facilitate Spatial Subsidy in a Detrital-Based Food Web in a Shoreline Ecosystem. Front. Mar. Sci. 8:678222. doi: 10.3389/fmars.2021.678222
Received: 09 March 2021; Accepted: 24 May 2021;
Published: 05 July 2021.
Edited by:
Mariana Mayer Pinto, University of New South Wales, AustraliaReviewed by:
Melanie Bishop, Macquarie University, AustraliaMegan Dethier, University of Washington, United States
Copyright © 2021 Singh, Huggett, Lavery, Säwström and Hyndes. This is an open-access article distributed under the terms of the Creative Commons Attribution License (CC BY). The use, distribution or reproduction in other forums is permitted, provided the original author(s) and the copyright owner(s) are credited and that the original publication in this journal is cited, in accordance with accepted academic practice. No use, distribution or reproduction is permitted which does not comply with these terms.
*Correspondence: Glenn A. Hyndes, Zy5oeW5kZXNAZWN1LmVkdS5hdQ==
†ORCID: Megan J. Huggett, orcid.org/0000-0002-3401-0704; Paul S. Lavery, orcid.org/0000-0001-5162-273X; Christin Säwström, orcid.org/0000-0001-9297-3093; Glenn A. Hyndes, orcid.org/0000-0002-3525-1665