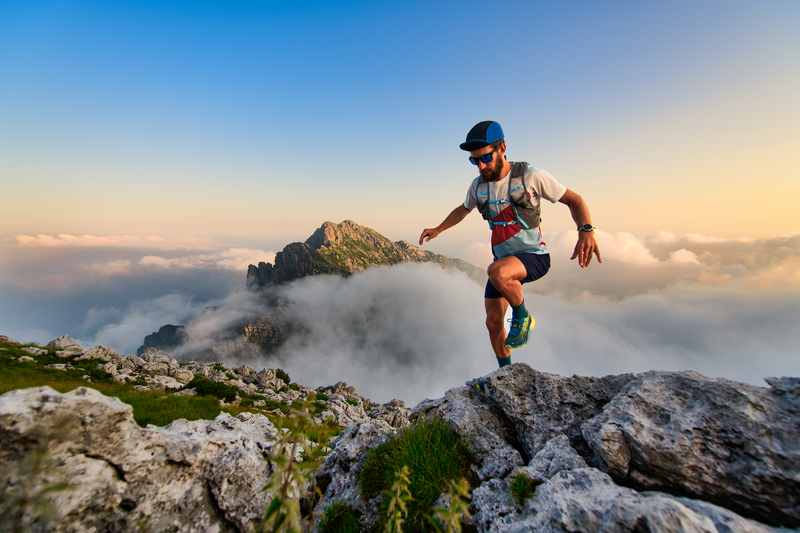
95% of researchers rate our articles as excellent or good
Learn more about the work of our research integrity team to safeguard the quality of each article we publish.
Find out more
ORIGINAL RESEARCH article
Front. Mar. Sci. , 07 June 2021
Sec. Marine Biology
Volume 8 - 2021 | https://doi.org/10.3389/fmars.2021.675807
This article is part of the Research Topic Biological Invasions in the Mediterranean Sea View all 13 articles
Invasive alien species threaten biodiversity and ecosystem structure and functioning, but incomplete assessments of their origins and temporal trends impair our ability to understand the relative importance of different factors driving invasion success. Continuous time-series are needed to assess invasion dynamics, but such data are usually difficult to obtain, especially in the case of small-sized taxa that may remain undetected for several decades. In this study, we show how micropaleontologic analysis of sedimentary cores coupled with radiometric dating can be used to date the first arrival and to reconstruct temporal trends of foraminiferal species, focusing on the alien Amphistegina lobifera and its cryptogenic congener A. lessonii in the Maltese Islands. Our results show that the two species had reached the Central Mediterranean Sea several decades earlier than reported in the literature, with considerable implications for all previous hypotheses of their spreading patterns and rates. By relating the population dynamics of the two foraminifera with trends in sea surface temperature, we document a strong relationship between sea warming and population outbreaks of both species. We conclude that the micropaleontologic approach is a reliable procedure for reconstructing the bioinvasion dynamics of taxa having mineralized remains, and can be added to the toolkit for studying invasions.
Global trade and worldwide transport of people and goods have largely altered the natural distribution of species (e.g., Sardain et al., 2019), but large gaps of knowledge remain in assessing spatial and temporal patterns of invasions, especially in the marine environment (Seebens et al., 2017; Ojaveer et al., 2018). Incomplete assessments of temporal trends, origins and drivers of their spread may affect our ability to understand the ecology and history of communities and the relative importance of different factors driving invasion success (Carlton, 2009). Sporadic monitoring and surveillance, weak taxonomic knowledge, or elusive behavior of some species have left several marine invasions undetected for years, decades or even centuries (Carlton, 2009; Griffiths et al., 2010; Zenetos et al., 2019). Several approaches have been attempted to reconstruct the timing of first introduction events and subsequent stages of invasion: re-examination of old museum or herbarium collections (Ahnelt, 2016; Steen et al., 2017), analysis of published descriptions (Zullo, 1992; Galil et al., 2018), interviews to local fishermen (Bariche et al., 2014; Azzurro et al., 2019), molecular tools (Ordóñez et al., 2016; Deldicq et al., 2019) and radiometric dating (Petersen et al., 1992; Albano et al., 2018). In spite of different approaches utilized, the gap still persists because long and continuous time-series are difficult to obtain, due to the often sporadic and intermittent availability of marine biodiversity data; hence several studies are restricted to inter-decadal comparisons (Parravicini et al., 2015) or are based on population model simulations (Clark et al., 2013; Walsh et al., 2016). Moreover, most of the current knowledge of invasions is from larger organisms, whereas very little is known about spatial and temporal patterns of bioinvasions by microscopic taxa such as foraminifera or other unicellular eukaryotes (Langer et al., 2012; Skarlato et al., 2018; Reavie and Cangelosi, 2020). These small-sized invaders can remain unnoticed for several years after their introduction and gain attention from beach visitors or scientists when population sizes reach a certain threshold and become visible to the naked eye (Guastella et al., 2019).
An example is provided by two algal symbiont-bearing benthic foraminifera: Amphistegina lobifera Larsen, 1976, an Indo-Pacific species that entered the Mediterranean via the Suez Canal (Prazeres et al., 2020), and its congener A. lessonii d’Orbigny, 1826, a species of uncertain native origin. Discussions on the non-indigenous status of A. lobifera and cryptogenic status (sensu Carlton, 2009) of A. lessonii are provided in Guastella et al. (2019). In particular, fossil specimens of A. lobifera have never been found in ancient Mediterranean records, while fossil remains of A. lessonii are common in Mediterranean shallow-water strata, late Pliocene to early Pleistocene in age (Di Bella et al., 2005). Both species are currently abundant in the Red Sea, but only A. lobifera is successfully spreading in the Mediterranean Sea, following a westward colonization process (Langer et al., 2012; Caruso and Cosentino, 2014; Langer and Mouanga, 2016; Guastella et al., 2019). On the contrary, A. lessonii is extremely rare in the Mediterranean Sea, and is mainly characterized by smaller-sized specimens compared to A. lobifera (Guastella et al., 2019). This sporadic occurrence and size range has been attributed to its different sensitivity to colder temperature that may limit test calcification (Titelboim et al., 2019).
Both species commonly proliferate in shallow-waters (<20 m depth) of warm tropical and subtropical areas, and their latitudinal distribution is strongly regulated by sea surface temperatures, with winter minimum temperatures around 18°C (Hallock, 1984; Langer and Hottinger, 2000; Hohenegger, 2004; Renema, 2018). In the Mediterranean Sea, their geographic distribution and population abundance is believed to be primarily controlled by wintry sea surface temperature (winter isotherm 15°C, Hollaus and Hottinger, 1997). However, little is known about Amphistegina spp. populations established in the Central Mediterranean region, which may have been selected for their higher tolerance to colder temperatures, compared to Red Sea and Eastern Mediterranean populations (Schmidt et al., 2016a; Titelboim et al., 2019). Nonetheless, a further spread of amphisteginids into the Western Mediterranean region could be expected in the near future, also promoted by the ongoing sea surface warming (Langer et al., 2013; Guastella et al., 2019). Profound ecological changes have been documented in the Eastern Mediterranean, where A. lobifera populations have become the dominant component of benthic foraminiferal assemblages. Massive deposits of amphisteginids produced an increased accumulation of carbonatic sands, which progressively altered native substrates. This, in turn, reduced biodiversity and caused the displacement of previously established foraminiferal biota (Langer et al., 2012; Mouanga and Langer, 2014).
In this study, we aim to reconstruct the invasion history of A. lobifera and A. lessonii using a multidisciplinary approach based on the analysis of sediment cores by techniques of micropaleontology, paleoecology, and radiometric dating. We document their population abundance along the timeline, by analyzing two sedimentary cores (CORE18 and CORE19) collected from Malta island (Central Mediterranean Sea). Additionally, we relate the temporal dynamics of the two populations with the trends of sea surface temperature (SST) measured in the area in the last 60 years, in order to assess whether sea warming has driven their invasion pattern. Finally, we elaborate hypotheses on the possible future spreading of both species in the Mediterranean Sea.
The Maltese Archipelago is composed of three main islands (Malta, Gozo and Comino) and is located within the Sicily channel in the Central Mediterranean Sea, at a latitude of 35°48′28″–36°05′00″ North and a longitude of 14°11′04″–14°34′37″ East (Figure 1A). The coasts of the archipelago, with a total perimeter of about 271 km, are mainly characterized by high rocky shores; only a few natural bays are present and most of them exhibit high levels of anthropogenic impact (Magri, 2006).
Figure 1. (A) Study area (Central Mediterranean Sea); (B) Current distribution and relative abundance of A. lobifera (in red) and A. lessonii (in green) along the coasts of the Maltese Islands. The pie charts in this panel derived from data available in Guastella et al. (2019) and are supplemented with new data regarding relative abundance of reworked fossil species (in light-blue); (C) Sampling site in Marsamxett Harbor. In the selected site, the relative abundance of A. lobifera and of reworked fossil species are about 40 and 3%, respectively. Additionally, the collection site is close to one site previously studied (Yokeş et al., 2007) (black star). Maps derived from Google Earth (https://www.google.it/intl/it/earth/; data providers: Google Landsat/Copernicus Data SIO, NOAA, U.S. Navy, NGA, GEBCO IBCAO) and modified with GIMP v. 2.10.20 (https://www.gimp.org/).
In order to identify suitable sites for collecting the sediment cores, where the sea-floor could reasonably reflect the pattern of sedimentation that occurred in the past decades and thus the history of colonization of Amphistegina spp. in the area, we complied with the following criteria: (I) occurrence of a well-established population of A. lobifera and subordinately of A. lessonii (Figure 1B); (II) absence of fossil amphisteginids in neighboring outcropping rocks, in order to avoid their reworked occurrence in the studied cores, that may confuse the record of current foraminiferal assemblages of marine sediments. To satisfy requirement II, relative abundance of reworked foraminiferal species present in sediment samples was calculated through the comparison with fossil assemblages preserved in seven rocky samples collected in Malta from Miocene outcrops: three rocky samples were collected from the Globigerina Limestone Formation and prepared as washed residues, and four rocky samples were collected from the Upper Coralline Limestone Formation and prepared as thin rock sections (Figure 1B and Supplementary Table 1 available on-line as Supplementary Data); (III) proximity to one of the four sites of the earliest record of A. lobifera in Malta (Yokeş et al., 2007), in order to have a temporal constraint for the reconstruction of the invasion dynamics; (IV) prevalence of fine-grained sediments, first requirement necessary for 210Pb dating, a widely applied method to date recent sediments and to assess sediment accumulation rates (Tylmann et al., 2016; Andersen, 2017); (V) location within an enclosed bay, to ensure protection from storm waves and littoral currents that would alter the normal sedimentation process. In fact, the presence of an undisturbed sediment deposition is the second requirement to correctly apply 210Pb-chronology (Tylmann et al., 2016); (VI) absence of dredging or beach nourishment operations in the area, for the same reason as above; (VII) depth >10 m to avoid sediment mixing by waves, as above, and <20 m, the maximum depth where A. lobifera has been observed to occur (Hallock, 1984; Hohenegger, 1995; Guastella et al., 2019).
Despite many efforts and surveys to search for multiple suitable sites along the coasts of the Maltese Archipelago, only a single locality satisfied all the stringent conditions listed above: Marsamxett Harbor (35°54′16.7″N; 14°30′27.5″E; Figure 1C). Located near station “4” of Yokeş et al. (2007) and known to have in 2018 a relative abundance of A. lobifera of ∼ 40% (Guastella et al., 2019), this natural bay displays the required condition of sediment grain size, shelter, depth and absence of human activities that could have altered sedimentation on the sea-floor. Furthermore, Amphistegina spp. populations are well established (Guastella et al., 2019) and the rocky outcrops around the selected site are exclusively characterized by rocks belonging to the Globigerina Limestone Formation (Aquitanian-early Langhian in age), formed in outer shelf to upper slope environments (Baldassini and Di Stefano, 2017). Thus, no fossil amphisteginids were recorded and the relative abundance of the other reworked fossil foraminifera (mainly deep-water taxa such as Cibicides pseudoungerianus, Heterolepa bellincionii, Neoeponides schreibersii, Reussella spinulosa, and Spiroplectammina carinata and the planktonic genera Globigerina, Globigerinoides, and Globoquadrina) is only 3% (Figure 1B and Supplementary Table 2 available on-line as Supplementary Data).
Within Marsamxett bay, two sediment cores were collected a few meters away from each other using a hand-corer operated by a scuba diver. The first core (CORE18) was sampled on 8th May 2018 at 16 m depth and the second one (CORE19) was collected on 4th September 2019 at 17 m depth. CORE18 was collected as a “pilot” sample in order to evaluate if all the stringent conditions listed above were respected and if the collected data had a high quality; CORE19 was sampled in order to have a replicate that could confirm the reconstruction of invasion dynamic provided by CORE18.
CORE18 is 41 cm long and CORE19 is 50 cm long, both of them mostly made up by fine-grained sediments (fine sands, silts and clays). After collection, both cores were longitudinally sectioned in two halves and then crosscut at each centimeter, obtaining 41 samples from the first one and 50 samples from the second one, respectively. Sediment samples were used for both grain-size and radiometric analyses and prepared for the micropaleontologic analyses.
Grain-size analysis was carried out for CORE18 in order to verify its compliance with two prerequisites for radiometric dating: fine-grained sediments and undisturbed record (not mixed vertically). Sediment samples were oven-dried at 40°C for 1 day, weighed (up to a maximum of 17 g) and separated by wet sieving using five overlapped sieves (meshes, respectively of 1 mm, 500, 250, 125, and 63 μm) as indicated in Blott and Pye (2012). The fraction retained on each sieve was oven-dried and weighed again, obtaining weight percentage data in the form of cumulative curves (distinguishing between very coarse, coarse, medium, fine and very fine sands and indistinct mud fraction); the weight of the mud fraction (silts plus clays) was calculated by difference of the total weight.
Both cores were chronologically constrained by measuring activities of 210Pb and 137Cs isotopes along the sedimentary record. 210Pb is a natural radionuclide belonging to the 238U decay series and is characterized by a half-life of 22.3 years. The total 210Pb activity in marine sediments has two components: supported 210Pb activity derived from the decay of in situ 226Ra, and unsupported 210Pb activity derived from atmospheric fallout (Kosnik et al., 2015; Andersen, 2017). In marine environments, unsupported 210Pb deposits associated with muddy particles accumulate at the sediment-water interface as excess 210Pb. The dating is based upon the determination of the vertical distribution of unsupported 210Pb, by subtracting supported 210Pb activity from the total activity of 210Pb (Hollins et al., 2011).
In the studied core samples, 210Pb activity was measured at the CNR-ISMAR Institute (Bologna, Italy) via alpha counting of its daughter isotope 210Po, assuming secular equilibrium between the two isotopes as described in Rizzo et al. (2009) and Incarbona et al. (2016). 210Po was extracted from the sediment using hot HNO3 and H2O2, and spiked with 209Po (NIST standard SRM 4326, diluted to 0.43 Bq g–1) used as a yield monitor. After separation of the leachate from the residue, the solution was evaporated to near dryness and the nitric acid was eliminated using concentrated HCl, the residue was then dissolved in 1.5 N HCl and Iron was reduced using ascorbic acid. Finally, Po isotopes were plated onto a silver disk overnight, at room temperature. Repeated measurements of a certain number of sediment samples was carried out to estimate the analytical precision. Sedimentation rates for the last decades were calculated based on the decreasing concentration of excess 210Pb, following the CF:CS model (Constant Flux: Constant Sedimentation) (Robbins, 1978), which assumes that both the atmospheric flux of excess 210Pb to the sediment-water interface and the sediment supply remain constant over time (Abril and Brunskill, 2014).
137Cs is an artificial radionuclide derived from nuclear fission and is commonly used as independent tracer for validation of the 210Pb chronologies (Smith, 2001). Its main sources in the atmosphere were the nuclear weapons testing (peaked in 1963) and in some parts of the northern hemisphere the input from the accident that occurred in Chernobyl in 1986. Dried samples for 137Cs measurements were placed in plastic containers and counted by gamma spectrometry. Gamma emissions of 137Cs were counted at 661.7 keV photo-peak for 24–72 h using Ortec germanium detectors (OrtecHPGeGMX-20195P and GEM-20200) calibrated against a sediment spiked with the Amersham reference standard solution QCY48A by using the same counting geometry. The detectors were coupled to a multi-channel analyzer and shielded by a 10 cm thick layer of lead.
Core samples were oven-dried at 40°C for 1 day, weighed (∼7 g), washed on a 63 μm sieve and then oven-dried again at 40°C for 1 day. Washed residues were separated in discrete aliquots using a precision micro-splitter and finally analyzed at the stereomicroscope for the analysis of foraminiferal content. Since it is quite difficult to morphologically distinguish between the two target species when specimens are small in size (diameter <500 μm; Hohenegger et al., 1999), the counting was limited only to those specimens that clearly displayed distinctive morphological features (following Hottinger et al., 1993) and for which the specific attribution was unambiguous. Specimens were attributed to A. lobifera (Figure 2, images 1–3) when characterized by tests with a more rounded margin, if observed in profile (p), with lobulated sutures (lobes usually visible on both sides) that were bent backwards forming an unbroken arch in dorsal view (d), while they were mainly sigmoidal in ventral view (v). On the contrary, specimens were attributed to A. lessonii (Figure 2, images 4–6) when their tests were: (I) more flattened and always characterized by an angular peripheral margin if observed in profile (p); (II) not as rounded as in similar-sized A. lobifera specimens; (III) with sutures without any folding nor complications, which sharply bent backwards forming a typical falciform arch in dorsal view (d), while they were slightly depressed in the last chambers but always maintaining the typical sickle shape, in ventral view (v).
Figure 2. Photomicrographs of adult and juvenile specimens of Amphistegina lobifera (images 1–3) and Amphistegina lessonii (4–6) collected from cm 2 to 3 bsf of CORE19. v) ventral view; p) profile; d) dorsal view. Note in juvenile specimens of A. lobifera (diameter size <300 μm, images 2–3) the presence of typical lobulated sutures (arrows) and the more rounded profile with respect to juvenile specimens of A. lessonii (5–6) characterized by more flattened tests with falciform sutures without any folding nor complication. Scale bar 300 μm.
The absolute abundances of A. lobifera and A. lessonii were calculated along the cores as number of individuals recorded per gram of dry sediment (N g–1).
Additionally, the absolute abundance of calcareous nannoplankton was calculated in smear-slides as total number of individuals recorded per mm2 and then used as proxy for hydrodynamism. Since nannoplankton deposition occurs only in low-energy systems (particle size <30 μm), this independent proxy was used to qualitatively estimate if the energy at the sampling site was low enough to permit deposition of fine-grained particles and, consequently, of radionuclides used for the radiometric dating.
In order to verify the relation between the abundance of Amphistegina spp. and the water temperature, Sea Surface Temperature (SST) of Sicily Channel was considered. SST was extracted from 3D temperature simulated by the reanalyses of Mediterranean Sea (ref. MEDSEA_REANALYSIS_ PHY_006_009, horizontal spatial resolution ∼ 6 km), available as monthly averages covering the period 1955–2015. The quality of the MEDSEA_REANALYSIS_PHY_006_009 was assessed for the entire period by comparing results with available observations, consolidated climatological products and current knowledge of the ocean circulation. The reanalysis data were simulated by means of the assimilation of in situ temperature profile and sea level anomaly in the Mediterranean basin.
In the framework of this study, the sea temperature was analyzed at 16 m depth in the grid point of the model closest to the sampling site of sediment cores [Lat. 35°56′15″N; Long. 14°30′0″E]. Starting from available data, curves of annual average SST and annual wintry average SST were obtained. The first one was elaborated by calculating the average SST for each year since 1955–2015, and the second one by calculating the average SST from values simulated during the winter season (January, February, and March). The average annual SST and wintry SST were plotted against the absolute abundances of A. lobifera and A. lessonii. In addition, the SST annual and wintry anomalies were computed taking as a reference the SST mean over the whole period and over the winter season, respectively. In particular, the annual anomalies were obtained by subtracting the SST averaged over the period 1955–2015 from each annual mean, while the wintry anomalies were obtained subtracting to each wintry mean the SST averaged over all January, February and March in the 1955–2015 period. All relationships were analyzed with Spearman correlation analysis using the software PAST v4.01 (Hammer et al., 2001). All graphs were generated in Microsoft Excel for Microsoft 365 MSO, and modified with GIMP v.2.10.201.
Granulometric curves obtained from CORE18 (see Figure 1 for map) show that the sample meets both prerequisites for radiometric dating through 210Pb: the mud fraction (particle size <63 μm) is continuously present with discrete abundances between 8 and 40%, and high-energy episodes (e.g., storm waves) that could have altered the normal deposition can be reasonably excluded, due to the absence of abrupt variations down core and the continuous occurrence of nannoplankton (Figure 3).
Figure 3. From left to right: photo of CORE18, granulometric curves according to Blott and Pye (2012), and curve of absolute abundance of calcareous nannoplankton down core. The content of finest particles continuously recorded without abrupt variations along the core is mandatory in order to proceed with radiometric dating.
The curves of 210Pb decay are very similar in the two cores, indicating a good replicability of the collected data. Both curves show the typical activity-depth profile, with higher activities at the core top that rapidly decrease down core (Figure 4 and Supplementary Table 3 available on-line as Supplementary Data) halving within the first 25 cm below sea floor (bsf). This interval was used to calculate a constant Sediment Accumulation Rate (SAR) of about 0.22 cm yr–1 (Figure 4A); the derived age model provides an estimated time interval of about 4.5 years for each centimeter of sediment. Unfortunately, no 137Cs was recorded in either core, thus an independent validation through this method was not possible. While the absence of the Chernobyl peak of 137Cs is common in sediments collected in the southern part of the Mediterranean Sea due to the dispersion pattern of 137Cs fallout that followed the accident, it is surprising to have no signal of nuclear bomb experiments. Nevertheless, the absence of 137Cs in both cores supports the finding that in this area the 137Cs supply is negligible, as also reported by other works (e.g., Hassen et al., 2019).
Figure 4. Curves reporting from left to right: 210Pb decay, showing the typical activity profile decreasing with depth, and absolute abundances of A. lobifera (in red) and A. lessonii (in green) recorded in both cores with a characteristic decreasing trend moving down core. The boxes on the bottom report a constant SAR, respectively of 0.20 cm yr–1 for CORE18 (A) and 0.22 cm yr–1 for CORE19 (B); this last value was utilized in the applied age model, which leads to an estimated time interval of about 4.5 years for each cm of sediment (see the text for further explanations).
Along the studied cores, the absolute abundance of both A. lobifera and A. lessonii is characterized by a decreasing trend starting from the core top to the lowest occurrence (Figure 4 and Supplementary Table 4 available on-line as Supplementary Data). All curves show the same distribution pattern down core indicating that the two records are well replicated and have not suffered episodes of mixing by high energy events. In CORE18, A. lobifera is continuously present from cm 0–1 to cm 14–15 bsf, while in CORE19 its lowest occurrence is slightly deeper and corresponds to cm 16–17 bsf. The highest abundances were recorded in the upper portion of sediment cores. In both cores, the absolute abundances of A. lobifera decrease abruptly starting from cm 6–7 bsf down to the lowest occurrences, where the minimum values were recorded. Similarly, A. lessonii shows the highest abundances in the upper portion of the cores and an abrupt decrease starting from cm 6–7 bsf down to the lowest occurrences, which correspond to cm 14–15 bsf in CORE18 and to cm 17–18 bsf in CORE19.
Based on the age model, where 1 cm of sediment corresponds to a time interval of 4.5 years, the first occurrence of A. lobifera in Marsamxett Harbor can be dated to the mid-1940s (Figure 5). During the first decades of its colonization, up to the end of the 1980s, A. lobifera is present but with very low abundances, probably in response to environmental conditions still not completely favorable for the growth of dense populations. Starting from the 1990s, A. lobifera increases more rapidly, reaching the maximum peak of abundance between 2005 and 2010, when it was recorded in the Maltese Islands by Yokeş et al. (2007) for the first time. Thus, the first occurrence of A. lobifera was reported in 2006 with a lag time of about 60 years from its true first arrival; additionally, the establishment of dense populations and their spread along the Maltese coasts were reported by Guastella et al. (2019) with a lag time of 30 years (Figure 5).
Figure 5. Curves of absolute abundance of A. lobifera (A) and A. lessonii (B) with the different steps of invasion process (on the left): (1) introduction of the species after the opening of Suez Canal in 1869; (2) first arrival of A. lobifera occurred in the mid-1940s and of A. lessonii at the end of 1930s, respectively; (3) subsequent gradual colonization and (4) the establishment and population increase since the 1990s for both species. Compared to the real first arrival and the beginning of colonization process of A. lobifera, the first record documented in 2006 (Yokeş et al., 2007) occurred with a lag time of about 60 years. Guastella et al. (2019) reported the first record of A. lessonii in Malta in 2018, with a lag time of about 80 years and the establishment/spread of A. lobifera and A. lessonii with a lag time of about 30 years.
The first occurrence of A. lessonii in Marsamxett Harbor can be dated at the end of 1930s. Although with lower abundances than A. lobifera, A. lessonii displays a similar invasion dynamic: its population is restricted to a few specimens during the first decades, then rapidly increases in abundance starting from the 1990s, up to the first record made in 2018 (Guastella et al., 2019). Thus, the first record of A. lessonii in Malta was reported with a lag time of 80 years, while the establishment of permanent populations with a lag time of 30 years (Figure 5).
From the comparison of the increasing abundances of A. lobifera and A. lessonii recorded along the sediment cores with the annual average SST measured during the last 60 years, a strong similarity emerges (Figure 6 and Supplementary Table 5 available on-line as Supplementary Data). Between the 1980s and the 1990s, a progressive rise of the annual average SST is recorded, with an average overall increase of ∼ 1°C; in the same timeframe, A. lobifera and A. lessonii abundances start to increase as well. The same pattern results when considering the annual average wintry SST, for both A. lobifera and A. lessonii. Since the 1980s, the average SST increased rapidly during the winter, exceeding 15°C at the beginning of the 1990s and leading to an average overall rise of ∼ 0.7°C (Figure 6). The aforementioned relation is further supported by the time series of annual SST anomalies evaluated against the 1955–2015 average, at 16 m depth in the grid point of the model closest to the sampling site of sediment cores in Malta (Figure 7 and Supplementary Table 5). In fact, Figure 7 shows that both A. lobifera and A. lessonii increase their abundance only when the annual SST anomaly curve exhibits positive values, corresponding to the warmer phase (with temperature higher than 1955–2015 average) starting from 1985 and still in progress (Marullo et al., 2011), and finally, the pattern is similar when considering also the annual wintry SST anomaly. All correlations between absolute abundance of both foraminiferal species and annual average SST, wintry SST, SST anomaly and wintry SST anomaly resulted positive and significant in both core samples, with an average correlation coefficient of 0.74 (Figures 6, 7 and Supplementary Table 6 available on-line as Supplementary Data).
Figure 6. Curves reporting from left to right: absolute abundance of A. lobifera (A) and A. lessonii (B) plotted against the annual SST and wintry SST simulated since 1955–2015. Beside: scatter-plots with Spearman’s coefficients (rs) relative to the absolute abundances of A. lobifera and A. lessonii recorded in CORE18 and CORE19 compared to the annual average and wintry SST (p-values of correlation indicated in brackets as follows: *p < 0.05; **p < 0.01; ***p < 0.001).
Figure 7. Curves reporting from left to right: absolute abundance of A. lobifera (A) and A. lessonii (B) plotted against the annual SST anomaly and wintry SST anomaly evaluated over the period 1955–2015. Beside: scatter-plots with Spearman’s coefficients (rs) relative to the absolute abundances of A. lobifera and A. lessonii recorded in CORE18 and CORE19 compared to the annual and wintry SST anomalies (p-values of correlation indicated in brackets as follows: *p < 0.05; **p < 0.01l; ***p < 0.001). The beginning of the exponential growth of both populations starts around the 1990s, when the warmer phases (in orange) abruptly increase in intensity and frequency.
This study is one of the few works (e.g., Ribeiro et al., 2012; Lishawa et al., 2013; Albano et al., 2018; Deldicq et al., 2019; Holman et al., 2019) applying analyses and methods commonly used in Micropaleontology and Paleoecology for the investigation of a relatively recent phenomenon, such as marine bioinvasions. Here we unified the recent fossil record of two alien foraminiferal species preserved in sediment cores with field observations, radiometric dating and environmental variables, such as SST trends. We also documented a possible causality link between temperature increase and population outbreaks.
Advantages of this approach include: (I) possibility of analyzing long historical records (up to about one century) on a continuous base; (II) possibility of analyzing early stages of invasions (e.g., see Walsh et al., 2016), which are very often unknown because several alien species tend to remain overlooked for a long time after their first arrival (Carlton, 2009); (III) possibility of directly exploring the response of the target species to environmental parameters, provided that long time-series are available. More in general, the analysis of sediment cores has proven useful in detecting anthropogenic change in a variety of coastal and marine communities, by reconstructing ecological scenarios before human intervention (e.g., Yamamuro and Kanai, 2005; de Boer et al., 2013; Lin et al., 2019; Handley et al., 2020; O’Dea et al., 2020).
Thanks to the availability of continuous, direct measurements of species abundance, the first three points above are here treated with a quantitative, replicable approach, differently from several other studies where bioinvasion patterns and dynamics are reconstructed from qualitative or non-structured observations (e.g., Delaney et al., 2008; Azzurro et al., 2019) or from modeling approaches (Salihoglu et al., 2011; Kanary et al., 2014; Mellin et al., 2016), to compensate for the lack of continuous and direct measurements.
However, using the analysis of sediment core samples to unravel invasion histories has some stringent restrictions. The first and most obvious restriction is that this method applies only to taxa with mineralized remains that can persist in thanatocoenoses of sediments (e.g., Albano et al., 2018; Deldicq et al., 2019). Another challenging requirement is related to the nature of the sediment containing the target species: it should reflect both its habitat (in terms of substratum, grain-size, depth, etc.) and its real distribution through time, should be sufficiently sheltered from wave/current motion to avoid vertical mixing, free from bioturbation and free from reworked fossil specimens that, in the case of living species having fossil counterparts, could misrepresent the original first occurrence along the core record. This may present a problem when one of these conditions is not met. Moreover, we must keep in mind that faunal variations observed down core strongly depend on the sediment accumulation rate (SAR): a single centimeter of sediment can correspond to multiple years, thus only in the case of very high SAR we can reconstruct annual or seasonal time series.
In spite of the restrictions mentioned above, this approach deserves to be included in the toolkit for studying invasion dynamics by these types of marine organisms, for which literature data are poorest and which are most likely to meet all the required conditions.
Sea surface warming in the semi-enclosed Mediterranean basin has been largely documented and predicted, based on satellite measurements coupled to complex ocean models (Pastor et al., 2017; Sakalli, 2017; Macias et al., 2018). Analysis of decadal SST trends has documented a consistent warming increase starting from 1980 to 1983, which could be part of a 70-year variation linked to the Atlantic multi-decadal oscillation (Marullo et al., 2011; Pastor et al., 2017). Despite being uneven across time and space, the warming trend of the Mediterranean Sea surface has notably accelerated during the last two decades (Pastor et al., 2017; Sakalli, 2017). Since a global increase in sea temperatures has been reported to promote the redistribution of marine biodiversity, supporting the spread of thermophilic invaders (Occhipinti-Ambrogi, 2007; Marras et al., 2015; Molinos et al., 2016; Walsh et al., 2016), an accelerated warming rate should be taken into serious account when studying relationships between invasion processes and climate change, especially in the semi-enclosed Mediterranean Sea where the climatic signals can be amplified (Pastor et al., 2017). In fact, there is evidence that sea warming has been progressively shifting the horizontal and vertical distribution of Mediterranean marine species (Lejeusne et al., 2010; Marbà et al., 2015), and favoring tropical alien species to the detriment of temperate native species (Raitsos et al., 2010; Azzurro et al., 2019).
Our results, derived from historical records preserved in radiometrically-dated sediment cores, provide a unique opportunity to unravel the invasion history of cryptic invaders and to follow the 70 year-long dynamics of two tropical species that have been exposed to the progressive heating of the central Mediterranean Sea. We document that these two target species, the Indo-Pacific A. lobifera and the cryptogenic A. lessonii, reached Malta at the beginning of the 1940s, several decades earlier than their first records (Yokeş et al., 2007; Guastella et al., 2019). However, they did not develop dense populations for at least 50 years. Protracted lag times between initial introduction and population explosion have been reported for other marine alien species, such as the mussel Brachidontes pharaonis (Fischer, 1870) in the Mediterranean Sea (Rilov et al., 2004), the barnacle Austrominius modestus (Darwin, 1854) in the North Sea (Witte et al., 2010) and the cladoceran (water flea) Bythotrephes longimanus (Leydig, 1860) in Lake Mendoka, United States (Spear et al., 2020). Such delayed population outbreaks may be due to multiple independent introduction events occurring along time, that may enrich the population gene pool and provide genotypes more fit for the local conditions (Dlugosch and Parker, 2008; Handley et al., 2011), or to changes of the receiving environment (e.g., increased vulnerability, relaxation of biotic pressure, more favorable abiotic environment, etc.), resulting in population growth of the alien species (Sakai et al., 2001; Crooks, 2005; Walsh et al., 2016).
In the case of A. lobifera and A. lessonii, a possible explanation for the delayed population outbreak could be the altered seasonal patterns of sea temperature triggered by global climate change. For both populations, in fact, the beginning of their exponential growth started only after A.D. 1990, and more pronouncedly after A.D. 2003, when the wintry SST repeatedly exceeded 15°C (Figure 6) and the warmer phase abruptly increased its intensity and frequency along the timeline (Figure 7). Indeed, laboratory experiments and direct observations have demonstrated that 15°C represents a thermal threshold for both species, which are unable to calcify the tests at temperatures below that value (Titelboim et al., 2019). In particular, A. lessonii displays higher sensitivity than A. lobifera, and this fact could explain why the latter has so far achieved a higher invasion success in the Mediterranean basin (Titelboim et al., 2019). Additionally, A. lobifera collected from the eastern Mediterranean has a similar thermal response to high temperatures than A. lobifera from the Red Sea, showing that the thermal tolerance is retained during its invasion (Schmidt et al., 2016a). On the other hand, laboratory experiments have shown that higher temperatures (>32°C) are better tolerated by A. lessonii than A. lobifera (Schmidt et al., 2016b; Titelboim et al., 2019). This implies that in the future, according to the predicted Mediterranean warming (Sakalli, 2017; Macias et al., 2018) and the different tolerance ranges of the two species, A. lessonii could be favored, overcoming A. lobifera in relative abundance.
Historical data suggest that such switch is not unlikely. The southern portion of the Sicily Channel, an area characterized today by mean annual SST varying between 19 and 21°C (Drago et al., 2010), during Late Pliocene (3.1–2.51 Ma), was warmer on average 6–7°C than modern conditions (Herbert et al., 2015; Plancq et al., 2015; Tzanova and Herbert, 2015). In that epoch, Mediterranean amphisteginid populations were strongly dominated by A. lessonii, with minor abundances of the congeneric species A. targionii and A. gibbosa (Di Bella et al., 2005). Their test remains were so abundant on the sea floor after death to form biogenic deposits named Amphistegina-rich beds, over 1 m thick and cropping out all over the Mediterranean basin (Di Bella et al., 2005; Caruso and Cosentino, 2014; Cau et al., 2019). Amphistegina lessonii probably developed its acclimation ability earlier, thanks to a longer colonization history during the warmer Pliocene Mediterranean, at least since ∼ 5.3 Ma, when the species recolonized the basin after the abrupt foraminiferal extinction following the Messinian salinity crisis (Hayward et al., 2009). This ability could advantage the species even in the future, taking over from the congeneric Indo-Pacific A. lobifera. On the other hand, A. lobifera is characterized by a high dispersal capacity, and can modify its geographic range in response to global warming without requiring further adaptation (Prazeres et al., 2020).
This fact, however, might be considered realistic only if invaders spread and acclimate to new recipient environments fast enough to keep pace with climate change (Hiddink et al., 2012; Marras et al., 2015; Molinos et al., 2016). Based on a literature search and meta-analyses, Sorte et al. (2010) estimated that the poleward shift of marine species in response to climate change happens at an average rate of ∼ 19 km yr–1, which is an order of magnitude faster than the estimated range shift of terrestrial species. According to a recent species distribution model (Guastella et al., 2019), A. lobifera is spreading in the Mediterranean Sea at a rate of 13.2 km yr–1. However, this estimate was based on the known sequence of first records of the species along the eastern and central Mediterranean coasts. Here, we demonstrate that a serious bias exists in the assessment of the “first arrival” of A. lobifera, showing that in Malta the species went overlooked for as much as 60 years. Additionally, it could be assumed that this bias also exists in other localities around the Mediterranean Sea, because our results precede the first record of A. lobifera in the entire Mediterranean, making all first records of this alien species presumably incorrect, as well as questioning hypothesis of spreading due to ichtyochory by lessepsian rabbitfish (Guy-Haim et al., 2017). On the other hand, we show that the species arrived in the central Mediterranean at least in the mid-1940s, that is only 70 years after the opening of the Suez Canal occurred in 1869. Malta is about 3,200 km far from Port Said, the outlet of the Suez Canal (as calculated along the North-African coasts), a distance that both A. lobifera and A. lessonii have covered in about 70 years with a spread rate of ∼ 45 km yr–1. This value is consistent with the spread rates estimated for other Erythraean invaders that move in response to climate change (referred to inter-quartile range of 25–75%: 17.0–49.8 km yr–1 for continental shelf dispersal and 12.6–35.1 km yr–1 for straight-line dispersal; Hiddink et al., 2012).
Accurate data on the arrival and temporal dynamics of alien taxa are essential to understand invasion patterns and their drivers, and hence to design and implement effective measures. Such data are often lacking, especially for small-sized taxa that often remain undetected or are only sporadically recorded until their populations reach outstanding densities. The present work demonstrates how, in the case of taxa such as foraminifera with mineralized remains that persist in sediments after their death, micropaleontologic analysis of radiometrically-dated sediment cores can be used to reconstruct invasion histories, accurately determining the true date of introduction and subsequent temporal changes in abundance. In turn, the population abundance time-series can be linked to changes in environmental parameters. Using this approach, we unraveled the invasion history of two foraminiferal species in the Central Mediterranean Sea, reporting a considerable lag-time between their arrival and their first record, and highlighting how inadequate knowledge can lead to misleading or incorrect conclusions (e.g., expansion rates and vectors).
Finally, by relating the temporal dynamics of the two Amphistegina populations with trends in sea surface temperature, we also show that a link exists between sea warming and population density of both species. This suggests that temperature could represent an important driver of invasion patterns, with sea surface warming having the potential to trigger population outbreaks of these thermophilic alien species. We therefore propose the micropaleontologic approach adopted in the present work as an important addition to the toolkit for studying bioinvasions, serving to elucidate invasion histories, identify the main drivers of invasion success, and ultimately better inform management decisions.
The original contributions presented in the study are included in the article/Supplementary Material, further inquiries can be directed to the corresponding author/s.
NM and AM developed the idea. AC, JE, and CC collected and sampled the cores. RG and MC performed the micropaleontologic analyses. LL carried out the radiometric dating calculations. RL provided and elaborated sea surface temperature data. All authors contributed to interpretation of results and discussions and wrote parts of the manuscript.
This work was part of a Ph.D. project of the University of Pavia (RG) financially supported by the MIUR (Italian Ministry of Education, University and Research) and by FRG-2018 and FFABR funds (to NM and AM, University of Pavia) and by R1D14-PLHA2010_MARGINE (to AC, University of Palermo). JE received financial support from the University of Malta’s Research Fund. Two reviewers have contributed to improve an earlier version of the manuscript.
The authors declare that the research was conducted in the absence of any commercial or financial relationships that could be construed as a potential conflict of interest.
We acknowledge Sonia Albertazzi (CNR-ISMAR) for help with 210Pb and 137Cs measurements, Maria Pia Riccardi (University of Pavia) for photos at the stereomicroscope, and Nadia Pinardi (University of Bologna) for facilitating access to SST data. The reviewers are gratefully acknowledged for their constructive comments.
The Supplementary Material for this article can be found online at: https://www.frontiersin.org/articles/10.3389/fmars.2021.675807/full#supplementary-material
Supplementary Table 1 | Geographic coordinates and other sampling details of the study sites.
Supplementary Table 2 | Census of benthic foraminifera recorded in the study sites from the Maltese islands.
Supplementary Table 3 | Radiometric data and chronological constrain based on 210Pb decay.
Supplementary Table 4 | Absolute abundances of A. lobifera and A. lessonii recorded along CORE18 and CORE19.
Supplementary Table 5 | Sea Surface Temperature (SST) in Marsamxett harbor measured at 16 m depth in the grid point of the model closest to the sampling site of sediment cores [Lat. 35°56′15″N; Long. 14°30′00″E].
Supplementary Table 6 | Spearman rank correlation coefficient (rs) and p-values between sea surface temperature -SST (annual average SST, average wintry SST, annual SST anomaly, and wintry SST anomaly) and abundance of A. lobifera and A. lessonii in the two sediment cores: CORE18 and CORE19.
Abril, J. M., and Brunskill, G. J. (2014). Evidence that excess 210Pb flux varies with sediment accumulation rate and implications for dating recent sediments. J. Paleolimnol. 52, 121–137. doi: 10.1007/s10933-014-9782-6
Ahnelt, H. (2016). Translocations of tropical and subtropical marine fish species into the Mediterranean. A case study based on Siganus virgatus (Teleostei: Siganidae). Biologia 71, 952–959. doi: 10.1515/biolog-2016-0106
Albano, P. G., Gallmetzer, I., Haselmair, A., Tomasovych, A., Stachowitsch, M., and Zuschin, M. (2018). Historical ecology of a biological invasion: the interplay of eutrophication and pollution determines time lags in establishment and detection. Biol. Invas. 20, 1417–1430. doi: 10.1007/s10530-017-1634-7
Andersen, T. J. (2017). “Some practical considerations regarding the application of 210pb and 137cs dating to estuarine sediments,” in Applications of Paleoenvironmental Techniques In Estuarine Studies, Developments in Paleoenvironmental Research, eds K. Weckstrom, K. Saunders, P. Gell, and C. Skilbeck (Dordrecht: Springer), 121–140. doi: 10.1007/978-94-024-0990-1_6
Azzurro, E., Sbragaglia, V., Cerri, J., Bariche, M., Bolognini, L., and Ben Souissi, J. (2019). Climate change, biological invasions, and the shifting distribution of Mediterranean fishes: a large scale survey based on local ecological knowledge. Glob. Change Biol. 25, 2779–2792. doi: 10.1111/gcb.14670
Baldassini, N., and Di Stefano, A. (2017). Stratigraphic features of the maltese archipelago: a synthesis. Nat. Hazards 86, S203–S231.
Bariche, M., Kazanjian, G., and Azzurro, E. (2014). A lag of 25 years: evidence from an old capture of Fistularia commersonii Rüppell, 1838 from Lebanon (Mediterranean Sea). J. Appl. Ichthyol. 30, 535–536. doi: 10.1111/jai.12394
Blott, S. J., and Pye, K. (2012). Particle size scale and classification of sediment types based on particle size distributions: review and recommended procedures. Sedimentology 59, 2071–2096. doi: 10.1111/j.1365-3091.2012.01335.x
Carlton, J. T. (2009). “Deep invasion ecology and the assembly of communities in historical time,” in Biological Invasions in Marine Ecosystems, eds G. Rilov and J. A. Crooks (Cham: Springer), 13–56. doi: 10.1007/978-3-540-79236-9_2
Caruso, A., and Cosentino, C. (2014). The first colonization of the Genus Amphistegina and other exotic benthic foraminifera of the Pelagian Islands and south-eastern Sicily (central Mediterranean Sea). Mar. Micropaleontol. 111, 38–52. doi: 10.1016/j.marmicro.2014.05.002
Cau, S., Laini, A., Monegatti, P., Roveri, M., Scarponi, D., and Taviani, M. (2019). Palaeoecological anatomy of shallow-water Plio-Pleistocene biocalcarenites (northern Apennines, Italy). Paleogeogr. Paleoclimatol. Paleoecol. 514, 838–851. doi: 10.1016/j.palaeo.2018.08.011
Clark, G. F., Johnston, E. L., and Leung, B. (2013). Intrinsic time dependence in the diversity-invasibility relationship. Ecology 94, 25–31. doi: 10.1890/12-0592.1
Crooks, J. A. (2005). Lag times and exotic species: the ecology and management of biological invasions in slow-motion. Ecoscience 12, 316–329. doi: 10.2980/i1195-6860-12-3-316.1
de Boer, E. J., Slaikovska, M., Hooghiemstra, H., Rijsdijk, K. F., MariaIVèlez, M., Prins, M., et al. (2013). Multi-proxy reconstruction of environmental dynamics and colonization impacts in the Mauritian uplands. Paleogeogr. Paleoclimatol. Paleoecol. 383–384, 42–51. doi: 10.1016/j.palaeo.2013.04.025
Delaney, D. G., Sperling, C. D., Adams, C. S., and Leung, B. (2008). Marine invasive species: validation of citizen science and implications for national monitoring networks. Biol. Invas. 10, 117–128. doi: 10.1007/s10530-007-9114-0
Deldicq, N., Alve, E., Schweizer, M., Polovodova Asteman, I., Hess, S., Darling, K., et al. (2019). History of the introduction of a species resembling the benthic foraminifera Nonionella stella in the oslofjord (Norway): morphological, molecular and paleo-ecological evidences. Aquat. Invas. 14, 182–205. doi: 10.3391/ai.2019.14.2.03
Di Bella, L., Carboni, M. G., and Pignatti, J. (2005). Paleoclimatic significance of the Pliocene Amphistegina levels from the Tyrrhenian margin of Central Italy. Boll. Soc. Paleontol. Ital. 44, 219–229.
Dlugosch, K. M., and Parker, I. M. (2008). Founding events in species invasions: genetic variation, adaptive evolution, and the role of multiple introductions. Mol. Ecol. 17, 431–449. doi: 10.1111/j.1365-294x.2007.03538.x
Drago, A., Sorgente, R., and Olita, A. (2010). Sea temperature, salinity and total velocity climatological fields for the south-central Mediterranean Sea. MedSudMed Techn. Doc. 35, pp.
Galil, B. S., Douek, J., Gevili, R., Goren, M., Yudkovsky, Y., Paz, G., et al. (2018). The resurrection of Charybdis (Gonioinfradens) giardi (Nobili, 1905), newly recorded from the SE Mediterranean Sea. Zootaxa 4370, 580–590. doi: 10.11646/zootaxa.4370.5.9
Griffiths, C. L., Mead, A., and Robinson, T. B. (2010). A brief history of marine bio-invasions in South Africa. Afr. Zool. 44, 241–247. doi: 10.3377/004.044.0212
Guastella, R., Marchini, A., Caruso, A., Cosentino, C., Evans, J., Anna, E., et al. (2019). “Hidden invaders” conquer the Sicily Channel and knock on the door of the Western Mediterranean sea. Est., Coast. Shelf Sci. 225:106234. doi: 10.1016/j.ecss.2019.05.016
Guy-Haim, T., Hyams-Kaphzan, O., Yeruham, E., Almogi-Labin, A., and Carlton, J. T. (2017). A novel marine bioinvasion vector: ichthyochory, live passage through fish. Limnol. Oceanogr. Lett. 2, 81–90. doi: 10.1002/lol2.10039
Hallock, P. (1984). Distribution of larger foraminiferal assemblages on two Pacific coral reefs. J. Foraminiferal Res. 11, 195–208.
Hammer, Ø, Harper, D. A. T., and Ryan, P. D. (2001). PAST: Paleontological statistics software package for education and data analysis. Palaeontol. Electron. 4:9.
Handley, L. J. L., Estoup, A., Evans, D. M., Thomas, C. E., Lombaert, E., Facon, B., et al. (2011). Ecological genetics of invasive alien species. Biocontrol 56, 409–428.
Handley, S. J., Swales, A., Horrocks, M., Gibbs, M., Carter, M., Ovenden, R., et al. (2020). Historic and contemporary anthropogenic effects on granulometry and species composition detected from sediment cores and death assemblages, Nelson Bays, Aotearoa-New Zealand. Cont. Shelf Res. 202:104147. doi: 10.1016/j.csr.2020.104147
Hassen, N., Reguigui, N., Helali, M., Mejjad, N., Laissaoui, A., Benkdad, A., et al. (2019). Evaluating the historical sedimentation patterns in two different Mediterranean deep environments (Sardinia and Sicily Channels). Med. Mar. Sci. 20, 542–548. doi: 10.12681/mms.19558
Hayward, B. W., Sabaa, A. T., Kawagata, S., and Grenfell, H. R. (2009). The Early Pliocene re-colonisation of the deep Mediterranean Sea by benthic foraminifera and their pulsed Late Pliocene-Middle Pleistocene decline. Mar. Micropaleontol. 71, 97–112. doi: 10.1016/j.marmicro.2009.01.008
Herbert, T. D., Ng, G., and Cleaveland Peterson, L. (2015). Evolution of Mediterranean sea surface temperature 3.5-1.5 Ma: regional and hemispheric influences. Earth Planet. Sci. Lett. 409, 307–318. doi: 10.1016/j.epsl.2014.10.006
Hiddink, J. G., Ben Rais, Lasram, F., Cantrill, J., and Davies, A. J. (2012). Keeping pace with climate change: what can we learn from the spread of Lessepsian migrants? Glob. Change Biol. 18, 2161–2172. doi: 10.1111/j.1365-2486.2012.02698.x
Hollaus, S. S., and Hottinger, L. (1997). Temperature dependance of endosymbiontic relationships? Evidence from the depth range of mediterranean Amphistegina lessonii (Foraminiferida) truncated by the thermocline. Eclogae Geol. Helv. 90, 591–598.
Hohenegger, J. (1995). Depth estimation by proportions of living larger foraminifera. Mar. Micropaleontol. 26, 31–47. doi: 10.1016/0377-8398(95)00044-5
Hohenegger, J. (2004). Depth coenoclines and environmental considerations of Western Pacific larger foraminifera. J. Foram. Res. 34, 9–33. doi: 10.2113/0340009
Hohenegger, J., Yordanova, E., Nakano, Y., and Tatzreiter, F. (1999). Habitats of larger foraminifera on the upper reef slope of Sesoko Island, Okinawa, Japan. Mar. Micropaleontol. 36, 109–168. doi: 10.1016/s0377-8398(98)00030-9
Hollins, S. E., Harrison, J. J., Jones, G. J., Zawadzki, A., Heijnis, H., and Hankin, S. (2011). Reconstructing recent sedimentation in two urbanised coastal lagoons (NSW, Australia) using radioisotopes and geochemistry. J. Paleolimnol. 46, 579–596. doi: 10.1007/s10933-011-9555-4
Holman, L. E., de Bruyn, M., Creer, S., Carvalho, G., Robidart, J., and Rius, M. (2019). Detection of introduced and resident marine species using environmental DNA metabarcoding of sediment and water. Sci. Rep. 9:11559.
Hottinger, L., Halicz, E., and Reiss, Z. (1993). Recent Foraminifera from the Gulf of Aqaba, Red Sea, Vol. 33. Ljubljana: Dela SAZU, 1–179.
Incarbona, A., Martrat, B., Mortyn, P. G., Sprovieri, M., Ziveri, P., Gogou, A., et al. (2016). Mediterranean circulation perturbations over the last five centuries: relevance to past Eastern Mediterranean Transient-type events. Sci. Rep. 6:29623.
Kanary, L., Musgrave, J., Tyson, R. C., Locke, A., and Lutscher, F. (2014). Modelling the dynamics of invasion and control of competing green crab genotypes. Theor. Ecol. 7, 391–406. doi: 10.1007/s12080-014-0226-8
Kosnik, M. A., Hua, Q., Kaufman, D. S., and Zawadzki, A. (2015). Sediment accumulation, stratigraphic order, and the extent of time-averaging in lagoonal sediments: a comparison of 210Pb and 14C/amino acid racemization chronologies. Coral Reefs 34, 215–229. doi: 10.1007/s00338-014-1234-2
Langer, M. R., and Hottinger, L. (2000). Biogeography of selected “larger” foraminifera. Micropaleontology 46, 105–126.
Langer, M. R., and Mouanga, G. H. (2016). Invasion of amphisteginid foraminifera in the Adriatic Sea. Biol. Invas. 18:1335. doi: 10.1007/s10530-016-1070-0
Langer, M. R., Weinmann, A. E., Lotters, S., Bernhard, J. M., and Rodder, D. (2013). Climate-driven range extension of Amphistegina (Protista, Foraminiferida): models of current and predicted future ranges. PLoS One 8:e54443. doi: 10.1371/journal.pone.0054443
Langer, M. R., Weinmann, A. E., Lotters, S., and Rodder, D. (2012). “Strangers” in paradise: modelling the biogeographic range expansion of the foraminifera Amphistegina in the Mediterranean Sea. J. Foraminif. Res. 42, 234–244. doi: 10.2113/gsjfr.42.3.234
Lejeusne, C., Chevaldonné, P., Pergent-Martini, C., Boudouresque, C. F., and Pérez, T. (2010). Climate change effects on a miniature ocean: the highly diverse, highly impacted Mediterranean Sea. Trends Ecol. Evol. 25, 250–260. doi: 10.1016/j.tree.2009.10.009
Lin, C. H., De Gracia, B., Pierotti, M. E., Andrews, A. H., Griswold, K., and O’Dea, A. (2019). Reconstructing reef fish communities using fish otoliths in coral reef sediments. PLoS One 14:e0218413. doi: 10.1371/journal.pone.0218413
Lishawa, S. C., Treering, D. J., Vail, L. M., McKenna, O., Grimm, E. G., and Tuchman, N. C. (2013). Reconstructing plant invasions using historical aerial imagery and pollen core analysis: Typha in the Laurentian Great Lakes. Divers. Distrib. 19, 14–28. doi: 10.1111/j.1472-4642.2012.00929.x
Macias, D., Garcia-Gorriz, E., Dosio, A., and Stips, A. (2018). Obtaining the correct sea surface temperature: bias correction of regional climate model data for the Mediterranean Sea. Clim. Dynam. 51, 1095–1117. doi: 10.1007/s00382-016-3049-z
Magri, O. (2006). A geological and geomorphological review of the maltese islands with special reference to the coastal zone. Territoris 6, 7–26.
Marbà, N., Jordà, G., Agustí, S., Girard, C., and Duarte, C. M. (2015). Footprints of climate change on Mediterranean sea biota. Front. Mar. Sci. 2:56. doi: 10.3389/fmars.2015.00056
Marras, S., Cucco, A., Antognarelli, A., Azzurro, E., Milazzo, M., Bariche, M., et al. (2015). Predicting future thermal habitat suitability of competing native and invasive fish species: from metabolic scope to oceanographic modelling. Conserv. Physiol. 3:cou059. doi: 10.1093/conphys/cou059
Marullo, S., Artale, V., and Santoleri, R. (2011). The SST multidecadal variability in the atlantic-mediterranean region and its relation to AMO. J. Clim. 24, 4385–4401. doi: 10.1175/2011jcli3884.1
Mellin, C., Lurgi, M., Matthews, S., MacNeil, M. A., Caley, M. J., Bax, N., et al. (2016). Forecasting marine invasions under climate change: biotic interactions and demographic processes matter. Biol. Conserv. 204, 459–467. doi: 10.1016/j.biocon.2016.11.008
Molinos, G. J., Halpern, B. J., Schoeman, D. S., Brown, C. J., Kiessling, W., Moore, P. J., et al. (2016). Climate velocity and the future global redistribution of marine biodiversity. Nat. Clim. Change 6, 83–88. doi: 10.1038/nclimate2769
Mouanga, G. H., and Langer, M. R. (2014). At the front of expanding ranges: shifting community structures at amphisteginid species range margins in the Mediterranean Sea. Neues Jahrbuch Geol. Palaontol. Abhand. 271/2, 141–150. doi: 10.1127/0077-7749/2014/0381
Occhipinti-Ambrogi, A. (2007). Global change and marine communities: alien species and climate change. Mar. Poll. Bull. 55, 342–352. doi: 10.1016/j.marpolbul.2006.11.014
O’Dea, A., Lepore, M., Altieri, A. H., Chan, M., Morales-Saldaña, J. M., Muñoz, N. H., et al. (2020). Defining variation in pre-human ecosystems can guide conservation: an example from a Caribbean coral reef. Sci. Rep. 10, 1–10.
Ojaveer, H., Galil, B. S., Carlton, J. T., Alleway, H., Goulletquer, P., Lehtiniemi, M., et al. (2018). Historical baselines in marine bioinvasions: implications for policy and management. PLoS One 13:e0202383. doi: 10.1371/journal.pone.0202383
Ordóñez, V., Pascual, M., Fernández-Tejedor, M., and Turon, X. (2016). When invasion biology meets taxonomy: Clavelina oblonga (Ascidiacea) is an old invader in the Mediterranean Sea. Biol. Invas. 18, 1203–1215. doi: 10.1007/s10530-016-1062-0
Parravicini, V., Mangialajo, L., Mousseau, L., Peirano, A., Morri, C., Montefalcone, M., et al. (2015). Climate change and warm-water species at the northwestern boundary of the Mediterranean Sea. Mar. Ecol. 36, 897–909. doi: 10.1111/maec.12277
Pastor, F., Valiente, J. A., and Palau, J. L. (2017). Sea surface temperature in the Mediterranean: trends and spatial patterns (1982-2016). Pure Appl. Geophys. 175, 4017–4029. doi: 10.1007/s00024-017-1739-z
Petersen, K. S., Rasmussen, K. L., Heinemeler, J., and Rud, N. (1992). Clams before columbus? Nature 359:679. doi: 10.1038/359679a0
Plancq, J., Grossi, V., Pittet, B., Huguet, C., Rosell-Melè, A., and Mattioli, E. (2015). Multi-proxy constraints on sapropel formation during the late Pliocene of central Mediterranean (southwest Sicily). Earth Planet. Sci. Lett. 420, 30–44. doi: 10.1016/j.epsl.2015.03.031
Prazeres, M., Morard, R., Roberts, T. E., Doo, S. S., Jompa, J., Schmidt, C., et al. (2020). High dispersal capacity and biogeographic breaks shape the genetic diversity of a globally distributed reef-dwelling calcifier. Ecol. Evol. 10, 5976–5989. doi: 10.1002/ece3.6335
Raitsos, D. E., Beaugrand, G., Georgopoulos, D., Zenetos, A., Pancucci-Papadopoulou, A. M., Theocharis, A., et al. (2010). Global climate change amplifies the entry of tropical species into the Eastern Mediterranean Sea. Limnol. Oceanog. 55, 1478–1484. doi: 10.4319/lo.2010.55.4.1478
Reavie, E. D., and Cangelosi, A. A. (2020). Ballast water management system certification testing requirements for protists are a poor fit for the Great Lakes. J. Great Lakes Res. 46, 237–242. doi: 10.1016/j.jglr.2019.10.020
Renema, W. (2018). Terrestrial influence as a key driver of spatial variability in large benthic foraminiferal assemblage composition in the Central Indo-Pacific. Earth Sci. Rev. 177, 514–544. doi: 10.1016/j.earscirev.2017.12.013
Ribeiro, S., Amorim, A., Andersen, T. J., Abrantes, F., and Ellegaard, M. (2012). Reconstructing the history of an invasion: the toxic phytoplankton species Gymnodinium catenatum in the Northeast Atlantic. Biol. Invas. 14, 969–985. doi: 10.1007/s10530-011-0132-6
Rilov, G., Benayahu, Y., and Gasith, A. (2004). Prolonged lag in population outbreak of an invasive mussel: a shifting-habitat model. Biol. Invas. 6, 347–364. doi: 10.1023/b:binv.0000034614.07427.96
Rizzo, S., Basile, S., Caruso, A., Cosentino, C., Tranchina, L., and Brai, M. (2009). Dating of a Sediment core by 210Pbex method and pb pollution chronology in the palermo Gulf (Italy). Wat. Air Soil Poll. 202, 109–120. doi: 10.1007/s11270-008-9961-z
Robbins, J. A. (1978). “Geochemical and geophysical applications of radioactive lead,” in Biogeochemistry of Lead in the Environment, ed. J. O. Nriagu (Amsterdam: Elsevier), 285–393.
Sakai, A. K., Allendorf, F. W., Holt, J. S., Lodge, D. M., Molofsky, J., With, K. A., et al. (2001). The population biology of invasive species. Ann. Rev. Ecol. Systemat. 32, 305–332.
Sakalli, A. (2017). Sea surface temperature change in the Mediterranean Sea under climate change: a linear model for simulation of the sea surface temperature up to 2100. Appl. Ecol. Environ. Res. 15, 707–716. doi: 10.15666/aeer/1501_707716
Salihoglu, B., Fach, B. A., and Oguz, T. (2011). Control mechanisms on the ctenophore Mnemiopsis population dynamics: a modeling study. J. Mar. Syst. 87, 55–65. doi: 10.1016/j.jmarsys.2011.03.001
Sardain, A., Sardain, E., and Leung, B. (2019). Global forecasts of shipping traffic and biological invasions to 2050. Nat. Sustain. 2, 274–282. doi: 10.1038/s41893-019-0245-y
Schmidt, C., Morard, R., Prazeres, M., Barak, H., and Kucera, M. (2016a). Retention of high thermal tolerance in the invasive foraminifera Amphistegina lobifera from the Eastern Mediterranean and the Gulf of Aqaba. Mar. Biol. 163:228.
Schmidt, C., Titelboim, D., Brandt, J., Herut, B., Abramovich, S., Almogi-Labin, A., et al. (2016b). Extremely heat tolerant photo-symbiosis in a shallow marine benthic foraminifera. Sci. Rep. 6:30930.
Seebens, H., Blackburn, T. M., Dyer, E. E., Genovesi, P., Hulme, P. E., Jeschke, J. M., et al. (2017). No saturation in the accumulation of alien species worldwide. Nat. Commun. 8:14435.
Skarlato, S., Filatova, N., Knyazev, N., Berdieva, M., and Telesh, I. (2018). Salinity stress response of the invasive dinoflagellate Prorocentrum minimum. Est. Coast. Shelf Sci. 211, 199–207. doi: 10.1016/j.ecss.2017.07.007
Smith, J. N. (2001). Why should we believe 210Pb sediment geochronologies? J. Environ. Radioact. 55, 121–123. doi: 10.1016/s0265-931x(00)00152-1
Sorte, C. J. B., Williams, S. L., and Carlton, J. T. (2010). Marine range shifts and species introductions: comparative spread rates and community impacts. Glob. Ecol. Biogeogr. 19, 303–316. doi: 10.1111/j.1466-8238.2009.00519.x
Spear, M. J., Walsh, J. R., Ricciardi, A., and Vander Zanden, M. J. (2020). The invasion ecology of sleeper populations: prevalence, persistence, and abrupt shifts. Bioscience 71, 357–369. doi: 10.1093/biosci/biaa168
Steen, F., Aragay, J., Zuljevic, A., Verbruggen, H., Mancuso, F. P., Bunker, F., et al. (2017). Tracing the introduction history of the brown seaweed Dictyota cyanoloma (Phaeophyceae, Dictyotales) in Europe. Eur. J. Phycol. 52, 31–42. doi: 10.1080/09670262.2016.1212998
Titelboim, D., Almogi-Labin, A., Herut, B., Kucera, M., Asckenazi-Polivoda, S., and Abramovich, S. (2019). Thermal tolerance and range expansion of invasive foraminifera under climate changes. Sci. Rep. 9, 1–5.
Tylmann, W., Bonk, A., Goslar, T., Wulf, S., and Grosjean, M. (2016). Calibrating 210Pb dating results with varve chronology and independent chronostratigraphic markers: problems and implications. Quat. Geochronol. 32, 1–10. doi: 10.1016/j.quageo.2015.11.004
Tzanova, A., and Herbert, T. D. (2015). Regional and global significance of pliocene sea surface temperatures from the Gulf of Cadiz (Site U1387) and Mediterranean. Glob. Planet. Change 133, 371–377. doi: 10.1016/j.gloplacha.2015.07.001
Walsh, J. R., Munoz, S. E., and Vander Zanden, M. J. (2016). Outbreak of an undetected invasive species triggered by a climate anomaly. Ecosphere 7:e01628.
Witte, S., Buschbaum, C., van Beusekom, J. E. E., and Karsten, R. (2010). Does climatic warming explain why an introduced barnacle finally takes over after a lag of more than 50 years? Biol. Invas. 12:3579. doi: 10.1007/s10530-010-9752-5
Yamamuro, M., and Kanai, Y. (2005). A 200-year record of natural and anthropogenic changes in water quality from coastal lagoon sediments of Lake Shinji, Japan. Chem. Geol. 218, 51–61. doi: 10.1016/j.chemgeo.2005.01.021
Yokeş, M. B., Meriç, E., and Avşar, N. (2007). On the presence of alien foraminifera Amphistegina lobifera larsen on the coasts of the Maltese Islands. Aquat. Invas. 2, 439–441. doi: 10.3391/ai.2007.2.4.15
Zenetos, A., Gratsia, E., Cardoso, A., and Tsiamis, K. (2019). Time lags in reporting of biological invasions: the case of Mediterranean Sea. Mediterr. Mar. Sci. 20, 469–475. doi: 10.12681/mms.20716
Keywords: sea warming, SST, invasive species, foraminifera, radiometric dating, Mediterranean Sea, lessepsian invasion
Citation: Guastella R, Marchini A, Caruso A, Evans J, Cobianchi M, Cosentino C, Langone L, Lecci R and Mancin N (2021) Reconstructing Bioinvasion Dynamics Through Micropaleontologic Analysis Highlights the Role of Temperature Change as a Driver of Alien Foraminifera Invasion. Front. Mar. Sci. 8:675807. doi: 10.3389/fmars.2021.675807
Received: 03 March 2021; Accepted: 14 May 2021;
Published: 07 June 2021.
Edited by:
Jason Michael Hall-Spencer, University of Plymouth, United KingdomReviewed by:
Alexa Fredston, Rutgers, The State University of New Jersey, United StatesCopyright © 2021 Guastella, Marchini, Caruso, Evans, Cobianchi, Cosentino, Langone, Lecci and Mancin. This is an open-access article distributed under the terms of the Creative Commons Attribution License (CC BY). The use, distribution or reproduction in other forums is permitted, provided the original author(s) and the copyright owner(s) are credited and that the original publication in this journal is cited, in accordance with accepted academic practice. No use, distribution or reproduction is permitted which does not comply with these terms.
*Correspondence: Agnese Marchini, YWduZXNlLm1hcmNoaW5pQHVuaXB2Lml0
Disclaimer: All claims expressed in this article are solely those of the authors and do not necessarily represent those of their affiliated organizations, or those of the publisher, the editors and the reviewers. Any product that may be evaluated in this article or claim that may be made by its manufacturer is not guaranteed or endorsed by the publisher.
Research integrity at Frontiers
Learn more about the work of our research integrity team to safeguard the quality of each article we publish.