- 1Center for Marine and Environmental Studies, University of the Virgin Islands, St. Thomas, VI, United States
- 2Mote Marine Laboratory, Sarasota, FL, United States
- 3Department of Oceanography and Coastal Sciences, Louisiana State University, Baton Rouge, LA, United States
- 4Department of BioSciences, Rice University, Houston, TX, United States
- 5Department of Biology, University of Texas at Arlington, Arlington, TX, United States
- 6Woods Hole Oceanographic Institution, Woods Hole, MA, United States
Stony coral tissue loss disease (SCTLD) was initially documented in Florida in 2014 and outbreaks with similar characteristics have since appeared in disparate areas throughout the northern Caribbean, causing significant declines in coral communities. SCTLD is characterized by focal or multifocal lesions of denuded skeleton caused by rapid tissue loss and affects at least 22 reef-building species of Caribbean corals. A tissue-loss disease consistent with the case definition of SCTLD was first observed in the U.S. Virgin Islands (USVI) in January of 2019 off the south shore of St. Thomas at Flat Cay. The objective of the present study was to characterize species susceptibility to the disease present in St. Thomas in a controlled laboratory transmission experiment. Fragments of six species of corals (Colpophyllia natans, Montastraea cavernosa, Orbicella annularis, Porites astreoides, Pseudodiploria strigosa, and Siderastrea siderea) were simultaneously incubated with (but did not physically contact) SCTLD-affected colonies of Diploria labyrinthiformis and monitored for lesion appearance over an 8 day experimental period. Paired fragments from each corresponding coral genotype were equivalently exposed to apparently healthy colonies of D. labyrinthiformis to serve as controls; none of these fragments developed lesions throughout the experiment. When tissue-loss lesions appeared and progressed in a disease treatment, the affected coral fragment, and its corresponding control genet, were removed and preserved for future analysis. Based on measures including disease prevalence and incidence, relative risk of lesion development, and lesion progression rates, O. annularis, C. natans, and S. siderea showed the greatest susceptibility to SCTLD in the USVI. These species exhibited earlier average development of lesions, higher relative risk of lesion development, greater lesion prevalence, and faster lesion progression rates compared with the other species, some of which are considered to be more susceptible based on field observations (e.g., P. strigosa). The average transmission rate in the present study was comparable to tank studies in Florida, even though disease donor species differed. Our findings suggest that the tissue loss disease affecting reefs of the USVI has a similar epizootiology to that observed in other regions, particularly Florida.
Introduction
The emergence and outbreaks of various coral diseases have led to drastic declines in both coral cover and diversity over the last several decades (Aronson and Precht, 2001; Miller et al., 2009; Walton et al., 2018; Alvarez-Filip et al., 2019). However, no disease documented to date has been as persistent, widespread, and has affected as many species as stony coral tissue loss disease (SCTLD). SCTLD was first observed in Florida off the coast of Miami-Dade County in 2014 and was initially described as a white plague disease outbreak (Precht et al., 2016; Walton et al., 2018). White plague is a rapid tissue loss disease that affects many species of scleractinian corals and was previously one of the largest drivers of modern Caribbean coral community change (Miller et al., 2009). However, due to differences in the species affected by the disease, as well as faster associated rates of lesion progression, lower probability of colony survival, and a lack of seasonal restriction, SCTLD was distinguished from white plague disease and identified as a more severe disease of Caribbean corals (Walton et al., 2018).
Known to affect at least 22 Caribbean reef building species of coral (NOAA, 2018), SCTLD is having an unprecedented impact to affected reefs. Spatial modeling of disease reports has suggested that SCTLD is both contagious and water-borne (Dobbelaere et al., 2020; Muller et al., 2020). Ex situ transmission experiments have also shown both inter- and intra-species transmission via direct contact and/or shared water with a diseased colony (Aeby et al., 2019; Eaton and Muller, 2019). These previously conducted transmission experiments and field observations have demonstrated differences in susceptibility among species, based on incidence rates and lesion progression rates (Sharp and Maxwell, 2018; Aeby et al., 2019). Long term field-monitoring stations in Florida showed significant declines in coral density and diversity, with different disease prevalence and survivorship among species (Walton et al., 2018; Sharp et al., 2020). Additionally, small scale epidemiology suggests there is no effect of coral density on disease prevalence, but larger colonies are more likely to become affected (Sharp et al., 2020).
Four years after its initial detection in the Florida region, outbreaks of tissue loss on scleractinian corals with signs similar to SCTLD began emerging at various disparate locations across the Caribbean basin (Kramer et al., 2019). The disease was documented first in the Mexican Caribbean on the windward coast of Cozumel beginning in 2018 (Alvarez-Filip et al., 2019). SCTLD spread throughout the Mexican Caribbean rapidly and manifested on reefs affecting species in similar patterns as Florida, except that Agaricia agaricites and Porites astreoides exhibited low disease prevalence in the Mexican Caribbean (Alvarez-Filip et al., 2019; Estrada-Saldivar et al., 2020), but were not affected in Florida (NOAA, 2018).
A disease matching the phenotypic description of SCTLD was observed in the U.S. Virgin Islands (USVI) off the south shore of St. Thomas at Flat Cay in January 2019. This presented an opportunity to test species susceptibility in a relatively naive population, with the goal of experimentally quantifying species susceptibility to SCTLD. Using a laboratory-based transmission experiment following the framework of Williams et al. (2020), we tested coral susceptibility to SCTLD exposure. Susceptibility was characterized by lesion prevalence, time to lesion development, risk of lesion development, and lesion progression rates. We hypothesized that the species challenged with disease in the laboratory would exhibit different susceptibilities to SCTLD. We expected there would be variation in the lesion prevalence, time to development, relative risk, and progression rates among species (NOAA, 2018).
Materials and Methods
This study was conducted at the Center for Marine and Environmental Sciences (CMES), located on the St. Thomas campus of the University of the Virgin Islands in the USVI. All experimental corals were collected from reefs surrounding St. Thomas at depths shallower than 15 m.
Healthy coral colonies (ntotal = 60, nspecies = 10) from 6 species (Colpophyllia natans, Montastraea cavernosa, Orbicella annularis, Porites astreoides, Pseudodiploria strigosa, and Siderastrea siderea), no larger than 25 cm × 25 cm, were collected from Rupert’s Rock (18°19′39.6ʺN 64°55′33.5ʺW) by divers on SCUBA with hammers and chisels (22 and 26 March 2019). Prior to the collection of the corals, the site was scouted for any signs of SCTLD, or other rapid tissue loss diseases, and no disease was observed. Colonies of the same species were collected at least 5 m apart and of different phenotypes when available to maximize intraspecies genetic variation. Coral colonies were placed in individual bags that were sealed and then transported to CMES in coolers with seawater. Once at CMES, the corals were fragmented in half with a sterilized chop saw or bandsaw, given identification numbers, photographed, then placed into running seawater tables under shade. Coral fragments were allowed to acclimate for at least 1 week prior to the experiment. Any corals that appeared unhealthy (e.g., exhibiting bleaching, fragmentation stress, discoloration) during the acclimation period were not used in the experiment. One day prior to the start of the experiment (3 April 2019), ten healthy colonies of Diploria labyrinthiformis (approximately 20 cm × 20 cm) were collected from Rupert’s Rock to be utilized as controls (the site was again scoped for SCTLD, and no SCTLD was observed). Simultaneously, a separate team of divers on SCUBA collected SCTLD-affected colonies of D. labyrinthiformis from the Flat Cay fringing reef (18°19′02.9ʺN 64°59′27.0ʺW). SCTLD-affected corals were identified based on the appearance of large, multifocal lesions consistent with the case description of SCTLD (NOAA, 2018). All corals, both apparently healthy and SCTLD-affected, were removed using hammers and chisels, placed in individual gallon bags, and then transported to CMES and kept separate from all other experimental corals until the experiment commenced the following day. Diseased corals were observed for 24 h to confirm expansion of tissue loss, indicating active disease lesions. All diseased D. labyrinthiformis corals exhibited active lesion expansion and no healthy (control) D. labyrinthiformis corals exhibited lesion development over the duration of the experiment.
The present study applied the experimental methodology developed by Williams et al. (2020) and applied by MacKnight et al. (in press). For the control treatment, fragments of six coral species (1 fragment each of C. natans, M. cavernosa, O. annularis, P. astreoides, P. strigosa, and S. siderea) were arranged in a random order at equal distances (with no physical contact with other corals) around an apparently healthy colony of D. labyrinthiformis (Figure 1A). For the disease treatment, corresponding fragments of each genet of the six experimental species were then arranged in a random order (with no physical contact with other corals) around a SCTLD-affected D. labyrinthiformis colony (Figure 1B). This paired design was replicated eight times. All containers (26 L) were filled with filtered seawater, equipped with an air stone, and randomly arranged among three outdoor shaded seawater tables with chilled running seawater to maintain a constant temperature (∼28°C). Seawater at CMES is first pumped from Brewer’s Bay up to a sediment settling cistern, then travels by gravity flow to a second sediment settling tank. Next, water is pumped through a filtration system (20 μm pleated sediment filter) that includes ultraviolet light (UV) exposure (80 watt then 40 watt at tables) and then finally out to the running seawater tables. Water in the containers was changed daily (100%) and the containers were then randomly re-arranged among the three running seawater tables. The coral fragment locations within the containers were also randomly rearranged around the central coral each day.
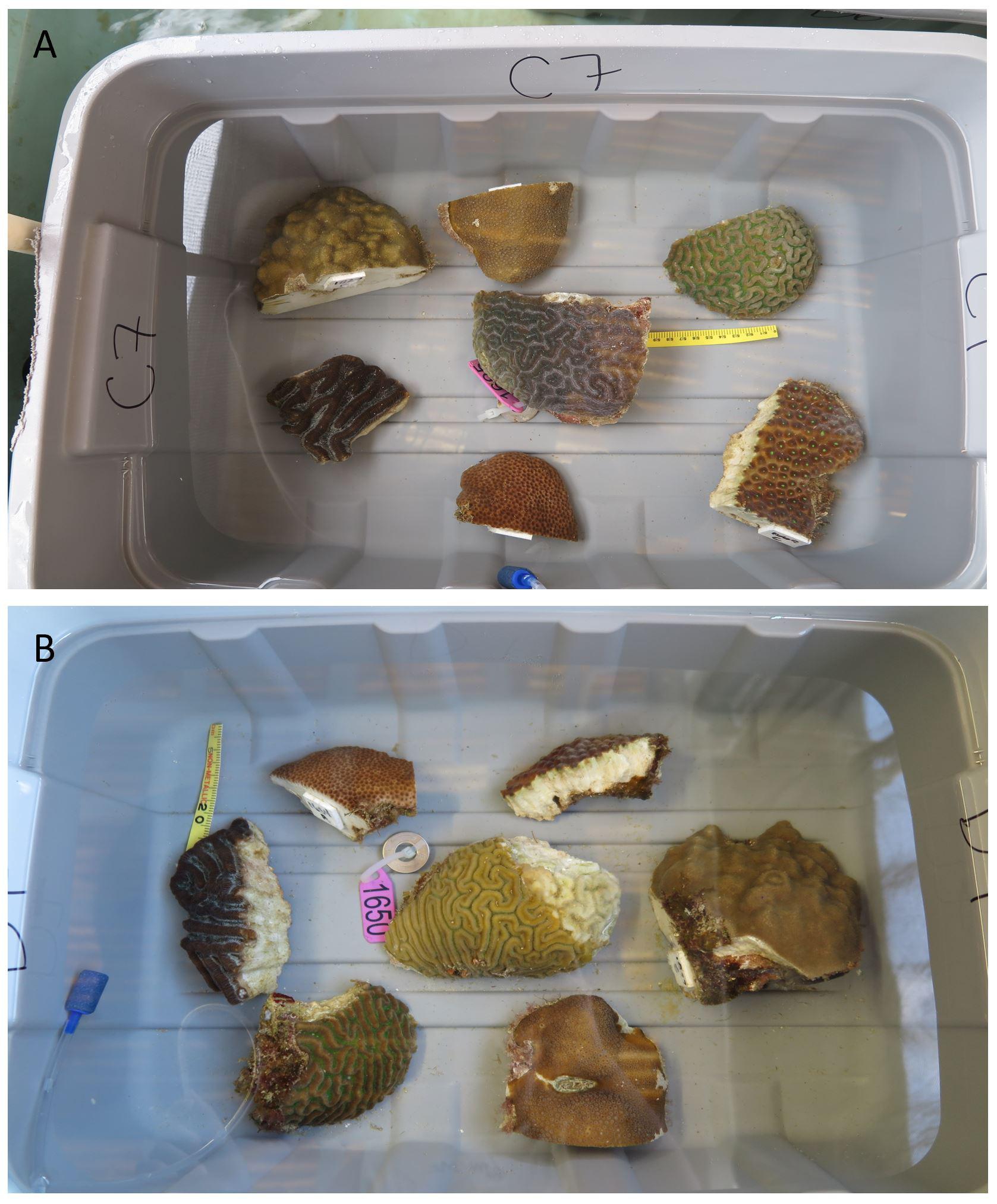
Figure 1. Experimental Design. (A) One control replicate containing an air stone, ruler, a visually healthy central colony of Diploria labyrinthiformis, and fragments of 6 different species: Colpophyllia natans, Montastraea cavernosa, Orbicella annularis, Porites astreoides, Pseudodiploria strigosa, and Siderastrea siderea. (B) One disease exposure replicate containing an air stone, ruler, a diseased central colony of D. labyrinthiformis with lesion signs matching SCTLD, and fragments of 6 species. The central coral is a distinct genotype from the D. labyrinthiformis colony in the control replicate (A), but the experimental fragments surrounding it are the same genets used in the control replicate (A). This paired design was replicated with 8 distinct sets of experimental genets.
Corals were examined and photographed twice daily (morning and afternoon) to identify the emergence of lesions indicative of SCTLD and assess the visual health of corals. When a lesion appeared, the affected coral was monitored at more frequent intervals to determine whether the lesion was actively expanding and appeared consistent with SCTLD (Figure 2). If a lesion was determined to be expanding for at least 12 h, the fragment was photographed, removed from the experimental container, and processed for future analyses. The corresponding control fragment was removed at the same time and processed identically. The experiment was run for 8 days, at which point, all remaining fragments were processed as above.
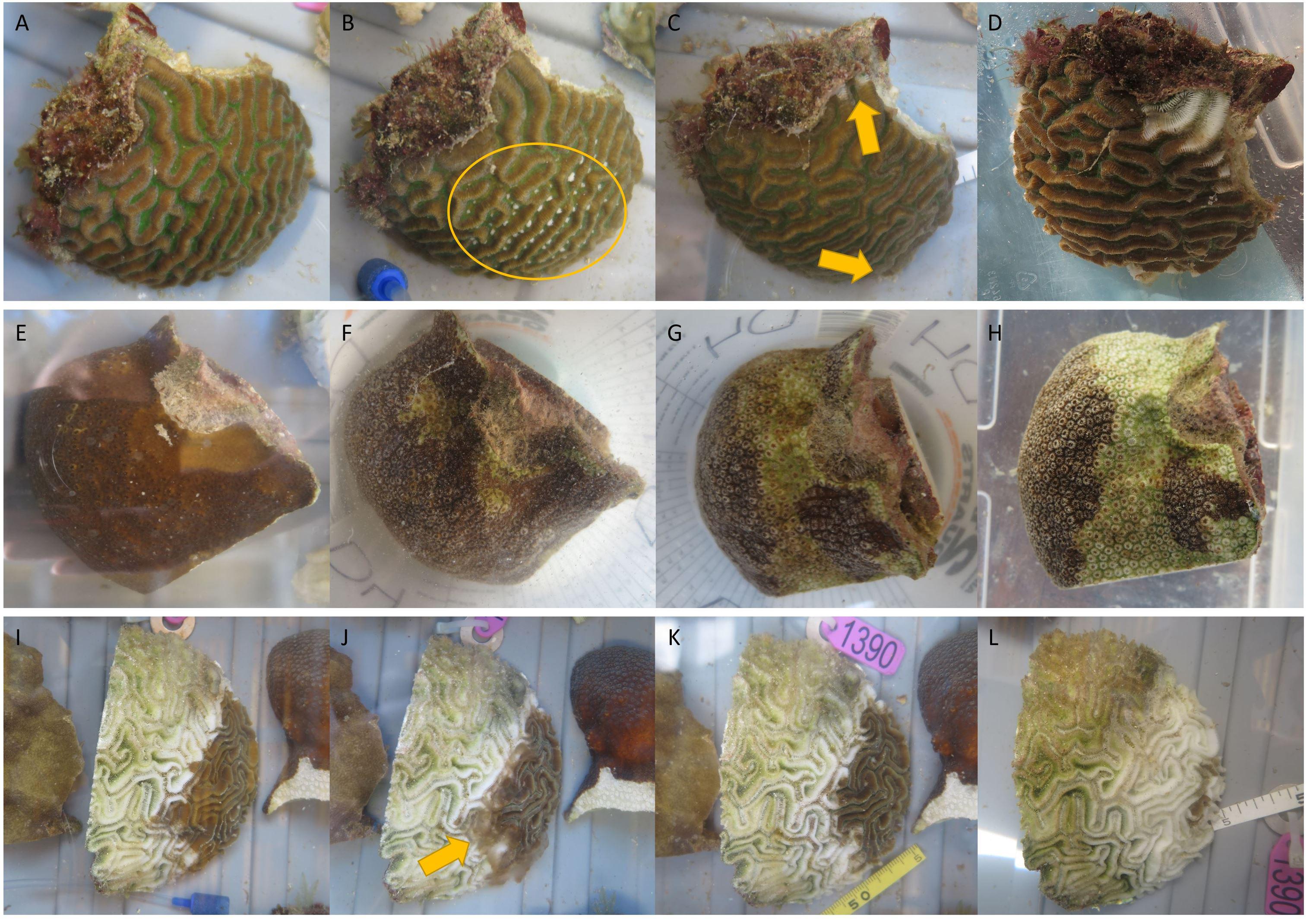
Figure 2. Rapid expansion of lesions on a Pseudodiploria strigosa fragment (A–D) and an Orbicella annularis fragment (E–H) exposed to stony coral tissue loss disease (SCTLD; exemplified by the central colony of diseased Diploria labyrinthiformis in I–L). (A) Day 0, fragment is visually healthy. (B) Day 4, fragment has mesentery filaments extended suggesting signs of stress (yellow circle). (C) Day 5, fragment develops tissue loss (yellow arrows). (D) Day 6, both lesions progressed. (E) Day 0, fragment is visually healthy. (F) Day 1, fragment is partially bleached and develops tissue loss. (G) Day 2 morning, lesion progressed and bleaching area has increased. (H) Day 2 afternoon, lesion progressed and bleaching area has increased. (I) Day 1, colony has signs of rapid tissue loss suspected to be SCTLD. (J) Day 2 morning pre-water change, lesion has progressed and sloughed tissue resides immediately above the skeleton (yellow arrow). (K) Day 2 morning post-water change, recently dead tissue has been removed from the skeleton. (L) Day 5, lesion has continued to rapidly progress.
Dates of the first observation of disease lesions on corals were used to calculate and compare disease incidence with Kaplan-Meier plots and log-rank analysis using the “survfit” and “survdiff” functions within the “survival” R package (Therneau, 2019). Fragments exposed to a diseased central colony that never showed signs of SCLTD were censored in analyses. An identical survival analysis was performed using the hours to infection, however, are not reported here because the trends in the results were identical to the analysis comparing lesion development among days.
The number of fragments with active lesions on each day was used to calculate species-specific disease prevalence. Fragments considered diseased and removed for further processing were included in a cumulative total of prevalence, assuming that if they had not been removed, they would continue to be diseased. Using a Fisher’s Exact test with simulated P-values in R, we analyzed whether there were significant differences in prevalence values among species.
Using the photo of the first observation of a lesion and the last photo of the fragment before processing, we calculated the lesion progression rate (cm2/day) for each fragment that showed signs of SCTLD. Within the program National Institutes of Health Image J (Schneider et al., 2012), a scale was established using a ruler placed within the frame of each photo. Due to slightly different heights and angles of photos, scales were inconsistent across timepoints when using the rulers. Thus, using the ruler only in the initial photo, a measurement on the fragment was selected that could be used as a standardized scale in sequential photos. For areal progression rates, we measured the area of each lesion for a single timepoint and added them together to calculate total fragment areal tissue loss. These same distances and areas were re-measured using the scale standardized on the coral fragment for the photograph at the final time point. Areal lesion progression rates were standardized by living tissue surface area at the previous timepoint to calculate proportional lesion progression rates.
Areal progression rates were log transformed to meet statistical test assumptions, and then an Analysis of Variance (ANOVA) was used to test for differences in lesion progression rates among species. A Tukey-HSD post hoc analysis was used to determine pair-wise differences among species. These same analyses, including the log transformation, were conducted using the proportional lesion progression rates. Analysis of Covariance (ANCOVA) tests were initially run using fragment size (cm) as a covariate; however, there was no effect of size on total nor proportional lesion progression rates. Therefore, only the ANOVA results are reported here. D. labyrinthiformis lesion progression rates were excluded from these tests because they developed SCTLD lesions in the field rather than within the laboratory experiment. The relative risk of each species was calculated to determine whether there was a significant risk of developing lesions after exposure to SCTLD. Here, the relative risk was identified as the risk in exposed individuals developing signs of SCTLD compared with the risk in non-exposed individuals developing signs of SCTLD throughout the 8-day experiment. Therefore, we examined relative risk using the following equation:
where a was the number of fragments showing signs of SCTLD after exposure to a diseased colony, b was the number of fragments showing no signs of SCTLD after being exposed to a diseased colony, c was the number of fragments showing signs of SCTLD after being exposed to an apparently healthy control colony, and d was the number of fragments showing no signs of SCTLD after being exposed to an apparently healthy control colony. Relative risk was calculated using a Bayesian approach (Gelman et al., 2004; Lawson, 2009) and was estimated using a binomial likelihood distribution and a uniform-Beta prior distribution. To obtain an estimate of relative risk, Markov chain Monte Carlo simulations (100,000 iterations with a burn-in of 10,000) were used with Gibbs sampling in OpenBUGS. A 95% credible interval was calculated for each estimate of relative risk. Credible intervals that did not include a value of one were considered significant, with a credible interval above one signifying a higher risk of a species showing signs of SCTLD after exposure to a diseased colony. A credible interval below one signified a reduced risk of showing signs of SCTLD after exposure to a diseased colony. To determine whether the relative risk of species differed from each other, we conducted a risk ratio analysis using the pairwiseCI R package (Schaarschmidt and Gerhard, 2019) with the “Prop.ratio” method. This approach conducted a pairwaise comparison of the relative risk of one species to another with a Bonferroni correction. Similar to the Bayesian relative risk analysis, a pairwise risk ratio with a confidence interval above the value of 1 indicates a significantly higher risk of SCTLD, whereas a confidence interval below the value of 1 indicates a significantly lower risk of SCTLD. When the confidence interval spanned the value of 1 the two species did not significantly differ in their risk to SCTLD.
For histological analysis, a subsample of the diseased (ensuring to include the lesion edge when available) or control fragment was placed into Z-fix (Anatech Ltd.) diluted 1:4 in filtered seawater for at least 24 h. It was then rinsed with freshwater for 12 h and transferred to ethanol for transport to Louisiana State University. Samples were decalcified in 1% EDTA decalcifier solution and stored in 70% EtOH until processing using a Leica ASP6025 tissue processor, embedded in wax blocks on a Leica EG1150H embedding machine, and sectioned (five slides per sample) at 4 micron thickness on a Leica RM2125RTS microtome. Slides were stained with hematoxylin and eosin on a Leica ST5020 and analyzed on an Olympus BX41 microscope with an Olympus SC180 camera attachment. 145 total coral samples were histologically processed and analyzed (C. natans 15 DD, 7 HD, 15 HH; D. labyrinthiformis: 4 DD, 8 HH; M. cavernosa 8 DD, 8 HH; O. annularis 11 DD, 2 HD, 9 HH; Porites astreoides 8 DD, 10 HH; Pseudodiploria strigosa 9 DD, 2 HD, 10 HH; Siderastrea siderea 9 DD, 7 HH). Histopathological images were analyzed for symbiont vacuolization and exocytosis, measured within randomly selected gridded image subsections. Vacuolization was measured as the ratio of 2-dimensional symbiont cell area to vacuole area for 25 symbiont cells per sample, and exocytosis was measured as the proportion of total symbiont cells outside of a visible vacuole. An effect of sample health condition was tested on sample mean vacuolization using a one-way ANOVA, and on sample mean exocytosis using a beta regression in RStudio. Slides were also assessed for gastrodermal separation, body wall breakage, and necrosis.
Results
All the visually healthy D. labyrinthiformis colonies remained healthy for the duration of the experiment, while all diseased D. labyrinthiformis colonies had rapidly progressing lesions indicative of SCTLD. No fragments exposed to apparently healthy corals developed visual signs of lesions, or evidence of SCTLD. The majority (77.1%) of fragments exposed to diseased corals developed active tissue loss lesions during the experiment. Visually, the lesions on experimental fragments exposed to disease developed liquefactive lesions, some of which were multifocal. Prior to water changes, sloughed tissue sat above the coral skeleton at the lesion borders (Figure 2J) indicating rapid progression and minimal water circulation within the experimental containers.
Cumulative disease prevalence varied among species from 100% in C. natans and O. annularis to 37.5% in M. cavernosa (Figure 3), but did not significantly differ among species (p = 1; Figure 3). However, disease incidence differed among species (X2 = 17.6, p = 0.003; Figure 4). Overall, fragments exposed to diseased corals developed lesions at a median of 6 days after exposure. There were pairwise differences in incidence of lesion development between M. cavernosa and O. annularis as well as M. cavernosa and C. natans (p < 0.05), with M. cavernosa having the slowest incidence within both pairs. O. annularis fragments had the lowest median time to lesion development at 4 days, while P. astreoides fragments had the highest median time to lesion development at 7.5 days (Figure 4). An insufficient number of fragments of M. cavernosa developed lesions (n = 3) to calculate an average time to lesion development.
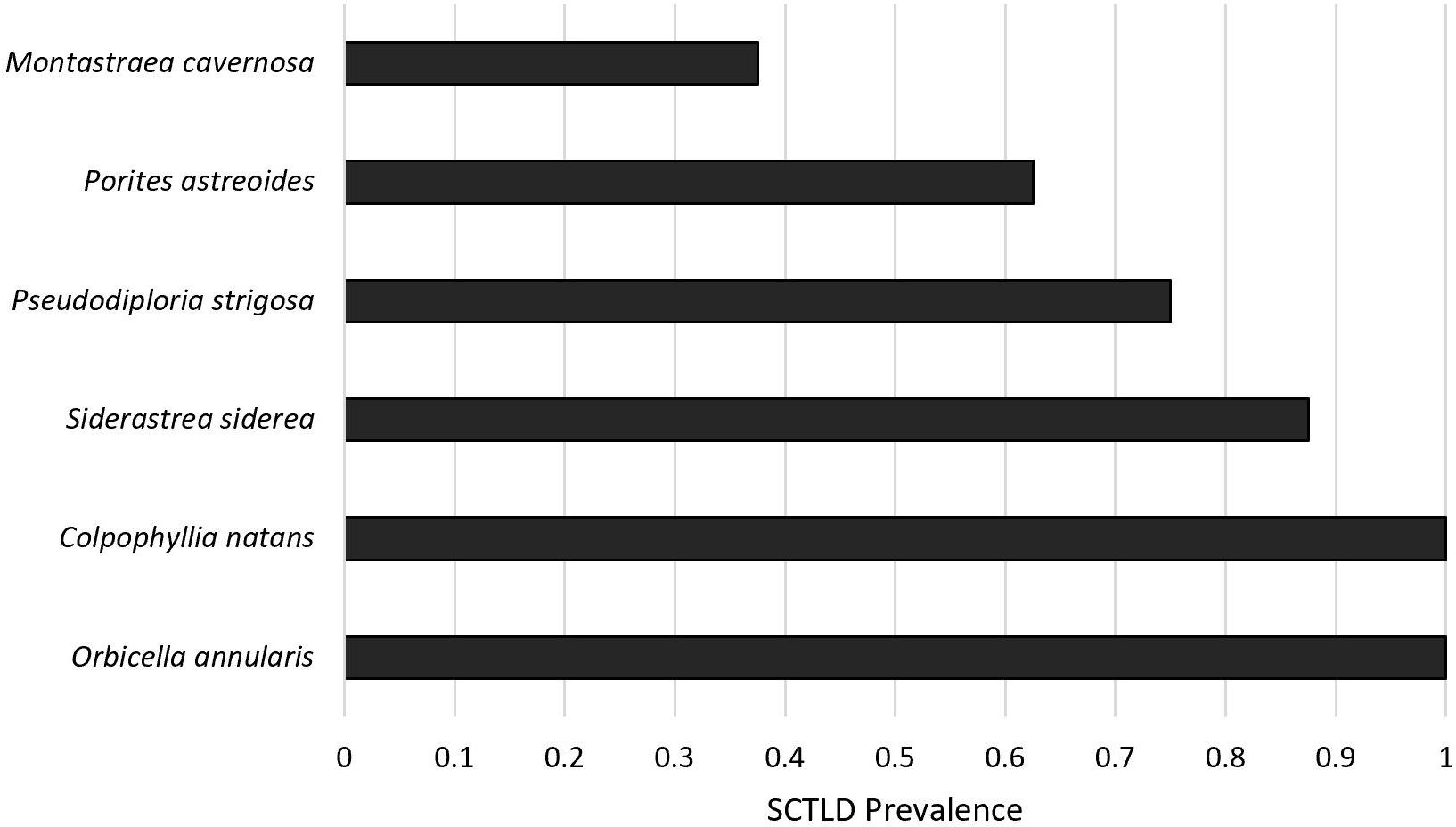
Figure 3. Disease prevalence. Proportion of healthy fragments (n = 8 per species) exposed to a diseased Diploria labyrinthiformis that developed lesions corresponding to stony coral tissue loss disease (SCTLD) after 8 days.
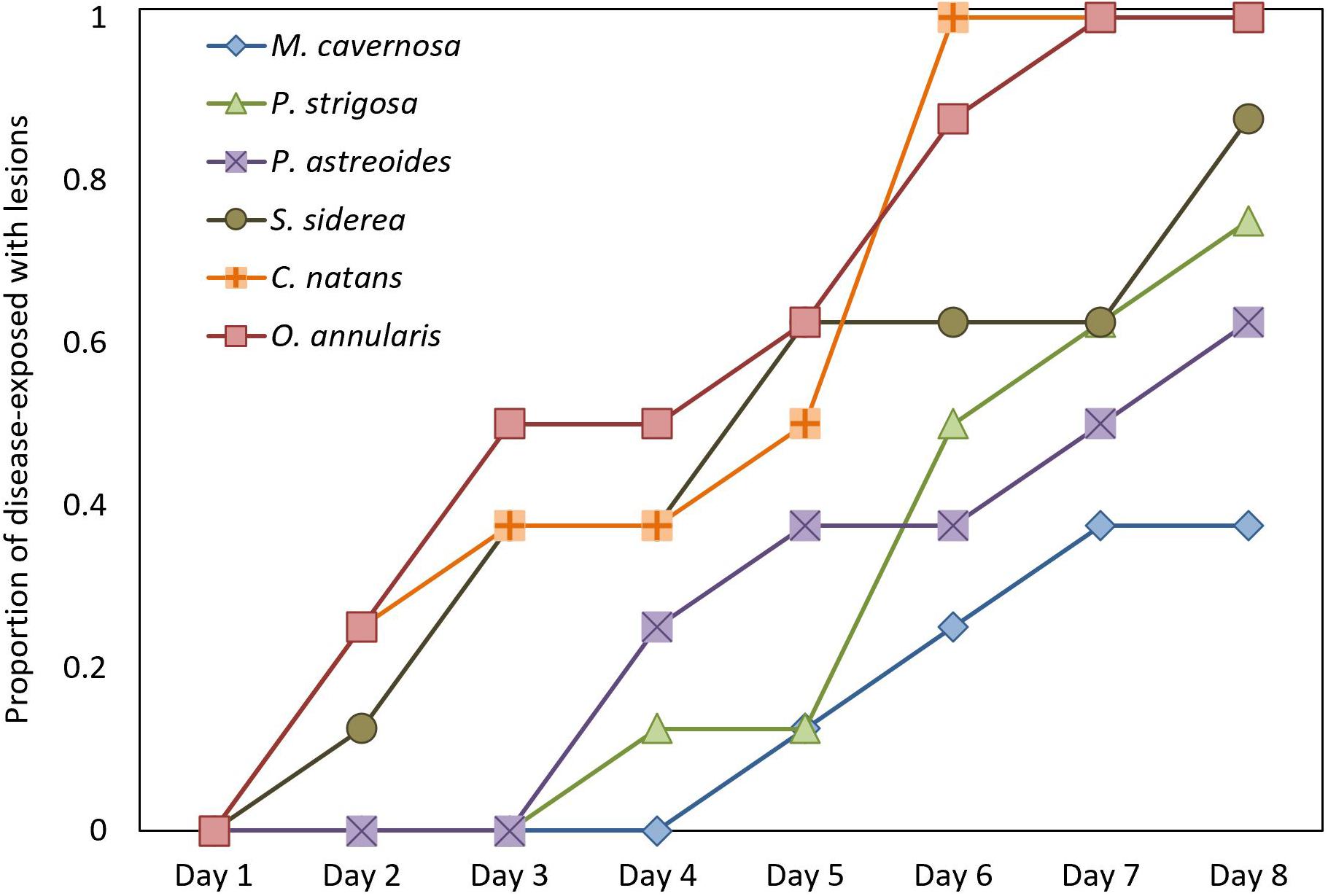
Figure 4. Disease Incidence. Time to appearance of disease lesions consistent with stony coral tissue loss disease (SCTLD) on healthy fragments exposed to a SCTLD-affected colony of Diploria labyrinthiformis (n = 8 per species).
The relative risk assessment showed that there was a significant increase in risk of developing lesions for most species tested after exposure to SCTLD. Although M. cavernosa trended toward elevated levels of risk to SCTLD after exposure, it was the only species in which the credible interval spanned the value of 1 (Figure 5) and, therefore, was not significantly different from controls. However, three of the eight replicates of this species showed disease signs after exposure, suggesting that M. cavernosa is indeed susceiptible. However, the low number of replicates likely resulted in a lack of statistical power. Additionally, the pairwise risk ratio for species comparison showed there was a significantly higher risk of SCTLD for C. natans (median: 2.67, 95% CI = 1.42–7.31), O. annularis (median: 2.67, 95% CI = 1.42–7.31), and S. siderea (median: 2.33, 95% CI = 1.05–6.53) when compared with M. cavernosa (Figure 6). Although all species did not significantly differ from each other, we used their median relative risk to qualitatively compare among species. C. natans (median risk: 12.19) and O. annularis (median risk: 12.09) had the greatest risk of developing SCTLD-type lesions after exposure. An intermediate risk was detected for P. astreoides (median risk: 7.91), P. strigosa (median risk: 9.30), and S. siderea (median risk: 10.63), whereas M. cavernosa (median risk: 5.10) showed the least risk of developing SCTLD-type lesions and was statistically at less risk compared with three other species.
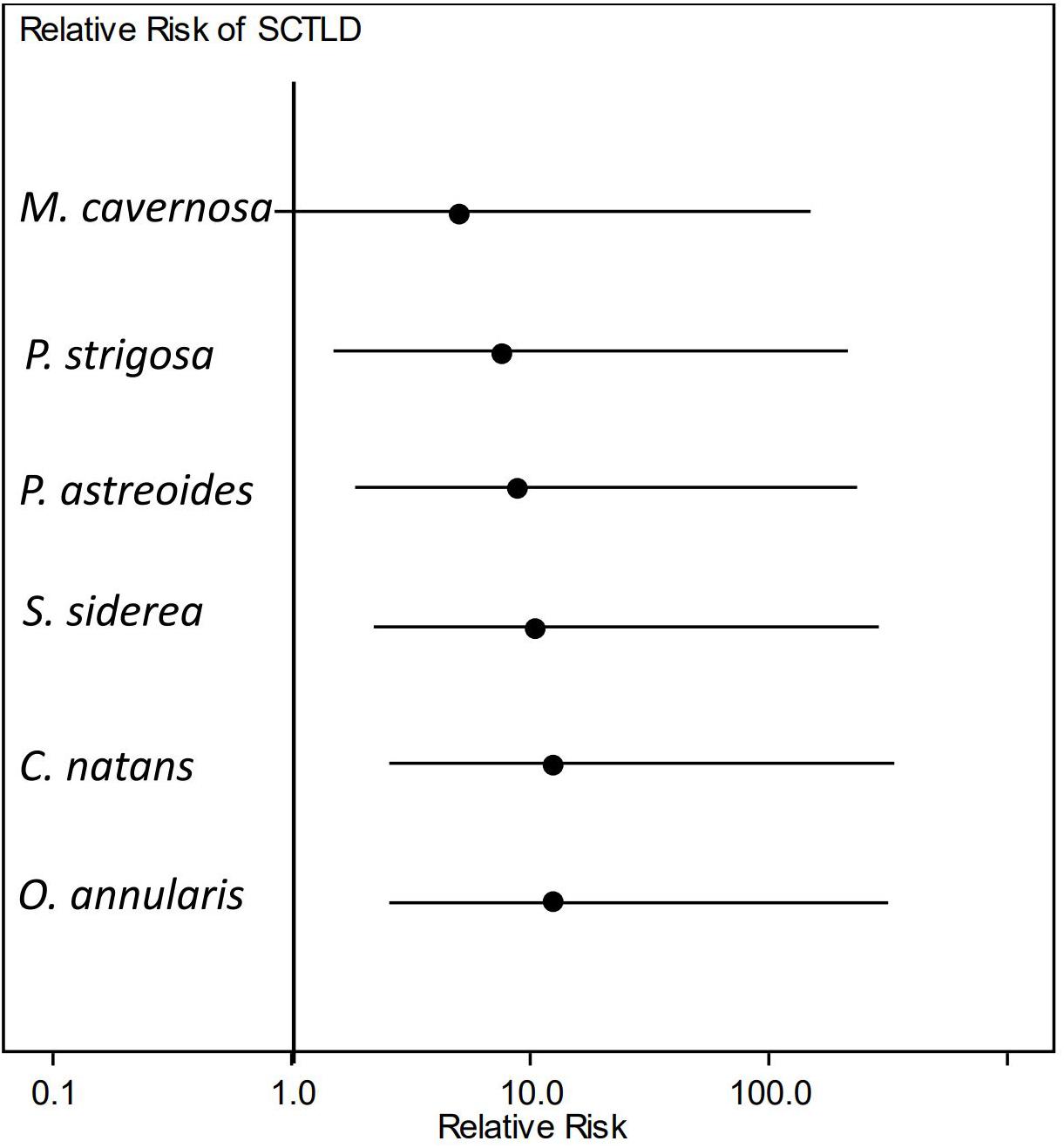
Figure 5. Relative risk of stony coral tissue loss disease (SCTLD) incidence. Dots represent the median and lines represent the credible intervals for relative risk of developing lesions consistent with SCTLD after exposure to a diseased coral. Only Montastraea cavernosa showed no significant elevated risk of SCTLD-type lesion development when compared with controls.
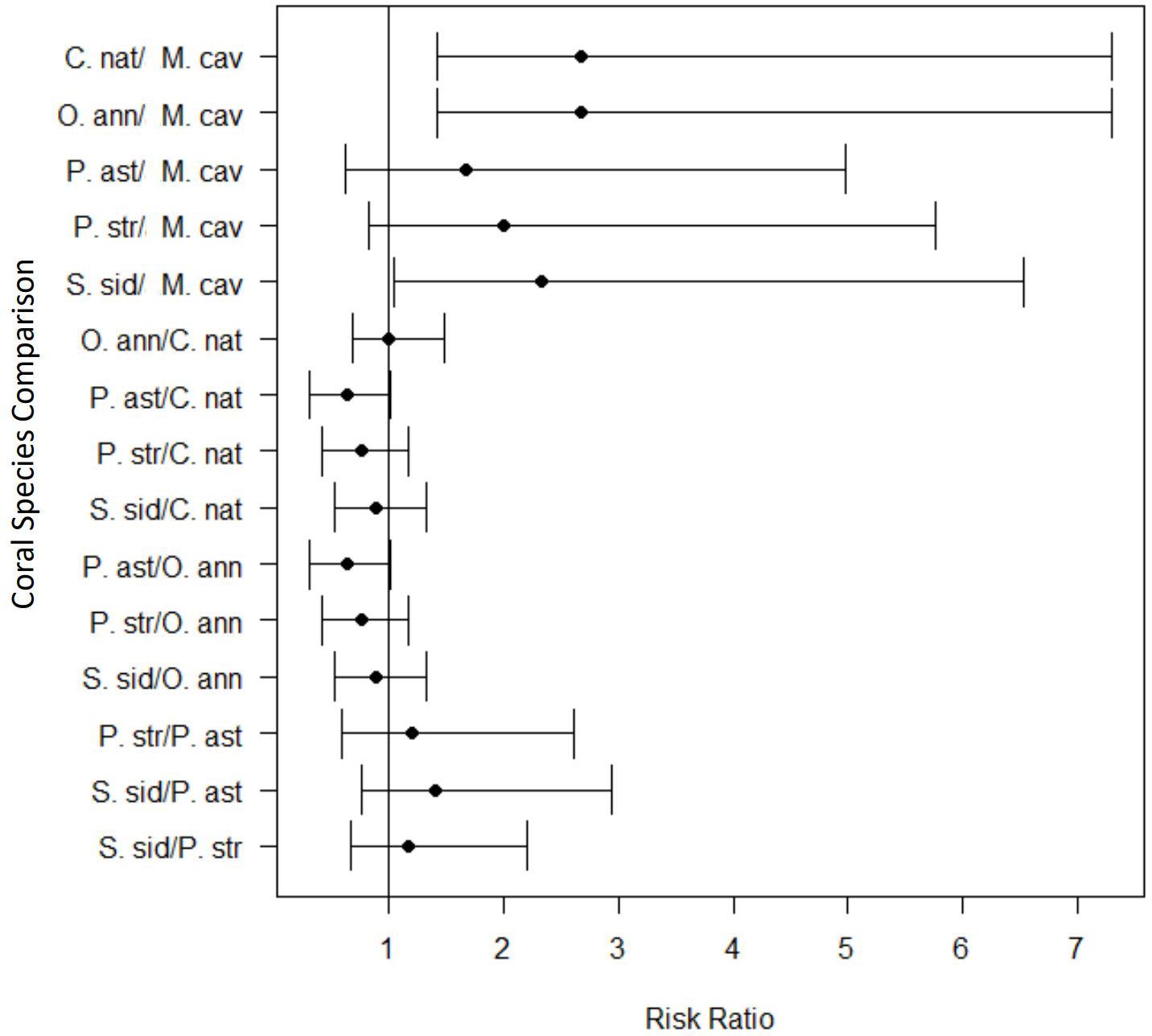
Figure 6. Pairwise comparisons of the risk ratio for each coral species exposed to stony coral tissue loss disease within the transmission experiment. The black circles represent the mean risk ratio and the brackets represent the 95% confidence interval. The vertical black line identifies the risk value of one. When the 95% confidence interval spans this line, there is no significant difference in the risk of lesion development between the two species tested. Values completely above the line indicate a significantly higher risk of lesion development for the first species listed in the pairwise comparison. Values completely below the line indicate a lower risk of lesion development for the first species listed in the pairwise comparison.
There was a significant difference in lesion progression rates among species (F = 2.622, p = 0.046, Figure 7A). C. natans had the fastest average (±SEM) lesion progression rate of 7.03 ± 3.93 cm2/day and P. astreoides had the slowest average lesion progression rate of 0.84 ± 0.37 cm2/day. However, there were no statistical pair-wise differences between species (Tukey HSD post hoc α = 0.05). Although not included in statistical analyses because they contracted SCTLD from field transmission, the average lesion progression rate of D. labyrinthiformis was 1.64 ± 0.17 cm2/day. There were no differences in proportional lesion progression rates among species (F = 2.051, p = 0.102, Figure 7B).
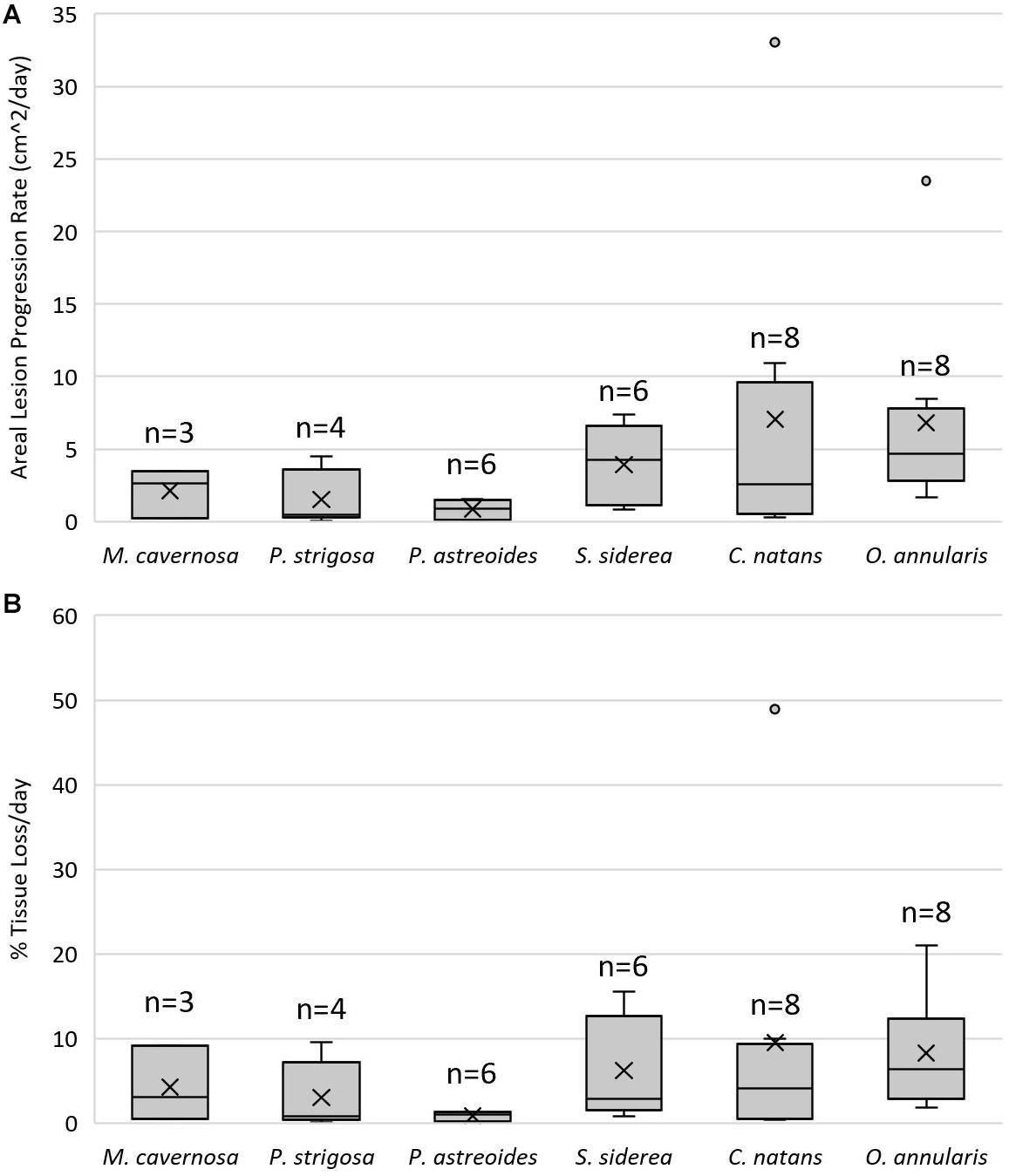
Figure 7. Lesion progression rates. Lower and upper error lines represent the 10th and 90th percentiles, respectively. Shaded boxes represent 25th and 75th percentiles, intersected by a line marking the median. “X” marks the mean and shaded circles represent data outside the 90th percentile. (A) Areal lesion progression rates (cm2/day) on coral fragments that exhibited lesions after being exposed to a stony coral tissue loss disease (SCTLD)-affected colony of Diploria labyrinthiformis. (B) Percent tissue loss per day standardized by healthy tissue area (proportional lesion progression rate) of coral fragments that exhibited lesions after being exposed to a SCTLD-affected colony of D. labyrinthiformis.
Histological analysis confirmed tissue responses as seen in the case definition and other reports from the Florida Keys (Landsberg et al., 2020), in which diseased tissue had high levels of liquifying necrosis (Figures 8E,F). The analysis revealed significantly increased vacuolization surrounding Symbiodiniaceae in diseased corals (measured as a ratio of symbiont cell area to vacuole area, p < 0.001; Figures 8A,B,D,E,F,G,H), and the proportion of exocytosis of symbiont cells (p < 0.05; Figures 8B,C,E,F,H,I). Surface body wall breakage was seen in diseased corals (Figures 8F,I), as well as gastrodermal separation (Figures 8B,C,E,F,G). While all tissue signs were seen in all three tissue health conditions (Figure 8), the most severe and most common were from diseased portions of diseased colonies (Figures 8C,E,F,H,I).
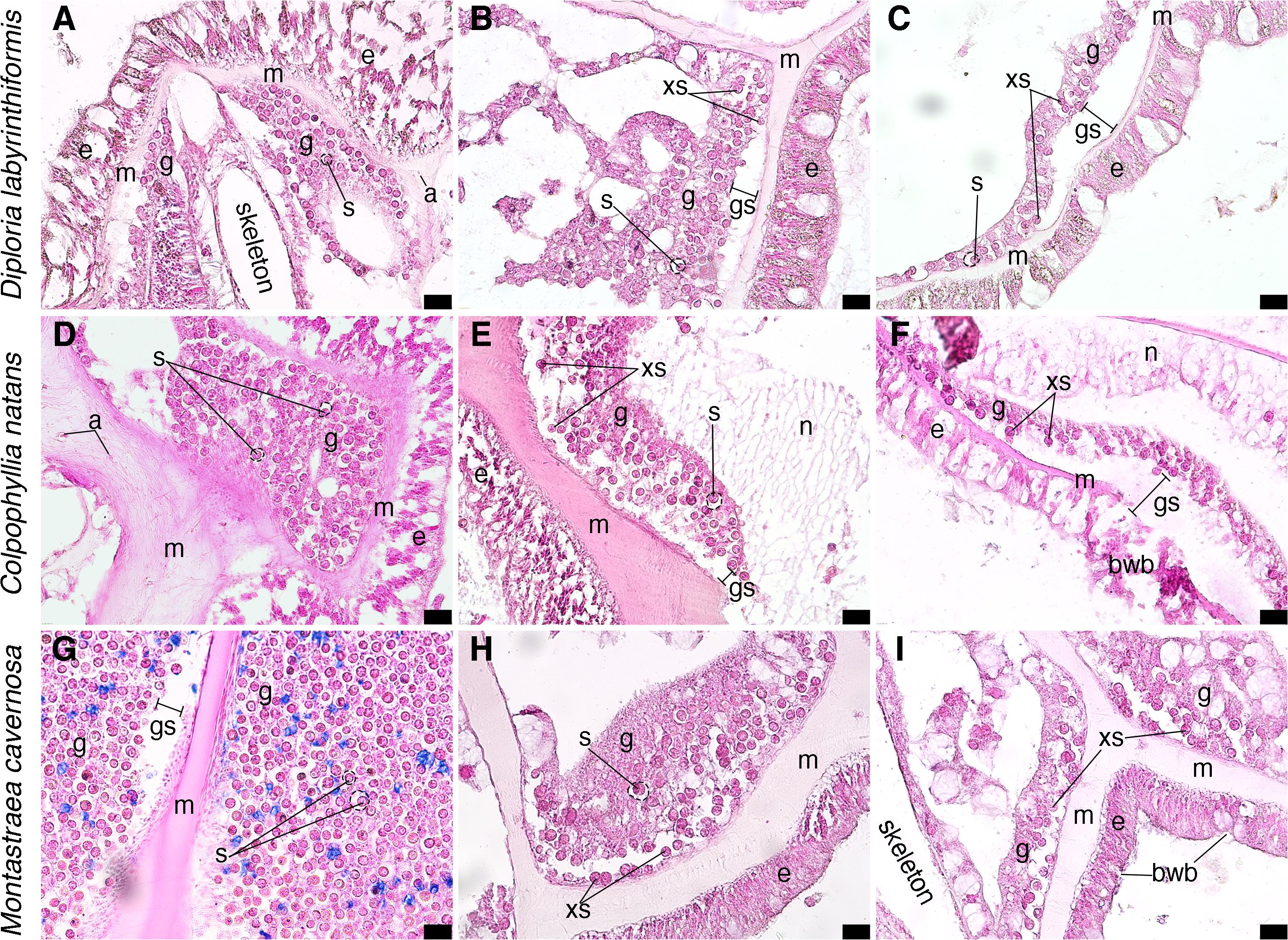
Figure 8. Micrographs of coral tissue from apparently healthy and diseased colonies stained with hematoxylin and eosin. (A–C) Diploria labrynthiformis. (A) Apparently healthy tissue from a visually healthy coral, epidermis surrounds mesoglea and gastrodermis, Symbiodinaceae in gastrodermis with clearvacuoles; location of skeleton of septa prior to decalcification indicated. (B) Diseased portion of a diseased coral, epidermis bounds mesoglea which has some separation from gastrodermis with some exocytosis of Symbiodinaceae. (C) Diseased portion of a diseased coral, mesoglea separated from gastrodermis leading to exocytosis of Symbiodinaceae. (D–F) Colpophyllia natans. (D) Apparently healthy tissue from a visually healthy coral, Symbiodinaceae in gastrodermis with clear vacuoles surrounded by mesoglea containing a few presumed amoebocytes. Epidermis visible on right-hand side. (E) Apparently healthy tissue from a diseased colony, mesoglea has separated from gastrodermis, leading to exocytosis of Symbiodinaceae; webbed tissue is likely necrotic. (F) Diseased portion of a diseased coral, epidermis displays breakage and mesoglea has separated from gastrodermis leading to exocytosis of Symbiodinaceae; necrotic tissue in gastrodermis shown as fibrous tissue. (G–I) Montastraea cavernosa. (G) Apparently healthy portion of a visually healthy coral, gastrodermis on both sides of mesoglea (epidermis not visible); there may be slight gastrodermal separation from mesoglea, potentially an artifact of histological processing; Symbiodinaceae are in clear vacuoles. (H) Diseased portion of a diseased coral, mesoglea has begun to separate from gastrodermis and exocytosis of Symbiodinaceae. (I) Diseased portion of a diseased coral, epidermis is broken, mesoglea has separated from gastrodermis, and subsequent exocytosis of Symbiodinaceae; no clearly defined vacuoles around Symbiodinaceae. S, Symbiodinaceae cell with vacuoles outlined in dashed lines; g, gastrodermis; m, mesoglea; a, potential amoebocyte; e, epidermis; xs, exocytosis of Symbiodinaceae cell; gs, gastrodermal separation; bwb, body wall breakage. Scale bars indicate 20 μm, all micrographs taken at 40X.
Discussion
In a controlled laboratory experiment, we successfully transmitted what we believe to be SCTLD to six species of Caribbean reef building corals. Visually healthy coral colonies that shared water (but did not come in physical contact) with a SCTLD-affected colony developed lesions consistent with SCTLD. We expect water-borne disease transmission occurred, which agrees with results from other SCTLD transmission experiments (Aeby et al., 2019), as well as epidemiologic and hydrodynamic models (Dobbelaere et al., 2020; Muller et al., 2020; Sharp et al., 2020). However, we acknowledge that transmission may have also occurred from vectors that may have resided on the diseased donor colonies or associated algae. Water-borne transmission of the possible pathogen(s)/pathogenic material could explain the rapid spread of the disease outbreak both within and among regions in the Atlantic and Caribbean.
Without a pathogen there is no definitive way to identify SCTLD, thus there is the possibility that the disease signs observed in the USVI represent a different disease than that observed in Florida and throughout the Caribbean. However, we find this extremely unlikely given the nearly identical ecological characteristics of the disease in the USVI (Brandt et al., in preparation) that are distinct from other rapid tissue loss diseases that have been studied in the region for almost two decades (Smith et al., 2008, 2010, 2013, 2016; Brandt et al., 2013). As with most coral diseases, a pathogen for SCTLD has not been identified despite considerable efforts to do so (Meyer et al., 2019; Rosales et al., 2020), likely due to the complexity of coral microbial communities that vary within and among individuals, species, regions, and across other scales (Thurber et al., 2020). In the present study, the gross lesion morphology on experimental corals matched descriptions of SCTLD from other regions, however, there were some notable differences in the spectrum of species susceptibility. Typically, C. natans and P. strigosa are the most susceptible (of the species tested in the present study), followed by O. annularis, M. cavernosa, S. siderea, then P. astreoides (NOAA, 2018). In the present study, we documented comparable susceptibilities between O. annularis and C. natans, followed by S. siderea, P. astreoides, P. strigosa, then M. cavernosa. Since we are confident that the field-collected diseased colonies that served as the source of transmission in this study were affected by SCTLD, the differences between susceptibility characteristics in the experiment vs. others suggest some important characteristics of SCTLD. The order in which different species developed lesions over the course of the present experiment does not match previous observations of SCTLD. Often, lesions appear earlier in C. natans and P. strigosa than O. annularis and S. siderea (NOAA, 2018 yet the opposite pattern was observed in our experiment. While O. annularis displayed signs of disease earlier, O. annularis and C. natans had equal cumulative prevalence, relative risks of lesion development, and comparable lesion progression rates leading to an overall similar susceptibility. Further, in Florida, P. astreoides is defined as not susceptible (NOAA, 2018), and Mexico defines the species as very low susceptibility (Alvarez-Filip et al., 2019), and the present study documented lesion development consistent with moderately susceptible species.
The lesions that appeared on P. astreoides fragments in the present study resembled fish predation scrapes (Figure 9). We are confident the tissue loss in the transmission experiment was not caused by predation because the corals were in a controlled setting devoid of predators. No fish were added to experimental containers and any macroscopic invertebrates were immediately removed upon identification. We also believe the lesions were the result of disease and not stress because they quickly expanded in subsequent timepoints, and no similar lesions were observed in control corals. Due to their distinctive gross lesion morphology, it is possible that field observations of SCTLD lesions on P. astreoides have previously been misidentified as predation marks. If this occurred, then previous estimates of P. astreoides susceptibility to the disease could be significant underestimates.
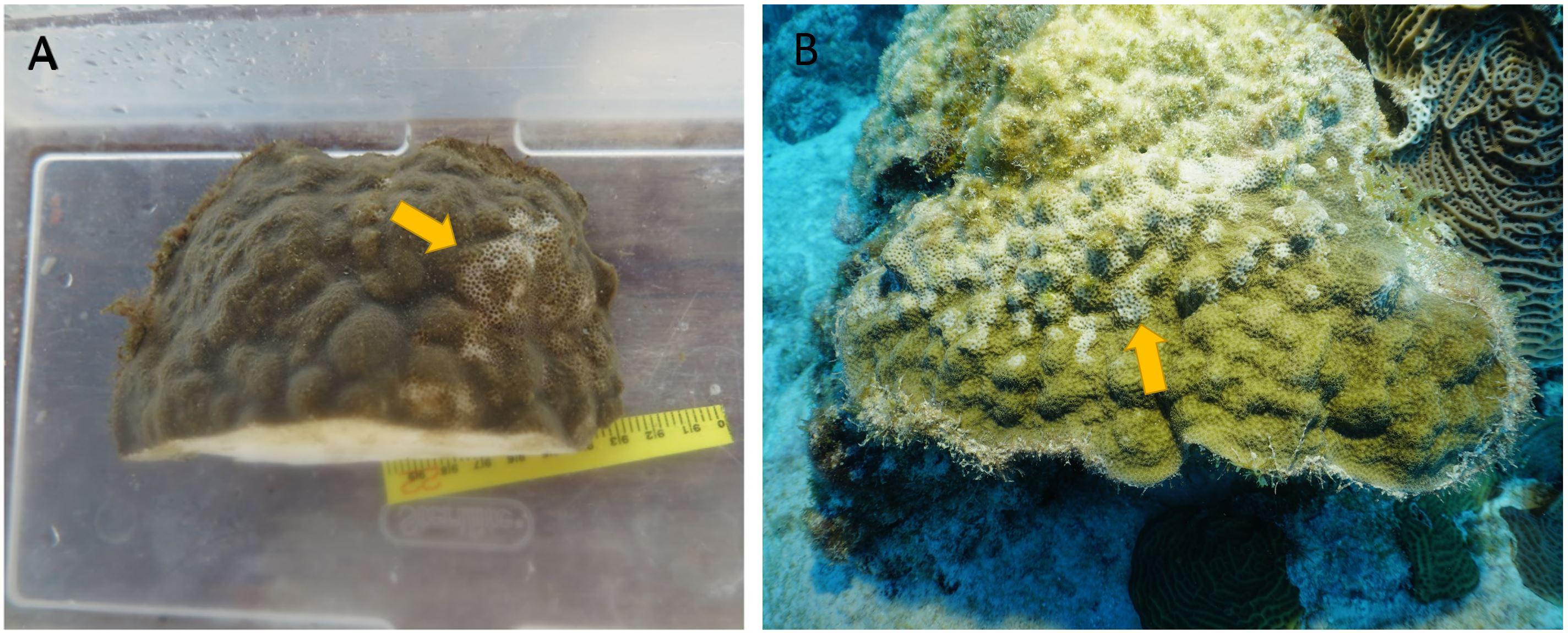
Figure 9. (A) A Porites astreoides fragment exposed to a diseased colony of Diploria labyrinthiformis exhibiting lesions (yellow arrow) potentially indicative of early stony coral tissue loss disease (SCTLD) infection. (B) A Porites astreoides colony with severe damselfish predation (yellow arrow).
It is notable that M. cavernosa did not show a significant increase in the relative risk of lesion development when fragments were exposed to the disease compared to when they were exposed to an apparently healthy coral, although the median risk increased. Additionally, the risk ratio showed that this species had significantly less risk compared with C. natans, O. annularis, and S. siderea. These results suggest USVI M. cavernosa may be slightly more resistant to SCTLD than other species tested in this study. The M. cavernosa fragments in the present experiment had the lowest lesion prevalence and one of the slowest lesion progression rates. Variable SCTLD lesion progression rates (Meiling et al., 2020) and survival (Aeby et al., 2019) among colonies of M. cavernosa suggest intra-species genotypic differences in disease resistance. In the field, M. cavernosa has the highest prevalence of SCTLD compared to other species (Brandt et al., in preparation), likely because it is one of the first few species affected, yet it is one of the slowest to experience full mortality. Case fatalities of marked M. cavernosa colonies were of the lowest across species in the USVI (∼40% over 5 months; Meiling et al., 2020). This may be because M. cavernosa is better at fighting the SCTLD infection compared to other species. Interestingly, M. cavernosa is susceptible to many of the coral diseases in the Caribbean (Pinzón et al., 2014) which frequently cause high tissue mortality in species such as Orbicella, but these diseases do not cause significant mortality of M. cavernosa colonies (MacKnight et al., in press). Immune activity of M. cavernosa is often comparable to Porites species (Mydlarz and Palmer, 2011), which are significantly less susceptible to the same diseases (Pinzón et al., 2014). Further, M. cavernosa is susceptible to similar diseases as Orbicella spp. (Pinzón et al., 2014). Perhaps M. cavernosa colonies, like Porites spp., are better at tolerating the diseases or mitigating the negative effects of the pathogens than other species.
The present study showed a difference in lesion progression rates among species, with C. natans having the fastest average rate of tissue loss. The rapid progression of lesions matches other SCTLD studies (Sharp and Maxwell, 2018; Meiling et al., 2020) and the case definition, which categorizes this species as highly susceptible (NOAA, 2018). The order from fastest to slowest lesion progression rates of the species in the present study (C. natans, O. annularis, S. siderea, M. cavernosa, P. strigosa, then P. astreoides) also matches the susceptibility hierarchy in the case definition, with the exception of P. strigosa having a lower mean rate than S. siderea. Comparisons with other studies suggest that the lesion progression rates from this study were lower on average than rates reported from the field, with the exception of O. annularis. Sharp and Maxwell (2018) found much higher progression rates on P. strigosa in Florida than reported in this study (Table 1). However, Aeby et al. (2019) found only slightly higher rates in Florida than this study (Table 1). This may suggest a regional difference, but Meiling et al. (2020) reports in situ rates from the USVI for P. strigosa that are comparable to Florida (Aeby et al., 2019; Table 1). This suggests a greater effect from lack of in situ stressors such as predation, water quality, or temperature fluctuations on lesion progression rates in the present study, rather than a regional difference. Similar to P. strigosa, the areal lesion progression rates of C. natans and M. cavernosa were much lower in the present experiment than in the field (Meiling et al., 2020; Table 1). However, areal lesion progression rates of O. annularis in the present study were much higher than local in situ rates (Meiling et al., 2020; Table 1), which suggests the experimental fragments had a different response to SCTLD exposure than the typical presentation on in situ colonies. In contrast to the total lesion progression rates, there was no difference in proportional lesion progression rates among species, which concurs with field-based rates recorded in the USVI (Meiling et al., 2020). This may suggest an influence of size of fragments on the difference of total lesion progression rates among species, however, there was no effect of size in initial ANCOVA analyses. Field monitoring in Florida also noted no effect of size on mortality rates of affected colonies (Aeby et al., 2019). However, epidemiological modeling suggests an increased risk of infection with greater surface area (Sharp et al., 2020).
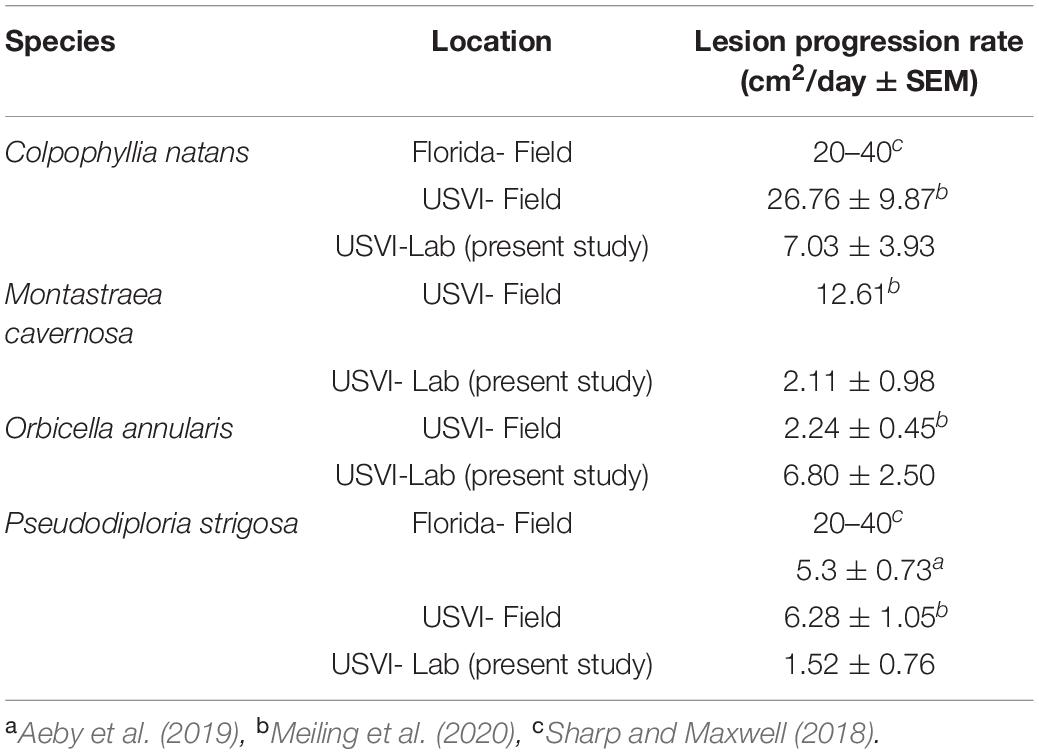
Table 1. Stony coral tissue loss disease (SCTLD) lesion progression rates across regions and studies.
While there are differences in areal lesion progression rates among regions, studies, and species, even the lowest rates for SCTLD are a magnitude higher than previously reported rapid tissue loss diseases such as white plague (Clemens and Brandt, 2015). Even though the SCTLD lesion progression rates reported in this study are lower than other studies, they are still more than double the rates reported in the USVI for white plague (Williams et al., 2020; MacKnight et al., in press) which emphasizes the severity of this new disease. Various diseases affect Caribbean corals, many of which have generalist etiologies and affect multiple species (Bruckner, 2007). Until recently, white plague outbreaks were one of the largest threats to modern Caribbean corals (Miller et al., 2009). Coral cover declines from SCTLD (Walton et al., 2018; Brandt et al., in preparation) are comparable to white plague outbreaks (Miller et al., 2009); however, the SCTLD outbreaks do not seem to be temporally restricted like white plague. Active SCTLD has been found on reefs years after initial invasion (Muller et al., 2020), while white plague is typically confined to seasonal outbreaks following thermal stress events (Miller et al., 2009) or other causes (Brandt et al., 2012).
The aforementioned differences between the present study and other SCTLD studies may suggest, regional differences in species susceptibility to disease, differences in etiology based on how long the disease has been present at a reef, or more likely, an effect of the ex situ experimental conditions on host health and disease transmission. For example, O. annularis and S. siderea fragments which are moderately susceptible species developed lesions around the same time as the highly susceptible species C. natans and earlier than the highly susceptible species P. strigosa. We would have expected O. annularis and S. siderea to develop lesions at the same time as M. cavernosa since they are all moderately susceptible species (NOAA, 2018). This expedited lesion development for O. annularis and S. siderea in the lab may be because the two species are more susceptible to the stressors of the experimental environment compared to the natural conditions on the reef. Further, M. cavernosa lesion development may have been delayed in the experiment compared to similar susceptible species due to greater tolerance of ex situ conditions. The experimental containers may have also altered feeding strategies, mucus production, or other physiological activities which may have effected disease response. Further, the closed systems and standardized sizes of the coral fragments among species in the present experiment may have affected disease transmission. All coral fragments were placed at an equal distance from the diseased coral. This, combined with the small volume of the container may have allowed pathogenic material sloughing from the central diseased coral to have nearly equivalent contact with all experimental corals. In the natural environment, coral surfaces vary substantially among species due to their differing morphologies and size structures. For instance, on the reef, colonies of C. natans grow as large hemispherical domes compared to O. annularis which grows in lobes. This may result in a differing in situ exposure to pathogenic material, especially since the SCTLD pathogen(s) is thought to be neutrally buoyant (Dobbelaere et al., 2020). Therefore, this experimental design may have “leveled the playing field,” allowing for equivalent exposure among species to pathogenic material, which may not exist in nature.
Histopathological analysis of a small subset of samples from the experiment found similar tissue markers of disease as previous SCTLD studies (Landsberg et al., 2020; Thome et al., 2021), suggesting similar physiological impacts once infected. Diseased samples of all six experimental species showed greater symbiont vacuolization and exocytosis than healthy samples. Most, if not all, presumed healthy samples did present signs of necrosis or vacuolization around Symbiodiniaceae (Figures 8A,B,D,G); however, this is not uncommon (Landsberg et al., 2020), and could be due to sampling stress or other environmental stress. Samples from apparently healthy tissue on a diseased coral (Figure 8E) may have shown signs of stress due to the impact of the disease (prior to tissue lesions or sloughing). We suspect samples from apparently healthy tissue on control corals (Figures 8A,D,G) exhibited signs of stress from containment or sampling, rather than disease because no healthy fragments developed lesions. Molecular and immunological investigations of the pathogen(s) and infection should be compared across regions to better qualify the separate disease outbreaks.
SCTLD is causing one of the greatest documented changes to occur in modern Caribbean coral communities. Although there are varying susceptibilities to SCTLD among species, affected reef communities are drastically altered. Defining susceptibilities is important for resource managers to prioritize efforts, especially direct interventions such as antibiotic application (Neely et al., 2020). Many of the species susceptible to SCTLD are massive corals, which are important to Caribbean reefs because they are the main reef builders (Ginsburg et al., 2001). Not only will this disease impact coral communities, but likely other reef inhabitants such as fish which prefer more rugose and complex structures (Kuffner et al., 2007) provided by living corals.
The rapid inter-species transmission via water ensures SCTLD will continue to spread throughout the Caribbean basin. Although a putative pathogen(s) has yet to be identified, studies comparing microbial communities between healthy and affected corals suggest a bacterial component (Meyer et al., 2019; Iwanowicz et al., 2020; Rosales et al., 2020; Ushijima et al., 2020). Efficacy of antibiotic applications in arresting active lesions (Neely et al., 2020) further supports a key role of bacteria in SCTLD, and also offers hope that not all susceptible species will be lost. While highly unlikely that resource managers can preserve the current state of unaffected reefs, aggressive intervention and management strategies can have positive effects on mitigating loss of coral cover and diversity. Regions without a disease response plan, especially those yet to be affected, should focus efforts on preparing for action and identifying the necessary resources for a proper outbreak response.
Data Availability Statement
The raw data supporting the conclusions of this article will be made available by the authors, without undue reservation.
Author Contributions
SM, EM, DL, AC, NH, TS, DH, and MB were involved with experimental design. SM, DL, AR, AV, NM, BD, AC, TS, DH, and MB contributed to data collection. SM, EM, and MB were involved with statistical analyses. SM, EM, AV, AR, AC, NM, BD, DH, LM, TS, and MB assisted with editing. All authors contributed to the article and approved the submitted version.
Funding
This work was supported by the National Science Foundation (Biological Oceanography) award number 1928753 to MB and TS, 1928609 to AC, 1928817 to EM, 19228771 to LM, 1927277 to DH, and 1928761 to AA as well as by VI EPSCoR (NSF #0814417 and NSF #1946412).
Conflict of Interest
The authors declare that the research was conducted in the absence of any commercial or financial relationships that could be construed as a potential conflict of interest.
Acknowledgments
This work could not have been completed without the hours of lab and fieldwork dedicated by Adam Glahn, Amanda Long, Brad Arrington, Daniel Mele, Kathryn Cobleigh, and Alex Gutting. Field collections were authorized by the Department of Planning and Natural Resources Coastal Zone Management permit #DFW19057U. This is contribution #229 from the Center for Marine and Environmental Studies at the University of the Virgin Islands.
References
Aeby, G. S., Ushijima, B., Campbell, J. E., Jones, S., Williams, G. J., Meyer, J. L., et al. (2019). Pathogenesis of a tissue loss disease affecting multiple species of corals along the Florida reef tract. Front. Mar. Sci. 6:678. doi: 10.3389/fmars.2019.00678
Alvarez-Filip, L., Estrada-Saldívar, N., Pérez-Cervantes, E., Molina-Hernández, A., and González-Barrios, F. J. (2019). A rapid spread of the stony coral tissue loss disease outbreak in the Mexican Caribbean. PeerJ 7, 1–17.
Aronson, R. B., and Precht, W. F. (2001). White-band disease and the changing face of Caribbean coral reefs. Hydrobiologia 460, 25–38. doi: 10.1007/978-94-017-3284-0_2
Brandt, M. E., Ruttenberg, B. I., Waara, R., Miller, J., Witcher, B., Estep, A. J., et al. (2012). Dynamics of an acute coral disease outbreak associated with the Macroalgae Dictyota spp. in Dry Tortugas National Park, Florida, USA. Bull. Mar. Sci. 88, 1035–1050. doi: 10.5343/bms.2011.1104
Brandt, M. E., Smith, T. B., Correa, A. M., and Vega-Thurber, R. (2013). Disturbance driven colony fragmentation as a driver of a coral disease outbreak. PLoS One 8:e57164. doi: 10.1371/journal.pone.0057164
Bruckner, A. W. (2007). Field Guide to Western Atlantic Coral Diseases and Other Causes of Coral Mortality. NOAA, UNEP-WCMC, PADI. Available online at: https://digitallibrary.un.org/record/503557?ln=en (accessed October 16, 2018).
Clemens, E., and Brandt, M. (2015). Multiple mechanisms of transmission of the Caribbean coral disease white plague. Coral Reefs 34, 1179–1188. doi: 10.1007/s00338-015-1327-6
Dobbelaere, T., Muller, E. M., Gramer, L. J., Holstein, D. M., and Hanert, E. (2020). Coupled epidemio-hydrodynamic modeling to understand the spread of a deadly coral disease in Florida. Front. Mar. Sci. 7:591881. doi: 10.3389/fmars.2020.591881
Eaton, K., and Muller, E. (2019). “2019 SWG disease transmission experiments: a summary,” in Powerpoint Presentation Presented to the Florida Coral Disease Advisory Council in June 2020.
Estrada-Saldivar, N., Molina-Hernandez, A., Perez-Cervantes, E., Medellin-Maldonado, F., Gonzalez-Barrios, F. J., and Alvarez-Filip, L. (2020). Reef-scale impacts of the stony coral tissue loss disease outbreak. Coral Reefs 39, 861–866. doi: 10.1007/s00338-020-01949-z
Gelman, A., Carlin, J. B., Rubin, D. B., Stern, H. S., Stern, H. S., and Gelman, A. (2004). Bayesian Data Analysis. United Kingdom: Chapman & Hall/CRC.
Ginsburg, R. N., Gischler, E., and Kiene, W. E. (2001). Partial mortality of massive reef-building corals: an index of patch reef condition, Florida Reef Tract. Bull. Mar. Sci. 69, 1149–1173.
Iwanowicz, D., Schill, W. B., Woodley, C. M., Bruckner, A. W., Neely, K., and Briggs, K. M. (2020). Exploring the stony coral tissue loss disease bacterial pathobiome. bioRxiv [Preprint]. doi: 10.1101/2020.05.27.120469
Kramer, P. R., Roth, L., and Lang, J. (2019). Map of Stony Coral Tissue Loss Disease Outbreak in the Caribbean. Available online at: www.agrra.org (accessed February, 2021).
Kuffner, I. B., Brock, J. C., Grober-Dunsmore, R., Bonito, V. E., Hickey, T. D., and Wright, C. W. (2007). Relationships between reef fish communities and remotely sensed rugosity measurements in Biscayne National Park, Florida, USA. Environ. Biol. Fish. 78, 71–82. doi: 10.1007/s10641-006-9078-4
Landsberg, J. H., Kiryu, Y., Wilson, P., Perry, N., Waters, Y., and Huebner, L. (2020). “Disease lab analysis updates,” in Powerpoint Presentation Presented to the Florida Coral Disease Advisory Council in December 2018.
Lawson, A. B. (2009). Bayesian Disease Mapping: Hierarchical Modeling in Spatial Epidemiology. United Kingdom: Taylor & Francis.
MacKnight, N., Cobleigh, K., Lasseigne, D., Chaves-Fonnegra, A., Gutting, A., and Dimos, B., et al. (in press). Microbial dysbiosis reflects disease resistance in diverse coral species. Submitted Commun. Biol.
Meiling, S., Muller, E. M., Smith, T. B., and Brandt, M. E. (2020). 3D photogrammetry reveals dynamics of stony coral tissue loss disease (SCTLD) lesion progression across a thermal stress event. Front. Mar. Sci. 7:597643. doi: 10.3389/fmars.2020.597643
Meyer, J. L., Castellanos-Gell, J., Aeby, G. S., Häse, C. C., Ushijima, B., and Paul, V. J. (2019). Microbial community shifts associated with the ongoing stony coral tissue loss disease outbreak on the Florida Reef Tract. Front. Microbiol. 10:2244. doi: 10.3389/fmicb.2019.02244
Miller, J., Muller, E., Rogers, C., Waara, R., Atkinson, A., Whelan, K. R. T., et al. (2009). Coral disease following massive bleaching in 2005 causes 60% decline in coral cover on reefs in the US Virgin Islands. Coral Reefs 28:925. doi: 10.1007/s00338-009-0531-7
Muller, E., Sartor, C., Alcaraz, N. I., and van Woesik, R. (2020). Spatial epidemiology of the stony-coral-tissue-loss-disease in Florida. Front. Mar. Sci. 7:163. doi: 10.3389/fmars.2020.00163
Mydlarz, L. D., and Palmer, C. (2011). The presence of multiple phenoloxidases in Caribbean reef-building corals. Comp. Biochem. Physiol. Part A 159, 372–378. doi: 10.1016/j.cbpa.2011.03.029
Neely, K., Macauly, K., Hower, E., and Dobler, M. (2020). “Intervention work: Florida keys,” in Powerpoint Presentation Presented to the Florida Coral Disease Advisory Council in 2020.
NOAA (2018). Stony Coral Tissue Loss Disease Case Definition. Available online at: https://floridadep.gov/sites/default/files/Copy%20of%20StonyCoralTissueLossDisease_CaseDefinition%20final%2010022018.pdf (accessed September 15, 2020).
Pinzón, C. J. H., Beach-Letendre, J., Weil, E., and Mydlarz, L. D. (2014). Relationship between Phylogeny and immunity suggests older caribbean coral lineages are more resistant to disease. PLoS One 9:e104787. doi: 10.1371/journal.pone.0104787
Precht, W. F., Gintert, B. E., Robbart, M. L., Fura, R., and van Woesik, R. (2016). Unprecedented disease related coral mortality in Southeastern Florida. Sci. Rep. 6:31374.
Rosales, S. M., Clark, A. S., Huebner, L. K., Ruzicka, R. R., and Muller, E. M. (2020). Rhodobacterales and Rhizobiales are associated with stony coral tissue loss disease and its suspected sources of transmission. Front. Microbiol. 11:681. doi: 10.3389/fmicb.2020.00681
Schaarschmidt, F., and Gerhard, D. (2019). pairwiseCI: Confidence Intervals for Two Sample Comparisons. R Package Version 0.1-27. Available online at: https://CRAN.R-project.org/package=pairwiseCI (accessed March 11, 2019).
Schneider, C. A., Rasband, W. S., and Eliceiri, K. W. (2012). NIH Image to ImageJ: 25 years of image analysis. Nat. Methods 9, 671–675. doi: 10.1038/nmeth.2089
Sharp, W., and Maxwell, K. (2018). Investigating the Ongoing Coral Disease Outbreak in the Florida Keys: Collecting Corals to Diagnose the Etiological Agent(s) and Establishing Sentinel Sites to Monitor Transmission Rates and the Spatial Progression of the Disease. Marathon FL: Florida Fish and Wildlife Conservation Commission.
Sharp, W. C., Shea, C. P., Maxwell, K. E., Muller, E. M., and Hunt, J. H. (2020). Evaluating the small-scale epidemiology of the stony-coral -tissue-loss disease in the middle Florida Keys. PLoS One 15:e0241871. doi: 10.1371/journal.pone.0241871
Smith, T. B., Blondeau, J., Nemeth, R. S., Pittman, S. J., Calnan, J. M., Kadison, E., et al. (2010). Benthic structure and cryptic mortality in a Caribbean mesophotic coral reef bank system, the Hind Bank Marine Conservation District, US Virgin Islands. Coral Reefs 29, 289–308. doi: 10.1007/s00338-009-0575-8
Smith, T. B., Brandt, M. E., Calnan, J. M., Nemeth, R. S., Blondeau, J., Kadison, E., et al. (2013). Convergent mortality responses of Caribbean coral species to seawater warming. Ecosphere 4:87.
Smith, T. B., Ennis, R. S., Kadison, E., Weinstein, D. W., Jossart, J., and Gyory, J. (2016). The United States Virgin Islands Territorial Coral Reef Monitoring Program 2016 Annual Report. United States Virgin Islands,: University of the Virgin Islands, 286.
Smith, T. B., Nemeth, R. S., Blondeau, J., Calnan, J. M., Kadison, E., and Herzlieb, S. (2008). Assessing coral reef health across onshore to offshore stress gradients in the US Virgin Islands. Mar. Pollut. Bull. 56, 1983–1991. doi: 10.1016/j.marpolbul.2008.08.015
Therneau, T. (2019). A Package for Survival Analysis in R. R Package Version 3.2-7. Available online at: https://CRAN.R-project.org/package=survival (accessed March 16, 2021).
Thome, P. E., Rivera-Ortega, J., Rodríguez-Villalobos, J. C., Cerqueda-García, D., Guzmán-Urieta, E. O., García-Maldonado, J. Q., et al. (2021). Local dynamics of a white syndrome outbreak and changes in the microbial community associated with colonies of the scleractinian brain coral Pseudodiploria strigosa. PeerJ 9, e10695. doi: 10.7717/peerj.10695
Thurber, R. V., Mydlarz, L. D., Brandt, M., Harvell, D., Weil, E., Raymundo, L., et al. (2020). Deciphering coral disease dynamics: integrating host, microbiome, and the changing environment. Front. Ecol. Evol. 8:575927. doi: 10.3389/fevo.2020.575927
Ushijima, B., Meyer, J. L., Thompson, S., Pitts, K., Marusich, M. F., Tittl, J., et al. (2020). Disease diagnostics and potential coinfections by Vibrio coralliilyticus during an ongoing coral disease outbreak in Florida. Front. Microbiol. 11:569354. doi: 10.3389/fmicb.2020.569354
Walton, C. J., Hayes, N. K., and Gilliam, D. S. (2018). Impacts of a regional, multi-year, multi-species coral disease outbreak in Southeast Florida. Front. Mar. Sci. 5:323. doi: 10.3389/fmars.2018.00323
Keywords: stony coral tissue loss disease, coral disease, transmission experiment, susceptibility, lesion progression rate, Caribbean, United States Virgin Islands, histopathology
Citation: Meiling SS, Muller EM, Lasseigne D, Rossin A, Veglia AJ, MacKnight N, Dimos B, Huntley N, Correa AMS, Smith TB, Holstein DM, Mydlarz LD, Apprill A and Brandt ME (2021) Variable Species Responses to Experimental Stony Coral Tissue Loss Disease (SCTLD) Exposure. Front. Mar. Sci. 8:670829. doi: 10.3389/fmars.2021.670829
Received: 22 February 2021; Accepted: 08 April 2021;
Published: 30 April 2021.
Edited by:
Lorenzo Alvarez-Filip, National Autonomous University of Mexico, MexicoReviewed by:
Karen Lynn Neely, Nova Southeastern University, United StatesAdán Guillermo Jordán-Garza, Universidad Veracruzana, Mexico
Copyright © 2021 Meiling, Muller, Lasseigne, Rossin, Veglia, MacKnight, Dimos, Huntley, Correa, Smith, Holstein, Mydlarz, Apprill and Brandt. This is an open-access article distributed under the terms of the Creative Commons Attribution License (CC BY). The use, distribution or reproduction in other forums is permitted, provided the original author(s) and the copyright owner(s) are credited and that the original publication in this journal is cited, in accordance with accepted academic practice. No use, distribution or reproduction is permitted which does not comply with these terms.
*Correspondence: Sonora S. Meiling, sonora.meiling@gmail.com