- 1School of Life Sciences, University of Warwick, Coventry, United Kingdom
- 2Department of Chemistry, University of Warwick, Coventry, United Kingdom
- 3UCL Department of Chemistry, University College London, London, United Kingdom
- 4Department of Biology, University of the Balearic Islands, Palma, Spain
Cerium oxide nanoparticles (nCeO2) are used at an ever-increasing rate, however, their impact within the aquatic environment remains uncertain. Here, we expose the ecologically significant marine cyanobacterium Prochlorococcus sp. MED4 to nCeO2 at a wide range of concentrations (1 μg L–1 to 100 mg L–1) under simulated natural and nutrient rich growth conditions. Flow cytometric analysis of cyanobacterial populations displays the potential of nCeO2 (100 μg L–1) to significantly reduce Prochlorococcus cell density in the short-term (72 h) by up to 68.8% under environmentally relevant conditions. However, following longer exposure (240 h) cyanobacterial populations are observed to recover under simulated natural conditions. In contrast, cell-dense cultures grown under optimal conditions appear more sensitive to exposure during extended incubation, likely as a result of increased rate of encounter between cyanobacteria and nanoparticles at high cell densities. Exposure to supra-environmental nCeO2 concentrations (i.e., 100 mg L–1) resulted in significant declines in cell density up to 95.7 and 82.7% in natural oligotrophic seawater and nutrient enriched media, respectively. Observed cell decline is associated with extensive aggregation behaviour of nCeO2 upon entry into natural seawater, as observed by dynamic light scattering (DLS), and hetero-aggregation with cyanobacteria, confirmed by fluorescent microscopy. Hence, the reduction of planktonic cells is believed to result from physical removal due to co-aggregation and co-sedimentation with nCeO2 rather than by a toxicological and cell death effect. The observed recovery of the cyanobacterial population under simulated natural conditions, and likely reduction in nCeO2 bioavailability as nanoparticles aggregate and undergo sedimentation in saline media, means that the likely environmental risk of nCeO2 in the marine environment appears low.
Introduction
Cerium oxide nanoparticles (nCeO2) represent an emerging contaminant with widespread use across a range of industries. Nano-sized cerium possesses unique properties compared to the bulk material and exhibit properties such as redox activity, scavenging of free radicals and inhibition of biofilm formation (Singh et al., 2020), leading to their use across a variety of applications including; biomedical applications (Das et al., 2013; Nyoka et al., 2020), drug delivery and therapeutics (Nadeem et al., 2020), glass production and incorporation into electronic components (Merrifield et al., 2013).
Annual production of nCeO2 is estimated to be approximately 10,000 tonnes (European Commission, 2012), and their ever-increasing use is likely to result in greater release of engineered nCeO2 into the environment (Collin et al., 2014). The use of nCeO2 as an additive for diesel fuels is a major ecological concern, and is believed to be the main source of nCeO2 particles into the natural environment (Johnson and Park, 2012). Overall, it is predicted up to 70 million metric tonnes of ceria could be released annually by road transport worldwide (Dale et al., 2017), with the highest environmental levels of nCeO2 predicted to be present in water draining from road surfaces (Johnson and Park, 2012), where nanoparticles may then enter the aquatic environment. Additionally, aquatic transport responsible for 80% of global trade shipping (Hallquist et al., 2013) may represent an understudied source of nCeO2 release. Boat engines have been recorded to utilise fuels containing nanosized additives including cerium (Somasundaram et al., 2017; Jiaqiang et al., 2018; Somasundaram et al., 2020). It is possible that nCeO2 may enter the ocean in this manner if used in boat fuels to enhance efficiency (Somasundaram et al., 2020), and may represent a previously understudied source of nCeO2 entry into the environment. Aside from transport, nCeO2 may enter the natural environments via release into wastewater, use of wastewater treatment sludge as fertiliser, or as a consequence of industrial production of nCeO2 or related products (Hu et al., 2006; Limbach et al., 2008).
Research carried out to date suggests that the environmental risk of nCeO2 is low (Johnson and Park, 2012), however, limited evidence exists outlining the potential impact of nCeO2 upon marine microorganisms. Upon entry into the environment, airborne and waterborne nCeO2 may become widely dispersed (Merrifield et al., 2013). In some cases, nanoparticulate matter emitted from diesel engines has been traced internationally, for example nCeO2 released in the United Kingdom has been identified in mainland Europe (Charron and Harrison, 2005). However, insufficient data exists to accurately predict the current levels of nCeO2 in the natural environment (Johnson and Park, 2012; Neil et al., 2021), and great variation exists in such estimations. For example, Dale et al. (2017) predict a global release of 70 million metric tonnes of Ce-based pollution into the environment by road transport, a value considerably higher than the estimated 15.6–114.9 kg year–1 predicted in the United Kingdom by Johnson and Park (2012). To address this uncertainty, increased efforts must be placed into improving methods by which we are able to accurately monitor the release of engineered nanomaterials into the environment. Subsequently, experimental work can be carried out under environmentally relevant conditions, facilitating the effective assessment of the environmental risk of nanosized contaminants in natural systems.
The marine environment represents the sink for pollutants entered into aquatic systems. Here, microbial organisms which display high surface area-to-volume ratios are at significant risk from waterborne pollutants, particularly in coastal regions (Lucas et al., 2011). These microorganisms represent the base of the marine food chain and play key roles in the functioning of the entire marine ecosystem (Field et al., 1998; Flombaum et al., 2013). Therefore, understanding the impact of pollutants upon these key species is of great importance. Metal oxide nanomaterials including; titanium dioxide (Clement et al., 2013; Manzo et al., 2015; Xia et al., 2015; Deng et al., 2017), zinc oxide (Miao et al., 2010; Wong et al., 2010) and iron oxide (Demir et al., 2015) have previously been reported to exert adverse effects upon marine phytoplankton. Such effects include growth inhibition (Wong et al., 2010; Clement et al., 2013), reduced photosynthetic performance (Deng et al., 2017), induction of oxidative stress (Xia et al., 2015; Deng et al., 2017) and physical attachment (Demir et al., 2015; Manzo et al., 2015; Deng et al., 2017). However, limited evidence is available examining the impact of nCeO2 upon marine microbial species. A significant reduction in growth of marine phytoplankton has been recorded in response to nCeO2 exposure at concentrations in the range 10–40 mg L–1 (Deng et al., 2017; Sendra et al., 2017), although findings vary. At concentrations of up to 5 mg L–1 no adverse effects of nCeO2 exposure upon the green algae Chlorella autotrophica and Dunaliella salina were observed (Sendra et al., 2018). Currently, the effect of nCeO2 upon marine microbial species under environmental conditions remain uncertain and further research is required to reveal potential impacts.
In this study, we examine the impact of nCeO2 exposure upon the ecologically significant marine cyanobacterium Prochlorococcus sp. MED4. This phototroph has previously been shown to display increased sensitivity to nano-pollutants (i.e., silver) (Dedman et al., 2020) and represents the most abundant phototroph in the world’s oligotrophic oceans (Scanlan et al., 2009; Bagby and Chisholm, 2015). Cultures were incubated with nCeO2 at both environmentally relevant and supra-environmental concentrations under simulated natural conditions (i.e., ambient cell densities grown in oligotrophic natural seawater) (Mella-Flores et al., 2011; Ma et al., 2017), or in cell-dense cultures grown in nutrient-rich media, for a period of 72–240 h. Alterations in cyanobacterial cell density were monitored by flow cytometry. An assessment of nCeO2 behaviour in saline media was carried out by dynamic light scattering (DLS) and microscopic methods, revealing extensive aggregation behaviour, believed to drive the observed declines in cyanobacterial abundance recorded during exposures. Such research presents novel insight into the likely behaviour and impact of an emerging contaminant within the marine environment for which limited information exists, hence, facilitating the effective evaluation of their likely environmental risk and interaction with microbial species.
Materials and Methods
Materials
Nanomaterials utilised for research were purchased from Sigma Aldrich [cerium oxide, <25 nm (Material No. 544841)]. Natural oligotrophic seawater (NSW) used to culture cyanobacteria and during experimental work was collected from Station L4, Plymouth (50°15.0’N; 4°13.0’W). Prior to use, NSW was autoclaved (120°C, 20 min) and filtered through a 0.22 μm polyethersulfone membrane (Corning®). For each experiment nCeO2 stocks were added directly to NSW prepared as described and sonicated for 15–30 min using a Branson 1210 Sonicator operating at 40 kHz to ensure suspensions were well-mixed and minimise aggregation of nanoparticles. MilliQ ultrapure water used throughout laboratory work was 0.22 μm filter operated at 18.2 MΩ at 298 K. Sterile filter capped tissue culture flasks were used for all experimental work with cyanobacteria and glassware was acid-washed before use. Axenic Prochlorococcus sp. MED4 cultures (isolate source: Mediterranean Sea, South France) were grown on-site using Pro99 media (see Supplementary Section “Pro99 Media”; Moore et al., 2002).
Characterisation of nCeO2 and Dynamic Light Scattering Assessment of Behaviour in Natural Seawater
To determine primary particle size and examine the morphology of nCeO2 utilised during study, transmission electron microscopy (TEM) was used. Here, images were taken using a JEOL 2100 TEM (200 kV, LaB6 instrument; beam current ∼115 mA), equipped with a Gatan Orius 11-megapixel camera. To prepare samples for TEM imaging, nCeO2 stock was made up in MilliQ ultrapure water and 10 μL deposited into formvar-coated 300 mesh copper grids (EM Resolutions). Following image acquisition, the average particle diameter was determined by measuring 100 nanoparticles using Image J v.3.2 software. Visual observation of particle morphology was also carried out at this time. UV-visible spectra were collected using an Agilent Cary 60 UV-vis spectrophotometer of a sample of 100 mg L–1 nCeO2 in MilliQ ultrapure water. Dynamic light scattering (DLS) was used to evaluate the aggregation behaviour of nCeO2 (1 and 100 mg L–1) when entered into NSW for a period of 240 h. Using a Malvern Zetasizer Nano ZS instrument, equipped with a 4 mW He-Ne 633 nm laser module, measurements of z-average size and polydispersity in MilliQ ultrapure water were first obtained. Following this, triplicate suspensions of nCeO2 were made up in 20 mL NSW in 50 mL tissue culture flasks, maintained at ambient room temperature under shaking (orbital shaker, 100 rpm) to simulate natural water motion. At 0, 1, 2, 4, 24, 48, 72, 168, and 240 h, a 200 μL sub-sample was collected from the mid-point flasks, representing the suspended fraction, and average values for z-average size, polydispersity index (PDI) and mean count rate recorded based on 3 measurements consisting of 11 sampling runs each lasting 10 s. Zeta-potential of nCeO2 (1 and 100 mg L–1) in MilliQ ultrapure water, and NSW diluted 1:1 in MilliQ ultrapure water, was additionally monitored at 0 h.
Short-Term (72 h) Toxicity Testing at Environmentally Relevant Concentrations
Prochlorococcus sp. MED4 was exposed to nCeO2 (0, 1, 10, and 100 μg L–1) for a period of 72 h under two test conditions: (i) ambient cell density (2.8 × 104 cells mL–1) grown in NSW and (ii) cell-dense cultures grown in nutrient-rich Pro99 media (8.9 × 105 cells mL–1). Seventy-two hours prior to experimentation, axenic culture was added to NSW or Pro99 media, respectively, and preadapted to experimental conditions: 23°C and 10 μmol photons m–2 s–1 light intensity (LifeliteTM full spectrum bulb) whilst shaking (100 rpm). To establish exposures, triplicate 30 mL samples were added to 50 mL filter-capped tissue culture flasks under sterile conditions and spiked with a defined volume of nCeO2 stock to make up test concentrations. Flasks were subsequently maintained under the experimental conditions described and the cyanobacterial population monitored at 0, 24, 48, and 72 h using a Becton Dickinson Fortessa Flow Cytometer. Briefly, a 1 mL sample was collected from the mid-point of flasks and for NSW cultures and analysed directly, whilst those obtained from cultures grown in Pro99 media were first diluted 10-times in NSW. To calculate values for cell density, FACSDiva software was used to gate Prochlorococcus cells based on their natural autofluorescence and their abundance relative to standard polystyrene reference beads (2.2 μm high Intensity fluorescent Nile Red particles, Spherotech FH-2056-2) was recorded.
Extended (240 h) Exposure
Following 72 h toxicity testing, incubations with nCeO2 were repeated and extended to 240 h to examine longer-term impacts of exposure. Initial cell densities were 1.7 × 105 and 6.6 × 105 cells mL–1 in NSW and Pro99 media, respectively. The nCeO2 test concentrations utilised were also expanded to include both environmentally relevant and supra-environmental values (0, 1, 10, and 100 μg L–1; and 1, 10, and 100 mg L–1), hence allowing for inference of likely impacts in hot spots of pollution or should release of nCeO2 into the marine environment increase. Exposures were established as described above and the cyanobacterial population monitored by flow cytometry at 0, 24, 48, 72, 168, and 240 h. A summary of test conditions examined is provided in Supplementary Table 2.
Fluorescent Microscopic Analysis of Cyanobacterial-nCeO2 Interactions
To investigate the presence of cyanobacteria within precipitated material observed during extended (240 h) toxicity testing (section “Extended (240 h) Exposure”), sub-samples of deposited material were collected from the base of culture flasks and stained using SYBR Gold nuclear acid stain (diluted to 1X, ThermoFisher) for > 15 min under darkness. After staining, material was imaged under brightfield and GFP fluorescence at 40x magnification using a Nikon Widefield Fluorescence Microscope. Images obtained from each channel were subsequently merged to produce a composite image and assess hetero-aggregation of cyanobacteria and nCeO2.
Statistical Analysis
To examine the occurrence of statistically significant variations in Prochlorococcus MED4 populations exposed to nCeO2 in short-term (section “Short-Term (72 h) Toxicity Testing at Environmentally Relevant Concentrations”) and extended toxicity tests (section “Extended (240 h) Exposure”), one-way analysis of variance ANOVA tests were carried out using IBM SPSS Statistics v27. Additionally, post hoc Dunnett’s T-tests were utilised to identify significant alterations in cell density in treated cultures compared to the untreated control at respective timepoints (p ≤ 0.05).
Results
Characterisation of nCeO2 and Aggregation Behaviour in Natural Seawater
Primary Particle Size and Morphology
Analysis of TEM images revealed a primary particle size of 20.6 ± 12.1 nm, close to that stated by the manufacturer (<25 nm). However, nanoparticles displayed a great variation in primary particle size. The size distribution of primary nCeO2 particles measured during analysis is presented in Supplementary Figure 1. In terms of morphology, nCeO2 were generally cuboid in shape, although a range of forms could be observed, including diamond- and triangular-shaped particles (Figures 1A,B). As is common in TEM analysis, nCeO2 readily formed aggregates, as a result of drying onto TEM grids (Figure 1C). UV-vis spectroscopy demonstrated an absorption band between 250 and 400 cm–1 with a maximum absorbance at 317 nm (Supplementary Figure 2), attributed to the electronic structure of ceria and defects, including oxygen vacancies, and is in line with literature (Fronzi et al., 2009; Calvache-Munoz et al., 2017).
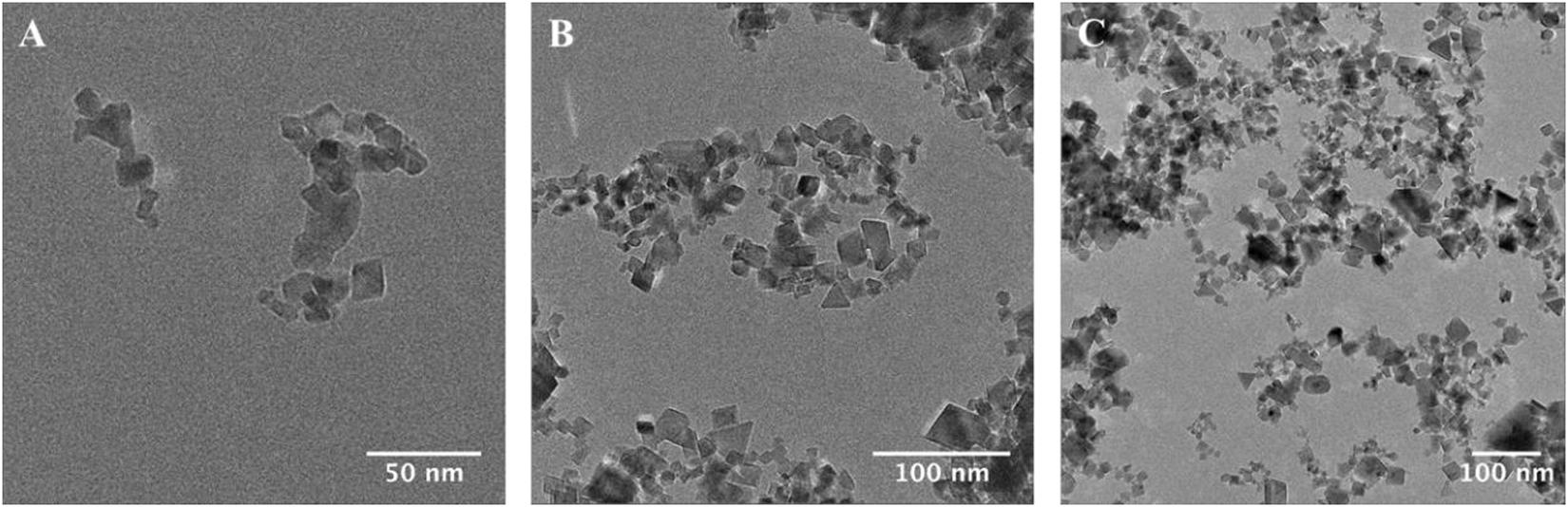
Figure 1. Transmission electron microscope imaging of nCeO2 (Sigma-Aldrich, <25 nm) used for experimental work (A–C).
Behaviour of nCeO2 in NSW
The aggregation behaviour of nCeO2 (20.6 ± 12.1 nm, TEM) was examined by monitoring the z-average size of nanoparticles, as measured by DLS, after entry into NSW over a period from 0 to 240 h (Table 1). Analysis of nCeO2 suspensions made up in MilliQ ultrapure water revealed respective z-average sizes of 136 ± 2 nm and 125 ± 11 nm for concentrations of 1 and 100 mg L–1. Upon entry into NSW (0 h), z-average size was recorded as 1,293 ± 141 nm and 1,196 ± 140 nm for 1 and 100 mg L–1 samples, respectively, indicating the immediate aggregation of nCeO2. The aggregation of nCeO2 within saline media with high ionic strength is expected and in accordance with previous research (Keller et al., 2010; Ottofuelling et al., 2011; Quik et al., 2014; Sendra et al., 2017). Measurements of zeta-potential were obtained in MilliQ ultrapure water and NSW at 0 h, revealing respective surface charges of −8.6 ± 0.5 mV and −2.8 ± 0.1 mV in ultrapure water; and −10.6 ± 1.5 mV and 1.4 ± 0.3 mV in NSW for 1 and 100 mg L–1 nCeO2 samples. Zeta-potential appeared to vary between 1 and 100 mg L–1 samples, likely due to concentration-dependent effects which appear exacerbated in NSW where ionic strength is increased. Upon entry into NSW, zeta-potential values appeared to vary between the two concentrations, likely due to the extensive aggregation behaviour of nanoparticles upon entry into saline media, as demonstrated by DLS measurements (Table 1). The highly variable nature of the surface charge of nCeO2 nanoparticles, and related metal oxides (e.g., TiO2), upon addition to various experimental media has previously been reported in the literature (Rodea-Palomares et al., 2010; Sendra et al., 2017).
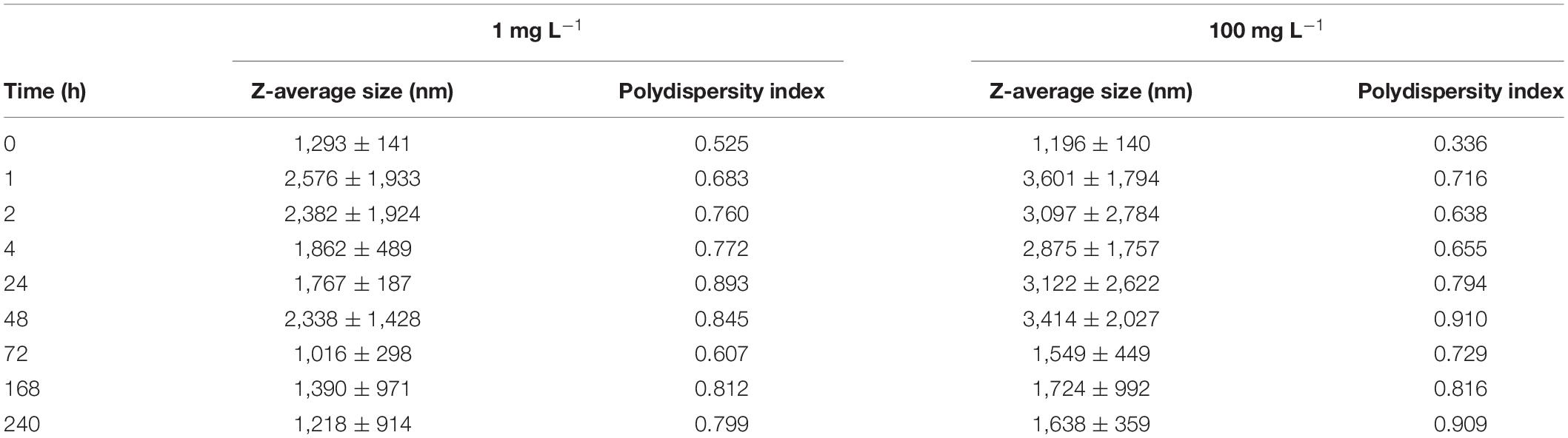
Table 1. Summary of data obtained during DLS analysis of nCeO2 (20.6 ± 12.1 nm) added to natural seawater for a period of 240 h at a concentration 1 and 100 mg L–1 (n = 3).
During the first 2 h, nCeO2 displayed an increase in z-average size at both concentrations, indicating a continuation of the aggregation process. Alongside increases in z-average size, the polydispersity index (PDI) also increased, rising from 0.525 to 0.760 in 1 mg L–1 samples, and 0.336 to 0.638 in 100 mg L–1 during the initial 2 h, further indicating the presence of aggregation of the particles. For both concentrations, a slight decrease in z-average size is recorded at the 4 h timepoint, however, in this case PDI continued to increase, implying continued aggregation (slight reductions in size observed may be due to precipitation of the largest aggregates, leaving smaller particle species in suspension). The highest z-average size of nCeO2 were reached after 48 h entry into NSW at both concentrations, reaching average values of 2,338 ± 1,428 nm and 3,414 ± 2,027 nm for 1 and 100 mg L–1, respectively. Generally, following this the average particle size at both concentrations displayed a gradual decrease for the rest of the 240-h experiment, ultimately reaching sizes similar to those recorded at the start of the experiment. This gradual decrease likely represents the reduction in abundance of larger particles which precipitated out of the water column at a faster rate. PDI values displayed a less generalised trend and remained considerably higher than those recorded at 0 h, indicating the consistent presence of aggregates in suspension. Throughout the experiment, the deposition of nCeO2 at the bottom of flasks was observed, clearly evident from 24 h onwards, indicating the precipitation of aggregated particles (Supplementary Figure 3). Mean count rate was recorded to vary throughout the 240-h experiment (Supplementary Table 1), however, the reduction of average count rate from 225 kcps at 0 h to 108 kcps at 240 h observed in the 100 mg L–1 treatment supports the visual observation of nCeO2 sedimentation.
Short-Term (72 h) Exposure of nCeO2 Toward Prochlorococcus
Figure 2 displays the effects of nCeO2 (20.6 ± 12.1 nm) upon 72 h growth of Prochlorococcus sp. MED4. At ambient cell densities grown in NSW (Figure 1A) ANOVA revealed significant alterations in cell density of Prochlorococcus between treatments at both the 48 and 72 h timepoints (p ≤ 0.05). However, only exposure to the highest concentration (100 μg L–1) had a significant negative effect upon growth of Prochlorococcus. After 48 h incubation, cell density was decreased on average 46.6% in this treatment compared to the untreated control, increasing to 68.8% by the end of the 72-h incubation (Dunnett’s T-tests, p ≤ 0.05). At the final 72 h timepoint the average cell density of the 10 μg L–1 treatment was also lower than that of the control, however, this result was not significant. Likewise, when grown in nutrient rich Pro99 media (Figure 1B), significant variations in cell density of various treatments were identified by ANOVA at the 24 and 48 h timepoints (p ≤ 0.05). However, no negative effect upon Prochlorococcus following 72 h incubation with nCeO2 was recorded at any concentration. Significantly reduced growth was observed at the 24 h and 48 h timepoints in the 10 μg L–1 treatment, where cell density was recorded on average 14.4 and 17.8% lower than the untreated control (Dunnett’s T-tests, p ≤ 0.05). Additionally, at the 48 h timepoint a 25.2% decrease was also recorded in the 100 μg L–1 treatment (Dunnett’s T-tests, p ≤ 0.05).
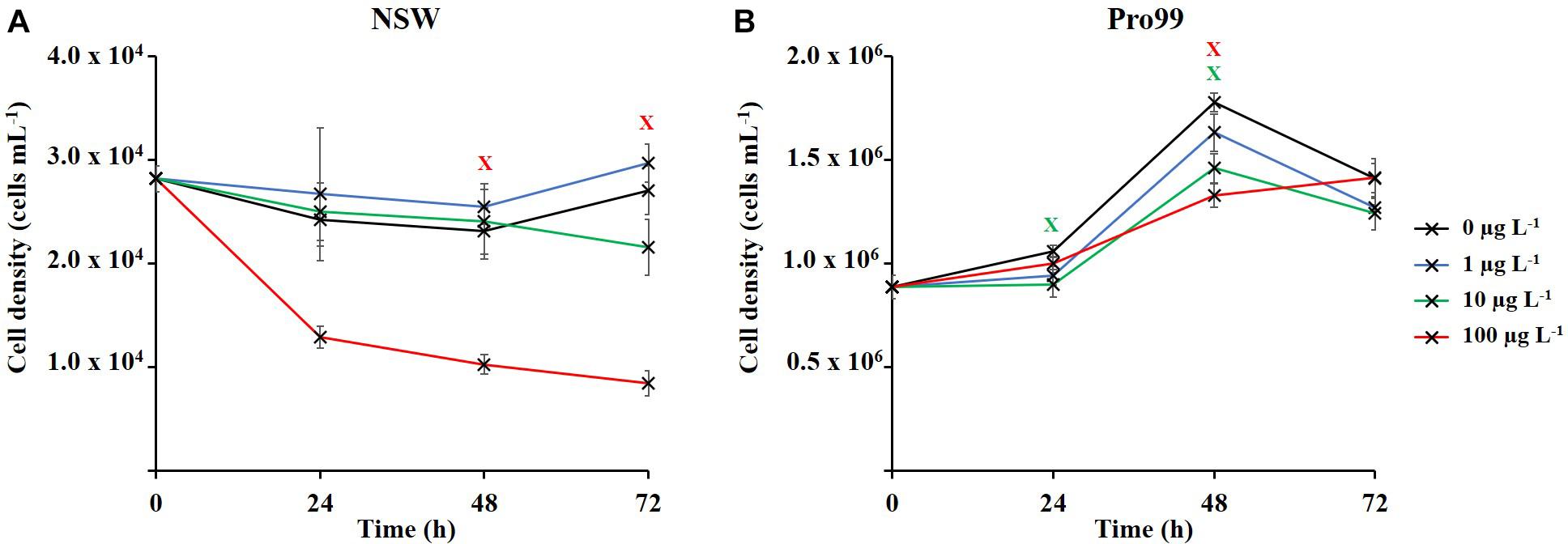
Figure 2. Seventy-two hours exposure of Prochlorococcus sp. MED4 to nCeO2 (0–100 μg L–1) in (A) natural oligotrophic seawater, and (B) nutrient-rich Pro99 media. Data points represent the mean of three culture replicates ± standard error (n = 3). Crosses indicate where Dunnett’s T-tests revealed cell density to significantly vary from the untreated control (p < 0.05).
Alterations in Cell Density of Prochlorococcus in Extended Exposure (240 h)
Environmentally Relevant Concentrations (μg L–1)
Extended (240 h) exposure of Prochlorococcus to nCeO2 is displayed in Figure 3. In accordance with short-term (72 h) experiments, the lowest tested concentrations of 1 and 10 μg L–1 had no effect on Prochlorococcus grown in NSW (Figure 3A1) throughout the 240-h experiment. As recorded in 72 h toxicity testing (section “Short-Term (72 h) Exposure of nCeO2 Toward Prochlorococcus”), exposure to 100 μg L–1 nCeO2 within NSW caused a decline in average cell density at each timepoint during the initial 72 h of exposure. Here, significant declines in cell density were recorded at both the 48 and 72 h timepoints, representing declines of 41.4 and 45.7% compared to the untreated control (Dunnett’s T-test, p ≤ 0.05). The extent of population decline in this treatment appears less severe as recorded in 72 h toxicity testing presented above (68.8%), likely due to the initial cell density of Prochlorococcus being relatively lower upon the establishment of the first experiment, indicating a possible impact of varying cell:nanoparticle ratios as recorded in previous research examining AgNP toxicity (Dedman et al., 2020). However, despite early declines, at later stages (i.e., 192 and 240 h), no significant variation was recorded between the cell density of Prochlorococcus in the 100 μg L–1 treatment and untreated cultures. Between 192 and 240 h, all cultures grown in NSW declined in cell density, likely due to nutrient depletion. In contrast, Prochlorococcus grown in the nutrient-enriched Pro99 media did not suffer any adverse effect of exposure to nCeO2 concentrations in the μg L–1 range during early stages of exposure (Figure 3A2). Due to the presence of nutrient-rich culture medium, no cell decline was expected to occur because of depleted nutrient availability as observed with cultures exposed in oligotrophic conditions. By the end of the 240-h incubation, both the 10 and 100 μg L–1 treatments were observed to reduce average cell growth. Unexpectedly, the 10 μg L–1 treatment had the greatest effect, reducing cell density on average by 27.3% compared to the untreated control. However, neither of these declines were recorded to be statistically significant. The lowest concentration of 1 μg L–1 exerted no negative impact on Prochlorococcus throughout the 240-h exposure in Pro99 media but resulted in an increase in cell density (38.8%) than the control after 240 h. However, this increase was not statistically significant.
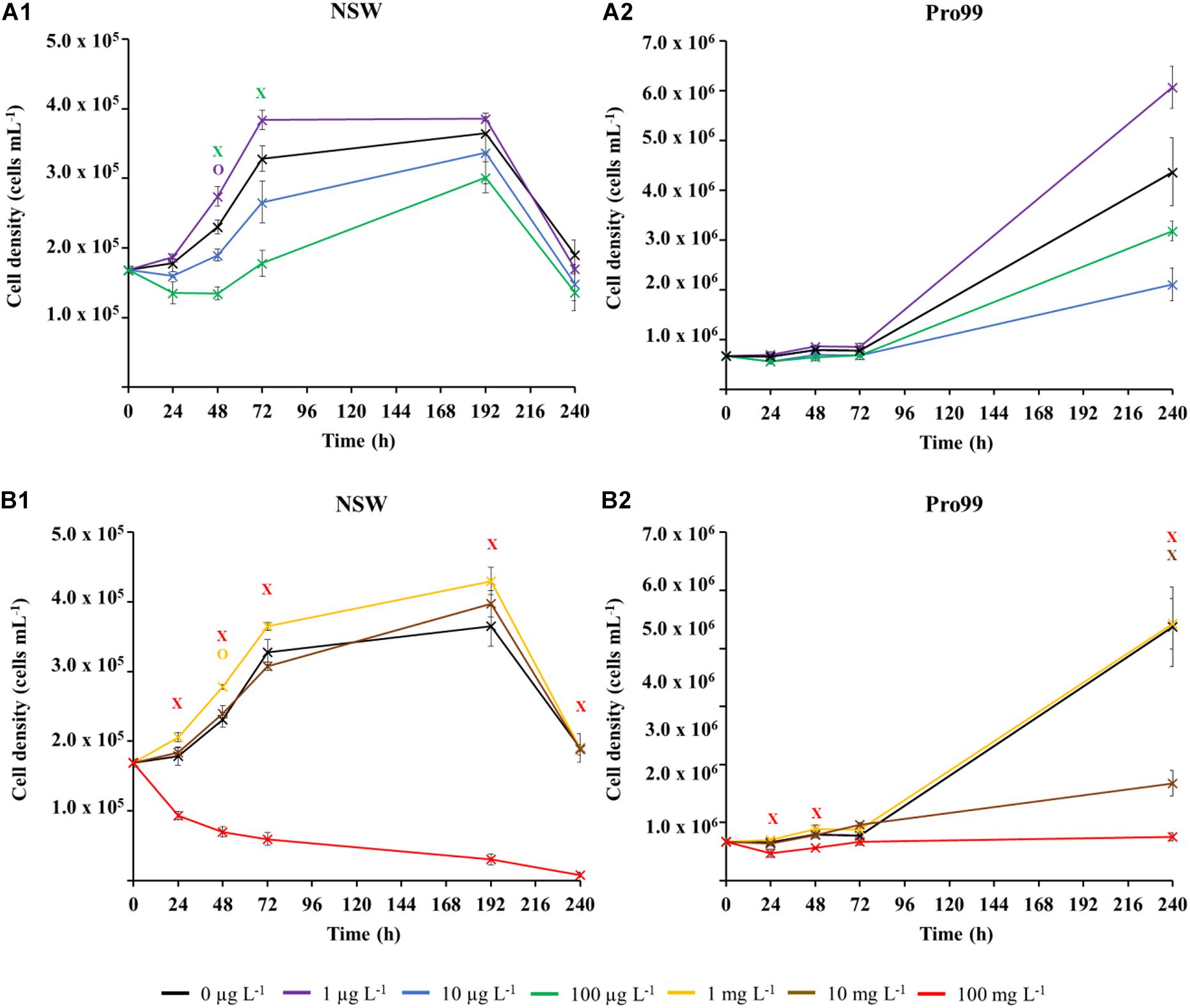
Figure 3. Extended exposure (240 h) of Prochlorococcus sp. MED4 to nCeO2 in the μg L–1 (A) and mg L–1 (B) concentration range, when exposed in natural oligotrophic seawater (A1,B1) or Pro99 media (A2,B2). Data is presented as the mean of three culture replicates ± standard error (n = 3). Dunnett’s T-tests were used to identify significant variations in cell density between nCeO2-treated cultures and the untreated control at each timepoint (p ≤ 0.05); Crosses indicate where cell density was significantly lowered in the nCeO2 treatment; Circles represent where a significant increase in cell density was recorded.
Supra-Environmental Concentrations (mg L–1)
Concentrations in the mg L–1 range were investigated to examine the impact of supra-environmental concentrations of nCeO2 should nanoparticles accumulate in the environment or their release increase. ANOVA revealed significant variations in cell density between treatment at all but one timepoint (p ≤ 0.05). In NSW (Figure 3B1) 100 mg L–1 nCeO2 caused a significant decline in Prochlorococcus at every timepoint with a maximal response observed after 192 h where cell density declined by over one order of magnitude in comparison to the untreated control, representing an approximate 95.7% decline (Dunnett’s T-test, p ≤ 0.05). Exposure to ≤10 mg L–1 nCeO2 in NSW did not appear to have any negative effect upon growth at any timepoint. As mentioned above, in NSW all cultures displayed a reduction in cell density at 240 h, this had no additional effect upon toxicity, only the highest concentration continued to exert an adverse effect. In cultures supplemented with Pro99 media (Figure 3B2), 100 mg L–1 nCeO2 also exerted a negative effect upon growth of Prochlorococcus observed at the 24, 48, and 240 h timepoints. In addition, the 10 mg L–1 treatment also resulted in a significant decrease in cell density after 240 h. By the end of the 240-h experiment, cell density was reduced approximately 61.6 and 82.9% in the 10 and 100 mg L–1 groups, respectively, in comparison with the untreated control (Dunnett’s T-test, p ≤ 0.05). As expected, throughout these supra-concentration treatments, small aggregates were observed by flow cytometry and large aggregates were visible at the bottom of the culture flasks confirming nanoparticle aggregation and possible cyanobacteria-nanoparticle aggregation which deserved further investigation.
Physical Cyanobacterial-nCeO2 Interactions
nCeO2 aggregates alone do not fluoresce and, hence, would not be picked up during flow cytometric analysis. Nevertheless, the occurrence of large fluorescent particles were observed only at mg L–1 treatments and are believed to represent hetero-aggregates of Prochlorococcus cells and nCeO2. By gating these aggregates using FACSDiva software, it was possible to estimate their abundance relative to free Prochlorococcus cells (described above, section “Alterations in Cell Density of Prochlorococcus in Extended Exposure (240 h)”) and reference beads. Here, stocks of NSW in the absence of cyanobacteria and nCeO2, as well as untreated cultures were utilised to control for the occurrence of such hetero-aggregated material. It should be noted that no such events were recorded during analysis of samples derived from cultures exposed to nCeO2 in the μg L–1 range and therefore these are not described herein.
The estimated concentration of cyanobacterial-nCeO2 aggregates in both NSW and Pro99 media, recorded at the 24, 48, 72, and 240 h timepoints is presented in Figure 4. The number of aggregates in suspension appeared considerably higher in cell-dense cultures grown in Pro99 media compared to those grown in NSW, reaching peak concentrations of 2.8 × 105 and ∼7.0 × 104 aggregates mL–1, respectively, at the 24 h timepoint. In NSW, aggregate density appeared to follow a dose-wise trend, increasing with increased concentration, however, this was not the case at the 240 h timepoint, where it is likely that aggregated material had precipitated out of the media and was therefore not present in the suspended fraction monitored by flow cytometry. In Pro99 media, this dose-wise trend was not observed, and highest values were recorded in the 10 mg L–1 treatment. Notably, aggregate density decreased rapidly throughout the course of the experiment, up to 73% decrease recorded between the 24 and 48 h timepoints. This decrease is likely the result of rapid aggregation and precipitation of large, aggregated material due to increased density and, interestingly, at the last time point i.e., 240 h the number of aggregates estimated at each concentration were in the same order of magnitude in both NSW and Pro99 media (Figure 4).
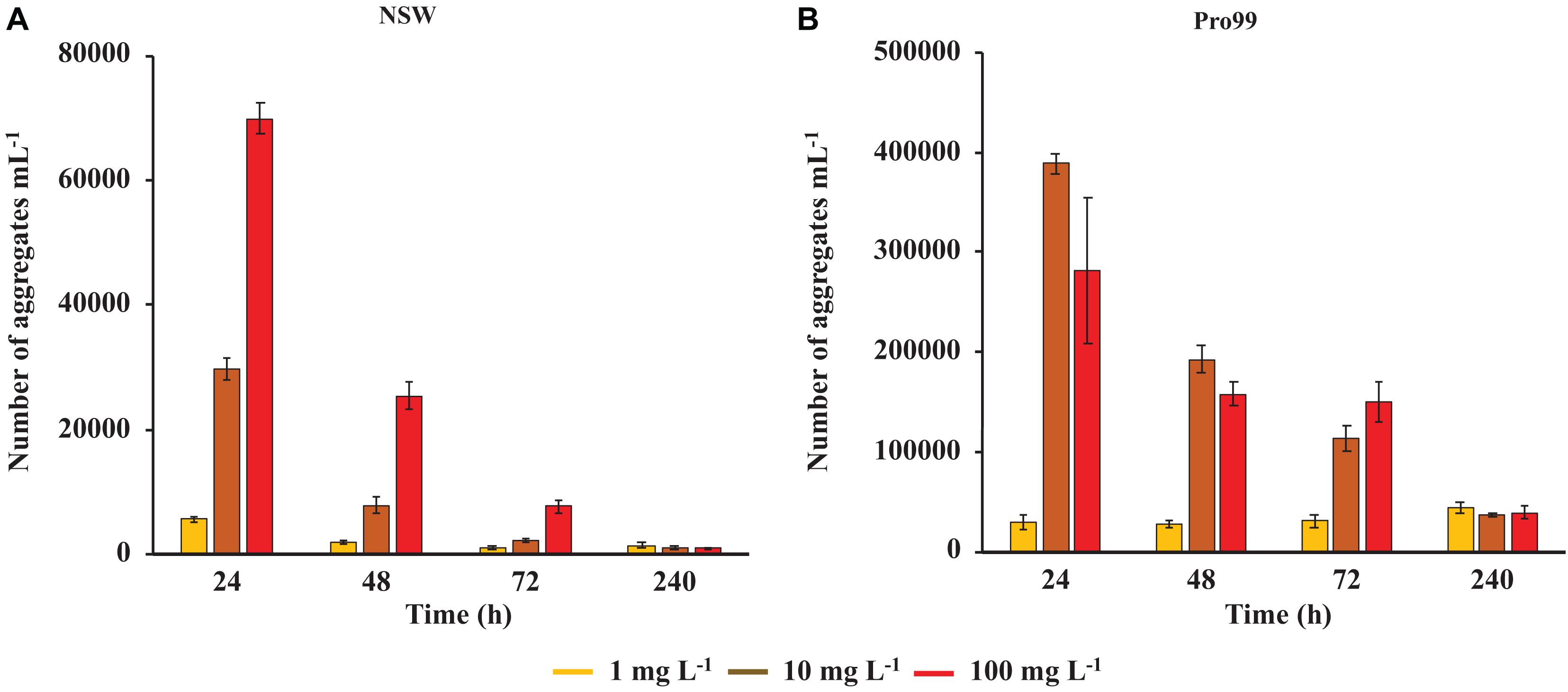
Figure 4. Estimated number of cyanobacterial-nCeO2 aggregates as monitored during flow cytometric analysis of Prochlorococcus sp. MED4 cultures grown in NSW (A) or Pro99 media (B) exposed to nCeO2 in the mg L–1 range (1–100 mg L–1). Data represents the mean of three culture replicates ± standard error (n = 3). No such aggregates were observed during analysis of cultures exposed to lower concentrations (i.e., 1–100 μg L–1).
Fluorescent microscopy was utilised to confirm the presence of cyanobacteria within aggregated material. Sub-samples were collected from the bottom of culture flasks and imaged using brightfield and GFP fluorescent channels after staining with SYBR Gold nuclear stain (Figure 5 and Supplementary Figure 4). Here, clear evidence of cyanobacterial-nCeO2 hetero-aggregation was observed at concentrations of 10 and 100 mg L–1 in both NSW (Figures 5A,B) and Pro99 media (Figures 5C,D). Observed hetero-aggregates reached sizes in the 10s-of-micron range but showed great variation in size and morphology. Some evidence of hetero-aggregation was observed within the 1 mg L–1 treatment; however, this was not apparent in cultures exposed to lower concentrations (1–100 μg L–1).
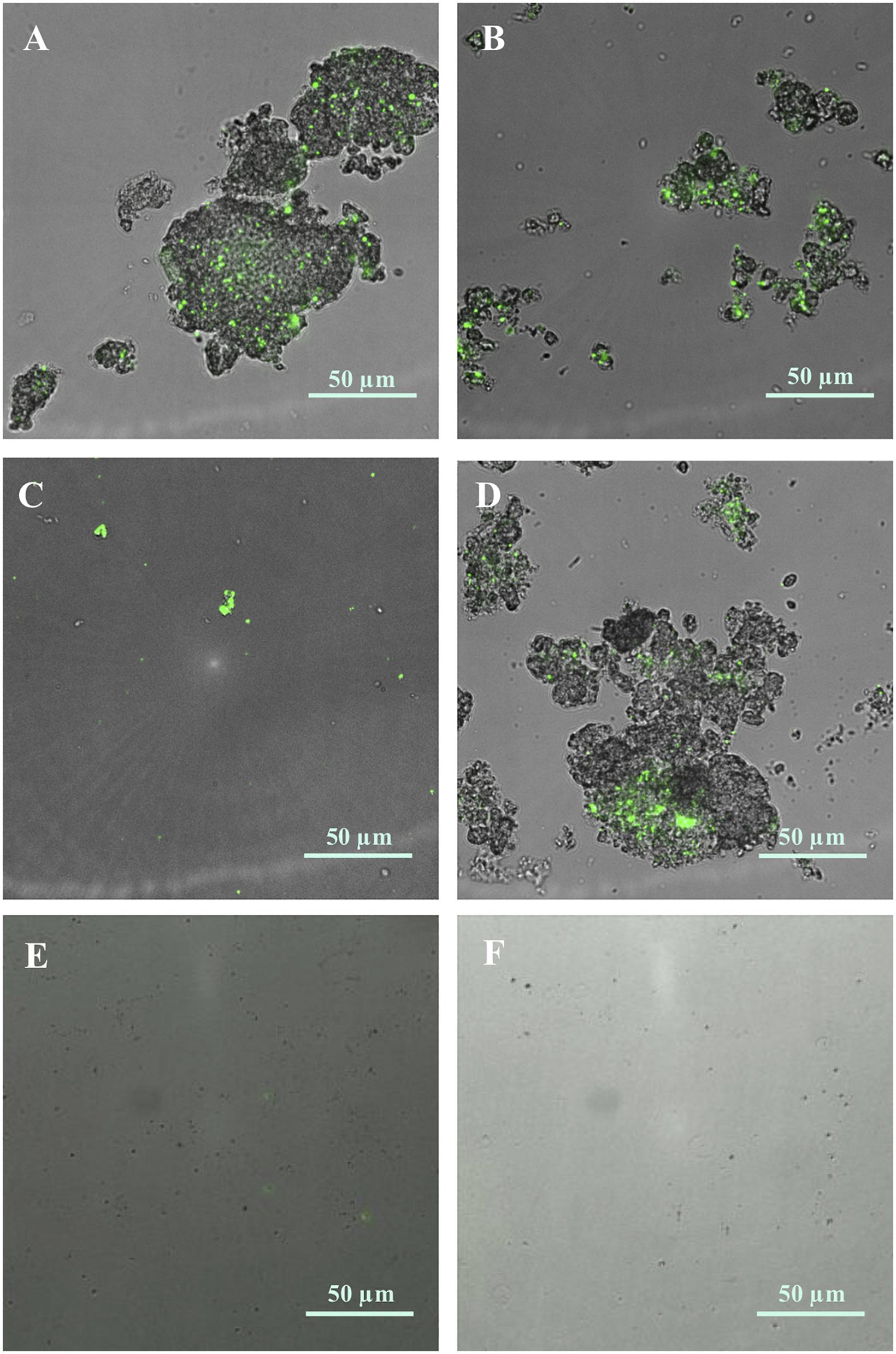
Figure 5. Hetero-aggregation of Prochlorococcus sp. MED4 (green) and nCeO2 (10 and 100 mg L–1) formed in natural oligotrophic seawater [(A); 10 mg L–1 and (B); 100 mg L–1] and Pro99 media [(C); 10 mg L–1 and (D); 100 mg L–1)]. Images were captured using fluorescent microscopy under brightfield and GFP fluorescent and subsequently were merged. No such aggregates were visible in Prochlorococcus culture (E) or natural oligotrophic seawater (F) in the absence of nanoparticles.
Discussion
Herein, the toxicity of the emerging nano-pollutant nCeO2 toward the marine cyanobacterium Prochlorococcus sp. MED4 was examined under environmentally relevant (ambient cell density within oligotrophic NSW) and optimal growth (cell-dense cultures grown in nutrient-enriched Pro99 seawater) conditions during short-term and extended exposure. Investigation revealed a potential for nCeO2 to exert significant declines in cyanobacterial cell density, dependent on nCeO2 concentration, culture media and exposure length. Examination of nCeO2 behaviour within NSW, and during incubation with cyanobacteria, displays the extensive aggregation of nCeO2 under saline conditions and ability to form hetero-aggregates with microbial cells. The physical interaction between cyanobacteria and nCeO2 is believed the key driver of significant alterations to cell density recorded during laboratory investigation.
It is widely acknowledged that the fate and behaviour of nanomaterials within the natural environment will determine their bioavailability and hence likely effect upon biota (Rodea-Palomares et al., 2010; Hazeem et al., 2016; Thiagarajan et al., 2019). Upon entry into the aquatic environment, nCeO2 may be transported freely in suspension, aggregate to other nCeO2 particles (homo-aggregation) or aggregate to other particulate matter (hetero-aggregation). To improve our understanding of nCeO2 behaviour in the marine environment, the aggregation behaviour of nanoparticles in NSW was assessed using DLS for a period of 240 h as previously described (Petosa et al., 2010; Afrooz et al., 2014; Clavier et al., 2019). Concentrations of 1 and 100 mg L–1 were selected for analysis, as limitations of DLS at lower concentrations meant that environmental concentrations (i.e., μg L–1) could not be accurately measured (Handy et al., 2012). Evidence of nCeO2 aggregation was recorded immediately upon entry into saline media, reaching sizes of up to 1,293 ± 141 nm, considerably higher than the primary particle size determined by TEM (20.6 ± 12.1 nm). Average size of nCeO2 remained high throughout the 240-h experiment, indicating that particles remained in an aggregated state, with a slight decrease recorded in later stages suggesting the possible sedimentation of larger aggregated material. The aggregation of particles causes a reduction in the surface-area-to-volume ratio of nanomaterials and is likely to influence the toxicity and reactivity of particles (Lowry et al., 2012). Following aggregation, particles may sink through the water column due to increased mass and sedimentation may occur. This process was evident through visual inspection of nCeO2 suspensions and supported by mean count data at high concentrations (100 mg L–1).
In accordance with previous research, it is clear that nCeO2 is likely to aggregate upon entry into saline media with high ionic strength such as seawater (Keller et al., 2010; Ottofuelling et al., 2011; Quik et al., 2014; Sendra et al., 2017). In the natural environment a number of additional factors will also govern the fate and behaviour of engineered nanomaterials, including; particle specific physicochemical properties (Dale et al., 2017) and local water chemistry with pH, natural organic matter (NOM) and particulate matter all playing influential roles (Quik et al., 2010; Collin et al., 2014; Velzeboer et al., 2014; Van Koetsem et al., 2015). For example, in the presence of NOM, Quik et al. (2010) observed up to 88% of nCeO2 added to deionised water to remain stable in suspension. Therefore, the presence of NOM in natural waters is likely to influence particle stability, although this will also largely depend on specific surface characteristics of nanoparticles. Hence, in our work we utilised NSW to simulate natural waters that nCeO2 may enter. Given nCeO2 nanoparticles’ propensity to aggregate and undergo sedimentation in saline media, they have the potential to interact with marine biota throughout the water column as they are transported toward deeper zones.
Flow cytometry was used to monitor alterations in populations of the marine cyanobacterium Prochlorococcus. It is known that dead cells of Prochlorococcus lose fluorescence rapidly (Christie-Oleza et al., 2017; Roth-Rosenberg et al., 2020), therefore flow cytometry can be used to monitor changes in the viable cyanobacterial population. During short-term (up to 72 h) exposure, presence of μg L–1 nCeO2 was recorded to significantly reduce the cell density of marine cyanobacterium Prochlorococcus, when grown under environmentally relevant conditions in NSW, causing up to a 68.8% decrease in cell density. However, no such effect was observed in cultures grown to higher cell densities in nutrient rich Pro99 media after 72 h exposure, highlighting the influence of specific exposure conditions. Upon extending incubations to 240 h; the trend observed in early stages of exposure (<72 h) continued. However, despite the significant declines recorded during the initial 72 h to 100 μg L–1 nCeO2, Prochlorococcus cultures grown in NSW were observed to recover to cell densities comparable to that of the untreated control by the 192 h timepoint. Whilst in short-term exposure, cell-dense cultures appeared more resilient to nCeO2, in the long-term this trend was reversed as cultures grown in Pro99 media experienced declines in average cell density up to 27.3% in response to both 10 and 100 μg L–1 treatments.
To further evaluate the impact of nCeO2 should emissions into the environment rise or hotspots of pollution occur due to accumulation of nanoparticles, the response of Prochlorococcus toward supra-environmental concentrations was also assessed. In both NSW and Pro99 exposure to the highest concentration (100 mg L–1) exerted a negative impact upon Prochlorococcus, causing a maximum decline in cell density of 95.7%. As with lower concentrations, differences in response between the two culture conditions could also be observed in mg L–1 treatments. Whilst 10 mg L–1 nCeO2 exerted no effect on Prochlorococcus grown in NSW, cell-dense cultures experienced a 61.6% decrease in cell density by the end of the experiment. The enhanced cell decline observed in cell-dense cultures, may arise due to increased likelihood of encounter between nanoparticles and cyanobacteria. Previous research has also shown that cultures grown to higher cell densities suffer greater adverse effect to metal oxide nanomaterial exposure (Bessa da Silva et al., 2016). Interestingly, following 240 h exposure no effect was recorded in 1 and 10 mg L–1 nCeO2 treatments in NSW, or the 1 mg L–1 treatment in Pro99 media. However, declines were observed at lower concentration at specific timepoints (10 and 100 μg L–1). We propose that the difference in effect caused by these treatments is likely due to the extensive aggregation of nCeO2 upon entering saline media. It is possible that in μg L–1 treatments, stability of nCeO2 in suspension is increased following lowered homo-aggregation due to reduced rate of encounter between individual nCeO2 particles, hence facilitating their interaction with planktonic cells. In contrast, at higher concentrations (1–10 mg L–1) homo-aggregation occurs at a higher rate causing nCeO2 to aggregate and sink before interacting with cyanobacteria. At the highest concentration (100 mg L–1) where effects are most severe, and in the 10 mg L–1 in cell-dense cultures where particle-cell contact is more likely, the aggregation process is extensive enough to significantly reduce Prochlorococcus numbers.
Adverse effects associated with nCeO2 exposure have previously been recorded in studies with marine phytoplankton (Deng et al., 2017). The diatom P. tricornutum suffered adverse effects following exposure to 10–30 nm nanoparticles at concentrations >10 mg L–1, resulting in significant reductions in growth (Deng et al., 2017). However, interestingly, lower concentrations <5 mg L–1 were recorded to stimulate growth of this diatom (Deng et al., 2017). Such enhanced growth following exposure to nCeO2 has also been recorded in marine algal species (Sendra et al., 2018).
Due to the limited evidence available, the exact mechanisms by which nCeO2 drives significant declines in phytoplankton abundance remain unclear. Based on work in freshwater, nCeO2 is thought to exert oxidative stress upon microbial species via photocatalytic ROS production (Rogers et al., 2010). This suggests that the presence of natural light may be a key factor in determining toxicity of nCeO2, hence in our study full-spectrum bulbs were used to best simulate natural conditions. Oxidative stress and associated lipid peroxidation have been recorded in the marine diatom P. tricornutum in response to nCeO2 (Deng et al., 2017). Although, whether such effects arise due to the nanoparticles’ presence, or as a consequence of physical interactions with cells is not clear. In that work, concentrations of 10–40 mg L–1 led to considerable increases in activity of superoxide dismutase, peroxidase and malondialdehyde, associated with oxidative stress and lipid peroxidation, respectively (Deng et al., 2017). It is possible ROS production and associated oxidative stress may have played a role in the significant declines of Prochlorococcus recorded during our study. However, it has also been suggested that due to nCeO2 being a redox catalyst, nanoparticles display the potential to both mitigate, as well as exert, oxidative stress (Arnold et al., 2013; Pulido-Reyes et al., 2015). For example, nCeO2 has been recorded to display antioxidant activities toward secondary oxidative stress exerted upon phagocytic and human bronchial epithelial cell lines (Xia et al., 2008), and during incubation with phytoplankton (Sendra et al., 2017). It is possible any antioxidant properties may account for the enhancement of phytoplankton growth recorded in previous research in response to nCeO2, and lack of decline observed at concentrations of 1 and 10 mg L–1 observed in our study. Although, such enhanced growth can also be attributed to hormetic effects (Mattson, 2008). The exact mechanisms by which nCeO2 exerts and mitigates oxidative stress requires attention, particularly in seawater. Additionally, nCeO2 exposure has been linked to a decrease in photosynthetic activity (Taylor et al., 2016; Deng et al., 2017), as well as reducing carbon fixation in freshwater phytoplankton (Taylor et al., 2016), suggesting phototoxic properties of nCeO2. Deng et al. (2017) recorded a decline in quantum yield of PSII by approximately 29% following 96 h exposure to nCeO2 in the diatom P. tricornutum. However, such effects are only observed at relatively high concentrations (2.5–40 mg L–1) (Taylor et al., 2016). As such, it is unlikely photosynthetic processes will be adversely affected by nCeO2 in the natural marine environment at current levels.
During exposures of Prochlorococcus to nCeO2 the formation and precipitation of aggregates of cyanobacteria and nanoparticles (which aggregate readily in the high ionic strength of seawater, as demonstrated and described by DLS analysis) were visible in culture flasks. Whilst monitoring cyanobacterial populations by flow cytometry, it was possible to estimate the number of hetero-aggregates within suspension (Figure 4). Only freely suspended Prochlorococcus were used to calculate cell density presented in the work above (section “Alterations in Cell Density of Prochlorococcus in Extended Exposure (240 h)”) as those entrapped within hetero-aggregates would likely be removed from the water column by co-precipitation with nCeO2. The presence of cyanobacteria within precipitated aggregates of nCeO2 was confirmed by carrying out fluorescent microscopy on precipitated material collected from the bottom of culture flasks that was subsequently stained with SYBR Gold (Figure 5). Here, Prochlorococcus was clearly visible within aggregates of nCeO2 which reached sizes in the 10s-of-micron range. The hetero-aggregation between metal oxide nanomaterials and phytoplankton has previously been reported in the literature (Rodea-Palomares et al., 2010; Manzo et al., 2015; Xia et al., 2015; Wang et al., 2016; Morelli et al., 2018; Dedman et al., 2021), and has been observed with nCeO2 during 96 h exposure (Deng et al., 2017). It is our belief that the process of entrapment and subsequent co-precipitation of cyanobacteria and nCeO2 from the water column (Figure 6) is a key mechanism behind the temporary declines in Prochlorococcus presented herein (sections “Short-Term (72 h) Exposure of nCeO2 Toward Prochlorococcus” and “Alterations in Cell Density of Prochlorococcus in Extended Exposure (240 h)”). However, given the various impacts of nCeO2 upon phytoplankton physiology described above, further testing such as proteomic analysis and the determination of ROS generation is required to confirm this.
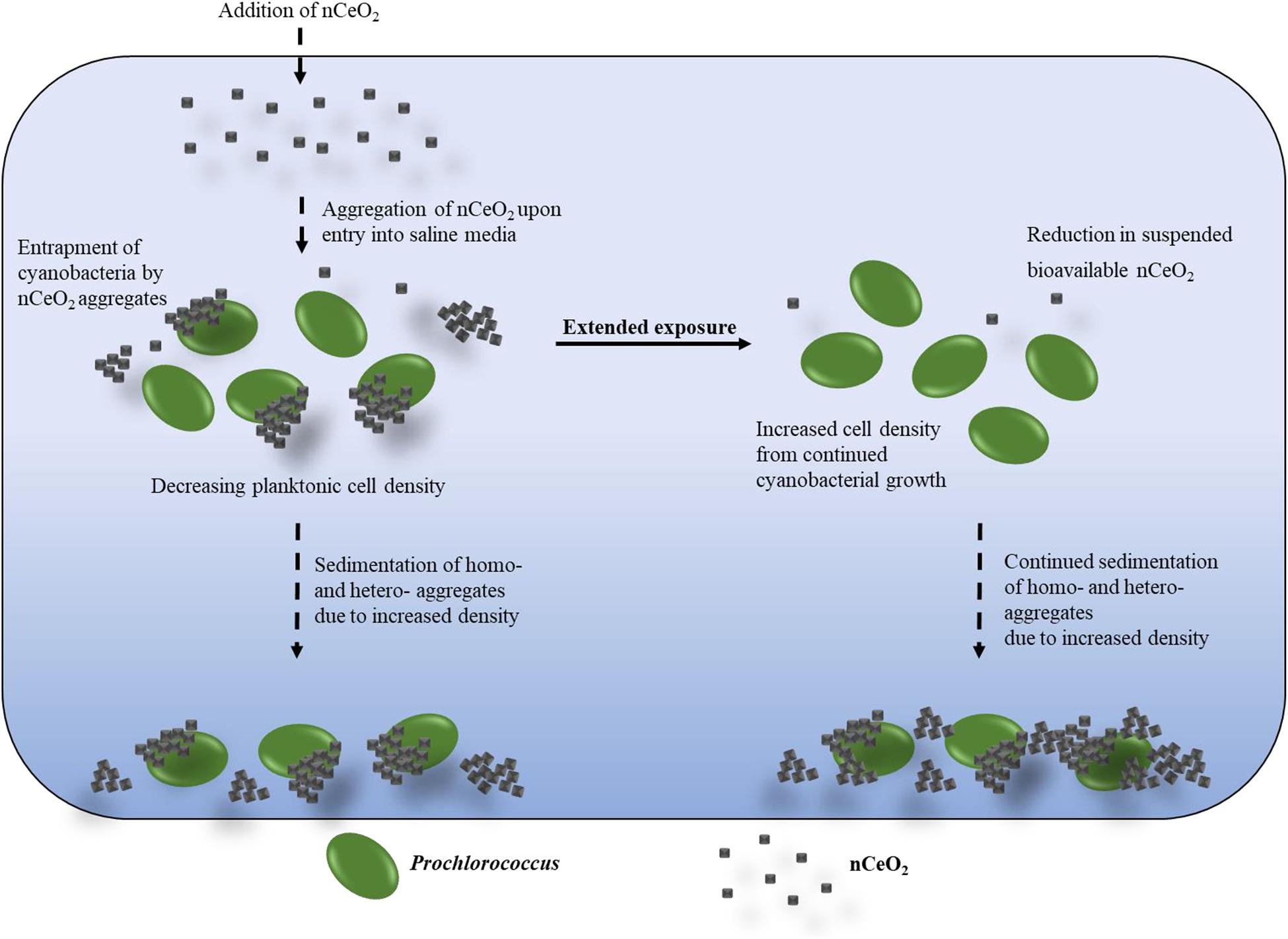
Figure 6. Graphical representation of the proposed mode of cell decline recorded in exposures of Prochlorococcus to nCeO2.
As expected, the estimated number of hetero-aggregates was substantially higher in cell-dense cultures (Figure 4B) than those grown in NSW (Figure 4A), likely due to the increased rate of encounter between nanoparticles and cyanobacteria. The number of hetero-aggregates in suspension within culture flasks was recorded to decline rapidly, with up to 73% decrease between the 24 and 48 h timepoints, suggesting their rapid precipitation out of the water column along with attached cyanobacteria. Previous research using the filamentous cyanobacterium, Anabaena CPB4337, also observed physical interaction between nCeO2 and cyanobacterial cells (Rodea-Palomares et al., 2010). Here, positively charged particles appeared to be attracted to the negatively charged cell walls of cyanobacteria (Rodea-Palomares et al., 2010). The researchers proposed that toxicity may be induced by the direct contact of nCeO2 to the cell wall; causing mechanical damage to the cell membrane, disrupting the exchange of nutrients and metabolites and exerting oxidative stress (Rodea-Palomares et al., 2010). The same research group investigated the effects of nCeO2 upon P. subcapitata in freshwater, observing damage to the cell membrane, and leakage of the cytoplasm (Rodea-Palomares et al., 2010). Once again, this was believed to result from direct contact between nanoparticles and cells. Similar findings upon P. subcapitata have been recorded in previous studies (Rogers et al., 2010), providing further evidence that direct contact induces the toxicity of nCeO2. Hetero-aggregation between phytoplankton and metal oxide nanomaterials has also been proposed to cause shading effects which may reduce photosynthetic efficiency (Hu et al., 2018), possibly causing the declines in photosynthetic output previously described (Taylor et al., 2016; Deng et al., 2017). Thus, it is concluded by Rodea-Palomares et al. (2010) that the cytotoxicity of nCeO2 requires direct contact of particles to the cell wall or membrane. During this process it is likely that physical damage to cells will be caused, such as that described above, although additional study is required to confirm this. The belief that physical effects are a key driver of cyanobacterial decline in our work, rather than oxidative stress, is supported by the fact that Prochlorococcus is highly susceptible to ROS and is sensitive to H2O2 concentrations as low as 200 nM (Morris et al., 2011; Zinser, 2018). Hence, given that the viable free-living population recorded by flow cytometry is able to recover to ambient cell densities in extended exposure, this suggests that ROS-mediated stress is likely not a feature of our work.
Over time the concentration of suspended nCeO2 in Prochlorococcus cultures will display a continual decline, hence reducing its bioavailability and allowing the surviving cyanobacterial population to recover, as observed in Prochlorococcus populations grown in NSW (Figure 6). It is important to note that in our work, uncoated nCeO2 was utilised during experiments. The behaviour of these nanoparticles likely differs to those which are surface-modified, likely playing a key role in the effects recorded herein. It has been suggested that surface characteristics influence differences in toxicity by altering NM bioavailability (Booth et al., 2015). For example, in contrast to the nanoparticles used in our work, Poly-(acylic acid)-stabilised nCeO2 appear well-dispersed in saline media, remaining biologically available to species occupying the water column, and hence enhancing toxicity toward these organisms (Booth et al., 2015). However, findings vary and appear case-specific. Dextran-coated nCeO2 have been recorded to exert negligible toxicity upon E. coli (Shah et al., 2012), believed likely due to stabilisation of the oxidative state nanoparticles by the polymer coating, as well as a reduction in contact between particles and cells (Shah et al., 2012). Going forward it will be increasingly important to assess the surface characteristics of nCeO2 entering the environment, such that greater insight into their likely fate and interaction with biota can be gained.
The occurrence of hetero-aggregation between nanoparticles and phytoplankton may increase the risk of bioaccumulation and transfer of particles to higher trophic levels by ingestion. Evidence of trophic transfer of nCeO2 has previously been reported in a terrestrial plant model (Hawthorne et al., 2014). However, it must be noted that the widespread occurrence of hetero-aggregation between phytoplankton and nanoparticles recorded in laboratory investigation is likely driven at least partially by the relatively high concentrations examined and the closed-system nature of most experimental set-ups. As a result, such physical interactions between cells and nanoparticles are less likely in the natural environment at lower concentrations where nanoparticles appear more dilute and may alternatively interact with other particulate matter.
The recovery of Prochlorococcus recorded under environmentally relevant conditions in extended exposure and lack of adverse effect of concentrations <10 mg L–1 in NSW suggests that at current, the likely environmental risk of nCeO2 exposure toward marine microbial species under natural conditions is low. The recovery of microbial populations in long-term exposure has previously been recorded during investigation with metal oxide nanomaterials (Hazeem et al., 2016; Li et al., 2019; Mitchell et al., 2019), often attributed to the aggregation behaviour of metal oxide nanomaterials, leading to their reduced bioavailability over time (Hazeem et al., 2016; Thiagarajan et al., 2019), in accordance with DLS analysis and microscopic imaging described above. This evidence suggests that similarities between the impact of various metal oxide nanomaterials exist, allowing researchers to better understand their likely effect.
Conclusion
In this study we provide new insight into the effect of nCeO2 upon marine microbial species using the ecologically significant marine cyanobacterium Prochlorococcus. To date, limited research has been carried out to assess the potential impact of this emerging contaminant upon marine microbial organisms, and hence the works presented herein address this gap in knowledge. Additionally, information is provided on the possible ecotoxic effects of engineered NMs upon an ecologically significant marine cyanobacterium, for which little ecotoxicological data exists. We have shown that despite evidence of short-term (<72 h) declines in cell density, nCeO2 appears to exert little toxicity toward Prochlorococcus under natural conditions in the longer term, except at exceptionally high concentrations (i.e., 100 mg L–1). Cultures grown to higher cell densities in nutrient-rich media appear to suffer greater effects of exposure when incubations are extended to 240 h, believed due to the increased rate of encounter between cyanobacteria and nanoparticles. The occurrence of hetero-aggregation between nCeO2 and Prochlorococcus is a key feature of exposure, in accordance with a number of studies examining the ecotoxicity of metal oxide NMs, (Rodea-Palomares et al., 2010; Manzo et al., 2015; Xia et al., 2015; Wang et al., 2016; Morelli et al., 2018) driven by aggregation behaviour of nCeO2 in saline media confirmed by DLS. The processes of entrapment and subsequent co-precipitation of nanoparticles and cyanobacteria are believed to be important mechanisms of the declines in cell number recorded, although additional testing is required to confirm this. Over time, the concentration of suspended nCeO2 is reduced, hence reducing the likelihood of interaction between nanoparticles and biota, allowing the remaining free-living cyanobacterial population to resume normal growth. However, it remains that the mechanism of cell decline observed in laboratory investigation is far less likely to occur at the low nCeO2 concentrations expected in the natural environment, where dilution effects likely limit the extent of both homo-aggregation and hetero-aggregation with phytoplankton, where nanoparticles may also interact with natural colloids or NOM. As such, in accordance with previous research (Johnson and Park, 2012), it appears that the likely environmental risk of nCeO2 toward marine microorganisms is low. One drawback from the works presented herein is the use of pristine research-grade nanomaterials. Such particles may not accurately represent those particles released into the environment. nCeO2 is likely to undergo transformation upon its release into the environment, particularly nCeO2 released from fuel via combustion (Dale et al., 2017). Echoing the beliefs of Dale et al. (2017), our future experimental work will focus upon investigating the environmental impact of nCeO2 and other metal oxide nanomaterials using materials that best represent those released into the environment. It will be important to comprehensively characterise physicochemical properties as well as nanoparticle state in the environment. Here, synthetic approaches may be a useful tool to produce nanomaterials which accurately match environmental particles. Efforts must also be directed toward accurately predicting the likely environmental concentration of nCeO2, where marine transport may contribute substantially to the entry of nanoparticles into the ocean. In future studies, experiments conducted on whole communities may aid analysis of impacts upon ecological function and identify any taxonomic groups particularly susceptible to exposure.
Data Availability Statement
The raw data supporting the conclusions of this article will be made available by the authors, without undue reservation.
Author Contributions
CD performed all experiments, data acquisition, data analysis, and wrote the manuscript. MR assisted with nanomaterial characterisation. G-LD and JC-O conceived the idea, assisted with data analysis and interpretation and co-wrote the manuscript. All authors contributed to the article and approved the submitted version.
Funding
CD was supported by the NERC CENTA DTP studentship NE/L002493/1. JC-O was funded by a NERC Independent Research Fellowship NE/K009044/1, Ramón y Cajal contract RYC-2017-22452 (funded by the Ministry of Science, Innovation and Universities, the National Agency of Research, and the European Social Fund) and Project PID2019-109509RB-I00/AEI/10.13039/501100011033. In addition, we thank the BBSRC/EPSRC Synthetic Biology Research Centre WISB (Grant Ref.: BB/M017982/1) for access to equipment.
Conflict of Interest
The authors declare that the research was conducted in the absence of any commercial or financial relationships that could be construed as a potential conflict of interest.
Acknowledgments
We thank to our respective funding agencies and technical staff at the University of Warwick for their support during the completion of experimental work. In particular, thanks are given to the WISB team and Advanced Bioimaging RTP.
Supplementary Material
The Supplementary Material for this article can be found online at: https://www.frontiersin.org/articles/10.3389/fmars.2021.668097/full#supplementary-material
References
Afrooz, A. R. M. N., Hussain, S. M., and Saleh, N. B. (2014). Aggregate size and structure determination of nanomaterials in physiological media: importance of dynamic evolution. J. Nanopart. Res. 2771:16.
Arnold, M. C., Badireddy, A. R., Wiesner, M. R., Di Giulio, R. T., and Meyer, J. N. (2013). Cerium oxide nanoparticles are more toxic than equimolar bulk cerium oxide in Caenorhabditis elegans. Arch. Environ. Contam. Toxicol. 65, 224–233. doi: 10.1007/s00244-013-9905-5
Bagby, S. C., and Chisholm, S. W. (2015). Response of Prochlorococcus to varying CO2:O2 ratios. ISME J. 9, 2232–2245. doi: 10.1038/ismej.2015.36
Bessa da Silva, M., Abrantes, N., Nogueira, V., Goncalves, F., and Pereira, R. (2016). TiO2 nanoparticles for the remediation of eutrophic shallow freshwater systems: efficiency and impacts on aquatic biota under a microcosm experiment. Aquat. Toxicol. 178, 58–71. doi: 10.1016/j.aquatox.2016.07.004
Booth, A., Storseth, T., Altin, D., Fornara, A., Ahniyaz, A., Jungnickel, H., et al. (2015). Freshwater dispersion stability of PAA-stabilised cerium oxide nanoparticles and toxicity towards Pseudokirchneriella subcapitata. Sci. Total Environ. 505, 596–605. doi: 10.1016/j.scitotenv.2014.10.010
Calvache-Munoz, J., Prado, F. A., and Rodriguez-Paez, J. E. (2017). Cerium oxide nanoparticles: synthesis, characterization and tentative mechanism of particle formation. Coll. Surf. A Physicochem. Eng. Asp. 529, 146–159. doi: 10.1016/j.colsurfa.2017.05.059
Charron, A., and Harrison, R. M. (2005). Fine (PM2.5) and coarse (PM2.5-10) particulate matter on a heavily trafficked London highway: sources and processes. Environ. Sci. Technol. 39, 7768–7776. doi: 10.1021/es050462i
Christie-Oleza, J. A., Sousoni, D., Lloyd, M., Armengaud, J., and Scanlan, D. J. (2017). Nutrient recycling facilitates long-term stability of marine microbial phototroph-heterotroph interactions. Nat. Microbiol. 2:17100.
Clavier, A., Praetorius, A., and Stoll, S. (2019). Determination of nanoparticle heteroaggregation attachment efficiencies and rates in presence of natural organic matter monomers. Monte Carlo modelling. Sci. Total Environ. 650 (Pt 1), 530–540. doi: 10.1016/j.scitotenv.2018.09.017
Clement, L., Hurel, C., and Marmier, N. (2013). Toxicity of TiO(2) nanoparticles to cladocerans, algae, rotifers and plants – effects of size and crystalline structure. Chemosphere 90, 1083–1090. doi: 10.1016/j.chemosphere.2012.09.013
Collin, B., Auffan, M., Johnson, A. C., Kaur, I., Keller, A. A., Lazareva, A., et al. (2014). Environmental release, fate and ecotoxicological effects of manufactured ceria nanomaterials. Environ. Sci. Nano 1, 533–548. doi: 10.1039/c4en00149d
Dale, J. G., Cox, S. S., Vance, M. E., Marr, L. C., and Hochella, M. F. Jr. (2017). Transformation of cerium oxide nanoparticles from a diesel fuel additive during combustion in a diesel engine. Environ. Sci. Technol. 51, 1973–1980. doi: 10.1021/acs.est.6b03173
Das, S., Dowding, J. M., Klump, K. E., McGinnis, J. F., Self, W., and Seal, S. (2013). Cerium oxide nanoparticles: applications and prospects in nanomedicine. Nanomedicine (Lond.) 8, 1483–1508. doi: 10.2217/nnm.13.133
Dedman, C. J., King, A. M., Christie-Oleza, J., and Davies, G.-L. (2021). Environmentally relevant concentrations of titanium dioxide nanoparticles pose negligible risk to marine microbes. Environ. Sci. Nano. doi: 10.1039/D0EN00883D
Dedman, C. J., Newson, G. C., Davies, G.-L., and Christie-Oleza, J. A. (2020). Mechanisms of silver nanoparticle toxicity on the marine cyanobacterium Prochlorococcus under environmentally-relevant conditions. Sci. Total Environ. 747, 141229. doi: 10.1016/j.scitotenv.2020.141229
Demir, V., Ates, M., Arslan, Z., Camas, M., Celik, F., Bogatu, C., et al. (2015). Influence of Alpha and Gamma-iron oxide nanoparticles on marine microalgae species. Bull. Environ. Contam. Toxicol. 95, 752–757. doi: 10.1007/s00128-015-1633-2
Deng, X. Y., Cheng, J., Hu, X. L., Wang, L., Li, D., and Gao, K. (2017). Biological effects of TiO2 and CeO2 nanoparticles on the growth, photosynthetic activity, and cellular components of a marine diatom Phaeodactylum tricornutum. Sci. Total Environ. 575, 87–96. doi: 10.1016/j.scitotenv.2016.10.003
European Commission (2012). Commission Staff Working Paper: Types and uses of Nanomaterials, Including Safety Aspects. Companying the Communication from the Commission to the European Parliament, the Council and the European Economic and Social Committee on the Second Regulatory Review on Nanomaterials. Brussels: European Commission.
Field, C. B., Behrenfeld, M. J., Randerson, J. T., and Falkowski, P. (1998). Primary production of the biosphere: integrating terrestrial and oceanic components. Science 281, 237–240. doi: 10.1126/science.281.5374.237
Flombaum, P., Gallegos, J. L., Gordillo, R. A., Rincon, J., Zabala, L. L., Jiao, N., et al. (2013). Present and future global distributions of the marine Cyanobacteria Prochlorococcus and Synechococcus. Proc. Natl. Acad. Sci. U.S.A. 110, 9824–9829.
Fronzi, M., Soon, A., Dolley, B., Traversa, E., and Stampfl, C. (2009). Stability and morphology of cerium oxide surfaces in an oxidizing environment: a first-principles investigation. J. Chem. Phys. 131:104701. doi: 10.1063/1.3191784
Hallquist, A. M., Fridell, E., Westerlund, J., and Hallquist, M. (2013). Onboard measurements of nanoparticles from a SCR-equipped marine diesel engine. Environ. Sci. Technol. 47, 773–780. doi: 10.1021/es302712a
Handy, R. D., van den Brink, N., Chappell, M., Muhling, M., Behra, R., Dusinska, M., et al. (2012). Practical considerations for conducting ecotoxicity test methods with manufactured nanomaterials: what have we learnt so far? Ecotoxicology 21, 933–972. doi: 10.1007/s10646-012-0862-y
Hawthorne, J., De la Torre Roche, R., Xing, B., Newman, L. A., Ma, X., Majumdar, S., et al. (2014). Particle-size dependent accumulation and trophic transfer of cerium oxide through a terrestrial food chain. Environ. Sci. Technol. 48, 13102–13109. doi: 10.1021/es503792f
Hazeem, L. J., Bououdina, M., Rashdan, S., Brunet, L., Slomianny, C., and Boukherroub, R. (2016). Cumulative effect of zinc oxide and titanium oxide nanoparticles on growth and chlorophyll a content of Picochlorum sp. Environ. Sci. Pollut. Res. Int. 23, 2821–2830. doi: 10.1007/s11356-015-5493-4
Hu, J., Wang, J., Liu, S., Zhang, Z., Zhang, H., Cai, X., et al. (2018). Effect of TiO2 nanoparticle aggregation on marine microalgae Isochrysis galbana. J. Environ. Sci. (China) 66, 208–215. doi: 10.1016/j.jes.2017.05.026
Hu, Z. Y., Haneklaus, S., Sparovek, G., and Schnug, E. (2006). Rare earth elements in soils. Commun. Soil Sci. Plant Anal. 37, 1381–1420.
Jiaqiang, E., Zhang, Z., Chen, J., Pham, M., Zhao, X., Peng, Q., et al. (2018). Performance and emission evaluation of a marine diesel engine fueled by water biodiesel-diesel emulsion blends with a fuel additive of a cerium oxide nanoparticle. Energy Conv. Manag. 169, 194–205. doi: 10.1016/j.enconman.2018.05.073
Johnson, A. C., and Park, B. (2012). Predicting contamination by the fuel additive cerium oxide engineered nanoparticles within the United Kingdom and the associated risks. Environ. Toxicol. Chem. 31, 2582–2587. doi: 10.1002/etc.1983
Keller, A. A., Wang, H., Zhou, D., Lenihan, H. S., Cherr, G., Cardinale, B. J., et al. (2010). Stability and aggregation of metal oxide nanoparticles in natural aqueous matrices. Environ. Sci. Technol. 44, 1962–1967. doi: 10.1021/es902987d
Li, M., Jiang, Y., Chuang, C. Y., Zhou, J., Zhu, X., and Chen, D. (2019). Recovery of Alexandrium tamarense under chronic exposure of TiO2 nanoparticles and possible mechanisms. Aquat. Toxicol. 208, 98–108. doi: 10.1016/j.aquatox.2019.01.007
Limbach, L. K., Bereiter, R., Muller, E., Krebs, R., Galli, R., and Stark, W. J. (2008). Removal of oxide nanoparticles in a model wastewater treatment plant: influence of agglomeration and surfactants on clearing efficiency. Environ. Sci. Technol. 42, 5828–5833. doi: 10.1021/es800091f
Lowry, G. V., Gregory, K. B., Apte, S. C., and Lead, J. R. (2012). Transformations of nanomaterials in the environment. Environ. Sci. Technol. 46, 6893–6899. doi: 10.1021/es300839e
Lucas, A., Dupont, C., Tai, V., Largier, J., Palenik, B., and Franks, P. (2011). The green ribbon: multiscale physical control of phytoplankton productivity and community structure over a narrow continental shelf. Limnol. Oceanogr. 56, 611–626. doi: 10.4319/lo.2011.56.2.0611
Ma, L., Calfee, B. C., Morris, J. J., Johnson, Z. I., and Zinser, E. R. (2017). Degradation of hydrogen peroxide at the ocean’s surface: the influence of the microbial community on the realized thermal niche of Prochlorococcus. ISME J. 12, 473–484. doi: 10.1038/ismej.2017.182
Manzo, S., Buono, S., Rametta, G., Miglietta, M., Schiavo, S., and Di Francia, G. (2015). The diverse toxic effect of SiO(2) and TiO(2) nanoparticles toward the marine microalgae Dunaliella tertiolecta. Environ. Sci. Pollut. Res. Int. 22, 15941–15951. doi: 10.1007/s11356-015-4790-2
Mella-Flores, D., Mazard, S., Humily, F., Partensky, F., Mahe, F., Bariat, L., et al. (2011). Is the distribution of Prochlorococcus and Synechococcus ecotypes in the Mediterranean Sea affected by global warming? Biogeosciences 8, 2785–2804. doi: 10.5194/bg-8-2785-2011
Merrifield, R. C., Wang, Z. W., Palmer, R. E., and Lead, J. R. (2013). Synthesis and characterization of polyvinylpyrrolidone coated cerium oxide nanoparticles. Environ. Sci. Technol. 47, 12426–12433. doi: 10.1021/es402541z
Miao, A. J., Zhang, X. Y., Luo, Z., Chen, C. S., Chin, W. C., Santschi, P. H., et al. (2010). Zinc oxide-engineered nanoparticles: dissolution and toxicity to marine phytoplankton. Environ. Toxicol. Chem. 29, 2814–2822. doi: 10.1002/etc.340
Mitchell, S. L., Hudson-Smith, N. V., Cahill, M. S., Reynolds, B. N., Frand, S. D., Green, C. M., et al. (2019). Chronic exposure to complex metal oxide nanoparticles elicits rapid resistance in Shewanella oneidensis MR-1. Chem. Sci. 10, 9768–9781. doi: 10.1039/c9sc01942a
Moore, L. R., Post, A. F., Rocap, G., and Chisholm, S. W. (2002). Utilization of different nitrogen sources by the marine cyanobacteria Prochlorococcus and Synechococcus. Limnol. Oceanogr. 47, 989–996. doi: 10.4319/lo.2002.47.4.0989
Morelli, E., Gabellieri, E., Bonomini, A., Tognotti, D., Grassi, G., and Corsi, I. (2018). TiO2 nanoparticles in seawater: aggregation and interactions with the green alga Dunaliella tertiolecta. Ecotoxicol. Environ. Saf. 148, 184–193. doi: 10.1016/j.ecoenv.2017.10.024
Morris, J. J., Johnson, Z. I., Szul, M. J., Keller, M., and Zinser, E. R. (2011). Dependence of the cyanobacterium Prochlorococcus on hydrogen peroxide scavenging microbes for growth at the ocean’s surface. PLoS One 6:e16805. doi: 10.1371/journal.pone.0016805
Nadeem, M., Khan, R., Afridi, K., Nadhman, A., Ullah, S., Faisal, S., et al. (2020). Green synthesis of cerium oxide nanoparticles (CeO2 NPs) and their antimicrobial applications: a review. Int. J. Nanomed. 15, 5951–5961.
Neil, C. W., Wu, X., Kim, D., Jung, H., Zhu, Y., Ray, J. R., et al. (2021). Arsenite oxyanions affect CeO2 nanoparticle dissolution and colloidal stability. Environ. Sci. Nano 8, 233–244. doi: 10.1039/d0en00970a
Nyoka, M., Choonara, Y. E., Kumar, P., Kondiah, P. P. D., and Pillay, V. (2020). Synthesis of cerium oxide nanoparticles using various methods: implications for biomedical applications. Nanomaterials (Basel) 10:242. doi: 10.3390/nano10020242
Ottofuelling, S., Von der Kammer, F., and Hofmann, T. (2011). Commercial titanium dioxide nanoparticles in both natural and synthetic water: comprehensive multidimensional testing and prediction of aggregation behavior. Environ. Sci. Technol. 45, 10045–10052. doi: 10.1021/es2023225
Petosa, A. R., Jaisi, D. P., Quevedo, I. R., Elimelech, M., and Tufenkji, N. (2010). Aggregation and deposition of engineered nanomaterials in aquatic environments: role of physicochemical interactions. Environ. Sci. Technol. 44, 6532–6549. doi: 10.1021/es100598h
Pulido-Reyes, G., Rodea-Palomares, I., Das, S., Sakthivel, T. S., Leganes, F., Rosal, R., et al. (2015). Untangling the biological effects of cerium oxide nanoparticles: the role of surface valence states. Sci. Rep. 5:15613.
Quik, J. T., Lynch, I., Van Hoecke, K., Miermans, C. J., De Schamphelaere, K. A., Janssen, C. R., et al. (2010). Effect of natural organic matter on cerium dioxide nanoparticles settling in model fresh water. Chemosphere 81, 711–715. doi: 10.1016/j.chemosphere.2010.07.062
Quik, J. T., Velzeboer, I., Wouterse, M., Koelmans, A. A., and van de Meent, D. (2014). Heteroaggregation and sedimentation rates for nanomaterials in natural waters. Water Res. 48, 269–279. doi: 10.1016/j.watres.2013.09.036
Rodea-Palomares, I., Boltes, K., Fernández-Piñas, F., Leganés, F., García-Calvo, E., Santiago, J., et al. (2010). Physicochemical characterization and ecotoxicological assessment of CeO2 nanoparticles using two aquatic microorganisms. Toxicol. Sci. 119, 135–145. doi: 10.1093/toxsci/kfq311
Rogers, N. J., Franklin, N. M., Apte, S. C., Batley, G. E., Angel, B., Lead, J. R., et al. (2010). Physico-chemical behaviour and algal toxicity of nanoparticulate CeO2 in freshwater. Environ. Chem. 7, 50–60. doi: 10.1071/en09123
Roth-Rosenberg, D., Aharonovich, D., Luzzatto-Knaan, T., Vogts, A., Zoccarato, L., Eigemann, F., et al. (2020). Prochlorococcus rely on microbial interactions rather than on chlorotic resting stages to survive long-term nutrient starvation. bioRxiv [Preprint]. doi: 10.1128/mbio.01846-20
Scanlan, D. J., Ostrowski, M., Mazard, S., Dufresne, A., Garczarek, L., Hess, W. R., et al. (2009). Ecological genomics of marine picocyanobacteria. Microbiol. Mol. Biol. Rev. 73, 249–299. doi: 10.1128/mmbr.00035-08
Sendra, M., Blasco, J., and Araujo, C. V. M. (2018). Is the cell wall of marine phytoplankton a protective barrier or a T nanoparticle interaction site? Toxicological responses of Chlorella autotrophica and Dunaliella salina to Ag and CeO2 nanoparticles. Ecol. Indic. 95, 1053–1067. doi: 10.1016/j.ecolind.2017.08.050
Sendra, M., Yeste, P. M., Moreno-Garrido, I., Gatica, J. M., and Blasco, J. (2017). CeO2 NPs, toxic or protective to phytoplankton? Charge of nanoparticles and cell wall as factors which cause changes in cell complexity. Sci. Total Environ. 590-591, 304–315. doi: 10.1016/j.scitotenv.2017.03.007
Shah, V., Shah, S., Shah, H., Rispoli, F. J., McDonnell, K. T., Workeneh, S., et al. (2012). Antibacterial activity of polymer coated cerium oxide nanoparticles. PLoS One 7:e47827. doi: 10.1371/journal.pone.0047827
Singh, K. R. B., Nayak, V., Sarkar, T., and Singh, R. P. (2020). Cerium oxide nanoparticles: properties, biosynthesis and biomedical application. RSC Adv. 10:27194. doi: 10.1039/d0ra04736h
Somasundaram, D., Elango, A., and Karthikeyan, S. (2017). Combustion and emission analysis of fishing – boat diesel engine running on diesel-ethanol-biodiesel-ceria-alumina nano blends. Indian J. Geo Mar. Sci. 46, 1704–1709.
Somasundaram, D., Elango, A., and Karthikeyan, S. (2020). Estimation of carbon credits of fishing boat diesel engine running ondiesel-ethanol-bio-diesel blends with nano alumina doped ceria-zirconia. Mater. Today Proc. 33, 2923–2928. doi: 10.1016/j.matpr.2020.02.887
Taylor, N. S., Merrifield, R., Williams, T. D., Chipman, J. K., Lead, J. R., and Viant, M. R. (2016). Molecular toxicity of cerium oxide nanoparticles to the freshwater alga Chlamydomonas reinhardtii is associated with supra-environmental exposure concentrations. Nanotoxicology 10, 32–41.
Thiagarajan, V., Pavani, M., Archanaa, S., Seenivasan, R., Chandrasekaran, N., Suraishkumar, G. K., et al. (2019). Diminishing bioavailability and toxicity of P25 TiO2 NPs during continuous exposure to marine algae Chlorella sp. Chemosphere 233, 363–372. doi: 10.1016/j.chemosphere.2019.05.270
Van Koetsem, F., Verstraete, S., Van der Meeren, P., and Du Laing, G. (2015). Stability of engineered nanomaterials in complex aqueous matrices: settling behaviour of CeO2 nanoparticles in natural surface waters. Environ. Res. 142, 207–214. doi: 10.1016/j.envres.2015.06.028
Velzeboer, I., Quik, J. T., van de Meent, D., and Koelmans, A. A. (2014). Rapid settling of nanoparticles due to heteroaggregation with suspended sediment. Environ. Toxicol. Chem. 33, 1766–1773. doi: 10.1002/etc.2611
Wang, Y., Zhu, X., Lao, Y., Lv, X., Tao, Y., Huang, B., et al. (2016). TiO2 nanoparticles in the marine environment: physical effects responsible for the toxicity on algae Phaeodactylum tricornutum. Sci. Total Environ. 565, 818–826. doi: 10.1016/j.scitotenv.2016.03.164
Wong, S. W., Leung, P. T., Djurisic, A. B., and Leung, K. M. (2010). Toxicities of nano zinc oxide to five marine organisms: influences of aggregate size and ion solubility. Anal. Bioanal. Chem. 396, 609–618. doi: 10.1007/s00216-009-3249-z
Xia, B., Chen, B., Sun, X., Qu, K., Ma, F., and Du, M. (2015). Interaction of TiO2 nanoparticles with the marine microalga Nitzschia closterium: growth inhibition, oxidative stress and internalization. Sci. Total Environ. 508, 525–533. doi: 10.1016/j.scitotenv.2014.11.066
Xia, T., Kovochich, M., Liong, M., Madler, L., Gilbert, B., Shi, H., et al. (2008). Comparison of the mechanism of toxicity of zinc oxide and cerium oxide nanoparticles based on dissolution and oxidative stress properties. ACS Nano 2, 2121–2134. doi: 10.1021/nn800511k
Keywords: Prochlorococcus, ecotoxicology, nanomaterials, cerium oxide, marine pollution, phytoplankton
Citation: Dedman CJ, Rizk MMI, Christie-Oleza JA and Davies G-L (2021) Investigating the Impact of Cerium Oxide Nanoparticles Upon the Ecologically Significant Marine Cyanobacterium Prochlorococcus. Front. Mar. Sci. 8:668097. doi: 10.3389/fmars.2021.668097
Received: 15 February 2021; Accepted: 26 April 2021;
Published: 19 May 2021.
Edited by:
Anastasia Tsiola, Hellenic Centre for Marine Research (HCMR), GreeceReviewed by:
Roselyne Ferrari, Université de Paris, FranceRavindra Pratap Singh, Indira Gandhi National Tribal University, India
Giacomo Grassi, Université Paris-Saclay, France
Copyright © 2021 Dedman, Rizk, Christie-Oleza and Davies. This is an open-access article distributed under the terms of the Creative Commons Attribution License (CC BY). The use, distribution or reproduction in other forums is permitted, provided the original author(s) and the copyright owner(s) are credited and that the original publication in this journal is cited, in accordance with accepted academic practice. No use, distribution or reproduction is permitted which does not comply with these terms.
*Correspondence: Craig J. Dedman, Yy5kZWRtYW5Ad2Fyd2ljay5hYy51aw==; Joseph A. Christie-Oleza, am9zZXBoLmNocmlzdGllQHVpYi5ldQ==; Gemma-Louise Davies, Z2VtbWEtbG91aXNlLmRhdmllc0B1Y2wuYWMudWs=