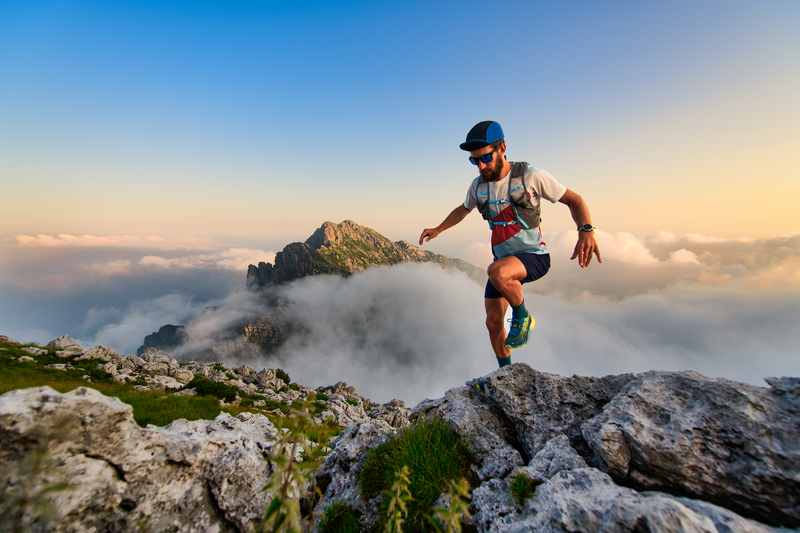
94% of researchers rate our articles as excellent or good
Learn more about the work of our research integrity team to safeguard the quality of each article we publish.
Find out more
REVIEW article
Front. Mar. Sci. , 21 April 2021
Sec. Global Change and the Future Ocean
Volume 8 - 2021 | https://doi.org/10.3389/fmars.2021.666662
This article is part of the Research Topic ICYMARE - Early Career Researchers in Marine Science View all 32 articles
Aquaculture is one of the fastest growing food production sectors and has great potential for food security and livelihoods. However, it generates concerning consequences for the environment, including chemical and biological pollution, disease outbreaks, unsustainable feeds and competition for coastal space. Recent investigations are focusing on sustainable techniques (e.g., polyculture, offshore facilities) to improve the relationship between the industry, environment and society. This review provides an overview of the main factors of ecological concern within marine finfish aquaculture, their interactions with the environment, and highlights sustainable alternatives that are currently in use or development. Adequate environmental monitoring and location of farms, the reduction and exploitation of wastes and chemicals being used is crucial to ensure the growth and continuity of aquaculture production.
Aquaculture can be traced back as far as 4000 years in Egypt (Chimits, 1957) and more than 2000 years in China (Beveridge and Little, 2002; Edwards, 2004; Lu and Li, 2006) and Europe (Beveridge and Little, 2002; Buschmann and Muñoz, 2019). Aquaculture was further developed because the capacities of traditional aquatic ecosystems could not support the human population growth (Costa-Pierce, 2002), thus, it became a social necessity. The rapid development of the aquaculture sector by producing great amounts of diverse fish products for human consumption has been called the “Blue Revolution” (Costa-Pierce, 2002) which started during the 1960s in Asia (Thia-Eng, 1997) and in the West in the late 1970s and early 1980s (MacKay, 1983). Since then, the aquaculture sector is continuing to significantly develop towards new diversification and intensification (Ahmed and Thompson, 2018). The economic demand for fish protein is increasing, however, capture fisheries production remains static or is diminishing (depending on the species in question) during the last decades (FAO, 2018). Currently, aquaculture produces more than 30% of the total fish consumed worldwide (FAO, 2018).
In recent years, society has become increasingly concerned about the effects that anthropogenic activities have on the environment. The assessment of impacts from aquaculture farm activities has not been examined extensively enough to cope with the growth of this industry (Bostock et al., 2009; Bohnes and Laurent, 2021). These assessments are mostly based on physical-chemical measures and/or sediment characteristics with comparably limited focus on environmental carrying capacity studies, required for aquaculture sustainability. Environmental characteristics of the receiving environment define the Environmental Carrying Capacity (ECC) of the selected site, which will determine the discharge load (i.e., dissolved and particulate organic matter, chemicals) that might be assimilated by the affected ecosystem. These environmental characteristics include bathymetry conditions, physical-chemical characteristics of water and substrate, trophic status, and colonizing capacity (fouling) (Garmendia et al., 2012).
Since its appearance on the international agenda, the concept of sustainability has been the topic of much debate, both in terms of how to achieve it as well as how to define it. A popular definition is the one coined by the 1992 United Nations Conference on Environment and Development report Our common future in the context of sustainable development: “meeting the needs of the present without compromising the ability of future generations to meet their own needs” (Brundtland, 1987). However, not all ecosystems can be conserved unaltered (Brundtland, 1987). Principles of sustainability include three dimensions: (1) the economy (ability to be maintained without an outside influx of money; Copus and Crabtree, 1996), (2) the society [equity and cultural capital that can be passed to succeeding generations (Copus and Crabtree, 1996)], and (3) the environment [maintenance of an ecosystem’s characteristic diversity, productivity and biogeochemical cycling (Amundsen and Osmundsen, 2018)]. In this chapter, we focus on environmental aspects of sustainability.
Environmental aspects of sustainability in aquaculture may not be achieved until several problems are solved: eutrophication of receiving ecosystems, destruction of natural habitats, dependence on fishmeal and fish oil, introduction of exotic species and inadequate medication practices (Martinez-Porchas and Martinez-Cordova, 2012; Figure 1).
Figure 1. The main pollution sources from marine finfish aquaculture and their related effects on the environment.
Worldwide, there are many developed co-operational projects that are or have been researching for more sustainable methods (Table 1). The main topics studied address general sustainable intensification of aquaculture, educational purposes, animal welfare, alternative feeds, land-based and aquaponics systems. According to Life Cycle Assessment studies (a tool to assess the environmental impacts to get a product, including processing, transport, use and disposal), fish feed and burning of fossil fuels (required for mobility and electricity) are the main environmental impacts from net cage aquaculture (Samuel-Fitwi et al., 2012; Ramos et al., 2019). From a feed efficiency point of view, aquatic livestock use less energy than terrestrial livestock to grow and, therefore, produce less greenhouse gasses (Ayer and Tyedmers, 2009; Pelletier et al., 2009). For example, eutrophication is not considered due to the inexistence of specific impact methodologies for the marine environment (Samuel-Fitwi et al., 2012; Woods et al., 2016; Winter et al., 2017; Ramos et al., 2019). Nevertheless, more research is needed to measure life cycles covering all environmental aspects (Samuel-Fitwi et al., 2012; Woods et al., 2016; Winter et al., 2017).
Table 1. Summary about co-operational projects (ongoing or finished) concerning sustainable methods in aquaculture.
Optimal environmental monitoring plans help to understand the impacts of waste management, their utilization and possibilities of how it can be reduced. The exploitation of farming wastes implies a double benefit; less environmental contamination and higher economic profits. Thus, polycultures and technological advances should help the aquaculture industry and are necessary towards aquaculture sustainability.
This review focuses on marine finfish aquaculture. The majority of world aquaculture occurs inland, producing nearly twice as much as coastal and marine aquaculture (Li et al., 2018). However, these are mostly small operations while multinational companies are mainly focused on marine farming. Those companies highly contribute to aquaculture research (including minimizing economic losses from uneaten feed or ineffective chemical treatment), define best management practices, develop more detailed monitoring studies and help governments in defining environmental regulations. Marine aquaculture, also known as mariculture, includes those cultures located in the sea while coastal aquaculture implies human-made structures in areas close to the sea (e.g., coastal ponds and gated lagoons). Most of the finfish aquaculture from the Americas, Europe and Oceania is produced through mariculture (FAO, 2018). Most African production belongs to inland aquaculture because infrastructure, technical expertise and investment are needed to promote marine culturing (FAO, 2020). In terms of live weight, molluscs represent the most biomass from mariculture. Nevertheless, when edible animal-source food is considered, a conversion factor of 6 and 1.15 is applied to the commonly reported weight from molluscs and finfish, respectively. Therefore, finfish are the main contributor from whole aquatic animal production to human nutrition (FAO, 2018; Edwards et al., 2019).
Mariculture concerning finfish production is dominated by salmonid aquaculture, especially Atlantic salmon (Salmo salar), which is also the most cultured carnivorous fish worldwide (Føre et al., 2018; Filgueira et al., 2019; Figure 2).
Figure 2. Main finfish species produced in marine aquaculture globally, salmonid species on the right (percentages extracted from FAO, 2018).
From an ecological point of view, intensive fish farming represents the highest environmental risk when compared to other aquaculture sectors due to the feeding needs and the chemicals used associated with the production process (Tornero and Hanke, 2016). This chapter will further describe the main effects that the marine finfish aquaculture creates for the environment.
As the aquaculture sector is developing and expanding, it has an increasing effect on the surrounding environment. These effects include nutrient pollution from uneaten feed and metabolic waste, chemical pollution from various substances used in the production process (such as medical treatments, including antibiotics and antiparasitics) as well as the spread of farmed fish genes, parasites, and diseases to wild populations (Sarà, 2007; Burridge et al., 2010; Little et al., 2016; Weitzman, 2019). An important indirect effect of the production process is the high impact on aquatic ecosystems through the need to use wild fish to feed carnivorous species of farmed fish (Little et al., 2016). However, it must be noted that there are also other direct effects, such as landscape visual impact, noise, odor, and marine litter from intensively farmed areas (Figure 3; Martinez-Porchas and Martinez-Cordova, 2012; Radford and Slater, 2019). Untreated wastewater from fish farms includes elevated nutrient levels, but also chemicals used to prevent diseases. In the case of open net cages, the ability to prevent these contaminants from entering the surrounding water is very limited. The focus of this chapter is on the main sources of contamination in marine finfish aquaculture (as highlighted, e.g., by Duarte et al., 2009).
Figure 3. Intensive marine land-based fish farm discharging effluents directly into the sea (NW Spain). Authorship: Carlos Carballeira.
During the production process, uneaten feed and the metabolic waste of fish release nitrogen and phosphorus into the water. This process causes problems in intensive farming areas where food sources from a broader region are concentrated in a smaller area (Dempster and Sanchez-Jerez, 2008). Waste originated from aquaculture farms may create a significant source of excess nutrients within the coastal areas. These excess nutrients are mainly related to the proliferation of primary producers that may trigger micro- and macroalgal blooms that may be toxic (Wang et al., 2018). The causes of emergence of toxic algae are still unknown and seem to be more related with natural or anthropogenic nutrient and silica fluxes from terrestrial sources (Masó and Garcés, 2006; Kumar et al., 2018), but nutrients are required for algal blooms and they may originate at pisciculture facilities. Up to 50% of supplied feed can be lost to the surrounding water and sediment, posing not only an economic, but also an environmental threat (Ballester-Moltó et al., 2017b). Particulate waste from aquaculture farms, such as feeds, feces, and other organic material, traps harmful lipophilic compounds, increasing their incorporation into the trophic chain, elevates water turbidity and, thus, decreases photic depth, and is finally deposited close to fish farms, modifying chemical and biochemical properties of the sediment (Price et al., 2015; Brager et al., 2016; Ballester-Moltó et al., 2017a).
Nitrogen species make up the largest volume of aquaculture contaminants and provide a source of nutrients for primary producers. When discharged into the surrounding environment, they affect the trophic balance and can lead to eutrophication at low hydrodynamic sites, where less mixing occurs (Price et al., 2015; Urbina, 2016; Herrera et al., 2018). Ammonia is excreted directly by the fish and produced through decomposition of uneaten food. It is toxic to animals, especially at high pH levels, and when levels of unionized ammonia are also high, this reduces fertility and increases susceptibility to diseases (Ip and Chew, 2010). Ammonia is rapidly taken up by primary producers because of the higher preference for reduced forms of nitrogen or oxidized by Nitrobacteraceae bacteria into nitrite, which is 50 to 100 times more toxic to freshwater animals than those from seawater (Kroupova et al., 2005). Nitrification continues when nitrite is oxidized into nitrate, which is less toxic than its previous forms. At low levels of oxygen as found in sediment, nitrate is reduced into dinitrogen gas, then sulfate-reducing bacteria transfer electrons to sulfur forms (sulfate, thiosulfate, and elemental sulfur) releasing toxic sulfides (Wang and Chapman, 1999).
Nitrogen and phosphorous support and limit the growth of aquatic primary producers because they are needed for the synthesis of proteins, DNA, RNA, and the energy transfer, respectively (Conley et al., 2009). The vast majority, about 80%, of phosphorus contained in fish feed has been found to be lost to surrounding waters, with a large portion ending up in the sediment (Holby and Hall, 1991). Phosphorous introduced from aquaculture pollution can be more mobilizable (more easily released to the overlying water and posteriorly taken up by primary producers) than what is usually found in the environment, hindering recovery and increasing the potential to cause eutrophication (Jia et al., 2015).
Numerous chemicals are being used in aquaculture production (Table 2) to prevent and treat disease outbreaks, ranging from medicines such as antiparasitics and antibiotics to disinfectants (Burridge et al., 2010; Martinez-Porchas and Martinez-Cordova, 2012; Ozbay et al., 2014; Rico et al., 2018). Antifouling chemicals are also being used at aquaculture facilities to avoid the clogging of meshes.
Table 2. Main chemical pollution sources in marine finfish aquaculture and their related effects on the environment.
Chemicals used in aquaculture enter surrounding marine environments and may be toxic to non-target organisms in proximity of the farming sites because they are not highly selective, e.g., benzoylurea pesticides affect naupliar development of copepod Tisbe battagliai (Macken et al., 2015). Moreover, the effectiveness of most chemical treatments is low within high fish densities because of increased host availability. This is especially the case in open cage systems where chemical inputs are directly released to the marine environment.
Antibiotics are used in aquaculture to control the spread of pathogenic bacteria (Burridge et al., 2010; Martinez-Porchas and Martinez-Cordova, 2012; Rico et al., 2018). This raises concerns about the possible effects on biological diversity and of selection of antibiotic resistant bacteria when antibiotics are used excessively over time, e.g., Erythromycin and bacterial kidney disease (Burridge et al., 2010). Moreover, the use of a single pharmaceutical can cause insignificant consequences while it may have a highly adverse effect when acting in combination with other chemical substances (Fent et al., 2006). It is estimated that around 70 - 80% of antibiotics given as medicated feed ends up in the marine environment around the farming sites (Ferreira et al., 2007). Another environmental risk is associated with parasiticides, which contaminate the water column and seabed either as uneaten medicated feed, fecal material or in soluble form in urine (Tornero and Hanke, 2016). Additionally, parasiticides that are being used in aquaculture to control ectoparasite infestations can lack specificity to target organisms thereby posing a threat to non-target species including small crustaceans in the proximity of the farm site (Burridge et al., 2010).
Due to increasing resistance, the approach in some cases is to use even larger concentrations of chemical treatments. Use of antibiotics is subjected to reporting but quantities are not always publicly accessible (e.g., Chile) (Burridge et al., 2010). However, there are examples of diseases that classically have been considered as occurring only in freshwater but today cause problems in marine aquaculture, e.g., furunculosis (Aeromonas salmonicida), bacterial kidney disease (Renibacterium salmoninarum) and some types of streptococcosis in such species as salmonids and turbot (Toranzo et al., 2005).
Antifouling products are used in aquaculture on submerged structures (cage nets and supporting infrastructure) to avoid biofouling by various epibiotic organisms, such as marine algae, barnacles, bivalves, bryozoans and others. Marine finfish aquaculture biofouling can result in compromised cage structures and have negative effects on farmed fish lowering water flow through cages, leading to poor oxygen availability or increased risk of disease (Fitridge et al., 2012). Antifouling products are associated with increased copper levels, which are toxic to several marine invertebrates, especially molluscs (Fitridge et al., 2012). In addition, the immune defense of exposed organisms around farming sites is affected by antifouling and copper-based products (Fitridge et al., 2012; Tornero and Hanke, 2016).
There is little information available regarding disinfectant quantities and particular substances, and not so much research has been performed on the effect of disinfectants, which are used on land-based facilities, nets, platforms, boats, ponds, and other surfaces (Buschmann et al., 2006).
Anesthetics are being used in marine aquaculture to reduce stress during handling and to improve the efficiency of procedures (Sneddon, 2012). However, the use of those chemicals does not pose significant risks to the environment due to infrequent and limited use and practically no discharge into the environment when good management practices are followed (Burridge et al., 2010).
Outbreaks of fish diseases are a result of the interaction between the pathogen, the host, and the environment. Several drivers may cause a disease outbreak: high fish density, compressed rearing cycle and a limited genetic diversity (Kennedy et al., 2016). Considering the high stocking densities within aquaculture farms, bacterial, viral, and fungal diseases as well as the spread of parasites is taking place at an increasing rate. For example, in marine aquaculture such spread of diseases occurred during Infectious Salmon Anemia outbreaks (Lyngstad et al., 2018). Another recently growing problem is dealing with parasites (e.g., salmon lice Leopephtheirus salmonis) (Overton et al., 2018). While the culture period is shortened to minimize the production costs, this may lead to higher pathogen virulence due to evolution (Kennedy et al., 2016). In addition, the limited genetic diversity due to selective breeding may lead to the development of a pathogen specialization towards a particular group of hosts (Kennedy et al., 2016).
Another issue concerning aquaculture-based negative effects on the environment is the risk of biological pollution. Biological pollution can be caused by the farming of exotic species, which also act as vectors for new parasites and diseases, and of native but cultured individuals with a reduced genetic diversity that may also pose a threat to wild populations. After shipping, aquaculture is the second largest sector causing the introduction of exotic species worldwide and likely to increase because of the spread of farms into more pristine areas (Hewitt and Campbell, 2007). Although some escaped fish have shown homing behavior (remaining in the vicinity of the cages), other studies found escaped fish dozens of kilometers away from the place of cultivation (Toledo-Guedes et al., 2014; Dempster et al., 2016). Recapture methods have been only partially effective, therefore, efforts should be focused on preventive measures.
Biological pollution can take place as the accidental release of fish during operation, damage to cages (e.g., caused by harsh weather conditions), or attacks by wild predators. It may also occur during spawning when farmed fish are kept in open cages to a size in which they can become sexually mature (e.g., Atlantic cod (Gadus morhua)), allowing drifting of fertilized eggs into the surrounding environment (Jensen et al., 2010). Although accidental escapes (especially large scale) do not occur often, the number of individuals escaping can be large when compared to the abundance of their wild conspecifics as is the case with wild Atlantic salmon (S. salar) (Thorstad et al., 2008). Negative effects to the wild populations are related to ecological interactions, such as possible genetic impacts in case of inter-breeding and competition for both food and habitat (Izquierdo-Gómez et al., 2017). Around 80-83% of the European aquaculture production originates from selective breeding to improve growth performance (Janssen et al., 2017). In the wild, farmed fish may transmit these genetic characteristics, which may result in reduced life span, increased susceptibility to diseases and lower individual fitness leading to a lower survival rate of future wild populations (Glover et al., 2017; Faust et al., 2018).
Fish farms are considered as fish aggregation devices (FAD) due to the significant increase of surrounding populations after installation of facilities because their wastewater is a source of food and their structure acts as artificial reefs (Dempster et al., 2006; Grigorakis and Rigos, 2011; Sanchez-Jerez et al., 2011; Aguado-Giménez et al., 2016; Riera et al., 2017; Figure 4). Cage aquaculture is colonized similarly to artificial and rocky reefs but unique fish assemblages have been observed around deployed aquaculture facilities. The combination of both floating and bottom artificial structures (e.g., Integrated multi-trophic aquaculture, IMTA) enhances the population of local species and increases the complexity of fish assemblages (Wang Y. et al., 2015). However, artificial reefs for sea cucumber cultivation may trigger the settlement of polyps whose jellyfish blooms may generate severe gill problems on farmed fish (e.g., salmon) (Purcell et al., 2007). Furthermore, microbial densities are increasing, as a result of the medusae preying on the predators of microbes like small copepods and ciliates (Turk et al., 2008). Wild organisms may improve the quality of sediment and water by reducing the organic load released by farms (Katz et al., 2002; Vita et al., 2004). However, fishing in the vicinity of farms must be restricted because of public health concerns (disease and antibiotic transmission) and ecological reasons. Fishing at FADs will reduce local populations and subsequently adjacent populations that are attracted by the empty ecological niches and the food supply (Riera et al., 2017). Other wild populations are attracted by the increased biomass and may damage farming facilities (e.g., sea lions, dolphins, cormorants, and bluefish) easing biological pollution (Aguado-Giménez et al., 2016).
Figure 4. Opportunistic species from turbot land-based farms: (a) mullets [Nerga, Cangas do morrazo (Spain)], (b) birds [Quilmas, Carnota (Spain)], (c) green algae [Grove, Salnés (Spain)]. (a,d) Recreational fishermen are usually found in the dumping area, attracted by the high concentration of fish [d – Aguiño, Barbanza (Spain)]. Authorship: Carlos Carballeira.
The control of the main marine aquaculture production by multinational companies helps the development of environmental regulations and technological breakthroughs, improves feeding techniques by reducing feed losses, and decreases the usage of antibiotics by the increasing utilization of vaccines (Føre et al., 2018). However, these regulations are mainly based on the economically dominant type of fish farming; net cages on protected coasts. This is why Environmental Monitoring Plans (EMPs) are usually based on the assessment of water and sediment changes. Meanwhile, the location of farms at high dispersive sites (high flow velocities and great depths) may disable this type of monitoring (Sutherland et al., 2018). Changes on physicochemical properties of the water column are most times undetectable (Sarà, 2007) even between input and output water from land-based farms. Sediment is a matrix that integrates and concentrates contaminants from the water column and facilitates studies of ecological integrity of benthic communities (Caeiro et al., 2012) but this may not be present at hard bottom coastal areas and offshore sites because organic waste is highly dispersed (Carballeira et al., 2012). The following sections describe the current type of assessment, monitoring plans and methods, and how to improve and adapt it to different types of facilities.
Nutrient release may affect water quality (e.g., water turbidity, dissolved oxygen), increase trophic resources, and modify the geochemical properties of sediment (Qi et al., 2019). Nevertheless, most times the effect of a fish farm on the water column is negligible due to the high dilution and recycling of nutrients at sites where farms are established (Soto and Norambuena, 2004). For this reason, environmental impact assessments are mainly based on the study of sediment, but this is not possible in hard-bottom sites. To reach sustainable alternatives, effective assessment methods must be used without being sediment dependent. Traditional water monitoring measures physicochemical parameters mainly related with organic contamination (dissolved oxygen, nitrogen forms, phosphorus, salinity, turbidity, pH, chlorophyll, temperature, sulfides, and redox potential). When a parameter represents a certain pollution threshold, the water sample is sent to a laboratory for further analysis. Every step is performed manually, which is time- and cost-consuming, and contamination episodes might be missed (Li and Liu, 2018). New sensors that utilize fiber optics, laser technology, biosensors, optical sensors, and microelectronic mechanical systems to detect different water quality parameters in situ and in real-time are being developed (Simbeye et al., 2014; Li and Liu, 2018). Nevertheless, these methods are either under development or expensive.
Chemical treatments used in aquaculture depend on several factors - disease, the location of the facility, system parameters, treatment type, and legislation. There are specific regulations concerning the use and quantities of specific substances in aquaculture but this highly differs between countries (Burridge et al., 2010). These include mandatory risk evaluation and authorization processes before a particular substance can be used. There are also various modeling tools, based on the assessment of dilution and dispersion of both chemical treatments and particles (from medicated feed), that calculate chemical exposure and ecotoxicological risks close to cages (Rico et al., 2018). Even though there are only few monitoring and control requirements regarding accumulation of chemicals used, their components and derivatives (FAO, 2009).
Authorities are setting regulations concerning allowed substances but also routes of delivery, dosage by fish species and other limitations (Dawood et al., 2018). Fish do not metabolize antibiotics efficiently; releasing a large part of the substance to the marine environment that should be posteriorly monitored or reused within polycultures (Burridge et al., 2010).
Overall, the use of antimicrobials in aquaculture has declined because of improved rearing practices and hygienic conditions, as well as the development of vaccines. Also, the use of antibiotics as growth promoters is not practiced in aquaculture (Burridge et al., 2010; Done et al., 2015; Watts et al., 2017; Okocha et al., 2018). Producers in the largest farming countries are required to report particular diseases and the chemicals prescribed to avoid their outbreaks and minimize chemical treatments, e.g., allowable number of salmon lice (L. salmonis) per fish in Atlantic salmon (S. salar) farming which is monitored regularly during the production process (Hjeltnes et al., 2018).
Fish from open net cages may escape and enter directly into the surrounding environment. The ability to assess the escape rate depends on factors determined by species behavior, including the time escaped fish are spending close to the aquaculture facilities after the escape and their mortality rates. These behaviors are important for calculating the efficiency of potential recapture methods (Izquierdo-Gómez and Sanchez-Jerez, 2016; Dempster et al., 2016; Arechavala-Lopez et al., 2017). Recapture is said to be mostly ineffective (around 50% of escaped fish) although required in many jurisdictions (Dempster et al., 2016). According to that, a contingency plan consisting of notification of the escape to responsible authorities, recapture actions and a final report must be carried out (Arechavala-Lopez et al., 2018).
Recapture methods are similar to those that have been used in wild fisheries and include the identification of farmed fish (Dempster et al., 2016). Farmed fish can be tagged and thereby identified in case of escape using acoustic telemetry or mark and recapture techniques (Arechavala-Lopez et al., 2017). Methods to detect escaped fish are based on genetic differences (Karlsson et al., 2011) and external characteristics, as a consequence of high crop densities and handling (fin erosion, opercular deformities and body lesions) (Fiske et al., 2005). A simple and less expensive method includes the identification of scales based on the fact that fish in aquaculture lose some scales when handling or during feeding. These lost scales are then regenerated, and this can be detectable on the farmed fish (Izquierdo-Gómez et al., 2017).
Triploid organisms are being used in commercial farming (e.g., oysters and trout) but triploidy attempts with some species have been unsuccessful with an increase in deformities, e.g., Atlantic salmon (S. salar) (Lovatelli et al., 2013). Triploidy might be a potential solution to prevent interbreeding but it will not solve the problem of potential disease transmission or competition for food and space. The location of the farm (to avoid harsh weather conditions) is important as a preventive measure for escapes. The same is said about the provision of appropriate technical standards for the operation of facilities (e.g., appropriate mooring and netting of the cages) and development of farming routines (Arechavala-Lopez et al., 2017).
The effects of marine aquaculture on the surrounding environments (especially in open production systems) may be limited to a minimum. To establish these limits, monitoring data are needed at different levels of organization, so that, ecological changes can be detected and Ecological Quality Standards can be defined and included within an Environmental Impact Assessment (EIA) (Telfer and Beveridge, 2001; Holmer et al., 2008). Monitoring techniques must be effective, scientifically rigorous, cost-effective, dynamic, and regionally/site adapted to facilitate their usage and avoid unwanted damage to the ecosystem. Research is still needed to improve the monitoring programs, in particular those related to eutrophication at different scales and the ecosystem approach at larger scales (Holmer et al., 2008). Only few countries apply the ECC approach (e.g., Norway) and regulations from the majority are aimed at favoring farmers’ production (e.g., feed efficiency and absence of anoxic sediments) and regulate food standards (FAO, 2009; Weitzman and Filgueira, 2019). Determination of ECC of an aquatic ecosystem is a complex process generally based on mathematical models, making difficult to assess and implement standards (Silva et al., 2012; Bueno et al., 2017).
Environmental monitoring programs for marine open land-based aquaculture effluents are generally based on maximum percentages of increase or decrease of several physicochemical parameters between input and output water. These parameters are related to the eutrophication risk by measuring nutrient content (ammonia, nitrites, nitrates, and phosphorus) and related parameters such as turbidity, dissolved oxygen, chlorophyll, and pH (Carballeira et al., 2012).
One of the most critical problems from marine land-based aquaculture monitoring is the absence of sensitive methods able to determine the amount and toxic effects of pollutants in highly diluted seawater discharges. This is because there are no industries with similar discharges; moreover, intensive aquaculture is relatively modern and was previously considered as a non-polluting activity (Carballeira et al., 2018). This is not the case with net cages and soft bottom samples, because there is usually sediment underneath and its integrative capacity concentrates contaminants from the water column and allows its determination (Caeiro et al., 2012).
Net cages involve higher: (i) losses of nutrients (feed and biological waste) and chemicals; (ii) emergence and transmission of diseases; (iii) risk of escapees and attraction of predators; and (iv) greater problems in the shared use of waters (Price and Morris, 2013). The environmental requirements of cage production are very similar worldwide; they are based on the same parameters of study, by focusing the study on the ecosystemic effects in the benthic macrofauna of the sediment and the appearance of bacterial mats (Holmer et al., 2008; Wilson et al., 2009). Moreover, implications of EIA’s and routine monitoring involve high costs and time because there is a lack of harmonization between federal and regional roles in regulating aquaculture activities, and appropriately qualified scientific personnel (particularly in the area of benthic ecology and this is regarded as a major issue for the EIA).
Norway, being the largest salmon producer in the world, is developing the aquaculture practice that is based on the sustainable development (one that does not exceed the carrying capacity of the ecosystem) (Hersoug, 2015; Michaelsen-Svendsen, 2019). Even if each particular farming site meets the requirements independently, overall, they may exceed the load capacity in the area in question and cause environmental problems. Therefore, the presence of salmon lice (L. salmonis) in salmon farming is considered as an important factor relating to the ECC. Overall, close cooperation between the Norwegian government and producers allows better enforcement of sustainable measures, but this approach does regulate production carrying capacity instead of ECC (Chapman and Byron, 2018). The first may not guarantee long-term sustainability. Thus ECC should be considered.
Unfortunately, EMPs do not usually consider ECC measures and there are problems associated with current monitoring methods regarding sampling, selection of indicator species, use of biotic indices and the absence of sediment (hard-bottom sites). Most of the time methods lack standardization and effectiveness at different aquaculture scenarios.
Before and After Control Impact (BACI) is a common design proposed to evaluate anthropogenic perturbations on ecological variables. Using powerful statistics, different approaches allow adjusting to the particular real conditions (Underwood, 1993). However, the BACI method depends on the preoperational study (which is only done once just before starting the activity) and requires choosing control sites arbitrarily and assumes the control and impact sites to be similar before the impact. When a contaminant disperses with distance from a point source it is suggested that a gradient design will be more sensitive to change than BACI sampling designs (Rubio-Portillo et al., 2019). Gradient designs avoid the problem of arbitrarily selecting a control site, enable chemical, physical, and biological changes to be assessed as a function of distance, and results are easier to interpret and to use in public policy decisions (Ellis and Schneider, 1997).
Geochemical changes of the sediment due to organic enrichment alter the composition and structure of the infauna, favoring the dominance of the tolerant-generalist versus the sensitive-specialized species (Resende et al., 2010; Martinez-Garcia et al., 2013). The effects of the farms on the benthic communities are widely studied, especially on the communities of macro and meio invertebrates from the infauna, and occasionally on the benthic microbial communities (Verhoeven et al., 2016; Weitzman, 2019). It is usual to determine the degradation of the sediment by using the formation and coverage of Beggiatoa spp., a chemotrophic filamentous bacterium common in sulfur-rich environments (Verhoeven et al., 2016; Weitzman, 2019).
Benthic communities (e.g., bacterial mats, polychaetes, amphipods) are the most studied group as they are those that manifest the greatest changes when environmental conditions are altered (Buschmann et al., 2006; Borja et al., 2009; Keeley et al., 2012; Martinez-Porchas and Martinez-Cordova, 2012; Ozbay et al., 2014; Martinez-Garcia et al., 2013; Weitzman, 2019; Verhoeven et al., 2016). It is very common to use them as bioindicators of the state of the benthic ecosystem (both indicator species and communities), such as the increase of opportunistic species (e.g., Capitella capitata or Malacoceros fuliginosus) (Maldonado et al., 2005) or the reduction of sensitive species (e.g., Pennatula phosphorea) (Wilding, 2011) up to macroscopic parameters (e.g., ABC Curves, Shannon-Wiener Index, ITI, AMBI, OSI, BHQ) of the invertebrate community (Borja et al., 2009; Cromey et al., 2012; Keeley et al., 2012).
However, there are no standardized protocols for the selection of indicator species (Carballeira et al., 2019), and biotic indices need to be regionally adapted and may show higher biodiversity at transitional zones of disturbances than reference sites because of low-medium concentrations of organic matter (Galand et al., 2016). Thus, it might be hindering the interpretation of results.
Moreover, sampling and the studies related to benthic fauna are very onerous, and very little has been done regarding the combined effects of pollutants and the ecological preferences of marine indicator species, impeding the development of sensitive biomonitoring tools (Belando et al., 2017). The application of frequency analysis techniques, the calculation of specific renewal rates along environmental gradients and multiple regression techniques allow to select the explanatory variables and define the physicochemical thresholds that best characterize the significant changes in the ecological status of the system represented by the community of polychaetes (Martinez-Garcia et al., 2013).
Sessile organisms are good candidates for indicator species because they cannot change their habitat, have to deal with and/or adapt to shifts to less favorable conditions (Scheu et al., 2011). Being bioaccumulators, the study of their body burdens and pollution effects allow the determination of influence area and extent of impact, respectively (Carballeira et al., 2012). The uptake of organic matter from fish farms by associated invertebrates can be traced by examining changes in their fatty acid profile due to the assimilation of terrestrial-based oils used in fish feed (Gonzalez-Silvera et al., 2015; White et al., 2019). Additionally, metabolomics is emerging as a technique to assess the impact of aquaculture effluents on wild populations (Maruhenda-Egea et al., 2015).
Deducing the fate of nutrients in an aquaculture or natural system can be done based on balance calculations of the nutrient content in plants or animals (Vandermeulen and Gordin, 1990; Brown et al., 1999; Shpigel et al., 2013).
However, there are shortcomings to this technique when trying to account for all nutrient transformation in the system (Gribsholt et al., 2005). A more detailed view can be obtained through stable isotope analysis (SIA), a widely accepted tool to reconstruct diets and trophic relationships of organisms and their food (DeNiro and Epstein, 1978; Middelburg, 2014). The analysis of stable isotopes is often applied in marine or estuarine sciences and can be used to examine fluxes of carbon and nitrogen from ecosystems and pollution from coastal aquaculture (Peterson and Fry, 1987; Herbeck et al., 2014; Viana et al., 2015). Compared to the natural aquatic ecosystem, aquaculture derived nutrients are usually enriched in their δ15N and depleted in their δ13C values, allowing this source to be traced along gradients and into sinks (Holmer et al., 2007; Watai et al., 2015). In case larger isotopic differences are needed, isotope labeling (the introduction of compounds high in the heavy isotope) can be applied to trace the fate of the labeled matter over time, through specific metabolic pathways or along trophic chains (Berthold et al., 1991; Burford et al., 2002; Gribsholt et al., 2005; Barrón et al., 2006). A number of studies have worked with labeled feed in an aquaculture context, tracing the fate of feed derived nutrients in the vicinity of fish cages (Felsing et al., 2006; Gonzalez-Silvera et al., 2015; White et al., 2017, 2019), monitoring nutrient uptake in different fish tissues (Felip et al., 2012; Maruhenda-Egea et al., 2015), or identifying uptake of cyanobacteria in an aquaculture pond (Wang Z. et al., 2015).
Establishing a monitoring system based on isotope values of an indicator species gives the opportunity to trace the impact of aquaculture effluents in the surrounding ecosystem and check the effectiveness of mitigation measures (Costanzo et al., 2005).
Eutrophication and toxicity assessment methods of hard bottom sites should always integrate physicochemical and biological indicators as a basis for management decisions although some methods use only selected water column parameters (Ferreira et al., 2011). Contaminant concentrations may not be useful parameters (most times below the detection limits of analytical methods) while properly selected indicators should show a gradient that reflects the level of human-induced impairment.
No single set of indicators will meet the needs worldwide so general guidelines for the selection of indicator species must be established, both for soft and hard substrates (Rice et al., 2012). Hard substrates sites require the selection of sensitive or tolerant sessile organisms (Scheu et al., 2011), such as macroalgae, bivalves, cnidarians, and defined ecological state groups for both unpolluted and contaminated situations.
Other indicator species, such as sponges (Porifera) have shown to be strong indicators of environmental changes (Luter et al., 2014) and are highly sensitive to environmental shifts (Holmes, 2000; Hill and Hill, 2002).
In the case that no sessile organisms or study matrices (apart from the water column) are present, studies on accumulation as well as on diversity or abundance of species could be focused on the study of the colonizing communities of artificial surfaces previously placed in areas affected by aquaculture activities.
One of the main challenges for the sustainable development of aquaculture is the distribution of water, land, and other resources with alternative uses, such as fishing, agriculture, and tourism. Marine Spatial Planning must consider the zoning of aquaculture (to define suitable areas for fish farming or mixed activities) and identifying the most appropriate places for the specific location of farms (site selection) (Chen et al., 2007). Environmental impacts of a single farm may not be significant when considered individually but may be relevant if other farms, fishing grounds, or activities are located in the same area. Environmentally sound selections of the site, away from habitats of ecological interest, together with adequate management, are the best tools to prevent or minimize the negative environmental effects of farming (Porporato et al., 2020). Therefore, aquaculture operators must act as environmental managers to ensure a pollution-free environment in which to culture healthy organisms. Impacts on the benthos and water column may happen because of an improper site selection, administrative issues, and/or overproduction (Aguilar-Manjarrez et al., 2017; Shetty et al., 2018).
Geographic Information Systems (GIS), remote sensing, and modeling are commonly used for aquaculture site selection because they provide preliminary information on site assessment. However, they are mainly based on physical variables, e.g., water temperature and chlorophyll a concentration (chl a) (Huber et al., 2016; Stelzenmüller et al., 2017). From a sustainable point of view, the location of farms must consider the ECC of the waterbody and get in situ background information (e.g., isotopic signal 15N, biodiversity and metal contents) to enable the necessary production with the least possible adverse impact on the environment and consequently, the farm itself. For this reason, assessment of suitability must consider conservative estimates (i.e., precautionary principle), presence of predators, spacing between the proposed site and other farms, industries, recreational activities, cultural or ecological assets, and environmental characteristics (FAO, 2018).
In general, more carrying capacity will be achieved at oligotrophic sites with higher coastal exposure, waves, seafloor slope, depth, current speed, salinity, dissolved oxygen and lower temperature, suspended solids, and colonizing capacity (Garmendia et al., 2012). However, the multifactorial nature of the ecological capacity makes it difficult to evaluate it theoretically. Moreover, the contribution of other specific or diffuse sources of pollution (e.g., wastewater treatment plants, agricultural activities, river mouths) that occur in a specific physical space must also be considered.
The requirements for appropriate sites are directly related to the interplay of the farm with the surrounding environment. Recirculating Aquaculture Systems (RAS) provide the possibility to cultivate fish in a closed system, minimizing the threat of parasites, diseases, and changing environmental conditions. It also allows for the immediate treatment of waste. As land-based facilities, RAS may be located almost everywhere while net cages must be located at protected coasts with high dispersive capacity and far from other sources of pollution. However, RAS operations require specific infrastructure and know-how of the system and have high starting and operational costs (Badiola et al., 2012). While they are becoming increasingly important in the aquaculture sector of developed countries, their successful widespread application needs further economic and political incentives as well as widespread sharing of information (Badiola et al., 2012). Unlike offshore cultures, RAS facilities also compete for land space with other industries.
Land-based marine fish farms are economically limited by the pumping of water inside the facilities (elevation from sea) and need to be located at exposed coasts with high dispersive capacity (Carballeira et al., 2012). Facilities may be ordered (from the highest to the lowest) according to the level of site requirements as follows: Net cages, marine IMTA, land-based, offshore, IMTA land-based, RAS.
Site selection must also integrate multiple use marine spatial plans to consider economic and social objectives from all affected sectors and achieve successful management. Wind farming and energy production from micro and macroalgae are compatible candidates, being the best ones that are based on nutrient removal (Stelzenmüller et al., 2017).
An important step towards sustainable aquaculture is to consider excess food and fecal matter not as a waste product, but as a resource that contains high amounts of nutrients and essential fatty acids that should be recycled and not discarded (Bischoff et al., 2009). Based on this idea the concept of IMTA was created, which applies a simplified food web structure to a farming system of fed-species, such as fish and shrimp, together with extractive organisms, such as molluscs and seaweed that take up particles and nutrients from the environment (Neori et al., 2004). Integrated aquaculture also produces higher yields than mono-species systems in addition to satisfying rising consumer demands for environmental standards (Neori et al., 2004). The practice of IMTA aims to perfect this principle by combining species at different trophic levels for a balanced-ecosystem approach (Chopin et al., 2007). Reducing the load of nutrients and organic matter released by IMTA systems, preserves the quality of the receiving ecosystem, a secondary economic benefit is obtained and the social image of aquaculture is improved (Barrington et al., 2009).
Macroalgae are a popular component of IMTA setups and have a number of advantages over conventional mechanical or microbial filtration systems. Common nitrifications filters use up dissolved oxygen and require additional equipment and monitoring (Chopin et al., 2001). Contrary to this, integrating algae into an aquaculture system counterbalances nutrients, CO2 levels, acidity, and increases dissolved oxygen while producing valuable biomass (Chopin et al., 2001; Neori et al., 2003). Macroalgae are fast and easy to grow, highly productive, with a higher yield of cultivation than land plants, no need of pesticides and with great potential for various economic applications (Gao and McKinley, 1994; Ditchburn and Carballeira, 2019). An example of ideal seaweed for a biofilter is Ulva lactuca, which is fast-growing, has high filtration performance, thrives under high nutrient conditions, tolerates a wide range of water quality, and has a high market value (Neori et al., 2003, 2004). Sales of seaweed grown in biofilters can cover the additional costs of implementation (Neori et al., 2004; Ditchburn and Carballeira, 2019), but economic validity is not always ensured and the area required can be extremely large (Kang et al., 2003; Shpigel et al., 2013).
Rearing shellfish may have positive effects on the environment and promote biodiversity (Ozbay et al., 2014). Oysters and mussels cultivated close to fish farms benefit from the suspended organic matter, show improved growth and reduce organic nutrient load in the water (Sarà et al., 2009; Troell et al., 2009; Reid et al., 2010) and integrating mussel longline cultivation to existing operations should be economically viable (Whitmarsh et al., 2006). Their culture is, however, not a panacea as particle absorption is restricted to appropriate size ranges (Wang et al., 2012) and the benefits of bivalve culture sometimes fail to be achieved at all (Navarrete-Mier et al., 2010). Moreover, bivalves also produce particulate waste in the form of pseudofeces, creating a need for further remediation of impacted sediment (Cubillo et al., 2016).
Particulate waste of fed species or shellfish cultivation can be used as a source of feed for detritivorous animals such as polychaetes and sea cucumbers. Some sea cucumber species have been found to thrive on the debris of fish farms (Ahlgren, 1998; Slater et al., 2011; Hannah et al., 2013). They can constitute an additional crop and have beneficial effects on sediments by re-working upper sediment layers and influencing the development of microbial communities (Moriarty and Pollard, 1982; Moriarty et al., 1985; Uthicke, 1999; MacTavish et al., 2012). In closed aquaculture systems, such bioturbating detritivores can also be integrated into sand filters where oxic and anoxic conditions in the sediment become the site of nitrogen conversion and removal (Palmer, 2010; Robinson et al., 2015).
In the case of land-based farms in the littoral zone, IMTA can be arranged inside (indoor) or outside (outdoor) of the facilities (Figure 5). Outdoor systems require the aquatic installation of devices close to the source of pollution and in the direction of the prevailing current. This is a complex management option and may be limited by physiological requirements of the extractive species to specific seasons or hydrodynamic conditions. The strong energy of the sea in exposed coasts can endanger the structures or detach organisms, e.g., Saccharina is able to withstand strong waves but can only be grown in winter (Guerrero and Cremades, 2012; Freitas, 2015). On the contrary, indoor systems with their controlled conditions are more easily managed and are theoretically the most respectful option for the environment (Guerrero and Cremades, 2012), but compete for land space.
Figure 5. Example of integrated multi-trophic aquaculture outside (OUT) and inside (IN) of a facility.
Wastewater lagooning is a highly effective, low-cost solution (initial installation and maintenance) for purifying wastewater from land-based farms (Porrello et al., 2003). The treatment of wastewater consists of a series of physical, chemical, and biological processes to remove contaminants and separate clean or at least reusable water and solid waste, which can be used for a number of industrial or agricultural purposes. Such types of artificial wetlands are already widely used for the treatment of municipal waste and are especially effective at removing excess nitrogen and storing excess phosphorus in the soil (Vymazal, 2010; De Lange et al., 2013; Almuktar et al., 2018). This type of phytotreatment has already been tested with wastewater from fish ponds as means of algal ponds and wetlands and has shown to be an efficient system by reducing nutrient contents and modifying physico-chemical parameters of water (Porrello et al., 2003; Adeoye et al., 2009; Omitoyin et al., 2017).
However, lagooning systems require large surface areas, thus, competing for land space with other sectors. The installation of aeration systems makes artificial wetlands more efficient by enhancing the oxidation rate of pollutants and allows reducing the space needed (El-Kamah et al., 2011; Almuktar et al., 2018). Using the area for additional production makes this method more profitable. Various halophytes in different types of natural and constructed wetlands are already successfully used as down-stream filters of municipal, industrial, or agricultural wastewater (Verhoeven and Meuleman, 1999; Vymazal, 2010). Their use can have great economic benefits when compared to conventional water treatment and they are being investigated as possible extractive species in integrated aquaculture as they are able to utilize high levels of ammonia, which can be toxic to other plants (Glenn et al., 1991; Cardoch et al., 2000; Kudo and Fujiyama, 2010). Different species of halophytes have been successfully cultivated in the effluents of European sea bass (Dicentrarchus labrax) in RAS (Waller et al., 2015) and can be integrated in artificial wetlands or cultivated hydroponically (Singh et al., 2014). While still a niche market, they are gaining popularity as a delicacy vegetable (Custódio et al., 2017).
Maintaining the integrity and function of ecosystems is vital for the sustainable spread of aquaculture operations. An example is the practice of Silvofishery or Integrated Mangrove Aquaculture, which maintains a certain degree of tree cover in and around aquaculture ponds constructed in mangrove areas (Budihastuti et al., 2012; Bosma et al., 2016). Mangroves are important tropical ecosystems that provide a habitat and nursery to many species, prevent sedimentation of reefs and seagrass meadows, protect coasts from storm surges and flooding, and store atmospheric carbon dioxide (Nagelkerken et al., 2000, 2008). Many mangrove areas globally have already been lost to the conversion of coastal habitats, including for aquaculture production (Valiela et al., 2001; Alongi, 2002). Constructing aquaculture ponds while maintaining a level of tree cover in and around the ponds is a strategy how to combine food production with mangrove protection (Yunus et al., 2015). Sustainable aquaculture is seen as an important way to protect these ecosystems, preserve their biodiversity and secure their continued contribution to climate protection (Primavera, 2005; Ahmed et al., 2016). Such an approach to integrated cultivation is especially important in the context of tropical developing countries where the importance of aquaculture goes beyond intensive production of high-value species and includes its vital contribution to food production and livelihoods in rural areas (Harrison, 1996; Edwards, 2000). Especially in Asia, low-input, integrated fish farming systems have a long tradition (Chen et al., 2019). Large, government driven projects have small success rates if they create dependencies and have low adaptation rates by stakeholders (White et al., 2005). Community-based projects put local actors in the focus and aim to improve livelihoods through capacity building, diversification and business development (Pomeroy, 2012; Yuerlita et al., 2013). Extensive, co-managed aquaculture operations require low input and technologies while still being vital sources of income (Partelow et al., 2018; Senff et al., 2018).
Sourcing of aquaculture feed is one of the sustainability core challenges of marine finfish aquaculture (Klinger and Naylor, 2012). Intensified production and the cultivation of high value carnivorous fish largely depends upon the use of fish meal and fish oil as the main feed ingredients, making it a consumer of capture fisheries products, especially of nontargeted fisheries and small forage fish (Cao et al., 2015). This has caused environmental as well as economic concerns, with feed costs being a large part of total production expenses, and important progress has been made towards sustainability by improving feed efficiency (Verdal et al., 2017), turning fish offal into useful silage (Cunha et al., 2019; Gonçalves et al., 2019) or designing plant-based, polychaete-based, and insect-based protein feeds (Boyd, 2015; Pahlow et al., 2015; Rhodes et al., 2016; Gómez et al., 2019; Llagostera et al., 2019). The challenge has been to replace fish oil with other alternatives and ensure the high content of highly unsaturated fatty acids within the feed to maintain the nutritional quality of the fish for human consumption (Little et al., 2016). While the use of land-based feed may reduce the pressure on fisheries, it can significantly increase the pressure on freshwater resources (water footprint), due to water consumption and pollution in crop production (Pahlow et al., 2015).
However, recently new approaches have been developed to reduce excess feed used and loss of food. In intensive fish farming where feeding is taking place by an automatic system, it is important to monitor the feeding activity of the fish and adjust the amount of feed to the feeding behavior. Such monitoring can be done by using, for example, an underwater camera technology or other similar methods that detect uneaten feed and stopping the feeding process (Zhou et al., 2017).
More sustainable feed management can be achieved through IMTA in a number of ways. Dissolved nitrogen in wastewater used to promote the growth of micro- or macroalgae replaces the need for fertilizer or growth media. These extractive species can in turn be cultivated as a feed source, increasing their practical value. The macroalgae Ulva lactuca, which has low economic value when sold directly, provides high quality feed for abalone or sea urchins (Neori et al., 2000) and microalgae serve as feed for mussels (Shpigel et al., 1993). Thus, algae production can be considered as a more sustainable industry than continuing to harvest fish for fishmeal. It is estimated that if microalgae were used as fishmeal replacement, the effect would be to remove 30% of the fishing pressure thereby helping to conserve marine ecosystems (Beal et al., 2018). Algae may substitute fish oil, be viable and even improve growth of farmed fish so it should be considered to reduce harvesting fish for fishmeal (Sarker et al., 2020).
Effluents can be directly valorised by growing primary producers (Milhazes-Cunha and Otero, 2017; Wei et al., 2017; Li et al., 2019). Sludges and effluents from aquaculture activities may also be bioremediated by decomposers, detritivores, and biofilms, whose biomass in turn presents a useful resource for feed production (Martinez-Porchas et al., 2014; Barnharst et al., 2018; Gómez et al., 2019; Robinson et al., 2019). Solid wastes are an appropriate feed for polychaetes, which provide protein and important fatty acids as feed for fish, shrimp, or crab production, reducing or eliminating the need for manufactured feed based on farmed crops or fish meal (Brown et al., 2011; Alava et al., 2017; Pajand et al., 2017). Fish feed can also be supplemented or even replaced through the application of biofloc technology. In this approach, microbial growth in the water column of fish tanks or ponds is stimulated through the addition of carbohydrates in the form of sugar, starch, or cellulose (Avnimelech, 2012). Heterotrophic bacteria, using these as a substrate to build proteins for growth, require nitrogen, which they take from the surrounding water. Dissolved nitrogen species in the water are, thus, transformed into bacterial biomass, aggregating into bioflocs that can be consumed by shrimp or fish (Avnimelech, 2012). Biofloc technology in tilapia ponds can provide 50% of the protein consumed by the fish (Avnimelech, 2007) and when used as a protein source in shrimp feed, microbial floc meal showed the same results or even outperformed soybean or fishmeal ingredients (Kuhn et al., 2009, Kuhn et al., 2010; Bauer et al., 2012). Biofloc systems have been mainly used in freshwater ecosystem and for herbivorous organisms but recent studies included mullets and pacific shrimp (Legarda et al., 2019). The successful application of biofloc technology, however, requires knowledge of the system, close monitoring to maintain appropriate C:N ratios and microbial densities and upscaling from laboratory to economic application (Kuhn et al., 2010; Hende et al., 2014).
There is an increasing tendency to develop methods with the aim of reducing extensive chemical substance use, and, therefore, minimizing environmental pollution. Such alternative methods can have other positive effects on production such as cost minimization for the producer and increased consumer acceptance.
Methods that have been used vary between several factors such as the fish species and disease in question as well as the production methods used and characteristics of the area where the facility is located. They can be divided as precautionary methods (e.g., limiting fish density), methods concerning disease prevention (e.g., immunostimulant feeds and herbal medicine additives), co-culturing of different marine species which both benefits from such a production model, different physical techniques used such as so called “lice skirts,” plankton nets around the fish cage, to avoid parasite infestations (Stien et al., 2018) as well as other varying from above mentioned aspects (He et al., 2016; Haugland et al., 2017; Dawood et al., 2018; Jahangiri and Esteban, 2018; Escobar-Dodero et al., 2019).
The largest aquaculture producing countries have been using regulations to report disease occurrence and to limit the fish density according to those measures because high density of fish or cages highly increase the risk of disease transmission, e.g., infectious salmon anemia or infectious pancreatic necrosis (Escobar-Dodero et al., 2019).
Other methods that have been used include functional feed additives, which can be administered via feed (He et al., 2016). They are non-nutritive ingredients that affect fish performance concerning, for example, feed utilization, survival, and growth rate (Jahangiri and Esteban, 2018). These additives promote fish growth and can replace antibiotics in those countries where it is still used as preventing and growth promotional measures, and thereby avoiding associated negative effects (Done et al., 2015). Most widely researched options include probiotics, prebiotics, synbiotics, acidifiers, plant extracts, nucleotides, and such immunostimulants as β-glucan and lactoferrin (Dawood et al., 2018). Chinese herbal medicines can be used as an alternative in disease prevention in aquaculture because of antibacterial, antiviral, immunostimulant, and growth promoting substances (He et al., 2016).
Co-culturing of different fish species can be used to limit pathogens during production. An example of this could be the use of cleaner fishes, which can limit the spread of ectoparasites such as salmon lice (Lepeophtheirus salmonis) because of predation by those fish species towards these small crustaceans. However, this implies that appropriate health and welfare conditions must be provided for both species, as farming together different species would also generate a risk of common disease spread, e.g., amoebic gill disease when lumpfish (Cyclopterus lumpus) are farmed together with Atlantic salmon (Haugland et al., 2017).
Genetic improvement through selective breeding has been used in aquaculture mostly to improve growth performance of fish. In Europe, around 80% of aquaculture originates from selective breeding mainly because of this reason. However, it is possible to select fish for other traits such as disease susceptibility (Yáñez et al., 2014). Also, vaccines are being used to minimize the need for antibiotic treatments. However, the method can be associated with some negative effects such as stress caused to fish during the treatment when handling of fish is involved (Miccoli et al., 2019).
Lytic bacteriophages can be used as therapeutic agents against marine bacterial diseases such as those associated with vibrios. However, more research is still needed to apply these viruses under field conditions and to avoid dispersal of unwanted genes and effects on fish microbiota (Kalatzis et al., 2018; Plaza et al., 2018).
Generally, mariculture facilities are located close to human settlements, in protected coasts or inland, with shallow waters and low hydrodynamic energy. Recently, more aquaculture facilities are being placed in semi-exposed areas with a potential of offshore aquaculture development. To have a regular supply of oxygen and temperature, and to avoid diseases, parasites, algal blooms, and user conflicts, the aquaculture industry has been developing new technologies for offshore facilities. Offshore systems are not environmentally sustainable in the long term (there is no reduction on contaminant outputs) but are a more respectful alternative with the environment since the load capacity increases with the depth and the higher dispersion of the waste (Kapetsky and Aguilar Manjarez, 2013; Lovatelli et al., 2013; Gentry et al., 2016). From a spatial perspective, offshore production has a lot of potential; it may be expanded to many countries, move away from sensitive marine biocenosis areas, be combined with wind, oyster, and mussel farms, increase biodiversity by creating an artificial reef, etc. (Lovatelli et al., 2013). However, the open sea is considerably rougher than coastal waters and strong waves can break structures or detach organisms, worldwide distribution of species is uncertain, and there are political, technical, and cost-distance limitations (Troell et al., 2009; Forster, 2013). Free-floating and propelled installations may be too expensive and improvements on mooring systems are required to expand farms at deeper areas.
Some finfish farms are already located at open sea, most of them are round and submersible net cages to dissipate water currents and to avoid waves (Benetti et al., 2010). Seaweed and bivalve become profitable more quickly because they do not need to be fed and operational costs are lower. Several farms including IMTA have been developed, mainly in Asian countries, but all at shallow waters and close to the coast (Buck et al., 2018). Moreover, it is still difficult to avoid the detachment of seaweed and mussels with traditional longline systems (Troell et al., 2009).
Wind-powered water pumps and solar-powered water heating systems are proposed to be integrated with offshore systems because will reduce long-term operating costs and environmental implications and increase competitiveness and profitability.
For aquaculture to expand, greater social acceptance, adapted regulations and long-term sustainability are necessary (Kapetsky and Aguilar Manjarez, 2013; Lovatelli et al., 2013). However, offshore production development is sometimes limited because few countries have regulations that explicitly mentions offshore or open-ocean aquaculture, and fewer still have codified definitions of this type of culture (Davies et al., 2019). Sometimes governance of offshore industries is ambiguous or undefined, presenting obstacles to allow their activities (e.g., United States and Australia) (Davies et al., 2019).
Biofouling development reduces the water flow inside the fish cage forcing farmers to clean and change polymer nets frequently, or use antifouling coatings, thus, increasing operational costs (Berillis et al., 2017). Antifouling coatings are discarded every year producing toxic effects because of the release of their main active ingredients to the environment (copper oxide, cadmium, and zinc) (Kalantzi et al., 2016). Organisms grown inside and outside of trials using copper nets were safe for human consumption and no accumulation of copper was detected in sediment, but assessment and environmental monitoring is needed because copper alloy released more copper to the surrounding environment and affect the biota more compared to the conventional net (Kalantzi et al., 2016).
There is a recent sustainable framework of fish farming called Precision Fish Farming (PFF), which developed from the concept of Precision Livestock Farming (PLF) (Norton and Berckmans, 2018) to pisciculture. PLF and PFF use hardware (e.g., sensors), observers, and intelligent software to improve animal health and welfare while increasing productivity, yield, and environmental sustainability (Figure 6). In contrast to livestock production, intensive fish farming methods are more recent, crop is largely determined by environmental conditions and monitoring methods are more expensive and complex at aquatic ecosystems (Føre et al., 2018).
Figure 6. Example of precision fish farming system including (a) surface camera, (b) underwater camera with multiparameter water probe, (c) sonar, (d) acoustic telemetry system with hydrophone, and (e) smart trap (based on Føre et al., 2018).
The majority of components proposed to be included in PFF systems are already developed (e.g., hydrophones, sonars, acoustic telemetry systems, cameras) but in many cases they need to be adapted for mariculture activities. For example, smart sediment traps will greatly improve knowledge on particle settling flux data, oceanic nutrient cycles, food efficiency through online monitoring and detection of temporal changes of sedimentation rates (Jurg, 1996). Studies of smart traps include autonomous neutrally buoyant sediment traps (Sherman et al., 2011) and sedimentation accumulation sensors with a wiper arm connected to a logger and a transmitter/receiver (Thomas and Ridd, 2005; Whinney et al., 2017).
Fish farming monitoring knowledge is required to understand the complex interactions between growing methods and farmed fish with the aquatic environment. An increase on the knowledge of behavioral and physiological responses may help reach sustainability (by reducing feed loose, disease and parasitic outbreaks, enhance animal vital development, etc.) but it seems that the general aim is to improve intensive fish farming conditions from a production perspective instead of an environmental approach (carrying capacity, feed alternative, etc.). To apply a more sustainable concept of PFF, predictive models should include the information supplied by the application of technological advances to monitor other ecosystem parameters potentially affected by crops.
The key to affordable and sustainable aquaculture practices lies in the independence from natural resources through recycling or remediation of fish production wastes. However, sustainability and the reduction of ecological impacts depend on the governance of infrastructure and environmental agencies must improve legislation and regulations worldwide. Many countries with important aquaculture production have some environmental regulations but lack clear frameworks for emerging technologies such as offshore farming. Every farmer may develop sustainable principles, methodologies, or practices. Therefore, it is recommended to promote certifications of good practices.
Environmental monitoring costs may be too expensive and onerous, hindering its application or interpretation and make sustainable development more difficult. So that, just representative, non-redundant, reliable, cost-effective, and significant measures should be included within environmental monitoring plans to harmonize production with the ECC of the selected site.
Different methods are being used to reduce the environmental impact of aquaculture practices, but further research is still needed. For example, the use of selective breeding to reduce the use of chemicals for disease treatment may diminish the population genetic difference and possibly increase the virulence of the pathogenic organisms that may specialize in particular organisms. Also, the use of vaccines represents a sustainable method but can cause significant stress for the fish during the vaccination which itself can increase the disease risk.
Land-based IMTA aquaculture with recirculation systems seems to be the best alternative option in terms of wastewater reduction and exploitation, prevention of disease outbreaks, escapees, monitoring costs and efficiency. However, there is a competition for land space that can be complemented by well-managed offshore polyculture facilities integrated with solar and wind farms. Despite those, numerous other sustainable measures were proposed (Figure 7).
Figure 7. Summary of main sustainability issues of marine mariculture and respective methodological (black boxes) and infrastructure based (gray boxes) solutions.
Aquaculture sustainability is an on-going process that requires integration of all stakeholders. Government, farmers, ecologists, and consumers should drive aquaculture practices under a risk assessment approach to reduce wastes, disease outbreaks, and operational costs enhancing sustainability potential.
All authors contributed to conception and design of the study, wrote sections of the manuscript, prepared the figures, and revised, read, and approved the submitted version.
CC is a postdoctoral researcher supported by the FONDECYT Postdoctoral Programme (3170795).
The authors declare that the research was conducted in the absence of any commercial or financial relationships that could be construed as a potential conflict of interest.
We are grateful for all the valuable comments from the academic editor and reviewer, who we believe have strengthened our manuscript significantly. We thank Dr. Simon Jungblut, Christina Hörterer, Stacy Ballyram, Arley Muth, and Francine Beaujot whose insightful comments and suggestions have helped to improve this review.
Adeoye, P., Musa, J. J., and Akinyemi, B. A. (2009). Design of aerated lagoon for fish pond wastewater treatment. Aust. J. Dairy Technol. 12, 188–192.
Aguado-Giménez, F., Sallent-Sánchez, A., Eguía-Martínez, S., Martínez-Ródenas, J., Hernández-Llorente, M. D., Palanca-Maresca, C., et al. (2016). Aggregation of European storm-petrel (Hydrobates pelagicus ssp. melitensis) around cage fish farms. Do they benefit from the farms resources? Mar. Environ. Res. 122, 46–558.
Aguilar-Manjarrez, J., Soto, D., and Brummett, R. (2017). Aquaculture Zoning, Site Selection and Area Management Under the Ecosystem Approach to Aquaculture. A Handbook. Rome: Food and Agriculture Organization of the United Nations
Ahlgren, M. O. (1998). Consumption and assimilation of salmon net pen fouling debris by the red sea cucumber parastichopus californicus: implications for polyculture. J. World Aquac. Soc. 29, 133–139. doi: 10.1111/j.1749-7345.1998.tb00972.x
Ahmed, N., and Thompson, S. (2018). The blue dimensions of aquaculture: a global synthesis. Sci. Total. Environ. 652, 851–861. doi: 10.1016/j.scitotenv.2018.10.163
Ahmed, N., Bunting, S. W., Glaser, M., Flaherty, M. S., and Diana, J. S. (2016). Can greening of aquaculture sequester blue carbon? Ambio 46, 468–477. doi: 10.1007/s13280-016-0849-7
Alava, V. R., Biñas, J. B., and Mandario, M. A. E. (2017). “Breeding and culture of the polychaete, marphysa mossambica, as feed for the mud crab,” in Philippines: In the Forefront of the Mud Crab Industry Development: Proceedings of the 1st National Mud Crab Congress, 16-18 November 2015, Iloilo City, Philippines, eds E.T. Quinitio, F.D. Parado-Estepa, and R.M. Coloso (Philippines: Southeast Asian Fisheries Development Center), 39–45
Almuktar, S. A. A. A. N., Abed, S. N., and Scholz, M. (2018). Wetlands for wastewater treatment and subsequent recycling of treated effluent: a review. Environ. Sci. Pollut. Res. 25, 23595–23623. doi: 10.1007/s11356-018-2629-3
Alongi, D. M. (2002). Present state and future of the world’s mangrove forests. Environ. Conserv. 29, 331–349. doi: 10.1017/S0376892902000231
Amundsen, V. S., and Osmundsen, T. C. (2018). Sustainability indicators for salmon aquaculture. Data Brief 20, 20–29.
Arechavala-Lopez, P., Toledo-Guedes, K., Izquierdo-Gomez, D., Šegviæ-Bubiæ, T., and Sanchez-Jerez, P. (2018). Implications of sea bream and sea bass escapes for sustainable aquaculture management: a review of interactions, risks and consequences. Rev. Fish. Sci. Aquacult. 26, 214–234.
Arechavala-Lopez, P., Uglem, I., Izquierdo-Gomez, D., Fernandez-Jover, D., and Sanchez-Jerez, P. (2017). Rapid dispersion of escaped meagre (Argyrosomus regius) from a coastal mediterranean fish farm. Aquac. Res. 48, 1502–1512.
Avnimelech, Y. (2007). Feeding with microbial flocs by tilapia in minimal discharge bio-flocs technology ponds. Aquaculture 264, 140–147. doi: 10.1016/j.aquaculture.2006.11.025
Avnimelech, Y. (2012). Control of microbial activity in aquaculture systems: active suspension ponds. World Aquaculture 34, 19–21.
Ayer, N. W., and Tyedmers, P. H. (2009). Assessing alternative aquaculture technologies: life cycle assessment of salmonid culture systems in Canada. J. Clean. Prod. 17, 362–373. doi: 10.1016/j.jclepro.2008.08.002
Badiola, M., Mendiola, D., and Bostock, J. (2012). Aquacultural engineering recirculating aquaculture systems (ras) analysis: main issues on management and future challenges. Aquac. Eng. 51, 26–35. doi: 10.1016/j.aquaeng.2012.07.004
Ballester-Moltó, M., Sanchez-Jerez, P., and Aguado-Giménez, F. (2017a). Consumption of particulate wastes derived from cage fish farming by aggregated wild fish. An experimental approach. Mar. Environ. Res. 130, 166–173.
Ballester-Moltó, M., Sanchez-Jerez, P., Cerezo-Valverde, J., and Aguado-Giménez, F. (2017b). Particulate waste outflow from fish-farming cages. How much is uneaten feed? Mar. Poll. Bull. 119, 23–30.
Barnharst, T., Rajendran, A., and Hu, B. (2018). Bioremediation of synthetic intensive aquaculture wastewater by a novel feed-grade composite biofilm. Int. Biodeter. Biodegr. 126, 131–142. doi: 10.1016/j.ibiod.2017.10.007
Barrington, K., Chopin, T., and Robinson, S. (2009). “Integrated multi-trophic aquaculture (IMTA) in marine temperate waters”, in Integrated Mariculture: A Global Review, ed. D. Soto (Rome: FAO) FAO Fisheries and Aquaculture Technical Paper. N° 529.
Barrón, C., Middelburg, J. J., and Duarte, C. M. (2006). Phytoplankton trapped within seagrass (Posidonia oceanica) sediments are a nitrogen source: an in situ isotope labeling experiment. Limnol. Oceanogr. 51, 1648–1653.
Bauer, W., Prentice-Hernandez, C., Tesser Borges, M., Wasielesky, W., and Poesch, L. H. S. (2012). Substitution of fishmeal with microbial floc meal and soy protein concentrate in diets for the pacific white shrimp Litopenaeus vannamei. Aquaculture 342–343, 112–116. doi: 10.1016/j.aquaculture.2012.02.023
Beal, C. M., Gerber, L. N., Thongrod, S., Promkunthong, W., Kiron, V., Granados, J., et al. (2018). Marine microalgae commercial production improves sustainability of global fisheries and aquaculture. Sci. Rep. 8:15064. doi: 10.1038/s41598-018-33504-w
Belando, M. D., Marín, A., Aboal, M., García-Fernández, A. J., and Marín-Guirao, L. (2017). Combined in situ effects of metals and nutrients on marine biofilms: shifts in the diatom assemblage structure and biological traits. Sci. Total. Environ. 574, 381–389.
Benetti, D., Benetti, G. I., Rivera, J. A., Sardenberg, B., and O’Hanlon, B. (2010). Site selection criteria for open ocean aquaculture. Mar. Technol. Soc. J. 44, 22–35.
Berillis, P., Mente, E., and Kormas, K. A. (2017). The use of copper alloy in aquaculture fish net pens: mechanical, economic and environmental advantages. J. FisheriesScicom 11, 1–3.
Berthold, H. K., Hachey, D. L., Reeds, P. J., Thomas, O. P., Hoeksema, S., and Klein, P. D. (1991). Uniformly 13C-labeled algal protein used to determine amino acid essentiality in vivo. Proc. Natl. Acad. Sci. U.S.A. 88, 8091–8095.
Beveridge, M., and Little, D. (2002). “The history of aquaculture in traditional societies,” in Ecological Aquaculture: The Evolution of the Blue Revolution, ed. B.A. Costa-Pierce (Oxford: Blackwell Science), 3–29
Bischoff, A. A., Fink, P., and Waller, U. (2009). The fatty acid composition of Nereis diversicolor cultured in an integrated recirculated system: possible implications for aquaculture. Aquaculture 296, 271–276. doi: 10.1016/j.aquaculture.2009.09.002
Bohnes, F. A., and Laurent, A. (2021). Environmental impacts of existing and future aquaculture production: comparison of technologies and feed options in Singapore. Aquaculture 532:736001.
Borja, Á., Rodríguez, J. G., Black, K., Bodoy, A., Emblow, C., Fernandes, T. F., et al. (2009). Assessing the suitability of a range of benthic indices in the evaluation of environmental impact of fin and shellfish aquaculture located in sites across Europe. Aquaculture 293:231240.
Bosma, R. H., Nguyen, T. H., Siahainenia, A. J., Tran, H. T. P., and Tran, H. N. (2016). Shrimp-based livelihoods in mangrove silvo-aquaculture farming systems. Rev. Aquac. 8, 43–60. doi: 10.1111/raq.12072
Bostock, J., Murray, F., Muir, J., Telfer, T., Lane, A., Anagnopoulos, N., et al. (2009). European Aquaculture Competitiveness: Limitations and Possible Strategies. Brussels: Directorate General For Internal Policies, Policy Department B: Structural and Cohesion Policies Fisheries. Brussels: European Parliament.
Boyd, C. E. (2015). “Overview of aquaculture feeds: global impacts of ingredient use,” in Feed and Feeding Practices in Aquaculture, ed. D. Allen Davis (Sawston: Woodhead Publishing), 3–25. doi: 10.1016/B978-0-08-100506-4.00001-5
Brager, L. M., Cranford, P. J., Jansen, H., and Stranch, Ø. (2016). Temporal variations in suspended particulate waste concentrations at open-water fish farms in Canada and Norway. Aquacult. Environ. Interact. 8, 437–452. doi: 10.3354/aei00190
Brown, J. J., Glenn, E. P., Fitzsimmons, K. M., and Smith, S. E. (1999). Halophytes for the treatment of saline aquaculture effluent. Aquaculture 175, 255–268. doi: 10.1016/S0044-8486(99)00084-8
Brown, N., Eddy, S., and Plaud, S. (2011). Utilization of waste from a marine recirculating fish culture system as a feed source for the polychaete worm, Nereis virens. Aquaculture 322–323, 177–183. doi: 10.1016/j.aquaculture.2011.09.017
Brundtland, G. H. (1987). Our common future: report of the world commission on environment and development. Med. Confl. Surviv. 4:300. doi: 10.1080/07488008808408783
Buck, B. H., Troell, M. F., Krause, G., Angle, D. L., Grote, B., and Chopin, T. (2018). State of the art and challenges for offshore integrated multi-trophic aquaculture (IMTA). Front. Mar. Sci. 5:165. doi: 10.3389/fmars.2018.00165
Budihastuti, R., Anggoro, S., and Saputra, S. W. (2012). The application of silvofishery on tilapia (Oreochromis niloticus) and milkfish (Chanos chanos) fattening within mangrove ecosystem of the Northern coastal area of Semarang City. J. Coast. Dev. 16, 1410–5217.
Bueno, G. W., Bureau, D., Skipper-Norton, J.O. Roubach, R., Tavares de Mattos, F., and Bernal, F. E. M. (2017). Mathematical modelling for the management of the carrying capacity of aquaculture enterprises in lakes and reservoirs. Pesq. Agropec. Bras. 52, 695–706.
Burford, M., Preston, N. P., Gilbert, P. M., and Dennison, W. C. (2002). Tracing the fate of 15N-enriched feed in an intensive shrimp system. Aquaculture 206, 199–216.
Burridge, L., Weis, J. S., Cabello, F., Pizarro, J., and Bostick, K. (2010). Chemical use in salmon aquaculture: a review of current practices and possible environmental effects. Aquaculture 306, 7–23.
Buschmann, A. H., Riquelme, V. A., Hernández-González, M. C., Varela, D., Jiménez, J. E., Henríquez, L. A., et al. (2006). A review of the impacts of salmonid farming on marine coastal ecosystems in the southeast Pacific. ICES J. Mar. Sci. 63, 1338–1345.
Buschmann, A. H., and Muñoz, J. L. (2019). “Challenges for future salmonid farming,” in Encyclopedia of Ocean Sciences, 3rd Edn, eds J.K. Cochran, H. Bokuniewicz, and P. Yager (Amsterdam: Elsevier), 2, 313–319
Caeiro, S. S., Del Valls, T. A., and Chapman, P. M. (2012). Considerations for integrative environmental assessments of contaminated estuarine sediments. Manag. Environ. Qual. 23, 400–413.
Cao, L., Naylor, R., Henriksson, P., Leadbitter, D., Metian, M., Troell, M., et al. (2015). China’s aquaculture and the world’s wild fisheries. Science 347, 133–135.
Carballeira, C., Carballeira, A., Aboal, J. R., and Fernández, J. A. (2019). Biomonitoring freshwater FISH farms by measuring nitrogen concentrations and the δ15N signal in living and devitalized moss transplants. Environ. Poll. 245, 1014–1021.
Carballeira, C., Cebro, A., Villares, R., and Carballeria, A. (2018). Assessing changes in the toxicity of effluents from intensive marine fish farms over time by using a battery of bioassays. Environ. Sci. Poll. Res. 25, 12739–12748.
Carballeira, C., Ramos Gómez, J., Martín Díaz, M. L., DelValls, T. A., and Carballeira, A. (2012). Designing an integrated environmental monitoring plan for land-based marine fish farms located at exposed and hard bottom coastal areas. J. Environ. Monit. 14, 1305–1316.
Cardoch, L., Day, J. W., Rybczyk, J. M., and Kemp, G. P. (2000). An economic analysis of using wetlands for treatment of shrimp processing wastewater - a case study in Dulac, LA. Ecol. Econ. 33, 93–101. doi: 10.1016/S0921-8009(99)00130-5
Chapman, E. J., and Byron, C. J. (2018). The flexible application of carrying capacity in ecology. Glob. Ecol. Conserv. 13:e00365.
Chen, J., Guang, C., Xu, H., Chen, Z., Xu, P., Yan, X., et al. (2007). “A review of cage and pen aquaculture: China,” in Cage Aquaculture – Regional Reviews and Global Overview, eds M. Halwart, D. Soto, and J.R. Arthur (Rome: FAO), FAO Fisheries Technical Paper. No. 498, 50–68
Chen, W., He, B., Nover, D., Lu, H., Liu, J., Sun, W., et al. (2019). Farm ponds in southern China: challenges and solutions for conserving a neglected wetland ecosystem. Sci. Total. Environ. 659, 1322–1334.
Chopin, T., Buschmann, A. H., Halling, C., Troell, M., Kautsky, M., Neori, A., et al. (2001). Integrating seaweeds into marine aquaculture systems: a key toward sustainability. J. Phycol. 37, 975–986. doi: 10.1046/j.1529-8817.2001.01137.x
Chopin, T., Yarish, C., and Sharp, G. (2007). “Beyond the monospecific approach to animal aquaculture — the light of integrated multi-trophic aquaculture,” in Ecological and Genetic Implications of Aquaculture Activities, ed. T. Bert (Dordrecht: Springer), 447–458
Conley, D. J., Paerl, H. W., Howarth, R. W., Boesch, D. F., Seitzinger, S. P., Havens, K. E., et al. (2009). Controlling eutrophication: nitrogen and phosphorus. Science 323, 1014–1015. doi: 10.1126/science.1167755
Copus, A. K., and Crabtree, J. R. (1996). Indicators of socio-economic sustainability: an application to remote rural Scotland. J. Rural Stud. 12, 41–54. doi: 10.1016/0743-0167(95)00050-X
Costanzo, S. D., Udy, J., Longstaff, B., and Jonas, A. (2005). Using nitrogen stable isotope ratios (δ15N) of macroalgae to determine the effectiveness of sewage upgrades: changes in the extent of sewage plumes over four years in Moreton Bay, Australia. Mar. Pollut. Bull. 51, 212–217. doi: 10.1016/j.marpolbul.2004.10.018
Costa-Pierce, B. (2002). Ecological Aquaculture: The Evolution of the Blue Revolution. Oxford: Blackwell Publishing
Cromey, C., Thetmeyer, H., Lampadariou, N., Black, K. D., Kögeler, J., and Karakassis, I. (2012). MERAMOD: predicting the deposition and benthic impact of aquaculture in the eastern Mediterranean Sea. Aquacult. Environ. Interact. 2, 157–176.
Cubillo, A. M., Ferreira, J. G., Robinson, S. M. C., Pearce, C. M., Corner, R. A., and Johansen, J. (2016). Role of deposit feeders in integrated multi-trophic aquaculture — a model analysis. Aquaculture 453, 54–66.
Cunha, M. E., Quental-Ferreira, H., Parejo, A., Gamito, S., Ribeiro, L., Moreira, M., et al. (2019). Understanding the individual role of fish, oyster, phytoplankton and macroalgae in the ecology of integrated production in earthen ponds. Aquaculture 512:734297.
Custódio, M., Villasante, S., Cremades, J., Calado, R., and Lillebø, A. I. (2017). Unravelling the potential of halophytes for marine integrated multi-trophic aquaculture (IMTA) - a perspective on performance, opportunities and challenges. Aquac. Environ. Interact. 9, 445–460. doi: 10.3354/AEI00244
Davies, I. P., Carranza, V., Froehlich, H. E., Gentry, R. R., Kareiva, P., and Halpern, B. S. (2019). Governance of marine aquaculture: Pitfalls, potential, and pathways forward. Mar. Policy 104, 29–36.
Dawood, M. A. O., Koshio, S., and Esteban, M. Á. (2018). Beneficial roles of feed additives as immunostimulants in aquaculture: a review. Rev. Aquacult. 10, 950–974.
De Lange, H. J., Paulissen, M. P. C. P., and Slim, P. A. (2013). ‘Halophyte filters’: the potential of constructed wetlands for application in saline aquaculture. Int. J. Phytoremediation 15, 352–364. doi: 10.1080/15226514.2012.702804
Dempster, T., and Sanchez-Jerez, P. (2008). “Aquaculture and coastal space management in europe: an ecological perspective,” in Aquaculture in the Ecosystem, eds M. Holmer, C.M. Duarte, I. Karakassis, et al. (Dordrecht: Springer), 87–116
Dempster, T., Arechavala-Lopez, P., Barrett, L. T., Fleming, I. A., Sanchez-Jerez, P., and Uglem, I. (2016). Recapturing escaped fish from marine aquaculture is largely unsuccessful: alternatives to reduce the number of escapees in the wild. Rev. Aquac. 10, 153–167.
Dempster, T., Sanchez-Jerez, P., Tuya, F., Fernandez-Jover, D., Bayle-Sempere, J., Boyra, A., et al. (2006). Coastal aquaculture and conservation can work together. Mar. Ecol. Prog. Ser. 314, 309–310.
DeNiro, M. J., and Epstein, S. (1978). Influence of diet on the distribution of carbon isotopes in animals. Geochim. Cosmochim. Acta. 425, 495–506. doi: 10.1016/0016-7037(78)90199-0
Ditchburn, J. L., and Carballeira, C. B. (2019). Versatility of the humble seaweed in biomanufacturing. Procedia Manuf. 32, 87–94.
Done, H. Y., Venkatesan, A. K., and Halden, R. U. (2015). Does the recent growth of aquaculture create antibiotic resistance threats different from those associated with land animal production agriculture? AAPS J. 17, 513–524. doi: 10.1208/s12248-015-9722-z
Duarte, C. M., Holmer, M., Olsen, Y., Soto, D., Marbá, N., Guiu, J., et al. (2009). Will the oceans help feed humanity? Bioscience 59, 967–976.
Edwards, P. (2000). Aquaculture, poverty impacts and livelihoods. ODI Nat. Resour. Perspect. 56, 1–4.
Edwards, P. (2004). Traditional Chinese aquaculture and its impact outside China. J. World Aquacult. Soc. 35, 24–27.
Edwards, P., Zhang, W., Belton, B., and Little, D.C. (2019). Misunderstandings, myths and mantras in aquaculture: Its contribution to world food supplies has been systematically over reported. Mar. Policy 106, 103547–103556.
El-Kamah, H. M., Badr, S. A., and Moghazy, R. M. (2011). Reuse of wastewater treated effluent by lagoon for agriculture and aquaculture purposes. Aust. J. Basic. Appl. Sci. 5, 9–17.
Ellis, J. I., and Schneider, D. C. (1997). Evaluation of a gradient sampling design for environmental impact assessment. Environ. Monit. Assess. 48, 157–172. doi: 10.1023/A:1005752603707
Escobar-Dodero, J., Kinsley, A., Perez, A.M., Ibarra, R., Tello, A., Monti, G., et al. (2019). Risk factors for infectious pancreatic necrosis in farmed Chilean Atlantic salmon (Salmo salar L.) from 2010 to 2013. Prev. Vet. Med. 167, 182–189.
FAO (2018). The State of World Fisheries and Aquaculture 2018 - Meeting the Sustainable Development Goals. Rome: Food and Agriculture Organization of the United Nations
FAO (2009). Environmental Impact Assessment and Monitoring in Aquaculture: Requirements, Practices, Effectiveness and Improvements No. 527. Rome: FAO Fisheries and Aquaculture Department
FAO (2020). The State of World Fisheries and Aquaculture 2020. Sustainability in Action. Rome: Food and Agriculture Organization of the United Nations, 224. doi: 10.4060/ca9229en
Faust, E., Halvorsen, T. K., Andersen, P., Knutsen, H., and André, C. (2018). Cleaner fish escape salmon farms and hybridize with local wrasse populations. R. Soc. Open Sci. 5:171752. doi: 10.1098/rsos.171752
Felip, O., Ibarz, A., Fernández-Borràs, J., Beltrán, M., Martin-Pérez, M., Planas, J. V., et al. (2012). Tracing metabolic routes of dietary carbohydrate and protein in rainbow trout (Oncorhynchus mykiss) using stable isotopes ([13C]starch and [15N]protein): effects of gelatinisation of starches and sustained swimming. Br. J. Nutr. 107, 834–844. doi: 10.1017/S0007114511003709
Felsing, M., Telfer, T., and Glencross, B. (2006). 15N-enrichment of an aquaculture diet and tracing of cage culture waste in an estuarine environment. J. Appl. Ichthyol. 22, 419–426. doi: 10.1111/j.1439-0426.2006.00744.x
Fent, K., Weston, A. A., and Caminada, D. (2006). Ecotoxicology of human pharmaceuticals. Aquat. Toxicol. 76, 122–159.
Ferreira, C. S. G., Nunes, B. A., Henriques-Almeida, J. M. M., and Guilhermino, L. (2007). Acute toxicity of oxytetracycline and florfenicol to the microalgae Tetraselmis chuii and to the crustacean Artemia parthenogenetica. Ecotoxicol. Environ. Saf. 67, 452–458.
Ferreira, J. G., Andersen, J. H., Borja, A., Bricker, S. B., Camp, J., and Cardoso da Silva, M. (2011). Indicators of human-induced eutrophication to assess the environmental status within the european marine strategy framework directive. Estuar. Coast. Shelf Sci. 93, 117–131.
Filgueira, R., Strople, L. C., Strohmeier, T., Rastrick, S., and Strand, Ø. (2019). Mussels or tunicates: That is the question. Evaluating efficient and sustainable resource use by low-trophic species in aquaculture settings. J. Clean Prod. 231, 132–143.
Fiske, P., Lund, R.A., and Hansen, L. P. (2005). “Identifying fish farm escapees,” in Stock Identification Methods; Applications in Fishery Science, eds S.X. Cadrin, K.D. Friedland, and J.R. Waldman (Amsterdam: Elsevier), 659–680
Fitridge, I., Dempster, T., Guenther, J., and de Nys, R. (2012). The impact and control of biofouling in marine aquaculture: a review. Biofouling 28, 649–669.
Føre, M., Frank, K., Norton, T., Svendsen, E., Alfredsen, J. A., Dempster, T., et al. (2018). Precision fish farming: a new framework to improve production in aquaculture. Biosyst. Eng. 173, 176–193.
Forster, J. (2013). “A review of opportunities, technical constraints and future needs of offshore mariculture – temperate waters,” in Expanding Mariculture Farther Offshore: Technical, Environmental, Spatial and Governance Challenges, eds A. Lovatelli, J. Aguilar-Manjarrez, and D. Soto (Rome: FAO) FAO Fisheries and Aquaculture Proceedings N° 24. 77–99
Freitas, J. R. C. (2015). Desarrollo y Aplicaciones Del Cultivo De “Saccharina latissima” (Laminariales, Ochrophyta) en Sistemas de Acuicultura Multitrófica Integrada (MTI). Dissertation. Coruña: Universidade da Coruña
Galand, P. E., Lucas, S., Fagervold, S. K., Peru, E., Pruski, A. M., Vétion, G., et al. (2016). Disturbance increases microbial community diversity and production in marine sediments. Front. Microbiol. 7:1950. doi: 10.3389/fmicb.2016.01950
Gao, K., and McKinley, K. R. (1994). Use of macroalgae for marine biomass production and CO2 remediation: a review. J. Appl. Phycol. 6, 45–60. doi: 10.1007/BF02185904
Garmendia, M., Bricker, S., Revilla, M., Borja, Á., Franco, J., Bald, J., et al. (2012). Eutrophication assessment in basque estuaries: comparing a North American and a European method. Estuar. Coast. 35, 991–1006. doi: 10.1007/s12237-012-9489-8
Gentry, R. R., Lester, S. E., Kappel, C. V., White, C., Bell, T. W., Stevens, J., et al. (2016). Offshore aquaculture: spatial planning principles for sustainable development. Ecol. Evol. 7, 733–743.
Glenn, E., O’Leary, J., and Watson, M. (1991). Salicornia bigelovii Torr.: an oilseed halophyte for seawater irrigation. Science 251, 1065–1067.
Glover, K. A., Solberg, M. F., McGinnity, P., Hindar, K., Verspoor, E., Coulson, M. W., et al. (2017). Half a century of genetic interaction between farmed and wild Atlantic salmon: status of knowledge and unanswered questions. Fish. Fish. 18, 890–927.
Gómez, B., Munekata, P. E. S., Zhu, Z., Barba, F. J., Toldrá, F., Putnik, P., et al. (2019). Challenges and opportunities regarding the use of alternative protein sources: aquaculture and insects. Adv. Food Nutr. Res. 89, 259–295. doi: 10.1016/bs.afnr.2019.03.003
Gonçalves, A. A., Emerenciano, M. G. C., Ribeiro, F. A. S., and Soares Neto, J. D. R. (2019). The inclusion of fish silage in Litopenaeus vannamei diets and rearing systems (biofloc and clear-water) could affect the shrimp quality during subsequent storage on ice? Aquaculture 507, 493–499.
Gonzalez-Silvera, D., Izquierdo-Gomez, D., Fernandez-Gonzalez, V., Martínez-López, F. J., López-Jiménez, J. A., and Sanchez-Jerez, P. (2015). Mediterranean fouling communities assimilate the organic matter derived from coastal fish farms as a new trophic resource. Mar. Pollut. Bull. 91, 45–53.
Gribsholt, B., Boschker, H. T. S., Struyf, E., Andersson, M., Tramper, A., de Brabandere, L., et al. (2005). Nitrogen processing in a tidal freshwater marsh: a whole-ecosystem 15N-labeling study. Limnol. Oceanogr. 50, 1945–1959.
Grigorakis, K., and Rigos, G. (2011). Aquaculture effects on environmental and public welfare - the case of Mediterranean mariculture. Chemosphere 85, 899–919.
Guerrero, S., and Cremades, J. (2012). Acuicultura Multitrófica Integrada: Una Alternativa Sostenible y de Futuro Para los Cultivos Marinos en Galicia, ed De Galicia, X., Consellería do Medio Rural e do Mar [CIMAR]: Vilanova de Arousa
Hannah, L., Pearce, C. M., and Cross, S. F. (2013). Growth and survival of California sea cucumbers (Parastichopus californicus) cultivated with sable fish (Anoplopoma fimbria) at an integrated multi-trophic aquaculture site. Aquaculture 406–407, 34–42. doi: 10.1016/j.aquaculture.2013.04.022
Harrison, E. (1996). “Options for small-scale aquaculture development,” in Report of the Expert Consultation on Small-Scale Aquaculture, ed. M. Martínez-Espinosa (Rome: FAO) FAO Fisheries Report 548, 31–68.
Haugland, G. T., Olsen, A. B., Rønneseth, A., and Andersen, L. (2017). Lumpfish (Cyclopterus lumpus L.) develop amoebic gill disease (AGD) after experimental challenge with Paramoeba perurans and can transfer amoebae to Atlantic salmon (Salmo salar L.). Aquaculture 478, 48–55.
He, Z., Cheng, X., Kyzas, G. Z., and Fu, J. (2016). Pharmaceuticals pollution of aquaculture and its management in China. J. Mol. Liq. 223, 781–789.
Hende, S., Van Den Beelen, V., Bore, G., Boon, N., and Vervaeren, H. (2014). Bioresource technology up-scaling aquaculture wastewater treatment by microalgal bacterial flocs: from lab reactors to an outdoor raceway pond. Bioresour. Technol. 159, 342–354. doi: 10.1016/j.biortech.2014.02.113
Herbeck, L. S., Sollich, M., Unger, D., Holmer, M., and Jennerjahn, T. C. (2014). Impact of pond aquaculture effluents on seagrass performance in NE hainan, tropical China. Mar. Pollut. Bull. 85, 190–203. doi: 10.1016/j.marpolbul.2014.05.050
Herrera, J., Cornejo, P., Sepúlveda, H. H., Artar, O., and Quiñones, R. A. (2018). A novel approach to assess the hydrodynamic effects of a salmon farm in a patagonian channel: coupling between regional ocean modeling and high resolution les simulation. Aquaculture 495, 115–129. doi: 10.1016/j.aquaculture.2018.05.003
Hersoug, B. (2015). The greening of norwegian salmon production. Marit. Stud. 14:16. doi: 10.1186/s40152-015-0034-9
Hewitt, C. L., and Campbell, M. L. (2007). Mechanisms for the prevention of marine bioinvasion for better biosecurity. Mar. Pollut. Bull. 55, 395–401
Hill, M.S., and Hill, A.L. (2002). “Freshwater sponges as indicators of water pollution: an investigative undergraduate lab. Pages 385–389 in tested studies for laboratory teaching,” Proceedings of the 23rd Workshop/Conference of the Association for Biology Laboratory Education (ABLE), ed. M.A. O’Donnell, Connecticut.
Hjeltnes, B., Bang-Jensen, B., Bornø, G., Haukaas, A., and Walde, C. S. (2018). The Health Situation in Norwegian Aquaculture 2017. Norwegian Veterinary Institute, Oslo. 68–78
Holby, O., and Hall, P. O. J. (1991). Chemical fluxes and mass balances in a marine fish cage farm. II Phosphorus. Mar. Ecol. Prog. Ser. 70, 263–272. doi: 10.3354/meps070263
Holmer, M., Hansen, P.K., Karakassis, I., Borg, J. A., and Schembri, P. J. (2008). “Monitoring of environmental impacts of marine aquaculture,” in Aquaculture in the Ecosystem, eds M. Holmer, K. Black, and C.M. Duarte et al. (Dodrecht: Springer), 47–85
Holmer, M., Marba, N., Diaz-Almela, E., Duarte, C. M., Tsapakis, M., and Danovaro, R. (2007). Sedimentation of organic matter from fish farms in oligotrophic Mediterranean assessed through bulk and stable isotope (∂13C and ∂15N) analyses. Aquaculture 262, 268–280. doi: 10.1016/j.aquaculture.2006.09.033
Holmes, K. E. (2000). Effects of eutrophication on bioeroding sponge communities with the description of new West Indian sponges, Cliona spp. (Porifera: Hadromerida: Clionidae). Invertebr. Biol. 119, 125–138.
Huber, S., Hansen, L. B., Rasmussen, M. O., and Kaas, H. (2016). “Characterisation of danish waters with EO and modelling for aquaculture site selection,” in Proceedings of the Living Planet Symposium Proceedings (Noordwijk: European Space Agency) 740:262.
Ip, Y. K., and Chew, S. F. (2010). Ammonia production, excretion, toxicity, and defense in fish: a review. Front. Physiol. 1:134. doi: 10.3389/fphys.2010.00134
Izquierdo-Gómez, D., and Sanchez-Jerez, P. (2016). Management of fish escapes from Mediterranean Sea cage aquaculture through artisanal fisheries. Ocean Coast. Manage. 122, 57–63
Izquierdo-Gómez, D., Arechavala-Lopz, P., Bayle-Sempere, J. T., and Sánchez-Jerez, P. (2017). Assessing the influence of gilthead sea bream escapees in landings of Mediterranean fisheries through a scale-based methodology. Fish. Manag. Ecol. 24, 62–72.
Jahangiri, L., and Esteban, M. Á. (2018). Administration of probiotics in the water in finfish aquaculture systems: a review. Fishes 3:33.. doi: 10.3390/fishes3030033
Janssen, K., Chavanne, H., Berentsen, P., and Komen, H. (2017). Impact of selective breeding on European aquaculture. Aquaculture 472, 8–16.
Jensen, Ø., Dempster, T., Throstad, E. B., Uglem, I., and Fredheim, A. (2010). Escapes of fishes from Norwegian sea-cage aquaculture: causes, consequences and prevention. Aquacult. Environ. Interact. 171–83
Jia, B., Tang, Y., Tian, L., Franz, L., Alewell, C., and Huang, J. H. (2015). Impact of fish farming on phosphorus in reservoir sediments. Sci. Rep. 5:16617. doi: 10.1038/srep16617
Jurg, B. (1996). Towards a new generation of sediment traps and a better measurement/understanding of settling particle flux in lakes and oceans: a hydrodynamical protocol. Aquat. Sci. 58, 283–296. doi: 10.1007/BF00877472
Kalantzi, I., Zeri, C., Catsiki, V. A., Tsangaris, C., Strogyloudi, E., Kaberi, H., et al. (2016). Assessment of the use of copper alloy aquaculture nets: potential impacts on the marine environment and on the farmed fish. Aquaculture 465, 209–222.
Kalatzis, P. G., Castillo, D., Katharios, P., and Middelboe, M. (2018). Bacteriophage interactions with marine pathogenic vibrios: implications for phage therapy. Antibiotics 7:15.
Kang, C. K., Kim, J. B., Lee, K. S., Kim, J. B., Lee, P. Y., and Hong, J. S. (2003). Trophic importance of benthic microalgae to macrozoobenthos in coastal bay systems in Korea: dual stable C and N isotope analyses. Mar. Ecol. Prog. Ser. 259, 79–92. doi: 10.3354/meps259079
Kapetsky, J. M. D., and Aguilar Manjarez, J. (2013). A Global Assessment of Offshore Mariculture Potential from a Spatial Perspective. Rome: FAO. FAO Fisheries and Aquaculture Technical paper 549.
Karlsson, S., Moen, T., Lien, S., Glover, K. A., and Hindar, K. (2011). Generic genetic differences between farmed and wild Atlantic salmon identified from a 7K SNP-chip. Mol. Ecol. Reour. 11, 247–253.
Katz, T., Herut, B., Genin, A., and Angel, D. L. (2002). Gray mullets ameliorate organically enriched sediments below a fish farm in the oligotrophic Gulf of Aqaba (Red Sea). Mar. Ecol. Prog. Ser. 234, 205–214. doi: 10.3354/meps234205
Keeley, N. B., Forrest, B. M., Crawford, C., and Macleod, C. K. (2012). Exploiting salmon farm benthic enrichment gradients to evaluate the regional performance of biotic indices and environmental indicators. Ecol. Indic. 23, 453–466.
Kennedy, D. A., Kurath, G., Brito, I. L., Purcell, M. K., Read, A. F., Winton, J. R., et al. (2016). Potential drivers of virulence evolution in aquaculture. Evol. Appl. 9, 344–354.
Klinger, D., and Naylor, R. (2012). Searching for solutions in aquaculture: charting a sustainable course. Annu. Rev. Environ. Resour. 37, 247–276. doi: 10.1146/annurev-environ-021111-161531
Kroupova, H., Machova, J., and Svobodova, Z. (2005). Nitrite influence on fish: a review. Vet. Med. 50, 461–471
Kudo, N., and Fujiyama, H. (2010). Responses of halophyte salicornia bigelovii to different forms of nitrogen source. Pedosphere 20, 311–317. doi: 10.1016/S1002-0160(10)60019-7
Kuhn, D. D., Boardman, G. D., Lawrence, A.L., Marsh, L., and Flick Jr., G.J. (2009). Microbial floc meal as a replacement ingredient for fish meal and soybean protein in shrimp feed. Aquaculture, 296, 51–57. doi: 10.1016/j.aquaculture.2009.07.025
Kuhn, D. D., Lawrence, A. L., Boardman, G. D., Patnaik, S., Marsh, L., and Flick, G. J, Jr. (2010). Evaluation of two types of bioflocs derived from biological treatment of fish effluent as feed ingredients for Pacific white shrimp, Litopenaeus vannamei. Aquaculture 303, 28–33. doi: 10.1016/j.aquaculture.2010.03.001
Kumar, P. S., Kumaraswami, M., Rao, G. D., Ezhilarasan, P., Sivasankar, R., Rao, V. R., et al. (2018). Influence of nutrient fluxes on phytoplankton community and harmful algal blooms along the coastal waters of southeastern Arabian Sea. Cont. Shelf Res. 161, 20–28.
Legarda, E. C., Poli, M. A., Martins, M. A., Pereira, S. A., Martins, M. L., Machado, C., et al. (2019). Integrated recirculating aquaculture system for mullet and shrimp using biofloc technology. Aquaculture 512:734308.
Li, D., and Liu, S. (2018). Water Quality Monitoring and Management: Basis, Technology and Case Studies. London: Elsevier
Li, M., Callier, M. D., Blancheton, J. P., Galés, A., Nahon, S., Triplet, S., et al. (2019). Bioremediation of fishpond effluent and production of microalgae for an oyster farm in an innovative recirculating integrated multi-trophic aquaculture system. Aquaculture 504, 314–325.
Li, Z., Liu, J., Wang, Q., and De Silva, S. S. (2018). “Inland aquaculture: trends and prospects,” in Aquaculture in China: Success Stories and Modern Trends, eds J. Gui, Q. Tang, Z. Li, J. Liu, and S. S. De Silva (Oxford: Wiley Blackwell) 25–37. doi: 10.1002/9781119120759.ch1_2
Little, D. C., Newton, R. W., and Beveridge, C. M. (2016). Aquaculture: a rapidly growing and significant source of sustainable food? Status, transitions and potential. Proc. Nutr. Soc. 75, 274–286.
Llagostera, P. F., Kallas, Z., Reig, L., and de Gea, D. A. (2019). The use of insect meal as a sustainable feeding alternative in aquaculture: current situation, Spanish consumers’ perceptions and willingness to pay. J. Clean. Prod. 229, 10–21.
Lovatelli, A., Aguilar-Manjarrez, J., and Soto, D. (2013). Expanding Mariculture Farther Offshore. Technical, Environmental, Spatial and Governance Challenges. FAO Technical Workshop, Orbetello, Italy, 22-25 March 2010 (No. 24). Rome: FAO
Lu, J., and Li, X. (2006). Review of rice–fish– farm systems in China. One of the globally important ingenious agricultural heritage systems (GIAHS). Aquaculture 260, 106–113.
Luter, H. M., Gibb, K., and Webster, N. S. (2014). Eutrophication has no short-term effect on the Cymbastela stipitata holobiont. Front. Microbiol. 5:216. doi: 10.3389/fmicb.2014.00216
Lyngstad, T. M., Qviller, L., Sindre, H., Brun, E., and Kristoffersen, A. B. (2018). Risk factors associated with outbreaks of infectious salmon anemia (ISA) with unknown source of infection in Norway. Front. Vet. Sci. 5:308. doi: 10.3389/fvets.2018.00308
MacKay, K. T. (1983). Ecological aquaculture, new approaches to aquaculture in North America. J. World Maricult. Soc. 14, 704–713.
Macken, A., Lillicrap, A., and Langford, K. (2015). Benzoylurea pesticides used as veterinary medicines in aquaculture: risks and developmental effects on nontarget crustaceans. Environ. Toxicol. Chem. 34, 1533–1542.
MacTavish, T., Stenton-Dozey, J., Vopel, K., and Savage, C. (2012). Deposit-feeding sea cucumbers enhance mineralization and nutrient cycling in organically-enriched coastal sediments. PLoS One 7:e50031. doi: 10.1371/journal.pone.0050031
Maldonado, M., Carmona, M. C., Echeverría, Y., and Riesgo, A. (2005). The environmental impact of Mediterranean cage fish farms at semi-exposed locations: does it need a re-assessment? Helgoland Mar. Res. 59, 121–135.
Martinez-Garcia, E., Sanchez-Jerez, P., Aguado-Giménez, F., Ávila, P., Guerroro, A., Sánchez-Lizaso, J. L., et al. (2013). A meta-analysis approach to the effects of fish farming on soft bottom polychaeta assemblages in temperate regions. Mar. Pollut. Bull. 69, 165–171.
Martinez-Porchas, M., and Martinez-Cordova, L. R. (2012). World aquaculture: environmental impacts and troubleshooting alternatives. Sci. World J. 2012:389623. doi: 10.1100/2012/389623
Martinez-Porchas, M., Martinez-Cordova, L.R., Lopez-Elias, J.A., and Porchas-Cornejo, M.A. (2014). “Bioremediation of aquaculture effluents,” in Microbial Biodegradation and Bioremediation, ed. S. Das (London: Elsevier), 539–553
Maruhenda-Egea, F. C., Toledo-Guedes, K., Sanchez-Jerez, P., Ibanco-Cañete, R., Uglem, I., and Saeteher, B. S. (2015). A metabolomics approach to detect effects of Salmon farming on wild Saithe (Pollacius virens) populations. J. Agric. Food Chem. 63, 10717–10726.
Masó, M., and Garcés, E. (2006). Harmful microalgae blooms (HAB); problematic and conditions that induce them. Mar. Pollut. Bull. 53, 620–630.
Miccoli, A., Saraceni, P. R., and Scapigliati, G. (2019). Vaccines and immune protection of principal Mediterranean marine fish species. Fish Shellfish Immun. 94, 800–809.
Michaelsen-Svendsen, B. (2019). Implementation of the Traffic Light System in Norwegian Salmon Aquaculture: Success or Failure for Whom?. Master’s thesis. Norway: UiT The Arctic University of Norway
Middelburg, J. J. (2014). Stable isotopes dissect aquatic food webs from the top to the bottom. Biogeosciences 11, 2357–2371. doi: 10.5194/bg-11-2357-2014
Milhazes-Cunha, H., and Otero, A. (2017). Valorisation of aquaculture effluents with microalgae: the integrated multi-trophic aquaculture concept. Algal Res. 24, 416–424.
Moriarty, D. J. W., and Pollard, P. C. (1982). Diel variation of bacterial productivity in seagrass (Zostera capricorni) beds measured by rate of thymidine incorporation into DNA. Mar. Biol. 72, 165–173. doi: 10.1007/BF00396917
Moriarty, D. J. W., Pollard, P. C., Hunt, W. G., Moriarty, C. M., and Wassenerg, T. J. (1985). Productivity of bacteria and microalgae and the effect of grazing by holothurians in sediments on a coral reef flat. Mar. Biol. 85, 293–300. doi: 10.1007/BF00393250
Nagelkerken, I., Blaber, S. J. M., Bouillon, S., Green, P., Haywood, M., Kirton, L. G., et al. (2008). The habitat function of mangroves for terrestrial and marine fauna: a review. Aquat. Bot. 89, 155–185. doi: 10.1016/j.aquabot.2007.12.007
Nagelkerken, I., Van Der Velde, G., Gorissen, M. W., Meijer, G. J., Van’t Hof, T., and Hartog, C. (2000). Importance of mangroves, seagrass beds and the shallow coral reef as a nursery for important coral reef fishes, using a visual census technique. Estuar. Coast. Shelf. Sci. 51, 31–44. doi: 10.1006/ecss.2000.0617
Navarrete-Mier, F., Sanz-Lázaro, C., and Marín, A. (2010). Does bivalve mollusc polyculture reduce marine fin fish farming environmental impact? Aquaculture 306, 101–107. doi: 10.1016/j.aquaculture.2010.06.013
Neori, A., Chopin, T., Troell, M., Buschmann, A. H., Kraemer, G. P., Halling, C., et al. (2004). Integrated aquaculture: rationale, evolution and state of the art emphasizing seaweed biofiltration in modern mariculture. Aquaculture 231, 361–391. doi: 10.1016/j.aquaculture.2003.11.015
Neori, A., Msuya, F. E., Shauli, L., Schuenhoff, A., Kopel, F., and Shpigel, M. (2003). A novel three-stage seaweed (Ulva lactuca) biofilter design for integrated mariculture. J. Appl. Phycol. 15, 543–553. doi: 10.1023/B:JAPH.0000004382.89142.2d
Neori, A., Shpigel, M., and Ben-Ezra, D. (2000). A sustainable integrated system for culture of fish, seaweed and abalone. Aquaculture 186, 279–291. doi: 10.1016/S0044-8486(99)00378-6
Norton, T., and Berckmans, D. (2018). Engineering advances in precision livestock farming. Biosyst. Eng. 173, 1–3. doi: 10.1016/j.biosystemseng.2018.09.008
Okocha, R. C., Olatoye, I. O., and Adedeji, O. B. (2018). Food safety impacts of antimicrobial use and their residues in aquaculture. Public Health News 39:21.
Omitoyin, B. O., Ajani, E. K., Okeleye, O. L., Akpoilih, B. U., and Ogunjobi, A. A. (2017). Biological treatments of fish farm effluent and its reuse in the culture of nile tilapia (Oreochromis niloticus). J. Aquac. Res. Dev. 8:1000469.
Overton, K., Dempster, T., Oppedal, F., Kristiansen, T. S., Gismervik, K., and Stien, L. H. (2018). Salmon lice treatments and salmon mortality in Norwegian aquaculture: a review. Rev. Aquacult. 11, 1398–1417.
Ozbay, G., Blank, G., and Thunjai, T. (2014). “Impacts of aquaculture on habitats and best management practices (BMPs),” in Sustainable Aquaculture Techniques, eds M.P. Hernandez- Vergara and C.I. Perez-Rostro (London: IntechOpen), 67. doi: 10.5772/57471
Pahlow, M., van Oel, P. R., Mekonnen, M. M., and Hoekstra, A. Y. (2015). Increasing pressure on freshwater resources due to terrestrial feed ingredients for aquaculture production. Sci. Total. Environ. 536, 847–857.
Pajand, Z. O., Soltani, M., Bahmani, M., and Kamali, A. (2017). The role of polychaete Nereis diversicolor in bioremediation of wastewater and its growth performance and fatty acid composition in an integrated culture system with Huso (Linnaeus, 1758). Aquac. Res. 48, 5271–5279. doi: 10.1111/are.13340
Palmer, P. J. (2010). Polychaete-assisted sand filters. Aquaculture 306, 369–377. doi: 10.1016/j.aquaculture.2010.06.011
Partelow, S., Senff, P., Buhari, N., and Schlüter, A. (2018). Operationalizing the social-ecological systems framework in pond aquaculture. Int. J. Commons 12, 485–518.
Pelletier, D., Tyedmers, P., Sonesson, U., Scholz, A., Ziegler, F., Flysjo, A., et al. (2009). Not all salmon are created equal: life cycle assessment (LCA) of global salmon farming systems. Envirn. Sci. Technol. 43, 8730–8736.
Peterson, B. J., and Fry, B. (1987). Stable isotopes in ecosystem studies. Annu. Rev. Ecol. Syst. 18, 293–320.
Plaza, N., Castillo, D., Pérez-Reytor, D., Higuera, G., García, K., and Bastías R. (2018). Bacteriophages in the control of pathogenic vibrios. Electron. J. Biotechn. 31, 24–33.
Pomeroy, R. S. (2012). Managing overcapacity in small-scale fisheries in Southeast Asia. Mar. Policy. 36, 520–527. doi: 10.1016/j.marpol.2011.10.002
Porporato, E. M.D., Pastres, R., and Brigolin, D. (2020). Site suitability for finfish marine aquaculture in Central Mediterranean Sea. Front. Mar. Sci. 6:772. doi: 10.3389/fmars.2019.00772
Porrello, S., Lenzi, M., Persia, E., Tomassetti, P., and Finoia, M. G. (2003). Reduction of aquaculture wastewater eutrophication by phytotreatment ponds system I. Dissolved and particulate nitrogen and phosphorus. Aquaculture 219, 515–529.
Price, C. S., and Morris, J. A. (2013). Marine Cage Culture and the Environment: Twenty-first Century Science Informing a Sustainable Industry. NOAA Technical Memorandum NOS NCCOS, Washington, DC: NOAA. 164
Price, C., Black, D. K., Hargrave, B. T., and Morris Jr., J. A. (2015). Marine cage culture and the environment: Effects on water quality and primary production. Aquacul. Environ. Int. 6, 151–174.
Primavera, J. H. (2005). Mangroves, fishponds, and the quest for sustainability. Science 310, 57–59.
Purcell, E. J., Uye, S. -I., and Wen-Tseng, L. (2007). Anthropogenic causes of jellyfish blooms and their direct consequences for humans: a review. Mar. Ecol. Prog. Ser. 350, 153–174.
Qi, Z., Shi, R., Yu, Z., Han, T., Li, C., Xu, S., et al. (2019). Nutrient reléase from fish cage aquaculture and mitigation strategies in Daya Bay, southern China. Mar. Poll. Bull. 146, 399–407.
Radford, C., and Slater, M. (2019). Soundscapes in aquaculture systems. Aquacult. Environ. Int. 11, 53–62.
Ramos, S., Cidad, M., and Aguilera, C. (2019). Evaluación del Impacto Ambiental de la Producción de Dorada y Lubina en el Mediterráneo. XVII Congreso Nacional de Acuicultura. IRTA: Cartagena, 346–347
Reid, G. K., Liutkus, M., Bennett, A., Robinson, S. M. C., MacDonald, B., and Page, F. (2010). Absorption efficiency of blue mussels (Mytilus edulis and M. trossulus) feeding on Atlantic salmon (Salmo salar) feed and fecal particulates: implications for integrated multi-trophic aquaculture. Aquaculture 299, 165–169. doi: 10.1016/j.aquaculture.2009.12.002
Resende, P. C., Resende, P., Pardal, M., Almeida, S., and Azeiteiro, U. (2010). Use of biological indicators to assess water quality of the Ul River (Portugal). Environ. Monit. Assess. 170, 535–544. doi: 10.1007/s10661-009-1255-4
Rhodes, L. D., Johnson, R. B., and Myers, M. S. (2016). Effects of alternative plant-based feeds on hepatic and gastrointestinal histology and the gastrointestinal microbiome of sablefish (Anoplopoma fimbria). Aquaculture 464, 683–691.
Rice, J., Arvanitidis, C., Borja, A., Frid, C., Hiddinik, J. G., Krause, J., et al. (2012). Indicators for sea-floor integrity under the European marine strategy framework directive. Ecol. Ind. 12, 174–184.
Rico, A., Vighi, M., Van den Brink, P. J., Horst, M., Macken, A., Lillicrap, A., et al. (2018). Use of models for the environmental risk assessment of veterinary medicines in European aquaculture: current situation and future perspectives. Rev. Aquacult. 11, 969–988.:Google Scholar
Riera, R., Tuset, V., Rodríguez, M., Monterroso, Ó., and Lombarte, A. (2017). Analyzing functional diversity to determine the effects of fish cages in insular coastal wild fish assemblages. Aquaculture 479, 384–395.
Robinson, G., Caldwell, G. S., Jones, C. L. W., Slater, M. J., and Stead, S. M. (2015). Redox stratification drives enhanced growth in a deposit-feeding invertebrate: implications for aquaculture bioremediation. Aquac. Environ. Interact. 8, 1–13. doi: 10.3354/aei00158
Robinson, G., Caldwell, G. S., Jones, C. L., and Stead, S. M. (2019). The effect of resource quality on the growth of Holothuria scabra during aquaculture waste bioremediation. Aquaculture 499, 101–108.
Rubio-Portillo, E., Villamor, A., Fernandez-Gonzalez, V., Antón, J., and Sanchez-Jerez, P. (2019). Exploring changes in bacterial communities to assess the influence of fish farming on marine sediments. Aquaculture 506, 459–464.
Samuel-Fitwi, B., Wuertz, S., Schroeder, J. P., and Schulz, C. (2012). Sustainability assessment tools to support aquaculture development. J. Clean. Prod. 32, 183–192.
Sanchez-Jerez, P., Fernandez-Jover, D., Uglem, I., Arechavala-Lopez, P., Dempster, T., Bayle-Sempere, J.T., et al. (2011). “Coastal fish farms as fish aggregation devices (FADs),” in Artificial Reefs in Fisheries Management, eds S.A. Bortone, P. Brandini, and G. Fabi et al. (Boca Raton, FL: CRC Press), 187–208
Sarà, G. (2007). A meta-analysis on the ecological effects of aquaculture on the water column: dissolved nutrients. Mar. Environ. Res. 63, 390–408.
Sarà, G., Zenone, A., and Tomasello, A. (2009). Growth of Mytilus galloprovincialis (Mollusca, Bivalvia) close to fish farms: a case of integrated multi-trophic aquaculture within the Tyrrhenian Sea. Hydrobiologia 636, 129–136. doi: 10.1007/s10750-009-9942-2
Sarker, P. K, Kapuscinski, A. R., McKuin, B., Fitzgerald, D. S., Nash, H. M., and Greenwood, C. (2020). Microalgae-blend tilapia feed eliminates fishmeal and fish oil, improves growth, and is cost viable. Sci. Rep. 10:19328.
Scheu, S., Steidel, J. L. M., Brose, U., and Kronberg, I. (2011). “Ökologie der individuen: umweltbedingungen, ressourcen, nischen und verbreitungsgebiet,” in TLB Biologie: Ökologie, Biodiversität, Evolution, ed. K. Munk (Stuttgart: Thieme)
Senff, P., Partelow, S., Indriana, L. F. Buhari, N., and Partelow, S. (2018). Improving pond aquaculture production on Lombok, Indonesia. Aquaculture 497, 64–73. doi: 10.1016/j.aquaculture.2018.07.027
Sherman, A. D., Hobson, B. W., McGill, P. R., Davis, R. E., McClune, M. C., and Smith, Jr., K.L. (2011). Lagrangian sediment traps for sampling at discrete depths beneath free-drifting icebergs. Deep Sea Res. II Top. Stud. Oceanogr. 58, 1327–1335. doi: 10.1016/j.dsr2.2010.11.008
Shetty, S., Pai, R. M., and Pai, M. M. M. (2018). Design and implementation of aquaculture resource planning using underwater sensor wireless network. Cogent. Eng. 5:1542576. doi: 10.1080/23311916.2018.1542576
Shpigel, M., Ben-Ezra, D., Shauli, L., Sagi, M., Ventura, Y., Samocha, T., et al. (2013). Constructed wetland with Salicornia as a biofilter for mariculture effluents. Aquaculture 413, 52–63. doi: 10.1016/j.aquaculture.2013.06.038
Shpigel, M., Neori, A., Popper, D. M., and Gordin, H. (1993). A proposed model for “environmentally clean” land-based culture of fish, bivalves and seaweeds. Aquaculture 117, 115–128. doi: 10.1016/0044-8486(93)90128-L
Silva, C., Barbieri, M. A., Yánez, E., Gutiérrez-Estrada, J. C., and DelValls, T. A. (2012). Using indicators and models for an ecosystem approach to fisheries and aquaculture management: anchovy fishery and pacific oyster culture- cases studies in Chile. Lat. Am. J. Aquat. Res. 40, 955–969.
Simbeye, D. S., Zhao, J., and Yang, S. (2014). Design and deployment of wireless sensor networks for aquaculture monitoring and control based on virtual instruments. Comput. Electron. Agr. 102, 31–42.
Singh, D., Buhmann, A. K., Flowers, T. J., Seal, C. E., and Paperbrock, J. (2014). Salicornia as a crop plant in temperate regions: selection of genetically characterized ecotypes and optimization of their cultivation conditions. AoB Plants 6:lu071. doi: 10.1093/aobpla/plu071
Slater, M. J., Jeffs, A. G., and Sewell, M. A. (2011). Organically selective movement and deposit-feeding in juvenile sea cucumber, Australostichopus mollis determined in situ and in the laboratory. J. Exp. Mar. Bio. Ecol. 409, 315–323. doi: 10.1016/j.jembe.2011.09.010
Soto, D., and Norambuena, F. (2004). Evaluation of salmon farming effects on marine systems in the inner seas of southern Chile: a large-scale mensurative experiment. J. Appl. Ichthyol. 20, 493–501. doi: 10.1111/j.1439-0426.2004.00602.x
Stelzenmüller, V., Gimpel, A., Gopnik, M., and Gee, K. (2017). “Aquaculture site-selection and marine spatial planning: the roles of GIS-based tools and models,” in Aquaculture Perspective of Multi-Use Sites in the Open Ocean, eds B. Buck and R. Langan (Cham: Springer), 131–148
Stien, L. H., Lind, M. B., Oppedal, F., Wright, D. W., and Seternes, T. (2018). Skirts on salmon production cages reduced salmon lice infestations without affecting fish welfare. Aquaculture 490, 281–287.
Sutherland, T. F., Sterling, A. M., and Ou, M. (2018). Influence of salmonid aquaculture activities on a rock-cliff epifaunal community in jervis inlet, British columbia. Mar. Pollut. Bull. 127, 297–309. doi: 10.1016/j.marpolbul.2017.12.005
Telfer, T., and Beveridge, M. C. M. (2001). “Monitoring environmental effects of marine fish aquaculture,” in Environmental Impact Assessment of Mediterranean Aquaculture Farms, Cahiers Options Méditerranéennes, 55. eds A. Uriarte and B. Basurco (Zaragoza: CIHEA), 75–83.
Thia-Eng, C. (1997). “Sustainable aquaculture and integrated coastal management,” in Sustainable Aquaculture, ed. J.E. Bardach (New York, NY: Wiley), 177–200
Thomas, S., and Ridd, P. (2005). Field assessment of innovative sensor for monitoring of sediment accumulation at inshore coral reefs. Mar. Pollut. Bull. 51, 470–480. doi: 10.1016/j.marpolbul.2004.10.026
Thorstad, E. B., Fleming, I. A., McGinnity, P., Soto, D., Wennevik, V., and Whoriskey, F. (2008). Incidence and impacts of escaped farmed Atlantic salmon Salmo salar in nature: Report from the Technical Working Group on Escapes of the Atlantic Salmon Aquaculture Dialog. Available online at: http://www.fao.org/3/aj272e/aj272e00.pdf (accessed March 2020).
Toledo-Guedes, K., Sanchez-Jerez, P., and Brito, A. (2014). Influence of a massive aquaculture escape event on artisanal fisheries. Fish. Manag. Ecol. 21, 113–121.
Toranzo, A. E., Magariños, B., and Romalde, J. L. (2005). A review of the main bacterial fish diseases in mariculture systems. Aquaculture 246, 37–61.
Tornero, V., and Hanke, G. (2016). Chemical contaminants entering the marine environment from sea-based sources: a review with a focus on European seas. Mar. Pollut. Bull. 112, 17–38.
Troell, M., Joyce, A., Chopin, T., Neori, A., Buschmann, A. H., and Fang, J. G. (2009). Ecological engineering in aquaculture - potential for integrated multi-trophic aquaculture (IMTA) in marine offshore systems. Aquaculture 297, 1–9. doi: 10.1016/j.aquaculture.2009.09.010
Turk, V., Lučić, D., Flander Putrle, V., and Malej, A. L. (2008). Feeding of Aurelia sp. (Scyphozoa) and links to the microbial food web. Mar. Ecol. 29, 495–505. doi: 10.1111/j.1439-0485.2008.00250.x
Underwood, A. J. (1993). The mechanics of spatially replicated sampling programmes to detect environmental impacts in a variable world. Aust. J. Ecol. 18, 99–116.
Urbina, M. A. (2016). Temporal variation on environmental variables and pollution indicators in marine sediments under sea Salmon farming cages in protected and exposed zones in the Chilean inland Southern Sea. Sci. Total Environ. 573, 841–853.
Uthicke, S. (1999). Sediment bioturbation and impact of feeding activity of Holothuria (Halodeima) atra and Stichopus chloronotus, two sediment feeding holothurians, at Lizard Island, great barrier reef. Bull. Mar. Sci. 64, 129–141.
Valiela, I., Bowen, J. L., and York, J. K. (2001). Mangrove forests: one of the world’s threatened major tropical environments. Bioscience 51, 807–815.
Vandermeulen, H., and Gordin, H. (1990). Ammonium uptake using Ulva (Chlorophyta) in intensive fishpond systems: mass culture and treatment of effluent. J. Appl. Phycol. 2, 363–374. doi: 10.1007/BF02180927
Verdal, H., Mekkawy, W., Lind, C. E., Vandeputtede, M., Chataine, B., and Benziebf, J. A. H. (2017). Measuring individual feed efficiency and its correlations with performance traits in Nile tilapia, Oreochromis niloticus. Aquaculture 468, 489–495.
Verhoeven, J. T. A., and Meuleman, A. F. M. (1999). Wetlands for wastewater treatment: opportunities and limitations. Ecol. Eng. 12, 5–12.
Verhoeven, J. T. P., Salvo, F., Hamoutene, D., and Dufour, S. C. (2016). Bacterial community composition of flocculent matter under a salmonid aquaculture site in Newfoundland, Canada. Aquacult. Environ. Interact. 8, 637–646. doi: 10.3354/aei00204
Viana, I. G., Bode, A., Bartholomew, M., and Valiela, I. (2015). Experimental assessment of the macroalgae Ascophyllum nodosum and Fucus vesiculosus for monitoring N sources at different time-scales using stable isotope composition. J. Exp. Mar. Bio. Ecol. 466, 24–33. doi: 10.1016/j.jembe.2015.01.014
Vita, R., Marín, A., Madrid, J. A., Jiménez-Brinquis, B., Cesar, A., and Marín-Guirao, L. (2004). Effects of wild fishes on waste exportation from a Mediterranean fish farm. Mar. Ecol. Prog. Ser. 277, 253–261.
Vymazal, J. (2010). Constructed wetlands for wastewater treatment. Water 2, 530–549. doi: 10.3390/w2030530
Waller, U., Buhmann, A. K., Ernst, A. Hanke, V., Kulakowski, A., Wecker, B., et al. (2015). Integrated multi-trophic aquaculture in a zero-exchange recirculation aquaculture system for marine fish and hydroponic halophyte production. Aquac. Int. 23, 1473–1489. doi: 10.1007/s10499-015-9898-3
Wang, B., Xin, M., Wei, Q., and Xie, L. (2018). A historical overview of coastal eutrophication in the China Seas. Mar. Pollut. Bull. 136, 394–400.
Wang, F., and Chapman, P. M. (1999). Biological implications of sulfide in sediment—a review focusing on sediment toxicity. Environ. Toxicol. Chem. 18, 2526–2532. doi: 10.1002/etc.5620181120
Wang, X., Olsen, L. M., Reitan, K. I., and Olsen, Y. (2012). Discharge of nutrient wastes from salmon farms: environmental effects, and potential for integrated multi-trophic aquaculture. Aquacult. Environ. Interact. 2, 267–283. doi: 10.3354/aei00044
Wang, Y., Gu, X., Zeng, Q., Mao, Z., Gu, X., and Li, X. (2015). Fate of 15N-enriched cyanobacteria feed for planktivorous fish in an enclosure experiment: a stable isotope tracer study. Fish. Sci. 81, 821–830. doi: 10.1007/s12562-015-0897-0
Wang, Z., Chen, Y., Zhang, S., Wang, K., Zhao, J., and Xu, Q. (2015). A comparative study of fish assemblages near aquaculture, artificial and natural habitats. J. Ocean Univ. China 14, 149–160. doi: 10.1007/s11802-015-2455-x
Watai, M., Nakamura, Y., Honda, K., Bolisay, K. O., Miyajima, T., and Nakaoka, M. et al. (2015). Diet, growth, and abundance of two seagrass bed fishes along a pollution gradient caused by milkfish farming in Bolinao, northwestern Philippines. Fish. Sci. 81, 43–51. doi: 10.1007/s12562-014-0824-9
Watts, J. E. M., Schreier, H. J., Lanska, L., and Hale, M. S. (2017). The rising tide of antimicrobial resistance in aquaculture: sources, sinks and solutions. Mar. Drugs. 15:158. doi: 10.3390/md15060158
Wei, Z., You, J., Wu, H., Yang, F., Long, L., Liu, Q., et al. (2017). Bioremediation using Gracilaria lemaneiformis to manage the nitrogen and phosphorous balance in an integrated multi-trophic aquaculture system in Yantian Bay, China. Mar. Pollut. Bull. 121, 313–319.
Weitzman, J. (2019). Applying the ecosystem services concept to aquaculture: a review of approaches, definitions, and uses. Ecosyst. Serv. 35, 194–206. doi: 10.1016/j.ecoser.2018.12.009
Weitzman, J., and Filgueira, R. (2019). The Evolution and Application of Carrying Capacity in Aquaculture: Towards a Research Agenda. Hoboken, NJ: Wiley. doi: 10.1111/raq.12383.
Whinney, J. C., Jones, R., Duckworth, A., and Ridd, P. (2017). Continuous in situ monitoring of sediment deposition in shallow benthic environments. Coral Reefs 36, 521–533. doi: 10.1007/s00338-016-1536-7
White, A. T., Bannister, R. J., Dworjanyn, S. A., Husa, V., Nichols, P. D., Kutti, T., et al. (2017). Consumption of aquaculture waste affects the fatty acid metabolism of a benthic invertebrate. Sci. Total Environ. 586, 1170–1181.
White, A. T., Christie, P., D’Agnes, H., Lowry, K., and Milne, N. (2005). Designing ICM projects for sustainability: lessons from the Philippines and Indonesia. Ocean. Coast. Manag. 48, 271–296. doi: 10.1016/j.ocecoaman.2005.04.007
White, C. A., Woodcock, S. H., Bannister, R. J., and Nichols, P. D. (2019). Terrestrial fatty acids as tracers of finfish aquaculture waste in the marine environment. Rev. Aquacult. 11, 133–148.
Whitmarsh, D. J., Cook, E. J., and Black, K. D. (2006). Searching for sustainability in aquaculture: an investigation into the economic prospects for an integrated salmon-mussel production system. Mar. Policy 30, 293–298. doi: 10.1016/j.marpol.2005.01.004
Wilding, T. A. (2011). A characterization and sensitivity analysis of the benthic biotopes around Scottish salmon farms with a focus on the sea pen Pennatula phosphorea L. Aquac. Res. 42, 35–40.
Wilson, A., Magill, S., and Black, K. D. (2009). Review of Environmental Impact Assessment and Monitoring in Salmon Aquaculture, in Environmental Impact Assessment and Monitoring in Aquaculture. FAO Fisheries and Aquaculture Technical Paper No. 527 (Rome: FAO), 455–535
Winter, L., Lehmann, A., Finogenova, N., and Finkbeiner, M. (2017). Including biodiversity in life cycle assessment – state of the art, gaps and research needs. Environ. Impact Assess. Rev. 67, 88–100.
Woods, J. S., Veltman, K., Huijbregts, M. A. J., Verones, F., and Hertwich, E. G. (2016). Towards a meaningful assessment of ecological impacts in life cycle assessment (LCA). Env. Int. 89-90, 48–61.
Yáñez, J. M., Houston, R. D., and Newman, S. (2014). Genetics and genomics of disease resistance in salmonid species. Front. Genet. 5:415. doi: 10.3389/fgene.2014.00415
Yuerlita, Perret, S. R., and Shivakoti, G. P. (2013). Fishing farmers or farming fishers? Fishing typology of inland small-scale fishing households and fisheries management in Singkarak Lake, West Sumatra, Indonesia. Environ. Managem. 52, 85–98. doi: 10.1007/s00267-013-0050-8
Yunus, B., Dirawan, G. D., and Saru, A. (2015). The behavior of fishpond farmers with silvofishery insight and its effects on biodiversity of macrozoobenthos in mangrove ecosystem of coastal area. Int. J. Agric. Syst. 3, 65–77.
Keywords: IMTA, finfish farms, environmental pollution, environmental assessement, eutrophication
Citation: Carballeira Braña CB, Cerbule K, Senff P and Stolz IK (2021) Towards Environmental Sustainability in Marine Finfish Aquaculture. Front. Mar. Sci. 8:666662. doi: 10.3389/fmars.2021.666662
Received: 10 February 2021; Accepted: 17 March 2021;
Published: 21 April 2021.
Edited by:
Viola Liebich, Bremen Society for Natural Sciences, GermanyReviewed by:
Christina Hoerterer, Alfred Wegener Institute Helmholtz Centre for Polar and Marine Research (AWI), GermanyCopyright © 2021 Carballeira Braña, Cerbule, Senff and Stolz. This is an open-access article distributed under the terms of the Creative Commons Attribution License (CC BY). The use, distribution or reproduction in other forums is permitted, provided the original author(s) and the copyright owner(s) are credited and that the original publication in this journal is cited, in accordance with accepted academic practice. No use, distribution or reproduction is permitted which does not comply with these terms.
*Correspondence: Carlos Brais Carballeira Braña, Y2FybG9zLmNhcmJhbGxlaXJhQHB1Y3YuY2w=
Disclaimer: All claims expressed in this article are solely those of the authors and do not necessarily represent those of their affiliated organizations, or those of the publisher, the editors and the reviewers. Any product that may be evaluated in this article or claim that may be made by its manufacturer is not guaranteed or endorsed by the publisher.
Research integrity at Frontiers
Learn more about the work of our research integrity team to safeguard the quality of each article we publish.