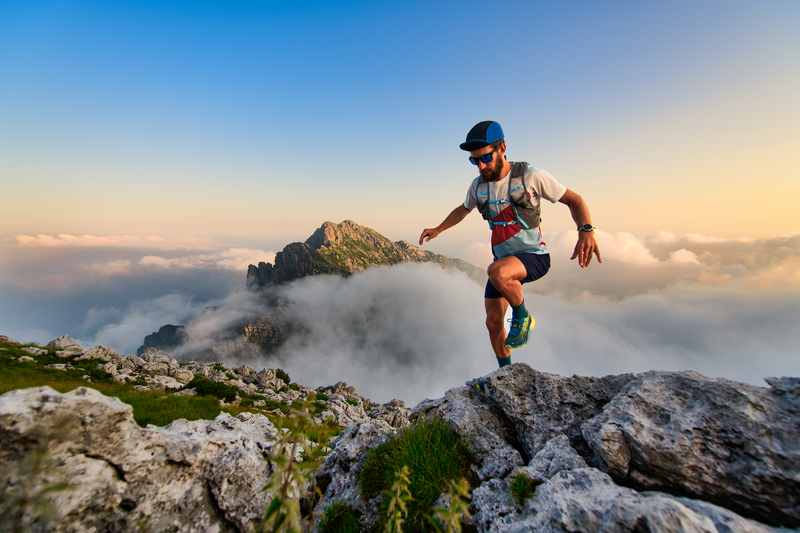
95% of researchers rate our articles as excellent or good
Learn more about the work of our research integrity team to safeguard the quality of each article we publish.
Find out more
ORIGINAL RESEARCH article
Front. Mar. Sci. , 31 May 2021
Sec. Marine Fisheries, Aquaculture and Living Resources
Volume 8 - 2021 | https://doi.org/10.3389/fmars.2021.664701
This article is part of the Research Topic Understanding the Interplay Between Diet, Feed Ingredients and Gut Microbiota for Sustainable Aquaculture View all 11 articles
A 121-day feeding trial was undertaken to test the effects of two dietary lipid levels (16 and 21% L16, L21) in triplicated gilthead sea bream groups (initial weight: 67.5 g) reared at two different water temperatures (high, H 23°C and low, L 17°C) in the same recirculation system but exposed to a switch in temperature after 58 days. Fish kept at H were transferred to L (HL transition, autumn shift), and the fish kept at L were exposed to H (LH transition, summer shift), while continuing to receive the same diet to apparent satiation in each group. At the end of the trial, no significant diet effect on specific growth rate (SGR), feed intake (FI), and feed conversion rate (FCR) were detected in fish exposed to HL transition compared with those exposed to LH transition, while gross lipid efficiency (GLE) and lipid efficiency ratio (LER) were higher in L16. After temperature changes, L16 displayed higher SGR, FI, GLE, and LER, while mesenteric fat index was reduced. After temperature changes, the combined effects of low lipid diet and low temperature conditions resulted in higher pepsin activity, while trypsin, chymotrypsin, and lipase activities were generally higher at high lipid content. The combined effect of diet and temperature did not alter the metabolic plasma profile, except for the observed final higher aspartate aminotransferase (AST) and alkaline phosphatase (ALP) values when combining high dietary lipid (L21) and temperature changes. Different diets showed a significantly different gut microbiome layout, only at high temperature with L16 diet resulting in a higher load of Lactobacillus. On the contrary, no dietary impact on ecosystem diversity was observed, independently from the temperature. In addition, L16 diet in the HL transition favored an increase in Weissella and Bradyrhizobium genera in the gut microbiome, while in the final condition of LH transition, L21 diet favored a significant increase in Streptococcus and Bacillus. According to the results, the utilization of 16% dietary lipid levels in gilthead sea bream should be preferred during seasonal temperature changes in order to optimize feed utilization and gut health.
Today, feeding strategy optimization related to environmental conditions is extremely necessary to pursue more intensive and more efficient aquaculture production in the Mediterranean basin. Feed production is the greatest cost in the aquaculture sector and can account for up to 60–80% of the overall expenses (Hasan et al., 2007; Luna et al., 2019). Dietary lipid supplementation has been largely developed to reduce feed cost and reduce the need for limited and expensive protein ingredients in many farmed fish species (Leaver et al., 2008; Bell and Koppe, 2010; Bonaldo et al., 2010) including gilthead sea bream (Sparus aurata), which is one of the most important marine finfish species farmed in the Mediterranean area (Guillen, 2019). Currently, commercial diet composition for the grow-out phase of this species consists, on average, of 43% protein and 20% fat (Koven, 2002; Vasconi et al., 2017; Arantzamendi et al., 2019). Among abiotic factors, water temperature is the key environmental factor, playing a crucial role on metabolism, nutrient utilization, fat deposition and welfare, in particular for this species which is highly susceptible to thermal seasonal changes and fluctuation (Ibarz et al., 2010; Sánchez-Nuño et al., 2018a). Although it is known that the optimal temperature range is between 18 and 26°C (Davis, 1988; Jobling and Peruzzi, 2010), this species is yearly subjected to large temperature fluctuations (from 11 to 26°C) in most farming conditions. Previous works found that increasing dietary lipids from 16 to 24% produced no significant differences in final body weight and specific growth rate at summer temperatures between 24 and 27°C (Velázquez et al., 2006; Bonaldo et al., 2010; Mongile et al., 2014). On the other hand, several studies have also been devoted to developing winter feeds for overcoming metabolic alterations, immune suppression, and nutritional disorders (Silva et al., 2014; Richard et al., 2016; Schrama et al., 2017). While most of these diets were formulated in order to test the effectiveness of functional ingredients such as immunostimulants and antioxidants, their lipid content ranged from 17 to 19.7%. In addition, especially at temperatures below 13°C, if there is an excess in dietary lipid, it can be accumulated as a fat depot in perivisceral tissue due to low metabolic activity (Ibarz et al., 2007, 2010).
To the best of our knowledge, few studies have been carried out to assess optimal lipid composition during water temperature changes. Sánchez-Nuño et al. (2018a) found that dietary lipid content 18% vs. 14% did not affect growth in fish subjected to temperature fluctuations from 22 to 14°C; however, the authors suggested adopting lower lipid levels to avoid excessive fat deposition and putative oxidative stress during recovery. Environmental temperature fluctuation during seasonal changes may also affect fish metabolism, digestive enzyme activity, and gut bacterial community, which may influence performance, tissue composition, and fish health (Couto et al., 2012; García-Meilán et al., 2013; Guerreiro et al., 2016; Zarkasi et al., 2016; Sepulveda and Moeller, 2020). To date, very limited studies have investigated how changes in water temperature interact with diet in shaping the gut microbiome structure in teleost species, and none of them deals with sea bream. The aim of the present study was to explore the effects of dietary lipid level and temperature switch on growth, digestive enzyme activity, plasma biochemistry, and gut microbiome structure during the on-growing of gilthead sea bream.
Ingredients and proximate composition of the experimental diets are represented in Table 1. Two isonitrogenous (43.7%) extruded diets (sinking pellet size diameter 4.00 mm) were produced to contain a low 16% (L16) and high 21% (L21) dietary lipid level. Diets were formulated with fish meal and with a mixture of vegetable ingredients currently used for sea bream in aquafeed (Parma et al., 2016). Diets were produced by Sparos Lda (Olhão, Portugal).
Before the beginning of the trial (October), fish were adapted to the laboratory facilities at the constant water rearing temperature of 20°C for 10 days. At the beginning of the trial, triplicate tanks were randomly divided into two groups: one at high (H) temperature 23.17 ± 1.11°C and one at low (L) temperature 17.34 ± 0.92°C, respectively, and maintained at these constant temperatures for 58 days. In the RAS, water temperature was maintained warmer in six tanks by a heater (H03609-00.B-2012/01, Zodiac Pool Care, Saint-Barthélemy-d’Anjou, France), while the water in the remaining tanks was kept cooler (AWP 16 SP R407C, GENCOLD S.r.l., Cesena, Italy) for the whole experiment.
On day 58, fish were exposed to a switch in temperature [fish kept at H were transferred to L, HL, and the fish kept at L were transferred to H (LH)], while continuing to receive the same diet in each group. The thermal variation of 6°C occurred at a rate of 3°C day–1. Thus, fish that were brought from 23°C to 17°C (HL) were subjected to summer–autumn temperature variation, while fish brought from 17°C to 23°C (LH) underwent spring–summer temperature changes. In order to exclude the effect of light regime on performance, photoperiod was maintained constant at 12-h light and 12-h dark through artificial light (light intensity on the water surface 400 lx).
The experiment was carried out at the Laboratory of Aquaculture, Department of Veterinary Medical Sciences of the University of Bologna, Cesenatico, Italy. Gilthead sea bream juveniles were obtained from Panittica Pugliese (Torre Canne di Fasano, Brindisi, Italy). At the beginning of the trial, 30 fish (initial average weight: 67.50 ± 1.66 g) per tank were randomly distributed into 12 450-L square tanks. Experimental diets (L16 and L21) were administered to triplicate groups to visual satiation twice a day (h 8.30 and h 16.00) for 6 days a week. While temperatures were switched after intermediate day sampling, each tank continued to receive the same dietary treatment until the end of the trial. Tanks were provided with natural seawater and connected to a closed recirculating aquaculture system (RAS) with an overall water volume capacity of 6,000 L. The rearing system consisted of a mechanical sand filter (0.4 m3 of silica sand, 0.4–0.8 mm. PTK 1200, Astral Pool, Servaqua S.A. Barsareny, Spain), ultraviolet lights (SH-63, BLUGEO S.r.l., Parma, Italy), and a biofilter (PTK 1200, Astral Pool, Servaqua S.A. Barsareny, Spain). The oxygen level was kept constant (8.0 ± 1.0 mg L–1) by a liquid oxygen system regulated by a software program (B&G Sinergia snc, Chioggia, Italy). Ammonia (total ammonia nitrogen, TAN ≤ 0.1 mg L–1), nitrite (NO2 ≤ 0.2 mg L–1), nitrate (NO3 ≤ 50 mg L–1), and salinity (25–30 g L–1) were daily monitored spectrophotometrically (Spectroquant Nova 60, Merck, Lab business, Darmstadt, Germany). Sodium bicarbonate was added daily to keep pH constant at 7.8–8.0. The feeding trial lasted for a total of 121 days.
The samples size for each analysis are reported in Supplementary Table 1. At the beginning, just before thermal change (58 days), and at the end of the experiment, all the fish in each tank were anesthetized by tricaine methanesulfonate at 100 mg L–1 and individually weighed. The proximate composition of the carcasses was determined on pooled samples at the beginning (10 fish per tank), before the temperature switch (three fish per tank), and at the end of the trial (five fish per tank).
Furthermore, wet weight of the viscera, liver, and perivisceral fat were individually recorded for intermediate (six fish per tank) and final (five fish per tank) pools to determine viscerosomatic index (VSI), hepatosomatic index (HSI), and mesenteric fat index (MFI). Moreover, liver pooled samples (from six individuals per tank) were taken out at the end of the trial and stored at −20°C until analyzed to access the fat liver content in animals subjected to temperature switch. At 5 h post meal (hpm), three fish per tank (n = 9/treatment) on day 58 (before temperature changes) and five fish per tank (n = 15/treatment) on day 121 were sampled and dissected to obtain their whole gastrointestinal tract; then they were first stored at −80°C and subsequently freeze dried until digestive enzyme activity analysis according to Busti et al. (2020a).
Digesta content (n = 3 fish per tank on intermediate sampling day 58, n = 9 fish per diet treatment; n = 3 fish per tank on final sampling day 121st, n = 9 fish per diet treatment) from posterior intestine was also individually sampled and immediately stored at −80°C for gut microbiota investigation according to Parma et al. (2016).
Blood was collected from the caudal vein in the three fish per tank on intermediate sampling (n = 9 fish per treatment) and in the five fish per tank (n = 15 fish per treatment) on the final sampling. Samples were then centrifuged (3,000 × g for 10 min at 4°C), and plasma aliquots were stored at −80°C until analysis according to Bonvini et al. (2018a).
All experimental procedures were evaluated and approved by the Ethical–Scientific Committee for Animal Experimentation of the University of Bologna, in accordance with European directive 2010/63/UE on the protection of animals used for scientific purposes (protocol ID 942/2019).
The formulae employed to calculate growth performance, somatic indices, nutritional indices, and relative variations were used according to Bonvini et al. (2018b) and Parma et al. (2020) as follows:
Specific growth rate (SGR) (% day–1) = 100 ∗ (ln FBW - ln IBW)/days (where FBW and IBW represent the final and the initial body weights).
FI = Feed intake (% ABW–1 day–1) = [(100∗total ingestion)/(ABW)/days].
Feed conversion ratio (FCR) = feed intake/weight gain.
Viscerosomatic index (VSI) (%) = 100 ∗ (viscera weight/body weight).
Hepatosomatic index (HSI) (%) = 100 ∗ (liver weight/body weight).
Mesenteric fat index (MFI) (%) = 100 ∗ (mesenteric fat weight/body weight).
Protein efficiency rate (PER) = (FBW – IBW)/protein intake.
Gross protein efficiency (GPE) (%) = 100 ∗ [(% final body protein ∗ FBW) - (% initial body protein ∗ IBW)]/total protein intake fish.
Lipid efficiency rate (LER) = (FBW - IBW)/lipid intake.
Gross lipid efficiency (GLE) (%) = 100 ∗ [(% final body lipid ∗ FBW) - (% initial body lipid ∗ IBW)]/total lipid intake fish.
Relative variation = (final considered value - initial considered value)/initial considered value.
Diets and whole bodies were analyzed for proximate composition. Moisture content was obtained by weight loss after drying samples in a stove at 105°C overnight. Crude protein was determined as total nitrogen (N∗6.25) after performing Kjeldahl’s method. Ash content was estimated by incineration in a muffle oven at 450°C overnight (AOAC, 2010). Total lipids were determined according to the extraction method of Bligh and Dyer (1959). The same method was performed also on final liver pool samples in order to estimate their fat content. Gross energy was determined by a calorimetric bomb (Adiabatic Calorimetric Bomb Parr 1261; PARR Instrument, IL, United States).
Stomach and proximal intestine, including the pyloric ceca, of each individual were separately homogenized in distilled water (1:3 w/v) and were centrifuged at 4°C, 13,000 × g, for 10 min. Supernatants were stored at –20°C until being processed. Using the stomach homogenate, pepsin activity was measured according to the methodology described in Anson (1938). In brief, 10 μl of the enzyme extract was diluted in 1 mL of 0.1 M HCl-glycine buffer (pH 2.0) containing 0.5% bovine hemoglobin. The mixture was incubated for 20 min at room temperature (approximately 25°C). The reaction was terminated by adding 0.5 ml of 20% trichloroacetic acid (TCA) and was cooled at 4°C for 15 min to facilitate precipitation. After centrifuging at 13,000 × g for 15 min at 4°C, 200 μl of the supernatant was used to measure absorbance at 280 nm. One unit of enzyme activity was defined as 1 μg tyrosine released per minute using a specific absorptivity of 0.008 μg–1 cm–1 at 280 nm.
In the proximal intestine homogenate, trypsin and chymotrypsin activity were measured using Nα-benzoyl-DL-arginine 4-nitroanilide hydrochloride (BAPNA) and N-glutaryl-L-phenylalanine p-nitroanilide (GAPNA) as substrates, according to Erlanger et al. (1961, 1966), respectively. For each of these enzymes, substrate stock (0.5 mM of BAPNA or GAPNA in dimethyl sulfoxide) was brought to the working concentration by 1/10th dilutions using 50 mM Tris-HCl and 20 mM CaCl2 buffer (pH 8.5). The change in absorbance at 405 nm was measured over 10 min at room temperature, for 10–15 μl of the enzyme extract and 200 μl of substrate per each microplate well. For these enzymes, one unit of activity was defined as 1 μmol p-nitroaniline released per minute using coefficients of molar extinction of 8,270 M–1 cm–1 at 405 nm.
Amylase activity was measured following the 3,5-di-nitrosalicylic acid (DNSA) method (Bernfeld, 1955). In brief, 30 μl of enzyme extract and 300 μl of substrate [2% soluble starch in 100 mM phosphate and 20 mM NaCl2 buffer (pH 7.5) were incubated at 37°C for 30 min]. The reaction was stopped by the addition of 150 μl of DNSA and was heated in boiling water for 5 min. After cooling on ice, 1.5 ml of distilled water was added to the mixture, and the absorbance was measured at 530 nm. One unit of amylase activity was defined as the amount of enzyme needed to catalyze the formation of 1 μg of maltose equivalent per minute.
Lipase activity was measured using 4-nitrophenyl myristate as substrate, according to Albro et al. (1985). Briefly, 10 μl of enzyme extract was added to 50 μl of sodium taurocholate (0.4 mg ml–1) and 130 μl of 100 mM Tris-HCL buffer (pH 8.0) per each microplate well. The change in the absorbance at 405 nm was measured over 10 min at room temperature. One unit of amylase activity was defined as the amount of enzyme needed to catalyze the production of 1 μg of p-nitrophenol per minute.
All the activities were expressed in units per g of wet weight of fish, considering both the total amount of tissue used for enzyme determination and the live weight of each sampled fish.
The chemistry profile evaluated in the study was determined using an automated analyzer (AU 480; Olympus/Beckman Coulter, Brea, CA, United States) using dedicated methods (Olympus system reagent, OSR). The type of reaction used in the assay and OSR identification number were reported in brackets after the reported variables. The profile included glucose (GLU; exochinase reaction, OSR6121), urea (urease reaction, OSR6134), creatinine (CREA, Jaffè method, OSR6178), uric acid (Uric Ac, uricase reaction, OSR6198), total bilirubin (Tot Bil, colorimetric reaction, OSR6112), bile acid (Bil Ac, colorimetric method, OSR17000801), cholesterol (CHOL, enzymatic method, OSR6116), triglycerides (TRIG, enzymatic method, OSR61118), high-density lipoprotein (HDL, enzymatic method, OSR6187), total protein (TP, biuret method, OSR6132), albumin (ALB, bromocresol green method, OSR6102), aspartate aminotransferase (AST), alkaline phosphatase (ALP), creatine kinase (CK), and lactate dehydrogenase (LDH) (enzymatic reaction, OSR6009, OSR6004, OSR6179, and OSR6128, respectively), calcium (Ca+2, Arsenazo reaction, OSR6017), inorganic phosphorus (P, molybdate reaction, OSR6122), potassium (K+), sodium (Na+), and chloride (Cl) (ion selective electrode indirect method), iron (Fe; colorimetric reaction, OSR6186), and magnesium (Mg; xylidyl blue reaction, OSR6189). The albumin-to-globulin ratio (ALB/GLO), CaxP, and Na+ to K+ ratio (Na/K) were calculated. Plasma cortisol (CORT) concentration was determined using a chemiluminescence immunoassay (Immulite cortisol, Diagnostic Product Corporation, Los Angeles, CA, United States) using an automated analyzer (Immulite XP2000, Siemens).
Total DNA was extracted and analyzed from individual distal intestine content obtained from three fish per tank (300 mg per fish) on day 58 and day 121, as previously reported in Parma et al. (2020). The analyses were performed to target the transient bacterial community. Amplification of V3–V4 hypervariable regions of the 16S rRNA bacterial gene was carried out using the 341F and 785R primers (Klindworth et al., 2013) with added Illumina adapter overhang sequences and 2 × KAPA HiFi HotStart ReadyMix (KAPA Biosystems). For this step, the thermal cycle consisted of an initial denaturation phase at 95°C for 3 min, 30 cycles of denaturation at 95°C for 30 s, annealing at 55°C for 30 s, and extension at 72°C for 30 s, and a final extension step at 72°C for 5 min. To purify PCR products and to prepare samples for Library for Illumina sequencing, the Illumina protocol “16S Metagenomic Sequencing Library Preparation” was followed, as used in several other publications (Biagi et al., 2019; Musella et al., 2020). Sequencing was performed on Illumina MiSeq platform using a 2 × 250-bp paired-end protocol according to the manufacturer’s instructions (Illumina, San Diego, CA, United States). Raw sequences were processed using the QIIME2 pipeline1 (Bolyen et al., 2019). High-quality reads, obtained by a filtering step for length (minimum/maximum = 250/550 bp) and quality with default parameters, were cleaned using DADA2 (Callahan et al., 2016) and clustered into amplicon sequence variants (ASVs) using VSEARCH (Rognes et al., 2016). Taxonomy was assigned using RDP classifier against SILVA database (Quast et al., 2013).
Alpha diversity was assessed using Faith’s Phylogenetic Diversity (PD_whole_tree), Chao1 index for microbial richness, and observed_ASVs, while beta diversity was estimated by computing UniFrac distances, which were used as input for principal coordinate analysis (PCoA).
All data are represented as mean ± standard deviation (SD). A tank was used as the experimental unit for analyzing growth performance, and a pool of three (on intermediate sampling, day 58) and five (on final sampling, day 121) fish was considered as the experimental unit for analyzing carcass composition, liver fat content, and nutritional indices, whereas nine (on intermediate sampling, day 58) and 15 (on final sampling, day 121) individual fish per treatment were used for analyzing somatic indices, digestive enzyme activity, blood biochemistry, and gut bacterial community profiles. Data of growth performance, nutritional indices, somatic indices, fat liver content, enzyme activity, and plasma parameters were analyzed by a two-way analysis of variance (ANOVA) with Tukey’s post hoc test. In order to assess the amplitude of variations occurring before and after the temperature change, relative variations in growth parameters, morphometric indices, nutritional indices, digestive enzyme activity, and plasma biochemistry were calculated and analyzed by a two-way analysis of variance (ANOVA) with Tukey’s post hoc test. The normality and homogeneity of variance assumptions were validated for all data preceding ANOVA using Levene’s test for homogeneity of variance and Shapiro–Wilks normality test. All gut microbiota statistical analyses were performed using R project2. PCoA plots were generated using the ‘‘vegan’’3 and “Made4” packages (Culhane et al., 2005), and for all PCoAs, betadisper and permutest functions were used to assess homogeneity of dispersion of our data (in all tests, p-value was > 0.05). PERMANOVA (Permutational Multivariate Analysis Of Variance) using distance matrices was used to asses data separation among groups (function “Adonis” in “vegan,” numbers of permutations = 999), in order to assess the influence of temperature changes in each diet group and the influence of diet in the two temperature conditions. When required, Wilcoxon and Kruskal–Wallis test was used to assess significant differences in alpha diversity and taxon relative abundance between groups. A p-value ≤ 0.05 was considered statistically significant, while a p-value between 0.05 and 0.1 was seen as a trend.
Results on growth performance parameters and nutritional indices are summarized in Table 2. Concerning the temperature change occurring in the overall experimental period (days 0–121 period), no significant effects of diet nor temperature (p > 0.05) on growth (FBW, WG, SGR), FI, and survival were detected, while FCR was significantly influenced by temperature (p < 0.05) with lower values in LH (animals first exposed to L temperature and then switched to H). At the same time, diet and temperature had a significant effect on LER and GLE (p < 0.05), both of which were found to be higher in animals fed L16, while among groups fed the same dietary treatment, they were slightly lower in HL. Over days 0–58 period, no dietary effect was detected on FBW, WG, SGR, FCR, FI, and survival (p > 0.05), while temperature had a significant effect (p < 0.05) on FBW, WG, SGR, and FI with higher values in fish reared at 23°C (H groups) compared with those at 17°C (L groups). FCR was moderately lower (p = 0.0505) at high temperature. A significant dietary effect was recorded on LER and GLE (p < 0.05) but not on PER and GPE (p > 0.05). At the same time, significant temperature effect (p < 0.05) was found on PER, GPE, and LER, with lower levels in animals held at 17°C (L).
Table 2. Growth performance and nutritional indices of gilthead sea bream fed experimental diets and exposed to water temperature switch.
In the period following the temperature switch (days 59–121), diet and temperature had a significant effect (p < 0.05) on SGR and FI, with higher values in animals fed diet L16 and maintained at H temperature. Significant temperature effects (p < 0.05) were also found on WG and FCR showing higher WG and lower FCR in H temperature. At the same time, significant (p < 0.05) dietary and temperature effects were found on GLE and LER, with the highest values in fish fed L16 and kept at H and the lowest levels in individuals fed L21 and reared at L (17°C). No significant dietary effect (p > 0.05) was found on PER and GPE. A significant temperature effect (p < 0.05) was noticed on PER, which showed higher in animals reared at H temperature. No significant temperature effect was detected on GPE (p < 0.05). Relative variations in growth performance and nutritional indices calculated between day 58 and day 121 (before–after temperature switch) are represented in Supplementary Table 2. WG, SGR, and FI showed a higher increment/lower reduction in L16 compared with L21 (diet effect p ≤ 0.05). A significant temperature effect was found on the relative variation in FBW, WG, SGR, FCR, and FI with lower increment in HL compared with LH. In addition, the relative variation in FI displayed a significant interaction effect (p < 0.05). Diet had no significant effect on the relative variations in nutritional indices (p < 0.05), while temperature affected PER, GPE, and LER relative variations showing decreasing values in HL compared with LH (temperature effect < 0.05). No significant dietary nor temperature effect were found on GLE relative variation (p < 0.05).
Proximate body composition and somatic indices data are shown in Table 3. Before temperature switch (day 58), protein displayed significant effects of diet, temperature, and interaction (p < 0.05) with higher amounts in L16 and H temperature. For lipid content, there were significant effects of temperature and interaction (p < 0.05), where groups fed L21 retained both the highest and the lowest percentages for H and L, respectively. Ash body percentage was significantly affected (p > 0.05) only by diet, with higher values in groups fed L16. Significant dietary and temperature effects (p < 0.05) were also found for moisture. At the same time, no significant dietary effect (p > 0.05) was found on HSI, MFI, and VSI, while a significant temperature effect occurred on HSI (p < 0.05) with higher levels in fish kept at low temperature (L 17°C).
Table 3. Body composition and somatic indices of gilthead sea bream fed experimental diets and exposed to water temperature switch.
At the end of the trial (day 121), no significant dietary and temperature effects were found in body protein, lipid, and ash percentages (p > 0.05). Moisture showed a significant dietary effect (p < 0.05), displaying a higher level in L21. Fat liver content was not significantly affected by diet nor temperature (p > 0.05). Concerning somatic indices, diet showed a significant effect on MFI and VSI (p < 0.05) with MFI values higher in L21 than in L16, while an additional interaction effect in VSI was also observed, where animals brought to low temperature (L) displayed higher values in L21, and fish exposed to rise in water temperature (H) displayed higher levels in L16. HSI was significantly affected by temperature with higher values observed in the L groups for both dietary regimes.
Relative variations in proximate body composition and somatic indices calculated between day 58 and day 121 (before–after temperature switch) are represented in Supplementary Table 3. No significant dietary or temperature effects were found on relative variations in protein, lipid, ash, and moisture (p > 0.05). Relative variation in HSI was not significantly affected by diet (p > 0.05); however, HL groups displayed a relative increment, while LH groups showed decreasing values (temperature effect p < 0.05). MFI and VSI relative variations were not significantly affected by diet nor temperature (p > 0.05).
Digestive enzymes activities measured before and after water temperature change are shown in Figure 1. Before temperature switch, pepsin activity was significantly (p < 0.05) higher in fish fed on diet L21, but no significant differences were observed between groups maintained at low or high temperature. After temperature switch, the only significant differences observed were associated with interactions between the effects of dietary lipid level and low temperature; the highest and lowest activities were measured in fish fed on low-lipid and high-lipid diets, respectively, maintained at low temperature. On the other hand, no significant effect of temperature change was evidenced, irrespective of diet composition.
Figure 1. Digestive gut enzymes activity (expressed as U g fish body weight– 1) of gilthead sea bream fed the experimental diets and exposed to temperature switch over 121 days. Data are given as the mean of triplicate tank individual samples (n = 3 per tank before temperature change, n = 5 per tank after temperature change) ± SD. Different letters indicate significant difference (two-way ANOVA P ≤ 0.05) between treatments. L16, low-lipid 16% diet; L21, high-lipid 21% diet. HL, constant temperature exposure to high (H) 23°C until temperature switch (days 0–58), then to constant low (L) 17°C until end of trial (day 59–21); LH, constant temperature exposure to low (L) 17°C until temperature switch (days 0–58), then to constant high (H) 23°C until end of trial (day 59–121).
In the case of trypsin, a significant effect of rearing temperature and none of dietary lipid level was evidenced during the first part of the assay, with higher values measured in fish maintained at 17°C when compared with those at 23°C. After temperature inversion, significantly higher values were measured in fish fed on high lipids and maintained at high temperature when compared with those fed on low lipids and maintained at low temperature. On the other hand, significantly higher values of chymotrypsin activity were measured in fish fed on high dietary lipids, both before and after temperature switch.
Amylase activity was not significantly affected either by diet or temperature during the initial period of the experiment, but a significant interaction of diet × temperature occurred (p < 0.05).
After temperature inversion, no significant effect of dietary lipid level was evidenced on amylase activity, while significantly higher values of this enzyme were measured in fish maintained at high temperature.
Relating to lipase activity, while no significant effect of diet or temperature were measured during the initial period, these were evidenced after temperature change. Significantly lower activity was linked to the consumption of low lipid diet, and within the same dietary treatment, a higher activity was detected in fish kept at 23°C compared with those at 17°C.
Relative variations in digestive enzyme activity calculated between day 58 and day 121 are represented in Supplementary Table 4. Relative variations in digestive enzymes were not significantly affected by diet nor temperature (p > 0.05), except for amylase, which displayed a significant effect of temperature. In particular, a lower reduction was observed for LH treatment, and this reduction was less evident under L21 than under L16.
The results of plasma parameters are shown in Tables 4, 5. Before temperature change, significant dietary effect (p < 0.05) was displayed in Tot Bil, Ca2+, P, Na+, CORT, and CaxP, having higher levels in animals fed L21, except for CORT that appeared to be more elevated in groups treated with L16. At the same time, a significant temperature effect was noticed on TRIG, TP, AST, LDH, HDL, ALP, and Fe where all subjects reared at L temperature tended to have higher values than those at H temperature, except for ALP and Fe. Significant dietary and temperature effects (p < 0.05) were observed on ALB/GLO before temperature switch (day 58). Moreover, significant interaction effect on CREA was found in the same period of time (p < 0.05). Before temperature switch, no significant dietary and temperature effects (p > 0.05) were found in GLU, urea, uric Ac, CHOL, ALB, CK, K+, Cl, Mg, and Na/K.
Table 4. Plasma biochemistry values for gilthead sea bream fed the experimental diets and exposed to two different temperatures before temperature switch.
Table 5. Plasma biochemistry values for gilthead sea bream fed the experimental diets and exposed to two different temperatures after temperature switch.
At the end of the experiment (day 121) diet significantly affected TP, ALB, AST, LDH, Ca2+, K+, Na+, Cl, and Na/K on day 121 (p < 0.05). Plasma AST, LDH, K+, Na+, and Cl were higher in L21-supplied individuals and contrariwise for TP, ALB, Ca2+, and Na/K. At the same time, temperature significantly affected GLU, CREA, Bil Ac, and CK (p < 0.05). Among them, GLU, CREA, and Bil Ac presented higher levels in individuals maintained at H temperature, while plasma CK concentration showed the opposite trend. Significant dietary and temperature effects (p < 0.05) were observed on Tot Bil, ALP, and ALB/GLO. Uric Ac was significantly affected by diet, temperature, and interaction (p < 0.05). Moreover, a significant interaction effect on TRIG was found at the same time (p < 0.05); no significant dietary and temperature effect (p > 0.05) was observed on urea, CHOL, P, Fe, Mg, CORT, CaxP, and HDL.
Relative variations in plasma parameters calculated between day 58 and day 121 are represented in Supplementary Table 5. Uric Ac, AST, and CK showed a higher relative increment in L21 compared with L16, while Ca2+ was significantly reduced in fish fed L21 compared with those fed L16 (dietary effect p < 0.05). HL animals displayed higher TP, AST, CK, LDH, and HDL relative increments compared to LH ones, while GLU, Bil Ac, ALP, and ALB/GLO relative increments were higher in LH animals than HL ones (temperature effect p < 0.05). In addition, significant interaction was found on relative variation of CK (p < 0.05). No significant dietary or temperature effect was found on relative variations of Urea, CREA, Tot Bil, CHOL, TRIG, ALB, P, K, Na+, Fe, Cl, Mg, CORT, and CaxP, Na/K (p > 0.05).
The 16S rRNA gene sequencing was performed on a total of 71 distal intestine content samples, yielding 1,724,306 high-quality reads (mean ± SD, 24,286 ± 6,505) and clustered into a total of 2,726 ASVs, of which 2,434 were assigned at family level and 2,002 were assigned at genus level. In order to assess whether the different diets (L16 and L21) result in a specific gut microbiome response to water temperature changes, for each dietary regime, the gut microbiome was sampled before and after the HL (autumn shift) and LH (summer shift) temperature transitions. The correspondent variations in the gut microbiome profiles were assessed by the PCoA of the unweighted UniFrac distances between samples collected at the different temperatures, the taxa most explaining sample segregation are superimposed on the bidimensional space. Finally, for each diet and temperature transition, changes in the gut microbiome internal diversity are shown according to three different metrics: PD_whole_tree, Chao1, and observed_ASVs.
According to our findings (Figures 2A,B), under the L16 dietary regime, only the HL transition (autumn shift) resulted in a significant variation in the overall gut microbiome composition, both in terms of overall compositional structure (“Adonis,” p = 0.001) and in terms of reduction in the internal ecosystem diversity (Kruskal–Wallis test p = 0.002; p = 0.002; p = 0.006). Particularly, the transition to low temperature brings about the reduction in Bacillus in the fish gut microbiome. Conversely, in fishes fed with the L21 diet, both the LH (summer shift) and HL (autumn shift) resulted in significant gut microbiome compositional changes (Figures 2C,D, “Adonis,” p < 0.01). However, in these conditions, no significant variations in the gut microbiome compositional diversity were observed.
Figure 2. Beta diversity and alpha diversity of gut microbiota of gilthead sea bream fed with the experimental diets and exposed to temperature switch over 121 days. (A,B) PCoA based on unweighted UniFrac distances between gut microbiota structure of animals fed with L16 diet and exposed, respectively, to summer shift (LH transition) and autumn shift (HL transition). Samples are significantly separated, only in the autumn shift condition (permutation test with pseudo-F ratios Adonis; p = 0.001). (C,D) PCoA based on unweighted UniFrac distances between gut microbiota structure of animals fed with L21 diet and exposed, respectively, to summer shift (LH transition) and autumn shift (HL transition). Samples are significantly separated in both conditions (permutation test with pseudo-F ratios Adonis; p = 0.002, p = 0.002). Black arrows are obtained by fitting the genus relative abundance values for each sample within the ordination space (function envfit of the vegan R package, with a p-value < 0.01). In each panel, boxplots show alpha diversity values, measured by Faith’s Phylogenetic Diversity (PD_whole_tree), Chao1 index, and amplicon sequence variants (observed_ASVs). Only for the HL group (B), all metrics showed a significant reduction (Kruskal–Wallis test p < 0.01) of alpha diversity in the final condition of group fed with L16 diet and subjected to a temperature switch toward autumn temperature (HL). L16, low-lipid 16% diet; L21, high-lipid 21% diet; HL, constant temperature exposure to high (H) temperature of 23°C before, and to low (L) temperature of 17°C after, temperature switch; LH, constant temperature exposure to low (L) temperature of 17°C before, and to high (H) temperature of 23°C after, temperature switch. Temperature switch occurred on day 58.
We next investigated whether the different diets were associated with specific gut microbiome compositional structure in fishes grown at high or low temperatures. To this end, the PCoA of the unweighted UniFrac distances of the gut microbiome composition of fishes consuming L16 or L21 diet is provided at both warm (Figure 3A) and cold (Figure 3B) growth temperature. For each temperature, the internal gut microbiome diversity corresponding to both diets is also provided. According to our findings, only at high temperature did the different diets show a significantly different gut microbiome layout (“Adonis,” p = 0.01), with L16 diet resulting in a higher load of Lactobacillus. On the contrary, no dietary impact on ecosystem diversity was observed, independent from the temperature.
Figure 3. Beta diversity and alpha diversity of gut microbiota of gilthead sea bream fed with the experimental diets at both warm and cold temperatures. (A) PCoA based on unweighted UniFrac distances between gut microbiota structure of animals fed with L16 and L21 diets and grown at warm temperature. Samples are significantly separated (permutation test with pseudo-F ratios Adonis; p = 0.017). (B) PCoA based on unweighted UniFrac distances between gut microbiota structure of animals fed with L16 and L21 diets and grown at cold temperature. Samples are not significantly separated (permutation test with pseudo-F ratios Adonis; p > 0.05). Black arrows are obtained by fitting the genus relative abundance values for each sample within the ordination space (function envfit of the vegan R package, with a p-value < 0.01). For both temperature conditions, all metrics used to assess alpha diversity did not show a significant variation between the two experimental diets (as highlighted by the boxplots in both panels). L16, low-lipid 16% diet; L21, high-lipid 21% diet.
The overall composition of the sea bream gut microbiome at different phylogenetic levels is represented in Figure 4: phylum in Figure 4A and family in Figure 4B. For all experimental groups, the most abundant taxa were Firmicutes, Proteobacteria, and Actinobacteria, which represented about 88% of the whole gilthead sea bream gut microbiota (Figure 4A and Supplementary Table 6). At family level, the gilthead sea bream gut bacterial community was dominated almost entirely by Lactobacillaceae, which represented around 60% of the whole ecosystem in all groups (Figure 4B). Interestingly, focusing on the genus level, specific compositional differences were detectable among the groups studied (Wilcoxon rank-sum test p < 0.05) (Figure 5). In particular, according to our data, for sea bream receiving L16 diet, the HL transition (autumn shift) resulted in a significant decrease in Bacillus and Planctomycetaceae, while for fish fed with L21 diet, the same shift resulted in the reduction of Planctomyces (Wilcoxon rank-sum test p = 0.008, p = 0.016, p = 0.033, respectively). On the other hand, for both diets, the LH transition (summer shift) gave a significant increase in Methylobacterium (Wilcoxon rank-sum test p = 0.012, p = 0.033). Finally, the L16 diet in the HL transition (autumn shift) favored an increase in Weissella and Bradyrhizobium genera in the gut microbiome, resulting in a significantly higher relative abundance of these genera in the final condition compared with fish fed with L21 diet in the same condition (Wilcoxon p = 0.014, p = 0.026), while L21 diet in the final condition of LH (summer shift) transition favored a significant increase in Streptococcus and Bacillus genera compared with L16 diet in the corresponding condition (Wilcoxon p = 0.015, p = 0.011).
Figure 4. Microbiota composition of distal gut content of gilthead sea bream fed with the experimental diets and exposed to temperature switch over 121 days. Bar plot summarizing the microbiota composition at phylum (A) and family level (B) of fish intestinal content. Only phyla with a relative abundance ≥ 0.1% in at least 10 samples, and families with relative abundance ≥ 0.1% in at least 10 samples are represented. L16, low-lipid 16% diet; L21, high-lipid 21% diet; HL, constant temperature exposure to high (H) temperature of 23°C before, and to low (L) temperature of 17°C after, temperature switch; LH, constant temperature exposure to low (L) temperature of 17°C before, and to high (H) temperature of 23°C after, temperature switch. Temperature switch occurred on day 59.
Figure 5. Taxonomic composition of bacterial communities of distal gut content of gilthead sea bream fed with the experimental diets and exposed to temperature switch over 121 days. Distributions of relative abundance of genera that showed a significant variation between groups fed with different diets or after the temperature switch (Wilcoxon rank-sum test, **p ≤ 0.01; *p ≤ 0.05), only genera with a mean relative abundance ≥ 1.0% in at least one group were represented. The central box of each dataset represents the distance between the 25th and the 75th percentiles. The median between them is marked with a black line. L16, low-lipid 16% diet; L21, high-lipid 21% diet; HL, constant temperature exposure to high (H) temperature of 23°C before, and to low (L) temperature of 17°C after, temperature switch; LH, constant temperature exposure to low (L) temperature of 17°C before, and to high (H) temperature of 23°C after, temperature switch. Temperature switch occurred on day 59.
Though many studies have been conducted on the effect of water temperature on growth, physiological responses, and health in gilthead sea bream, so far, very few have investigated the possible interaction between temperature switch simulating seasonal variation and dietary lipid level, and no work exists on its capability to affect gut microbiota.
The growth parameters observed throughout the overall trial (fish encountering temperature switch between 23 and 17°C and vice versa), within 16 or 21% dietary lipid levels, showed similar performance in terms of growth (FBW, WG, and SGR). However, overall FCR was higher in animals entering low temperature (17°C, HL) in both diets. This significant difference was mainly due to the observed negative effect of temperature on FCR when fish moving from high to low temperature exhibited higher values and higher relative increments. Similarly, our study agrees with the “winter growth arrest” described by Sánchez-Nuño et al. (2018a), where gilthead sea bream brought from 22°C down to 14°C showed a doubling of FCR and a fourfold drop of SGR. Before the temperature change (day 0–58), temperature alone regulated fish growth rather than dietary lipid, and no differences in overall performance were detected within the temperature regimes tested. Our findings are in agreement with previous studies that found no differences in growth and feed utilization when feeding sea bream juveniles at increasing dietary lipid levels at constant high temperatures (Velázquez et al., 2006; Bonaldo et al., 2010; Mongile et al., 2014). Interestingly, in our study, after temperature change (day 59–121), low dietary lipid gained more influence, bringing a compensatory growth effect. In fact, 62 days after temperature change, L16 diet seemed to compensate better for the differences in SGR occurring between days 0 and 59. Furthermore, L16 was better accepted (higher FI values and higher relative increment) by animals passing from 17 to 23°C. It should also be mentioned that the differences in the initial body weight between high and low temperature recorded after the temperature switch could have also interfered in the final overall results.
Concerning lipid efficiency, overall results of LER and GLE indicated that at the same temperature regime, low dietary lipids guaranteed better lipid utilization rather than high ones both before and after temperature change; again, low lipid diet and high temperatures led to better lipid utilization by fish, confirming previous study statements (Velázquez et al., 2006; Bonaldo et al., 2010; Mongile et al., 2014). Feeding 16 or 21% lipid diets did not make any difference in HSI, as observed in other previous studies (Velázquez et al., 2006; Bonaldo et al., 2010; Mongile et al., 2014; Melis et al., 2017). However, HSI increased by 22–35% from H to L temperature, and though no statistical difference occurred in fat liver content, liver lipid content tended to be higher at 23°C. In contrast, most of the current literature, focused on metabolic and physiological responses of this species to low temperatures, observed an increase in the hepatosomatic index (HSI), which is explained by a higher mobilization of lipids due to fat mobilization and hepatic deposition caused by cold temperatures (Ibarz et al., 2005, 2007; Mininni et al., 2014). In our study, before temperature change, MFI, VSI, and their relative variations were not influenced by diet nor temperature. These findings are consistent with those of Mongile et al. (2014), where dietary lipid from 20% up to 24% did not have any effect in gilthead sea bream maintained at 27°C. However, in contrast with the above mentioned author’s findings, 21% lipid diet caused slightly higher MFI levels after temperature change. In addition, a significant interaction indicated that the combined effect of temperature increase and L16 led to a higher VSI than L21.
To further explain the growth responses of gilthead sea bream after undertaking seasonal thermal changes, a spotlight on digestive enzymatic activity was performed. In our study, before the temperature change, pepsin activity was higher in fish fed high lipid (L21). However, after the temperature switch, a significant interaction indicated that the combined effect of low lipid (L16) and low temperature guaranteed a higher activity of this enzyme in animals subjected to a lower temperature of 17°C (HL). For both before and after temperature switch, our study reported no temperature-significant influence on pepsin activity. However, after the temperature switch, pepsin activity in L16 showed a general increasing pattern compared with that in the period before the temperature change, while its activity tended to decrease under L21. On the contrary, in on-growing cobia (Rachycentron canadum) reared at two different temperatures (30°C and 34°C), higher pepsin activity was attributed to animals reared at a high temperature of 34°C (Nguyen et al., 2019; Yúfera et al., 2019). Yet those subjects had higher FCR rather than others reared at 30°C and fed the same daily ration (Nguyen et al., 2019; Yúfera et al., 2019). The authors stated that in cobia reared at a higher temperature, increased pepsin activity could not improve growth owing to increased gastric transit rate (Yúfera et al., 2019). Unlike pepsin, trypsin appeared to be influenced by thermal changes. Before the temperature change, trypsin level was slightly higher at 17°C in each diet; then after the thermal switch, its activity displayed higher values at 23°C but relative decreasing changes ranging from 40 to 59% were observed for all the treatment. Our trypsin levels found before thermal change are consistent with results found in European sea bass (Dicentrarchus labrax) reared at three different water temperatures (17, 20, and 23°C) where the trypsin activity peaked at the lowest temperature of 17°C (Pereira et al., 2018). Temperature and dietary lipid level also affected FI, which could have resulted in variation of enzymatic activity. In addition, dietary lipid level may affect gastric transit rate as reported by Bonvini et al. (2018b) in European sea bass and by García-Meilán et al. (2013) in gilthead sea bream, with possible consequences on enzyme activity. In the present study, chymotrypsin activity was influenced only by diet, being more elevated in fish fed 21% dietary lipid level rather than 16% both before and after temperature change. Similarly, while temperature did not affect chymotrypsin, dietary regime was shown to improve consistently its activity in European sea bass reared at 17°C (Pereira et al., 2018). As regard amylase activity, while before the temperature change no dietary or thermal effect occurred, after the temperature switch, it was significantly impeded, with lower activity values and higher relative reduction in fish brought to 17°C (HL). This temperature influence reinforces the hypothesis that when fish are subjected to colder temperatures, feeding absorption drops, while in the liver, a metabolic reassessment takes place for glycogen synthesis, accumulation, and storage (Silva et al., 2014; Melis et al., 2017; Sánchez-Nuño et al., 2018b). Similarly, lipase activity also was not influenced by diet or temperature before temperature change. Afterward, lipase showed a general reduction in activity with higher values in L21 and at high temperature. This is in contrast with Arantzamendi et al. (2019), where bile salt-activated lipase activity (BAL) of gilthead sea bream maintained at constant optimal water temperature (within 20 and 24.2°C) tended to increase with age throughout the life cycle.
Plasma cortisol and glucose levels are the first and main metabolites being released into the plasma as response markers to stress (Barton, 2002). Before the seasonal temperature change, fish fed L16 showed cortisol levels higher than L21 at each considered temperature, and a similar tendency, though not significant (p = 0.066), was noticed also in glucose. Afterward, cortisol was not influenced by any factor, while glucose increased in animals brought from 17 to 23°C (LH). Our findings on glucose levels are in contrast with cold-induced hyperglycemia observed in studies on gilthead sea bream undertaken in both outdoor and indoor conditions (Rotllant et al., 2001; Faggio et al., 2014; Matias et al., 2018).
Total protein is a liver impairment marker, and increase in concentration can be caused by structural liver alterations such as aminotransferase activity reduction, leading to a concurrent reduction in deamination capacity (Bernet et al., 2001). Among them, ALB was found to be the major plasma protein in 16–18°C acclimated gilthead sea bream, representing 25–30% of the TP. In our study, TP level was greater at lower temperatures, and after temperature change, both TP and ALB were found to be higher in animals fed lower lipid (L16). Our findings are in accordance with the significantly higher plasma TP levels of gilthead sea bream exposed to 13°C compared with those with the same thermal history maintained at 23°C described by Mateus et al. (2017). On the other hand, Sala-Rabanal et al. (2003) reported a decrease in plasma protein fractions in gilthead sea bream exposed to both acute or gradual water thermal decrease to 8, 12, and 14°C during 15 and 20 days long. AST, ALP, and LDH are non-specific plasma enzymes, indicators of tissue damage owing to pathological processes, toxic chemical exposure, or traumatic fish handling (Peres et al., 2013). In the present study, AST, ALP, and LDH were influenced only by temperature before the temperature change. While LDH and AST levels were higher at 17°C, ALP was very high in animals kept at 23°C. Then, after the temperature switch, ALP, AST, and LDH were found to be influenced by dietary lipids, with greater levels in response to high dietary lipid diet (L21). In the present study, the elevated blood LDH activity found at low temperatures before temperature changes could probably be caused by lactate accumulation in aerobic tissues such as red muscle and heart indicating an activation of the anaerobic component of metabolism during exposure to cold (Faggio et al., 2014; Feidantsis et al., 2020b). Before temperature switch, TRIG and HDL were more elevated at 17°C. These results are consistent with increased triglyceride levels found during the colder months, interpreted as a mobilization of the lipid deposits to use as fuels by Faggio et al. (2014). Though most previous studies revealed that cold water conditions for gilthead sea bream caused an imbalance in plasma ion levels (Rotllant et al., 2001; Gallardo et al., 2003; Sala-Rabanal et al., 2003; Vargas-Chacoff et al., 2009; Mateus et al., 2017), in the present study, they mostly changed according to dietary lipid content rather than temperature changes. Indeed, before the temperature change, calcium, phosphorus, and sodium were more elevated in animals fed high lipid diet (L21). Then, after temperature change, while potassium, sodium, and chloride remained higher in accordance with a high lipid diet, calcium was found to be more elevated in fish fed L16 diet. In our study, iron was the only ion influenced by temperature with higher values in animals kept at 23°C before the temperature change. In the present study, it should be mentioned that the high rate of temperature change (3° day–1) could have induced physiological stress during the first days after the thermal switch. In fact, according to Feidantsis et al. (2020a, b). This species showed 3–5 days of adaptive cellular response to stress when a sudden thermal changes occurred in the range of 18–24°C.
The study of the gut microbiota has received great attention in the aquaculture sector as an indicator of productivity and fish health, and it is likely that its manipulation will be achieved in the near future in several fish species of commercial interest. Several studies have recently addressed the effect of diet (Huyben et al., 2020; Rimoldi et al., 2020), rearing density (Parma et al., 2020), age, sex (Piazzon et al., 2019), and genetic background (Piazzon et al., 2020) on the gut microbiota of gilthead sea bream; however more studies to detect dynamical changes of microbial composition during the farming cycle are necessary (Infante-Villamil et al., 2020). In the present study, at high phylogenetic levels, the overall gut microbiome structure was similar among groups, and the main represented taxa at phylum (Firmicutes, Proteobacteria, and Actinobacteria) and family (Lactobacillaceae) levels are consistent with previous trials on this species reared on similar aquafeed formulation and feeding protocols (Parma et al., 2016, 2020). According to our findings, the impact of the L16 and L21 diets on the overall gut microbiome was dependent on growth temperature. Indeed, only high temperature led the two diets associated with different gut microbiome compositional layouts with L16 diet resulting in a higher load of Lactobacillus. The dominance of Lactobacillaceae mainly Lactobacillus has been considered a valid indicator of optimal gut health condition in sea bream (Parma et al., 2016, 2020). Interestingly, the two diets performed differently in terms of microbiome response to the temperature transitions. In particular, while fish fed with L16 diet showed significant gut microbiome changes only at the autumn shift, parallel with a reduction in ecosystem diversity, for the L21 diet, both summer and autumn temperature shifts resulted in significant variations in the ecosystem. Temperature is known to modulate microbial diversity in animals especially in poikilothermic fish species (Sepulveda and Moeller, 2020); however, data explaining the interaction between diet and temperature changes in fish are scarce (Soriano et al., 2018; Busti et al., 2020b; Pelusio et al., 2020). Interestingly, among the few studies, which underlined the combined effect of temperature and dietary lipid level, Soriano et al. (2018), in yellowtail kingfish, detected a reduced bacterial abundance and richness associated to a suboptimal low temperature and low dietary lipid level, suggesting that gut microbiome composition could maintain high relative abundance after the decrease in temperature only in the presence of appropriate nutritional conditions, pointing out the importance of optimal lipid level at low temperatures. On the other hand, in the present study, the temperature increase from 17 to 23°C showed a significant impact on the diversity (β-diversity) only in a high-lipid diet. As concerns the specific gut microbiome compositional changes, the decrease in temperature from 23°C to 17°C leads to a significant reduction in Planctomycetaceae and Bacillus. Bacillus is one of the most important beneficial taxa in fish species, which can make a positive contribution to nutrition, to the immune system, and to disease resistance toward pathogens by producing bacteriocins. This decreasing effect may be in line with the sensibility of sea bream to thermal reduction; however, it should be mentioned that, although there has been a significant decrease in this bacterial taxa only under L16, its value was higher in comparison with L21 at the same time point examined. After the temperature decrease from 23 to 17°C, fish fed L16 also showed a significantly higher abundance of Weissella in comparison to L21. This taxon, belonging to lactic acid bacteria (LAB), is of potential interest for its application as a probiotic in aquaculture (Mortezaei et al., 2020; Ringø et al., 2018, 2020) and has been shown to improve intestinal health and the hemato-parameters of hybrid surubim (Pseudoplatystoma reticulatum female × P. corruscans) male (Jesus et al., 2017).
In L16, the change toward high temperature was characterized by a significant increase in the relative abundance of Methylobacterium. Although with contradictory results, the abundance of Methylobacteriaceae in fish gut has been previously associated with environmental temperature change in tench, Tinca tinca, and the sparids pinfish, Lagodon rhomboids (Givens, 2012; Dulski et al., 2020). Methylobacterium has also been associated as beneficial microbial taxa with Nile Tilapia fed functional ingredients (Zheng et al., 2018). Focusing on the dietary effect after the increase in temperature, L21 showed a significantly higher abundance of Bacillus and Streptococcus compared with L16. Dietary lipid content and composition is known to potentially affect gut microbiota composition of animals, although very few studies in aquatic species are available. In mice, high-calorie diets can affect gut microbiota, reducing bacterial diversity and altering the ecosystem in favor of opportunistic taxa (Bruce-Keller et al., 2020). Also, in zebrafish, the increase in dietary fat from 5 to 15% led to reduced gut microbiome diversity (Falcinelli et al., 2015), and a high-fat diet (24% vs. 8%) fed to overfeeding affected the gut microbiome composition (Navarro-Barró et al., 2019). In this last-mentioned study, the authors revealed an increase in the abundance of Proteobateria, which has been proposed as a possible sign of gut microbiome imbalance in fish species. This is also in agreement with the observed increased taxa (Enterobacteriaceae) belonging to this phylum in sea bass gut microbiome, which experienced inflammatory gut mucosa after exposure to high temperature and low-oxygen condition (Busti et al., 2020b). In the present study, we did not observe a gut microbiome imbalance related to the lipid level tested, which remains within a general optimal requirement for this species. However, the significant increase in Streptoccoccus under L21 compared with L16 at the end of the trial may deserve specific attention. Streptoccoccus is considered one of the most common pathogens in aquaculture (Ringø et al., 2018). These taxa were indicative of dysbiosis in olive flounder, Paralichthys olivaceus, after antibiotic treatment (Kim et al., 2019), and in gilthead sea bream, its significant increase was associated with low fishmeal diet and high rearing density conditions (Parma et al., 2020). Finally, it should be mentioned that fish gut microbiome may also change within the same individual in different parts of the intestine due to their physiological differences (Piazzon et al., 2019), and according to Jones et al. (2018), bacterial community in the midgut of rabbitfish (Siganus fuscescens) hosted operational taxonomic units (OTUs) related to environmental sources, while hindgut hosted OTUs that appeared to be specialized in the role of fermentation. In this regard, further studies in gilthead sea bream should be carried out to define the interaction between microbial community and environmental changes in different traits of the intestine.
In conclusion, high dietary lipid levels, 21% did not improve growth and feed efficiency during seasonal temperature changes in comparison with low dietary lipid (16%). On the other hand, low dietary lipid improved feed intake, growth, and nutrient utilization after temperature changes, especially in fish entering optimal temperature (23°C), which simulated the spring to summer water temperature switch. In addition, after temperature switch, L16 reduced perivisceral fat. Low temperature (17°C) strongly affected overall growth performance and nutrient efficiency parameters in comparison with 23°C with major negative effects in fish experiencing summer to autumn temperature changes. After temperature changes, the combined effects of low-lipid diet and low-temperature conditions resulted in higher pepsin activity, while trypsin, chymotrypsin, and lipase were generally higher at high lipid content. The absence of a significant interaction in most of the plasma parameters examined supports the hypothesis that the combined effect of diet and temperature did not alter the metabolic plasma profile. However, the higher AST and ALP observed at the end of the trial in L21 may deserve further attention of possible negative effect on liver status when combining high dietary lipid and temperature changes. Gut microbiome composition were similar among all groups with the dominance of beneficial taxa (such as Lactobacillus) representative of a healthy ecosystem in this species especially in high temperature condition when L16 diet resulted in a higher load of Lactobacillus. In addition, after the temperature reduction, L16 was characterized by a higher abundance of the potential beneficial taxa Weisella spp., while the increase in temperature and L21 diet supports the growth of the potential pathogens Streptococcus spp. According to the results, the utilization of 16% dietary lipid levels in gilthead sea bream should be preferred when fish are exposed to temperature changes. Although the combined effects of temperature and photoperiod was not addressed in this study, the results of the present study could give useful indication to optimize feeding strategy during summer to autumn and spring to summer temperature changes.
The original contributions presented in the study are included in the article/Supplementary Material, further inquiries can be directed to the corresponding author/s.
The animal study was reviewed and approved by Ethical-Scientific Committee for Animal Experimentation of the University of Bologna (protocol ID 942/2019).
AB, NP, LP, and PG conceived and designed the experiment. NP, DS, and LP wrote the first draft of the manuscript. NP and LP carried out the fish maintenance and sample collection. DS, FD’A, and MC carried out the microbiota analysis and data processing. FD and EB carried out the plasma biochemistry analysis. MY, NG, and FM carried out the enzyme activity analysis. All the authors reviewed, improved the writing, and approved the final manuscript.
This research was undertaken under the MedAID (Mediterranean Aquaculture Integrated Development) project, which has received funding from the European Union’s Horizon 2020 Research and Innovation Programme, Call H2020-SFS-23-2016, Grant agreement no. 727315 (http://www.medaid-h2020.eu/). MY and NG received support from the Spanish Ministry of Science, Innovation and Universities (MCIU), the State Research Agency (AEI), the European Regional Development Fund (FEDER/ERDF), and project Thermodigest (RTI2018-096134-B-I00) granted to MY.
The authors declare that the research was conducted in the absence of any commercial or financial relationships that could be construed as a potential conflict of interest.
This study represents partial fulfillment of the requirements for the Ph.D. thesis of DS at the Ph.D. course of Innovative Technologies and Sustainable Use of Mediterranean Sea Fishery and Biological Resources (FishMed – University of Bologna, Italy). The authors would like to thank Gillian Forlivesi Heywood for English language editing.
The Supplementary Material for this article can be found online at: https://www.frontiersin.org/articles/10.3389/fmars.2021.664701/full#supplementary-material
Albro, P. W., Hall, R. D., Corbett, J. T., and Schroeder, J. (1985). Activation of nonspecific lipase (EC 3.1.1.-) by bile salts. Biochim. Biophys. Acta Lipids Lipid Metab. 835, 477–490. doi: 10.1016/0005-2760(85)90117-1
Anson, M. L. (1938). The estimation of pepsin, trypsin, papain, and cathepsin with hemoglobin. J. Gen. Physiol. 22, 79–89. doi: 10.1085/jgp.22.1.79
AOAC (2010). Officials Methods of Analysis, 17th Edn. Washington. DC: Association of Official Analytical Chemists.
Arantzamendi, L., Roo, F., Hernández-Cruz, C. M., Fernández-Palacios, H., and Izquierdo, M. (2019). Lipid digestion capacity in gilthead seabream (Sparus aurata) from first feeding to commercial size. Fish Physiol. Biochem. 45, 469–484. doi: 10.1007/s10695-018-0577-y
Barton, B. A. (2002). Stress in fishes: a diversity of responses with particular reference to changes in circulating corticosteroids. Integr. Comp. Biol. 42, 517–525. doi: 10.1093/icb/42.3.517
Bell, J. G., and Koppe, W. (2010). “Lipids in aquafeeds,” in Fish Oil Replacement and Alternative Lipid Sources in Aquaculture Feeds, eds G. M. Turchini, W.-K. Ng, and D. R. Tocher (Boca Raton, FL: CRC Press), 2010.
Bernet, D., Schmidt, H., Wahli, T., and Burkhardt-Holm, P. (2001). Effluent from a sewage treatment works causes changes in serum chemistry of brown trout (Salmo trutta L.). Ecotoxicol. Environ. Saf. 48, 140–147. doi: 10.1006/eesa.2000.2012
Biagi, E., D’Amico, F., Soverini, M., Angelini, V., Barone, M., Turroni, S., et al. (2019). Faecal bacterial communities from Mediterranean loggerhead sea turtles (Caretta caretta). Environ. Microbiol. Rep. 11, 361–371. doi: 10.1111/1758-2229.12683
Bligh, E. G., and Dyer, W. J. (1959). A rapid method of total lipid extraction and purification. Can. J. Biochem. Physiol. 37, 911–917. doi: 10.1139/o59-099
Bolyen, E., Rideout, J. R., Dillon, M. R., Bokulich, N. A., Abnet, C. C., Al-Ghalith, G. A., et al. (2019). Reproducible, interactive, scalable and extensible microbiome data science using QIIME 2. Nat. Biotechnol. 37, 852–857. doi: 10.1038/s41587-019-0209-9
Bonaldo, A., Isani, G., Fontanillas, R., Parma, L., Grilli, E., and Gatta, P. P. (2010). Growth and feed utilization of gilthead sea bream (Sparus aurata, L.) fed to satiation and restrictively at increasing dietary energy levels. Aquac. Int. 18, 909–919. doi: 10.1007/s10499-009-9312-0
Bonvini, E., Bonaldo, A., Mandrioli, L., Sirri, R., Dondi, F., Bianco, C., et al. (2018a). Effects of feeding low fishmeal diets with increasing soybean meal levels on growth, gut histology and plasma biochemistry of sea bass. Animal 12, 923–930. doi: 10.1017/S1751731117002683
Bonvini, E., Bonaldo, A., Parma, L., Mandrioli, L., Sirri, R., Grandi, M., et al. (2018b). Feeding European sea bass with increasing dietary fibre levels: impact on growth, blood biochemistry, gut histology, gut evacuation. Aquaculture 494, 1–9. doi: 10.1016/j.aquaculture.2018.05.017
Bruce-Keller, A. J., Richard, A. J., Fernandez-Kim, S.-O., Ribnicky, D. M., Salbaum, J. M., Newman, S., et al. (2020). Fenugreek counters the effects of high fat diet on gut microbiota in mice: links to metabolic benefit. Sci. Rep. 10:1245. doi: 10.1038/s41598-020-58005-7
Busti, S., Bonaldo, A., Dondi, F., Cavallini, D., Yúfera, M., Gilannejad, N., et al. (2020a). Effects of different feeding frequencies on growth, feed utilisation, digestive enzyme activities and plasma biochemistry of gilthead sea bream (Sparus aurata). Aquaculture 529:735616. doi: 10.1016/j.aquaculture.2020.735616
Busti, S., Rossi, B., Volpe, E., Ciulli, S., Piva, A., D’Amico, F., et al. (2020b). Effects of dietary organic acids and nature identical compounds on growth, immune parameters and gut microbiota of European sea bass. Sci. Rep. 10:21321. doi: 10.1038/s41598-020-78441-9
Callahan, B. J., McMurdie, P. J., Rosen, M. J., Han, A. W., Johnson, A. J. A., and Holmes, S. P. (2016). DADA2: high-resolution sample inference from Illumina amplicon data. Nat. Methods 13, 581–583. doi: 10.1038/nmeth.3869
Couto, A., Enes, P., Peres, H., and Oliva-Teles, A. (2012). Temperature and dietary starch level affected protein but not starch digestibility in gilthead sea bream juveniles. Fish Physiol Biochem. 38, 595–601. doi: 10.1007/s10695-011-9537-5
Culhane, A. C., Thioulouse, J., Perrière, G., and Higgins, D. G. (2005). MADE4: an R package for multivariate analysis of gene expression data. Bioinformatics 21, 2789–2790. doi: 10.1093/bioinformatics/bti394
Davis, P. S. (1988). Two occurrences of the gilthead, Sparus aurata Linnaeus 1758, on the coast of Northumberland, England. J. Fish Biol. 33, 951–951. doi: 10.1111/j.1095-8649.1988.tb05545.x
Dulski, T., Kozłowski, K., and Ciesielski, S. (2020). Habitat and seasonality shape the structure of tench (Tinca tinca L.) gut microbiome. Sci. Rep. 10:4460. doi: 10.1038/s41598-020-61351-1
Erlanger, B., Kokowsky, N., and Cohen, N. (1961). The preparation and properties of two new chromogenic substrates of trypsin. Arch. Biochem. Biophys. 95, 271–278. doi: 10.1016/0003-9861(61)90145-X
Erlanger, B. F., Cooper, A. G., and Cohent, W. (1966). The inactivation of chymotrypsin by diphenylcarbamyl chloride and its reactivation by nucleophilic agents. Biochemistry 5, 190–196. doi: 10.1021/bi00865a025
Faggio, C., Piccione, G., Marafioti, S., Arfuso, F., Fortino, G., and Fazio, F. (2014). Metabolic response to monthly variations of Sparus aurata reared in mediterranean on-shore tanks. Turk. J. Fish. Aquat. Sci. 14, 567–574. doi: 10.4194/1303-2712-v14_2_28
Falcinelli, S., Picchietti, S., Rodiles, A., Cossignani, L., Merrifield, D., and Taddei, A. (2015). Lactobacillus rhamnosus lowers zebrafish lipid content by changing gut microbiota and host transcription of genes involved in lipid metabolism. Sci. Rep. 5:9336. doi: 10.1038/srep09336
Feidantsis, K., Georgoulis, I., Zachariou, A., Campaz, B., Christoforou, M., Pörtner, H. O., et al. (2020a). Energetic, antioxidant, inflammatory and cell death responses in the red muscle of thermally stressed Sparus aurata. J. Comp. Physiol. B 190, 403–418. doi: 10.1007/s00360-020-01278-1
Feidantsis, K., Pörtner, H. O., Giantsis, I. A., and Michaelidis, B. (2020b). Advances in understanding the impacts of global warming on marine fishes farmed offshore: Sparus aurata as a case study. J. Fish Biol. doi: 10.1111/jfb.14611 [Epub ahead of print].
Gallardo, M. A., Sala-Rabanal, M., Ibarz, A., Padrós, F., Blasco, J., Fernández-Borras, J., et al. (2003). Functional alterations associated with “winter syndrome” in gilthead sea bream (Sparus aurata). Aquaculture 223, 15–27. doi: 10.1016/S0044-8486(03)00164-9
García-Meilán, I., Valentín, J. M., Fontanillas, R., and Gallardo, M. A. (2013). Different protein to energy ratio diets for gilthead sea bream (Sparus aurata): effects on digestive and absorptive processes. Aquaculture 412–413, 1–7. doi: 10.1016/j.aquaculture.2013.06.031
Givens, C. E. (2012). A Fish Tale: Comparison of the Gut Microbiome of 15 Fish Species and the Influence of Diet and Temperature on Its Composition. Ph.D. thesis. Athens, GA: University of Georgia.
Guerreiro, I., Enes, P., Rodiles, A., Merrifield, D., and Oliva-Teles, A. (2016). Effects of rearing temperature and dietary short-chain fructooligosaccharides supplementation on allochthonous gut microbiota, digestive enzymes activities and intestine health of turbot (Scophthalmus maximus L.) juveniles. Aquac. Nutr. 22, 631–642. doi: 10.1111/anu.12277
Guillen, J. (2019). European and Mediterranean Aquaculture Data Collection and Reporting Under the STECF. Available online at: http://www.medaid-h2020.eu/index.php/2019/02/25/aquaculture-data-collection-stecf/
Hasan, M. R., Hecht, T., De Silva, S. S., and Tacon, A. G. J. (2007). Global synthesis of feeds and nutrients for sustainable aquaculture development. FAO Fish. Tech. Paper 497:3.
Huyben, D., Rimoldi, S., Ceccotti, C., Montero, D., Betancor, M., Iannini, F., et al. (2020). Effect of dietary oil from Camelina sativa on the growth performance, fillet fatty acid profile and gut microbiome of gilthead Sea bream (Sparus aurata). PeerJ 8:10430. doi: 10.7717/peerj.10430
Ibarz, A., Beltrán, M., Fernández-Borràs, J., and Gallardo, M. (2007). Alterations in lipid metabolism and use of energy depots of gilthead sea bream (Sparus aurata) at low temperatures. Aquaculture 262, 470–480. doi: 10.1016/j.aquaculture.2006.11.008
Ibarz, A., Blasco, J., Beltrán, M., Gallardo, M., and Sánchez, J. (2005). Cold-induced alterations on proximate composition and fatty acid profiles of several tissues in gilthead sea bream (Sparus aurata). Aquaculture 249, 477–486. doi: 10.1016/j.aquaculture.2005.02.056
Ibarz, A., Padrós, F., Ngeles Gallardo, M. A., Fernández-Borrà, J., Blasco, J., and Tort, L. (2010). Low-temperature challenges to gilthead sea bream culture: review of cold-induced alterations and “Winter Syndrome.”. Rev. Fish Biol. Fish. 20, 539–556. doi: 10.1007/s11160-010-9159-5
Infante-Villamil, S., Huerlimann, R., and Jerry, D. R. (2020). Microbiome diversity and dysbiosis in aquaculture. Rev. Aquac. 13, 1077–1096. doi: 10.1111/raq.12513
Jesus, G. F. A., Vieira, F. D. N., Silva, B. C., Junior, M. M. D. S., Ushizima, T. T., Schmidt, E. C., et al. (2017). Probiotic bacteria may prevent haemorrhagic septicaemia by maturing intestinal host defences in Brazilian native surubins. Aquac. Nutr. 23, 484–491. doi: 10.1111/anu.12416
Jobling, M., and Peruzzi, S. (2010). “Seabreams and Porgies (Family: Sparidae),” in Finfish Aquaculture Diversification, eds M. Jobling and C. Carter (Wallingford: CABI).
Jones, J., DiBattista, J. D., Stat, M., Bunce, M., Boyce, M. C., Fairclough, D. V., et al. (2018). The microbiome of the gastrointestinal tract of a range-shifting marine herbivorous fish. Front. Microbiol. 9:2000. doi: 10.3389/fmicb.2018.02000
Kim, A., Kim, N., Roh, H. J., Chun, W.-K., Ho, D. T., Lee, Y., et al. (2019). Administration of antibiotics can cause dysbiosis in fish gut. Aquaculture 512:734330. doi: 10.1016/j.aquaculture.2019.734330
Klindworth, A., Pruesse, E., Schweer, T., Rg Peplies, J., Quast, C., Horn, M., et al. (2013). Evaluation of general 16S ribosomal RNA gene PCR primers for classical and next-generation sequencing-based diversity studies. Nucleic Acids Res. 41:e1. doi: 10.1093/nar/gks808
Koven, W. (2002). “Gilthead sea bream, Sparus aurata,” in Nutrient Requirements and Feeding of Finfish for Aquaculture, eds C. D. Webster and C. Lim (Wallingford: CABI), 448.
Leaver, M. J., Bautista, J. M., Björnsson, T., Jönsson, E., Krey, G., Tocher, D. R., et al. (2008). Towards fish lipid nutrigenomics: current state and prospects for fin-fish aquaculture. Rev. Fish Sci. 16, 73–94. doi: 10.1080/10641260802325278
Luna, M., Llorente, I., and Cobo, A. (2019). Determination of feeding strategies in aquaculture farms using a multiple-criteria approach and genetic algorithms. Ann. Oper. Res. doi: 10.1007/s10479-019-03227-w [Epub ahead of print].
Mateus, A. P., Costa, R., Gisbert, E., Pinto, P. I. S., Andree, K. B., Estévez, A., et al. (2017). Thermal imprinting modifies bone homeostasis in cold-challenged sea bream (Sparus aurata). J. Exp. Biol. 220, 3442–3454. doi: 10.1242/jeb.156174
Matias, A. C., Ribeiro, L., Araujo, R. L., and Pousão-Ferreira, P. (2018). Preliminary studies on haematological and plasmatic parameters in gilthead sea bream (Sparus aurata) held under day/night temperature variations. Fish Physiol. Biochem. 44, 273–282. doi: 10.1007/s10695-017-0432-6
Melis, R., Sanna, R., Braca, A., Bonaglini, E., Cappuccinelli, R., Slawski, H., et al. (2017). Molecular details on gilthead sea bream (Sparus aurata) sensitivity to low water temperatures from 1H NMR metabolomics. Comp. Biochem. Physiol. Part A Mol. Integr. Physiol. 204, 129–136. doi: 10.1016/j.cbpa.2016.11.010
Mininni, A. N., Milan, M., Ferraresso, S., Petochi, T., Di Marco, P., Marino, G., et al. (2014). Liver transcriptome analysis in gilthead sea bream upon exposure to low temperature. BMC Genomics 15:765. doi: 10.1186/1471-2164-15-765
Mongile, F., Bonaldo, A., Fontanillas, R., Mariani, L., Badiani, A., Bonvini, E., et al. (2014). Effects of dietary lipid level on growth and feed utilisation of gilthead seabream (Sparus aurata L.) reared at mediterranean summer temperature. Ital. J. Anim. Sci. 13:2999. doi: 10.4081/ijas.2014.2999
Mortezaei, F., Royan, M., Noveirian, H. A., Babakhani, A., Kordghashlaghi, H. A., and Balc Azar, J. L. (2020). In vitro assessment of potential probiotic characteristics of indigenous Lactococcus lactis and Weissella oryzae isolates from rainbow trout (Oncorhynchus mykiss Walbaum). J. Appl. Microbiol. 129, 1004–1019. doi: 10.1111/jam.14652
Musella, M., Wathsala, R., Tavella, T., Rampelli, S., Barone, M., Palladino, G., et al. (2020). Tissue-scale microbiota of the Mediterranean mussel (Mytilus galloprovincialis) and its relationship with the environment. Sci. Total Environ. 717:137209. doi: 10.1016/j.scitotenv.2020.137209
Navarro-Barró, E., Herná Ndez, C., Llera-Herrera, R. L., García-Gasca, A., and Gó Mez-Gil, B. (2019). Overfeeding a high-fat diet promotes sex-specific alterations on the gut microbiota of the Zebrafish (Danio rerio). Zebrafish 16, 268–279. doi: 10.1089/zeb.2018.1648
Nguyen, M., Van Espe, M., Conceição, L. E. C., Le, H. M., Yúfera, M., Engrola, S. A. D., et al. (2019). The role of dietary methionine concentrations on growth, metabolism and N-retention in cobia (Rachycentron canadum) at elevated water temperatures. Aquac. Nutr. 25, 495–507. doi: 10.1111/anu.12875
Parma, L., Candela, M., Soverini, M., Turroni, S., Consolandi, C., Brigidi, P., et al. (2016). Next-generation sequencing characterization of the gut bacterial community of gilthead sea bream (Sparus aurata, L.) fed low fishmeal based diets with increasing soybean meal levels. Anim. Feed Sci. Technol. 222, 204–216. doi: 10.1016/j.anifeedsci.2016.10.022
Parma, L., Pelusio, N. F., Gisbert, E., Esteban, M. A., D’Amico, F., Soverini, M., et al. (2020). Effects of rearing density on growth, digestive conditions, welfare indicators and gut bacterial community of gilthead sea bream (Sparus aurata, L. 1758) fed different fishmeal and fish oil dietary levels. Aquaculture 518:734854. doi: 10.1016/j.aquaculture.2019.734854
Pelusio, N. F., Rossi, B., Parma, L., Volpe, E., Ciulli, S., Piva, A., et al. (2020). Effects of increasing dietary level of organic acids and nature-identical compounds on growth, intestinal cytokine gene expression and gut microbiota of rainbow trout (Oncorhynchus mykiss) reared at normal and high temperature. Fish Shellfish Immunol. 107, 324–335. doi: 10.1016/j.fsi.2020.10.021
Pereira, L. F., Peixoto, M. J., Carvalho, P., Sansuwan, K., Santos, G. A., Gonçalves, J. F. M., et al. (2018). Cross-effects of dietary probiotic supplementation and rearing temperature on growth performance, digestive enzyme activities, cumulative mortality and innate immune response in seabass (Dicentrarchus labrax). Aquac. Nutr. 24, 453–460. doi: 10.1111/anu.12578
Peres, H., Santos, S., and Oliva-Teles, A. (2013). Selected plasma biochemistry parameters in gilthead seabream (Sparus aurata) juveniles. J. Appl. Ichthyol. 29, 630–636. doi: 10.1111/j.1439-0426.2012.02049.x
Piazzon, M. C., Naya-Català, F., Perera, E., Palenzuela, O., Sitjà-Bobadilla, A., and Pérez-Sánchez, J. (2020). Genetic selection for growth drives differences in intestinal microbiota composition and parasite disease resistance in gilthead sea bream. Microbiome 8:168. doi: 10.1186/s40168-020-00922-w
Piazzon, M. C., Naya-Català, F., Simó-Mirabet, P., Picard-Sánchez, A., Roig, F. J., Calduch-Giner, J. A., et al. (2019). Sex, age, and bacteria: how the intestinal microbiota is modulated in a protandrous hermaphrodite fish. Fish. Front Microbiol. 10:2512. doi: 10.3389/fmicb.2019.02512
Quast, C., Klindworth, A., Pruesse, E., Schweer, T., Horn, M., and Glo, F. O. (2013). Evaluation of general 16S ribosomal RNA gene PCR primers for classical and next-generation sequencing-based diversity studies. Nucleic Acids Res. 41, 1–11. doi: 10.1093/nar/gks808
Richard, N., Silva, T., Wulff, T., Schrama, D., Dias, J., Rodrigues, P. M., et al. (2016). Nutritional mitigation of winter thermal stress in gilthead seabream: associated metabolic pathways and potential indicators of nutritional state. J. Proteomics 142, 1–14. doi: 10.1016/j.jprot.2016.04.037
Rimoldi, S., Gini, E., Koch, J. F. A., Iannini, F., Brambilla, F., and Terova, G. (2020). Effects of hydrolyzed fish protein and autolyzed yeast as substitutes of fishmeal in the gilthead sea bream (Sparus aurata) diet, on fish intestinal microbiome. BMC Vet Res. 16:118. doi: 10.1186/s12917-020-02335-1
Ringø, E., Hoseinifar, S. H., Ghosh, K., Van Doan, H., Beck, B. R., and Song, S. K. (2018). Lactic acid bacteria in finfish-An update. Front. Microbiol. 9:1818. doi: 10.3389/fmicb.2018.01818
Ringø, E., Van Doan, H., Lee, S. H., Soltani, M., Hoseinifar, S. H., Harikrishnan, R., et al. (2020). Probiotics, lactic acid bacteria and bacilli: interesting supplementation for aquaculture. J. Appl. Microbiol. 129, 116–136. doi: 10.1111/jam.14628
Rognes, T., Flouri, T., Nichols, B., Quince, C., and Mahé, F. (2016). VSEARCH: a versatile open source tool for metagenomics. PeerJ 2016:e2584. doi: 10.7717/peerj.2584
Rotllant, J., Balm, P. H. M., and Pe, J. (2001). Pituitary and interrenal function in gilthead sea bream (Sparus aurata L., Teleostei) after handling and confinement stress. Gen. Comp. Endocrinol. 342, 333–342. doi: 10.1006/gcen.2001.7604
Sala-Rabanal, M., Sánchez, J., Ibarz, A., Fernández-Borràs, J., Blasco, J., and Gallardo, M. A. (2003). Effects of low temperatures and fasting on hematology and plasma composition of gilthead sea bream (Sparus aurata). Fish Physiol. Biochem. 29, 105–115. doi: 10.1023/B:FISH.0000035904.16686.b6
Sánchez-Nuño, S., Eroldogan, O. T., Sanahuja, I., özşnahinoğlu, I., Blasco, J., Fernández-Borràs, J., et al. (2018a). Cold-induced growth arrest in gilthead sea bream Sparus aurata: metabolic reorganisation and recovery. Aquac. Environ. Interact. 10, 511–528. doi: 10.3354/AEI00286
Sánchez-Nuño, S., Sanahuja, I., Fernández-Alacid, L., Ordóñez-Grande, B., Fontanillas, R., Fernández-Borràs, J., et al. (2018b). Redox challenge in a cultured temperate marine species during low temperature and temperature recovery. Front. Physiol. 9:923. doi: 10.3389/fphys.2018.00923
Schrama, D., Richard, N., Silva, T. S., Figueiredo, F. A., Conceição, L. E., Burchmore, R., et al. (2017). Enhanced dietary formulation to mitigate winter thermal stress in gilthead sea bream (Sparus aurata): a 2D-DIGE plasma proteome study. Fish Physiol Biochem. 43, 603–617. doi: 10.1007/s10695-016-0315-2
Sepulveda, J., and Moeller, A. H. (2020). The effects of temperature on animal gut microbiomes. Front. Microbiol. 11:384. doi: 10.3389/fmicb.2020.00384
Silva, T., da Costa, A., Conceição, L., Dias, J., Rodrigues, P., and Richard, N. (2014). Metabolic fingerprinting of gilthead seabream (Sparus aurata) liver to track interactions between dietary factors and seasonal temperature variations. PeerJ 2:e527. doi: 10.7717/peerj.527
Soriano, E. L., Ramírez, D. T., Araujo, D. R., Gómez-Gil, B., Castro, L. I., and Sánchez, C. G. (2018). Effect of temperature and dietary lipid proportion on gut microbiota in yellowtail kingfish Seriola lalandi juveniles. Aquaculture 497, 269–277. doi: 10.1016/j.aquaculture.2018.07.065
Vargas-Chacoff, L., Arjona, F. J., Polakof, S., del Río, M. P. M., Soengas, J. L., and Mancera, J. M. (2009). Interactive effects of environmental salinity and temperature on metabolic responses of gilthead sea bream Sparus aurata. Comp. Biochem. Physiol. Part A Mol. Integr. Physiol. 154, 417–424. doi: 10.1016/j.cbpa.2009.07.015
Vasconi, M., Caprino, F., Bellagamba, F., and Moretti, V. M. (2017). Fatty acid composition of gilthead sea bream (Sparus aurata) fillets as affected by current changes in aquafeed formulation. Turk. J. Fish. Aquat. Sci. 17, 51–459. doi: 10.4194/1303-2712-v17_3_01
Velázquez, M., Zamora, S., and Martínez, F. J. (2006). Effect of dietary energy content on gilthead sea bream (Sparus aurata) feeding behaviour and nutritional use of the diet. Aquac. Nutr. 12, 127–133. doi: 10.1111/j.1365-2095.2006.00391.x
Yúfera, M., Nguyen, M. V., Navarro-Guillén, C., Moyano, F. J., Jordal, A. E. O., Espe, M., et al. (2019). Effect of increased rearing temperature on digestive function in cobia early juvenile. Comp. Biochem. Physiol. Part A Mol. Integr. Physiol. 230, 71–80. doi: 10.1016/j.cbpa.2019.01.007
Zarkasi, K. Z., Taylor, R. S., Abell, G. C. J., Tamplin, M. L., Glencross, B. D., and Bowman, J. P. (2016). Atlantic Salmon (Salmo salar L.) gastrointestinal microbial community dynamics in relation to digesta properties and diet. Microb. Ecol. 71, 589–603. doi: 10.1007/s00248-015-0728-y
Keywords: gilthead sea bream (Sparus aurata L.), feeding strategies, growth, digestive enzyme, plasma biochemistry, gut microbiota, gut health
Citation: Pelusio NF, Scicchitano D, Parma L, Dondi F, Brini E, D’Amico F, Candela M, Yúfera M, Gilannejad N, Moyano FJ, Gatta PP and Bonaldo A (2021) Interaction Between Dietary Lipid Level and Seasonal Temperature Changes in Gilthead Sea Bream Sparus aurata: Effects on Growth, Fat Deposition, Plasma Biochemistry, Digestive Enzyme Activity, and Gut Bacterial Community. Front. Mar. Sci. 8:664701. doi: 10.3389/fmars.2021.664701
Received: 05 February 2021; Accepted: 21 April 2021;
Published: 31 May 2021.
Edited by:
Fotini Kokou, Wageningen University and Research, NetherlandsReviewed by:
Eduardo Almansa, Spanish Institute of Oceanography (IEO), SpainCopyright © 2021 Pelusio, Scicchitano, Parma, Dondi, Brini, D’Amico, Candela, Yúfera, Gilannejad, Moyano, Gatta and Bonaldo. This is an open-access article distributed under the terms of the Creative Commons Attribution License (CC BY). The use, distribution or reproduction in other forums is permitted, provided the original author(s) and the copyright owner(s) are credited and that the original publication in this journal is cited, in accordance with accepted academic practice. No use, distribution or reproduction is permitted which does not comply with these terms.
*Correspondence: Luca Parma, bHVjYS5wYXJtYUB1bmliby5pdA==
†These authors have contributed equally to this work and share first authorship
Disclaimer: All claims expressed in this article are solely those of the authors and do not necessarily represent those of their affiliated organizations, or those of the publisher, the editors and the reviewers. Any product that may be evaluated in this article or claim that may be made by its manufacturer is not guaranteed or endorsed by the publisher.
Research integrity at Frontiers
Learn more about the work of our research integrity team to safeguard the quality of each article we publish.